- Department of Radiation Oncology, The Third Affiliated Hospital of Soochow University, Changzhou, China
Radiotherapy is one important treatment for malignant tumours. It is widely believed today that radiotherapy has not only been used as a local tumour treatment method, but also can induce systemic anti-tumour responses by influencing the tumour microenvironment, but its efficacy is limited by the tumour immunosuppression microenvironment. With the advancement of technology, immunotherapy has entered a golden age of rapid development, gradually occupying a place in clinical tumour treatment. Regulatory T cells (Tregs) widely distributing in the tumour microenvironment play an important role in mediating tumour development. This article analyzes immunotherapy, the interaction between Tregs, tumours and radiotherapy. It briefly introduces immunotherapies targeting Tregs, aiming to provide new strategies for radiotherapy combined with Immunotherapy.
1 Introduction
Radiotherapy is a major method in malignant tumour therapy. Studies have shown that about 70% of malignant tumours receive radiotherapy during the course of treatment. On one hand, radiotherapy destroys the DNA structure of tumour cells through direct and indirect effects, leading to cell necrosis and apoptosis (1); On the other hand, it also modifies the tumour microenvironment (TME) that can stimulate and augment the inherent and adaptive immunity of the body in order to achieve a systematic anti-tumour response.
Radiosensitivity is a key factor affecting the efficacy of radiotherapy. Currently, researches indicate that radiosensitivity is associated with both the biological features and the microenvironment of the tumour (2). The TME is composed of non-cancerous cells, including fibroblasts, blood vessel forming cells, and immune cells (3). These cells are related to tumour growth, invasion and metastasis, and are closely related to the effect of treatment. Complex systemic effects always occur during radiotherapy, it has the potential to produce both anti-tumour and pro-tumour immune response (4). On one hand, most of the antigens expressed by tumour cells are not specific, but tissue differentiation antigens that are also expressed by normal cells (5), and it is difficult to be identified and killed by immune cells. However, when tumour cells death is caused by radiotherapy, they will release tumour-associated antigen(TAAs)and damage-associated pattern molecules (DAMPs), which can activate T cells through antigen presentation (6). At the same time, radiotherapy will induce the production of pro-inflammatory factors, such as type I interferon(IFN) (7), CXCL16 (8) and CXCL10 (9), which recruit T cells into the irradiated TME and kill tumour cells. Radiotherapy can also increase the expression of NK Group 2 member D (NKG2D) ligands on tumour cells and promote the killing of natural killer cells (NK cells) (10). Furthermore, it can also achieve a systematic anti-tumour response through activating the immune system, commonly known as the abscopal effect (11). On the other hand, radiotherapy induces the aggregation of immunosuppressive cells in the TME, such as regulatory T cells (Tregs), tumour-associated macrophages (TAMs) of M2 phenotype, myeloid-derived suppressor cells (MDSCs), and so on (12). These cells hinder the immune response by limiting the activation and proliferation of T cells. It can also cause TME hypoxia and induce TGF-β expression on tumour cells to trigger hypoxia-inducible factor 1(HIF-1)/vascular endothelial growth factor (VEGF) pathway to promote tumour angiogenesis (13, 14). Radiotherapy can induce cancer-associated fibroblasts (CAFs) to secrete various growth factors, such as transforming growth factor β(TGF-β) and matrix metalloproteinases (MMPs), then further promote tumour growth (15) (Figure 1).
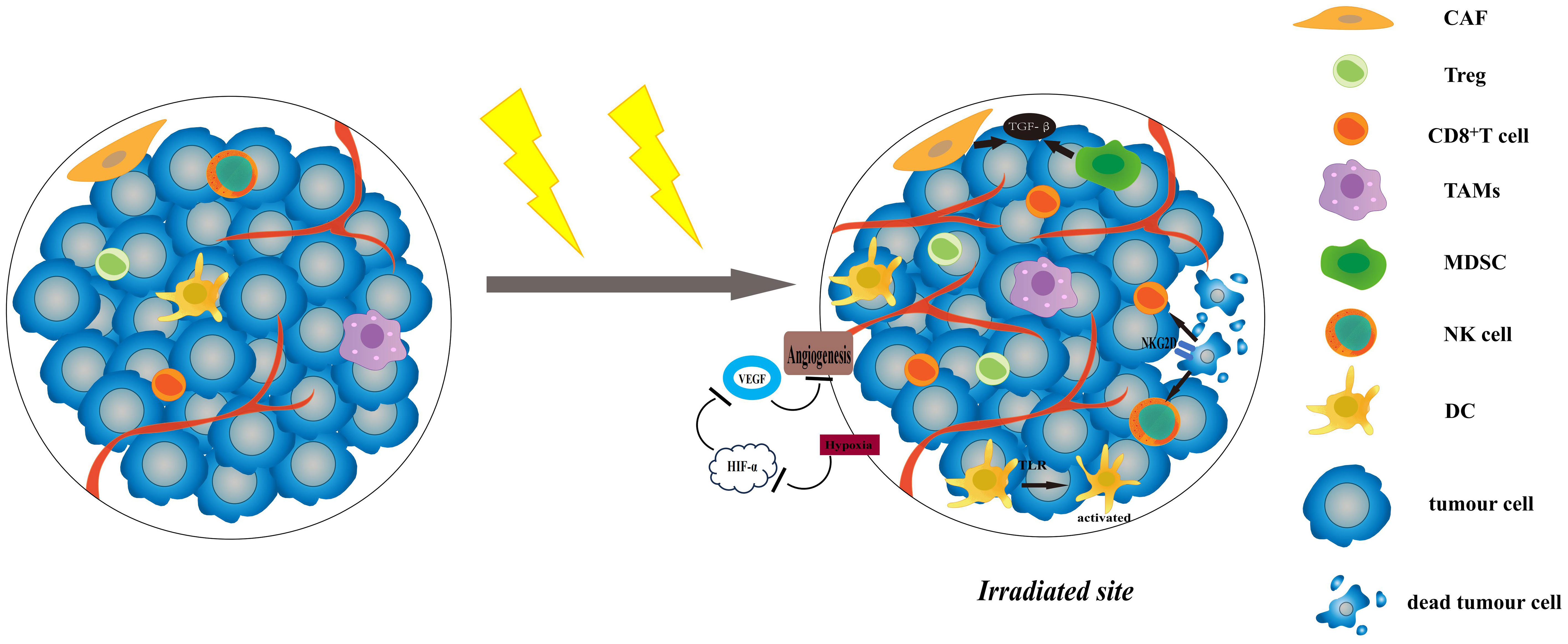
Figure 1 The effects of radiotherapy on TME. Radiotherapy can increase the expression of NKG2D ligands, and promote the killing of NK cells and CD8+ T cells. The increased levels of proinflammatory factors and DAMP-related TLRs can activate DCs and recruit T cells to improve anti-cancer effect. Radiotherapy induces the aggregation of immunosuppressive cells in the TME, like Tregs and MDSCs. It can cause TME hypoxia and induce TGF-β expression on tumour cells to trigger HIF-1/VEGF pathway. CAFs are activated after radiotherapy and can secrete various growth factors, such as TGF-β and MMPs, then further promote tumour growth. TME, tumour microenvironment; NKG2D, NK Group 2 member D; NK cell, natural killer cell; DC, dendritic cell; TRL, Toll-like receptors; MMPs, matrix metalloproteinases; HIF-1, hypoxia-inducible factor 1; VEGF, vascular endothelial growth factor; CAF, cancer-associated fibroblasts.
The efficacy of radiotherapy is limited, using it alone is not enough to destroy the immune tolerance of tumour and kill it all (16). One of the primary problems is that the residual tumour cells are radioresistant and can result in tumour recurrence and metastasis (4). In this case, it is essential to kill the radioresistant tumour cells as many as possible through alternative approaches to optimise tumour control, which has a good development prospect is radiotherapy combined with immunotherapy.
Cancer immunoediting theory states that the process of tumour development can be divided into three stages: Elimination, Equilibration and Escape (17). T cells are an important part of the immune system and can be divided into two categories according to their function in tumour development: CD4+ helper T cells (Th cells) and CD8+ T cells, which play an anti-tumour role, and Treg cells, which play a pro-tumour role. During tumour development, dendritic cells (DCs) present MHC-I molecules of tumour antigens to CD8+ T cells, activating them into cytotoxic T lymphocytes (CTLs) (18), whereas Th cells further promote the proliferation and activation of CD8+ T cells (19). CTL can inhibit tumour growth by directly killing tumour cells through secreting granzyme and perforin, or by inducing apoptosis through the Fas/FasL pathway (18). As a type of immunosuppressive cells, Treg cell is an important factor in creating an immunosuppressive TME and promoting tumour growth. Recent studies have demonstrated that decreasing Tregs infiltration can enhance the efficacy of radiotherapy (20, 21). This article focuses on Tregs to introduce the development of radiotherapy combined with immunotherapy in current tumour therapy.
2 Immunotherapy
2.1 Introduction of immunotherapy
Tumours are genomic diseases, the antigens they expressed are usually the products of mutated cellular genes, aberrantly expressed normal genes, or genes encoding viral proteins (17). These antigens can be recognised by immune system, inducing an immune response that regulates tumour progression. Tumour immunotherapy refers to the method of breaking tumour immune tolerance and inhibiting tumour growth by activating immune cells and enhancing the anti-tumour immune response. It mainly includes: (i) cancer vaccines, such as sipuleucel-T using for prostate cancer (22); (ii) cytokine therapies, such as INF (23), interleukin-2 (IL-2) (24); (iii) Oncolytic virus therapies, such as T-VEC (25); and (iv) chimeric antigen receptor T-cell (CAR-T) and adoptive cell transfer (ACT) (26); (v) antibody therapies or immune checkpoint inhibitors (ICI) such as rituximab and Trastuzumab, as well as CTLA-4 and PD-1 (27, 28). Immunotherapy is a landmark breakthrough in the treatment of tumours and is gradually becoming another pillar of tumour treatment in addition to surgery, chemotherapy and radiotherapy.
