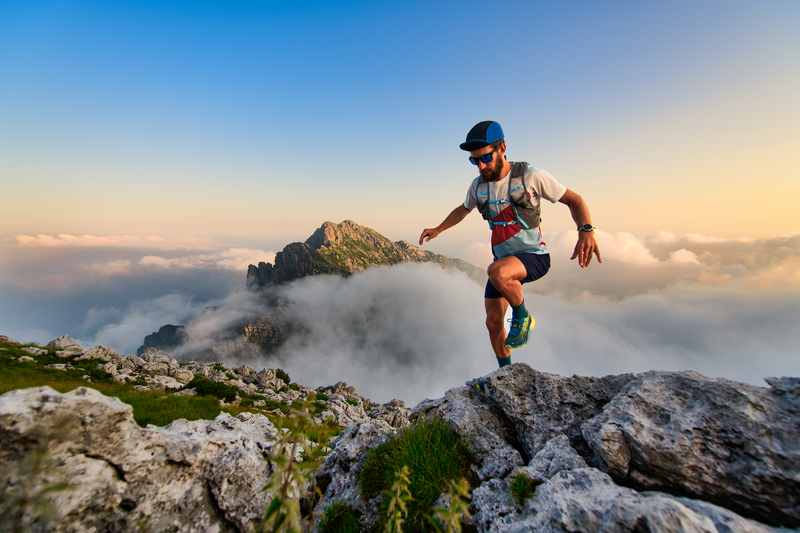
94% of researchers rate our articles as excellent or good
Learn more about the work of our research integrity team to safeguard the quality of each article we publish.
Find out more
REVIEW article
Front. Immunol. , 13 December 2023
Sec. Cytokines and Soluble Mediators in Immunity
Volume 14 - 2023 | https://doi.org/10.3389/fimmu.2023.1323797
Sepsis currently remains a major contributor to mortality in the intensive care unit (ICU), with 48.9 million cases reported globally and a mortality rate of 22.5% in 2017, accounting for almost 20% of all-cause mortality worldwide. This highlights the urgent need to improve the understanding and treatment of this condition. Sepsis is now recognized as a dysregulation of the host immune response to infection, characterized by an excessive inflammatory response and immune paralysis. This dysregulation leads to secondary infections, multiple organ dysfunction syndrome (MODS), and ultimately death. PD-L1, a co-inhibitory molecule expressed in immune cells, has emerged as a critical factor in sepsis. Numerous studies have found a significant association between the expression of PD-1/PD-L1 and sepsis, with a particular focus on PD-L1 expressed on neutrophils recently. This review explores the role of PD-1/PD-L1 in immunostimulatory and anti-inflammatory pathways, illustrates the intricate link between PD-1/PD-L1 and sepsis, and summarizes current therapeutic approaches against PD-1/PD-L1 in the treatment and prognosis of sepsis in preclinical and clinical studies.
Sepsis is one of the leading causes of death in intensive care units (ICU) (1). In 2017, there were approximately 48.9 million globally reported cases of sepsis, resulting in a mortality rate of 22.5% and accounting for nearly 20% of all-cause mortality worldwide (2). While advancements in sepsis treatments such as antibiotics and fluid resuscitation have led to a reduction in mortality rates over the past few decades, there is still ample room for improvement (3). Furthermore, even sepsis survivors face a notable mortality risk after discharge, with rates as high as 15% within the first year (4). These statistics underscore the insufficiency of current treatments and emphasize the need to enhance our understanding of sepsis’s etiology and progression (5).
Sepsis is recognized as an immune dysregulation to various infections (6). This dysregulation manifests as a simultaneous presence of excessive inflammatory response and persistent immune paralysis (7–9). Although the patient’s immune status fluctuates, immune paralysis emerges from the onset of sepsis and serves as a critical factor contributing to multiple organ dysfunction syndrome (MODS). The programmed cell death 1 (PD-1) and its ligand PD-L1 pathway play a pivotal role in sepsis occurrence, development, and prognosis from various perspectives and levels. A considerable number of researches have demonstrated the correlation between PD-1/PD-L1 expression level and sepsis mortality, but the range of cells covered in previous articles was not comprehensive, and some were not sufficiently in-depth because the topic was not so focused (5, 10–13).
Different from those studies mentioned above, this review explores the role of PD-L1 in immunostimulatory and anti-inflammatory pathways, elucidates the intricate relationship between PD-L1 and the pathogenesis, development, and prognosis of sepsis, and summarizes current therapeutic approaches to PD-L1 in the treatment and prognosis of sepsis in preclinical and clinical studies, especially with a particular focus on PD-L1 expressed on neutrophils.
PD-1 (CD279) is a co-inhibitory receptor expressed in various locations, including the spleen, lymph nodes, bone marrow cells, and immature immune cells like CD4+CD8+T cells (11, 14–16). As a ligand for PD-1, PD-L1 (CD274) is widely expressed in hematopoietic cells and non-hematopoietic healthy tissue cells such as vascular endothelial cells and astrocytes (10). Another ligand of PD-1 is PD-L2 (CD273), which is mainly expressed in DCs and macrophages, but this review will not cover it in detail (17). Both PD-1 and PD-L1 belong to the type I transmembrane immunoglobulin (Ig) superfamily and interact via their extracellular domains, leading to a conformational change in PD-1. This prompts Src-family kinases to phosphorylate the inhibitory motif (ITIM) and switching motif (ITSM), attracting Src homology-2 containing protein tyrosine phosphatase 2 (SHP-2) and SHP-1 protein tyrosine phosphatases (9). SHP-2 dephosphorylates phosphatidylinositol 3 kinase (PI3K), thereby inhibiting Akt and ERK/MAPK signaling pathways. In the absence of SHP-2, SHP-1 acts as a compensator. Simultaneously, CD28 co-stimulatory receptors are also dephosphorylated, resulting in the inhibition of T lymphocyte activation (18–22).
The expression of PD-1 on different cells is regulated by a variety of factors. In T cells, PD-1 is increasingly expressed after antigen activation (16, 23). If the antigen is promptly eliminated, the PD-1 expression level on responding T cells decreases; otherwise, it remains elevated (24, 25). The expression of PD-1 on T cells is regulated by various factors, including activated T nuclear factor (NFAT), cytoplasm 1, Recombinant Forkhead Box Protein O1(FOXO1), T-bet, B lymphocyte-induced Maturation protein 1 (BLIMP-1), and serine-threonine kinase glycogen synthetase kinase 3 (GSK3) (26, 27). T cell receptor (TCR) activation is the primary controlling factor for PD-1 expression in T cells. Similarly, PD-L1, which is generally expressed in a variety of cells in an inflammatory environment, is regulated by multiple factors. Pro-inflammatory signals promote PD-L1 expression, in which interferon γ (IFN-γ) is considered the most effective soluble inducer (28). Also, noncoding RNAs are responsible for post-transcriptional regulation (28, 29). Additionally, protein cycling, ubiquitination, and glycosylation all influence PD-L1 expression levels (27, 30).
PD-1/PD-L1 plays a critical role in maintaining physiological health by downregulating inflammatory responses and restoring immune system balance. The interaction of PD-1 and PD-L1 facilitates autoimmune tolerance (31, 32). Reduction or deficiency of PD-L1 and PD-1 may result in species-specific autoimmunity (33, 34). Nishimura et al. found that IgG3 deposition in C57BL/6-PD-1(-/-) mice caused characteristic lupus-like proliferative arthritis and glomerulonephritis, and dilated cardiomyopathy occurred in BALB/c mice with PD-1 gene destruction (35, 36). Severe impaired myocardial contraction eventually leads to congestive heart failure and even sudden death. Wang et al. also found that PD-1 deficiency significantly increased the frequency of type 1 diabetes in mice (37). Moreover, the PD-1/PD-L1 pathway also regulates atherosclerotic inflammatory responses, as demonstrated by increased atherosclerotic lesions in animals with deficiency in low-density lipoprotein receptors and PD-L1 (38).
The interaction between PD-1 and PD-L1 is closely associated with the main mechanisms of sepsis, such as inhibiting T cells function, impairing myeloid cell function, and triggering non-immune cell death (11, 39, 40). The expression of their genes significantly increases during sepsis, and PD-L1 gene deficiency improves survival in septic patients (41). Even in sepsis survivors who develop chronic critical illness (CCI), serum sPD-L1 remains elevated (42). The role of PD-L2 in sepsis is unknown, and PD-L2 gene deletion has no significant effect on the mortality of sepsis mice (41).
The definition of sepsis has been revised to refer to life-threatening organ dysfunction caused by the host’s dysfunctional immune response to various microbial infections, rather than solely excessive inflammation (43). Numerous clinical studies over the past few decades have attempted to target various mediators, such as pattern recognition receptors (PRRs), pathogen-associated molecular patterns (PAMPs), and cytokines, to suppress excessive inflammation in sepsis (44–46). However, none of these studies have yielded clinically valid results, leading to a shift in research focus. For instance, administering a single dose of tumor necrosis factor receptor-FC (TNFR-Fc) fusion protein to 141 patients with septic shock not only failed to improve patient outcomes but also increased mortality in a dose-dependent manner (47). Additionally, patients with sepsis are more prone to nosocomial infections, indicating the possible presence of persistent immunosuppression (48–52). While the clinical manifestations of immune response disorders may vary, immune paralysis occurs early in sepsis and persists due to innate and acquired immune dysfunction (Figure 1) (9, 53).
Figure 1 Cellular network diagram of immunoparalysis in sepsis. (I) The expression of PD-1 on T cells and PD-L1 on other immune cells are upregulated during sepsis. (II) Phagocytosis of monocytes/macrophages is compromised, and cytokine production is reduced. (III) The differentiation, proliferation, and activation of DCs are impaired, with inhibitory effect on T cell function. The endotoxin-tolerant DCs express immunosuppressive genes and have direct inhibitory effects on other immune cells. (IV) The apoptosis of B cells increases and the number of Bregs increases. (V) Increased MDSCs inhibit T cells, decrease IL-6 production, and increase the number of Tregs. (VI) Exosomal PD-L1 and PD-L1 increase and induce T cell dysfunction. (VII) The phagocytosis and migration ability of neutrophils were weakened, and TNF-α production was reduced. Neutrophils promote T cell apoptosis, inhibit T cell activation, and increase the amount of Tregs through direct contact, while IFN-γ secreted by T cells in turn increases the number of PD-L1+ neutrophils. PD-1, programmed cell death- 1; PD-L1, programmed cell death-ligand 1; MHC, major history complex; TCR, T cell receptor; CTLA-4, cytotoxic T-lymphocyte associated protein 4; TGF, transforming growth factor; IL-6, interleukin 6; TNF-α, tumor necrosis factor α; IFN-γ, interferon γ; IRAK, interleukin receptor-associated kinase; ITSM, immunoreceptor tyrosine-based switching motif; ITIM, immunoreceptor tyrosine-based inhibitory motif; SHP1/2, Src homology-2 containing protein tyrosine phosphatase 1/2; PI3K, phosphatidylinositol 3 kinase; AKT (PKB), protein kinase B; ERK, extracellular regulated protein kinases; MAPK, mitogenactivated protein kinase; APC, antigen-presenting cell; DCs, dendritic cells; Bcl-2, B lymphoblastoma-2 gene.
Studies have found that the expression of both PD-1 and PD-L1 is upregulated on T cells and monocytes respectively (54–57). During early sepsis, activation of the PD-1/PD-L1 pathway leads to innate immune cell dysfunction, and treatment with anti-PD-L1 antibodies can reverse monocyte dysfunction and inhibit T-cell apoptosis (56). Studies have demonstrated that mice deficient in PD-1 or PD-L1 exhibit improved survival during early sepsis (54). Moreover, elevated PD-L1 expression in neutrophils is associated with a higher risk of sepsis-related mortality due to increased expression of inflammatory cytokines (57). In advanced sepsis, therapy with anti-PD-1 antibodies reactivates antigen-presenting cells and T cells, thereby mitigating secondary infections (58, 59).
Multiple studies have shown that the PD-1/PD-L1 pathway plays a significant role in the immunosuppression seen in sepsis (60). The expressions of PD-1 on T cells and PD-L1 on macrophages and endothelial cells increase during advanced sepsis (60). Interfering with this pathway can restore T-cell activity (61, 62). In a mouse model of Candida auris infection, it was found that the positive rate of PD-1 on T cells and the frequency of PD-L1 positive macrophages were significantly higher in infected mice compared to uninfected mice (63). Additionally, the PD-L1 expression level was strongly positively correlated with the fungal tissue load. In patients with severe corona virus disease 2019 (COVID-19), progressive lymphocytopenia and depletion of lymphocyte subsets were observed, and PD-1 expression on CD4+ and CD8+ T cells was significantly increased in patients with poor prognosis (64).
In addition to the aforementioned T cell activation disorders, the depletion of T lymphocytes by the PD-1/PD-L1 pathway is also a possible internal mechanism (65–68). In sepsis, T cells are constantly exposed to antigens and inflammatory signals, leading to gradual depletion and loss of effector function, and the long non-coding RNA HOTAIRM1 may be the culprit (69). Initially, T cells experience a reduction in their proliferative capacity and production of interleukin 2 (IL-2) (70), which is followed by decreased production of IFN-γ, TNF, and chemokines, ultimately resulting in immune paralysis (70). Lymphocyte activation gene 3 (LAG3) and PD-1 have a potential synergistic effect in regulating the progressive depletion of T cells in sepsis (71). Furthermore, Zinselmeyer et al. discovered that during persistent viral infection, immune paralysis is anatomically localized in the limbic region of the spleen/red marrow and characterized by drawn-out motor paralysis of virus-specific T cells (72). Planar bilayer data indicated that localized PD-L1 within the central supramolecular activation cluster (cSMAC) can hinder the movement of CD8+ T cells and promote stable p-ZAP-70 immune synapse formation (73). Moreover, soluble PD-L1 was negatively correlated with TCRβ, CD4, and CD8 mRNA (74). Moreover, phosphatidylcholine (PC), 2-ethyl-2-hydroxybutyric acid, and glyceraldehyde can also be used to modulate the PD-1 expression on CD4+ T cells with the help of related environmental factors such as IL-2 or Lac, thus affecting the 7-day prognosis of septic patients (75).
Under physiological conditions, regulatory T cells (Tregs) maintain self-tolerance by inhibiting the activation of autoreactive T cells. And Tregs also generate pathologic immunosuppression in sepsis by suppressing the functionality of specific immune cells (76). Previous research showed that, during sepsis, there was an increase in the number of Tregs, resulting in immune paralysis by inhibiting the function of effector T cells, monocytes, and neutrophils (76).
Umakoshi et al. observed a significant increase in bone marrow cells of mice and a decrease in circulating B cells 6 hours after cecal ligation and puncture (CLP) (77). Additionally, CD5-expressing regulatory B cells (B regs) were found to emerge and secrete IL-10, with increased levels of IL-10 and PD-L1 mRNA detected in the spleen. In the later stages of sepsis, the expression of the B lymphoblastoma-2 gene (Bcl-2) in the spleen gradually declined, while the pro-apoptotic protein Bim showed an obvious increase. These findings suggest that B-lymphocytopenia accompanied by the increase of B regs occurs early in sepsis and may contribute to immune paralysis during septic conditions. In addition, PD-1/PD-L1 may be involved with CD72/CD100 in the formation of immune disorders during human immunodeficiency virus (HIV)-1 infection. In addition to HIV-1-specific T-cell dysfunction, it was also observed that PD-1/PD-L1 and CD72/CD100 markers on B cells were significantly enhanced during active HIV-1 infection (78).
