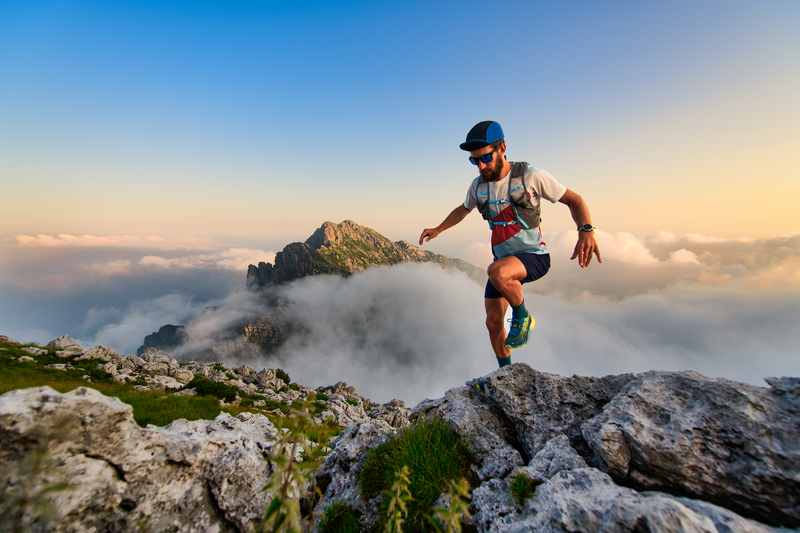
94% of researchers rate our articles as excellent or good
Learn more about the work of our research integrity team to safeguard the quality of each article we publish.
Find out more
REVIEW article
Front. Immunol. , 08 January 2024
Sec. Immunological Tolerance and Regulation
Volume 14 - 2023 | https://doi.org/10.3389/fimmu.2023.1323676
This article is part of the Research Topic Community Series in Harnessing the Participation of Dendritic Cells in Immunity and Tolerance: Volume II View all 6 articles
Radiation-induced lung injury is a common complication associated with radiotherapy. It is characterized by early-stage radiation pneumonia and subsequent radiation pulmonary fibrosis. However, there is currently a lack of effective therapeutic strategies for radiation-induced lung injury. Recent studies have shown that tolerogenic dendritic cells interact with regulatory T cells and/or regulatory B cells to stimulate the production of immunosuppressive molecules, control inflammation, and prevent overimmunity. This highlights a potential new therapeutic activity of tolerogenic dendritic cells in managing radiation-induced lung injury. In this review, we aim to provide a comprehensive overview of tolerogenic dendritic cells in the context of radiation-induced lung injury, which will be valuable for researchers in this field.
Radiation-induced lung injury (RILI) refers to any damage to the lungs caused by exposure to ionizing radiation (1, 2). The recent Fukushima nuclear sewage incident has raised significant concerns regarding its impact on human health (3, 4). Since the lung is a radiation-sensitive organ, nuclear pollution can potentially lead to radioactive damage in the lungs (5, 6). Clinical manifestations of RILI are typically divided into two stages: pneumonia in the early stages and pulmonary fibrosis in the late stages (7–9). However, the pathological process of RILI is complex, and the molecular mechanism underlying its development remains poorly understood. Currently, there are no specific therapeutic drugs available for this condition.
Recently, numerous studies have demonstrated the potential of dendritic cell (DC)-based therapy as a promising treatment for diseases associated with lung injury (10–13). DCs, which are strategically located between the airway epithelial cells and the matrix, are known for their strong antigen-presenting abilities and immune monitoring of the lungs (14, 15). DCs are a heterogeneous group of myeloid-derived cells that can be found in almost all tissues (16, 17). When there is an invasion of pathogens or tissue inflammation, DCs quickly migrate to the damaged tissue site, process antigens, and present them to T lymphocytes, thereby bridging the innate immune response and adaptive immunity (18). Indeed, DCs have diverse functions and plasticities within the immune system. They have been extensively studied in various fields such as autoimmune diseases, inflammation, cancer, and fibrosis in different organs including the lungs, liver, kidneys, and heart (19–21).
Recent studies have also highlighted the role of tolerogenic DCs (tolDCs) in negatively regulating the immune response and maintaining immune tolerance. They promote the elimination of autoreactive immature T-cells in the thymus and induce immune tolerance in the periphery by interacting with T-cells, leading to T-cell deletion, T-cell incompetence, and differentiation of regulatory T-cells (Treg) subgroups (22–24). Based on the available evidence, there has been an increasing interest in exploring the therapeutic potential of tolDCs for the management of radiation-related diseases. Here, we review the latest developments in the potential application and treatment mechanisms of tolDCs in RILI. Specifically, we focused on the immunosuppressive effects of tolDCs, which may represent an important therapeutic advantage in the treatment of RILI and pave the way for clinical trials.
RILI is a complex pathological process that involves multiple cells. Following radiation exposure, acute exudation occurs in the lungs, which is characterized by inflammatory cell infiltration, varying degrees of transparency in small arteries, fibrous thickening, alveolar edema filled with exudate, and collagen fibrous hyperplasia. It is important to note that this process is irreversible (25, 26). However, the precise molecular mechanism of RILI remains unclear. So far, the existing research, both domestic and foreign, has mainly focused on a few key areas. These areas include the generation of reactive oxygen species (ROS), direct damage to target cells, and activation of the immune system, as shown in Figure 1.
Figure 1 Pulmonary tissue undergoes changes following irradiation. When ionizing radiation is applied to lung tissue, it ionizes water molecules and generates a significant amount of ROS. These persistent ROS can exacerbate damage to target cells such as alveolar epithelial cells and vascular endothelial cells. The damaged target cells then release inflammatory cytokines and chemokines. Additionally, imDCs located between the pulmonary epithelium and interstitium promptly migrate to the site of injury. They not only enhance T-cell activity, but also activate and mobilize other immune cells. As a result, the immune system becomes abnormally activated. Inflammatory cells and fibrosis-associated cells are activated and infiltrate the interstitium. Eventually, the epithelium undergoes the EMT process, and fibroblasts differentiate into myofibroblasts, leading to excessive collagen deposition. Ultimately, this excessive collagen deposition contributes to the development of pulmonary fibrosis. IR, ionizing radiation;ROS, reactive oxygen species; imDCs, immature dendritic cells; AT1 cells, alveolar type 1 cells; AT2 cells, alveolar type 2 cells; EMT, epithelial-mesenchymal transition.
When ionizing radiation is applied to lung tissue, it ionizes water molecules and generates a significant amount of ROS (27–29). These ROS can damage the DNA, proteins, and lipid membranes of target cells. If the damage is not promptly repaired, it can lead to oxidative stress damage. Research has shown that oxidative stress damage can still be detected weeks or even months after the completion of radiotherapy (30). Moreover, these persistent ROS can worsen the damage to target cells and perpetuate lung lesions (31–33).
According to extensive research, vascular endothelial cells and alveolar epithelial cells have been identified as the main target cells for RILI (34, 35). In the early stages after radiation exposure, vascular endothelial cells exhibit increased vascular permeability and inflammatory exudation (33). As the duration of irradiation and the dose of radiation increase, vascular endothelial cells undergo rupture and detachment, leading to platelet attachment and resulting in capillary embolism and fibrosis (36–38). Type I alveolar epithelial cells, which lack the ability to proliferate, undergo direct necrosis or apoptosis following irradiation. Damage to type II alveolar epithelial cells can trigger excessive proliferation of fibroblasts, leading to fibrosis (39–41). Moreover, the abnormal proliferation of type II alveolar epithelial cells reduces the secretion of alveolar surface-active substances, resulting in decreased alveolar surface tension and causing pulmonary tissue edema and atelectasis (42, 43).
Of note, damaged alveolar epithelial cells and vascular endothelial cells also secrete various cytokines, including tumor necrosis factors (TNF-α) involved in local injury and inflammatory response, transforming growth factor β-1 (TGF-β1) that promotes tissue repair and organ fibrosis, platelet-derived growth factor (PDGF), interleukins (IL-1β, IL-6, IL-8, IL-10), and monocyte chemotactic peptides (32, 44, 45). Among them, some studies have shown that TGF-β1-based CRISPR/Cas9 gene therapy improves lung tissue pathological damage, reduces the secretion and expression of inflammatory factors, and ultimately inhibits the progression of radiation fibrosis (46).
More importantly, the immune system, which serves as the body’s primary defense against external damage, has been extensively studied and found to play a crucial role in the onset and progression of RILI (47). Among the various components of the immune system, DCs are the most potential professional antigen presenting cells and play a dominate role in immune system as commanders during RILI. In the early stages of RILI, immature DCs(imDCs) situated between the pulmonary epithelium and interstitium promptly detect endogenous damage-associated molecular patterns (DAMPs) released from damaged or dying cells through their surface pattern recognition receptors (PRRs) (48–53). They swiftly migrate to the site of injury and initiate an initial immune response by efficiently capturing, processing, and presenting antigens to T-cells in nearby lymph nodes (54). T-cells play a crucial role in the immune system as communication experts. They activate B-cells, which release a large number of antibodies and contribute to the ultimate defense (55–57). Furthermore, DCs secrete cytokines and growth factors to enhance and regulate various immune responses, including those of macrophages, mast cells, NK cells, and cytokine-induced killer (CIK) cells (58–60). Simultaneously, these activated immune cells release high levels of pro-inflammatory cytokines, including IL-1β and TNF-α, as well as chemokines like chemokine C-C-motif ligand 1 (CCL1). These cytokines further stimulate fibroblasts to differentiate into myofibroblasts, leading to excessive collagen deposition (61–63). Ultimately, this excessive collagen deposition contributes to the development of radiation-induced pulmonary fibrosis (64–66).