2.2 Immunotherapy and radiotherapy
Radiotherapy is used to kill tumours through rays. It has a better local tumour control, not only can eliminate tumours and prevent tumour recurrence after surgery, but also can relieve symptoms and improve the quality of life of patients with advanced tumours (11). However, the specificity of radiotherapy is low, while killing the tumour cells, it also damages normal cells and may cause irreversible damage when it exceeds the tolerance of the tissues (29). Most tumours have low sensitivity to radiotherapy, making it difficult to achieve radical tumour cure. On the contrary, immunotherapy has high specificity, most of them can precisely kill tumour cells with fewer side effects. Tumours are usually classified as immunogenic (hot) tumours or non-immunogenic (cold) tumours based on the abundance or paucity of T-lymphocyte infiltration (30). The former has a better efficacy using immunotherapy. Unfortunately, because the majority of tumours are cold tumours, only about 20-40% of patients can benefit from monoimmunotherapy (31).
In order to identify which patients will benefit from immunotherapy, we need to evaluate TME. Considering the dynamic and complex nature of tumour immune regulation, it is difficult to use a single immune biomarker to select patients (32). We usually use assessment methods like immunohistochemistry, flow cytometry, NGS sequencing and others (33). Currently, single-cell protein analysis and single-cell RNA sequencing (scRNA-seq) have been used to assess TME (28), such as melanoma (34), lung cancer (35), renal cell carcinoma (36) and colorectal cancer (37).
One of the major challenges of immunotherapy is how to convert cold tumours into hot tumours to improve efficacy. Radiotherapy has the potential. The main mechanisms responsible for the lack of tumour T-cell infiltration, include tumour antigen deficiency, defective antigen presentation, poor T-cell activation and defective homing to the tumour (38). Radiotherapy can lead to immunogenic cell death that release TAAs (6), and induce increased expression of MHC-I molecules in tumour cells and increase their sensitivity to CTL killing (39), as well as activate the DNA-cGAS-STING pathway to enhance anti-tumour T-cell responses by DCs (7). It also can induce chemokine secretion to recruit T-cells in tumour (11), and at low doses, radiotherapy can normalise dysfunctional tumour blood vessels thus allowing antigen-specific T cells to infiltrate into the tumour tissue (40). Neoadjuvant local low-dose radiation induces iNOS expression in TAMs, triggering efficient recruitment of tumour-specific T cells, which prolongs survival in immune-refractory mouse tumour models (41). Preclinical trials have demonstrated that radiotherapy can convert tumours unresponsive to CTLA-4 into responsive tumours (42, 43).
In summary, radiotherapy and immunotherapy can be mutually reinforcing. There are many existing clinical trials that combine radiotherapy with immunotherapy aiming to improve efficacy (Table 1).
3 Tregs and tumour
3.1 Introduction to Tregs
Regulatory T cells (Tregs) can regulate the response of the immune system to self-antigens, graft antigens, and tumour antigens. Normally, Tregs account for an average of 6% of the peripheral CD4+ T cells (44). There are two types of Tregs: natural regulatory T cells (nTreg), which are generated through thymic selection; and inducible regulatory T cells (iTreg), which are transforming from CD4+CD25-T cells in peripheral blood through microenvironmental induction (45). Except CD25, Tregs also express the cytotoxic T-lymphocyte-associated protein 4 (CTLA-4), the glucocorticoid inducible tumour necrosis factor receptor (GITR), lymphocyte activation gene 3(LAG-3), neuropilin-1, membrane-bound TGF-β, CD62Lhigh, CD45RBlow, CD103, CD5, CD27, CD38, CD39,CD69, CD73, CD122, OX-40 (CD134), CCR4, CCR7, CCR8,TNF-R2 and FOXP3 (46). FOXP3 is an important differentiation marker (47), and the expression of FOXP3 can transform naive CD4+ T cells into Tregs (48). The expression of FOXP3 plays a variety of roles, for example, FOXP3 can inhibit the transcription of IL-2 by Treg and promote the expression of CD25 (49). It also reprogrammed the metabolism of Treg, making it play a role in the environment of a low-glucose and high-lactate, and more suitable for the TME (50).
3.2 Relationship between Tregs and tumours
Previous studies have shown that Tregs are abundant in the microenvironment of several tumours such as head and neck (51), breast (52), lung (53), liver (54), esophageal (55), gastric (56), pancreatic (57), colon (58) and ovarian cancers (59). There are three possible reasons for its enrichment in the tumour microenvironment: firstly, proliferation: TGF-β secreted by tumour cells can prompt Tregs proliferation in the TME (60); secondly, recruitment: Tregs can be recruited from the peripheral blood to the tumour by interacting with chemokines such as CCL22, which are secreted by tumour cells and macrophages in the TME (59, 61). and thirdly, transformation: TGF-β (62), retinoic acid (63), and others can promote the transformation of CD4+Foxp3-T cells into Tregs.
Evidences showed that Tregs are associated with tumour prognosis and that high expression of Foxp3 in tumour-infiltrating lymphocytes has been linked to a poor prognosis and a high risk of recurrence (64). Curiel et al. analysed the number of Tregs in the tumour of 70 ovarian cancer patients and found that the increase in Treg counts can led to an increased risk of death and reduced survival in patients (59). Gao et al. found that for patients with hepatocellular carcinoma, the quantity of Tregs in the tumour was inversely related to the overall and disease-free survival of the patients, while positively related to the envelope and vascular invasive capacity of the tumour (65). Bates et al. studied Tregs in tumours of breast cancer patients and found that increased levels of Tregs significantly shortened patients’ overall survival and recurrence-free survival, and the accumulation of Tregs was a marker of breast cancer progression (66). McCoy et al. investigated the relationship between the number of Tregs in tumour tissue and prognosis in rectal cancer patients who had received preoperative chemoradiotherapy. The results showed that patients with fewer Tregs tended to achieve complete pathological remission and had longer survival (67).
Tregs regulate the immune response of cells in the tumour microenvironment through the following mechanisms:(a) secreting immunosuppressive factors, such as TGF-β and IL-10, which enhances immunosuppression by decreasing CD8+ T-cell toxicity, suppressing CD4+ T-cell differentiation, promoting Treg transformation, and inhibiting NK-cell proliferation (68).(b) killing effector T cells and antigen-presenting cells by granzymes and perforins (45). (c) interfering with normal immune cell metabolism and functions, such as depriving IL-2 to interference CD4+ T cells activation (69), hydrolysing ATP to produce adenosine to inhibit effector cell proliferation (70). (d) interacting with DCs to achieve immunosuppression: by binding to DCs through CTLA-4,not only inhibits T cell activation, but also triggers indoleamine 2,3-dioxygenase (IDO) expressed by DCs to suppress T cell proliferation (71), and expands Tregs (72). (e) Enhanced immunosuppressive microenvironment through interaction with MDSCs, such as secretion of IL-10 and TGF-β by MDSCs on stimulation with IFN-γ to induce Tregs (73), and secretion of TGF-β and IL-35 by Tregs to enhance the suppressive function of MDSCs (74). Additionally, an in vitro study showed that the presence of large numbers of Tregs can disable the effector function of self-antigen and tumour antigen-specific CD8+ T cells (75). These incompetent CD8+ T cells have a naïve phenotype and respond poorly to tumour antigen stimulation, that is to say, they have a low proliferative capacity after antigen stimulation, produce little IL-2 and other cytokines, and have an up-regulated expression of CTLA-4 (76). Tregs inhibit the body’s immune response through the above actions, thereby promoting tumour growth and affecting the efficacy of treatment.
4 Radiotherapy and Tregs
4.1 Effect of radiotherapy on Tregs
Different immune cells have different radiosensitivity whereby Tregs are more resistant to radiation than other T and B cells (11). Radiation does not enhance the suppressive function of Tregs in the physiological situation. However, in the tumour environment, Tregs acquire a highly suppressive phenotype, termed tumour-infiltrating effector Treg cells, with increased expression of FOXP3 and CD25 (76) and upregulation of a variety of cell-surface molecules including CTLA-4, GITR, OX40, TIGIT, LAG-3, and TIM-3 (77), that is further enhanced by radiotherapy (78). These Tregs have a stronger immunosuppressive function than the Tregs during normal physiology. It was previously stated that the suppressive function of Tregs is significantly dependent on TGF-β and IL-10, and radiation can affect these factors. A previous study found that low-dose irradiation inhibited IL-10 production, whereas high-dose irradiation (HDR) stimulated IL-10 production (79). In contrast, Hussien et al. noted a significant reduction of IL-10 in serum after HDR (80). The reason for the difference may be due to the fact that 2Gy rays were used in the former, whereas 5Gy rays were used in the latter. Both high and low doses of irradiation can consistently activate TGF-β (2). Dong et al. found that HDR may induce TGF-β1 secretion via activating the cAMP-PKA signaling pathway or inhibiting the PLC-PIP2 signaling pathway, and IL-10 secretion was increased (81). The experiments of Jobling et al. demonstrated that after irradiation, reactive oxygen species(ROS) can induce a conformational change in latency-associated peptide (LAP)-TGFβ1, triggering TGF-β1 release (82).