It is well-established that neutrophils undergo phenotypic, functional, and morphological changes in the circulatory system several hours after sepsis onset, and the upregulation of PD-L1 expression may play a key role in this process (Figure 2) (74, 79–82). Under physiological conditions, neutrophils eliminate pathogenic microorganisms through adhesion, migration, phagocytosis, and respiratory eruption (83). However, in sepsis, neutrophils have abnormal mobilization, delayed apoptosis, migration dysfunction, phagocytosis dysfunction, and other abnormal manifestation (84–88). At the same time, neutrophils also inhibited the function of lymphocytes through contact and non-contact inhibition (89, 90).
Figure 2 Dysfunction of PD-L1+ neutrophils. (I) The mobilization of PD-L1+ neutrophils is impaired. During fungal infection, β-glucans activate Dectin-1 and regulate the production of CXCL1/2, resulting in the accumulation of neutrophils in the bone marrow and further weakening the host immunity. (II) The migration dysfunction of PD-L1+ neutrophils and delayed apoptosis mediated by PKM2/STAT1 lead to excessive accumulation of neutrophils in non-targeted organs, resulting in organ damage. (III) Phagocytosis of PD-L1+ neutrophils is impaired, and the release of TNF-α is reduced. PD-L1, programmed cell death-ligand 1; JAK2, janus kinase 2; STAT3, recombinant signal transducer and activator of transcription 3; PKM2, recombinant pyruvate kinase M2; CXCL1/2, chemokine (C-X-C motif) ligand 1/2; TNF-α, tumor necrosis factor α.
Research has confirmed that PD-L1 expression on neutrophils increases during sepsis through the p38α−MSK1/−MK−2 pathway, and neutrophils migrate from the bone marrow to the blood and peritoneal cavity (81, 90). Patera et al. found that during sepsis, while the expression of PD-L1 was up-regulated, the ability of neutrophils to phagocytose bacteria was decreased, and the production of TNF-α was reduced, which may be related to immune paralysis (91). The impaired migration ability of PD-L1 positive neutrophils might also be associated with sepsis-induced immune paralysis, and septic neutrophils can induce lymphocyte apoptosis through direct contact, which can be reversed by anti-PD-L1 antibodies (81, 90). In addition, neutrophils can also inhibit T cell activation, promote T cell differentiation, and increase Tregs through direct contact (81). Yu et al. also found that during fungal sepsis of Candida albicans infection, PD-L1 expression is upregulated on mouse and human neutrophils. PD-L1 translocates into the nucleus under β-glucan-induction, modulates the production of chemokine (C-X-C motif) ligand 1/2 (CXCL1/2), and eventually leads to the accumulation of bone marrow neutrophils, weakening the host’s anti-infection ability (92). At the same time, IFN-γ secreted by T lymphocytes induces the production of polymorphonuclear leukocytes (PMN) with high PD-L1 expression via the JAK2/STAT1 pathway, and the Jak2 inhibitor fedratinib has been shown to significantly reduce PD-L1 expression levels in neutrophils (93). This suggests that the upregulation of PD-L1 expression on neutrophils, interacting with T cells, plays a critical role in immune paralysis. Both the p38α−MSK1/−MK−2 pathway and the JAK2/STAT1 pathway mentioned above have an impact on the expression of PD-L1 in neutrophils, but it is difficult to say which one is more important because the regulation of intracellular signal transduction often has a complex interactive network and many life activities are not controlled by a single signaling pathway. However, it has been found that the signal transduction of JAK-STAT1 may also depend on the activity of p38, and there may be a p38-JAK-STAT1 axis, so more studies need to be carried out to further explore the underlying mechanism (94). In addition, PD-L1-positive neutrophils have the potential to predict and diagnose sepsis (95).
Studies have shown that PD-1 and PD-L1 expression on mononuclear/macrophage increases at 24 hours of CLP treatment in mice (56). Macrophage phagocytosis and stimulus-induced cytokine production (TNF-α, IL-6, IL-1β, IFN-γ, and IL-12) were significantly decreased after CLP treatment, but random migration and cell diffusion were enhanced (54, 96). PD-1 on T cells and PD-L1 on macrophages are significantly up-regulated in mice infected with Candida auris AR-0384 (63). Shikonin can reduce PD-L1 expression on macrophages through phosphorylation of recombinant pyruvate kinase M2 (PKM2) and downregulation of nuclear input, and PKM2 can bind to the hormone receptor enzyme-1(HRE-1) and HRE-4 sites of the PD-L1 promoter (97). In addition, blood oxygen saturation at admission can be used as an effective predictor of monocyte PD-L1 expression level and immune response impairment (98). The ratio between the total level of activated CD86+ macrophages and the PD-1+ population in CLP mice was also associated with susceptibility to secondary fungal infection (99).
Previous studies have shown that in the early stage after CLP treatment, spleen DCs are mainly activated, and in the late stage, negative co-stimulatory molecules PD-L1 and PD-1 expression are up-regulated (100, 101). Their upregulation inhibits the activation and proliferation of DCs and affects the activation of T cells, thereby inducing immunosuppression (102). Tumor necrosis factor α-induced protein-8-like 1 (TIPE1) may play a negative regulatory role in sepsis by inhibiting DCs maturation and T cells functionality through PD-L1/PD-1 (103). In addition, endotoxin-tolerant DCs express negative regulatory genes of inflammation and have direct regulatory effects on other immune cells, which may be mediated by Interleukin-1 Receptor Associated Kinase (IRAK)-M and PD-L1 (104).
One important mechanism contributing to immune paralysis in sepsis is the expansion of myeloid-derived suppressor cells (MDSCs) (105–107). MDSCs are a heterogeneous population of immature bone marrow cells that inhibit antigen-specific activation of CD4+ and CD8+ T cells (108). Although typically undetected, elevated levels of MDSCs are observed in both cancer and sepsis, often accompanied by an increase in regulatory T cells (109–111). Hess et al. found that the increase of Tregs is mediated by transforming growth factor β (TGF-β) and IL-10 secreted by MDSCs (112). In addition, MDSCs may play a key inhibitory role on T cells through the PD-1/PD-L1 axis (53). Active Toll-like receptor 4 (TLR4) can induce monocyte MDSC expansion, thereby impacting antigen-specific T-cell initiation and IgG production (113). Clinical data analysis indicates a correlation between increased blood MDSC levels and a higher prevalence of nosocomial infections in septic patients (114). Ao et al. demonstrated that the Gr-1hi cells mediated by PD-1/PD-L1 is crucial for the development of immune paralysis in later stages of sepsis (115). The Gr-1hi cells were identified as MDSCs and exhibited a polymorphonuclear phenotype expressing CD11b and Ly6G markers (116). Following the injection of lipopolysaccharide (LPS) into mice treated with zymosan (ZM) on day 21, serum IL-6 production was reduced, while CD11b+ Gr-1hi cells accumulated in the peripheral blood (115). Additionally, transferring Gr-1hi cells to control mice decreased IL-6 production, but this inhibitory effect was not observed in PD-1/PD-L1-deficient d21-ZM mice. Conversely, treatment with anti-GR-1 monoclonal antibody (mAb) or anti-PD-1 and anti-PD-L1 mAb improved ZM-induced immune paralysis during sepsis induction. These findings suggest that the accumulation of Gr-1hi cells mediated by PD-1/PD-L1 is another critical contributor to immune paralysis in sepsis.
Studies have revealed that exosomes (EVs) play a crucial role in tumor-associated immune paralysis by acting as carriers for PD-L1 on PD-1 and inducing potent inhibitory signals (117–119). Recently, the involvement of EVs in the pathogenesis of sepsis has also attracted attention (120). EVs are lipid bilayer nanoparticles containing RNA, DNA, and proteins (121). Studies have shown that normal cells or tumor cells release exosomes through exocytosis, which are multivesicular body (MVB) formed by inward budding of vesicles in the late endosome, and PD-L1 on the cell surface enters MVB during endocytosis (122). Huang et al. found that the expression level of circulating EVs in septic patients was higher than that in healthy controls, and it inhibited T cell function in a concentration-dependent manner, which was represented by significantly reduced expression of CD69, up-regulated expression of PD-1 and increased proportion of Treg, which may be one of the mechanisms leading to immunosuppression of sepsis (119). Kawamoto et al. reported the presence of PD-L1 and PD-L2 on circulating exosomes in the plasma of septic patients and found elevated levels of β2 integrin and PD-L2 on exosomes in these patients (123). Although there was no significant difference in PD-L1 levels on exosomes, the overall concentration of sPD-L1 increased. Furthermore, the expression levels of sPD-L1 and leukocyte β2 integrin were strongly associated with organ dysfunction. Further investigation is warranted to explore the role of exosomal PD-L1 and PD-L2 in sepsis-induced immune paralysis.
Sepsis is a condition that can result in multiple organ failure, leading to fatal outcomes, and the interaction of PD-1/PD-L1 with CD80, PI3K/Akt pathway, or STAT1 molecules plays an important role in the mechanism of sepsis-induced organ dysfunction as described below (124, 125).
The lung is so vulnerable to sepsis that respiratory dysfunction has been reported to almost 70% (126). And sepsis-induced lung damage is a leading cause of mortality in patients (127, 128). In a study investigating lung injury in neonatal sepsis, Fallon et al. observed significant increases in pulmonary edema (PE), neutrophil infiltration, myeloperoxidase (MPO) levels, and cytokine expression in wild-type (WT) mice 24 hours after treatment with cecal serous fluid (CS) (129). Neonatal mice lacking PD-1 (PD-1−/−) showed improved survival rates compared to WT mice, particularly with noticeable differences in lung damage. However, the survival rate of neonatal mice lacking PD-L1 (PD-L1−/−) did not show significant improvement. Additionally, the number of PD-1+ cells in the lungs of human newborns with intrauterine infection was prominently higher than those who died from non-infectious causes (129). Therefore, PD-1 plays a pivotal role in the mechanism of sepsis-induced lung injury, and PD-1/PD-L1 inhibitors may be potential therapeutic targets (130). Alfred Ayala et al. constructed a mouse model of sepsis-induced lung injury using septic aggression-induced sepsis after hemorrhagic shock (Hem-CLP) and found that PD-L1 expression was significantly upregulated on vascular endothelial cells (ECs) or lung epithelial cells (EpiCs) in mice with indirect acute lung injury (iALI) 24 hours after sepsis injury (131). Moreover, inhibiting PD-L1 expression on ECs using PD-L1 siRNA encapsulated by liposomes inhibited the iALI-induced increase in cytokine/chemokine levels, as well as pulmonary myeloperoxidase and caspase 3 activities. This treatment also preserved normal tissue structure, alleviated pulmonary edema, and reduced neutrophil influx caused by iALI. However, inhibiting PD-L1 expression on EpiCs through endotracheal administration did not yield the same effects. Thus, it can be concluded that ECs, but not EpiCs, play a significant role in sepsis-induced lung injury and are closely associated with PD-L1 expression. Zona occludens-1 (ZO-1), a protein found within ECs in the lungs of WT mice, is known to shift from membranous to perinuclear position 24 hours after treatment, while PD1−/− mice retain the membranous position (129). In summary, both the presence or absence of the PD-1 gene and the level of PD-L1 expression on ECs have a profound impact on lung injury induced by sepsis.
Although the precise mechanisms have not yet been fully elucidated, they generally involve the following aspects. Firstly, the upregulation of PD-1 expression on immune cells and PD-L1 expression on ECs can impair the barrier function of ECs and increase monolayer permeability (132). Moreover, under TNF-α stimulation, the expression of EC connexin on EC monolayer of PD-L1− mice was increased in vitro and EC activation was decreased through the angiopoietin/Tie2 pathway (132). Additionally, PD-L1 plays a key role in regulating the suppression of iALI by Treg cells, which may be related to the activation of SHP-1 of lung tissue (133, 134). The activation of SHP-1 is associated with the loss of the protective effect of Tregs in vivo (135). Equally important, PD-L1 binds to the p85 subunit of PI3K on the endoplasmic reticulum (ER) of neutrophils, inhibiting autophagy through the PI3K/Akt/mTOR pathway and promoting the release of neutrophil extracellular traps (NETs) (136). This process leads to severe acute inflammatory lung injury, including acute respiratory distress syndrome (ARDS) (136). Li et al. found that PKM2/STAT1 mediates the up-regulation of PD-L1 expression on neutrophils and its anti-apoptotic effect, which may lead to the increase of pulmonary neutrophils accumulation and promote the occurrence of lung injury (137). Additionally, Gao et al. found through in vitro studies that PD-L1 regulates LPS-induced inflammation in EpiCs and vascular ECs by interacting with the hypoxia-inducible factor 1α (HIF-1α) signaling pathway. Downregulation of HIF-1α can reduce PD-L1 expression, which further inhibits HIF-1α protein expression and related pathways. The exact mechanism underlying this relationship has yet to be clarified (138). In addition, Group 2 innate lymphoid cells (ILC2s) may also mediate pulmonary immune homeostasis through PD-1 (139).
Previous studies have demonstrated that PD-L1 expression in the liver is significantly increased at mRNA transcription and immunohistochemical levels following CLP treatment compared to sham-operated controls (140–142). When hepatitis B virus (HBV)-associated cirrhosis is complicated with severe sepsis (SS), HBV-related acute-on-chronic liver failure (HBV-ACLF) can be caused by the superposition of monocyte PD-L1 up-regulation, and monocyte PD-L1 expression can also predict the 28-day mortality of HBV-ACLF (142). Anti-PD-L1 antibodies have shown significant improvement in liver injury morphology in CLP mice by reducing glutamic pyruvic transaminase (ALT) and glutamic oxaloacetic transaminase (AST) release, as well as decreasing TNF-α, interleukin (IL)-6, and IL-10 mRNA levels in the liver after sepsis (143).
Hutchins et al. found that intrahepatic Kupffer cells can exacerbate hepatic sinusoid endothelial cell damage during sepsis by binding to PD-L1 (140). It is PKM2/STAT1 that mediates the up-regulation of PD-L1 expression of neutrophils and promotes the intrahepatic accumulation of neutrophils (137). However, recent studies have challenged the conventional hypothesis by suggesting that high expression of PD-L1 on liver cells can ameliorate liver damage and improve survival in mice with sepsis (144). Liver damage during sepsis is associated with the activation of cytotoxic T lymphocytes (CTLs), and PD-L1 serves as a co-receptor that negatively regulates T cell function (144). Downregulation of PD-L1 in hepatocytes has been observed in mouse sepsis models, and restoration of PD-L1 expression through adenovirus- and transposon-based gene transfer significantly improved survival and reduced liver injury. Therefore, administration of recombinant PD-L1 or inhibition of NADPH oxidase type 2 (NOX2) activity may offer new treatment options for sepsis (144). In conclusion, further studies are needed to elucidate the role of PD-1/PD-L1 in the pathogenesis of sepsis-induced liver injury.
