- 1Department of Radiation Oncology, The First Affiliated Hospital of Nanjing Medical University, Nanjing, China
- 2Institution of Radiation Medicine, Shanghai Medical College, Fudan University, Shanghai, China
Natural killer (NK) cells are innate lymphocytes possessing potent tumor surveillance and elimination activity. Increasing attention is being focused on the role of NK cells in integral antitumor strategies (especially immunotherapy). Of note, therapeutic efficacy is considerable dependent on two parameters: the infiltration and cytotoxicity of NK cells in tumor microenvironment (TME), both of which are impaired by several obstacles (e.g., chemokines, hypoxia). Strategies to overcome such barriers are needed. Radiotherapy is a conventional modality employed to cure solid tumors. Recent studies suggest that radiotherapy not only damages tumor cells directly, but also enhances tumor recognition by immune cells through altering molecular expression of tumor or immune cells via the in situ or abscopal effect. Thus, radiotherapy may rebuild a NK cells-favored TME, and thus provide a cost-effective approach to improve the infiltration of NK cells into solid tumors, as well as elevate immune-activity. Moreover, the radioresistance of tumor always hampers the response to radiotherapy. Noteworthy, the puissant cytotoxic activity of NK cells not only kills tumor cells directly, but also increases the response of tumors to radiation via activating several radiosensitization pathways. Herein, we review the mechanisms by which NK cells and radiotherapy mutually promote their killing function against solid malignancies. We also discuss potential strategies harnessing such features in combined anticancer care.
1 Introduction
Adoptive cell therapy (ACT) is an extremely personalized immunotherapy which involves adoptive transfer of autologous lymphocytes into patients with advanced malignancies, especially hematologic neoplasms, who have exhibited remarkable tumor eradication (1). Since 1988, when the first demonstration of autologous tumor-infiltrating T lymphocytes-based ACT mediated objective tumor regression in patients with metastatic melanoma, T lymphocytes were the only authoritative ACT target over almost twenty years (2). Nonetheless, numerous “unsuccessful” cases did remind us T lymphocytes-based ACT was not always as satisfactory as we thought, and rate of relapse or no-remission was significantly higher than we could accept (3–6). Mechanically, these confusions are derived from several barriers in T lymphocytes therapy, including severe life-threatening toxicities, restricted trafficking, limited tumor infiltration, and in particular, modest anti-tumor activity and antigen escape (7–10). Apart from seeking technological improvements for solving these obstacles, researchers also explored other antitumor lymphocytes, which could be chosen for high-avidity tumor recognition, as well as for effective agents required to induce cancer elimination in the use of ACT. The natural killer (NK) cell-based immunotherapy was then developed.
As a subset of innate lymphoid cells, NK cells are currently considered exerting natural cytotoxicity against primary malignancy and metastasis by suppressing proliferation, migration and colonization to distant tissues (11). In addition to their cytotoxic role, NK cells have been reported to produce plenty of cytokines, mainly interferon-γ (IFN-γ), to modulate adaptive immune responses or other related pathways (12, 13). Of note, NK cells are capable of distinguishing abnormal cells from healthy ones, providing accurate anticancer cytotoxicity and alleviating off-target complications (14, 15), which should be the more important reason for NK cells naturally emerging as a promising target for ACT. In the first pioneering clinical practice in 2005, Millier and colleagues adoptively transferred allogeneic activated NK cells into patients with acute myeloid leukemia, leading to major tumor regression (16). Up to now, numerous clinical studies revealed that adoptively transferred NK cells triggered robust eradication of primary leukemic blasts, substantially reduced the burden of acute myeloid leukemia, and lengthened the overall survival of patients (17–19). However in solid tumors, the therapeutic efficiency of NK cell-based ACT is much less than desired (20, 21), and results from more than 40 clinical trials have been inconsistent (22). The de facto clinical benefits of alloreactive NK cells in patients with neurologic tumors have been documented in two instances (23, 24). In contrast to hematologic malignancies, solid tumors are characterized by dense physical structures and a supportive TME that is often impervious to effector immune cells (25). Thus, two inevitable parameters are reported to mainly determine the therapeutic efficacy for alloreactive NK cells in patients with solid tumors: (i) intra-tumoral infiltration (26); (ii) persistence in a robust anti-tumor state (16). With respect to the determinants against infiltration of NK cells into tumors, C-X-C motif chemokine ligand 9 (CXCL9), CXCL10, or CXCL11 (which are all ligands to the C-X-C motif chemokine receptor 3 (CXCR3), a receptor that plays a crucial part in the recruitment of NK cells in solid tumors) have shown substantial secretion in an experimental model of lymphoma, lung adenocarcinomas, and melanomas tissues (27–29). Several studies have also uncovered the obstacles preventing persistence of an activated state for transferred NK cells, including the abundance of interleukin (IL) -2, IL-12, IL-15 (and many other cytokines) and hypoxia or reactive oxygen species (ROS) in the TME (30–33). Obviously, the two parameters mentioned above are deeply affected by the functional factors in TME. Under normal circumstances, the specificity of the TME tends to prevent the infiltration of NK cells into solid tumors, as well as inhibit anticancer activation. Efforts have been made to remove functional damage to NK cells by pharmaceutical or non-pharmaceutical means (34–36), but it takes years for these external interventions to enter clinical practice.