Radiation can also improve Tregs suppression by several mechanisms:(a) Tregs express the nucleotide exoenzymes CD39 and CD73, which catabolise nucleotides to produce adenosine for immunosuppression, and radiation can enhance the expression of CD39 (83). (b) Apoptotic tumour cells can induce the production of tolerogenic DCs, which increase their inhibitory capacity by enhancing their interaction with Tregs (78). (c) Radiation increases the Akt expression in Tregs (84, 85). Akt, through the DNA-PK/AKT/GSK3β pathway, can mediate the overexpression of cyclin D1, activate DNA damage response, and improve Tregs resistance to radiation-induced apoptosis (86). (d) Radiation induces the production of TGF-β in TME, which stimulates Treg aggregation (87). As a result, the number and suppressive capacity of Tregs in the TME are enhanced by radiotherapy, consequently strengthening the immunosuppression of the TME and affecting tumour therapy.
Several clinical trials have shown an increased number of Tregs in their peripheral blood following radiotherapy for patients with head and neck tumours (88), cervical cancer (89), glioma (90), and colorectal cancer (91). Son et al. showed that radiation increased the proportion of Tregs in irradiated tumours of lung and colon cancer (20). Kachikwu et al. found an increase in Treg cells in spleen, lymph nodes, blood and lungs after local irradiation of tumours in mice (92). Baba et al. irradiated mice inoculated with fibrosarcoma, and found that the percentage of Treg cells in the irradiated mice increased, and their function was not weakened. By using annexin V to detect the apoptotic ability of Tregs, it was found that their apoptotic ability decreased after radiation, indicating that they have a good anti-apoptotic ability against radiation (48). Schuler et al. found that Tregs increased and persisted after radiotherapy in patients with head and neck tumours and were highly expressive of CD39, LAP, GARP and anti-apoptotic proteins. These Tregs are resistant to activation-induced cell death and may lead to cisplatin resistance and tumour recurrence and metastasis (88). Qinfeng et al. conducted tumour biopsies on 59 cervical cancer patients before and after radiotherapy. The study demonstrated that radiotherapy induced the redistribution of immune cells in the tumour environment, specifically manifested as: CD8+T and CD4+T cells decreased, while Tregs did not change significantly, resulting in an impaired anti-tumour immune response (89). According to Balogh et al, consistently high absolute numbers of Tregs could be detected in the spleen of mice irradiated with 2 Gy or higher doses at different time points, and radiation promoted Tregs proliferation and enhanced CTLA-4 and IL-10 mRNA expression (93).
4.2 Effect of Tregs on radiotherapy
How to overcome radiation resistance to improve radiosensitivity is the key to improve the effect of radiotherapy. Previous studies have revealed that various pathways can be used to increase Tregs depletion, reduce their function, and improve radiosensitivity. The following are some related studies (Table 2).
4.2.1 IL-2 and CD25
It has been proposed that IL-2 plays a “key role” in the activation of Tregs and is required for the sustained expression of Foxp3 and CD25 in natural Tregs, as well as the improvement of their function (94, 95). A main feature of Tregs is that they constitutively express high levels of the high-affinity IL-2 receptor consisting of CD25, CD122 and CD132, but produce little IL-2 themselves. This means that Tregs can restrict T cell activation by “robbing” IL-2 (96). The addition of exogenous IL-2 can eliminate Tregs suppression in vitro (97). Thus, IL-2 and CD25 may be targets for controlling Tregs survival and suppressive function (98), and the combination of radiotherapy with this approach may enhance anti-tumour effect. After radiotherapy, there are an increase in CD25, and a decrease in CD122 (99). Yasuda et al. ‘s study in rectal cancer found that mice treated with RT+IL-2 had a higher percentage of CD4+T cells but a lower percentage of Tregs and MDSC in splenocytes, Administering IL-2 significantly improved the anti-tumour impact of RT (100). Oweida et al. developed a mouse head and neck tumour model using anti-CD25 antibodies to neutralise Tregs activity. They found that compared with radiotherapy alone, mice treated with combination therapy had more effector T cell infiltration in their tumours. And the combination therapy promoted more macrophages conversion to the M1 phenotype, resulting in more tumour regression (21). Kachikwu et al. also used anti-CD25 antibodies to deplete Tregs, and in prostate cancer, when combined with radiotherapy, tumour growth was delayed and temporary shrink, with improved efficacy compared to radiotherapy alone (92). Piper et al. used PD1-IL2v, a variant of IL-2 immunocytokine that abolishes the binding with CD25 and targets PD-1. It can enhance the infiltration and activation of tumour antigen-specific T cells and reduce Treg cells. Combining it with radiotherapy can significantly improve the efficacy of radiotherapy (99). A phase II clinical trial has shown that in metastatic melanoma, patients treated with IL-2+SBRT have a higher disease control rate compared with IL-2 monotherapy. 44 patients were included in the research. The result showed that the ORR in the SBRT + IL-2 group was 54%: 21% complete response (CR), 33% partial response (PR), 21% stable disease (SD) and 25% progressive disease (PD). The ORR in patients receiving IL-2 monotherapy was 35%: 15% CR, 20% PR, 25% SD and 40% PD (101).
4.2.2 CTLA-4
APC activates T cells by presenting antigens, with MHC as the primary stimulatory signal and CD80/86 as the secondary co-stimulatory signal interacting with TCRs and CD28 on T cells, respectively (102). CTLA-4 expressed by Tregs and CD28 expressed by conventional T cells (Tconv) have common ligands CD80 and CD86, but the affinity of CTLA-4 is significantly higher than CD28, so Tregs can compete with conventional T cells to bind CD80/CD86 and prevent the activation of effector T cells (103). In addition, CTLA-4 on Tregs can downregulate the expression of CD80 and CD86 on DCs, further impeding the activation of conventional T cells (104, 105). It has been demonstrated that CTLA-4 blocker enhances anti-tumour response mainly by selectively reducing Tregs, with T cell activation being a secondary role (106). Therefore, when RT combining with CTLA-4 blockage therapy, more durable T-cell activation can be achieved and the efficacy of radiotherapy can be increased.
In a study of poorly immunogenic metastatic breast cancer 4T1, Demaria et al. combined CTLA-4 blocker with radiotherapy and found a significant reduction in tumour growth rate. Efficient CD8+ T cell-dependent anti-tumour immunity was formed, which not only controlled local tumour growth but also inhibited the formation of lung metastasis (42). Ji et al. found that radiotherapy combining with CTLA-4 blocker reduced the percentage of Tregs and delayed tumour growth and metastasis compared to radiotherapy alone (107). Ipilimumab is a human monoclonal antibody against the CTLA-4 antigen, and several studies have reported significant effects of combination of radiotherapy and Ipilimumab in the treatment of metastatic melanoma (108), non-small cell lung cancer (109) and prostate cancer (110).
4.2.3 GITR
GITR is a co-stimulatory molecule that is expressed at low levels in resting CD4+ and CD8+ T cells and is constitutively expressed in CD4+ FoxP3+ Tregs (111). Activation of the GITR signaling pathway leads to instability and depletion of Tregs (112). Furthermore, GITR signaling acts as a co-stimulatory factor on effector T cells and stimulates T cell proliferation and activation (113). Schoenhals et al. showed that anti-GITR antibody could reverse the increase in Tregs induced by radiotherapy and increase the efficacy of radiotherapy. They also compared the efficacy of G1 and G2 antibodies, in which the G1-type antibody only agonises the GITR, whereas the G2-type antibody agonises the GITR signaling pathway and depletes Tregs meanwhile. The mice receiving the G2 antibody treatment had a more significant tumour regression and experienced longer survival, the abscopal effect was also observed, indicating that depletion of Tregs can increase the efficacy of radiotherapy (114).
4.2.4 Chemokines and their receptors
Tumour-infiltrating macrophages and tumour cells can produce chemokine ligand 22 (CCL22), which attracts cells expressing CCR4 to the tumour tissue (115). CCR4 is primarily expressed in effector Tregs, rather than naïve Tregs and Th2 cells. A study by Sugiyama et al. suggested that anti-CCR4 antibodies can selectively deplete effector Treg cells and increases tumour antigen-specific CD4+ and CD8+ T cells (116); an in vitro study using the antibody to neutralise CCL22 blocked Tregs migration to tumour tissue (59). These trials inspired us that blocking the transport of Tregs is also a potential therapeutic strategy. Similarly, CCR6, a chemokine receptor selectively expressed in Th17 cells and Tregs, can mediate the migration of circulating Tregs to the TME through the CCL20-CCR6 axis, leading to tumour progression and poor prognosis (117). Rutihinda et al. discovered that radiation promotes the secretion of CCL20 by tumour cells and inhibition of the CCL20-CCR6 axis reduces the infiltration of Tregs into the TME, thus inhibiting tumour growth and improving tumour response to radiation (118).