Regarding the brain, sepsis-associated encephalopathy (SAE) is characterized by acute and long-term cognitive impairment (145–147). While enhanced PD-L1 expression following surgical brain injury (SBI) can regulate neuroimmune and inflammatory responses through PD-L1+ astrocytes for self-protection and promote nerve repair, the opposite effect occurs in brain injury caused by sepsis (148). Our previous data showed that during sepsis, PD-L1 binds to P-Y705-Stat3, promoting nuclear translocation of PD-L1 and enhancing the transcription of GSDMD, resulting in increased release of neutrophil extracellular traps (NETs) (149). Neutrophils and NETs contribute to blood-brain barrier breakdown in the hippocampus, neuronal apoptosis, microglia activation, and hippocampal-dependent memory impairment (150–153). Treatment with anti-Gr-1 antibodies or DNase I has been shown to attenuate these sepsis-induced changes (149).
Sepsis is one of the leading causes of acute kidney injury (AKI) in the ICU, and its occurrence correlates positively with patient mortality (154–157). The pathogenesis of septic AKI is not yet fully understood. Serum sPD-L1 levels were significantly elevated in sepsis patients with impaired renal function (158). Xu et al. established a septic AKI model induced by CLP and found increased expressions of PD-1 and PD-L1 in septic AKI mice, leading to T cell apoptosis (159). Compared to the sham group, the number of lymphocytes was reduced by 64% in sepsis mice, including a 27% decrease in CD3+ T cells. The results also suggest that lactate upregulates PD-L1 expression in the kidney, and blocking the lactate receptor or PD-1/PD-L1 signaling may provide a novel treatment approach for septic AKI.
PD-1 upregulation in the spleen is observed early in sepsis, and spleen cell apoptosis increases over time (53, 100, 160). As evident from the aforementioned studies, the persistent expansion of myeloid-derived suppressor cells (MDSCs) during sepsis is closely associated with immune paralysis, characterized by splenocyte apoptosis, decreased T cell numbers, and upregulated PD-1 expression (114). Moreover, when human ghrelin and human growth hormone (GH) are used to correct immune paralysis, a decrease in PD-1 expression is observed in the spleens of elderly rats with sepsis (161). Additionally, the administration of anti-human PD-L1 nanobody KN035 alleviates splenocyte apoptosis, as well as lung and liver damage induced by septicemia in humanized mice, ultimately improving survival (162). These findings illustrate the close association between PD-1/PD-L1 and spleen injury caused by sepsis, although the specific mechanisms require further exploration.
Increased permeability of intestinal epithelia plays a vital role in the pathophysiology of numbers of gastrointestinal diseases, and its mucosal immune system plays a key role in the development and regulation of the immune system (163). Intestinal barrier dysfunction or increased intestinal permeability is a key component in the development of MODS during sepsis (164). PD-L1 is constitutively expressed in epithelial cells of the colon and stomach, contributing to the interaction between epithelial cells and lymphocytes in specific cases, participating in intestinal mucosal inflammation, and regulating intestinal immune tolerance (19). However, during sepsis, PD-L1 expression in mouse intestinal epithelial cells (IECs) is significantly increased, ileum permeability is increased, and tight junction (TJ) proteins (claudin-1, occludin, and ZO-1 proteins) are lost, resulting in severe intestinal injury (57, 165). Moreover, PD-L1 antibodies prevented the development of colitis in mice (166).
PD-1/PD-L1 has emerged as a critical player in the pathogenesis of sepsis, and numerous treatment approaches targeting immune checkpoints have shown a promising role of targeting PD-L1 against sepsis in preclinical and clinical studies (167–169).
Several anti-PD-L1 antibodies have been used in tumor therapy and achieved certain efficacy, including atezolizumab, avelumab, and durvalumab (55, 91, 170–173). Meanwhile, a large number of studies have shown that specific antibodies can also reverse the negative effects of high PD-1/PD-L1 expression in sepsis, both in terms of immunoparalysis and organ damage (66, 115, 136). Zhao et al. demonstrated that the anti-human PD-L1 nanobody KN035 alleviated sepsis-induced spleen cell apoptosis, as well as lung and liver injury in humanized mouse models. This led to an improvement in the overall survival rate (162).
Additionally, regulation of the expression level of PD-L1 has shown promising therapeutic potential. miR-142 can reduce CLP-induced inflammation by targeting PD-L1 in macrophages, thereby reducing sepsis (174). Shikonin, a PKM2 inhibitor extracted from a herbal medicine, also significantly decreases the PD-L1 expression on macrophages and alleviates various immune paralyzing factors through PKM2 phosphorylation and the downregulation of nuclear input (97). Farnesyl transferase inhibitor (FTI)-277, in a dose-dependent manner, downregulated PD-L1 in spleen lymphocytes of septic mice and mitigated sepsis-induced apoptosis of spleen lymphocytes with nuclear factor-κB (NF-κB) (175). The nuclear factor erythroid 2-related factor 2 (Nrf2) can interfere with the induction of PD-L1 and inhibit the expression of PD-L1 in the later stage of sepsis, to reduce the occurrence of immunosuppression in sepsis (176). Ascorbic acid prevents sepsis-induced organ dysfunction through the p-STAT1/PD-L1 signaling pathway (177). Zusanli (ST36), Guanyuan (CV4), and Qihai (CV6) acupoint electroacupuncture modulated the immune function of sepsis patients through the PD-1/PD-L1 pathway and improved clinical symptoms (169). Furthermore, adoptive transfer of bone marrow-derived dendritic cells (BMDCs), niacinamide nucleoside supplementation, fibronectin FN C-terminal heparin-binding domain polypeptide (rhFNHC-36), glutamine (GLN), recombinant enhancer of zeste homolog 2 (EZH2) inhibitor GSK343, mitogen-activated protein kinase phosphatase 1 (Mkp-1), mycophenolate mofetil (MMF), anti-ICAM (intercellular adhesion molecule)-1 antibody and other methods have shown improved survival rates in septic mice (178–184). Notably, downregulation of PD-L1 expression has been observed in these approaches.
The involvement of neutrophils in sepsis is notable, and more and more studies showed that PD-L1 positive neutrophils were crucial for the development of inflammatory and immunological disturbance (173). The reduction in immunosuppression subsequently decreased the apoptosis rate of T lymphocytes, thereby improving the survival rate in septic mice (173). In addition, β-glucan induces an increase in the production of the chemokine CXCL1/2 by PD-L1, which leads to the accumulation of bone marrow neutrophils, weakening the host’s ability to resist fungal infection, and therefore can be used as a potential target for the treatment of fungal sepsis (92). Treatment with anti-PD-L1 antibodies or DNase I has been shown to attenuate the sepsis-induced changes in the lungs and brains caused by neutrophils (136, 149, 185).
Sepsis is more prevalent in the elderly population (186–189). The percentage of PD-1+ T cells and Tregs increased in elderly patients with sepsis (190). Wang et al. found that combined treatment with human growth hormone (Ghr) and human growth hormone (GH) prevented the loss of spleen T cells in elderly septic rats, thereby reducing lymphocyte apoptosis. This treatment also inhibited an increase in Treg cell number and PD-1 expression (191). They further revealed that human Ghrelin and GH can inhibit TGF-β production in a vagal-dependent manner, thus correcting immunosuppression in elderly septic rats. Treatment reduced PD-1 expression in the spleen of elderly septic rats, increased human leukocyte antigen-DR (HLA-DR) expression, alleviated lymphocyte reduction, and decreased caspase-3 levels (161, 192, 193).
The area under ROC curve (AUC) of serum soluble PD-L1 (sPD-L1) combined with Sequential Organ Failure Assessment (SOFA) score is known to be of considerable value in the diagnosis of sepsis, and during the first week of ICU treatment, sPD-L1 was a valuable predictor of severe sepsis and septic shock severity and 28-day mortality (194–196). sPD-1 levels and CRP and PCT levels were positively correlated, so the correlation between sPD-1 and inflammatory markers may also serve as a potential biomarker for the diagnosis of sepsis (197).
In the therapy aspect, A Phase 1b randomized study evaluated the relevant aspects of Nivolumab (198). Nivolumab is a monoclonal antibody targeting PD-1 and it is approved for the treatment of various cancers (199–202). The study found that the pharmacokinetic profile of Nivolumab (480 mg or 960 mg) resulted in a receptor occupancy greater than >90% for at least 28 days, with no evidence of worsening symptoms such as fever, shock, or cytokine storms. Watanabe et al. found that when treated with Nivolumab, there was an observed increase in absolute lymphocyte counts and monocyte HLA-DR subtype expression levels over time (203). The incidence of adverse events in the 480 mg and 960 mg groups was reported as 80% and 50%, respectively. Notably, only one drug-related adverse event was observed in the 480 mg group, and no Nivolumab-related deaths occurred. In conclusion, a single dose of 960 mg of Nivolumab demonstrated good tolerability and maintained adequate blood concentration of the drug. Furthermore, both the 480 mg and 960 mg doses of Nivolumab appeared to improve immune system markers for the study (203). Moreover, Zusanli (ST36), Guanyuan (CV4) and Qihai (CV6) acupoint electroacupuncture can also regulate the immune function of septic patients through PD-1 pathway and improve clinical symptoms (169).
However, van den Haak et al. found that PD-1 suppression at a single high dose of 480mg or 960mg of Nivolumab lasted for more than 90 days in most cases, while the duration of sepsis was 7-10 days, so it may induce long-term immune-related side effects (204). In contrast, a single dose of 20mg of Nivolumab (median 23 days and effective) may be more appropriate as a therapeutic dose for sepsis. In addition, Hong et al. found that using selective beta-blockers (especially atenolol) improved sepsis incidence and course, significantly reduced serum sPD-L1 levels, and facilitated ROS-induced NF-κB and STAT3 activation, thus down-regulating PD-L1 expression on monocytes/macrophages (205). In addition, since PD-1 inhibitors often require modification to improve stability, poor modification may result in a higher incidence of irAEs than PD-L1 inhibitors (206). And PD-1 inhibitors block both PD-1/PD-L1 and PD-1/PD-L2 pathway simultaneously, thus reducing local homeostasis of macrophages, which may also increase the incidence of irAEs (207). But other studies have shown no significant difference, and there is currently no comparison between the two in the field of sepsis treatment (208, 209).
Coronavirus disease 19 (COVID-19) is a viral sepsis characterized by lymphocytopenia, which is particularly prominent in severe cases of COVID-19. One of the main changes in these patients was the increased counts of neutrophils and decreased counts of lymphocyte, thus the neutrophil-lymphocyte-ratio is a potential marker of severity of COVID-19 (210–212). Additionally, co-inhibitory molecules such as PD-1, PD-L1, cytotoxic T-lymphocyte-associated protein 4 (CTLA-4), and T cell immunoglobulin domain and mucin domain-3 (TIM-3) are found to be overexpressed in CD4+ and CD8+ T cells, and high levels of PD-L1 expression are associated with lymphocytopenia and increased mortality in COVID-19 patients (211, 213).
Vitamin D is a potentially effective treatment for COVID-19 (214–217). It was reported that vitamin D reduced PD-L1 levels when serum PD-L1 was very high, and vice versa (218). At the same time, vitamin D can also reduce PD-L1 expression by reducing pro-inflammatory cytokines such as IL-6, TNF-γ, etc (219, 220). But vitamin D supplementation also increased the expression of PD-L1 on Tregs, increased the depletion of T cells, and worsen the immunoparalysis (221). Therefore, the use of vitamin D might be a choice in treating COVID-19.
These findings suggested the potential use of anti-PD-1/PD-L1 antibodies in COVID-19 patients with or without cancer. Currently, there are five clinical trials registered on clinicaltrials.gov aiming to investigate the efficacy of anti-PD-1 antibodies in treating COVID-19 (NCT04333914, NCT04268537, NCT04356508, NCT04343144, NCT04413838). These studies include patients with metastatic and advanced cancer who have also been affected by COVID-19, as well as obese patients with COVID-19 infection. The trials aim to assess the effectiveness of various anti-PD-1 antibodies, such as nivolumab, either in combination with standard treatment regimens (NCT04333914) or as standalone treatments (222).
Despite the accumulation of preclinical evidence demonstrating the efficacy of various treatments, there is still a lack of robust clinical studies supporting their use. Immune checkpoint inhibitors (ICIs) used in cancer treatment research have been associated with immune-related adverse events (irAEs), such as rashes, colitis, thyroiditis, and pneumonia (223–225). Additionally, ICIs can also lead to systemic hyperinflammatory syndrome, although it is less common (226). Therefore, it is evident that PD-1/PD-L1-related treatments in sepsis may have similar side effects (227). The therapeutic effect was related to the immune status and initial pathogen load before treatment (228, 229). Studies have shown that anti-PD-L1 treatment failed to improve survival rates in a fatal Staphylococcus aureus pneumonia mouse model, but the exact reason is unclear (230).
To improve the positive rate of clinical trials and reduce side effects, many new ideas and attempts have been produced. For example, the above-mentioned KN035 is derived from a single-domain antibody with a lower molecular weight than normal monoclonal antibodies, which may give it more favorable physical and chemical properties, but the exact mechanism and effects are still unknown (162).
Peptide immune checkpoint antagonists present a potential alternative drug model. Unlike sustained blocking of the PD-1 pathway by antibodies, peptide-based therapies offer a rapid pharmacokinetic profile that reduces the likelihood of irAEs. In a mouse model of Candida albicans sepsis, Hotchkiss et al. evaluated the efficacy of a novel short-acting anti-PD-L1 peptide called compound 8, which demonstrated a twofold increase in survival compared to the control group (231). Gutierrez et al. also reported an effective peptide-based PD-1 checkpoint antagonist (LD01) that significantly improved survival by enhancing macrophage phagocytosis activity and T-cell production of IFN-γ (232).
The lack of success in clinical trials may be attributed to the use of animal models established using relatively uniform “inducers” in genetically homogenous strains of laboratory animals, which do not fully capture the pathophysiology of human sepsis and the heterogeneity of patient populations. Heterogeneity in patient factors such as genetic and social backgrounds, cause of sepsis, personal medical history, and disease course (233). Accurate sepsis diagnosis and clinical classification can help improve treatment efficacy to some extent. For example, early administration of Nivolumab 6mg/kg in combination with the antibiotic meropenem fully alleviated bacterial sepsis when the initial pathogen load was below 3,000 CFU/μL, but not when the initial load was above 5,000 CFU/μL (229). Artificial intelligence (AI) may also be valuable in this area. AI has shown a promising ability to predict early-stage organ dysfunction, such as acute kidney injury and ARDS, leading to improved outcomes (234). Furthermore, AI has facilitated the unprecedented classification of four sepsis subgroups based on big data analysis, which might guide a more precision clinical treatment (12).