However, several subsets of DCs have been discovered with the development of DC studies on lung tissue. It is crucial to note that these different subgroups of pulmonary DCs exhibit a division of labor. Therefore, it is necessary to gain a deeper understanding of the biological and functional characteristics of pulmonary DCs to identify a specific subset of DCs that plays a pivotal regulatory role in RILI.
DCs are the primary antigen-presenting cells in the lung tissue and are crucial for the immune response to RILI (67–69). Within the lung tissue, DCs are categorized into three main types: conventional DCs, plasmacytoid DCs, and monocyte-derived DCs, as shown in Table 1.
Notably, it has been reported that many conventional DCs(cDCs) are located in the mucous membranes of the airway ducts (107–109). These cells extend pseudopods between epithelial cells to capture antigens present within the airway cavity (102, 110, 111). Based on their functional differences, cDCs are further categorized into two subsets: cDC1 and cDC2. Among them, mice and humans cDC1 have been observed to exhibit a high degree of cross-presentation ability, effectively stimulating CD8+ T-cells in response to extracellular antigens like those found in bacteria and viruses (70, 71). These cells are known to secrete IL-12, type I, and III interferons (IFNs), and are believed to facilitate Th1-assisted T-cells and natural killer responses (72, 73). Some well-known markers for cDC1 include CD8a, CLEC9A,CD103, CD11c, CD141, and XCR1. Additionally, cDC2, which is the primary subset of DC found in the blood, tissues, and lymphatic organs, has been demonstrated to stimulate Th2 and Th17 auxiliary T-cell responses (74, 75). Moreover, cDC2 has been found to have several regulatory effects, such as the induction of Tregs in lung tissue and the maintenance of tolerance in the same tissue (76). Common markers used to identify cDC2 include CD1c, CD207, CD11b, NOTCH2, and SIRPα. However, recent evidence has accumulated, indicating that cDC2 is not a homogeneous population, but rather consists of two distinct subsets: cDC2A and cDC2B (77). These subsets are differentiated based on the expression levels of two key transcription regulators, T-bet and ROR-ƴt (77). The cDC2A subset demonstrates an anti-inflammatory function (78), while the cDC2B subset exhibits pro-inflammatory properties (78–80). Consequently, the cDC2 subsets have significant implications in maintaining lung tissue homeostasis and regulating immune responses. Of note, AXL+Siglec6+ DCs (AS DC), the precursor to mature cDC2, can potentially transition to this subset through the influence of two key regulators, AXL and Siglec6 (CD327) (81). It has been reported that this subset strongly stimulates T-cell response in lung tissue (82).
Plasmacytoid DCs (pDCs) undergo direct maturation from common DC progenitors (CDPs) in the bone marrow and subsequently migrate to the blood and surrounding lymphoid tissue (83). These round plasmacytoid cells are present in lower numbers compared to regular cells. pDCs are known for their ability to rapidly produce cytokines such as type I and type III IFNs (84–88), which makes them a crucial subset for managing RILI. pDCs also express major histocompatibility complex II (MHC II) and may function as antigen-presenting cells (89–92). There are different subsets of pDCs, including one population characterized by high expression of CD2, which has been observed to specifically induce CD4+ T-cell proliferation (93–95). Additionally, upon stimulation, pDCs have been reported to activate CD8+ T-cells (96–98). Currently, the primary markers used to identify pDCs include CLEC4C, LILRB4, NRP1, CCR7, B220 (in mice), and SiglecH (in mice).
Monocyte-derived DCs (moDCs) are a DC subset that has been gaining attention due to their strong impact on adaptive immune function and their rapid accumulation in response to an inflammatory stimulus (99–101). Initial studies have primarily focused on the infection aspect of moDCs. However, their significance in RILI is now being increasingly acknowledged. Indeed, moDCs have been observed in human lung mucosal tissue as well as in inflammatory settings (102). In such settings, they are often referred to as ‘pneumonic DCs’ and are generated from monocytes that are recruited from the blood to the lung tissue during inflammation. Although this particular subpopulation exhibits dendrite morphology, it also possesses the genetic signature of moDCs in vitro. Hence, it is generally accepted that moDCs are produced as part of the inflammatory response, and this subgroup plays a role in promoting CD4+ T-cells to generate a Th17 immune response (105, 106). Currently, reported moDC markers include CD14, CD206, CD209, SIRPα, CD11b, and CD1a.
Taken together, DCs are heterogeneous populations. Therefore, future research should focus on linking the phenotypic features of different DC subsets to the known functions of their biology, especially when considering clinical applications based on DCs.
DCs play a crucial role in maintaining a balance between immunity and tolerance (112–114). They act as messengers in the occurrence and development of respiratory diseases (115–117). Additionally, they serve as sensors and tolerant gatekeepers for airway mucosa pathogens (118–120). This multifunctional cell type combines innate signaling mechanisms such as pattern recognition and early inflammatory mediators with adaptive immune responses, including T-cell priming and Treg induction (121, 122). Ionizing radiation disrupts the balance between effector immunity and regulatory immunity in lung tissue, leading to alterations in the behavior of DCs in response to these changes (Figure 2).
Figure 2 DC phenotypes and functions undergo alterations in response to the microenvironment of lung tissue induced by ionizing radiation. The intrinsic immune cells are stimulated by ionizing radiation to release various soluble factors including TNF-α, IFN-ƴ, TSLP, IL-15, IL-10, CCL19, and CXCL4. These factors subsequently promote the differentiation of imDCs and their precursors into infDCs and tolDCs. This cascade of events further impacts other immune cells, ultimately disrupting the delicate balance between immunity and tolerance. DC, dendritic cell; IR, ionizing radiation; TNF-α, tumor necrosis factors; IFN-ƴ, interferons gama; TSLP, thymic stromal lymphopoietin(TSLP); IL-10, interleukin 10; CCL19, chemokine C-C-motif ligand 19; CXCL4, chemokine CXC-motif ligand 4; imDCs, immature DCs; infDCs, inflammatory DCs; tolDCs, tolerogenic DCs.
Ionizing radiation induces a cascade of cellular and molecular changes in lung tissue, leading to the release of numerous cytokines, chemokines, and growth factors (123–125). These substances attract immune cells, resulting in the formation of a microenvironment within the lung tissue. Within this microenvironment, immature DCs (imDCs) and their precursors can be activated by various factors, including those from intrinsic immune cells, to differentiate into mature cells with distinct phenotypes and functions (50, 126). Therefore, TNF-α, IFN-γ, thymic stromal lymphopoietin(TSLP), IL-15, IL-10, chemokine C-C-motif ligand 19 (CCL19) and chemokine CXC-motif ligand 4(CXCL4) differentiated DCs into TNF-DCs, IFN-DCs, TSLP-DCs, IL-15-DCs, IL-10-DCs, CCL19-DCs or CXCL4-DCs, respectively. These distinct phenotypes and functions of DCs play a crucial role in shaping different types of T-cell immunity. For example, TSLP-DCs develop T-cells into inflammatory type 2 cells, secreting large amounts of TNF and type 2 cytokines (127). IL-10-DCs promote the development of IL-10-secreted Treg (128). IFN-γ-DCs promote effective T-cell response by upregulating IL-12 secretion (129). CXCL4-DCs enhance the proliferation of autologous CD4+ T-cells and CD8+ T-cells and the production of IFN-γ and IL-4 (130, 131).
In light of the available evidence, we suggest that imDCs and their precursors possess remarkable functional plasticity, enabling the intrinsic immune system to modulate the specific immune system. Additionally, this process gives rise to two types of DCs: inflammatory DCs (infDCs) that initiate positive immune cell responses (132, 133), and tolDCs, which elicit negative regulatory immune responses and contribute to the maintenance of immune tolerance (134). As a result, the outcome of various inflammatory diseases is determined by the conflict between the exaggerated immune response activated by infDCs and the immune response negatively regulated by tolDCs. Collectively, conducting comprehensive research on the potential application mechanisms of tolDCs could offer a promising and innovative approach to treating RILI.