Over decades, radiotherapy has been a conventional therapeutic modality for patients with solid neoplasms. In recent years, radiotherapy has been employed not only for its direct tumoral-killing ability, but also TME modulation (37–40). An increasingly emphasized byproduct of the radiation damage to tumor cells is the secretion/release of various cytokines, chemokines or tumor-associated antigens (TAAs) in TME (41, 42), contributing to the underlying role of radiotherapy to serve as an “in situ vaccine”, rendering immune-insensitive tumors responsive to immunotherapy. Thus, it has been speculated that radiotherapy mediates a favorable TME for the infiltration and activation of NK cells in solid tumors. Additionally, despite survival rates of patients with solid malignancies are elevated by assistance of radiotherapy, radioresistant properties of tumors remain a significant barrier for curative treatment (43). Clinical interferences on traditional radioresistant pathways, including DNA damage repair or protective autophagy, still did not improve therapeutic efficiency to a gratifying level. In the era of cancer immunotherapy, a new appreciation of the role played by immune system in governing the therapeutic effect of radiotherapy has caused a major spike in interest. As a novel and pivotal part of cancer immunotherapy, NK cell-based ACT definitely has tight interaction with radiotherapy, which has obtained considerable support from preclinical and clinical data (Table 1). Underlying these trials are the hope that radiotherapy would optimize immune activity of NK cells in solid tumors, while alloreactive NK cells further amplify the tumor response of radiotherapy.
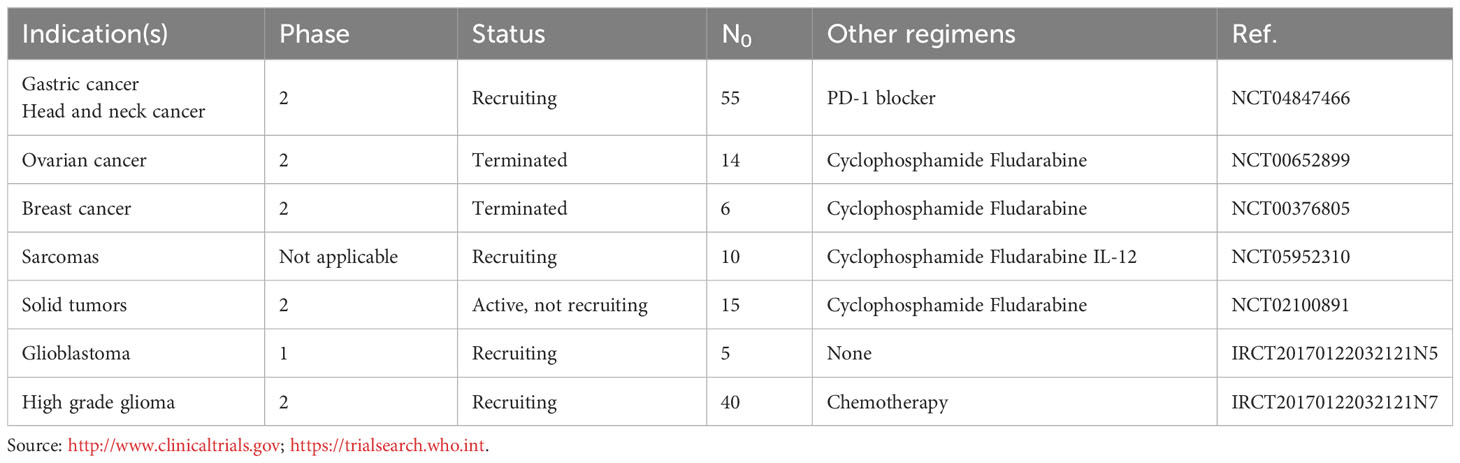
Table 1 Clinical trials testing allogenic NK cells therapy combined with radiotherapy in patients with solid tumors.
