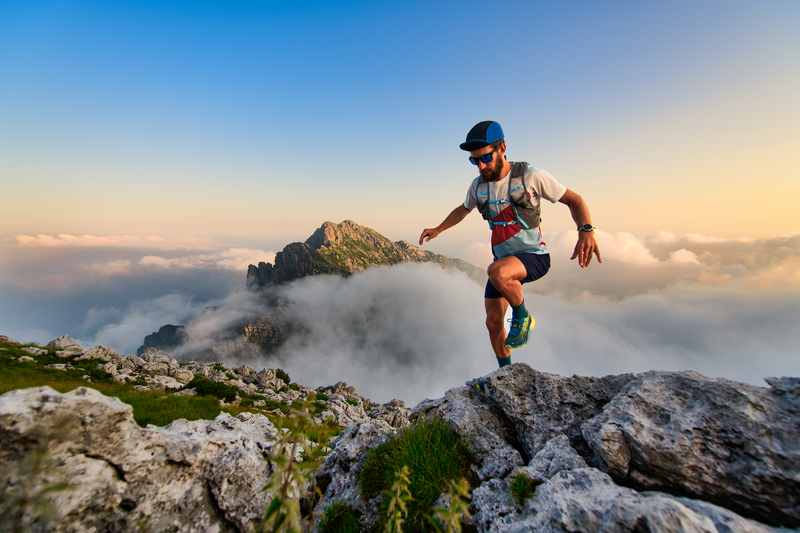
95% of researchers rate our articles as excellent or good
Learn more about the work of our research integrity team to safeguard the quality of each article we publish.
Find out more
ORIGINAL RESEARCH article
Front. Immunol. , 19 December 2023
Sec. Comparative Immunology
Volume 14 - 2023 | https://doi.org/10.3389/fimmu.2023.1319572
It is known that sperm and seminal plasma (SP) affect uterine immunity. In cattle, artificial insemination enables breeding by depositing frozen and largely diluted sperm with a negligible amount of SP into the uterus. Thus, the present study focused on the impact of frozen-thawed sperm on bovine uterine immunity. We have previously shown that in the bovine uterus, sperm swim smoothly over the luminal epithelium and some sperm interact with uterine glands to induce a weak inflammatory response mainly via the endometrial Toll-like receptor 2 (TLR2) signaling. However, the process by which sperm is encountered in the uterine glands is not completely clear. The present study intended to evaluate the role of sperm-TLR2 in sperm-uterine mucus penetration for reaching the glandular epithelium to induce the uterine immune response. To activate and block sperm-TLR2, they were treated with TLR2 agonist and antagonist, respectively. TLR2 activation enhanced sperm hyperactivation and improved its capacity to penetrate the artificial viscoelastic fluid and estrous-uterine-mucus. In contrast, TLR2-blocked sperm showed completely opposite effects. It is noteworthy, that the TLR2-activated sperm that penetrated the uterine mucus exhibited increased motile activity with hyperactivation. In the sperm-endometrial ex-vivo model, a greater amount of TLR2-activated sperm entered the uterine glands with an immune response, which was seen as the upregulation of mRNA expression for TNFA, IL1B, IL8, PGES, and TLR2 similar to those in control sperm. On the other hand, a lesser amount of TLR2-blocked sperm entered the uterine glands and weakened the sperm-induced increase only in PGES, suggesting that penetration of a certain number of sperm in the uterine gland is necessary enough to trigger the inflammatory response. Altogether, the present findings indicate that activation of sperm-TLR2 promotes their hyperactivation and mucus penetration with greater motility, allowing them to enter into the uterine glands more. This further suggests that the hyperactivated sperm contributes to triggering the pro-inflammatory cascade partly via TLR2 in the uterus.
In mammals, following natural mating or artificial insemination (AI), semen, which consists of sperm and seminal plasma (SP), enters the female reproductive tract (FRT) (1). During natural mating, in humans and cattle, semen is deposited in the cranial vagina, and from there, sperm migrate to enter the uterine lumen by leaving most of the SP behind in the vagina (1, 2). In pigs and horses, semen is directly deposited into the uterine body (1, 2). In mice, most of the semen is rapidly transported into the uterine cavity, and some remain in the vagina where it coagulates to form a copulatory plug (2). Sperm and SP are known to modulate the immune response of the FRT (3–5).
AI is the most common technique used for breeding cattle worldwide. During AI, sperm are directly deposited into the uterus, with a very low amount of SP, since they are considerably diluted during semen extension with the extender (6). Nevertheless, there are SP components that bind to the surface of sperm and are retained when sperm pass through the uterus (2, 7). Even though millions of sperm enter the uterus, only a few thousand sperm reach the oviduct (1). Our recent research has revealed that following AI, sperm are quickly transported from the site of semen deposition in the uterine body. Within an hour of AI, a large number of sperm pass through the uterine horn, while a massive number of sperm are excluded backward into the vagina. After 6 hours, only a few sperm are found remaining in the uterine lumen (8). Such rapid transport of sperm is independent of the active sperm motility and is regulated by the uterine smooth muscle contractions (9–12). During the sperm passage through the uterus, sperm encounter the uterus’s unique anatomical features such as the microarchitecture and mucus lining the endometrium (13).
The uterus not only facilitates the passage of sperm to the oviduct but also assists in eliminating the huge number of excess and dead sperm through its innate immunity (3). Of note, we have shown that in cattle, sperm interact with glandular epithelium to trigger an acute and transient inflammatory response against sperm (13, 14). Importantly, Toll-like receptor 2 (TLR2) (3, 13–16) in cattle and TLR4 in mice (17) have been recognized as the main mediators of uterine immune responses toward sperm.
TLRs are transmembrane proteins and an essential element of the innate immune system. They play a crucial role in pathogen-specific recognition (18). Even though TLRs are predominantly expressed in immune cells (19), several TLRs are generally present in cells of various tissues including in the male (20) and female reproductive tract (21). In cattle, endometrium expresses TLRs 1 to 10; specifically, TLRs 1 to 7 and 9 are expressed in the endometrial epithelial cells, while TLRs 1 to 4, 6, 7, 9, and 10 are expressed by stromal cells (22). TLR2 is localized in the surface and glandular epithelia of the endometrium of cows (15). In general, TLR2 recognizes various infectious pathogens and their products such as lipoproteins and peptidoglycans (PGN) of Gram-positive bacteria (18). Notably, TLR2 regulates physiological inflammation during fertilization when sperm interact with cumulus-oocyte complexes (23).
TLR2 is expressed also in the sperm of several species such as the Chinese soft-shelled turtle (24), mouse (25), rat (26), and human (27), but the role of TLR2 in sperm is almost unknown. It was reported that the presence of TLR2 in sperm is critical for long-term sperm survival (24) and protection against pathogens (27). It has been shown that sperm-TLR2 activation mediates the motility of sperm in humans and mice (27).
Recently, we investigated the interactions of bovine sperm with the endometrium using the ex-vivo sperm-endometrial model. Our results revealed that a few numbers of cultured sperm use their motility to swim smoothly over the luminal epithelium and invade the uterine glands to trigger the inflammatory response (13). This raises the question of which system regulates such particular features of the bovine sperm action to enhance their interaction with the uterine glands. Importantly, we have recently shown that during in-vitro fertilization (IVF), sperm-TLR2 activation stimulates sperm penetration to oocytes (28). This led us to hypothesize that sperm may acquire a particular motile pattern that is potentially induced by sperm-TLR2 activation, thereby efficiently penetrating the mucus to reach the glandular epithelium and induce uterine inflammatory responses.
To test the above hypothesis, at first, we confirmed the existence of TLR2 on the bull sperm, and then onwards we examined its impact on sperm motile pattern and subsequent mucus penetration since sperm needs to penetrate the viscoelastic mucus on the endometrial surface before interacting with the uterine glandular epithelium. Then, the impact of TLR2 activation/blockage on the penetration of sperm into the uterine gland and subsequent induction of uterine inflammatory response was examined.
The localization of TLR2 on sperm was evaluated using immunofluorescence staining. The impact of TLR2 activation and blockage on sperm were analyzed via flow cytometry and computer-assisted sperm analysis (CASA). The impact of sperm-TLR2 on mucus penetration as well as sperm penetration of the viscoelastic fluid and estrous-uterine-mucus was evaluated by the CASA outcomes. Then, we evaluated the role of sperm-TLR2 in sperm-uterine immune interactions, using the ex-vivo co-incubation model of sperm and endometrial tissue. The detailed experimental model is shown in Figure 1.