4.2.5 Cyclophosphamide
Cyclophosphamide is an alkylating agent that interferes with DNA replication and kills highly proliferating cells. High dose administration of cyclophosphamide has severe effects on all T cells. However, when used at low dose for long time, it has been shown to selectively reduce Tregs, resulting in enhanced anti-tumour immune responses in both humans and rodents (119–121). Zhao et al. attribute the ability of low-dose CTX to target and deplete Tregs to the down-regulation of a miRNA (miR-142-3p) and up-regulation of the nucleotide exonuclease CD39, which made the ATP levels lower than conventional T cells, increasing the sensitivity of Tregs to CTX (122). North et al. showed that cyclophosphamide eliminated CD4+ T cells that induced CD8+ T cell suppression and promoted immunotherapy of tumours (123). Son et al. demonstrated that radiotherapy combined with low-dose CTX significantly could reduce the number of Tregs in rectal and lung cancers while increasing the number of effector T-cells, tumours regression and survival rate in mice have been improved. Compared with anti-CD25 antibody, LD-CTX combined radiotherapy more effectively inhibited the growth of primary and distant tumours and significantly improved the survival rate of mice (20).
4.2.6 PD-1/PD-L1
In many tumours, immune cells are highly expressive of PD-1 (47). When CD4+ effector T cells in the TME express PD-1, they are actually transformed into Tregs (124). PD-L1 can not only cooperate with TGF-β to promote iTreg transformation, but also enhance its function by maintaining and enhancing the expression of Foxp3 (125). Increased PD-L1 expression in tumour cells after X-ray irradiation aggravates the immunosuppressive nature of the TME (3). Sharabi et al. used radiotherapy combined with PD-1 blockers to treat melanoma mice and found that that the combined therapy reversed the increase of Tregs, increased the ratio of CD8+ T cells to Tregs and improve prognosis compared with radiotherapy alone (126). Since anti-CTLA-4 and anti-PD-L1 use non-redundant mechanisms and act at different time points in the anti-tumour immune response, dual immune checkpoint inhibition in combination with RT may be a future therapeutic option (127). Ji et al. used a combination of radiotherapy, anti-CTLA-4, and anti-PD-L1 blocker to treat mice inoculated with colorectal cancer cells. When compared to using radiotherapy alone or combining it with anti-CTLA-4, the combined treatment led to a more significant decrease in Tregs, an increase in CD8+ T cells, and led to a more effective therapy (107).
4.2.7 TGF-β
TGF-β is an important factor in the regulation of immune homeostasis and immune tolerance, and it regulates the generation and functions of many kinds of immune cells (128). In TME, TGF-β is mainly produced by tumour cells and several other types of cells, including Tregs, fibroblasts, macrophages, etc (129). Elevated levels of TGF-β can inhibit anti-tumour immunity by activating SMADs and NFAT to induce the expression of FOXP3 in CD4+ T cells, which can promote their differentiation to a Treg phenotype (130). As previously stated, irradiation can continuously activate TGF-β (2), the activated TGF-β reduces cellular radiosensitivity by decreasing DNA damage and apoptosis (131). It has been shown that inhibiting TGF-β signaling reduces the number of Tregs and restores the tumour sensitivity to anti-PD-1 and anti-CTLA-4 therapy (132), and blocking TGF-β prior to radiotherapy increases clonogenic cell death and decreases tumour growth (133). A study constructed by Vanpouille-Box et al. found that PD-1-mediated immune escape limits the effect of TGF- β blockers combined with RT, while combination with anti-PD-1 therapy could further improve the efficacy (134). Rodriguez-Ruiz et al. demonstrated TGF-β blockade in conjunction with the anti-PD-1 plus anti-CD137 mAb combination can enhance the abscopal effects of radiotherapy (135). Currently, Yi et al. have developed anti-TGF-β/PD-L1 bispecific antibodies (YM101 and BiTP), which activities were superior to anti-TGF-β and anti-PD-L1 monotherapy, they could effectively inhibit TGF-β1-induced Treg differentiation and suppress the proliferation of tumour cells to slow down tumour growth (136, 137). However, their role with radiotherapy needs further study.
4.2.8 Others
STAT3 can enhance Tregs function and increase their conversion. Oweida et al. used STAT3 antisense nucleotide (ASO) to deplete Tregs, when combined with radiotherapy increased the efficacy of radiotherapy for head and neck tumours (21). Mondini et al. used FOXP3-DRT mice and normal mice to establish a head and neck tumour model, and depletion of Tregs using DT targeting resulted in a significant reduction in tumour burden compared to radiotherapy alone (138). 5-fluorouracil (5-FU) is often used in patients with locally advanced rectal cancer during neoadjuvant chemoradiotherapy and has been shown to induce immunogenic tumour cells death (139). Maeda et al. analysed blood samples from 27 colorectal cancer patients treated with 5-FU-based chemotherapy regimens, and found that there was a significant reduction in Tregs after chemotherapy without affecting CD4+ T cells (140). It was a selective Tregs depletion that made 5-FU a radiosensitizer.
5 Prospect
Radiotherapy not only kills tumour cells directly, but also regulates the immune response of tumours by reshaping the immune microenvironment, making it possible for radiotherapy combined with immunotherapy to achieve a “1 + 1>2” effect. Nowadays, immunotherapy is developing rapidly, more and more tumours can be treated with radiotherapy combined with immunotherapy to achieve a good tumour control. As a kind of immunosuppressive cells, Treg cells participate in the formation of self-immune tolerance, but also hinder the immune system’s surveillance of tumour cells, promoting tumour progression. Therefore, based on the depletion of Treg cells, immunotherapy combined with radiotherapy has a good development prospect. Nowadays, most combination therapies only exist in animal experiments and still require preclinical and clinical experimental data to confirm. However, enhancing anti-tumour immunity and enhancing the efficacy of radiotherapy by affecting Tregs has great development prospects and is worth our in-depth research.
Author contributions
DS: Writing – original draft. YD: Writing – review & editing.
Funding
The author(s) declare financial support was received for the research, authorship, and/or publication of this article. The present study was supported by grants from the Changzhou Sci & Tech Programs (Grant No. CJ20190089), the Young Talent Development Plan of Changzhou Health Commission (No. CZQM2020021).
Conflict of interest
The authors declare that the research was conducted in the absence of any commercial or financial relationships that could be construed as a potential conflict of interest.
Publisher’s note
All claims expressed in this article are solely those of the authors and do not necessarily represent those of their affiliated organizations, or those of the publisher, the editors and the reviewers. Any product that may be evaluated in this article, or claim that may be made by its manufacturer, is not guaranteed or endorsed by the publisher.
References
1. Rupnow BA, Knox SJ. The role of radiation-induced apoptosis as a determinant of tumor responses to radiation therapy. Apoptosis (1999) 4(2):115–43. doi: 10.1023/A:1009675028784
2. Barcellos-Hoff MH, Park C, Wright EG. Radiation and the microenvironment - tumorigenesis and therapy. Nat Rev Cancer (2005) 5(11):867–75. doi: 10.1038/nrc1735
3. Derer A, Frey B, Fietkau R, Gaipl US. Immune-modulating properties of ionizing radiation: rationale for the treatment of cancer by combination radiotherapy and immune checkpoint inhibitors. Cancer Immunol Immunother (2016) 65(7):779–86. doi: 10.1007/s00262-015-1771-8
4. Ozpiskin OM, Zhang L, Li JJ. Immune targets in the tumor microenvironment treated by radiotherapy. Theranostics (2019) 9(5):1215–31. doi: 10.7150/thno.32648
5. Rabinovich GA, Gabrilovich D, Sotomayor EM. Immunosuppressive strategies that are mediated by tumor cells. Annu Rev Immunol (2007) 25:267–96. doi: 10.1146/annurev.immunol.25.022106.141609
6. Tsoutsou PG, Zaman K, Martin Lluesma S, Cagnon L, Kandalaft L, Vozenin MC. Emerging opportunities of radiotherapy combined with immunotherapy in the era of breast cancer heterogeneity. Front Oncol (2018) 8:609. doi: 10.3389/fonc.2018.00609
7. Deng L, Liang H, Xu M, Yang X, Burnette B, Arina A, et al. STING-dependent cytosolic DNA sensing promotes radiation-induced type I interferon-dependent antitumor immunity in immunogenic tumors. Immunity (2014) 41(5):843–52. doi: 10.1016/j.immuni.2014.10.019
8. Matsumura S, Wang B, Kawashima N, Braunstein S, Badura M, Cameron TO, et al. Radiation-induced CXCL16 release by breast cancer cells attracts effector T cells. J Immunol (2008) 181(5):3099–107. doi: 10.4049/jimmunol.181.5.3099
9. Lugade AA, Sorensen EW, Gerber SA, Moran JP, Frelinger JG, Lord EM. Radiation-induced IFN-gamma production within the tumor microenvironment influences antitumor immunity. J Immunol (2008) 180(5):3132–9. doi: 10.4049/jimmunol.180.5.3132
10. Sayitoglu EC, Georgoudaki AM, Chrobok M, Ozkazanc D, Josey BJ, Arif M, et al. Boosting natural killer cell-mediated targeting of sarcoma through DNAM-1 and NKG2D. Front Immunol (2020) 11:40. doi: 10.3389/fimmu.2020.00040