Besides its therapeutic effects, PD-1/PD-L1 pathway is also promising in prognostic prediction both in terms of acquired immune cells and innate immune cells (235, 236). Li et al. conducted a two-phase cohort study to assess the predictive effect of PD-1 on 28-day mortality in sepsis patients (237). The analysis included a total of 120 patients, with 58 patients in phase I (test set) and 62 patients in phase II (validation set). The findings revealed that the expression of PD-1 in Tregs and the Sequential Organ Failure Assessment (SOFA) score were independent risk factors for 28-day mortality. Moreover, the expression of PD-1 on CD4+ and memory CD8+ T cells and the PD-1/CD28 ratio in CD8+ T cells are also significantly correlated with the severity and prognosis of sepsis patients (238–241). Furthermore, Zeng et al. conducted a cohort study involving 114 patients, demonstrating that the percentage of PD-L1+ NK cell and the SOFA score were independent risk factors for 28-day mortality (242). PD-L1 expression levels on monocytes, DCs, and neutrophils combined with SOFA or APACHE II scores have also been used to predict sepsis mortality (90, 142, 243–245). Overall, the evaluation of PD-1 and PD-L1 expression in immune cells is promising in prognostic prediction for sepsis patients. These markers, along with traditional factors such as SOFA score, provide valuable insights into patient outcomes, allowing for tailored treatment strategies and improved clinical decision-making.
PD-1/PD-L1 is known to play a crucial role in the occurrence and development of sepsis, affecting the functionality of various immune cells and the release of immune factors, which leads to dual functional abnormalities in innate and acquired immunity (241, 246, 247). During sepsis, the expression levels of PD-1/PD-L1 on T lymphocytes, B lymphocytes, neutrophils, macrophages, myeloid suppressor cells, and exosomes show significant differences compared to the control group, resulting in immunoparalysis (248–250). PD-1/PD-L1 may mediate the damage and dysfunction of organs such as the lung, liver, brain, kidney, spleen, and intestines in sepsis, although the specific mechanism requires further investigation (141, 251). While many preclinical and preliminary studies (Table 1) on PD-1/PD-L1 have shown promising results, large-scale clinical studies are warranted to confirm its therapeutic effect against sepsis (257).
YC: Writing – original draft, Writing – review & editing. DG: Writing – original draft, Writing – review & editing. CZ: Conceptualization, Writing – original draft. SR: Writing – review & editing. CS: Writing – original draft. YW: Conceptualization, Supervision, Writing – review & editing. JW: Conceptualization, Funding acquisition, Supervision, Writing – review & editing.
The author(s) declare financial support was received for the research, authorship, and/or publication of this article. This work was supported by the National Natural Science Foundation of China (82072147, 82272214); the Sci-Tech Innovation 2030 Brain Science and Brain-Like Intelligence Technology Project (2022ZD0208100); and Shanghai Rising-Star Program (21QA1411800).
All the figures in this review are made by BioRender (https://app.biorender.com/).
The authors declare that the research was conducted in the absence of any commercial or financial relationships that could be construed as a potential conflict of interest.
All claims expressed in this article are solely those of the authors and do not necessarily represent those of their affiliated organizations, or those of the publisher, the editors and the reviewers. Any product that may be evaluated in this article, or claim that may be made by its manufacturer, is not guaranteed or endorsed by the publisher.
1. Mayr FB, Yende S, Angus DC. Epidemiology of severe sepsis. Virulence (2014) 5:4–11. doi: 10.4161/viru.27372.Epub2013Dec11
2. Rudd KE, Johnson SC, Agesa KM, Shackelford KA, Tsoi D, Kievlan DR, et al. Global, regional, and national sepsis incidence and mortality 1990-2017: analysis for the global burden of disease study. Lancet (2020) 395:200–11. doi: 10.1016/S0140-6736(19)32989-7
3. Gavelli F, Castello LM, Avanzi GC. Management of sepsis and septic shock in the emergency department. Intern Emerg Med (2021) 16:1649–61. doi: 10.1007/s11739-021-02735-7
4. Prescott HC, Osterholzer JJ, Langa KM, Angus DC, Iwashyna TJ. Late mortality after sepsis: propensity matched cohort study. BMJ (2016) 353:i2375. doi: 10.1136/bmj.i2375
5. Liu D, Huang SY, Sun JH, Zhang HC, Cai QL, Gao C, et al. Sepsis-induced immunosuppression: mechanisms, diagnosis and current treatment options. Mil Med Res (2022) 9:56. doi: 10.1186/s40779-022-00422-y
6. Evans L, Rhodes A, Alhazzani W, Antonelli M, Coopersmith CM, French C, et al. Surviving sepsis campaign: international guidelines for management of sepsis and septic shock 2021. Intensive Care Med (2021) 47:1181–247. doi: 10.1007/s00134-021-06506-y
7. Opal SM. New perspectives on immunomodulatory therapy for bacteraemia and sepsis. Int J Antimicrob Agents (2010) 36 Suppl 2:S70–3. doi: 10.1016/j.ijantimicag.2010.11.008
8. Delsesto D, Opal SM. Future perspectives on regulating pro-and anti-inflammatory responses in sepsis. Contrib Microbiol (2011) 17:137–56. doi: 10.1159/000324030
9. Venet F, Monneret G. Advances in the understanding and treatment of sepsis-induced immunosuppression. Nat Rev Nephrol (2018) 14:121–37. doi: 10.1038/nrneph.2017.165
10. Keir ME, Butte MJ, Freeman GJ, Sharpe AH. PD-1 and its ligands in tolerance and immunity. Annu Rev Immunol (2008) 26:677–704. doi: 10.1146/annurev.immunol.26.021607.090331
11. Gianchecchi E, Delfino DV, Fierabracci A. Recent insights into the role of the PD-1/PD-L1 pathway in immunological tolerance and autoimmunity. Autoimmun Rev (2013) 12:1091–100. doi: 10.1016/j.autrev.2013.05.003
12. Nakamori Y, Park EJ, Shimaoka M. Immune deregulation in sepsis and septic shock: reversing immune paralysis by targeting PD-1/PD-L1 pathway. Front Immunol (2020) 11:624279. doi: 10.3389/fimmu.2020.624279
13. Zhang T, Yu-Jing L, Ma T. Role of regulation of PD-1 and PD-L1 expression in sepsis. Front Immunol (2023) 14:1029438. doi: 10.3389/fimmu.2023.1029438
14. Liang SC, Latchman YE, Buhlmann JE, Tomczak MF, Horwitz BH, Freeman GJ, et al. Regulation of PD-1, PD-L1, and PD-L2 expression during normal and autoimmune responses. Eur J Immunol (2003) 33:2706–16. doi: 10.1002/eji.200324228
15. Liu Q, Lv Y, Zhao M, Jin Y, Lu J. PD-L1 blockade improves immune dysfunction of spleen dendritic cells and T-cells in zymosan-induced multiple organs dysfunction syndromes. Int J Clin Exp Pathol (2015) 8:1374–83.
16. Dammeijer F, van Gulijk M, Mulder EE, Lukkes M, Klaase L, van den Bosch T, et al. The PD-1/PD-L1-checkpoint restrains T cell immunity in tumor-draining lymph nodes. Cancer Cell (2020) 38:685–700.e8. doi: 10.1016/j.ccell.2020.09.001
17. Fan Z, Wu C, Chen M, Jiang Y, Wu Y, Mao R, et al. The generation of PD-L1 and PD-L2 in cancer cells: From nuclear chromatin reorganization to extracellular presentation. Acta Pharm Sin B (2022) 12:1041–53. doi: 10.1016/j.apsb.2021.09.010
18. Chemnitz JM, Parry RV, Nichols KE, June CH, Riley JL. SHP-1 and SHP-2 associate with immunoreceptor tyrosine-based switch motif of programmed death 1 upon primary human T cell stimulation, but only receptor ligation prevents T cell activation. J Immunol (2004) 173:945–54. doi: 10.4049/jimmunol.173.2.945
19. Sharpe AH, Wherry EJ, Ahmed R, Freeman GJ. The function of programmed cell death 1 and its ligands in regulating autoimmunity and infection. Nat Immunol (2007) 8:239–45. doi: 10.1038/ni1443
20. Fernandes RA, Su L, Nishiga Y, Ren J, Bhuiyan AM, Cheng N, et al. Immune receptor inhibition through enforced phosphatase recruitment. Nature (2020) 586:779–84. doi: 10.1038/s41586-020-2851-2
21. Marasco M, Berteotti A, Weyershaeuser J, Thorausch N, Sikorska J, Krausze J, et al. Molecular mechanism of SHP2 activation by PD-1 stimulation. Sci Adv (2020) 6:eaay4458. doi: 10.1126/sciadv.aay4458
22. Doroshow DB, Bhalla S, Beasley MB, Sholl LM, Kerr KM, Gnjatic S, et al. PD-L1 as a biomarker of response to immune-checkpoint inhibitors. Nat Rev Clin Oncol (2021) 18:345–62. doi: 10.1038/s41571-021-00473-5
23. Tomino A, Tsuda M, Aoki R, Kajita Y, Hashiba M, Terajima T, et al. Increased PD-1 expression and altered T cell repertoire diversity predict mortality in patients with septic shock: A preliminary study. PLoS One (2017) 12:e0169653. doi: 10.1371/journal.pone.0169653
24. Crawford A, Angelosanto JM, Kao C, Doering TA, Odorizzi PM, Barnett BE, et al. Molecular and transcriptional basis of CD4+ T cell dysfunction during chronic infection. Immunity (2014) 40:289–302. doi: 10.1016/j.immuni.2014.01.005
25. Pauken KE, Wherry EJ. Overcoming T cell exhaustion in infection and cancer. Trends Immunol (2015) 36:265–76. doi: 10.1016/j.it.2015.02.008
26. Schildberg FA, Klein SR, Freeman GJ, Sharpe AH. Coinhibitory pathways in the B7-CD28 ligand-receptor family. Immunity (2016) 44:955–72. doi: 10.1016/j.immuni.2016.05.002
27. Taylor A, Harker JA, Chanthong K, Stevenson PG, Zuniga EI, Rudd CE. Glycogen synthase kinase 3 inactivation drives T-bet-mediated downregulation of co-receptor PD-1 to enhance CD8(+) cytolytic T cell responses. Immunity (2016) 44:274–86. doi: 10.1016/j.immuni.2016.01.018
28. Langereis JD, Pickkers P, De Kleijn S, Gerretsen J, De Jonge MI, Kox M. Spleen-derived IFN-γ induces generation of PD-L1(+)-suppressive neutrophils during endotoxemia. J Leukoc Biol (2017) 102:1401–9. doi: 10.1189/jlb.3A0217-051RR
29. Danbaran GR, Aslani S, Sharafkandi N, Hemmatzadeh M, Hosseinzadeh R, Azizi G, et al. How microRNAs affect the PD-L1 and its synthetic pathway in cancer. Int Immunopharmacol (2020) 84:106594. doi: 10.1016/j.intimp.2020.106594
30. Sun C, Mezzadra R, Schumacher TN. Regulation and function of the PD-L1 checkpoint. Immunity (2018) 48:434–52. doi: 10.1016/j.immuni.2018.03.014
31. Park JJ, Omiya R, Matsumura Y, Sakoda Y, Kuramasu A, Augustine MM, et al. B7-H1/CD80 interaction is required for the induction and maintenance of peripheral T-cell tolerance. Blood (2010) 116:1291–8. doi: 10.1182/blood-2010-01-265975
32. Boussiotis VA. Molecular and biochemical aspects of the PD-1 checkpoint pathway. N Engl J Med (2016) 375:1767–78. doi: 10.1056/NEJMra1514296
33. Probst HC, Mccoy K, Okazaki T, Honjo T, van den Broek M. Resting dendritic cells induce peripheral CD8+ T cell tolerance through PD-1 and CTLA-4. Nat Immunol (2005) 6:280–6. doi: 10.1038/ni1165
34. Okazaki T, Honjo T. The PD-1-PD-L pathway in immunological tolerance. Trends Immunol (2006) 27:195–201. doi: 10.1016/j.it.2006.02.001
35. Nishimura H, Nose M, Hiai H, Minato N, Honjo T. Development of lupus-like autoimmune diseases by disruption of the PD-1 gene encoding an ITIM motif-carrying Immunoreceptor. Immunity (1999) 11:141–51. doi: 10.1016/S1074-7613(00)80089-8
36. Nishimura H, Okazaki T, Tanaka Y, Nakatani K, Hara M, Matsumori A, et al. Autoimmune dilated cardiomyopathy in PD-1 receptor-deficient mice. Science (2001) 291:319–22. doi: 10.1126/science.291.5502.319
37. Wang J, Yoshida T, Nakaki F, Hiai H, Okazaki T, Honjo T. Establishment of NOD-Pdcd1-/- mice as an efficient animal model of type I diabetes. Proc Natl Acad Sci U S A (2005) 102:11823–8. doi: 10.1073/pnas.0505497102
38. Gotsman I, Grabie N, Dacosta R, Sukhova G, Sharpe A, Lichtman AH. Proatherogenic immune responses are regulated by the PD-1/PD-L pathway in mice. J Clin Invest (2007) 117:2974–82. doi: 10.1172/JCI31344
39. Freeman GJ, Long AJ, Iwai Y, Bourque K, Chernova T, Nishimura H, et al. Engagement of the PD-1 immunoinhibitory receptor by a novel B7 family member leads to negative regulation of lymphocyte activation. J Exp Med (2000) 192:1027–34. doi: 10.1084/jem.192.7.1027
40. Okazaki T, Honjo T. PD-1 and PD-1 ligands: from discovery to clinical application. Int Immunol (2007) 19:813–24. doi: 10.1093/intimm/dxm057
41. Rossi AL, Le M, Chung CS, Chen Y, Fallon EA, Matoso A, et al. A novel role for programmed cell death receptor ligand 2 in sepsis-induced hepatic dysfunction. Am J Physiol Gastrointest Liver Physiol (2019) 316:G106–g114. doi: 10.1152/ajpgi.00204.2018
42. Stortz JA, Murphy TJ, Raymond SL, Mira JC, Ungaro R, Dirain ML, et al. Evidence for persistent immune suppression in patients who develop chronic critical illness after sepsis. Shock (2018) 49:249–58. doi: 10.1097/SHK.0000000000000981
43. Singer M, Deutschman CS, Seymour CW, Shankar-Hari M, Annane D, Bauer M, et al. The third international consensus definitions for sepsis and septic shock (Sepsis-3). JAMA (2016) 315:801–10. doi: 10.1001/jama.2016.0287
44. Benjamim CF, Lundy SK, Lukacs NW, Hogaboam CM, Kunkel SL. Reversal of long-term sepsis-induced immunosuppression by dendritic cells. Blood (2005) 105:3588–95. doi: 10.1182/blood-2004-08-3251
45. Unsinger J, Burnham CA, Mcdonough J, Morre M, Prakash PS, Caldwell CC, et al. Interleukin-7 ameliorates immune dysfunction and improves survival in a 2-hit model of fungal sepsis. J Infect Dis (2012) 206:606–16. doi: 10.1093/infdis/jis383