Herein, we review the mechanisms by which NK cells and radiotherapy mutually promote their killing function against solid malignancies. We also discuss potential strategies harnessing such features in supporting anticancer care.
2 Radiotherapy encourages the optimal anti-tumor activity of NK cells
Several environmental features play essential roles in preventing the promising function of NK cells in solid tumors. Radiotherapy (a robust modulator of the TME) can eliminate such barriers and contributes (but is not limited) to ameliorating the trafficking of NK cells as well as enhanced cytotoxic functions.
2.1 Facilitation of trafficking of NK cells
For almost all anticancer chemo- or immune-therapy, the inevitable question in clinical practice is how to enhance the abundance of functional agents intratumorally. NK cell-based ACT has no exception. However, investigation of tumor biopsy specimens for various solid neoplasms, no matter patients derived or animal derived, have demonstrated little infiltration of NK cells into these tumors (44–46). The interactions between chemokines and their corresponding chemokine receptors have been widely explored for their roles in driving NK cells trafficking (47, 48). Chemokine receptors are grouped by structure into four types: C-X-C chemokine receptor (CXCR), C-C chemokine receptor (CCR), C-X3-C chemokine receptor (CX3CR), and XCR (49), among which CXCR3 expression is to date one of the marker providing the most reliable message about the accumulation of NK cells. In general, CXCR3 is highly expressed on the surface of NK cells, and it drives NK cell-specific chemotaxis toward the CXCR3 ligands CXCL9, CXCL10 and CXCL11, which are secreted by tumor cells into TME and usually expressed at relative low levels in homeostatic condition (50–52). Hence, strategies to increase CXCR3 expression by NK cells and/or raise the abundance of CXCR3 ligands secreted in the TME may lead to enhanced recruitment of NK cells into solid malignancies. Cytokine stimulation upon NK cells was proved to be able to modulate the expression of CXCR3. It was shown that ex vivo expansion of NK cells in the coculture of IL-2 and feeder cells (EBV-LCLs) significantly upregulated the expression of CXCR3 on NK cells, resulting in increased migratory capacity toward CXCL10-producing tumor cells in vitro (27). Besides, pharmaceutical interventions such as BXCL701 (inhibitor of dipeptidyl peptidase) and tazemetostat (a polycomb histone-lysine N-methyltransferase enzyme-EZH2 inhibitor) can stimulate CXCL9/10 production, which has been shown to lead to enhanced NK-cell infiltration as well as cytotoxicity and eradication of pancreatic ductal adenocarcinoma in a mouse model (53, 54). Radiotherapy has been reported to elicit similar results as those reported above in several experimental and clinical models. Specifically, radiotherapy combined with inhibitors of ATR serine/threonine kinase triggered CXCL10 expression and increased infiltration of innate immune cells (including NK cells) in models of human papillomavirus-driven malignancies (55). Also, in models of Kirsten rat sarcoma virus-mutated carcinomas, radiotherapy enhanced CXCL10 secretion, promoted anticancer immunity, and prolonged survival (56). Furthermore, the mechanisms by which radiotherapy influences expression of CXCR3 ligands have been postulated. Recent research has suggested that radiotherapy drives a type-I IFN response that culminates with CXCL10 secretion via accumulation of nuclear and mitochondrial DNA in the cytoplasm and consequent activation of cyclic guanosine monophosphate-adenosine monophosphate synthase (cGAS) (57). Moreover, radiotherapy can also lead to phosphorylation of p38 mitogen-activated protein kinase and increased expression of signal transducer and activator of transcription 1 (STAT1) which, ultimately, augments CXCL10 production (58, 59).