11. Jarosz-Biej M, Smolarczyk R, Cichoń T, Kułach N. Tumor microenvironment as A "Game changer" in cancer radiotherapy. Int J Mol Sci (2019) 20(13). doi: 10.3390/ijms20133212
12. Teng F, Kong L, Meng X, Yang J, Yu J. Radiotherapy combined with immune checkpoint blockade immunotherapy: Achievements and challenges. Cancer Lett (2015) 365(1):23–9. doi: 10.1016/j.canlet.2015.05.012
13. Zhu H, Zhang S. Hypoxia inducible factor-1alpha/vascular endothelial growth factor signaling activation correlates with response to radiotherapy and its inhibition reduces hypoxia-induced angiogenesis in lung cancer. J Cell Biochem (2018) 119(9):7707–18. doi: 10.1002/jcb.27120
14. Rodriguez-Ruiz ME, Vitale I, Harrington KJ, Melero I, Galluzz L. Immunological impact of cell death signaling driven by radiation on the tumor microenvironment. Nat Immunol (2020) 21(2):120–34. doi: 10.1038/s41590-019-0561-4
15. Zhao D, Mo Y, Neganova ME, Aleksandrova Y, Tse E, Chubarev VN, et al. Dual effects of radiotherapy on tumor microenvironment and its contribution towards the development of resistance to immunotherapy in gastrointestinal and thoracic cancers. Front Cell Dev Biol (2023) 11:1266537. doi: 10.3389/fcell.2023.1266537
16. Formenti SC, Demaria S. Combining radiotherapy and cancer immunotherapy: a paradigm shift. J Natl Cancer Inst (2013) 105(4):256–65. doi: 10.1093/jnci/djs629
17. Schreiber RD, Old LJ, Smyth MJ. Cancer immunoediting: integrating immunity's roles in cancer suppression and promotion. Science (2011) 331(6024):1565–70. doi: 10.1126/science.1203486
18. Farhood B, Najafi M, Mortezaee K. CD8(+) cytotoxic T lymphocytes in cancer immunotherapy: A review. J Cell Physiol (2019) 234(6):8509–21. doi: 10.1002/jcp.27782
19. Topalian SL, Taube JM, Anders RA, Pardoll DM. Mechanism-driven biomarkers to guide immune checkpoint blockade in cancer therapy. Nat Rev Cancer (2016) 16(5):275–87. doi: 10.1038/nrc.2016.36
20. Son CH, Bae J-H, Shin D-Y, Lee H-R, Jo WS, Yang K, et al. Combination effect of regulatory T-cell depletion and ionizing radiation in mouse models of lung and colon cancer. Int J Radiat Oncol Biol Phys (2015) 92(2):390–8. doi: 10.1016/j.ijrobp.2015.01.011
21. Oweida AJ, Darragh L, Phan A, Binder D, Bhatia S, Mueller A, et al. STAT3 modulation of regulatory T cells in response to radiation therapy in head and neck cancer. J Natl Cancer Inst (2019) 111(12):1339–49. doi: 10.1093/jnci/djz036
22. Kantoff PW, Higano CS, Shore ND, Berger ER, Small EJ, Penson DF, et al. Sipuleucel-T immunotherapy for castration-resistant prostate cancer. N Engl J Med (2010) 363(5):411–22. doi: 10.1056/NEJMoa1001294
23. Kirkwood JM, Strawderman MH, Ernstoff MS, Smith TJ, Borden EC, Blum RH, et al. Interferon alfa-2b adjuvant therapy of high-risk resected cutaneous melanoma: the eastern cooperative oncology group trial EST 1684. J Clin Oncol (2023) 41(3):425–35. doi: 10.1200/JCO.22.02264
24. Rosenberg SA, Packard BS, Aebersold PM, Solomon D, Topalian SL, Toy ST, et al. Use of tumor-infiltrating lymphocytes and interleukin-2 in the immunotherapy of patients with metastatic melanoma. A preliminary report. N Engl J Med (1988) 319(25):1676–80. doi: 10.1056/NEJM198812223192527
25. Andtbacka RH, Kaufman HL, Collichio F, Amatruda T, Senzer N, Chesney J, et al. Talimogene laherparepvec improves durable response rate in patients with advanced melanoma. J Clin Oncol (2015) 33(25):2780–8. doi: 10.1200/JCO.2014.58.3377
26. Dobosz P, Dzieciatkowski T. The intriguing history of cancer immunotherapy. Front Immunol (2019) 10:2965. doi: 10.3389/fimmu.2019.02965
27. Abbott M, Ustoyev Y. Cancer and the immune system: the history and background of immunotherapy. Semin Oncol Nurs (2019) 35(5):150923. doi: 10.1016/j.soncn.2019.08.002
28. Zhang Y, Zhang Z. The history and advances in cancer immunotherapy: understanding the characteristics of tumor-infiltrating immune cells and their therapeutic implications. Cell Mol Immunol (2020) 17(8):807–21. doi: 10.1038/s41423-020-0488-6
29. Giaj-Levra N, Borghetti P, Bruni A, Ciammella P, Cuccia F, Fozza A, et al. Current radiotherapy techniques in NSCLC: challenges and potential solutions. Expert Rev Anticancer Ther (2020) 20(5):387–402. doi: 10.1080/14737140.2020.1760094
30. Demaria S, Coleman CN, Formenti SC. Radiotherapy: changing the game in immunotherapy. Trends Cancer (2016) 2(6):286–94. doi: 10.1016/j.trecan.2016.05.002
31. Woo SR, Fuertes MB, Corrales L, Spranger S, Furdyna MJ, Leung MYK, et al. STING-dependent cytosolic DNA sensing mediates innate immune recognition of immunogenic tumors. Immunity (2014) 41(5):830–42. doi: 10.1016/j.immuni.2014.10.017
32. Sharma P, Allison JP. The future of immune checkpoint therapy. Science (2015) 348(6230):56–61. doi: 10.1126/science.aaa8172
33. Zhang J, Huang D, Saw PE, Song E. Turning cold tumors hot: from molecular mechanisms to clinical applications. Trends Immunol (2022) 43(7):523–45. doi: 10.1016/j.it.2022.04.010
34. Tirosh I, Izar B, Prakadan SM, Wadsworth MH, Treacy D, Trombetta JJ, et al. Dissecting the multicellular ecosystem of metastatic melanoma by single-cell RNA-seq. Science (2016) 352(6282):189–96. doi: 10.1126/science.aad0501
35. Guo X, Zhang Y, Zheng L, Zheng C, Song J, Zhang Q, et al. Global characterization of T cells in non-small-cell lung cancer by single-cell sequencing. Nat Med (2018) 24(7):978–85. doi: 10.1038/s41591-018-0045-3
36. Young MD, Mitchell TJ, Braga FAV, Tran MGB, Stewart BJ, Ferdinand JR, et al. Single-cell transcriptomes from human kidneys reveal the cellular identity of renal tumors. Science (2018) 361(6402):594–9. doi: 10.1126/science.aat1699
37. Li H, Courtois ET, Sengupta D, Tan Y, Chen KH, Goh JJL, et al. Reference component analysis of single-cell transcriptomes elucidates cellular heterogeneity in human colorectal tumors. Nat Genet (2017) 49(5):708–18. doi: 10.1038/ng.3818
38. Bonaventura P, Shekarian T, Alcazer V, Valladeau-Guilemond J, Valsesia-Wittmann S, Amigorena S, et al. Cold tumors: A therapeutic challenge for immunotherapy. Front Immunol (2019) 10:168. doi: 10.3389/fimmu.2019.00168
39. Reits EA, Hodge JW, Herberts CA, Groothuis TA, Chakraborty M, Wansley EK, et al. Radiation modulates the peptide repertoire, enhances MHC class I expression, and induces successful antitumor immunotherapy. J Exp Med (2006) 203(5):1259–71. doi: 10.1084/jem.20052494
40. Shevtsov M, Sato H, Multhoff G, Shibata A. Novel approaches to improve the efficacy of immuno-radiotherapy. Front Oncol (2019) 9:156. doi: 10.3389/fonc.2019.00156
41. Klug F, Prakash H, Huber PE, Seibel T, Bender N, Halama N, et al. Low-dose irradiation programs macrophage differentiation to an iNOS(+)/M1 phenotype that orchestrates effective T cell immunotherapy. Cancer Cell (2013) 24(5):589–602. doi: 10.1016/j.ccr.2013.09.014
42. Demaria S, Kawashima N, Yang AM, Devitt ML, Babb JS, Allison JP, et al. Immune-mediated inhibition of metastases after treatment with local radiation and CTLA-4 blockade in a mouse model of breast cancer. Clin Cancer Res (2005) 11(2 Pt 1):728–34. doi: 10.1158/1078-0432.728.11.2
43. Dewan MZ, Galloway AE, Kawashima N, Dewyngaert JK, Babb JS, Formenti SC, et al. Fractionated but not single-dose radiotherapy induces an immune-mediated abscopal effect when combined with anti-CTLA-4 antibody. Clin Cancer Res (2009) 15(17):5379–88. doi: 10.1158/1078-0432.CCR-09-0265
44. Dieckmann D, Plottner H, Berchtold S, Berger T, Schuler G, et al. Ex vivo isolation and characterization of CD4(+)CD25(+) T cells with regulatory properties from human blood. J Exp Med (2001) 193(11):1303–10. doi: 10.1084/jem.193.11.1303
45. Sakaguchi S, Yamaguchi T, Nomura T, Ono M. Regulatory T cells and immune tolerance. Cell (2008) 133(5):775–87. doi: 10.1016/j.cell.2008.05.009
46. La Cava A. Natural Tregs and autoimmunity. Front Biosci (Landmark Ed) (2009) 14(1):333–43. doi: 10.2741/3247
47. Karimi S, Chattopadhyay S, Chakraborty NG. Manipulation of regulatory T cells and antigen-specific cytotoxic T lymphocyte-based tumour immunotherapy. Immunology (2015) 144(2):186–96. doi: 10.1111/imm.12387
48. Baba J, Watanabe S, Saida Y, Tanaka T, Miyabayashi T, Koshio J, et al. Depletion of radio-resistant regulatory T cells enhances antitumor immunity during recovery from lymphopenia. Blood (2012) 120(12):2417–27. doi: 10.1182/blood-2012-02-411124
49. Tay C, Tanaka A, Sakaguchi S. Tumor-infiltrating regulatory T cells as targets of cancer immunotherapy. Cancer Cell (2023) 41(3):450–65. doi: 10.1016/j.ccell.2023.02.014
50. Wang J, Gong R, Zhao C, Lei K, Sun X, Ren H, et al. Human FOXP3 and tumour microenvironment. Immunology (2023) 168(2):248–55. doi: 10.1111/imm.13520
51. Schaefer C, Kim GG, Albers A, Hoermann K, Myers EN, Whiteside TL, et al. Characteristics of CD4+CD25+ regulatory T cells in the peripheral circulation of patients with head and neck cancer. Br J Cancer (2005) 92(5):913–20. doi: 10.1038/sj.bjc.6602407
52. Liyanage UK, Moore TT, Joo H-G, Tanaka Y, Herrmann V, Doherty G, et al. Prevalence of regulatory T cells is increased in peripheral blood and tumor microenvironment of patients with pancreas or breast adenocarcinoma. J Immunol (2002) 169(5):2756–61. doi: 10.4049/jimmunol.169.5.2756
53. Wolf AM, Wolf D, Steurer M, Gastl G, Gunsilius E, Grubeck-Loebenstein B, et al. Increase of regulatory T cells in the peripheral blood of cancer patients. Clin Cancer Res (2003) 9(2):606–12.