Figure 1 Schematic illustration of the experimental model. Initially, the localization of TLR2 in the frozen-thawed active bull sperm was analyzed using immunofluorescence staining. Frozen-thawed active (swim-up) bull sperm were treated with TLR1/2 agonist (to activate the TLR2) or antagonist (to block the TLR2). Sperm motion parameters and organelle functions were evaluated by CASA and flow cytometry analysis, respectively, to evaluate the effects of TLR2 activation and blockage on sperm. Since the sperm needs to penetrate the viscoelastic uterine mucus before entering into the uterine glands, at first, the specific sperm motile pattern (hyperactivated sperm) responsible for penetrating the mucus was calculated. Then, to observe the effect of activation of sperm-TLR2 on mucus penetration, percentages of sperm that penetrated the viscoelastic fluid (0.7% long-chain polyacrylamide, LC-PAM) and estrous-uterine-mucus were evaluated. Moreover, sperm motion patterns of uterine mucus-penetrated sperm were evaluated. Further, bovine endometrial explants were incubated with JC1-labeled sperm, and sperm-uterine gland interactions were observed using fluorescence microscopy. To evaluate the impact of sperm-TLR2 on uterine immune responses, TLR2-activated and -blocked sperm were incubated with endometrial explants, and mRNA expression of pro-inflammatory cytokines, PGES, and TLR2 was evaluated.
Chemicals were procured from Wako Pure Chemical Industries (Japan) except otherwise stated. The TALP (modified Tyrode balanced salt solution) (29) was used to wash and dilute sperm, as well as to incubate sperm with endometrial explants (13). The viscoelastic model mucus was prepared by adding Long-chain polyacrylamide, LC-PAM (5-6 MDa, Sigma-Aldrich, USA) into the TALP. The TALP and the model mucus were balanced in a 38.5°C incubator with 5% CO2.
The swim-up technique was used to separate the active sperm from the frozen-thawed semen (30). Briefly, frozen semen packed in 0.5 ml straws derived from three fertile Holstein bulls were used. The semen was collected and processed by the Genetics Hokkaido Association (Hokkaido, Japan) under controlled hygienic measures. The frozen-thawed semen was confirmed to be free from bacterial contamination. At first, sperm (0.25 ml) was layered under TALP (1 ml). After 1 h of incubation, the top sperm layers (0.5 ml) were collected, pooled, and washed at 200g for 5 min. To activate the TLR2 (15, 27, 28) in sperm, TLR1/2 agonist, Pam3Cys-Ser-(Lys)4 (Pam, ab142085, Abcam) (a synthetic analog of the triacylated N-terminal part of gram-positive bacterial peptidoglycan) was used. Initially, 100 µg/ml of stock agonist solution in 50% ethanol was prepared. To obtain TLR2-activated sperm (TLR2 ago.(+)), 100 ng/ml of agonist was incorporated into the sperm and incubated further for 2 h in a 38.5˚C incubator. Then, two times centrifugation at 200g for 10 min in 10 ml TALP was conducted to remove the agonist. Then, the sperm was suspended in TALP. TLR2 non-activated sperm (TLR2 ago (–).) were prepared in the same way (with the appropriate vehicle; 50% ethanol for TLR1/2 agonist) except without agonist. To block the TLR2 (15, 28) in sperm, TLR1/2 antagonist, a synthetic TLR2 blocker (CU-CPT22; Merck, Darmstadt, Germany) was used (31). Initially, 100 mM of stock antagonist solution in DMSO was prepared. To obtain TLR2-blocked sperm (TLR2 ant.(+)), 100 µM of the antagonist was incorporated into the sperm and incubated further for 30 min in a 38.5˚C incubator. Then, two times centrifugation at 200g for 10 min in 10 ml TALP was conducted to remove the antagonist. The resultant sperm were re-suspended in TALP. TLR2 non-blocked sperm (TLR2 ant (–).) were prepared in the same way (with the appropriate vehicle; DMSO for TLR1/2 antagonist) except without antagonists. The concentrations of the agonist and antagonist were chosen based on an earlier study (28). Sperm cell concentration was obtained via a hemocytometer (C-chip, NanoEnTek, Korea). To evaluate the sperm plasma membrane and acrosomal integrity (PMAI), high mitochondrial membrane potential (HMMP), plasma membrane stability, and sperm motion parameters, sperm were incubated for 0, 0.5, and 2 h in a 38.5˚C incubator.
Immunofluorescence was conducted to detect the TLR2 protein localization in bull sperm based on a previously described protocol (24) with modifications. The sperm (5x106 cells/ml) were spread on slides (S8226, Matsunami Glass Int., Osaka, Japan), air-dried at room temperature (RT), and fixed in 10% formalin in phosphate buffer (PB) at RT. After 10 min, the samples were immersed in absolute methanol for 5 min at -20˚C. Then, the samples were blocked with 5% BSA for 30 min at RT. Afterward, the slides were incubated overnight (4˚C in a humidified chamber) with the primary antibody (1:50, 10 µg/ml, rabbit polyclonal anti-TLR-2orb11487, Biorbyt, Cambridge, UK). Then, the sections were labeled with the secondary antibody (1:200, 10 µg/ml, goat anti-rabbit IgG labeled with Alexa Fluor 546, Invitrogen, Thermo Fisher Scientific, USA) for 1 h at RT. The samples were labeled with DAPI (1:50, 340-07971, Dojindo Laboratories, Japan) for 30 min. Slides were washed and mounted using VECTASHIELD mounting medium (H-1000; Vector Laboratories, Burlingame, CA 94010, USA). The Rabbit IgG isotype (1:500, 10 µg/ml, Invitrogen, Thermo Fisher Scientific) was applied as the IgG negative control. The sections were observed via fluorescence microscope (Keyence, BZ-X800, Osaka, Japan) through the BZ-X TexasRed (red wavelength) and BZ-X DAPI (blue wavelength) filters. Exposure time was maintained uniformly for all the sections.
The sperm PMAI and HMMP were assessed based on previous reports with minor modifications (32–34). Briefly, each treatment aliquot was labeled with Propidium Iodide (PI, 5 µg/ml, Sigma Aldrich) and Fluorescein isothiocyanate-conjugated peanut-agglutinin (FITC-PNA, 25 µg/ml, Vector Laboratories). PI and FITC-PNA negative cells were measured as sperm with intact plasma membrane and acrosome (PMAI). For evaluation of HMMP, each treatment aliquot was stained with 5,5’,6,6’-tetrachloro-1,1’3,3’-tetraethylbenzimidazolyl-carbocyanine iodide (JC1) fluorescent probe (5 µM, AdipoGen). The sperm emitting orange fluorescence were considered sperm with HMMP. The sperm plasma membrane stability of viable sperm was analyzed according to an earlier report (35). Each treatment aliquot was stained with cell viability indicator Yo-Pro 1 (50 nM, Y3603, Invitrogen) and plasma membrane destabilization indicator Merocyanine 540 (M540, 2.7 µM, 323756, Sigma Aldrich). Yo-Pro 1 and M540 negative cells were considered viable sperm with a stable plasma membrane. Percentages of sperm with stable plasma membranes were calculated out of total viable sperm. The labeled suspensions were incubated at 38.5˚C for 10 min under dark conditions. Sperm suspensions were analyzed using a spectral cell analyzer (Sony SA3800, Sony Imaging Products & Solutions., Tokyo, Japan). Each fluorochrome was excited by 488 nm laser, and emissions were detected on PMT channel 5-10 (515-546 nm, FITC), 18-19 (598-617 nm, PI), 5-8 (515-534 nm, JC1 Green), 15-17 (573-598 nm, JC1 Orange), 2 (503-507 nm, Yo-Pro 1), and 16 (581-589 nm, M540). The flow cytometer was used at short boost mode and a low flow rate of one. The acquisition was halted after recording 10,000 single sperm events. The representative flow cytometric dot plot diagrams are given in Supplementary Figure 1.