46. Shindo Y, Fuchs AG, Davis CG, Eitas T, Unsinger J, Burnham CD, et al. Interleukin 7 immunotherapy improves host immunity and survival in a two-hit model of Pseudomonas aeruginosa pneumonia. J Leukoc Biol (2017) 101:543–54. doi: 10.1189/jlb.4A1215-581R
47. Fisher CJ, Agosti JMJR, Opal SM, Lowry SF, Balk RA, Sadoff JC, et al. Treatment of septic shock with the tumor necrosis factor receptor:Fc fusion protein. The Soluble TNF Receptor Sepsis Study Group. N Engl J Med (1996) 334:1697–702. doi: 10.1056/NEJM199606273342603
48. Segreti J. Nosocomial infections and secondary infections in sepsis. Crit Care Clin (1989) 5:177–89. doi: 10.1016/S0749-0704(18)30457-3
49. Otto GP, Sossdorf M, Claus RA, Rödel J, Menge K, Reinhart K, et al. The late phase of sepsis is characterized by an increased microbiological burden and death rate. Crit Care (2011) 15:R183. doi: 10.1186/cc10332
50. Denstaedt SJ, Singer BH, Standiford TJ. Sepsis and nosocomial infection: patient characteristics, mechanisms, and modulation. Front Immunol (2018) 9:2446. doi: 10.3389/fimmu.2018.02446
51. Samantaray S, Karan P, Sharma A, Nag V, Dutt N, Garg MK, et al. Prevalence, presentation and outcome of secondary bloodstream infections among COVID-19 patients. Infect Disord Drug Targets (2022) 22:e180422203723. doi: 10.2174/1871526522666220418093450
52. Zhao GJ, Jiang DW, Cai WC, Chen XY, Dong W, Chen LW, et al. CD71(+) erythroid cell expansion in adult sepsis: potential causes and role in prognosis and nosocomial infection prediction. Front Immunol (2022) 13:830025. doi: 10.3389/fimmu.2022.830025
53. Ruan WS, Feng MX, Xu J, Xu YG, Song CY, Lin LY, et al. Early activation of myeloid-derived suppressor cells participate in sepsis-induced immune suppression via PD-L1/PD-1 axis. Front Immunol (2020) 11:1299. doi: 10.3389/fimmu.2020.01299
54. Huang X, Venet F, Wang YL, Lepape A, Yuan Z, Chen Y, et al. PD-1 expression by macrophages plays a pathologic role in altering microbial clearance and the innate inflammatory response to sepsis. Proc Natl Acad Sci U S A (2009) 106:6303–8. doi: 10.1073/pnas.0809422106
55. Brahmamdam P, Inoue S, Unsinger J, Chang KC, Mcdunn JE, Hotchkiss RS. Delayed administration of anti-PD-1 antibody reverses immune dysfunction and improves survival during sepsis. J Leukoc Biol (2010) 88:233–40. doi: 10.1189/jlb.0110037
56. Zhang Y, Zhou Y, Lou J, Li J, Bo L, Zhu K, et al. PD-L1 blockade improves survival in experimental sepsis by inhibiting lymphocyte apoptosis and reversing monocyte dysfunction. Crit Care (2010) 14:R220. doi: 10.1186/cc9354
57. Huang X, Chen Y, Chung CS, Yuan Z, Monaghan SF, Wang F, et al. Identification of B7-H1 as a novel mediator of the innate immune/proinflammatory response as well as a possible myeloid cell prognostic biomarker in sepsis. J Immunol (2014) 192:1091–9. doi: 10.4049/jimmunol.1302252
58. Chang KC, Burnham CA, Compton SM, Rasche DP, Mazuski RJ, Mcdonough JS, et al. Blockade of the negative co-stimulatory molecules PD-1 and CTLA-4 improves survival in primary and secondary fungal sepsis. Crit Care (2013) 17:R85. doi: 10.1186/cc12711
59. Vu CTB, Thammahong A, Yagita H, Azuma M, Hirankarn N, Ritprajak P, et al. Blockade of PD-1 attenuated Postsepsis aspergillosis via the activation of IFN-γ and the dampening of IL-10. Shock (2020) 53:514–24. doi: 10.1097/SHK.0000000000001392
60. Zhang Y, Li J, Lou J, Zhou Y, Bo L, Zhu J, et al. Upregulation of programmed death-1 on T cells and programmed death ligand-1 on monocytes in septic shock patients. Crit Care (2011) 15:R70. doi: 10.1186/cc10059
61. Boomer JS, To K, Chang KC, Takasu O, Osborne DF, Walton AH, et al. Immunosuppression in patients who die of sepsis and multiple organ failure. JAMA (2011) 306:2594–605. doi: 10.1001/jama.2011.1829
62. West EE, Jin HT, Rasheed AU, Penaloza-Macmaster P, Ha SJ, Tan WG, et al. PD-L1 blockade synergizes with IL-2 therapy in reinvigorating exhausted T cells. J Clin Invest (2013) 123:2604–15. doi: 10.1172/JCI67008
63. Wurster S, Albert ND, Kontoyiannis DP. Candida auris bloodstream infection induces upregulation of the PD-1/PD-L1 immune checkpoint pathway in an immunocompetent mouse model. mSphere (2022) 7:e0081721. doi: 10.1128/msphere.00817-21
64. Sabbatino F, Conti V, Franci G, Sellitto C, Manzo V, Pagliano P, et al. PD-L1 dysregulation in COVID-19 patients. Front Immunol (2021) 12:695242. doi: 10.3389/fimmu.2021.695242
65. Guignant C, Lepape A, Huang X, Kherouf H, Denis L, Poitevin F, et al. Programmed death-1 levels correlate with increased mortality, nosocomial infection and immune dysfunctions in septic shock patients. Crit Care (2011) 15:R99. doi: 10.1186/cc10112
66. Chang K, Svabek C, Vazquez-Guillamet C, Sato B, Rasche D, Wilson S, et al. Targeting the programmed cell death 1: programmed cell death ligand 1 pathway reverses T cell exhaustion in patients with sepsis. Crit Care (2014) 18:R3. doi: 10.1186/cc13176
67. Gao DN, Yang ZX, Qi QH. Roles of PD-1, Tim-3 and CTLA-4 in immunoregulation in regulatory T cells among patients with sepsis. Int J Clin Exp Med (2015) 8:18998–9005.
68. Thampy LK, Remy KE, Walton AH, Hong Z, Liu K, Liu R, et al. Restoration of T Cell function in multi-drug resistant bacterial sepsis after interleukin-7, anti-PD-L1, and OX-40 administration. PLoS One (2018) 13:e0199497. doi: 10.1371/journal.pone.0199497
69. Chen W, Liu J, Ge F, Chen Z, Qu M, Nan K, et al. Long noncoding RNA HOTAIRM1 promotes immunosuppression in sepsis by inducing T cell exhaustion. J Immunol (2022) 208:618–32. doi: 10.4049/jimmunol.2100709
70. Wherry EJ, Kurachi M. Molecular and cellular insights into T cell exhaustion. Nat Rev Immunol (2015) 15:486–99. doi: 10.1038/nri3862
71. Niu B, Zhou F, Su Y, Wang L, Xu Y, Yi Z, et al. Different expression characteristics of LAG3 and PD-1 in sepsis and their synergistic effect on T cell exhaustion: A new strategy for immune checkpoint blockade. Front Immunol (2019) 10:1888. doi: 10.3389/fimmu.2019.01888
72. Zinselmeyer BH, Heydari S, Sacristán C, Nayak D, Cammer M, Herz J, et al. PD-1 promotes immune exhaustion by inducing antiviral T cell motility paralysis. J Exp Med (2013) 210:757–74. doi: 10.1084/jem.20121416
73. Monks CR, Freiberg BA, Kupfer H, Sciaky N, Kupfer A. Three-dimensional segregation of supramolecular activation clusters in T cells. Nature (1998) 395:82–6. doi: 10.1038/25764
74. Derigs M, Heers H, Lingelbach S, Hofmann R, Hänze J. Soluble PD-L1 in blood correlates positively with neutrophil and negatively with lymphocyte mRNA markers and implies adverse sepsis outcome. Immunol Res (2022) 70:698–707. doi: 10.1007/s12026-022-09302-y
75. Bu Y, Wang H, Ma X, Han C, Jia X, Zhang J, et al. Untargeted metabolomic profiling of the correlation between prognosis differences and PD-1 expression in sepsis: A preliminary study. Front Immunol (2021) 12:594270. doi: 10.3389/fimmu.2021.594270
76. Venet F, Pachot A, Debard AL, Bohe J, Bienvenu J, Lepape A, et al. Human CD4+CD25+ regulatory T lymphocytes inhibit lipopolysaccharide-induced monocyte survival through a Fas/Fas ligand-dependent mechanism. J Immunol (2006) 177:6540–7. doi: 10.4049/jimmunol.177.9.6540
77. Umakoshi K, Choudhury ME, Nishioka R, Matsumoto H, Abe N, Nishikawa Y, et al. B lymphocytopenia and Bregs in a not-to-die murine sepsis model. Biochem Biophys Res Commun (2020) 523:202–7. doi: 10.1016/j.bbrc.2019.12.041
78. Correa-Rocha R, Lopez-Abente J, Gutierrez C, Pérez-Fernández VA, Prieto-Sánchez A, Moreno-Guillen S, et al. CD72/CD100 and PD-1/PD-L1 markers are increased on T and B cells in HIV-1+ viremic individuals, and CD72/CD100 axis is correlated with T-cell exhaustion. PLoS One (2018) 13:e0203419. doi: 10.1371/journal.pone.0203419
79. Demaret J, Venet F, Friggeri A, Cazalis MA, Plassais J, Jallades L, et al. Marked alterations of neutrophil functions during sepsis-induced immunosuppression. J Leukoc Biol (2015) 98:1081–90. doi: 10.1189/jlb.4A0415-168RR
80. Shen XF, Cao K, Jiang JP, Guan WX, Du JF. Neutrophil dysregulation during sepsis: an overview and update. J Cell Mol Med (2017) 21:1687–97. doi: 10.1111/jcmm.13112
81. Qi X, Yu Y, Sun R, Huang J, Liu L, Yang Y, et al. Identification and characterization of neutrophil heterogeneity in sepsis. Crit Care (2021) 25:50. doi: 10.1186/s13054-021-03481-0
82. Zhang H, Wang Y, Qu M, Li W, Wu D, Cata JP, et al. Neutrophil, neutrophil extracellular traps and endothelial cell dysfunction in sepsis. Clin Transl Med (2023) 13:e1170. doi: 10.1002/ctm2.1170
83. Dumas A, Pouliot M. [Neutrophil: foe or friend?]. Med Sci (Paris) (2009) 25:699–704. doi: 10.1051/medsci/2009258-9699
84. Taneja R, Parodo J, Jia SH, Kapus A, Rotstein OD, Marshall JC. Delayed neutrophil apoptosis in sepsis is associated with maintenance of mitochondrial transmembrane potential and reduced caspase-9 activity. Crit Care Med (2004) 32:1460–9. doi: 10.1097/01.CCM.0000129975.26905.77
85. Chiswick EL, Mella JR, Bernardo J, Remick DG. Acute-phase deaths from murine polymicrobial sepsis are characterized by innate immune suppression rather than exhaustion. J Immunol (2015) 195:3793–802. doi: 10.4049/jimmunol.1500874
86. Mare TA, Treacher DF, Shankar-Hari M, Beale R, Lewis SM, Chambers DJ, et al. The diagnostic and prognostic significance of monitoring blood levels of immature neutrophils in patients with systemic inflammation. Crit Care (2015) 19:57. doi: 10.1186/s13054-015-0778-z
87. Li X, Kondo Y, Bao Y, Staudenmaier L, Lee A, Zhang J, et al. Systemic adenosine triphosphate impairs neutrophil chemotaxis and host defense in sepsis. Crit Care Med (2017) 45:e97–e104. doi: 10.1097/CCM.0000000000002052
88. Patel JM, Sapey E, Parekh D, Scott A, Dosanjh D, Gao F, et al. Sepsis induces a dysregulated neutrophil phenotype that is associated with increased mortality. Mediators Inflammation (2018) 2018:4065362. doi: 10.1155/2018/4065362
89. Darcy CJ, Minigo G, Piera KA, Davis JS, Mcneil YR, Chen Y, et al. Neutrophils with myeloid derived suppressor function deplete arginine and constrain T cell function in septic shock patients. Crit Care (2014) 18:R163. doi: 10.1186/cc14003
90. Wang JF, Li JB, Zhao YJ, Yi WJ, Bian JJ, Wan XJ, et al. Up-regulation of programmed cell death 1 ligand 1 on neutrophils may be involved in sepsis-induced immunosuppression: an animal study and a prospective case-control study. Anesthesiology (2015) 122:852–63. doi: 10.1097/ALN.0000000000000525
91. Patera AC, Drewry AM, Chang K, Beiter ER, Osborne D, Hotchkiss RS. Frontline Science: Defects in immune function in patients with sepsis are associated with PD-1 or PD-L1 expression and can be restored by antibodies targeting PD-1 or PD-L1. J Leukoc Biol (2016) 100:1239–54. doi: 10.1189/jlb.4HI0616-255R
92. Yu Y, Wang RR, Miao NJ, Tang JJ, Zhang YW, Lu XR, et al. PD-L1 negatively regulates antifungal immunity by inhibiting neutrophil release from bone marrow. Nat Commun (2022) 13:6857. doi: 10.1038/s41467-022-34722-7
93. Huang J, Sun R, Yang Y, Li L, Liu L, Shao Y, et al. Splenic T lymphocytes induce the formation of immunosuppressive neutrophils through IFN-γ in sepsis. Inflammation Res (2022) 71:81–91. doi: 10.1007/s00011-021-01524-4
94. Shan X, Hu P, Ni L, Shen L, Zhang Y, Ji Z, et al. Serine metabolism orchestrates macrophage polarization by regulating the IGF1-p38 axis. Cell Mol Immunol (2022) 19:1263–78. doi: 10.1038/s41423-022-00925-7
95. Meghraoui-Kheddar A, Chousterman BG, Guillou N, Barone SM, Granjeaud S, Vallet H, et al. Two new neutrophil subsets define a discriminating sepsis signature. Am J Respir Crit Care Med (2022) 205:46–59. doi: 10.1164/rccm.202104-1027OC
96. Ayala A, Elphick GF, Kim YS, Huang X, Carreira-Rosario A, Santos SC, et al. Sepsis-induced potentiation of peritoneal macrophage migration is mitigated by programmed cell death receptor-1 gene deficiency. J Innate Immun (2014) 6:325–38. doi: 10.1159/000355888
97. Yuan L, Wang Y, Chen Y, Chen X, Li S, Liu X. Shikonin inhibits immune checkpoint PD-L1 expression on macrophage in sepsis by modulating PKM2. Int Immunopharmacol (2023) 121:110401. doi: 10.1016/j.intimp.2023.110401
98. Avendaño-Ortiz J, Maroun-Eid C, Martín-Quirós A, Lozano-Rodríguez R, Llanos-González E, Toledano V, et al. Oxygen saturation on admission is a predictive biomarker for PD-L1 expression on circulating monocytes and impaired immune response in patients with sepsis. Front Immunol (2018) 9:2008. doi: 10.3389/fimmu.2018.02008
99. Vu CTB, Thammahong A, Leelahavanichkul A, Ritprajak P. Alteration of macrophage immune phenotype in a murine sepsis model is associated with susceptibility to secondary fungal infection. Asian Pac J Allergy Immunol (2022) 40:162–71. doi: 10.12932/AP-170519-0565
100. Liu Q, Lu JY, Wang XH, Qu BJ, Li SR, Kang JR. Changes in the PD-1 and PD-L1 expressions of splenic dendritic cells in multiple-organ dysfunction syndrome mice and their significance. Genet Mol Res (2014) 13:7666–72. doi: 10.4238/2014.September.26.4
101. Wang F, Yang MY, Wang B, Qu ZL, Hu QR. [Effects of PD-L1 on immunosuppression of bacterial sepsis and its relevant mechanism]. Zhongguo Ying Yong Sheng Li Xue Za Zhi (2021) 37:606–10. 10.12047/j.cjap.6112.2021.091
102. Wang Z, Xie Z, Zhao Y, Bu T, Yu A, Wang S. [Effect of dendritic cells on immune function regulated by programmed cell death-1/programmed cell death-ligand 1 in sepsis]. Zhonghua Wei Zhong Bing Ji Jiu Yi Xue (2021) 33:1032–9.