54. Ormandy LA, Hillemann T, Wedemeyer H, Manns MP, Greten TF, Korangy F, et al. Increased populations of regulatory T cells in peripheral blood of patients with hepatocellular carcinoma. Cancer Res (2005) 65(6):2457–64. doi: 10.1158/0008-5472.CAN-04-3232
55. Ichihara F, Kono K, Takahashi A, Kawaida H, Sugai H, Fujii H, et al. Increased populations of regulatory T cells in peripheral blood and tumor-infiltrating lymphocytes in patients with gastric and esophageal cancers. Clin Cancer Res (2003) 9(12):4404–8.
56. Sasada T, Kimura M, Yoshida Y, Kanai M, Takabayashi A. CD4+CD25+ regulatory T cells in patients with gastrointestinal Malignancies: possible involvement of regulatory T cells in disease progression. Cancer (2003) 98(5):1089–99. doi: 10.1002/cncr.11618
57. Hiraoka N, Onozato K, Kosuge T, Hirohashi S. Prevalence of FOXP3+ regulatory T cells increases during the progression of pancreatic ductal adenocarcinoma and its premalignant lesions. Clin Cancer Res (2006) 12(18):5423–34. doi: 10.1158/1078-0432.CCR-06-0369
58. Salama P, Phillips M, Grieu F, Morris M, Zeps N, Joseph D, et al. Tumor-infiltrating FOXP3+ T regulatory cells show strong prognostic significance in colorectal cancer. J Clin Oncol (2009) 27(2):186–92. doi: 10.1200/JCO.2008.18.7229
59. Curiel TJ, Coukos G, Zou L, Alvarez X, Cheng P, Mottram P, et al. Specific recruitment of regulatory T cells in ovarian carcinoma fosters immune privilege and predicts reduced survival. Nat Med (2004) 10(9):942–9. doi: 10.1038/nm1093
60. Coe D, Addey C, White M, Simpson E, Dyson J, Chai J-G, et al. The roles of antigen-specificity, responsiveness to transforming growth factor-beta and antigen-presenting cell subsets in tumour-induced expansion of regulatory T cells. Immunology (2010) 131(4):556–69. doi: 10.1111/j.1365-2567.2010.03328.x
61. Gobert M, Treilleux I, Bendriss-Vermare N, Bachelot T, Goddard-Leon S, Arfi V, et al. Regulatory T cells recruited through CCL22/CCR4 are selectively activated in lymphoid infiltrates surrounding primary breast tumors and lead to an adverse clinical outcome. Cancer Res (2009) 69(5):2000–9. doi: 10.1158/0008-5472.CAN-08-2360
62. Liu VC, Wong LY, Jang T, Shah AH, Park I, Yang X, et al. Tumor evasion of the immune system by converting CD4+CD25- T cells into CD4+CD25+ T regulatory cells: role of tumor-derived TGF-beta. J Immunol (2007) 178(5):2883–92. doi: 10.4049/jimmunol.178.5.2883
63. Coombes JL, Siddiqui KRR, Arancibia-Cárcamo CV, Hall J, Sun C-M, Belkaid Y, et al. A functionally specialized population of mucosal CD103+ DCs induces Foxp3+ regulatory T cells via a TGF-beta and retinoic acid-dependent mechanism. J Exp Med (2007) 204(8):1757–64. doi: 10.1084/jem.20070590
64. Facciabene A, Motz GT, Coukos G. T-regulatory cells: key players in tumor immune escape and angiogenesis. Cancer Res (2012) 72(9):2162–71. doi: 10.1158/0008-5472.CAN-11-3687
65. Gao Q, Qiu S-J, Fan J, Zhou J, Wang X-Y, Xiao Y-S, et al. Intratumoral balance of regulatory and cytotoxic T cells is associated with prognosis of hepatocellular carcinoma after resection. J Clin Oncol (2007) 25(18):2586–93. doi: 10.1200/JCO.2006.09.4565
66. Bates GJ, Fox SB, Han C, Leek RD, Garcia JF, Harris AL, et al. Quantification of regulatory T cells enables the identification of high-risk breast cancer patients and those at risk of late relapse. J Clin Oncol (2006) 24(34):5373–80. doi: 10.1200/JCO.2006.05.9584
67. McCoy MJ, Hemmings C, Miller TJ, Austin SJ, Bulsara MK, Zeps N, et al. Low stromal Foxp3+ regulatory T-cell density is associated with complete response to neoadjuvant chemoradiotherapy in rectal cancer. Br J Cancer (2015) 113(12):1677–86. doi: 10.1038/bjc.2015.427
68. Wang Y, Ma Y, Fang Y, Wu S, Liu L, Fu D, et al. Regulatory T cell: a protection for tumour cells. J Cell Mol Med (2012) 16(3):425–36. doi: 10.1111/j.1582-4934.2011.01437.x
69. Boyman O, Sprent J. The role of interleukin-2 during homeostasis and activation of the immune system. Nat Rev Immunol (2012) 12(3):180–90. doi: 10.1038/nri3156
70. Ohta A, Kini R, Ohta A, Subramanian M, Madasu M, Sitkovsky M, et al. The development and immunosuppressive functions of CD4(+) CD25(+) FoxP3(+) regulatory T cells are under influence of the adenosine-A2A adenosine receptor pathway. Front Immunol (2012) 3:190. doi: 10.3389/fimmu.2012.00190
71. Munn DH, Sharma MD, Mellor AL. Ligation of B7-1/B7-2 by human CD4+ T cells triggers indoleamine 2,3-dioxygenase activity in dendritic cells. J Immunol (2004) 172(7):4100–10. doi: 10.4049/jimmunol.172.7.4100
72. Chung DJ, Rossi M, Romano E, Ghith J, Yuan J, Munn DH, et al. Indoleamine 2,3-dioxygenase-expressing mature human monocyte-derived dendritic cells expand potent autologous regulatory T cells. Blood (2009) 114(3):555–63. doi: 10.1182/blood-2008-11-191197
73. Huang B, Pan PY, Li Q, Sato AI, Levy DE, Bromberg J, et al. Gr-1+CD115+ immature myeloid suppressor cells mediate the development of tumor-induced T regulatory cells and T-cell anergy in tumor-bearing host. Cancer Res (2006) 66(2):1123–31. doi: 10.1158/0008-5472.CAN-05-1299
74. Li C, Jiang P, Wei S, Xu X, Wang J. Regulatory T cells in tumor microenvironment: new mechanisms, potential therapeutic strategies and future prospects. Mol Cancer (2020) 19(1):116. doi: 10.1158/1557-3125.HIPPO19-B11
75. Maeda Y, Nishikawa H, Sugiyama D, Ha D, Hamaguchi M, Saito T, et al. Detection of self-reactive CD8(+) T cells with an anergic phenotype in healthy individuals. Science (2014) 346(6216):1536–40. doi: 10.1126/science.aaa1292
76. Tanaka A, Sakaguchi S. Regulatory T cells in cancer immunotherapy. Cell Res (2017) 27(1):109–18. doi: 10.1038/cr.2016.151
77. De Simone M, Arrigoni A, Rossetti G, Gruarin P, Ranzani V, Politano C, et al. Transcriptional landscape of human tissue lymphocytes unveils uniqueness of tumor-infiltrating T regulatory cells. Immunity (2016) 45(5):1135–47. doi: 10.1016/j.immuni.2016.10.021
78. Persa E, Balogh A, Sáfrány G, Lumniczky K. The effect of ionizing radiation on regulatory T cells in health and disease. Cancer Lett (2015) 368(2):252–61. doi: 10.1016/j.canlet.2015.03.003
79. Liu SZ, Jin SZ, Liu XD, Sun YM. Role of CD28/B7 costimulation and IL-12/IL-10 interaction in the radiation-induced immune changes. BMC Immunol (2001) 2:8. doi: 10.1186/1471-2172-2-8
80. Hussien SM, Rashed ER. Immune system modulation by low-dose ionizing radiation-induced adaptive response. Int J Immunopathol Pharmacol (2023) 37:3946320231172080. doi: 10.1177/03946320231172080
81. Dong JC, Cheng GH, Shan Xing Y, Wu N, Shao ML, Li PW, et al. Role of PLC-PIP2 and cAMP-PKA signal pathways in radiation-induced immune-suppressing effect. BioMed Environ Sci (2014) 27(1):27–34. doi: 10.3967/bes2014.005
82. Jobling MF, Mott JD, Finnegan MT, Jurukovski V, Erickson AC, Walian PJ, et al. Isoform-specific activation of latent transforming growth factor beta (LTGF-beta) by reactive oxygen species. Radiat Res (2006) 166(6):839–48. doi: 10.1667/RR0695.1
83. Schaue D, Xie MW, Ratikan JA, McBride WH. Regulatory T cells in radiotherapeutic responses. Front Oncol (2012) 2:90. doi: 10.3389/fonc.2012.00090
84. Wang M, Gou X, Wang L. Protein kinase B promotes radiation-induced regulatory T cell survival in bladder carcinoma. Scand J Immunol (2012) 76(1):70–4. doi: 10.1111/j.1365-3083.2012.02707.x