CASA was conducted according to an earlier report (36). A measurement of 3 µL (10 x 106 cells/ml) of sperm was added onto a warmed (38.5°C) chamber slide (SC20-01-04-B, Leja, GN Nieuw-Vennep, Netherlands). More than 200 sperm tracks were tracked via the CASA analyzer (SMAS, DITECT, Tokyo, Japan) at 150 frames per second (fps), depending on the images gained through a phase-contrast microscope (ECLIPSE Ci-L, Nikon, Tokyo, Japan) in each replicate. The experiment was repeated five times. The percentage of total, progressive, hyperactivated motile sperm, straight-line velocity (VSL), curvilinear velocity (VCL), average path velocity (VAP), linearity (LIN), straightness (STR), beat cross frequency (BCF), and amplitude of lateral head (ALH) were measured.
It was reported that, in humans, sperm that could penetrate periovulatory cervical mucus had an increased velocity and side-to-side head movement, with a similar group of kinetic properties with VAP ≥ 25.0 µm/s and ALH ≥ 7.5 µm (37, 38). Moreover, sperm showing VCL ≥ 150 µm/s, LIN ≤ 50%, and ALH ≥ 7.0 µm were classified as hyperactivated sperm (39). In cattle, sperm with increased mean VCL and ALH and decreased LIN were characterized as hyperactivated sperm (40), and these sperm are known to advance through the viscoelastic mucus of the female tract and matrix of the cumulus oophorus (41). These reports indicate that hyperactivated sperm can penetrate the mucus efficiently. Therefore, in the present study, the hyperactivated sperm were characterized as sperm that could penetrate the mucus to a greater extent when compared to the non-hyperactivated sperm and computed based on the sperm motion parameters VCL, ALH, and LIN.
At first, threshold values for hyperactivated sperm (41) were evaluated with calcium ionophore (A23187)-induced hyperactivation (42). Briefly, 5 x 106 cells/ml of sperm were treated with A23187 (final concentration 1µM, C7522, Sigma Aldrich) for 30 min, washed at 200g for 10 min, and evaluated via CASA (SMAS, DITECT, Tokyo, Japan) at 150 frames per second (fps). Two observers independently evaluated at least 200 individual sperm tracks. The experiment was repeated three times with different sperm suspensions. Based on the individual sperm trajectories, hyperactivated sperm were judged and the VCL, ALH, and LIN of each hyperactivated sperm were recorded as previously suggested (41). Since all the sperm that were judged as hyperactivated showed VCL ≥ 200 µm/s, ALH ≥ 3 µm, and LIN ≤ 40%, these values were set as the threshold values for hyperactivated bull sperm in our CASA system (SMAS, DITECT, Tokyo, Japan). Then, the percentage of hyperactivated sperm along with other sperm motility parameters were measured in suspensions of interest.
We investigated the influence of sperm-TLR2 on the ability of sperm to penetrate mucus via sperm-model viscoelastic fluid and -estrous-uterine-mucus penetration assays.
We used 0.7% of LC-PAM in the TALP medium as the model viscoelastic mucus. This media mimics the bovine estrous cervical mucus (43, 44). Since the overall mucus in the uterus may contain the majority of the cervical mucus (2) and the endometrial secretion, we used 0.7% LC-PAM solution for comparison with the mucus of the uterus. The percentages of viscoelastic fluid penetrated sperm were assessed by layering sperm over a viscoelastic fluid layer in a 1.5 ml microcentrifuge tube. Phenol red (1 mg/ml) was incorporated into the viscoelastic fluid to differentiate the sperm suspension and viscoelastic fluid layers. A measurement of 1 ml of viscoelastic fluid was placed in a microcentrifuge tube. A measurement of 0.5 ml of 10x106 cells/ml sperm suspension was gently layered over the viscoelastic fluid layer and incubated at 38.5°C incubator for 30 min. After the incubation period, the top 0.5 ml of the sperm suspension layer was gently aspirated. Additionally, 0.1 ml of the top viscoelastic fluid layer was aspirated to avoid the mixing of sperm suspension with the viscoelastic fluid layer. The remaining 0.9 ml of viscoelastic fluid was mixed and sperm concentration in the viscoelastic fluid was evaluated. Based on initial sperm concentration in the sperm suspension and final sperm concentration in the viscoelastic fluid layer, the proportion of viscoelastic fluid-penetrated sperm was calculated.
The estrous-uterine-mucus was obtained from pre-ovulatory stage cows through mechanical pressure. The uterine mucus was centrifuged twice at 7200g for 10min at 4°C and used in the sperm-mucus penetration assay as mentioned above. The 0.5 ml of uterine mucus was placed in a microcentrifuge tube and 0.25 ml of 10x106 cells/ml sperm was gently layered over the uterine mucus layer and incubated at 38.5°C for 30 min. After the incubation period, the top 0.25 ml of the sperm layer was gently aspirated. Additionally, 0.05 ml of the top uterine mucus layer was aspirated to avoid mixing the sperm suspension with the uterine mucus layer. The remaining 0.45 ml of uterine mucus was mixed and sperm concentration and motion behavior (via CASA) in the uterine mucus were evaluated. Based on the initial sperm concentration in the sperm suspension and final sperm concentration in the uterine mucus layer, the proportion of uterine mucus penetrating sperm was calculated. The estrous-uterine-mucus obtained from five different cows was used in the five repeats of this experiment.
The healthy bovine female reproductive tracts were obtained from the abattoir (Doto plant Tokachi Factory, Obihiro, Japan). The phase of the tract was identified based on a previous report (45). The ipsilateral ovarian follicle-containing horns from the preovulatory stage (days 19-22) were separated and transported on ice-cold saline. Endometrial explants were extracted and incubated based on an earlier defined protocol (13). Briefly, with the aid of a biopsy punch, explants were extracted from the glandular endometrial regions and pre-incubated in a 38.5˚C incubator for 15 min before starting the co-incubation with sperm.
As per the previous description, we evaluated the number of sperm within the uterine glands (13). Briefly, JC1-stained sperm (106 cells/ml) were cultured with endometrial explants. After 30 min, sperm in endometrial explants were viewed using the fluorescence microscope, and sperm numbers within the uterine glands were assessed. Videos and images were taken using the BZ-X TexasRed (red wavelength) and BZ-X GFP (green wavelength) filters. Three glands were assessed in each treatment; the experiment was repeated five times using uteri explants obtained from different cows.
The immune response of endometrial explants to TLR2-activated or blocked sperm was investigated based on a previously described method (13). Briefly, explants were co-cultured with TLR2-activated or blocked sperm (106 cells/ml) for 2 h then the explants were collected and washed three times in TALP to get rid of sperm and stored in TRIZOL at -80˚C for total RNA extraction. After washing, very few sperm remained within the uterine glands; thus, the explant preparation for analysis of mRNA expression contained very few sperm. Our group previously showed that sperm express a negligible amount of mRNA for our selected genes (30), so the changes in mRNA expression of these genes were only attributed to uterine explants in response to sperm.
Endometrial explants were homogenized (13) and RNA extraction was conducted based on a previously described protocol (46). Prior to cDNA synthesis, NanoDrop Spectrophotometer (2000c, Thermo Scientific, Waltham, MA, USA) was used to obtain the concentration and purity of RNA. The cDNA was synthesized following a previously described protocol (47).
Quantitative real-time PCR was conducted by a MiniOpticon (Bio-Rad Laboratories, Tokyo, Japan) via SYBR Green PCR Master Mix (Bio-Rad Laboratories, USA). The targeted primer pairs are listed in Supplementary Table 1. The amplification program was set up according to a previously described protocol (13). The melting curve was evaluated at the end of the run to observe the specificity of the amplification. A negative control, reaction-containing nuclease-free water or non-reverse transcribed RNA was incubated in each run. The housekeeping gene, β-actin, was used as an internal standard for normalization of Ct values. The Delta-Delta comparative threshold method was used to determine the fold changes in relative mRNA expression and these fold changes were used in the statistical analysis (48).