103. Luan YY, Zhang L, Zhu FJ, Dong N, Lu JY, Yao YM. Effect of TIPE1 on immune function of dendritic cells and its signaling pathway in septic mice. J Infect Dis (2019) 220:699–709. doi: 10.1093/infdis/jiz158
104. Zhou Y, Xia Q, Wang X, Fu S. Endotoxin tolerant dendritic cells suppress inflammatory responses in splenocytes via interleukin-1 receptor associated kinase (IRAK)-M and programmed death-ligand 1 (PDL-1). Med Sci Monit (2018) 24:4798–806. doi: 10.12659/MSM.908242
105. Schrijver IT, Théroude C, Roger T. Myeloid-derived suppressor cells in sepsis. Front Immunol (2019) 10:327. doi: 10.3389/fimmu.2019.00327
106. Darden DB, Bacher R, Brusko MA, Knight P, Hawkins RB, Cox MC, et al. Single-cell RNA-seq of human myeloid-derived suppressor cells in late sepsis reveals multiple subsets with unique transcriptional responses: A pilot study. Shock (2021) 55:587–95. doi: 10.1097/SHK.0000000000001671
107. Zhang W, Fang X, Gao C, Song C, He Y, Zhou T, et al. MDSCs in sepsis-induced immunosuppression and its potential therapeutic targets. Cytokine Growth Factor Rev (2023) 69:90–103. doi: 10.1016/j.cytogfr.2022.07.007
108. Bruger AM, Vanhaver C, Bruderek K, Amodio G, Tavukçuoğlu E, Esendagli G, et al. Protocol to assess the suppression of T-cell proliferation by human MDSC. Methods Enzymol (2020) 632:155–92. doi: 10.1016/bs.mie.2019.05.046
109. Cuenca AG, Delano MJ, Kelly-Scumpia KM, Moreno C, Scumpia PO, Laface DM, et al. A paradoxical role for myeloid-derived suppressor cells in sepsis and trauma. Mol Med (2011) 17:281–92. doi: 10.2119/molmed.2010.00178
110. Albertsmeier M, Prix NJ, Winter H, Bazhin A, Werner J, Angele MK. Monocyte-dependent suppression of T-cell function in postoperative patients and abdominal sepsis. Shock (2017) 48:651–6. doi: 10.1097/SHK.0000000000000924
111. Gabrilovich DI. Myeloid-derived suppressor cells. Cancer Immunol Res (2017) 5:3–8. doi: 10.1158/2326-6066.CIR-16-0297
112. Hess NJ, Kink JA, Hematti P. Exosomes, MDSCs and Tregs: A new frontier for GVHD prevention and treatment. Front Immunol (2023) 14:1143381. doi: 10.3389/fimmu.2023.1143381
113. Tsukamoto H, Kozakai S, Kobayashi Y, Takanashi R, Aoyagi T, Numasaki M, et al. Impaired antigen-specific lymphocyte priming in mice after Toll-like receptor 4 activation via induction of monocytic myeloid-derived suppressor cells. Eur J Immunol (2019) 49:546–63. doi: 10.1002/eji.201847805
114. Uhel F, Azzaoui I, Grégoire M, Pangault C, Dulong J, Tadié JM, et al. Early expansion of circulating granulocytic myeloid-derived suppressor cells predicts development of nosocomial infections in patients with sepsis. Am J Respir Crit Care Med (2017) 196:315–27. doi: 10.1164/rccm.201606-1143OC
115. Ao X, Yang Y, Okiji T, Azuma M, Nagai S. Polymorphonuclear myeloid-derived cells that contribute to the immune paralysis are generated in the early phase of sepsis via PD-1/PD-L1 pathway. Infect Immun (2021) 89:e00771-20. doi: 10.1128/IAI.00771-20
116. Yamamoto Y, Ishigaki H, Ishida H, Itoh Y, Noda Y, Ogasawara K. Analysis of splenic Gr-1int immature myeloid cells in tumor-bearing mice. Microbiol Immunol (2008) 52:47–53. doi: 10.1111/j.1348-0421.2008.00009.x
117. Hashemian SM, Pourhanifeh MH, Fadaei S, Velayati AA, Mirzaei H, Hamblin MR. Non-coding RNAs and exosomes: their role in the pathogenesis of sepsis. Mol Ther Nucleic Acids (2020) 21:51–74. doi: 10.1016/j.omtn.2020.05.012
118. Murao A, Brenner M, Aziz M, Wang P. Exosomes in sepsis. Front Immunol (2020) 11:2140. doi: 10.3389/fimmu.2020.02140
119. Huang J, Huang G, Zhang C, Jian M, Li X, Jiang W. [Effect of circulating exosomes in patients with sepsis on T cell function]. Zhonghua Wei Zhong Bing Ji Jiu Yi Xue (2023) 35:586–91. doi: 10.3760/cma.j.cn121430-20230228-00122
120. Park EJ, Appiah MG, Myint PK, Gaowa A, Kawamoto E, Shimaoka M. Exosomes in sepsis and inflammatory tissue injury. Curr Pharm Des (2019) 25:4486–95. doi: 10.2174/1381612825666191116125525
121. Pegtel DM, Gould SJ. Exosomes. Annu Rev Biochem (2019) 88:487–514. doi: 10.1146/annurev-biochem-013118-111902
122. Poggio M, Hu T, Pai CC, Chu B, Belair CD, Chang A, et al. Suppression of exosomal PD-L1 induces systemic anti-tumor immunity and memory. Cell (2019) 177:414–427.e13. doi: 10.1016/j.cell.2019.02.016
123. Kawamoto E, Masui-Ito A, Eguchi A, Soe ZY, Prajuabjinda O, Darkwah S, et al. Integrin and PD-1 ligand expression on circulating extracellular vesicles in systemic inflammatory response syndrome and sepsis. Shock (2019) 52:13–22. doi: 10.1097/SHK.0000000000001228
124. Crouser E, Exline M, Knoell D, Wewers MD. Sepsis: links between pathogen sensing and organ damage. Curr Pharm Des (2008) 14:1840–52. doi: 10.2174/138161208784980572
125. Font MD, Thyagarajan B, Khanna AK. Sepsis and Septic Shock - Basics of diagnosis, pathophysiology and clinical decision making. Med Clin North Am (2020) 104:573–85. doi: 10.1016/j.mcna.2020.02.011
126. Kumar V. Pulmonary innate immune response determines the outcome of inflammation during pneumonia and sepsis-associated acute lung injury. Front Immunol (2020) 11:1722. doi: 10.3389/fimmu.2020.01722
127. Sadowitz B, Roy S, Gatto LA, Habashi N, Nieman G. Lung injury induced by sepsis: lessons learned from large animal models and future directions for treatment. Expert Rev Anti Infect Ther (2011) 9:1169–78. doi: 10.1586/eri.11.141
128. Nardiello C, Morty RE. World health observances in September 2020: sepsis, the lung and heart, and pulmonary fibrosis in focus. Am J Physiol Lung Cell Mol Physiol (2020) 319:L513–l517. doi: 10.1152/ajplung.00375.2020
129. Fallon EA, Chung CS, Heffernan DS, Chen Y, De Paepe ME, Ayala A. Survival and pulmonary injury after neonatal sepsis: PD1/PDL1's contributions to mouse and human immunopathology. Front Immunol (2021) 12:634529. doi: 10.3389/fimmu.2021.634529
130. Jia L, Liu K, Fei T, Liu Q, Zhao X, Hou L, et al. Programmed cell death-1/programmed cell death-ligand 1 inhibitors exert antiapoptosis and antiinflammatory activity in lipopolysaccharide stimulated murine alveolar macrophages. Exp Ther Med (2021) 21:400. doi: 10.3892/etm.2021.9831
131. Xu S, Yang Q, Bai J, Tao T, Tang L, Chen Y, et al. Blockade of endothelial, but not epithelial, cell expression of PD-L1 following severe shock attenuates the development of indirect acute lung injury in mice. Am J Physiol Lung Cell Mol Physiol (2020) 318:L801–l812. doi: 10.1152/ajplung.00108.2019
132. Lomas-Neira J, Monaghan SF, Huang X, Fallon EA, Chung CS, Ayala A. Novel role for PD-1:PD-L1 as mediator of pulmonary vascular endothelial cell functions in pathogenesis of indirect ARDS in mice. Front Immunol (2018) 9:3030. doi: 10.3389/fimmu.2018.03030
133. Iype T, Sankarshanan M, Mauldin IS, Mullins DW, Lorenz U. The protein tyrosine phosphatase SHP-1 modulates the suppressive activity of regulatory T cells. J Immunol (2010) 185:6115–27. doi: 10.4049/jimmunol.1000622
134. Tang L, Bai J, Chung CS, Lomas-Neira J, Chen Y, Huang X, et al. Programmed cell death receptor ligand 1 modulates the regulatory T cells' capacity to repress shock/sepsis-induced indirect acute lung injury by recruiting phosphatase SRC homology region 2 domain-containing phosphatase 1. Shock (2015) 43:47–54. doi: 10.1097/SHK.0000000000000247
135. Gu Q, Tung KS, Lorenz UM. Treg-specific deletion of the phosphatase SHP-1 impairs control of inflammation in vivo. Front Immunol (2023) 14:1139326. doi: 10.3389/fimmu.2023.1139326
136. Zhu CL, Xie J, Zhao ZZ, Li P, Liu Q, Guo Y, et al. PD-L1 maintains neutrophil extracellular traps release by inhibiting neutrophil autophagy in endotoxin-induced lung injury. Front Immunol (2022) 13:949217. doi: 10.3389/fimmu.2022.949217
137. Li Y, Tan R, Li R, Tian R, Liu Z, Wang X, et al. PKM2/STAT1-mediated PD-L1 upregulation on neutrophils during sepsis promotes neutrophil organ accumulation by serving an anti-apoptotic role. J Inflammation (Lond) (2023) 20:16. doi: 10.1186/s12950-023-00341-2
138. Zhao S, Gao J, Li J, Wang S, Yuan C, Liu Q. PD-L1 regulates inflammation in LPS-induced lung epithelial cells and vascular endothelial cells by interacting with the HIF-1α Signaling pathway. Inflammation (2021) 44:1969–81. doi: 10.1007/s10753-021-01474-3
139. Akama Y, Park EJ, Satoh-Takayama N, Gaowa A, Ito A, Kawamoto E, et al. Sepsis induces deregulation of IL-13 production and PD-1 expression in lung group 2 innate lymphoid cells. Shock (2021) 55:357–70. doi: 10.1097/SHK.0000000000001647
140. Hutchins NA, Wang F, Wang Y, Chung CS, Ayala A. Kupffer cells potentiate liver sinusoidal endothelial cell injury in sepsis by ligating programmed cell death ligand-1. J Leukoc Biol (2013) 94:963–70. doi: 10.1189/jlb.0113051
141. Zhu W, Bao R, Fan X, Tao T, Zhu J, Wang J, et al. PD-L1 blockade attenuated sepsis-induced liver injury in a mouse cecal ligation and puncture model. Mediators Inflammation (2013) 2013:361501. doi: 10.1155/2013/361501
142. Lu Y, Wang G, Li C. Expression of peripheral monocytic programmed death ligand-1 in severe sepsis combined with HBV-related cirrhosis. A pilot observational study. Cent Eur J Immunol (2021) 46:217–24. doi: 10.5114/ceji.2021.108179
143. Triantafyllou E, Gudd CL, Mawhin MA, Husbyn HC, Trovato FM, Siggins MK, et al. PD-1 blockade improves Kupffer cell bacterial clearance in acute liver injury. J Clin Invest (2021) 131:e140196. doi: 10.1172/JCI140196
144. von Knethen A, Schäfer A, Kuchler L, Knape T, Christen U, Hintermann E, et al. Tolerizing CTL by sustained hepatic PD-L1 expression provides a new therapy approach in mouse sepsis. Theranostics (2019) 9:2003–16. doi: 10.7150/thno.28057
145. Zhao L, Gao Y, Guo S, Lu X, Yu S, Ge ZZ, et al. Sepsis-associated encephalopathy: insight into injury and pathogenesis. CNS Neurol Disord Drug Targets (2021) 20:112–24. doi: 10.2174/1871527319999201117122158
146. Carter BL, Underwood J. Sepsis and the brain: a review for acute and general physicians. Clin Med (Lond) (2022) 22:392–5. doi: 10.7861/clinmed.2022-0346
147. Pan S, Lv Z, Wang R, Shu H, Yuan S, Yu Y, et al. Sepsis-induced brain dysfunction: pathogenesis, diagnosis, and treatment. Oxid Med Cell Longev (2022) 2022:1328729 . doi: 10.1155/2022/1328729
148. Gao X, Li W, Syed F, Yuan F, Li P, Yu Q. PD-L1 signaling in reactive astrocytes counteracts neuroinflammation and ameliorates neuronal damage after traumatic brain injury. J Neuroinflamm (2022) 19:43. doi: 10.1186/s12974-022-02398-x
149. Zhu CL, Xie J, Liu Q, Wang Y, Li HR, Yu CM, et al. PD-L1 promotes GSDMD-mediated NET release by maintaining the transcriptional activity of Stat3 in sepsis-associated encephalopathy. Int J Biol Sci (2023) 19:1413–29. doi: 10.7150/ijbs.79913
150. Moxon-Emre I, Schlichter LC. Neutrophil depletion reduces blood-brain barrier breakdown, axon injury, and inflammation after intracerebral hemorrhage. J Neuropathol Exp Neurol (2011) 70:218–35. doi: 10.1097/NEN.0b013e31820d94a5
151. Puy L, Corseaux D, Perbet R, Deramecourt V, Cordonnier C, Bérézowski V. Neutrophil extracellular traps (NETs) infiltrate haematoma and surrounding brain tissue after intracerebral haemorrhage: A post-mortem study. Neuropathol Appl Neurobiol (2021) 47:867–77. doi: 10.1111/nan.12733
152. Li C, Xing Y, Zhang Y, Hua Y, Hu J, Bai Y. Neutrophil extracellular traps exacerbate ischemic brain damage. Mol Neurobiol (2022) 59:643–56. doi: 10.1007/s12035-021-02635-z
153. Xie M, Hao Y, Feng L, Wang T, Yao M, Li H, et al. Neutrophil Heterogeneity and its Roles in the Inflammatory Network after Ischemic Stroke. Curr Neuropharmacol (2023) 21:621–50. doi: 10.2174/1570159X20666220706115957
154. Klenzak J, Himmelfarb J. Sepsis and the kidney. Crit Care Clin (2005) 21:211–22. doi: 10.1016/j.ccc.2005.01.002
155. Bellomo R, Kellum JA, Ronco C, Wald R, Martensson J, Maiden M, et al. Acute kidney injury in sepsis. Intensive Care Med (2017) 43:816–28. doi: 10.1007/s00134-017-4755-7
156. Poston JT, Koyner JL. Sepsis associated acute kidney injury. BMJ (2019) 364:k4891. doi: 10.1136/bmj.k4891
158. Loacker L, Egger A, Fux V, Bellmann-Weiler R, Weiss G, Griesmacher A, et al. Serum sPD-L1 levels are elevated in patients with viral diseases, bacterial sepsis or in patients with impaired renal function compared to healthy blood donors. Clin Chem Lab Med (2023) 61(12):2248–55. doi: 10.1515/cclm-2023-0232