85. Li CG, He MR, Wu FL, Li YJ, Sun AM. Akt promotes irradiation-induced regulatory T-cell survival in hepatocellular carcinoma. Am J Med Sci (2013) 346(2):123–7. doi: 10.1097/MAJ.0b013e31826ceed0
86. Shimura T, Kakuda S, Ochiai Y, Nakagawa H, Kuwahara Y, Takai Y, et al. Acquired radioresistance of human tumor cells by DNA-PK/AKT/GSK3beta-mediated cyclin D1 overexpression. Oncogene (2010) 29(34):4826–37. doi: 10.1038/onc.2010.238
87. Wu CT, Hsieh CC, Yen TC, Chen WC, Chen MF. TGF-beta1 mediates the radiation response of prostate cancer. J Mol Med (Berl) (2015) 93(1):73–82. doi: 10.1007/s00109-014-1206-6
88. Schuler PJ, Harasymczuk M, Schilling B, Saze Z, Strauss L, Lang S, et al. Effects of adjuvant chemoradiotherapy on the frequency and function of regulatory T cells in patients with head and neck cancer. Clin Cancer Res (2013) 19(23):6585–96. doi: 10.1158/1078-0432.CCR-13-0900
89. Qinfeng S, Depu W, Xiaofeng Y, Shah W, Hongwei C, Yili W, et al. In situ observation of the effects of local irradiation on cytotoxic and regulatory T lymphocytes in cervical cancer tissue. Radiat Res (2013) 179(5):584–9. doi: 10.1667/RR3155.1
90. Fadul CE, Fisher JL, Gui J, Hampton TH, Côté AL, Ernstoff MS, et al. Immune modulation effects of concomitant temozolomide and radiation therapy on peripheral blood mononuclear cells in patients with glioblastoma multiforme. Neuro Oncol (2011) 13(4):393–400. doi: 10.1093/neuonc/noq204
91. Schaue D, Comin-Anduix B, Ribas A, Zhang L, Goodglick L, Sayre JW, et al. T-cell responses to survivin in cancer patients undergoing radiation therapy. Clin Cancer Res (2008) 14(15):4883–90. doi: 10.1158/1078-0432.CCR-07-4462
92. Kachikwu EL, Iwamoto KS, Liao Y-P, DeMarco JJ, Agazaryan N, Economou JS, et al. Radiation enhances regulatory T cell representation. Int J Radiat Oncol Biol Phys (2011) 81(4):1128–35. doi: 10.1016/j.ijrobp.2010.09.034
93. Balogh A, Persa E, Bogdándi EN, Benedek A, Hegyesi H, Sáfrány G, et al. The effect of ionizing radiation on the homeostasis and functional integrity of murine splenic regulatory T cells. Inflammation Res (2013) 62(2):201–12. doi: 10.1007/s00011-012-0567-y
94. Thornton AM, Donovan EE, Piccirillo CA, Shevach EM. Cutting edge: IL-2 is critically required for the in vitro activation of CD4+CD25+ T cell suppressor function. J Immunol (2004) 172(11):6519–23. doi: 10.4049/jimmunol.172.11.6519
95. Shevach EM, DiPaolo RA, Andersson J, Zhao D-M, Stephens GL, Thornton AM, et al. The lifestyle of naturally occurring CD4+ CD25+ Foxp3+ regulatory T cells. Immunol Rev (2006) 212:60–73. doi: 10.1111/j.0105-2896.2006.00415.x
96. Pandiyan P, Zheng L, Ishihara S, Reed J, Lenardo MJ. CD4+CD25+Foxp3+ regulatory T cells induce cytokine deprivation-mediated apoptosis of effector CD4+ T cells. Nat Immunol (2007) 8(12):1353–62. doi: 10.1038/ni1536
97. Thornton AM, Shevach EM. CD4+CD25+ immunoregulatory T cells suppress polyclonal T cell activation in vitro by inhibiting interleukin 2 production. J Exp Med (1998) 188(2):287–96. doi: 10.1084/jem.188.2.287
98. Peng Y, Tao Y, Zhang Y, Wang J, Yang J, Wang Y, et al. CD25: A potential tumor therapeutic target. Int J Cancer (2023) 152(7):1290–303. doi: 10.1002/ijc.34281
99. Piper M, Hoen M, Darragh LB, Knitz MW, Nguyen D, Gadwa J, et al. Simultaneous targeting of PD-1 and IL-2Rbetagamma with radiation therapy inhibits pancreatic cancer growth and metastasis. Cancer Cell (2023) 41(5):950–969.e6. doi: 10.1016/j.ccell.2023.04.001
100. Yasuda K, Nirei T, Tsuno NH, Nagawa H, Kitayama J. Intratumoral injection of interleukin-2 augments the local and abscopal effects of radiotherapy in murine rectal cancer. Cancer Sci (2011) 102(7):1257–63. doi: 10.1111/j.1349-7006.2011.01940.x
101. Curti B, Crittenden M, Seung SK, Fountain CB, Payne R, Chang S, et al. Randomized phase II study of stereotactic body radiotherapy and interleukin-2 versus interleukin-2 in patients with metastatic melanoma. J Immunother Cancer (2020) 8(1). doi: 10.1136/jitc-2020-000773
102. Sansom DM. CD28, CTLA-4 and their ligands: who does what and to whom? Immunology (2000) 101(2):169–77. doi: 10.1046/j.1365-2567.2000.00121.x
103. Walunas TL, Lenschow DJ, Bakker CY, Linsley PS, Freeman GJ, Green JM, et al. CTLA-4 can function as a negative regulator of T cell activation. Immunity (1994) 1(5):405–13. doi: 10.1016/1074-7613(94)90071-X
104. Wing K, Onishi Y, Prieto-Martin P, Yamaguchi T, Miyara M, Fehervari Z, et al. CTLA-4 control over Foxp3+ regulatory T cell function. Science (2008) 322(5899):271–5. doi: 10.1126/science.1160062
105. Yamaguchi T, Kishi A, Osaki M, Morikawa H, Prieto-Martin P, Wing K, et al. Construction of self-recognizing regulatory T cells from conventional T cells by controlling CTLA-4 and IL-2 expression. Proc Natl Acad Sci USA (2013) 110(23):E2116–25. doi: 10.1073/pnas.1307185110
106. Selby MJ, Engelhardt JJ, Quigley M, Henning KA, Chen T, Srinivasan M, et al. Anti-CTLA-4 antibodies of IgG2a isotype enhance antitumor activity through reduction of intratumoral regulatory T cells. Cancer Immunol Res (2013) 1(1):32–42. doi: 10.1158/2326-6066.CIR-13-0013
107. Ji D, Song C, Li Y, Xia J, Wu Y, Jia J, et al. Combination of radiotherapy and suppression of Tregs enhances abscopal antitumor effect and inhibits metastasis in rectal cancer. J Immunother Cancer (2020) 8(2). doi: 10.1136/jitc-2020-000826
108. Grimaldi AM, Simeone E, Giannarelli D, Muto P, Falivene S, Borzillo V, et al. Abscopal effects of radiotherapy on advanced melanoma patients who progressed after ipilimumab immunotherapy. Oncoimmunology (2014) 3:e28780. doi: 10.4161/onci.28780
109. Golden EB, Demaria S, Schiff PB, Chachoua A, Formenti SC. An abscopal response to radiation and ipilimumab in a patient with metastatic non-small cell lung cancer. Cancer Immunol Res (2013) 1(6):365–72. doi: 10.1158/2326-6066.CIR-13-0115
110. Slovin SF, Higano CS, Hamid O, Tejwani S, Harzstark A, Alumkal JJ, et al. Ipilimumab alone or in combination with radiotherapy in metastatic castration-resistant prostate cancer: results from an open-label, multicenter phase I/II study. Ann Oncol (2013) 24(7):1813–21. doi: 10.1093/annonc/mdt107
111. Shimizu J, Yamazaki S, Takahashi T, Ishida Y, Sakaguchi S. Stimulation of CD25(+)CD4(+) regulatory T cells through GITR breaks immunological self-tolerance. Nat Immunol (2002) 3(2):135–42. doi: 10.1038/ni759
112. Amoozgar Z, Kloepper J, Ren J, Tay RE, Kazer SW, Kiner E, et al. Targeting Treg cells with GITR activation alleviates resistance to immunotherapy in murine glioblastomas. Nat Commun (2021) 12(1):2582. doi: 10.1038/s41467-021-22885-8
113. Shevach EM, Stephens GL. The GITR-GITRL interaction: co-stimulation or contrasuppression of regulatory activity? Nat Rev Immunol (2006) 6(8):613–8. doi: 10.1038/nri1867
114. Schoenhals JE, Cushman TR, Barsoumian HB, Li A, Cadena AP, Niknam S, et al. Anti-glucocorticoid-induced tumor necrosis factor-related protein (GITR) therapy overcomes radiation-induced treg immunosuppression and drives abscopal effects. Front Immunol (2018) 9:2170. doi: 10.3389/fimmu.2018.02170
115. Ishida T, Ueda R. CCR4 as a novel molecular target for immunotherapy of cancer. Cancer Sci (2006) 97(11):1139–46. doi: 10.1111/j.1349-7006.2006.00307.x