GraphPad Prism 5 software was applied for statistical analysis (GraphPad Software, La Jolla, CA, USA). The data in all experiments were normally distributed. The mean differences between the two groups were compared using the student t-test. The mean differences for more than two groups were compared using one-way ANOVA followed by Tukey’s tests. For mRNA expression, the animal was selected as a statistical unit and each experiment was repeated five times using explants from five different uteri (three explants/treatment/experiment). Each treatment had three replicates, and the mean of these replicates of each uterus was considered and these values were applied in the analysis and depicted in the figures. The values are presented as mean ± standard error of the mean (SEM). The significance of the data was determined based on their respective p-values where *P<0.05, **P<0.01, and ***P<0.001. All experiments were repeated five times.
Immunofluorescence analyses showed TLR2 protein localization in the bull sperm head’s posterior segment in all stained cells. The TLR2 activation or blockage did not change the TLR2 expression in the sperm head. The IgG controls did not show any fluorescent signal (Figure 2).
Figure 2 Immunofluorescence localization of TLR2 in bull sperm. The micrographs show the localization of TLR2 in non-treated, TLR2-activated (TLR2 ago.(+)), and TLR2-blocked (TLR2 ant. (+)) bull sperm. The localization was detected via immunofluorescence labeling with the Alexa-Fluor-conjugated anti-TLR2 antibody. For negative control, the sections were treated with Rabbit IgG isotype. DAPI stains the nucleus. The phase-contrast micrographs depict the morphology of the bull sperm. TLR2 was expressed in the posterior segment of the non-treated bull sperm head in all stained sperm. TLR2 localization did not change with the TLR2 activation or TLR2 blockage and was expressed in the same location as non-treated sperm. The result is representative of five separate experiments. In each experiment, more than 200 sperm per group were evaluated. Scale bar = 20µM.
Since sperm PMAI, HMMP, and plasma membrane stability were the important functional prerequisites for sperm action, we aimed to test whether TLR2 agonist (to activate TLR2) or antagonist (to block TLR2) had any negative effects on these sperm parameters via flow cytometric analysis. Moreover, mean values of sperm motion parameters were analyzed via CASA.
According to the flow cytometry analysis and CASA, neither the TLR2 activation nor blockage could affect the PMAI, HMMP, and plasma membrane stability (Supplementary Tables 2 and 3), as well as the percentage of total, progressive motile sperm and mean values of sperm motion parameters such as VSL, VCL, VAP, LIN, STR, BCF, and ALH of sperm when compared to non-treated sperm at 0, 0.5, and 2 h (Supplementary Tables 4 and 5).
The endometrial surface was coated with the viscoelastic mucus layer, and sperm had to penetrate this mucus before entering the uterine glands. Since sperm with hyperactivated motility were known to penetrate the mucus efficiently, we investigated whether regulation of sperm-TLR2 modulated sperm hyperactivation which was related to mucus penetration.
Initially, we determined the threshold values for hyperactivated sperm in the CASA system (please refer to the methodology section for more details). To do this, a calcium ionophore (A23187) was utilized to induce hyperactivation. All sperm with hyperactivation showed VCL ≥ 200 µm/s, ALH ≥ 3 µm, and LIN ≤ 40% (Figures 3A, B). As a result, these values were defined as the threshold values for hyperactivated bull sperm. The A23187 resulted in an increase in the mean values of VCL and ALH and a decrease in LIN (p<0.05) (Supplementary Table 6). The A23187 treatment pointedly enhanced the percentage of hyperactivated sperm compared to the control (p<0.001) (Figure 3C).
Figure 3 Sperm-TLR2 activation enhanced the hyperactivated sperm motility. (A) Sperm trajectory profiles of control and Calcium ionophore (A23187)-treated sperm. The arrows indicate the hyperactivated sperm trajectories. (B) Individual sperm trajectories of non-hyperactivated (VCL ≤ 200 µm/s, ALH ≤ 3 µm, and LIN ≥ 40%) and hyperactivated (VCL ≥ 200 µm/s, ALH ≥ 3 µm, and LIN ≤ 40%) sperm. (C) The percentage of hyperactivated sperm in control and A23187-treated groups. Data are presented as mean ± SEM of three independent experiments. In each experiment, 200 individual sperm tracks were evaluated. ***p<0.0001 denotes the significant difference. (D) The percentage of hyperactivated sperm in TLR2 non-activated (TLR2 ago (–).) and TLR2-activated (TLR2 ago.(+)) groups. (E) The percentage of hyperactivated sperm in TLR2 non-blocked (TLR2 ant (–).) and TLR2-blocked (TLR2 ant.(+)) groups. Data are presented as mean ± SEM of five independent experiments. In each experiment, 200 individual sperm tracks were evaluated. *p<0.05 denotes the significant difference.
Analyzing sperm motility revealed that a small portion of sperm (10-15%) acquired hyperactivation without any stimulus (Figures 3D, E). Notably, TLR2 activation increased the percentage of hyperactivated sperm at 0h compared to the non-activated sperm (p<0.05). However, activation of TLR2 did not modulate the percentage of hyperactivated sperm at 0.5 and 2h (Figure 3D). Meanwhile, TLR2 blockage reduced the percentage of hyperactivated sperm at 0.5h (p<0.05). The TLR2 blockage did not modulate the percentage of hyperactivated sperm at 0 and 2h (Figure 3E).
Since the endometrial surface was coated with a viscoelastic mucus layer, we tested whether sperm-TLR2 regulated sperm penetration to mucus via sperm-model viscoelastic fluid penetration assay (Figure 4A).
Figure 4 Sperm-TLR2 activation induced the sperm penetration of the viscoelastic fluid. (A) Brief methodology showing sperm penetrating the viscoelastic fluid (0.7% of long-chain polyacrylamide, LC-PAM). The number of sperm that penetrated the viscoelastic fluid was evaluated after 30 min following incubation of 10x106 cells/mL (0.5 ml) of sperm layering over the 1 ml of LC-PAM. (B) The percentage of penetrated TLR2 non-activated (TLR2 ago (–).) and TLR2-activated (TLR2 ago.(+)) sperm into the 0.9 ml LC-PAM fraction. (C) The percentage of penetrated TLR2 non-blocked (TLR2 ant (–).) and TLR2-blocked (TLR2 ant.(+)) sperm in the 0.9 ml LC-PAM fraction. Data are presented as mean ± SEM of five independent experiments. *p<0.05 denotes the significant difference.
In this assay, a significantly higher percentage of TLR2-activated sperm penetrated the viscoelastic fluid when compared to the non-activated sperm (p<0.05, ~ 20% increase vs. TLR2 non-activated sperm) (Figure 4B). Meanwhile, a lesser percentage of TLR2-blocked sperm penetrated the viscoelastic fluid when compared to the non-blocked sperm (p<0.05, ~ 22% reduction vs. TLR2 non-blocked sperm) (Figure 4C).
Since the sperm-TLR2 regulated the sperm penetration to model viscoelastic fluid, we, therefore, tested the sperm-TLR2 activation on sperm penetration to estrous-uterine-mucus (Figure 5A) and subsequent sperm motility behavior in this mucus. A significantly higher percentage of TLR2-activated sperm penetrated the estrous-uterine-mucus when compared to the non-activated sperm (p<0.05, ~ 36% increase vs. TLR2 non-activated sperm) (Figure 5B).
Figure 5 Sperm-TLR2 activation enhanced sperm penetration and motile activity in the estrous-uterine-mucus. (A) Brief methodology showing sperm penetrating the estrous-uterine-mucus. The number of sperm and sperm motion parameters that penetrated the estrous-uterine-mucus was evaluated after 30 min following incubation of 10x106 cells/mL (0.25 ml) of sperm layering over the 0.5 ml of uterine mucus. (B) The percentage of penetrated TLR2 non-activated (TLR2 ago (–).) and TLR2-activated (TLR2 ago.(+)) sperm into the 0.45 ml uterine mucus fraction. Data are presented as mean ± SEM of five independent experiments. *p<0.05 denotes the significant difference. (C) The motion parameters of estrous-uterine-mucus penetrated TLR2 ago (–). and TLR2 ago.(+) sperm analyzed via CASA. Data are presented as mean ± SEM of five independent experiments. In each experiment, 200 individual sperm tracks were evaluated. *p<0.05 denotes the significant difference.
Meanwhile, the percentage of total, progressive, and hyperactivated motility of TLR2-activated sperm that penetrated the estrous-uterine-mucus was significantly increased compared to the non-activated sperm (p<0.05). However, the mean values of other motion parameters such as VSL, VCL, VAP, LIN, STR, BCF, and ALH of TLR2-activated sperm were not affected in estrous-uterine-mucus (Figure 5C).