Apart from driving infiltration of NK cells into solid tumors, CXCR3 is also preferentially expressed on a number of cancer cells. In addition, several evidences reveal that CXCR3 on tumor cells surface facilitates the proliferation and metastasis of a variety of malignancies, including colon cancer (60) and melanoma (61), and CXCR3 blockade has shown a prospective anti-metastatic potential in breast cancer (62). Mechanically, the pairing of tumoral CXCR3 and its ligands may activate downstream Ras/ERK and PI3K/AKT pathways (63, 64). Thus, the relationship between CXCL9, CXCL10, CXCL11/CXCR3 axis and tumor development or patient prognosis is still controversial. In some cases, consensus is made upon the association between CXCR3 or its ligands expression and negative response to existing treatments (65, 66), while other reports reveal an opposite result (67–69). The theoretical base is still under study. At least, these results encourage us that patients with certain types of malignancies may benefit from radiotherapy-induced NK cells intratumoral infiltration, while not suffer from increased metastasis. Whereas, it also reminds us when combined NK cell-based ACT and radiotherapy in solid tumor treatment, we should detect CXCR3 and its ligands expression in cancer cells, and pathways (as Ras/ERK or PI3K/AKT) inhibitors should be introduced to prevent tumor metastasis in time. According to the interesting work by Liu WS and colleagues (70), strategy which systematically establishes a score utilizing CXCR3 and its ligands abundances in TME to predict tumor metastasis during NK cell-based ACT and radiotherapy combined therapy may be beneficial and promising.
Apart from the essential CXCR3, additional chemokine receptors, including CXCR4, CCR7 and CX3CR, have been reported to promote the recruitment of NK cells to the TME of solid neoplasms (71–74). Importantly, with no pharmaceutical strategies clinically available, radiotherapy seems to be the most cost-effective strategy to drive the expression of these chemokine receptors or their ligands. Specifically, expression of CXCR4 and its ligand CXCL12 were found to be increased after radiotherapy in patients with metastatic prostate cancer (75), rectal cancer (76), or nasopharyngeal carcinoma (77). When exploring tumor-infiltrating lymphocytes (TILs) after radiotherapy in patients with prostate cancer, researchers observed a higher number of CCR7+ TILs in tumor biopsies post-radiotherapy (78). In an ex vivo experiment, single-dose radiation promoted the migration of CX3CR1+ immune cells markedly and upregulated expression of its cognate ligand CX3CL1 (79, 80). In vivo and in vitro data have shown the positive regulation of radiotherapy on expression of CXCR4, CCR7, or CX3CR1, but the fundamental basis has yet to be identified.
However, similar with CXCR3, CXCR4 is not a NK cell-specific chemokine receptor either. CXCR4 expression is also abundant in immunosuppressive cells such as myeloid-derived suppressor cells (MDSCs). A recent study demonstrated that radiotherapy induced infiltration of CXCR4+ MDSCs into the microenvironment of glioblastoma, and led to diminished anti-tumor immunity (81). A similar contradiction can also be found via a mechanism involving CXCL8 secretion. The mammalian target of rapamycin (mTOR)-p65 axis showed sustained activation and was responsible for radiotherapy-induced CXCL8 release, which caused directional migration of NK cells to the TME in patients with pancreatic cancer (82). However, it has also been reported that expression of squamous cell carcinoma antigen 1 (clinical biomarker of a poor response to anticancer therapy) resulted in increased CXCL8 expression along with promotion of intra-tumoral trafficking of MDSCs in response to radiotherapy (83). Thus, combination therapy, such as radiotherapy plus SX-682 (CXCR1/2 inhibitor) (84), could abrogate the recruitment of tumor MDSCs and enhance the tumor infiltration and therapeutic efficacy of NK cells.
The data stated above reveal that trafficking of NK cells into solid tumors can be ameliorated by radiotherapy through driving abundant chemokines receptors expression on NK cells or corresponding ligands secretion by tumor cells into TME that favor NK-cell infiltration.