Accumulating clinical studies have demonstrated that breast cancer patients who receive unilateral chest wall radiotherapy exhibit a notable presence of activated lymphocytes in bilateral alveolar lavage (135). This finding suggests that the observed inflammatory change in RILI is not solely caused by tissue injury, but rather involves an exaggerated immune response of T and B-cells. Specifically, infDCs activate multiple immune cell inflammatory response processes in various diseases. Initially, the focus of understanding DCs in RILI was on this group. However, recent evidence indicates that tolDCs also play a crucial role in regulating inflammation and preventing excessive immune response-induced damage to lung tissue. Therefore, there is potential for utilizing tolDCs to address immune disorders in RILI through the following aspects (Figure 3):
Figure 3 Some mechanisms about tolDCs for RILI in current research. The main methods to apply tolDCs in RILI are shown in the picture. TolDCs can regulate multiple signaling molecules, which protect pulmonary cells. TolDCs inhibit leukocyte infiltration and regulate leukocyte function. TRAIL, TNF-related apoptosis-inducing ligand; FasL, fas ligand; TGF-β, transforming growth factor beta; Treg, regulatory T-cells; Breg, regulatory B cell; Teff, effector cells;PD-L1, programmed cell death 1 ligand 1; PD-1, programmed cell death protein 1;CTLA4, cytotoxic T-lymphocyte-associated protein 4.
TolDCs play a significant role in suppressing immune responses through various mechanisms, including the production of cytokines and cell-cell contact. Several studies have demonstrated that certain anti-inflammatory cytokines, including TGF-β and IL-27, secreted by various tolDCs, play a role in promoting the production of Tregs and stimulating the secretion of IL-10 (136). Besides, tolDC also promotes the Bregs to produce IL-10, TGF-β, and to some extent, IL-35, and further inhibit antigen-specific CD8+ T-cells in inflammation and autoimmune diseases (137–139). On the other hand, growing evidence has demonstrated that tolDCs exhibit limited capacity for cross-expression and low co-stimulation molecular phenotypes (140–142). In the absence of these co-stimulation signals, T-cells are unable to produce IL-2 and undergo further proliferation when they interact with tolDCs through the recognition of antigens presented by MHC via T-cell receptors (TCR) (143). As a result, the overreaction of T-cells in RILI is eventually blocked.
Of note, tolDCs express programmed cell death 1 ligand 1(PD-L1) and PD-L2, which bind with programmed cell death protein 1 (PD-1) in T-cells (144, 145). This interaction leads to the recruitment of SH2-containing inositol phosphatase 1(SHP-1) and SHP-2, instead of activating the TCR and CD28 signaling pathway. As a result, tolDCs induce clonal incompetence and promote the differentiation of Tregs, ultimately leading to tolerance in RILI. Furthermore, the study revealed that the interaction between TNF-related apoptosis-inducing ligand (TRAIL) in human DCs and T-cell death receptors can induce T-cell apoptosis by activating caspase. Likewise, the fas ligand (FasL) present on the surface of tolDCs binds to the upregulated Fas during T-cell activation, thereby promoting T-cell apoptosis (146).
In general, these findings demonstrate that tolDCs are capable of interacting with Tregs and Bregs, thus forming a feedback loop of tolerance. This means that once a regulating group initiates a tolerance signal, it can be sustained and enhanced through the aggregation of other cell groups. Therefore, tolDCs can be considered as a potential treatment option for radioactive lung injury, particularly during the initial stages of pneumonia. However, limited information is available regarding the origin of tolDCs.
In 1998, Steinman made a discovery that highlighted the dual role of DCs. While primarily involved in T-cell immune responses, DCs also have the paradoxical effect of inducing tolerance to autoantigens (147, 148). Subsequent research has revealed that a specific subset of DCs, known as tolDCs, possess functions that can inhibit T-cell profiles or induce Treg (149). According to domestic and foreign reports, the acquisition of tolDCs primarily involves the following aspects:
First, the stromal environment, particularly in the bone marrow, spleen, lung, kidney, and liver, contributes to the induction of tolDCs. The study revealed that co-culturing DCs with spleen stromal cells resulted in a negative immune function (150). Furthermore, co-culturing mesenchymal stem cells (MSCs) with imDCs or mature DCs (mDCs) resulted in the generation of a distinct subset of tolDCs (150). These tolDCs exhibited reduced expression of costimulatory molecules, decreased levels of IL-12, and increased levels of TGF-β and IL-10. Importantly, these changes were not reversed when the tolDCs were stimulated with lipopolysaccharides (LPS), indicating sustained immune toleration (151, 152).
Second, some drugs or chemical agents have been found to induce tolDCs. These drugs including immunosuppressants like sirolimus, tacrolimus, cyclosporin, and motidimethylphenol, have been shown to affect the expression of molecules such as CD40, CD80, and CD86 on the surface of DCs, with sirolimus having the most significant impact (144, 153, 154). In addition, numerous studies have shown that the immunomodulatory effect of vitamin D3 is achieved by binding to the vitamin D receptor expressed by DCs, whose immunosuppressive effect manifested as down-regulation of CD80, CD86, CD40, lower IL-12, and higher IL-10, thus inhibiting differentiation and maturation of DCs (155–158).
Third, genetic engineering technology was employed to modify the genes of DCs, preventing their transformation into mDCs and inducing them to secrete inhibitory cytokines, thereby functioning as tolDCs. The previous study has found that in vitro, adenovirus vectors are used to modify imDCs, which expressed cytotoxic T lymphocyte-associated antigen-4 (CTLA-4) and low levels of CD86, eventually differentiated into tolDCs (140, 159–163).
Fourth, it has been observed that anti-inflammatory cytokines or chemokines have the ability to stimulate imDCs or DC precursor cells to differentiate into tolDCs. The study has shown that the induction of anti-inflammatory cytokines, such as IL-37, TGF-β, prostaglandin E2 (PGE2), and TNF-α, can stimulate the enzyme activity of indoleamine 2,3-dioxygenase (IDO) in DCs, resulting in the generation of tolDCs (164–168). In addition, recent studies have shown that anti-inflammatory chemokines can transform into tolDCs by binding to their receptors. Azzaoui et al. (169) discovered that the chemokine CCL18, through an IL-10-mediated mechanism, is dependent on the production of IDO to differentiate DCs into tolerogenic cells capable of activating Tregs.
Taken together, with the recognition of tolDCs, there is an increasing focus on finding a quick and efficient acquisition method. This method will play a crucial role as a therapeutic tool in the future. The successful application of tolDCs in the clinical treatment of RILI will depend on the rational use and selection of appropriate access.
RILI is a serious and complex lung disease characterized by the infiltration of cytokines secreted by various inflammatory cells. However, tolDCs have the potential to regulate immune cell response and promote the production of anti-inflammatory factors, thereby maintaining immune balance. While tolDC-based therapy has shown promise in treating RILI in recent studies, further research is required to determine its safety, optimal dosage, and treatment timing. At present, genetically modified organism (GMO) tolDCs have garnered significant interest from experts in the field and could potentially serve as the next advancement in the development of novel therapeutic strategies. In essence, tolDC therapy holds immense promise for the treatment of RILI.
BL: Writing – original draft. YW: Writing – review & editing. GH: Writing – review & editing. MZ: Writing – review & editing.
The author(s) declare financial support was received for the research, authorship, and/or publication of this article. This research was supported by grants from the National Natural Science Foundation of China (81673095).
The authors declare that the research was conducted in the absence of any commercial or financial relationships that could be construed as a potential conflict of interest.
All claims expressed in this article are solely those of the authors and do not necessarily represent those of their affiliated organizations, or those of the publisher, the editors and the reviewers. Any product that may be evaluated in this article, or claim that may be made by its manufacturer, is not guaranteed or endorsed by the publisher.