Fluorescence microscopy revealed that 30 min after the co-incubation of TLR2-activated or blocked sperm with endometrial explants, the sperm entered the uterine glands (Figures 6A and 7A). A higher number of TLR2-activated sperm entered and remained within the uterine glands when compared to TLR2 non-activated sperm (p<0.01, ~ 32% increase vs. TLR2 non-activated sperm) (Figures 6A, B). A lesser number of TLR2-blocked sperm remained in the uterine glands when compared to TLR2 non-blocked sperm (p<0.001, ~ 44% reduction vs. TLR2 non-blocked sperm) (Figures 7A, B).
Figure 6 Sperm-TLR2 activation increased sperm numbers in glands and modulated the subsequent sperm-induced mRNA expression in explants. (A) JC1 stained midpiece mitochondria of TLR2 non-activated (TLR2 ago.(-)) and TLR2-activated (TLR2 ago.(+)) sperm within the uterine glands after 30 min incubation with endometrial explants. Bar = 50µM. (B) The number of sperm/glands in explants incubated with TLR2 ago.(-) and TLR2 ago.(+) sperm. Data are presented as mean ± SEM of five independent experiments. **p<0.01 denotes the significant difference. (C) Relative mRNA expression of pro-inflammatory cytokines, IL8, PGES, and TLR2 in endometrial explants incubated with 106 cells/mL TLR2 ago.(-), TLR2 ago.(+), and without sperm (control) for 2 (h) Data are presented as mean ± SEM of five independent experiments. Three uterine explants for each treatment were used from an individual cow in each experiment. Different letters denote a significant difference (p<0.05).
Figure 7 Sperm-TLR2 blockage reduced sperm numbers in glands and modulated the subsequent sperm-induced mRNA expression in explants. (A) JC1 stained midpiece mitochondria of TLR2 non-blocked (TLR2 ant.(-)) and TLR2-blocked (TLR2 ant.(+)) sperm within the uterine glands after 30 min incubation with endometrial explants. Bar = 50µM. (B) The number of sperm/glands in explants incubated with TLR2 ant.(-) and TLR2 ant.(+) sperm. Data are presented as mean ± SEM of five independent experiments. ***p<0.001 denotes the significant difference. (C) Relative mRNA expression of pro-inflammatory cytokines, IL8, PGES, and TLR2 in endometrial explants incubated with 106 cells/mL of TLR2 ant.(-), TLR2 ant.(+), and without sperm (control) for 2 (h) Data are presented as mean ± SEM of five independent experiments. Three uterine explants for each treatment were used from an individual cow in each experiment. Different letters denote a significant difference (p<0.05).
Incubation of TLR2 non-activated or non-blocked sperm with endometrial explants upregulated the mRNA expression of tumor necrosis factor-alpha (TNFA), interleukin 1-beta (IL1B), interleukin 8 (IL8), prostaglandin E synthase (PGES), and TLR2 compared to the control (p<0.05) (Figures 6C and 7C). Incubation of TLR2-activated sperm with endometrial explants did not further upregulate the mRNA expression of observed genes (Figure 6C), whereas the exposure to TLR2-blocked sperm inhibited the sperm-induced increase in PGES mRNA expression (p<0.05) (Figure 7C). Moreover, TLR2-blocked sperm in comparison to the control did not upregulate the TNFA and IL1B mRNA expressions, whereas they upregulated the IL8 and TLR2 mRNA expressions (p<0.05) (Figure 7C).
In the uterus, sperm are rapidly transported in the uterine cavity through the contraction of uterine smooth muscles. For the first time, the present investigation demonstrates that sperm undergo hyperactivation via TLR2 and utilize the activated motility to penetrate the lining uterine mucus and invade the uterine glands to induce transient uterine inflammatory responses in cattle. The findings suggest that this sperm-TLR2-mediated hyperactivation may occur in the uterus after insemination. The working hypothesis for the role of sperm-TLR2 in sperm-uterine gland immune interaction is illustrated in Figure 8.
Figure 8 Schematic illustration for the working hypothesis of the interaction between bovine sperm and uterine gland to induce the inflammatory response. The Toll-like receptor 2 (TLR2) is localized in the posterior segment of the bull sperm head. In the uterus, sperm glide over the mucus layer on the surface epithelium while migrating toward the oviduct. (1) Activation of sperm-TLR2, possibly by the endogenous TLR2 ligands regulates the sperm motile pattern by increasing hyperactivated motility. (2) Presumably, these hyperactivated sperm overcome the greater resistance and fluid shear to penetrate through the viscoelastic estrous-uterine-mucus and enter into the uterine glands. (3) Subsequently, this sperm interacts with the uterine gland (4) to trigger the inflammatory response towards sperm. However, the detailed molecular binding mechanism of sperm-TLR2 in the direct interaction with the uterine gland epithelium is unknown and yet to be investigated.
The present study mainly focuses on studying the impact of sperm in the uterus giving special emphasis on AI, the technique exclusively used in cattle breeding worldwide. During AI, sperm are directly deposited in the uterus where the amount of SP that accompanies the sperm is reduced since it is significantly diluted using different semen extenders (~1% of SP in frozen semen straws) to maximize the efficiency of a single ejaculate, and routine use of AI reduces maternal exposure to SP (6). Therefore, in the present study, frozen-thawed, washed, and active sperm (via swim-up technique) were used to investigate the impact of sperm-TLR2 on sperm behavior and subsequent penetration into the mucus and uterine glands to trigger the uterine inflammatory response.
The existence of the uterine mucus plays a vital part in supporting sperm passage through the uterus. A viscoelastic mucus exists on the bovine surface endometrium, and sperm have to penetrate this mucus coating to enter the uterine glands (13). Importantly, sperm hyperactivation (i.e., sperm with higher curvilinear velocity, side-to-side head movement, and lower path linearity) (39, 40) facilitates sperm progress through the viscoelastic mucus of the female tract and cumulus oophorus matrix (41).
The involvement of sperm-TLR2 in regulating sperm penetration to oocytes during IVF (28) prompted us to investigate whether the activation of sperm-TLR2 triggers sperm hyperactivation and subsequent mucus penetration. In the present study, the calcium ionophore, a trigger of hyperactivation, increased the percentage of hyperactivated sperm and modulated the mean values of hyperactivated-related parameters (i.e., VCL, ALH, and LIN). The TLR2 activation and blockage increased and decreased the proportion of hyperactivated sperm, respectively. These indicate that the activation of sperm-TLR2 enhances hyperactivated motility, which is associated with the ability to penetrate mucus.
Although the used concentrations of TLR2 agonist or antagonist increased or decreased the sperm hyperactivation, respectively, they did not affect the total motile sperm, mean values of sperm motility parameters, and other functional parameters such as sperm plasma and acrosomal membrane integrity, high mitochondrial membrane potential, and plasma membrane stability throughout the observed time points, which are the prerequisites for their action for fertilization (32, 34, 35). This suggests that sperm-TLR2 partially regulates the motility pattern (i.e., toward hyperactivation) without interfering with other sperm parameters. The detailed mechanism by which sperm-TLR2 regulates the hyperactivation is unclear. However, we have shown previously that the activation of sperm-TLR2 enhances Ca2+ influx into sperm (28) which is essential for the induction of hyperactivated sperm movement. Therefore, it is possible that TLR2 activation might modulate the activity of sperm ion channels, such as the CatSper channel, which is responsible for the Ca2+ influx and the hyperactivation of sperm (41).
Moreover, the increase in ATP levels is required to induce hyperactivation (41). The present results show that sperm become hyperactivated without changing the mitochondrial membrane potential which is a measure of the ability of the mitochondria to produce ATP. It is possible that the TLR2-activated sperm may have a slight or transient increase in the mitochondrial membrane potential that is sufficient to provide the energy for hyperactivation but not enough to cause significant changes in the mitochondrial membrane. However, more sensitive analysis such as a luciferin-luciferase bioluminescence assay is required to measure the ATP levels to get a deeper understanding of this mechanism. Presumably, these hyperactivated sperm are strong enough to overcome the greater resistance (49, 50) and fluid shear to penetrate through the mucus (51, 52).