2.2 Promoting the cytotoxicity of NK cells
The cytotoxicity of NK cells involves secretion of cytotoxic granules containing the pore-forming protein perforin and procaspase-cleaving granzyme B (GzmB), which induces apoptosis in targeted tumor cells (85). Also, NK cells can trigger target-cell apoptosis via the death receptors tumor necrosis factor (TNF)-related apoptosis-inducing ligand (TRAIL) and FAS ligand (86). Unfortunately, no report has shown a positive correlation between radiotherapy and secretion of perforin or GzmB by NK cells. However, numerous works have suggested a link between radiotherapy and expression/secretion of GzmB by cluster of differentiation (CD)8+ T cells (whose major cytotoxic executors are also GzmB and perforin) in models of prostate cancer (87), melanoma (88), or hepatocellular carcinoma (89). An ex vivo study in a model of colorectal cancer revealed that a radiotherapy-induced cGAS signaling pathway may favor GzmB expression by CD8+ T cells (90). A case report elucidated that upregulated expression of perforin in T cells was due to radiotherapy in a patient with metastatic gastric cancer (especially in the disease-resolution period) (91). Even though the synthesis, mobilization, and secretion of GzmB and perforin involve complex signal-transduction pathways, similar mechanisms are observed in T cells and NK cells (92–94). Therefore, it is reasonable to speculate that radiotherapy should be a promising strategy to stimulate secretion of GzmB and perforin in the TME by NK cells for solid tumors. However, direct evidence is needed to define this link.
Besides cytotoxic granules, NK cells also exert antitumor effects through secretion of cytokines such as TRAIL. The latter induces the apoptosis of cancer cells directly by bonding to its death receptors (DR4/DR5) (95). However, almost all tumors are not sensitive to TRAIL, including astrocytoma, chronic lymphocytic leukemia, medulloblastoma, and meningioma (96). The cause of this lack of sensitivity in erythroleukemia was investigated by Di Pietro and colleagues: the low surface expression of the TRAIL receptor DR4 was suggested to be the reason. Furthermore, they concluded that a low (1.5 Gy) or high (15 Gy) single dose of radiation could sensitize erythroleukemic cells to TRAIL mediated-cytotoxicity by selective upregulation of TRAIL-R1 (DR4) expression (97). A similar result was also observed in a mouse model of TRAIL-R2 (DR5) deficiency designed by Niklas and colleagues, whose study put radiotherapy “center stage” in TRAIL-induced organ-specific damage (98). Hence, we wonder whether radiotherapy could increase the response to TRAIL-induced apoptosis in other tumors and, ultimately, boost the cytotoxicity of NK cells. Apart from increasing expression of the death receptors of TRAIL, radiotherapy (a genotoxic agent) may sensitize cancer cells to TRAIL by activating diverse TNF-associated apoptotic pathways in tumor cells, such as caspase-8, p38, or nuclear factor-kappa B (99–101).
The degree to which NK cells are active against tumor cells is based on the activation state of NK cells, which is regulated in large part by cytokines (e.g., IL-12, IL-18, type-I IFN) derived from other immune cells, including dendritic cells (DCs) and monocytes (102–104). Interestingly, preclinical studies have been conducted over decades combining DCs-based immunotherapy with radiotherapy, and clinical benefit (at least a partial response) was exhibited when compared with administration of DCs alone. In a mouse-bearing sarcoma model, radiotherapy plus DCs treatment resulted in favorable infiltration of DCs into the TME to aid tumor eradication (105). However, in an ex vivo co-culture experiment, a significant difference in apoptosis or necrosis between DCs-administration alone and DCs plus radiotherapy was not detected in cancer cells. Explanation was not given in that study, but we hypothesize that radiotherapy promoted the antitumor effect of DCs via crosstalk between DCs and other immune-active cells (e.g., NK cells, CD8+ T cells) rather that DCs. Another study using models of pancreatic carcinoma and colorectal carcinoma showed that radiotherapy facilitated DCs maturation, followed by enhanced production of type-I IFN, and contributed to the activation of CD8+ T cells (106). Undoubtedly, NK cells could be activated simultaneously by type-I IFN also. To ascertain how radiotherapy activates DCs, researchers suggested that the cGAS-stimulator of interferon genes (STING; an endoplasmic reticulum-associated protein) axis was indispensable in radiation-induced production of type-I IFN by DCs. DNA fragments from irradiated cancer cells gained access to a cytosolic DNA-sensing pathway in DCs via direct cell–cell contact to trigger induction of STING-dependent type-I IFN, which further drove the adaptive immune response to radiation (107). The cGAS-STING pathway plays an essential part in expression of the NK-cell trafficking-associated ligand CXCL10 by irradiated tumor cells but also in the production of type-I IFN in DCs. Further studies may reveal more information about the cGAS-STING axis in radiotherapy-mediated activation of NK cells.