1. Giridhar P, Mallick S, Rath GK, Julka PK. Radiation induced lung injury: prediction, assessment and management. Asian Pac J Cancer Prev (2015) 16:2613–17. doi: 10.7314/apjcp.2015.16.7.2613
2. Roy S, Salerno KE, Citrin DE. Biology of radiation-induced lung injury. Semin Radiat Oncol (2021) 31:155–61. doi: 10.1016/j.semradonc.2020.11.006
3. Zhang Q, Yang F, Chen Y. The predicament and outlet of environmental impact assessment mechanism from the perspective of risk society: taking Japan’s accident-type nuclear sewage disposal as a cut-in. Int J Env Res Pub He (2023) 20:3929. doi: 10.3390/ijerph20053929
4. Yui K, Kuramochi H, Osako M. Understanding the behavior of radioactive cesium during the incineration of contaminated municipal solid waste and sewage sludge by thermodynamic equilibrium calculation. ACS Omega (2018) 3:15086–99. doi: 10.1021/acsomega.8b01113
5. Kamei-Ishikawa N, Ito A, Tagami K, Umita T. Fate of radiocesium in sewage treatment process released by the nuclear accident at Fukushima. Chemosphere (2013) 93:689–94. doi: 10.1016/j.chemosphere.2013.06.012
6. Chang YC, Zhao Y. The Fukushima Nuclear Power Station incident and marine pollution. Mar pollut Bull (2012) 64:897–901. doi: 10.1016/j.marpolbul.2012.01.038
7. Hu Z, Zheng J, Xiong Y, Tan K, Zhang X, Yu Y, et al. Radiation induced lung injury (RILI) after postoperative intensity modulated proton therapy (IMPT) in a patient with stage III locally advanced lung adenocarcinoma: a case report. Transl Cancer Res (2022) 11:3400–08. doi: 10.21037/tcr-22-256
8. Seto Y, Kaneko Y, Mouri T, Shimizu D, Morimoto Y, Tokuda S, et al. Changes in serum transforming growth factor-beta concentration as a predictive factor for radiation-induced lung injury onset in radiotherapy-treated patients with locally advanced lung cancer. Transl Lung Cancer Res (2022) 11:1823–34. doi: 10.21037/tlcr-22-229
9. Niu S, Zhang Y. Applications and therapeutic mechanisms of action of mesenchymal stem cells in radiation-induced lung injury. Stem Cell Res Ther (2021) 12:212. doi: 10.1186/s13287-021-02279-9
10. Mbongue J, Nicholas D, Firek A, Langridge W. The role of dendritic cells in tissue-specific autoimmunity. J Immunol Res (2014) 2014:857143. doi: 10.1155/2014/857143
11. Tabarkiewicz J. Dendritic cells: active and passive players in therapy of human diseases. Immunotherapy-Uk (2012) 4:975–78. doi: 10.2217/imt.12.97
12. Ilett EJ, Prestwich RJ, Melcher AA. The evolving role of dendritic cells in cancer therapy. Expert Opin Biol Ther (2010) 10:369–79. doi: 10.1517/14712590903559830
13. Banchereau J, Schuler-Thurner B, Palucka AK, Schuler G. Dendritic cells as vectors for therapy. Cell (2001) 106:271–74. doi: 10.1016/s0092-8674(01)00448-2
14. Upham JW, Stick SM. Interactions between airway epithelial cells and dendritic cells: implications for the regulation of airway inflammation. Curr Drug Targets (2006) 7:541–45. doi: 10.2174/138945006776818647
15. Makala LH, Nagasawa H. Dendritic cells: a specialized complex system of antigen presenting cells. J Vet Med Sci (2002) 64:181–93. doi: 10.1292/jvms.64.181
16. Nakamizo S, Dutertre CA, Khalilnezhad A, Zhang XM, Lim S, Lum J, et al. Single-cell analysis of human skin identifies CD14+ type 3 dendritic cells co-producing IL1B and IL23A in psoriasis. J Exp Med (2021) 218:e20202345. doi: 10.1084/jem.20202345
17. Patente TA, Pinho MP, Oliveira AA, Evangelista G, Bergami-Santos PC, Barbuto J. Human dendritic cells: their heterogeneity and clinical application potential in cancer immunotherapy. Front Immunol (2018) 9:3176. doi: 10.3389/fimmu.2018.03176
18. Steinman RM. Linking innate to adaptive immunity through dendritic cells. Novartis Found Symp (2006) 279:101–09. doi: 10.1002/9780470035399.ch9
19. Kvedaraite E, Ginhoux F. Human dendritic cells in cancer. Sci Immunol (2022) 7:m9409. doi: 10.1126/sciimmunol.abm9409
20. Liu J, Zhang X, Cao X. Dendritic cells in systemic lupus erythematosus: From pathogenesis to therapeutic applications. J Autoimmun (2022) 132:102856. doi: 10.1016/j.jaut.2022.102856
21. Brandum EP, Jørgensen AS, Rosenkilde MM, Hjortø GM. Dendritic cells and CCR7 expression: an important factor for autoimmune diseases, chronic inflammation, and cancer. Int J Mol Sci (2021) 22:8340. doi: 10.3390/ijms22158340
22. Passeri L, Marta F, Bassi V, Gregori S. Tolerogenic dendritic cell-based approaches in autoimmunity. Int J Mol Sci (2021) 22:8415. doi: 10.3390/ijms22168415
23. Moreau A, Alliot-Licht B, Cuturi MC, Blancho G. Tolerogenic dendritic cell therapy in organ transplantation. Transpl Int (2017) 30:754–64. doi: 10.1111/tri.12889
24. Torres-Aguilar H, Blank M, Jara LJ, Shoenfeld Y. Tolerogenic dendritic cells in autoimmune diseases: crucial players in induction and prevention of autoimmunity. Autoimmun Rev (2010) 10:8–17. doi: 10.1016/j.autrev.2010.07.015
25. Lu L, Sun C, Su Q, Wang Y, Li J, Guo Z, et al. Radiation-induced lung injury: latest molecular developments, therapeutic approaches, and clinical guidance. Clin Exp Med (2019) 19:417–26. doi: 10.1007/s10238-019-00571-w
26. Bledsoe TJ, Nath SK, Decker RH. Radiation pneumonitis. Clin Chest Med (2017) 38:201–08. doi: 10.1016/j.ccm.2016.12.004
27. Tan Y, Duan Y, Chi Q, Wang R, Yin Y, Cui D, et al. The role of reactive oxygen species in plant response to radiation. Int J Mol Sci (2023) 24:3346. doi: 10.3390/ijms24043346
28. Gómez-Alonso I, Baltierra-Uribe S, Sánchez-Torres L, Cancino-Diaz M, Cancino-Diaz J, Rodriguez-Martinez S, et al. Irradiation and parasitism affect the ability of larval hemocytes of Anastrepha obliqua for phagocytosis and the production of reactive oxygen species. Arch Insect Biochem Physiol (2022) 111:e21953. doi: 10.1002/arch.21953
29. Sies H, Jones DP. Reactive oxygen species (ROS) as pleiotropic physiological signalling agents. Nat Rev Mol Cell Biol (2020) 21:363–83. doi: 10.1038/s41580-020-0230-3
30. Hayashi T, Furukawa K, Morishita Y, Hayashi I, Kato N, Yoshida K, et al. Intracellular reactive oxygen species level in blood cells of atomic bomb survivors is increased due to aging and radiation exposure. Free Radic Biol Med (2021) 171:126–34. doi: 10.1016/j.freeradbiomed.2021.05.017
31. Shrishrimal S, Kosmacek EA, Oberley-Deegan RE. Reactive oxygen species drive epigenetic changes in radiation-induced fibrosis. Oxid Med Cell Longev (2019) 2019:4278658. doi: 10.1155/2019/4278658
32. Rao X, Zhou D, Deng H, Chen Y, Wang J, Zhou X, et al. Activation of NLRP3 inflammasome in lung epithelial cells triggers radiation-induced lung injury. Respir Res (2023) 24:25. doi: 10.1186/s12931-023-02331-7
33. Wijerathne H, Langston JC, Yang Q, Sun S, Miyamoto C, Kilpatrick LE, et al. Mechanisms of radiation-induced endothelium damage: Emerging models and technologies. Radiother Oncol (2021) 158:21–32. doi: 10.1016/j.radonc.2021.02.007
34. Hansel C, Jendrossek V, Klein D. Cellular senescence in the lung: the central role of senescent epithelial cells. Int J Mol Sci (2020) 21:3279. doi: 10.3390/ijms21093279
35. Singh S, Vaughan CA, Rabender C, Mikkelsen R, Deb S, Palit DS. DNA replication in progenitor cells and epithelial regeneration after lung injury requires the oncoprotein MDM2. JCI Insight (2019) 4:e128194. doi: 10.1172/jci.insight.128194
36. Ying H, Zhou C, Hang Q, Fang M. The preventive effect of endostar on radiation-induced pulmonary fibrosis. Curr Mol Med (2023) 21:3279. doi: 10.2174/1566524023666230406134640
37. Bouten RM, Dalgard CL, Soltis AR, Slaven JE, Day RM. Transcriptomic profiling and pathway analysis of cultured human lung microvascular endothelial cells following ionizing radiation exposure. Sci Rep (2021) 11:24214. doi: 10.1038/s41598-021-03636-7