In support of the idea above, the proportions of TLR2-activated and -blocked sperm that penetrated the artificial viscoelastic fluid were higher and lower, respectively, compared to non-treated sperm. Importantly, TLR2-activated sperm penetrated the estrous-uterine-mucus in a greater proportion. Finally, in the ex-vivo sperm-endometrial explant co-culture model, the fact that the TLR2-activated and -blocked sperm entered the uterine glands with a higher and lower number revealed a clear association between sperm-TLR2 activation and motile activity together with their penetration of mucus and the uterine glands.
More exactly, a lesser portion of non-treated sperm also showed a hyperactivated motile pattern, entered the viscoelastic fluid as well as estrous-uterine-mucus, and penetrated the uterine glands, suggesting that highly active sperm (i.e., hyperactivated sperm) are not exclusive population, but rather a major one to enter the uterine glands. In fact, TLR2-activated sperm showed higher motile activity with hyperactivation after moving into estrous-uterine-mucus, supporting the above interpretation that these activated sperm are the major population detected in the uterine gland. Collectively, our results reveal that TLR2 activation enhances the sperm motile pattern and mucus penetration, thereby contributing to entering into the uterine glands. Since the present ex-vivo model has limitations on investigating the features of sperm within the uterine glands, further advanced investigations (such as artificial uterine models) are necessary to clarify which kind of sperm enters the uterine glands in the non-treated sperm group; in particular, it is worth revealing whether the TLR2 is activated in these sperm.
In the uterine explant ex-vivo model, the sperm typically trigger the weak inflammatory response with a slight upregulation of TNFA, IL1B, IL8, and PGES mRNA expressions (13). Even though a higher percentage of TLR2-activated sperm (by 32% increase vs. TLR2 non-activated sperm) entered the uterine glands, this did not further increase the mRNA expression of the above-investigated cytokines in the present study. This suggests that a certain, but not large, number of sperm acting in the uterine glands is sufficient for triggering the cascade of the maximum physiological inflammatory response toward sperm.
On the other hand, a lesser number of TLR2-blocked sperm (by 44% reduction vs. TLR2 non-blocked sperm) in uterine glands weakened the above inflammatory response. Together, the data suggest that a certain number of sperm with the ‘narrow range’ in the uterine gland is sufficient to trigger the physiological uterine inflammatory response. This mechanism of interaction of a small population of sperm with uterine glands might serve to trigger mild and transient inflammatory responses and avoid the induction of massive and generalized inflammation throughout the entire uterine mucosa during sperm transport. Such a weak pro-inflammatory response is essential to switch on the maternal innate immunity, which has a crucial part in eliminating dead and excess sperm in preparing the endometrium without critical damage for implantation (3, 53).
The endogenous ligands to activate the sperm-TLR2 have not been identified in the present study. The endogenous TLR2 ligand such as the extracellular matrix molecule, hyaluronan (23, 54), recognized as a regulator of TLR2 (55), is one of the candidates along with the other possible physiological uterine microbiota-derived ligands (56, 57). Very recently, we reported that hyaluronan was present in the bovine endometrium and modulated the sperm-endometrial epithelial immune interaction through the cluster of differentiation 44 (CD44) and TLR2 (58). Thus, hyaluronan could also assist in activating the sperm-TLR2 in-vivo.The TLR1/2 and TLR2/6 are two types of heterodimers that TLR2 forms with either TLR1 or TLR6. These heterodimers have different ligand specificities and signaling pathways, thus having different impacts on the immune response (14). The agonist, Pam3Cys-Ser-(Lys), and antagonist, CU-CPT22, used in the present study are both specific to the TLR1/2 complex. Therefore, it is highly possible that the observed effects are due to the triggering of TLR1/2 heterodimerization of the sperm. In the physiological status, sperm are known to exhibit hyperactivated motility in the oviduct, which is essential for fertilization (41). The present study reveals that hyperactivated sperm also have a significant role in the uterus, at least in cattle, where they penetrate the uterine glands to trigger the uterine immune response. The hyperactivated sperm in the uterus are probably no longer able to migrate toward the oviduct to contribute to fertilization since sperm are exposed to polymorphonuclear neutrophils attack inside the uterine glands (13). Conversely, linear progressive motile sperm in the uterus are known to migrate toward the oviduct to participate in the fertilization process (59) and are also reported to have greater fertilizing capacity (60). These suggest that the inseminated sperm activated by endogenous TLR2 ligands in the uterus probably contribute to triggering the innate immune cascade in the bovine uterus.
In conclusion, our findings provide evidence that, in cattle, sperm-TLR2 activation induces hyperactivation of sperm, successive mucus penetration, and sperm entering into the uterine glands. Thus, it seems that sperm-TLR2 plays a role in the initiation of the whole cascade of sperm-induced uterine inflammatory response. The in-detail binding mechanism of sperm-TLR2 in the direct interaction with the uterine gland epithelium is yet to be intensively investigated.
The original contributions presented in the study are included in the article/Supplementary Material. Further inquiries can be directed to the corresponding author.
The animal study was approved by The Committee on the Ethics of Animal Experiments of the Obihiro University of Agriculture and Veterinary Medicine, Japan (Permit number 27-74). The study was conducted in accordance with the local legislation and institutional requirements.
IA: Conceptualization, Data curation, Formal Analysis, Investigation, Methodology, Visualization, Writing – original draft, Writing – review & editing. YK: Data curation, Formal Analysis, Investigation, Methodology, Writing – review & editing. TU: Conceptualization, Investigation, Methodology, Resources, Writing – review & editing. CK: Data curation, Formal Analysis, Investigation, Methodology, Software, Writing – review & editing. MSa: Investigation, Methodology, Resources, Supervision, Visualization, Writing – review & editing. MM: Conceptualization, Methodology, Project administration, Supervision, Validation, Writing – original draft, Writing – review & editing. MY: Conceptualization, Data curation, Investigation, Methodology, Supervision, Writing – review & editing. SH: Formal Analysis, Investigation, Methodology, Resources, Visualization, Writing – review & editing. MSh: Conceptualization, Methodology, Project administration, Resources, Supervision, Validation, Writing – review & editing. AM: Conceptualization, Funding acquisition, Methodology, Project administration, Resources, Software, Supervision, Validation, Visualization, Writing – original draft, Writing – review & editing.
The author(s) declare financial support was received for the research, authorship, and/or publication of this article. The present research work was funded by a Grant-in-Aid for Scientific Research (20H03122, 22KF0018, and 23H02356) of Japan Society for the Promotion of Science (JSPS), and, in part, by the Livestock Promotional Funds of Japan Racing Association (JRA).
This work was supported by the Station for Management of Common Equipment, Obihiro University of Agriculture and Veterinary Medicine, Obihiro, Japan. The authors thank the Genetics Hokkaido Association, Shimizu-Cho, Hokkaido, Japan, for providing the cryopreserved semen straws used in this study.
The authors declare that the research was conducted in the absence of any commercial or financial relationships that could be construed as a potential conflict of interest.
All claims expressed in this article are solely those of the authors and do not necessarily represent those of their affiliated organizations, or those of the publisher, the editors and the reviewers. Any product that may be evaluated in this article, or claim that may be made by its manufacturer, is not guaranteed or endorsed by the publisher.