159. Xu J, Ma X, Yu K, Wang R, Wang S, Liu R, et al. Lactate up-regulates the expression of PD-L1 in kidney and causes immunosuppression in septic Acute Renal Injury. J Microbiol Immunol Infect (2021) 54:404–10. doi: 10.1016/j.jmii.2019.10.006
160. Heffernan DS, Chung CS, Ayala A. Splenic invariant Natural Killer T-cells (iNKT-cells) play a significant role in the response to polymicrobial sepsis. Shock (2023) 60(3):443–9. doi: 10.1097/SHK.0000000000002185
161. Zhou M, Aziz M, Ochani M, Wang P. Correction of immunosuppression in aged septic rats by human ghrelin and growth hormone through the vagus nerve-dependent inhibition of TGF-β production. Mol Med (2020) 26:71. doi: 10.1186/s10020-020-00195-x
162. Zhao ZZ, Wang XL, Xie J, Chen LP, Li Q, Wang XX, et al. Therapeutic effect of an anti-human programmed death-ligand 1 (PD-L1) nanobody on polymicrobial sepsis in humanized mice. Med Sci Monit (2021) 27:e926820. doi: 10.12659/MSM.926820
163. Turner JR. Intestinal mucosal barrier function in health and disease. Nat Rev Immunol (2009) 9:799–809. doi: 10.1038/nri2653
164. Deitch EA. Role of the gut lymphatic system in multiple organ failure. Curr Opin Crit Care (2001) 7:92–8. doi: 10.1097/00075198-200104000-00007
165. Wu Y, Chung CS, Chen Y, Monaghan SF, Patel S, Huang X, et al. A novel role for programmed cell death receptor ligand-1 (PD-L1) in sepsis-induced intestinal dysfunction. Mol Med (2017) 22:830–40. doi: 10.2119/molmed.2016.00150
166. Kanai T, Totsuka T, Uraushihara K, Makita S, Nakamura T, Koganei K, et al. Blockade of B7-H1 suppresses the development of chronic intestinal inflammation. J Immunol (2003) 171:4156–63. doi: 10.4049/jimmunol.171.8.4156
167. Patil NK, Guo Y, Luan L, Sherwood ER. Targeting immune cell checkpoints during sepsis. Int J Mol Sci (2017) 18:2413. doi: 10.3390/ijms18112413
168. Rudick CP, Cornell DL, Agrawal DK. Single versus combined immunoregulatory approach using PD-1 and CTLA-4 modulators in controlling sepsis. Expert Rev Clin Immunol (2017) 13:907–19. doi: 10.1080/1744666X.2017.1357469
169. Yang G, Zheng B, Yu Y, Huang J, Zhu H, Deng D, et al. Electroacupuncture at Zusanli (ST36), Guanyuan (CV4), and Qihai (CV6) Acupoints Regulates Immune Function in Patients with Sepsis via the PD-1 Pathway. BioMed Res Int (2022) 2022:7037497. doi: 10.1155/2022/7037497
170. Rittmeyer A, Barlesi F, Waterkamp D, Park K, Ciardiello F, von Pawel J, et al. Atezolizumab versus docetaxel in patients with previously treated non-small-cell lung cancer (OAK): a phase 3, open-label, multicentre randomised controlled trial. Lancet (2017) 389:255–65. doi: 10.1016/S0140-6736(16)32517-X
171. Antonia SJ, Villegas A, Daniel D, Vicente D, Murakami S, Hui R, et al. Overall survival with durvalumab after chemoradiotherapy in stage III NSCLC. N Engl J Med (2018) 379:2342–50. doi: 10.1056/NEJMoa1809697
172. Motzer RJ, Penkov K, Haanen J, Rini B, Albiges L, Campbell MT, et al. Avelumab plus Axitinib versus Sunitinib for Advanced Renal-Cell Carcinoma. N Engl J Med (2019) 380:1103–15. doi: 10.1056/NEJMoa1816047
173. Chen J, Chen R, Huang S, Zu B, Zhang S. Atezolizumab alleviates the immunosuppression induced by PD−L1−positive neutrophils and improves the survival of mice during sepsis. Mol Med Rep (2021) 23:144. doi: 10.3892/mmr.2020.11783
174. Zhen J, Chen W. MiR-142 inhibits cecal ligation and puncture (CLP)-induced inflammation via inhibiting PD-L1 expression in macrophages and improves survival in septic mice. BioMed Pharmacother (2018) 97:1479–85. doi: 10.1016/j.biopha.2017.11.058
175. Antonangeli F, Natalini A, Garassino MC, Sica A, Santoni A, Di Rosa F. Regulation of PD-L1 expression by NF-κB in cancer. Front Immunol (2020) 11:584626. doi: 10.3389/fimmu.2020.584626
176. Zhang P, Wang Y, Yang W, Yin Y, Li C, Ma X, et al. 4-Octyl itaconate regulates immune balance by activating Nrf2 and negatively regulating PD-L1 in a mouse model of sepsis. Int J Biol Sci (2022) 18:6189–209. doi: 10.7150/ijbs.74456
177. Zhang X, Ji W, Deng X, Bo L. High-dose ascorbic acid potentiates immune modulation through STAT1 phosphorylation inhibition and negative regulation of PD-L1 in experimental sepsis. Inflammopharmacology (2023). doi: 10.1007/s10787-023-01319-5
178. Zhao YJ, Yi WJ, Wan XJ, Wang J, Tao TZ, Li JB, et al. Blockade of ICAM-1 improves the outcome of polymicrobial sepsis via modulating neutrophil migration and reversing immunosuppression. Mediators Inflammation (2014) 2014:195290. doi: 10.1155/2014/195290
179. Wang HW, Yang W, Gao L, Kang JR, Qin JJ, Liu YP, et al. Adoptive transfer of bone marrow-derived dendritic cells decreases inhibitory and regulatory T-cell differentiation and improves survival in murine polymicrobial sepsis. Immunology (2015) 145:50–9. doi: 10.1111/imm.12423
180. Hu YM, Hsiung YC, Pai MH, Yeh SL. Glutamine administration in early or late septic phase downregulates lymphocyte PD-1/PD-L1 expression and the inflammatory response in mice with polymicrobial sepsis. JPEN J Parenter Enteral Nutr (2018) 42:538–49. doi: 10.1177/0148607117695245
181. Barley TJ, Murphy PR, Wang X, Bowman BA, Mormol JM, Mager CE, et al. Mitogen-activated protein kinase phosphatase-1 controls PD-L1 expression by regulating type I interferon during systemic Escherichia coli infection. J Biol Chem (2022) 298:101938. doi: 10.1016/j.jbc.2022.101938
182. Wang Z, Guo Z, Wang X, Liao H, Chai Y, Wang Z, et al. Inhibition of EZH2 ameliorates sepsis acute lung injury (SALI) and non-small-cell lung cancer (NSCLC) proliferation through the PD-L1 pathway. Cells (2022) 11:3958. doi: 10.3390/cells11243958
183. Geng H, Wu Y, Chen Y. C-terminal fibronectin exerts beneficial effects in reducing tissue damage and modulating macrophage function in a murine septic model. J Inflammation Res (2023) 16:1509–21. doi: 10.2147/JIR.S398282
184. Zhao GJ, Yang XY, Zhang C, Dong W, Dong FB, Zhang J, et al. Supplementation with Nicotinamide riboside attenuates T cell exhaustion and improves survival in sepsis. Shock (2023) 60(2):238–47. doi: 10.1097/SHK.0000000000002153
185. Wang JF, Wang YP, Xie J, Zhao ZZ, Gupta S, Guo Y, et al. Upregulated PD-L1 delays human neutrophil apoptosis and promotes lung injury in an experimental mouse model of sepsis. Blood (2021) 138:806–10. doi: 10.1182/blood.2020009417
186. Lineberry C, Stein DE. Infection, sepsis, and immune function in the older adult receiving critical care. Crit Care Nurs Clin North Am (2014) 26:47–60. doi: 10.1016/j.ccell.2013.09.009
187. Clifford KM, Dy-Boarman EA, Haase KK, Maxvill K, Pass SE, Alvarez CA. Challenges with diagnosing and managing sepsis in older adults. Expert Rev Anti Infect Ther (2016) 14:231–41. doi: 10.1586/14787210.2016.1135052
188. Rowe TA, Mckoy JM. Sepsis in older adults. Infect Dis Clin North Am (2017) 31:731–42. doi: 10.1016/j.idc.2017.07.010
189. Yoshikawa TT, Reyes BJ, Ouslander JG. Sepsis in older adults in long-term care facilities: challenges in diagnosis and management. J Am Geriatr Soc (2019) 67:2234–9. doi: 10.1111/jgs.16194
190. Inoue S, Suzuki-Utsunomiya K, Okada Y, Taira T, Iida Y, Miura N, et al. Reduction of immunocompetent T cells followed by prolonged lymphopenia in severe sepsis in the elderly. Crit Care Med (2013) 41:810–9. doi: 10.1097/CCM.0b013e318274645f
191. Zhou M, Yang WL, Aziz M, Ma G, Wang P. Therapeutic effect of human ghrelin and growth hormone: Attenuation of immunosuppression in septic aged rats. Biochim Biophys Acta Mol Basis Dis (2017) 1863:2584–93. doi: 10.1016/j.bbadis.2017.01.014
192. Wang F, Huang X, Chung CS, Chen Y, Hutchins NA, Ayala A. Contribution of programmed cell death receptor (PD)-1 to Kupffer cell dysfunction in murine polymicrobial sepsis. Am J Physiol Gastrointest Liver Physiol (2016) 311:G237–45. doi: 10.1152/ajpgi.00371.2015
193. Xia Q, Wei L, Zhang Y, Sheng J, Wu W, Zhang Y. Immune checkpoint receptors tim-3 and PD-1 regulate monocyte and T lymphocyte function in septic patients. Mediators Inflammation (2018) 2018:1632902. doi: 10.1155/2018/1632902
194. Zhao Y, Jia Y, Li C, Shao R, Fang Y. Predictive value of soluble programmed death-1 for severe sepsis and septic shock during the first week in an intensive care unit. Shock (2019) 51:289–97. doi: 10.1097/SHK.0000000000001171
195. Sun S, Chen Y, Liu Z, Tian R, Liu J, Chen E, et al. Serum-soluble PD-L1 may be a potential diagnostic biomarker in sepsis. Scand J Immunol (2021) 94:e13049. doi: 10.1111/sji.13049
196. Yu X, Pan Y, Fei Q, Lin X, Chen Z, Huang H. Serum soluble PD-1 plays a role in predicting infection complications in patients with acute pancreatitis. Immun Inflammation Dis (2021) 9:310–8. doi: 10.1002/iid3.394
197. Bakhshiani Z, Fouladi S, Mohammadzadeh S, Eskandari N. Correlation of sPD1 with procalcitonin and C-reactive protein levels in patients with sepsis. Cell J (2021) 23:14–20. doi: 10.22074/cellj.2021.6941
198. Hotchkiss RS, Colston E, Yende S, Crouser ED, Martin GS, Albertson T, et al. Immune checkpoint inhibition in sepsis: a Phase 1b randomized study to evaluate the safety, tolerability, pharmacokinetics, and pharmacodynamics of nivolumab. Intensive Care Med (2019) 45:1360–71. doi: 10.1007/s00134-019-05704-z
199. Alsaab HO, Sau S, Alzhrani R, Tatiparti K, Bhise K, Kashaw SK, et al. PD-1 and PD-L1 checkpoint signaling inhibition for cancer immunotherapy: mechanism, combinations, and clinical outcome. Front Pharmacol (2017) 8:561. doi: 10.3389/fphar.2017.00561
200. Rotte A. Combination of CTLA-4 and PD-1 blockers for treatment of cancer. J Exp Clin Cancer Res (2019) 38:255. doi: 10.1186/s13046-019-1259-z
201. Friedman CF, Spencer C, Cabanski CR, Panageas KS, Wells DK, Ribas A, et al. Ipilimumab alone or in combination with nivolumab in patients with advanced melanoma who have progressed or relapsed on PD-1 blockade: clinical outcomes and translational biomarker analyses. J Immunother Cancer (2022) 10:e003853. doi: 10.1136/jitc-2021-003853
202. Ieranò C, Righelli D, D'Alterio C, Napolitano M, Portella L, Rea G, et al. In PD-1+ human colon cancer cells NIVOLUMAB promotes survival and could protect tumor cells from conventional therapies. J Immunother Cancer (2022) 10:e004032. doi: 10.1136/jitc-2021-004032
203. Watanabe E, Nishida O, Kakihana Y, Odani M, Okamura T, Harada T, et al. Pharmacokinetics, pharmacodynamics, and safety of nivolumab in patients with sepsis-induced immunosuppression: A multicenter, open-label phase 1/2 study. Shock (2020) 53:686–94. doi: 10.1097/SHK.0000000000001443
204. van den Haak DAC, Otten LS, Koenen H, Smeets RL, Piet B, Pickkers P, et al. Evidence-based rationale for low dose nivolumab in critically ill patients with sepsis-induced immunosuppression. Clin Transl Sci (2023) 16:978–86. doi: 10.1111/cts.13503
205. Hong SY, Lai CC, Teng NC, Chen CH, Hsu CC, Chan NJ, et al. Premorbid use of selective beta-blockers improves sepsis incidence and course: Human cohort and animal model studies. Front Med (Lausanne) (2023) 10:1105894. doi: 10.3389/fmed.2023.1105894
206. Elmakaty I, Abdo R, Elsabagh A, Elsayed A, Malki MI. Comparative efficacy and safety of PD-1/PD-L1 inhibitors in triple negative breast cancer: a systematic review and network meta-analysis of randomized controlled trials. Cancer Cell Int (2023) 23:90. doi: 10.1186/s12935-023-02941-7
207. Ramos-Casals M, Brahmer JR, Callahan MK, Flores-Chávez A, Keegan N, Khamashta MA, et al. Immune-related adverse events of checkpoint inhibitors. Nat Rev Dis Primers (2020) 6:38. doi: 10.1038/s41572-020-0160-6
208. Huang Q, Zheng Y, Gao Z, Yuan L, Sun Y, Chen H. Comparative efficacy and safety of PD-1/PD-L1 inhibitors for patients with solid tumors: A systematic review and Bayesian network meta-analysis. J Cancer (2021) 12:1133–43. doi: 10.7150/jca.49325
209. Yu H, Chen P, Cai X, Chen C, Zhang X, He L, et al. Efficacy and safety of PD-L1 inhibitors versus PD-1 inhibitors in first-line treatment with chemotherapy for extensive-stage small-cell lung cancer. Cancer Immunol Immunother (2022) 71:637–44. doi: 10.1007/s00262-021-03017-z
210. Diao B, Wang C, Tan Y, Chen X, Liu Y, Ning L, et al. Reduction and functional exhaustion of T cells in patients with coronavirus disease 2019 (COVID-19). Front Immunol (2020) 11:827. doi: 10.3389/fimmu.2020.00827
211. Tan L, Wang Q, Zhang D, Ding J, Huang Q, Tang YQ, et al. Lymphopenia predicts disease severity of COVID-19: a descriptive and predictive study. Signal Transduct Target Ther (2020) 5:33. doi: 10.1038/s41392-020-0148-4