38. Wiesemann A, Ketteler J, Slama A, Wirsdörfer F, Hager T, Röck K, et al. Inhibition of radiation-induced ccl2 signaling protects lungs from vascular dysfunction and endothelial cell loss. Antioxid Redox Signal (2019) 30:213–31. doi: 10.1089/ars.2017.7458
39. Xuan D, Du C, Zhao W, Zhou J, Dai S, Zhang T, et al. Downregulation of β-Catenin Contributes to type II Alveolar Epithelial Stem Cell Resistance to Epithelial-Mesenchymal Transition by Lowing Lin28/let-7 Ratios in Fibrosis-Resistant Mice after Thoracic Irradiation. Radiat Res (2023) 200:32–47. doi: 10.1667/RADE-22-00165.1
40. Huang JQ, Zhang H, Guo XW, Lu Y, Wang SN, Cheng B, et al. Mechanically activated calcium channel PIEZO1 modulates radiation-induced epithelial-mesenchymal transition by forming a positive feedback with TGF-β1. Front Mol Biosci (2021) 8:725275. doi: 10.3389/fmolb.2021.725275
41. Wang LK, Wu TJ, Hong JH, Chen FH, Yu J, Wang CC. Radiation induces pulmonary fibrosis by promoting the fibrogenic differentiation of alveolar stem cells. Stem Cells Int (2020) 2020:6312053. doi: 10.1155/2020/6312053
42. Ruaro B, Salton F, Braga L, Wade B, Confalonieri P, Volpe MC, et al. The history and mystery of alveolar epithelial type II cells: focus on their physiologic and pathologic role in lung. Int J Mol Sci (2021) 22:2566. doi: 10.3390/ijms22052566
43. Cooney AL, Wambach JA, Sinn PL, McCray PJ. Gene therapy potential for genetic disorders of surfactant dysfunction. Front Genome Ed (2021) 3:785829. doi: 10.3389/fgeed.2021.785829
44. Yan Y, Fu J, Kowalchuk RO, Wright CM, Zhang R, Li X, et al. Exploration of radiation-induced lung injury, from mechanism to treatment: a narrative review. Transl Lung Cancer Res (2022) 11:307–22. doi: 10.21037/tlcr-22-108
45. Lierova A, Jelicova M, Nemcova M, Proksova M, Pejchal J, Zarybnicka L, et al. Cytokines and radiation-induced pulmonary injuries. J Radiat Res (2018) 59:709–53. doi: 10.1093/jrr/rry067
46. Zhen S, Qiang R, Lu J, Tuo X, Yang X, Li X. TGF-β1-based CRISPR/cas9 gene therapy attenuates radiation-induced lung injury. Curr Gene Ther (2022) 22:59–65. doi: 10.2174/1566523220666201230100523
47. Tsoutsou PG. The interplay between radiation and the immune system in the field of post-radical pneumonitis and fibrosis and why it is important to understand it. Expert Opin Pharmacother (2014) 15:1781–83. doi: 10.1517/14656566.2014.938049
48. Yu H, Yang Y, Jiang T, Zhang X, Zhao Y, Pang G, et al. Effective Radiotherapy in Tumor Assisted by Ganoderma lucidum Polysaccharide-Conjugated Bismuth Sulfide Nanoparticles through Radiosensitization and Dendritic Cell Activation. ACS Appl Mater Interfaces (2019) 11:27536–47. doi: 10.1021/acsami.9b07804
49. Cerrato G, Liu P, Martins I, Kepp O, Kroemer G. Quantitative determination of phagocytosis by bone marrow-derived dendritic cells via imaging flow cytometry. Methods Enzymol (2020) 632:27–37. doi: 10.1016/bs.mie.2019.07.021
50. Balan S, Saxena M, Bhardwaj N. Dendritic cell subsets and locations. Int Rev Cell Mol Biol (2019) 348:1–68. doi: 10.1016/bs.ircmb.2019.07.004
51. Seya T, Shime H, Ebihara T, Oshiumi H, Matsumoto M. Pattern recognition receptors of innate immunity and their application to tumor immunotherapy. Cancer Sci (2010) 101:313–20. doi: 10.1111/j.1349-7006.2009.01442.x
52. van Vliet SJ, García-Vallejo JJ, van Kooyk Y. Dendritic cells and C-type lectin receptors: coupling innate to adaptive immune responses. Immunol Cell Biol (2008) 86:580–87. doi: 10.1038/icb.2008.55
53. Kanazawa N. Dendritic cell immunoreceptors: C-type lectin receptors for pattern-recognition and signaling on antigen-presenting cells. J Dermatol Sci (2007) 45:77–86. doi: 10.1016/j.jdermsci.2006.09.001
54. Chudnovskiy A, Pasqual G, Victora GD. Studying interactions between dendritic cells and T cells. vivo. Curr Opin Immunol (2019) 58:24–30. doi: 10.1016/j.coi.2019.02.002
55. Naderi W, Schreiner D, King CG. T-cell-B-cell collaboration in the lung. Curr Opin Immunol (2023) 81:102284. doi: 10.1016/j.coi.2023.102284
56. Poznyak AV, Bezsonov EE, Popkova TV, Starodubova AV, Orekhov AN. Immunity in atherosclerosis: focusing on T and B cells. Int J Mol Sci (2021) 22:8379. doi: 10.3390/ijms22168379
57. MacLennan IC, Gulbranson-Judge A, Toellner KM, Casamayor-Palleja M, Chan E, Sze DM, et al. The changing preference of T and B cells for partners as T-dependent antibody responses develop. Immunol Rev (1997) 156:53–66. doi: 10.1111/j.1600-065x.1997.tb00958.x
58. Oth T, Habets T, Germeraad W, Zonneveld MI, Bos G, Vanderlocht J. Pathogen recognition by NK cells amplifies the pro-inflammatory cytokine production of monocyte-derived DC via IFN-γ. BMC Immunol (2018) 19:8. doi: 10.1186/s12865-018-0247-y
59. Weiss E, Ziegler S, Fliesser M, Schmitt AL, Hünniger K, Kurzai O, et al. First insights in NK-DC cross-talk and the importance of soluble factors during infection with aspergillus fumigatus. Front Cell Infect Microbiol (2018) 8:288. doi: 10.3389/fcimb.2018.00288
60. Marcenaro E, Carlomagno S, Pesce S, Moretta A, Sivori S. NK/DC crosstalk in anti-viral response. Adv Exp Med Biol (2012) 946:295–308. doi: 10.1007/978-1-4614-0106-3_17
61. Liu SS, Liu C, Lv XX, Cui B, Yan J, Li YX, et al. The chemokine CCL1 triggers an AMFR-SPRY1 pathway that promotes differentiation of lung fibroblasts into myofibroblasts and drives pulmonary fibrosis. Immunity (2021) 54:2042–56. doi: 10.1016/j.immuni.2021.06.008
62. Öhlund D, Handly-Santana A, Biffi G, Elyada E, Almeida AS, Ponz-Sarvise M, et al. Distinct populations of inflammatory fibroblasts and myofibroblasts in pancreatic cancer. J Exp Med (2017) 214:579–96. doi: 10.1084/jem.20162024
63. Frangogiannis NG. The inflammatory response in myocardial injury, repair, and remodelling. Nat Rev Cardiol (2014) 11:255–65. doi: 10.1038/nrcardio.2014.28
64. Lee JG, Shim S, Kim MJ, Myung JK, Jang WS, Bae CH, et al. Pentoxifylline regulates plasminogen activator inhibitor-1 expression and protein kinase A phosphorylation in radiation-induced lung fibrosis. BioMed Res Int (2017) 2017:1279280. doi: 10.1155/2017/1279280
65. Duru N, Zhang Y, Gernapudi R, Wolfson B, Lo PK, Yao Y, et al. Loss of miR-140 is a key risk factor for radiation-induced lung fibrosis through reprogramming fibroblasts and macrophages. Sci Rep (2016) 6:39572. doi: 10.1038/srep39572
66. Judge JL, Owens KM, Pollock SJ, Woeller CF, Thatcher TH, Williams JP, et al. Ionizing radiation induces myofibroblast differentiation via lactate dehydrogenase. Am J Physiol Lung Cell Mol Physiol (2015) 309:L879–87. doi: 10.1152/ajplung.00153.2015
67. Bocchino M, Zanotta S, Capitelli L, Galati D. Dendritic cells are the intriguing players in the puzzle of idiopathic pulmonary fibrosis pathogenesis. Front Immunol (2021) 12:664109. doi: 10.3389/fimmu.2021.664109
68. Galati D, Zanotta S, Polistina GE, Coppola A, Capitelli L, Bocchino M. Circulating dendritic cells are severely decreased in idiopathic pulmonary fibrosis with a potential value for prognosis prediction. Clin Immunol (2020) 215:108454. doi: 10.1016/j.clim.2020.108454
69. Tort TM, Aschenbrenner F, Maus R, Stolper J, Schuette L, Knudsen L, et al. The FMS-like tyrosine kinase-3 ligand/lung dendritic cell axis contributes to regulation of pulmonary fibrosis. Thorax (2019) 74:947–57. doi: 10.1136/thoraxjnl-2018-212603
70. Christian DA, Adams TN, Shallberg LA, Phan AT, Smith TE, Abraha M, et al. cDC1 coordinate innate and adaptive responses in the omentum required for T cell priming and memory. Sci Immunol (2022) 7:q7432. doi: 10.1126/sciimmunol.abq7432
71. Gajewski TF, Cron KR. cDC1 dysregulation in cancer: An opportunity for intervention. J Exp Med (2020) 217:e20200816. doi: 10.1084/jem.20200816