The Supplementary Material for this article can be found online at: https://www.frontiersin.org/articles/10.3389/fimmu.2023.1319572/full#supplementary-material
1. Croxatto HB. Physiology of gamete and embryo transport through the fallopian tube. Reprod BioMed Online (2002) 4:160–69. doi: 10.1016/S1472-6483(10)61935-9
2. Suarez SS, Pacey AA. Sperm transport in the female reproductive tract. Hum Reprod Update (2006) 12:23–37. doi: 10.1093/humupd/dmi047
3. Akthar I, Marey MA, Kim Y, Shimada M, Suarez SS, Miyamoto A. Sperm interaction with the uterine innate immune system: toll-like receptor 2 (TLR2) is a main sensor in cattle. Reprod Fertil Dev (2021) 34:139–48. doi: 10.1071/RD21265
4. Marey MA, Ezz MA, Akthar I, Yousef MS, Imakawa K, Shimada M, et al. Sensing sperm via maternal immune system: a potential mechanism for controlling microenvironment for fertility in the cow. J Anim Sci (2020) 98:S88–95. doi: 10.1093/jas/skaa147
5. Robertson SA. Seminal plasma and male factor signalling in the female reproductive tract. Cell Tissue Res (2005) 322:43–52. doi: 10.1007/s00441-005-1127-3
6. Vishwanath R. Artificial insemination: The state of the art. Theriogenology (2003) 59:571–84. doi: 10.1016/s0093-691x(02)01241-4
7. Suarez SS. Mammalian sperm interactions with the female reproductive tract. Cell Tissue Res (2016) 363:185–94. doi: 10.1007/s00441-015-2244-2
8. Marey MA, Ma D, Yoshino H, Elesh IF, Zinnah MA, Fiorenza MF, et al. Sperm induce proinflammatory responses in the uterus and peripheral blood immune cells of artificially inseminated cows. J Reprod Dev (2023) 69:95–102. doi: 10.1262/jrd.2022-124
9. Bourke DA, Lindsay FEF. Uterine contractions in the cow and associated movement of inseminate and other substances. 11th Int Congr Anim Reprod Dublin Ireland. Congress Proc (1988) 3:233.
10. Katila T, Sankari S, Makela O. Transport of spermatozoa in the reproductive tracts of mares. J Reprod Fertil Suppl (2000) 56:571–78.
11. Madill S, Troedsson MH, Alexander SL, Shand N, Santschi EM, Irvine CH. Simultaneous recording of pituitary oxytocin secretion and myometrial activity in oestrous mares exposed to various breeding stimuli. J Reprod Fertil Suppl (2000) 56:351–61.
12. Sumransap P, Tummaruk P, Kunavongkrit A. Sperm distribution in the reproductive tract of sows after intrauterine insemination. Reprod Domest Anim (2007) 42:113–7. doi: 10.1111/j.1439-0531.2006.00696.x
13. Akthar I, Suarez SS, Morillo VA, Sasaki M, Ezz MA, Takahashi K, et al. Sperm enter glands of preovulatory bovine endometrial explants and initiate inflammation. Reproduction (2020) 159:181–92. doi: 10.1530/REP-19-0414
14. Mansouri A, Yousef MS, Kowsar R, Usui N, Akthar I, Miyamoto A. Sperm activate TLR2/TLR1 heterodimerization to induce a weak proinflammatory response in the bovine uterus. Front Immunol (2023) 14:1158090. doi: 10.3389/fimmu.2023.1158090
15. Ezz MA, Marey MA, Elweza AE, Kawai T, Heppelmann M, Pfarrer C, et al. TLR2/4 signaling pathway mediates sperm-induced inflammation in bovine endometrial epithelial cells. vitro. PloS One (2019) 14:e0214516. doi: 10.1371/journal.pone.0214516
16. Elesh IF, Marey MA, Zinnah MA, Akthar I, Kawai T, Naim F, et al. Peptidoglycan switches off the TLR2-mediated sperm recognition and triggers sperm localization in the bovine endometrium. Front Immunol (2021) 11:619408. doi: 10.3389/fimmu.2020.619408
17. Schjenken JE, Sharkey DJ, Green ES, Chan HY, Matias RA, Moldenhauer LM, et al. Sperm modulate uterine immune parameters relevant to embryo implantation and reproductive success in mice. Commun Biol (2021) 4:572. doi: 10.1038/s42003-021-02038-9
18. Mogensen TH. Pathogen recognition and inflammatory signaling in innate immune defenses. Clin Microbiol Rev (2009) 22:240–73. doi: 10.1128/CMR.00046-08
19. Liu G, Zhao Y. Toll-like receptors and immune regulation: Their direct and indirect modulation on regulatory CD4+ CD25+ T cells. Immunology (2007) 122:149–56. doi: 10.1111/j.1365-2567.2007.02651.x
20. Pudney J, Anderson DJ. Expression of toll-like receptors in genital tract tissues from normal and HIV-infected men. Am J Reprod Immunol (2011) 65:28–43. doi: 10.1111/j.1600-0897.2010.00877.x
21. Fazeli A, Bruce C, Anumba DO. Characterization of toll-like receptors in the female reproductive tract in humans. Hum Reprod (2005) 20:1372–78. doi: 10.1093/humrep/deh775
22. Davies D, Meade KG, Herath S, Eckersall PD, Gonzalez D, White JO, et al. Toll-like receptor and antimicrobial peptide expression in the bovine endometrium. Reprod Biol Endocrinol (2008) 6:53. doi: 10.1186/1477-7827-6-53
23. Shimada M, Yanai Y, Okazaki T, Noma N, Kawashima I, Mori T, et al. Hyaluronan fragments generated by sperm-secreted hyaluronidase stimulate cytokine/chemokine production via the TLR2 and TLR4 pathway in cumulus cells of ovulated COCs, which may enhance fertilization. Development (2008) 135:2001–11. doi: 10.1242/dev.020461
24. Hu L, Li Q, Yang P, Gandahi JA, Arain TS, Le Y, et al. Expression of TLR2/4 on epididymal spermatozoa of the chinese soft-shelled turtle Pelodiscus sinensis during the hibernation season. Anat Rec (2016) 299:1578–84. doi: 10.1002/ar.23463
25. Zhu X, Shi D, Li X, Gong W, Wu F, Guo X, et al. TLR signalling affects sperm mitochondrial function and motility via phosphatidylinositol 3-kinase and glycogen synthase kinase-3α. Cell Signal (2016) 28:148–56. doi: 10.1016/j.cellsig.2015.12.002
26. Palladino MA, Savarese MA, Chapman JL, Dughi MK, Plaska D. Localization of toll-like receptors on epididymal epithelial cells and spermatozoa. Am J Reprod Immunol (2008) 60:541–55. doi: 10.1111/j.1600-0897.2008.00654.x
27. Fujita Y, Mihara T, Okazaki T, Shitanaka M, Kushino R, Ikeda C, et al. Toll-like receptors (TLR) 2 and 4 on human sperm recognize bacterial endotoxins and mediate apoptosis. Hum Reprod (2011) 26:2799–806. doi: 10.1093/humrep/der234
28. Ma D, Marey MA, Shimada M, Miyamoto A. Toll-like receptor 2 is involved in calcium influx and acrosome reaction to facilitate sperm penetration to oocytes during in vitro fertilization in cattle. Front Cell Dev Biol (2022) 10:810961. doi: 10.3389/fcell.2022.810961
29. Parrish JJ, Susko-Parrish J, Winer MA, First NL. Capacitation of bovine sperm by heparin. Biol Reprod (1988) 38:1171–80. doi: 10.1095/biolreprod38.5.1171
30. Elweza AE, Ezz MA, Acosta TJ, Talukder AK, Shimizu T, Hayakawa H, et al. A proinflammatory response of bovine endometrial epithelial cells to active sperm. vitro. Mol Reprod Dev (2018) 85:215–26. doi: 10.1002/mrd.22955
31. Cheng K, Wang X, Zhang S, Yin H. Discovery of small-molecule inhibitors of the TLR1/TLR2 complex. Angew Chem Int Ed Engl (2012) 51:12246–49. doi: 10.1002/anie.201204910
32. Garner DL, Thomas CA. Organelle-specific probe JC-1 identifies membrane potential differences in the mitochondrial function of bovine sperm. Mol Reprod Dev (1999) 53:222–29. doi: 10.1002/(SICI)1098-2795(199906)53:2<222::AID-MRD11>3.0.CO;2-L
33. Kanno C, Kang SS, Kitade Y, Yanagawa Y, Takahashi Y, Nagano M. Simultaneous evaluation of plasma membrane integrity, acrosomal integrity, and mitochondrial membrane potential in bovine spermatozoa by flow cytometry. Zygote (2016) 24:529–36. doi: 10.1017/S0967199415000490
34. Malama E, Zeron Y, Janett F, Siuda M, Roth Z, Bollwein H. Use of computer-assisted sperm analysis and flow cytometry to detect seasonal variations of bovine semen quality. Theriogenology (2017) 87:79–90. doi: 10.1016/j.theriogenology.2016.08.002