116. Sugiyama D, Nishikawa H, Maeda Y, Nishioka M, Tanemura A, Katayama I, et al. Anti-CCR4 mAb selectively depletes effector-type FoxP3+CD4+ regulatory T cells, evoking antitumor immune responses in humans. Proc Natl Acad Sci USA (2013) 110(44):17945–50. doi: 10.1073/pnas.1316796110
117. Chen KJ, Lin SZ, Zhou L, Xie HY, Zhou WH, Taki-Eldin A, et al. Selective recruitment of regulatory T cell through CCR6-CCL20 in hepatocellular carcinoma fosters tumor progression and predicts poor prognosis. PloS One (2011) 6(9):e24671. doi: 10.1371/journal.pone.0024671
118. Rutihinda C, Haroun R, Saidi NE, Ordoñez JP, Naasri S, Lévesque D, et al. Inhibition of the CCR6-CCL20 axis prevents regulatory T cell recruitment and sensitizes head and neck squamous cell carcinoma to radiation therapy. Cancer Immunol Immunother (2023) 72:1089–102. doi: 10.1007/s00262-022-03313-2
119. Motoyoshi Y, Kaminoda K, Saitoh O, Hamasaki K, Nakao K, Ishii N, et al. Different mechanisms for anti-tumor effects of low- and high-dose cyclophosphamide. Oncol Rep (2006) 16(1):141–6. doi: 10.3892/or.16.1.141
120. Ghiringhelli F, Menard C, Puig PE, Ladoire S, Roux S, Martin F, et al. Metronomic cyclophosphamide regimen selectively depletes CD4+CD25+ regulatory T cells and restores T and NK effector functions in end stage cancer patients. Cancer Immunol Immunother (2007) 56(5):641–8. doi: 10.1007/s00262-006-0225-8
121. Ghiringhelli F, Larmonier N, Schmitt E, Parcellier A, Cathelin D, Garrido C, et al. CD4+CD25+ regulatory T cells suppress tumor immunity but are sensitive to cyclophosphamide which allows immunotherapy of established tumors to be curative. Eur J Immunol (2004) 34(2):336–44. doi: 10.1002/eji.200324181
122. Zhao J, Cao Y, Lei Z, Yang Z, Zhang B, Huang B, et al. Selective depletion of CD4+CD25+Foxp3+ regulatory T cells by low-dose cyclophosphamide is explained by reduced intracellular ATP levels. Cancer Res (2010) 70(12):4850–8. doi: 10.1158/0008-5472.CAN-10-0283
123. North RJ. Cyclophosphamide-facilitated adoptive immunotherapy of an established tumor depends on elimination of tumor-induced suppressor T cells. J Exp Med (1982) 155(4):1063–74. doi: 10.1084/jem.155.4.1063
124. Wang W, Lau R, Yu D, Zhu W, Korman A, Weber J, et al. PD1 blockade reverses the suppression of melanoma antigen-specific CTL by CD4+ CD25(Hi) regulatory T cells. Int Immunol (2009) 21(9):1065–77. doi: 10.1093/intimm/dxp072
125. Francisco LM, Salinas VH, Brown KE, Vanguri VK, Freeman GJ, Kuchroo VK, et al. PD-L1 regulates the development, maintenance, and function of induced regulatory T cells. J Exp Med (2009) 206(13):3015–29. doi: 10.1084/jem.20090847
126. Sharabi AB, Nirschl CJ, Kochel CM, Nirschl TR, Francica BJ, Velarde E, et al. Stereotactic radiation therapy augments antigen-specific PD-1-mediated antitumor immune responses via cross-presentation of tumor antigen. Cancer Immunol Res (2015) 3(4):345–55. doi: 10.1158/2326-6066.CIR-14-0196
127. Twyman-Saint Victor C, Rech AJ, Maity A, Rengan R, Pauken KE, Stelekati E, et al. Radiation and dual checkpoint blockade activate non-redundant immune mechanisms in cancer. Nature (2015) 520(7547):373–7. doi: 10.1038/nature14292
128. Sanjabi S, Oh SA, Li MO. Regulation of the immune response by TGF-beta: from conception to autoimmunity and infection. Cold Spring Harb Perspect Biol (2017) 9(6). doi: 10.1101/cshperspect.a022236
129. Batlle E, Massague J. Transforming growth factor-beta signaling in immunity and cancer. Immunity (2019) 50(4):924–40. doi: 10.1016/j.immuni.2019.03.024
130. Tone Y, Furuuchi K, Kojima Y, Tykocinski ML, Greene MI, Tone M, et al. Smad3 and NFAT cooperate to induce Foxp3 expression through its enhancer. Nat Immunol (2008) 9(2):194–202. doi: 10.1038/ni1549
131. An YS, Kim MR, Lee SS, Lee YS, Chung E, Song JY, et al. TGF-beta signaling plays an important role in resisting gamma-irradiation. Exp Cell Res (2013) 319(4):466–73. doi: 10.1016/j.yexcr.2012.12.008
132. Courau T, Nehar-Belaid D, Florez L, Levacher B, Vazquez T, Brimaud F, et al. TGF-beta and VEGF cooperatively control the immunotolerant tumor environment and the efficacy of cancer immunotherapies. JCI Insight (2016) 1(9):e85974. doi: 10.1172/jci.insight
133. Bouquet F, Pal A, Pilones KA, Demaria S, Hann B, Akhurst RJ, et al. TGFbeta1 inhibition increases the radiosensitivity of breast cancer cells in vitro and promotes tumor control by radiation in vivo. Clin Cancer Res (2011) 17(21):6754–65. doi: 10.1158/1078-0432.CCR-11-0544
134. Vanpouille-Box C, Diamond JM, Pilones KA, Zavadil J, Babb JS, Formenti SC, et al. TGFbeta is a master regulator of radiation therapy-induced antitumor immunity. Cancer Res (2015) 75(11):2232–42. doi: 10.1158/0008-5472.CAN-14-3511
135. Rodriguez-Ruiz ME, Rodríguez I, Mayorga L, Labiano T, Barbes B, Etxeberria I, et al. TGFbeta blockade enhances radiotherapy abscopal efficacy effects in combination with anti-PD1 and anti-CD137 immunostimulatory monoclonal antibodies. Mol Cancer Ther (2019) 18(3):621–31. doi: 10.1158/1535-7163.MCT-18-0558
136. Yi M, Wu Y, Niu M, Zhu S, Zhang J, Yan Y, et al. Anti-TGF-beta/PD-L1 bispecific antibody promotes T cell infiltration and exhibits enhanced antitumor activity in triple-negative breast cancer. J Immunother Cancer (2022) 10(12). doi: 10.1136/jitc-2022-005543
137. Yi M, Zhang J, Li A, Niu M, Yan Y, Jiao Y, et al. The construction, expression, and enhanced anti-tumor activity of YM101: a bispecific antibody simultaneously targeting TGF-beta and PD-L1. J Hematol Oncol (2021) 14(1):27. doi: 10.1186/s13045-021-01045-x
138. Mondini M, Loyher PL, Hamon P, Thoré MG, Laviron M, Berthelot K, et al. CCR2-dependent recruitment of Tregs and monocytes following radiotherapy is associated with TNFalpha-mediated resistance. Cancer Immunol Res (2019) 7(3):376–87. doi: 10.1158/2326-6066.CIR-18-0633
139. Frey B, Stache C, Rubner Y, Werthmöller N, Schulz K, Sieber R, et al. Combined treatment of human colorectal tumor cell lines with chemotherapeutic agents and ionizing irradiation can in vitro induce tumor cell death forms with immunogenic potential. J Immunotoxicol (2012) 9(3):301–13. doi: 10.3109/1547691X.2012.693547
Keywords: radiotherapy, Tregs, immunotherapy, radiosensitivity, immune microenvironment
Citation: Song D and Ding Y (2024) A new target of radiotherapy combined with immunotherapy: regulatory T cells. Front. Immunol. 14:1330099. doi: 10.3389/fimmu.2023.1330099
Received: 30 October 2023; Accepted: 11 December 2023;
Published: 08 January 2024.
Edited by:
Rosalinda Sorrentino, University of Salerno, ItalyReviewed by:
Saptak Banerjee, Chittaranjan National Cancer Institute (CNCI), IndiaMing Yi, Zhejiang University, China
Copyright © 2024 Song and Ding. This is an open-access article distributed under the terms of the Creative Commons Attribution License (CC BY). The use, distribution or reproduction in other forums is permitted, provided the original author(s) and the copyright owner(s) are credited and that the original publication in this journal is cited, in accordance with accepted academic practice. No use, distribution or reproduction is permitted which does not comply with these terms.
*Correspondence: Yun Ding, ZHl5c3diQDE2My5jb20=