72. Collin M, Bigley V. Human dendritic cell subsets: an update. Immunology (2018) 154:3–20. doi: 10.1111/imm.12888
73. Ferris ST, Durai V, Wu R, Theisen DJ, Ward JP, Bern MD, et al. cDC1 prime and are licensed by CD4(+) T cells to induce anti-tumour immunity. Nature (2020) 584:624–29. doi: 10.1038/s41586-020-2611-3
74. Izumi G, Nakano H, Nakano K, Whitehead GS, Grimm SA, Fessler MB, et al. CD11b(+) lung dendritic cells at different stages of maturation induce Th17 or Th2 differentiation. Nat Commun (2021) 12:5029. doi: 10.1038/s41467-021-25307-x
75. Anderson DR, Murphy KM, Briseño CG. Development, diversity, and function of dendritic cells in mouse and human. Cold Spring Harb Perspect Biol (2018) 10:a028613. doi: 10.1101/cshperspect.a028613
76. Mansouri S, Katikaneni DS, Gogoi H, Pipkin M, Machuca TN, Emtiazjoo AM, et al. Lung IFNAR1(hi) TNFR2(+) cDC2 promotes lung regulatory T cells induction and maintains lung mucosal tolerance at steady state. Mucosal Immunol (2020) 13:595–608. doi: 10.1038/s41385-020-0254-1
77. Brown CC, Gudjonson H, Pritykin Y, Deep D, Lavallée VP, Mendoza A, et al. Transcriptional basis of mouse and human dendritic cell heterogeneity. Cell (2019) 179:846–63. doi: 10.1016/j.cell.2019.09.035
78. Diener N, Fontaine JF, Klein M, Hieronymus T, Wanke F, Kurschus FC, et al. Posttranslational modifications by ADAM10 shape myeloid antigen-presenting cell homeostasis in the splenic marginal zone. Proc Natl Acad Sci U.S.A. (2021) 118:e2111234118. doi: 10.1073/pnas.2111234118
79. Xing E, Ma F, Wasikowski R, Billi AC, Gharaee-Kermani M, Fox J, et al. Pansclerotic morphea is characterized by IFN-γ responses priming dendritic cell fibroblast crosstalk to promote fibrosis. JCI Insight (2023) 8:e171307. doi: 10.1172/jci.insight.171307
80. Cyran L, Serfling J, Kirschner L, Raifer H, Lohoff M, Hermanns HM, et al. Flt3L, LIF, and IL-10 combination promotes the selective in vitro development of ESAM(low) cDC2B from murine bone marrow. Eur J Immunol (2022) 52:1946–60. doi: 10.1002/eji.202149663
81. Kang J, Kim M, Yoon DY, Kim WS, Choi SJ, Kwon YN, et al. AXL(+)SIGLEC6(+) dendritic cells in cerebrospinal fluid and brain tissues of patients with autoimmune inflammatory demyelinating disease of CNS. Clin Immunol (2023) 253:109686. doi: 10.1016/j.clim.2023.109686
82. Anselmi G, Vaivode K, Dutertre CA, Bourdely P, Missolo-Koussou Y, Newell E, et al. Engineered niches support the development of human dendritic cells in humanized mice. Nat Commun (2020) 11:2054. doi: 10.1038/s41467-020-15937-y
83. Shortman K, Sathe P, Vremec D, Naik S, O’Keeffe M. Plasmacytoid dendritic cell development. Adv Immunol (2013) 120:105–26. doi: 10.1016/B978-0-12-417028-5.00004-1
84. Ribeiro MS, Joshi G, Décembre E, Nuovo C, Bosseboeuf A, Bellomo A, et al. Tracking plasmacytoid dendritic cell response to physical contact with infected cells. Methods Mol Biol (2023) 2618:289–315. doi: 10.1007/978-1-0716-2938-3_21
85. Venet M, Ribeiro MS, Décembre E, Bellomo A, Joshi G, Nuovo C, et al. Severe COVID-19 patients have impaired plasmacytoid dendritic cell-mediated control of SARS-CoV-2. Nat Commun (2023) 14:694. doi: 10.1038/s41467-023-36140-9
86. Rajamanickam A, Kumar NP, Pandiaraj AN, Selvaraj N, Munisankar S, Renji RM, et al. Restoration of dendritic cell homeostasis and Type I/Type III interferon levels in convalescent COVID-19 individuals. BMC Immunol (2022) 23:51. doi: 10.1186/s12865-022-00526-z
87. Pang ES, Daraj G, Balka KR, De Nardo D, Macri C, Hochrein H, et al. Discordance in STING-induced activation and cell death between mouse and human dendritic cell populations. Front Immunol (2022) 13:794776. doi: 10.3389/fimmu.2022.794776
88. Cervantes-Barragan L, Vanderheiden A, Royer CJ, Davis-Gardner ME, Ralfs P, Chirkova T, et al. Plasmacytoid dendritic cells produce type I interferon and reduce viral replication in airway epithelial cells after SARS-CoV-2 infection. bioRxiv (2021) 179:846–63.e24. doi: 10.1101/2021.05.12.443948
89. Miura R, Kasakura K, Nakano N, Hara M, Maeda K, Okumura K, et al. Role of PU.1 in MHC class II expression via CIITA transcription in plasmacytoid dendritic cells. PloS One (2016) 11:e154094. doi: 10.1371/journal.pone.0154094
90. Sage AP, Murphy D, Maffia P, Masters LM, Sabir SR, Baker LL, et al. MHC Class II-restricted antigen presentation by plasmacytoid dendritic cells drives proatherogenic T cell immunity. Circulation (2014) 130:1363–73. doi: 10.1161/CIRCULATIONAHA.114.011090
91. Sadaka C, Marloie-Provost MA, Soumelis V, Benaroch P. Developmental regulation of MHC II expression and transport in human plasmacytoid-derived dendritic cells. Blood (2009) 113:2127–35. doi: 10.1182/blood-2008-10-178152
92. Young LJ, Wilson NS, Schnorrer P, Proietto A, Ten BT, Matsuki Y, et al. Differential MHC class II synthesis and ubiquitination confers distinct antigen-presenting properties on conventional and plasmacytoid dendritic cells. Nat Immunol (2008) 9:1244–52. doi: 10.1038/ni.1665
93. Rodrigues PF, Kouklas A, Cvijetic G, Bouladoux N, Mitrovic M, Desai JV, et al. pDC-like cells are pre-DC2 and require KLF4 to control homeostatic CD4 T cells. Sci Immunol (2023) 8:d4132. doi: 10.1126/sciimmunol.add4132
94. Sun L, Gang X, Li Z, Zhao X, Zhou T, Zhang S, et al. Advances in understanding the roles of CD244 (SLAMF4) in immune regulation and associated diseases. Front Immunol (2021) 12:648182. doi: 10.3389/fimmu.2021.648182
95. Dress RJ, Dutertre CA, Giladi A, Schlitzer A, Low I, Shadan NB, et al. Plasmacytoid dendritic cells develop from Ly6D(+) lymphoid progenitors distinct from the myeloid lineage. Nat Immunol (2019) 20:852–64. doi: 10.1038/s41590-019-0420-3
96. Fu C, Zhou L, Mi QS, Jiang A. Plasmacytoid dendritic cells and cancer immunotherapy. Cells-Basel (2022) 11:222. doi: 10.3390/cells11020222
97. Ram RR, Duatschek P, Margot N, Abram M, Geleziunas R, Hesselgesser J, et al. Activation of HIV-specific CD8(+) T-cells from HIV+ donors by vesatolimod. Antivir Ther (2020) 25:163–69. doi: 10.3851/IMP3359
98. Vanders RL, Gibson PG, Murphy VE, Wark PA. Plasmacytoid dendritic cells and CD8 T cells from pregnant women show altered phenotype and function following H1N1/09 infection. J Infect Dis (2013) 208:1062–70. doi: 10.1093/infdis/jit296
99. Hu X, Jiang C, Gao Y, Xue X. Human dendritic cell subsets in the glioblastoma-associated microenvironment. J Neuroimmunol (2023) 383:578147. doi: 10.1016/j.jneuroim.2023.578147
100. Shen Z, Luo W, Tan B, Nie K, Deng M, Wu S, et al. Roseburia intestinalis stimulates TLR5-dependent intestinal immunity against Crohn’s disease. Ebiomedicine (2022) 85:104285. doi: 10.1016/j.ebiom.2022.104285
101. Zafari R, Razi S, Rezaei N. The role of dendritic cells in neuroblastoma: Implications for immunotherapy. Immunobiology (2022) 227:152293. doi: 10.1016/j.imbio.2022.152293
102. Zhang M, Yu Q, Tang W, Wu Y, Lv J, Sun L, et al. Epithelial exosomal contactin-1 promotes monocyte-derived dendritic cell-dominant T-cell responses in asthma. J Allergy Clin Immunol (2021) 148:1545–58. doi: 10.1016/j.jaci.2021.04.025
103. Coutant F, Pin JJ, Miossec P. Extensive phenotype of human inflammatory monocyte-derived dendritic cells. Cells-Basel (2021) 10:1663. doi: 10.3390/cells10071663
104. Zeng Q, Zhou Y, Schwarz H. CD137L-DCs, potent immune-stimulators-history, characteristics, and perspectives. Front Immunol (2019) 10:2216. doi: 10.3389/fimmu.2019.02216
105. Coutant F. Shaping of monocyte-derived dendritic cell development and function by environmental factors in rheumatoid arthritis. Int J Mol Sci (2021) 22:13670. doi: 10.3390/ijms222413670