212. Tan M, Liu Y, Zhou R, Deng X, Li F, Liang K, et al. Immunopathological characteristics of coronavirus disease 2019 cases in Guangzhou, China. Immunology (2020) 160:261–8. doi: 10.1111/imm.13223
213. Bidar F, Hamada S, Gossez M, Coudereau R, Lopez J, Cazalis MA, et al. Correction to: Recombinant human interleukin-7 reverses T cell exhaustion ex vivo in critically ill COVID-19 patients. Ann Intensive Care (2022) 12:30. doi: 10.1186/s13613-022-01007-7
214. Mercola J, Grant WB, Wagner CL. Evidence regarding vitamin D and risk of COVID-19 and its severity. Nutrients (2020) 12:3361. doi: 10.3390/nu12113361
215. Mohan M, Cherian JJ, Sharma A. Exploring links between vitamin D deficiency and COVID-19. PloS Pathog (2020) 16:e1008874. doi: 10.1371/journal.ppat.1008874
216. Aygun H. Vitamin D can reduce severity in COVID-19 through regulation of PD-L1. Naunyn Schmiedebergs Arch Pharmacol (2022) 395:487–94. doi: 10.1007/s00210-022-02210-w
217. Pereira M, Dantas Damascena A, Galvão Azevedo LM, De Almeida Oliveira T, Da Mota Santana J. Vitamin D deficiency aggravates COVID-19: systematic review and meta-analysis. Crit Rev Food Sci Nutr (2022) 62:1308–16. doi: 10.1080/10408398.2020.1841090
218. Morita M, Okuyama M, Akutsu T, Ohdaira H, Suzuki Y, Urashima M. Vitamin D supplementation regulates postoperative serum levels of PD-L1 in patients with digestive tract cancer and improves survivals in the highest quintile of PD-L1: A Post Hoc analysis of the AMATERASU randomized controlled trial. Nutrients (2021) 13:1987. doi: 10.3390/nu13061987
219. Kraus AU, Penna-Martinez M, Shoghi F, Meyer G, Badenhoop K. Monocytic cytokines in autoimmune polyglandular syndrome type 2 are modulated by vitamin D and HLA-DQ. Front Immunol (2020) 11:583709. doi: 10.3389/fimmu.2020.583709
220. Tsuji A, Yoshikawa S, Morikawa S, Ikeda Y, Taniguchi K, Sawamura H, et al. Potential tactics with vitamin D and certain phytochemicals for enhancing the effectiveness of immune-checkpoint blockade therapies. Explor Target Antitumor Ther (2023) 4:460–73. doi: 10.37349/etat.2023.00145
221. Park HJ, Park JS, Jeong YH, Son J, Ban YH, Lee BH, et al. PD-1 upregulated on regulatory T cells during chronic virus infection enhances the suppression of CD8+ T cell immune response via the interaction with PD-L1 expressed on CD8+ T cells. J Immunol (2015) 194:5801–11. doi: 10.4049/jimmunol.1401936
222. Vivarelli S, Falzone L, Torino F, Scandurra G, Russo G, Bordonaro R, et al. Immune-checkpoint inhibitors from cancer to COVID−19: A promising avenue for the treatment of patients with COVID−19 (Review). Int J Oncol (2021) 58:145–57. doi: 10.3892/ijo.2020.5159
223. Kumar V, Chaudhary N, Garg M, Floudas CS, Soni P, Chandra AB. Current diagnosis and management of immune related adverse events (irAEs) induced by immune checkpoint inhibitor therapy. Front Pharmacol (2017) 8:49. doi: 10.3389/fphar.2017.00049
224. Wang X, Wu S, Chen Y, Shao E, Zhuang T, Lu L, et al. Fatal adverse events associated with programmed cell death ligand 1 inhibitors: A systematic review and meta-analysis. Front Pharmacol (2020) 11:5. doi: 10.3389/fphar.2020.00005
225. Wright JJ, Powers AC, Johnson DB. Endocrine toxicities of immune checkpoint inhibitors. Nat Rev Endocrinol (2021) 17:389–99. doi: 10.1038/s41574-021-00484-3
226. Liu LL, Skribek M, Harmenberg U, Gerling M. Systemic inflammatory syndromes as life-threatening side effects of immune checkpoint inhibitors: case report and systematic review of the literature. J Immunother Cancer (2023) 11:e005841. doi: 10.1136/jitc-2022-005841
227. Arana Y, Gálvez RI, Jacobs T. Role of the PD-1/PD-L1 pathway in experimental Trypanosoma cruzi infection and potential therapeutic options. Front Immunol (2022) 13:866120. doi: 10.3389/fimmu.2022.866120
228. Fuller MJ, Callendret B, Zhu B, Freeman GJ, Hasselschwert DL, Satterfield W, et al. Immunotherapy of chronic hepatitis C virus infection with antibodies against programmed cell death-1 (PD-1). Proc Natl Acad Sci U.S.A. (2013) 110:15001–6.
229. Gillis A, Ben Yaacov A, Agur Z. A new method for optimizing sepsis therapy by nivolumab and meropenem combination: importance of early intervention and CTL reinvigoration rate as a response marker. Front Immunol (2021) 12:616881. doi: 10.3389/fimmu.2021.616881
230. Curran CS, Busch LM, Li Y, Xizhong C, Sun J, Eichacker PQ, et al. Anti-PD-L1 therapy does not improve survival in a murine model of lethal staphylococcus aureus pneumonia. J Infect Dis (2021) 224:2073–84. doi: 10.1093/infdis/jiab274
231. Shindo Y, Mcdonough JS, Chang KC, Ramachandra M, Sasikumar PG, Hotchkiss RS. Anti-PD-L1 peptide improves survival in sepsis. J Surg Res (2017) 208:33–9. doi: 10.1016/j.jss.2016.08.099
232. Phares TW, Kotraiah V, Chung CS, Unsinger J, Mazer M, Remy KE, et al. A peptide-based checkpoint immunomodulator alleviates immune dysfunction in murine polymicrobial sepsis. Shock (2021) 55:806–15. doi: 10.1097/SHK.0000000000001682
233. Pei F, Yao RQ, Ren C, Bahrami S, Billiar TR, Chaudry IH, et al. Expert consensus on the monitoring and treatment of sepsis-induced immunosuppression. Mil Med Res (2022) 9:74. doi: 10.1186/s40779-022-00430-y
234. Le S, Pellegrini E, Green-Saxena A, Summers C, Hoffman J, Calvert J, et al. Supervised machine learning for the early prediction of acute respiratory distress syndrome (ARDS). J Crit Care (2020) 60:96–102. doi: 10.1016/j.jcrc.2020.07.019
235. Mansur A, Hinz J, Hillebrecht B, Bergmann I, Popov AF, Ghadimi M, et al. Ninety-day survival rate of patients with sepsis relates to programmed cell death 1 genetic polymorphism rs11568821. J Investig Med (2014) 62:638–43. doi: 10.2310/JIM.0000000000000059
236. Liu M, Zhang X, Chen H, Wang G, Zhang J, Dong P, et al. Serum sPD-L1, upregulated in sepsis, may reflect disease severity and clinical outcomes in septic patients. Scand J Immunol (2017) 85:66–72. doi: 10.1111/sji.12509
237. Jiang W, Li X, Ding H, Wang K, Liu X, Wang Q, et al. PD-1 in Tregs predicts the survival in sepsis patients using sepsis-3 criteria: A prospective, two-stage study. Int Immunopharmacol (2020) 89:107175. doi: 10.1016/j.intimp.2020.107175
238. Zhong W, Li J, Li D, Li X, Liu M, Zhang T, et al. ELEVATED PD-1/CD28 RATIO RATHER THAN PD-1 EXPRESSION IN CD8+ T CELLS PREDICTS NOSOCOMIAL INFECTION IN SEPSIS PATIENTS: A PROSPECTIVE, OBSERVATIONAL COHORT STUDY. Shock (2022) 58:111–8. doi: 10.1097/SHK.0000000000001967
239. Chen J, Wang H, Guo R, Li H, Cui N. Early expression of functional markers on CD4(+) T cells predicts outcomes in ICU patients with sepsis. Front Immunol (2022) 13:938538. doi: 10.3389/fimmu.2022.938538
240. Liu Q, Xue M, Song Q, Xie J, Yang Y, Liu S. Expression of PD-1 on memory T lymphocytes predicts 28-day mortality of patients with sepsis: A prospective observational study. J Inflammation Res (2022) 15:5043–52. doi: 10.2147/JIR.S376897
241. Wang L, Zhang Z, Chen X, Yu F, Huang H, Shen X, et al. Relationship between the expression of PD-1 and CTLA-4 on T lymphocytes and the severity and prognosis of sepsis. Int J Gen Med (2023) 16:1513–25. doi: 10.2147/IJGM.S402586
242. Jiang W, Li X, Wen M, Liu X, Wang K, Wang Q, et al. Increased percentage of PD-L1(+) natural killer cells predicts poor prognosis in sepsis patients: a prospective observational cohort study. Crit Care (2020) 24:617. doi: 10.1186/s13054-020-03329-z
243. Shao R, Fang Y, Yu H, Zhao L, Jiang Z, Li CS. Monocyte programmed death ligand-1 expression after 3-4 days of sepsis is associated with risk stratification and mortality in septic patients: a prospective cohort study. Crit Care (2016) 20:124. doi: 10.1186/s13054-016-1301-x
244. Tai H, Xing H, Xiang D, Zhu Z, Mei H, Sun W, et al. Monocyte programmed death ligand-1, A predicator for 28-day mortality in septic patients. Am J Med Sci (2018) 355:362–7. doi: 10.1016/j.amjms.2017.12.008
245. Li JB, Xie MR, Duan ML, Yu YN, Hang CC, Tang ZR, et al. Over-expression of programmed death-ligand 1 and programmed death-1 on antigen-presenting cells as a predictor of organ dysfunction and mortality during early sepsis: a prospective cohort study. World J Emerg Med (2023) 14:179–85. doi: 10.5847/wjem.j.1920-8642.2023.041
246. Monneret G, Gossez M, Venet F. Sepsis in PD-1 light. Crit Care (2016) 20:186. doi: 10.1186/s13054-016-1370-x
247. Jubel JM, Barbati ZR, Burger C, Wirtz DC, Schildberg FA. The role of PD-1 in acute and chronic infection. Front Immunol (2020) 11:487. doi: 10.3389/fimmu.2020.00487
248. Wilson JK, Zhao Y, Singer M, Spencer J, Shankar-Hari M. Lymphocyte subset expression and serum concentrations of PD-1/PD-L1 in sepsis - pilot study. Crit Care (2018) 22:95. doi: 10.1186/s13054-018-2020-2
249. Margaroli C, Horati H, Garratt LW, Giacalone VD, Schofield C, Dittrich AS, et al. Macrophage PD-1 associates with neutrophilia and reduced bacterial killing in early cystic fibrosis airway disease. J Cyst Fibros (2022) 21:967–76. doi: 10.1016/j.jcf.2022.06.001
250. Sari MI, Ilyas S. The expression levels and concentrations of PD-1 and PD-L1 proteins in septic patients: A systematic review. Diagnostics (Basel) (2022) 12:2004. doi: 10.3390/diagnostics12082004
251. Tang L, Bai J, Chung CS, Lomas-Neira J, Chen Y, Huang X, et al. Active players in resolution of shock/sepsis induced indirect lung injury: immunomodulatory effects of Tregs and PD-1. J Leukoc Biol (2014) 96:809–20. doi: 10.1189/jlb.4MA1213-647RR
252. Shankar-Hari M, Datta D, Wilson J, Assi V, Stephen J, Weir CJ, et al. Early PREdiction of sepsis using leukocyte surface biomarkers: the ExPRES-sepsis cohort study. Intensive Care Med (2018) 44:1836–48. doi: 10.1007/s00134-018-5389-0
253. Akhmaltdinova LL, Zhumadilova ZA, Kolesnichenko SI, Lavrinenko AV, Kadyrova IA, Avdienko OV, et al. The presence of PDL-1 on CD8+ Lymphocytes is linked to survival in neonatal sepsis. Children (Basel) (2022) 9:1171. doi: 10.3390/children9081171
254. Yadav P, Trehanpati N, Maiwall R, Sehgal R, Singh R, Islam M, et al. Soluble factors and suppressive monocytes can predict early development of sepsis in acute-on-chronic liver failure. Hepatol Commun (2022) 6:2105–20. doi: 10.1002/hep4.1949
255. Yan L, Chen Y, Han Y, Tong C. Role of CD8(+) T cell exhaustion in the progression and prognosis of acute respiratory distress syndrome induced by sepsis: a prospective observational study. BMC Emerg Med (2022) 22:182. doi: 10.1186/s12873-022-00733-2
256. Liu Q, An L, Qi Z, Zhao Y, Li C. Increased expression of programmed cell death-1 in regulatory T cells of patients with severe sepsis and septic shock: an observational clinical study. Scand J Immunol (2017) 86:408–17. doi: 10.1111/sji.12612
Keywords: sepsis, PD-1/PD-L1, immune homeostasis, immunoparalysis, organ damage, treatment
Citation: Chen Y, Guo D-z, Zhu C-l, Ren S-c, Sun C-y, Wang Y and Wang J-f (2023) The implication of targeting PD-1:PD-L1 pathway in treating sepsis through immunostimulatory and anti-inflammatory pathways. Front. Immunol. 14:1323797. doi: 10.3389/fimmu.2023.1323797
Received: 19 October 2023; Accepted: 29 November 2023;
Published: 13 December 2023.
Edited by:
J. Louise Lines, Dartmouth College, United StatesReviewed by:
Liyuan Zhang, Second Affiliated Hospital of Soochow University, ChinaCopyright © 2023 Chen, Guo, Zhu, Ren, Sun, Wang and Wang. This is an open-access article distributed under the terms of the Creative Commons Attribution License (CC BY). The use, distribution or reproduction in other forums is permitted, provided the original author(s) and the copyright owner(s) are credited and that the original publication in this journal is cited, in accordance with accepted academic practice. No use, distribution or reproduction is permitted which does not comply with these terms.
*Correspondence: Yi Wang, MTgwMTkwNTQ4ODBAMTYzLmNvbQ==; Jia-feng Wang, amZ3YW5nQHNtbXUuZWR1LmNu
†These authors have contributed equally to this work and share first authorship
Disclaimer: All claims expressed in this article are solely those of the authors and do not necessarily represent those of their affiliated organizations, or those of the publisher, the editors and the reviewers. Any product that may be evaluated in this article or claim that may be made by its manufacturer is not guaranteed or endorsed by the publisher.
Research integrity at Frontiers
Learn more about the work of our research integrity team to safeguard the quality of each article we publish.