35. Hallap T, Nagy S, Jaakma Ü, Johannisson A, Rodriguez-Martinez H. Usefulness of a triple fluorochrome combination Merocyanine 540/Yo-Pro 1/Hoechst 33342 in assessing membrane stability of viable frozen-thawed spermatozoa from Estonian Holstein AI bulls. Theriogenology (2006) 65:1122–36. doi: 10.1016/j.theriogenology.2005.07.009
36. Kanno C, Sakamoto KQ, Yanagawa Y, Takahashi Y, Katagiri S, Nagano M. Comparison of sperm subpopulation structures in first and second ejaculated semen from Japanese black bulls by a cluster analysis of sperm motility evaluated by a CASA system. J Vet Med Sci (2017) 79:1359–65. doi: 10.1292/jvms.17-0012
37. Mortimer D, Pandya IJ, Sawers RS. Relationship between human sperm motility characteristics and sperm penetration into human cervical mucus. vitro. Reprod (1986) 78:93–102. doi: 10.1530/jrf.0.0780093
38. Mortimer ST. CASA-practical aspects. J Androl (2000) 21:515–24. doi: 10.1002/j.1939-4640.2000.tb02116.x
39. Mortimer ST, Swan MA, Mortimer D. Effect of seminal plasma on capacitation and hyperactivation in human spermatozoa. Hum Reprod (1998) 13:2139–46. doi: 10.1093/humrep/13.8.2139
40. Marquez B, Suarez SS. Bovine sperm hyperactivation is promoted by alkaline-stimulated Ca 2+ influx. Biol Reprod (2007) 76:660–65. doi: 10.1095/biolreprod.106.055038
41. Suarez SS. Control of hyperactivation in sperm. Hum Reprod Update (2008) 14:647–57. doi: 10.1093/humupd/dmn029
42. Kanno C, Sun-Sik K, Sakamoto KQ, Yanagawa Y, Katagiri S, Nagano M. Relationship between frame rates and subpopulation structure of bovine sperm divided by their motility analyzed by a computer-assisted sperm analysis system. Anim Sci J (2022) 93:e13796. doi: 10.1111/asj.13796
43. Tung CK, Hu L, Fiore AG, Ardon F, Hickman DG, Gilbert RO, et al. Microgrooves and fluid flows provide preferential passageways for sperm over pathogen Tritrichomonas foetus. Proc Natl Acad Sci (2015) 112:5431–36. doi: 10.1073/pnas.1500541112
44. Tung CK, Lin C, Harvey B, Fiore AG, Ardon F, Wu M, et al. Fluid viscoelasticity promotes collective swimming of sperm. Sci Rep (2017) 7:1–9. doi: 10.1038/s41598-017-03341-4
45. Wijayagunawardane MP, Miyamoto A, Cerbito WA, Acosta TJ, Takagi M, Sato K. Local distributions of oviductal estradiol, progesterone, prostaglandins, oxytocin and endothelin-1 in the cyclic cow. Theriogenology (1998) 49:607–18. doi: 10.1016/S0093-691X(98)00011-9
46. Chomczynski P, Sacchi N. Single-step method of RNA isolation by acid guanidinium thiocyanate-phenol-chloroform extraction. Anal Biochem (1987) 162:156–59. doi: 10.1016/0003-2697(87)90021-2
47. Yousef MS, Marey MA, Hambruch N, Hayakawa H, Shimizu T, Hussien HA, et al. Sperm binding to oviduct epithelial cells enhances TGFB1 and IL10 expressions in epithelial cells as well as neutrophils in vitro: prostaglandin E2 as a main regulator of anti-inflammatory response in the bovine Oviduct. PloS One (2016) 11:e0162309. doi: 10.1371/journal.pone.0162309
48. Livak KJ, Schmittgen TD. Analysis of relative gene expression data using real-time quantitative PCR and the 2-ΔΔCT method. Methods (2001) 25:402–08. doi: 10.1006/meth.2001.1262
49. Barton M, Wiesner BP. Receptivity of cervical mucus to spermatozoa. Br Med J (1946) 2:606–10. doi: 10.1136/bmj.2.4477.606
50. Yudin AI, Hanson FW, Katz DF. Human cervical mucus and its interaction with sperm: A fine-structural view. Biol Reprod (1989) 40:661–71. doi: 10.1095/biolreprod40.3.661
51. Figueroa-Morales N, Dominguez-Rubio L, Ott TL, Aranson IS. Mechanical shear controls bacterial penetration in mucus. Sci Rep (2019) 9:9713. doi: 10.1038/s41598-019-46085-z
52. Zimmer RK, Riffell JA. Sperm chemotaxis, fluid shear, and the evolution of sexual reproduction. Proc Natl Acad Sci (2011) 108:13200–205. doi: 10.1073/pnas.1018666108
53. Rozeboom KJ, Troedsson MH, Crabo BG. Characterization of uterine leukocyte infiltration in gilts after artificial insemination. Reproduction (1998) 114:195–99. doi: 10.1530/jrf.0.1140195
54. Bourguignon LYW, Wong G, Earle CA, Xia W. Interaction of low molecular weight hyaluronan with CD44 and toll-like receptors promotes the actin filament-associated protein 110-actin binding and MyD88-NFκB signaling leading to proinflammatory cytokine/chemokine production and breast tumor invasion. Cytoskeleton (2011) 68:671–93. doi: 10.1002/cm.20544
55. Ebid R, Lichtnekert J, Anders HJ. Hyaluronan is not a ligand but a regulator of toll-like receptor signaling in mesangial cells: role of extracellular matrix in innate immunity. ISRN Nephrol (2014) 2014:1–11. doi: 10.1155/2014/714081
56. Ballas P, Reinlander U, Schlegl R, Ehling-Schulz M, Drillich M, Wagener K. Characterization of intrauterine cultivable aerobic microbiota at the time of insemination in dairy cows with and without mild endometritis. Theriogenology (2021) 159:28–34. doi: 10.1016/j.theriogenology.2020.10.018
57. Appiah MO, Wang J, Lu W. Microflora in the reproductive tract of cattle: A review. Agriculture (2020) 10:232. doi: 10.3390/agriculture10060232
58. Ezz MA, Mansouri A, Akthar I, Yousef MS, Kowsar R, Miyamoto A. Hyaluronan regulates sperm-induced inflammatory response by enhancing sperm attachment to bovine endometrial epithelial cells via CD44: in-silico and in-vitro approaches. Front Endocrinol (2023) 14:1134868. doi: 10.3389/fendo.2023.1134868
59. Shalgi R, Smith TT, Yanagimachi R. A quantitative comparison of the passage of capacitated and uncapacitated hamster spermatozoa through the uterotubal junction. Biol Reprod (1992) 46:419–24. doi: 10.1095/biolreprod46.3.419
Keywords: cattle, hyperactivation, immune response, mucus, sperm, Toll-like receptor 2, uterine gland
Citation: Akthar I, Kim Y, Umehara T, Kanno C, Sasaki M, Marey MA, Yousef MS, Haneda S, Shimada M and Miyamoto A (2023) Activation of sperm Toll-like receptor 2 induces hyperactivation to enhance the penetration to mucus and uterine glands: a trigger for the uterine inflammatory cascade in cattle. Front. Immunol. 14:1319572. doi: 10.3389/fimmu.2023.1319572
Received: 11 October 2023; Accepted: 07 December 2023;
Published: 19 December 2023.
Edited by:
Dirk Werling, Royal Veterinary College (RVC), United KingdomReviewed by:
Shavahn C. Loux, University of Kentucky, United StatesCopyright © 2023 Akthar, Kim, Umehara, Kanno, Sasaki, Marey, Yousef, Haneda, Shimada and Miyamoto. This is an open-access article distributed under the terms of the Creative Commons Attribution License (CC BY). The use, distribution or reproduction in other forums is permitted, provided the original author(s) and the copyright owner(s) are credited and that the original publication in this journal is cited, in accordance with accepted academic practice. No use, distribution or reproduction is permitted which does not comply with these terms.
*Correspondence: Akio Miyamoto, YWtpb21peWFAb2JpaGlyby5hYy5qcA==
Disclaimer: All claims expressed in this article are solely those of the authors and do not necessarily represent those of their affiliated organizations, or those of the publisher, the editors and the reviewers. Any product that may be evaluated in this article or claim that may be made by its manufacturer is not guaranteed or endorsed by the publisher.
Research integrity at Frontiers
Learn more about the work of our research integrity team to safeguard the quality of each article we publish.