106. Nowatzky J, Manches O, Khan SA, Godefroy E, Bhardwaj N. Modulation of human Th17 cell responses through complement receptor 3 (CD11 b/CD18) ligation on monocyte-derived dendritic cells. J Autoimmun (2018) 92:57–66. doi: 10.1016/j.jaut.2018.05.005
107. Aono Y, Suzuki Y, Horiguchi R, Inoue Y, Karayama M, Hozumi H, et al. CD109 on dendritic cells regulates airway hyperreactivity and eosinophilic airway inflammation. Am J Respir Cell Mol Biol (2023) 68:201–12. doi: 10.1165/rcmb.2022-0109OC
108. Pivniouk V, Gimenes-Junior JA, Ezeh P, Michael A, Pivniouk O, Hahn S, et al. Airway administration of OM-85, a bacterial lysate, blocks experimental asthma by targeting dendritic cells and the epithelium/IL-33/ILC2 axis. J Allergy Clin Immunol (2022) 149:943–56. doi: 10.1016/j.jaci.2021.09.013
109. Furlong-Silva J, Cook PC. Fungal-mediated lung allergic airway disease: The critical role of macrophages and dendritic cells. PloS Pathog (2022) 18:e1010608. doi: 10.1371/journal.ppat.1010608
110. Li X, Cao Y, Mou M, Li J, Huang S, Zhang E, et al. Enhanced TLR5-dependent migration and activation of antigen-loaded airway dendritic cells by flagellin. J Leukoc Biol (2023) 113:567–76. doi: 10.1093/jleuko/qiad030
111. Wu Y, Yu Q, Zhang M, Zhou Y, Su X, Wu M, et al. Hemin-primed dendritic cells suppress allergic airway inflammation through releasing extracellular vesicles. J Leukoc Biol (2022) 111:837–48. doi: 10.1002/JLB.3A0321-175R
112. Sen K, Pati R, Jha A, Mishra GP, Prusty S, Chaudhary S, et al. NCoR1 controls immune tolerance in conventional dendritic cells by fine-tuning glycolysis and fatty acid oxidation. Redox Biol (2023) 59:102575. doi: 10.1016/j.redox.2022.102575
113. Bosteels V, Maréchal S, De Nolf C, Rennen S, Maelfait J, Tavernier SJ, et al. LXR signaling controls homeostatic dendritic cell maturation. Sci Immunol (2023) 8:d3955. doi: 10.1126/sciimmunol.add3955
114. Devi KS, Anandasabapathy N. The origin of DCs and capacity for immunologic tolerance in central and peripheral tissues. Semin Immunopathol (2017) 39:137–52. doi: 10.1007/s00281-016-0602-0
115. Chakraborty K, Chatterjee S, Bhattacharyya A. Modulation of CD11c+ lung dendritic cells in respect to TGF-β in experimental pulmonary fibrosis. Cell Biol Int (2017) 41:991–1000. doi: 10.1002/cbin.10800
116. Liu H, Jakubzick C, Osterburg AR, Nelson RL, Gupta N, McCormack FX, et al. Dendritic cell trafficking and function in rare lung diseases. Am J Respir Cell Mol Biol (2017) 57:393–402. doi: 10.1165/rcmb.2017-0051PS
117. Racanelli AC, Kikkers SA, Choi A, Cloonan SM. Autophagy and inflammation in chronic respiratory disease. Autophagy (2018) 14:221–32. doi: 10.1080/15548627.2017.1389823
118. Waisman A, Lukas D, Clausen BE, Yogev N. Dendritic cells as gatekeepers of tolerance. Semin Immunopathol (2017) 39:153–63. doi: 10.1007/s00281-016-0583-z
119. Cerboni S, Gentili M, Manel N. Diversity of pathogen sensors in dendritic cells. Adv Immunol (2013) 120:211–37. doi: 10.1016/B978-0-12-417028-5.00008-9
120. Mortellaro A, Conforti-Andreoni C, Fric J, Ricciardi-Castagnoli P. Dendritic cells as sensors of environmental perturbations. Microbes Infect (2008) 10:990–94. doi: 10.1016/j.micinf.2008.07.013
121. Durai V, Murphy KM. Functions of murine dendritic cells. Immunity (2016) 45:719–36. doi: 10.1016/j.immuni.2016.10.010
122. Segura E, Touzot M, Bohineust A, Cappuccio A, Chiocchia G, Hosmalin A, et al. Human inflammatory dendritic cells induce th17 cell differentiation. Immunity (2013) 38:336–48. doi: 10.1016/j.immuni.2012.10.018
123. Cao W, Chen G, Wu L, Yu KN, Sun M, Yang M, et al. Ionizing radiation triggers the antitumor immunity by inducing gasdermin E-mediated pyroptosis in tumor cells. Int J Radiat Oncol Biol Phys (2023) 115:440–52. doi: 10.1016/j.ijrobp.2022.07.1841
124. Liubarets TF. Ionizing radiation and cytokines: the role in the pathogenesis of plasma cell myeloma (literature review). Probl Radiac Med Radiobiol (2022) 27:120–30. doi: 10.33145/2304-8336-2022-27-120-130
125. Wang J, Xu Z, Wang Z, Du G, Lun L. TGF-beta signaling in cancer radiotherapy. Cytokine (2021) 148:155709. doi: 10.1016/j.cyto.2021.155709
126. Srivastava N, Bishnoi A, Parsad D, Kumaran MS, Vinay K, Gupta S. Dendritic cells sub-sets are associated with inflammatory cytokine production in progressive vitiligo disease. Arch Dermatol Res (2021) 313:759–67. doi: 10.1007/s00403-020-02168-w
127. Tormo AJ, Gauchat JF. A novel role for STAT5 in DC: Controlling the Th2-response. JAKSTAT (2013) 2:e25352. doi: 10.4161/jkst.25352
128. Zhang A, Fu J, Ning B, Li D, Sun N, Wei W, et al. Tolerogenic dendritic cells generated with IL-10/TGFβ1 relieve immune thrombocytopenia in mice. Thromb Res (2013) 132:63–8. doi: 10.1016/j.thromres.2013.04.001
129. Gagliostro V, Seeger P, Garrafa E, Salvi V, Bresciani R, Bosisio D, et al. Pro-lymphangiogenic properties of IFN-γ-activated human dendritic cells. Immunol Lett (2016) 173:26–35. doi: 10.1016/j.imlet.2016.03.008
130. Gowhari SA, Haleem AZ, Markov A, Valerievich YA, Ezzatifar F, Ahmadi M, et al. Chemokine CXCL14; a double-edged sword in cancer development. Int Immunopharmacol (2021) 97:107681. doi: 10.1016/j.intimp.2021.107681
131. Silva-Cardoso SC, Tao W, Fernández BM, Boes M, Radstake T, Pandit A. CXCL4 suppresses tolerogenic immune signature of monocyte-derived dendritic cells. Eur J Immunol (2020) 50:1598–601. doi: 10.1002/eji.201948341
132. Hespel C, Moser M. Role of inflammatory dendritic cells in innate and adaptive immunity. Eur J Immunol (2012) 42:2535–43. doi: 10.1002/eji.201242480
133. Gu FF, Wu JJ, Liu YY, Hu Y, Liang JY, Zhang K, et al. Human inflammatory dendritic cells in Malignant pleural effusions induce Th1 cell differentiation. Cancer Immunol Immunother (2020) 69:779–88. doi: 10.1007/s00262-020-02510-1
134. Sim WJ, Ahl PJ, Connolly JE. Metabolism is central to tolerogenic dendritic cell function. Mediators Inflammation (2016) 2016:2636701. doi: 10.1155/2016/2636701
135. Roberts CM, Foulcher E, Zaunders JJ, Bryant DH, Freund J, Cairns D, et al. Radiation pneumonitis: a possible lymphocyte-mediated hypersensitivity reaction. Ann Intern Med (1993) 118:696–700. doi: 10.7326/0003-4819-118-9-199305010-00006
136. Mascanfroni ID, Yeste A, Vieira SM, Burns EJ, Patel B, Sloma I, et al. IL-27 acts on DCs to suppress the T cell response and autoimmunity by inducing expression of the immunoregulatory molecule CD39. Nat Immunol (2013) 14:1054–63. doi: 10.1038/ni.2695
137. Song M, Ma X. The immunobiology of interleukin-35 and its regulation and gene expression. Adv Exp Med Biol (2016) 941:213–25. doi: 10.1007/978-94-024-0921-5_10
138. Ren Y, Yang Y, Yang J, Xie R, Fan H. Tolerogenic dendritic cells modified by tacrolimus suppress CD4(+) T-cell proliferation and inhibit collagen-induced arthritis in mice. Int Immunopharmacol (2014) 21:247–54. doi: 10.1016/j.intimp.2014.05.004
139. Volchenkov R, Karlsen M, Jonsson R, Appel S. Type 1 regulatory T cells and regulatory B cells induced by tolerogenic dendritic cells. Scand J Immunol (2013) 77:246–54. doi: 10.1111/sji.12039
140. Yan F, Cai L, Hui Y, Chen S, Meng H, Huang Z. Tolerogenic dendritic cells suppress murine corneal allograft rejection by modulating CD28/CTLA-4 expression on regulatory T cells. Cell Biol Int (2014) 38:835–48. doi: 10.1002/cbin.10268
141. Rolig AS, Rose DC, McGee GH, Rubas W, Kivimäe S, Redmond WL. Combining bempegaldesleukin (CD122-preferential IL-2 pathway agonist) and NKTR-262 (TLR7/8 agonist) improves systemic antitumor CD8(+) T cell cytotoxicity over BEMPEG+RT. J Immunother Cancer (2022) 10:e004218. doi: 10.1136/jitc-2021-004218
142. Song HY, Sik KW, Moo HJ, Yong PW, Lim ST, Byun EB. HMOC, a chrysin derivative, induces tolerogenic properties in lipopolysaccharide-stimulated dendritic cells. Int Immunopharmacol (2021) 95:107523. doi: 10.1016/j.intimp.2021.107523
143. Mahnke K, Johnson TS, Ring S, Enk AH. Tolerogenic dendritic cells and regulatory T cells: a two-way relationship. J Dermatol Sci (2007) 46:159–67. doi: 10.1016/j.jdermsci.2007.03.002
144. Dahlqvist G, Renaud S, Barjon C, Lefebvre A, Aoudjehane L, Horsmans Y, et al. Modulatory effect of rapamycin and tacrolimus on monocyte-derived dendritic cells phenotype and function. Immunobiology (2021) 226:152031. doi: 10.1016/j.imbio.2020.152031
145. Kwiecień I, Rutkowska E, Raniszewska A, Sokołowski R, Bednarek J, Jahnz-Różyk K, et al. Immunosuppressive properties of human PD-1 + , PDL-1 + and CD80 + dendritic cells from lymph nodes aspirates of lung cancer patients. Cancer Immunol Immunother (2022) 71:2469–83. doi: 10.1007/s00262-022-03178-5
146. Lee IK, Son YM, Ju YJ, Song SK, Gu M, Song KD, et al. Survival of porcine fibroblasts enhanced by human FasL and dexamethasone-treated human dendritic cells. vitro. Transpl Immunol (2014) 30:99–106. doi: 10.1016/j.trim.2014.01.002
147. Steinman RM, Koide S, Witmer M, Crowley M, Bhardwaj N, Freudenthal P, et al. The sensitization phase of T-cell-mediated immunity. Ann N Y Acad Sci (1988) 546:80–90. doi: 10.1111/j.1749-6632.1988.tb21622.x
148. Steinman RM, Inaba K. The binding of antigen presenting cells to T lymphocytes. Adv Exp Med Biol (1988) 237:31–41. doi: 10.1007/978-1-4684-5535-9_4
149. Iberg CA, Jones A, Hawiger D. Dendritic cells as inducers of peripheral tolerance. Trends Immunol (2017) 38:793–804. doi: 10.1016/j.it.2017.07.007
150. Zhang M, Tang H, Guo Z, An H, Zhu X, Song W, et al. Splenic stroma drives mature dendritic cells to differentiate into regulatory dendritic cells. Nat Immunol (2004) 5:1124–33. doi: 10.1038/ni1130
151. Min Z, Zeng Y, Zhu T, Cui B, Mao R, Jin M, et al. Lipopolysaccharide-activated bone marrow-derived dendritic cells suppress allergic airway inflammation by ameliorating the immune microenvironment. Front Immunol (2021) 12:595369. doi: 10.3389/fimmu.2021.595369
152. Han X, Wei Q, Xu RX, Wang S, Liu XY, Guo C, et al. Minocycline induces tolerance to dendritic cell production probably by targeting the SOCS1/TLR4/NF-κB signaling pathway. Transpl Immunol (2023) 79:101856. doi: 10.1016/j.trim.2023.101856
153. Adnan E, Matsumoto T, Ishizaki J, Onishi S, Suemori K, Yasukawa M, et al. Human tolerogenic dendritic cells generated with protein kinase C inhibitor are optimal for functional regulatory T cell induction - A comparative study. Clin Immunol (2016) 173:96–108. doi: 10.1016/j.clim.2016.09.007
154. Xiong A, Duan L, Chen J, Fan Z, Zheng F, Tan Z, et al. Flt3L combined with rapamycin promotes cardiac allograft tolerance by inducing regulatory dendritic cells and allograft autophagy in mice. PloS One (2012) 7:e46230. doi: 10.1371/journal.pone.0046230
155. Garcia AM, Bishop EL, Li D, Jeffery LE, Garten A, Thakker A, et al. Tolerogenic effects of 1,25-dihydroxyvitamin D on dendritic cells involve induction of fatty acid synthesis. J Steroid Biochem Mol Biol (2021) 211:105891. doi: 10.1016/j.jsbmb.2021.105891
156. Anderson AE, Swan DJ, Wong OY, Buck M, Eltherington O, Harry RA, et al. Tolerogenic dendritic cells generated with dexamethasone and vitamin D3 regulate rheumatoid arthritis CD4(+) T cells partly via transforming growth factor-β1. Clin Exp Immunol (2017) 187:113–23. doi: 10.1111/cei.12870
157. Bscheider M, Butcher EC. Vitamin D immunoregulation through dendritic cells. Immunology (2016) 148:227–36. doi: 10.1111/imm.12610
158. Ferreira GB, Gysemans CA, Demengeot J, Da CJ, Vanherwegen AS, Overbergh L, et al. 1,25-Dihydroxyvitamin D3 promotes tolerogenic dendritic cells with functional migratory properties in NOD mice. J Immunol (2014) 192:4210–20. doi: 10.4049/jimmunol.1302350
159. Botturi K, Lacoeuille Y, Thomas P, Boniface S, Reynaud-Gaubert M, Magnan A. CTLA-4-mediated regulatory phenotype of T-cells in tolerant lung recipients. Eur Respir J (2008) 31:1167–76. doi: 10.1183/09031936.00093207
160. van Wijk F, Nierkens S, de Jong W, Wehrens EJ, Boon L, van Kooten P, et al. The CD28/CTLA-4-B7 signaling pathway is involved in both allergic sensitization and tolerance induction to orally administered peanut proteins. J Immunol (2007) 178:6894–900. doi: 10.4049/jimmunol.178.11.6894
161. Fallarino F, Bianchi R, Orabona C, Vacca C, Belladonna ML, Fioretti MC, et al. CTLA-4-Ig activates forkhead transcription factors and protects dendritic cells from oxidative stress in nonobese diabetic mice. J Exp Med (2004) 200:1051–62. doi: 10.1084/jem.20040942
162. Orabona C, Grohmann U, Belladonna ML, Fallarino F, Vacca C, Bianchi R, et al. CD28 induces immunostimulatory signals in dendritic cells via CD80 and CD86. Nat Immunol (2004) 5:1134–42. doi: 10.1038/ni1124
163. Lu L, Lee WC, Takayama T, Qian S, Gambotto A, Robbins PD, et al. Genetic engineering of dendritic cells to express immunosuppressive molecules (viral IL-10, TGF-beta, and CTLA4Ig). J Leukoc Biol (1999) 66:293–96. doi: 10.1002/jlb.66.2.293
164. Iberg CA, Bourque J, Fallahee I, Son S, Hawiger D. TNF-α sculpts a maturation process in vivo by pruning tolerogenic dendritic cells. Cell Rep (2022) 39:110657. doi: 10.1016/j.celrep.2022.110657
165. Rodrigues CP, Ferreira AC, Pinho MP, de Moraes CJ, Bergami-Santos PC, Barbuto JA. Tolerogenic IDO(+) dendritic cells are induced by PD-1-expressing mast cells. Front Immunol (2016) 7:9. doi: 10.3389/fimmu.2016.00009
166. Li Q, Harden JL, Anderson CD, Egilmez NK. Tolerogenic phenotype of IFN-γ-induced IDO+ Dendritic cells is maintained via an autocrine IDO-kynurenine/ahR-IDO loop. J Immunol (2016) 197:962–70. doi: 10.4049/jimmunol.1502615
167. Yang J, Yang Y, Fan H, Zou H. Tolerogenic splenic IDO (+) dendritic cells from the mice treated with induced-Treg cells suppress collagen-induced arthritis. J Immunol Res (2014) 2014:831054. doi: 10.1155/2014/831054
168. Heitger A. Regulation of expression and function of IDO in human dendritic cells. Curr Med Chem (2011) 18:2222–33. doi: 10.2174/092986711795656018
Keywords: radiation-induced lung injury(RILI), tolerogenic dendritic cells(tolDCs), regulatory T cells(Tregs), regulatory B cells(Bregs), inflammation
Citation: Liu B, Wang Y, Han G and Zhu M (2024) Tolerogenic dendritic cells in radiation-induced lung injury. Front. Immunol. 14:1323676. doi: 10.3389/fimmu.2023.1323676
Received: 18 October 2023; Accepted: 15 December 2023;
Published: 08 January 2024.
Edited by:
Silvia Beatriz Boscardin, University of São Paulo, BrazilCopyright © 2024 Liu, Wang, Han and Zhu. This is an open-access article distributed under the terms of the Creative Commons Attribution License (CC BY). The use, distribution or reproduction in other forums is permitted, provided the original author(s) and the copyright owner(s) are credited and that the original publication in this journal is cited, in accordance with accepted academic practice. No use, distribution or reproduction is permitted which does not comply with these terms.
*Correspondence: Yilong Wang, bmt3YW5neWlsb25nQDEyNi5jb20=; Maoxiang Zhu, emh1bXgyMDE2QDEyNi5jb20=
Disclaimer: All claims expressed in this article are solely those of the authors and do not necessarily represent those of their affiliated organizations, or those of the publisher, the editors and the reviewers. Any product that may be evaluated in this article or claim that may be made by its manufacturer is not guaranteed or endorsed by the publisher.
Research integrity at Frontiers
Learn more about the work of our research integrity team to safeguard the quality of each article we publish.