Activation of NK cells is also reliant on tumor-associated antigens (TAAs) expressed by cancer cells. TAAs are antigenic proteins produced in tumor cells, which can trigger an autoantibody response in patients (108). Numerous studies have reported that TAAs markedly influence the immunosurveillance of NK cells for aberrant cells or tumor cells, and partly determine the clinical benefits of adaptively transferred NK cells (109–112). The TAAs, New York esophageal squamous cell carcinoma-1 (NY-ESO-1), CD20, and CD48 are the most reported in terms of NK-cell stimulation. In recent years, radiotherapy-mediated genomic alteration of TAAs has attracted attention. In a single-arm feasibility study, patients with metastatic renal cell carcinoma were treated with stereotactic body radiotherapy (SBRT; 15 Gy) at the primary lesion in a single fraction, and specimens were analyzed for TAA expression at 4 weeks (113). Radiotherapy-treated tumors had enhanced expression of several TAAs, including carbonic anhydrase IX (CA9), trophoblast glycoprotein (TPBG), NY-ESO-1, and mucin-1. Interestingly, NY-ESO-1 seems to be the only intersection between radiotherapy-induced change in TAAs and NK cell stimulation-associated TAAs. Inspiringly, in a patient diagnosed with locally advanced unresectable gastric cancer, radiotherapy led to upregulation of NY-ESO-1 expression in the tumor, and enhanced the anticancer efficacy of anti-programmed cell death protein 1-based immunotherapy that correlated with a beneficial clinical outcome (91). Direct evidence identifying a pronounced relationship between radiotherapy, TAAs (e.g., NY-ESO-1), and NK-cell stimulation is lacking, but the above case report by Merhi M and colleagues suggests that radiotherapy may activate the NK-cell response to malignancies through upregulating expression of NY-ESO-1 or other TAAs.
Several metabolic features in the TME have been found to exert a considerable negative impact upon NK-cell activation. The rapid and uncontrolled proliferation of tumors leads to an insufficient blood supply and limits oxygen availability, so hypoxia is the most prominent metabolism-associated TME feature in nearly all solid tumors (114). Studies have reported that under hypoxia, there is a modulation of the genes associated with immunomodulatory effects and reduction in the number of activating receptors (e.g., NKG2D, NKp46), which result in impaired effector functions in NK cells (115–117). Hypoxia-inducible factor (HIF)-1α is considered to be responsible for this genetic regulation (though the specific pathway has yet to be uncovered). Reversing hypoxia is a promising method to reactivate NK cells, so radiotherapy has attracted attention. As one of the “4R” principles post-irradiation, reoxygenation occurs intratumorally in the early phase of radiotherapy. In 20 patients with head and neck cancer who received intensity-modulated radiotherapy (70 Gy/35 fractions), the intensity and volume of tumor hypoxia declined rapidly, and 18F-fluorodeoxyglucose-positron emission tomography suggested gradually decreased fluorodeoxyglucose uptake during intensity-modulated radiotherapy, which indicated a substantial tumoricidal effect over the entire treatment (118). Notably, a recent study demonstrated radiotherapy-induced tumoral reoxygenation to be dose-dependent. In six patients with lung cancer undergoing SBRT (18 Gy/fraction), increased (or at least persistent) tumor hypoxia was observed (119). In a prospective study of lung cancer, SBRT (12-13 Gy/fraction) caused a considerable reduction in tumor hypoxia and a favorable prognosis (120). Thus, at least in lung cancer, a high dose of radiation (18 Gy/fraction) should be the upper limit for radiotherapy-induced reoxygenation. Besides, tumor specificity may also be taken into consideration because a high dose (20 Gy) in patients with prostate cancer led to a reduced hypoxic volume in nearly all patients (121). In summary, using radiotherapy to eliminate hypoxia in solid tumors and thereby improve NK-cell cytotoxicity is a feasible and promising strategy. However, the radiation dose and tumor specificity must be evaluated individually. In addition, whether HIF-1α has a vital role in this regulation is not known.
The evidence stated above suggests that fractionated radiotherapy is a promising strategy for the recruitment and cytotoxic function of NK cells in solid tumors because it can eliminate inhibitory signals and augment enhancement signals simultaneously (Figure 1).
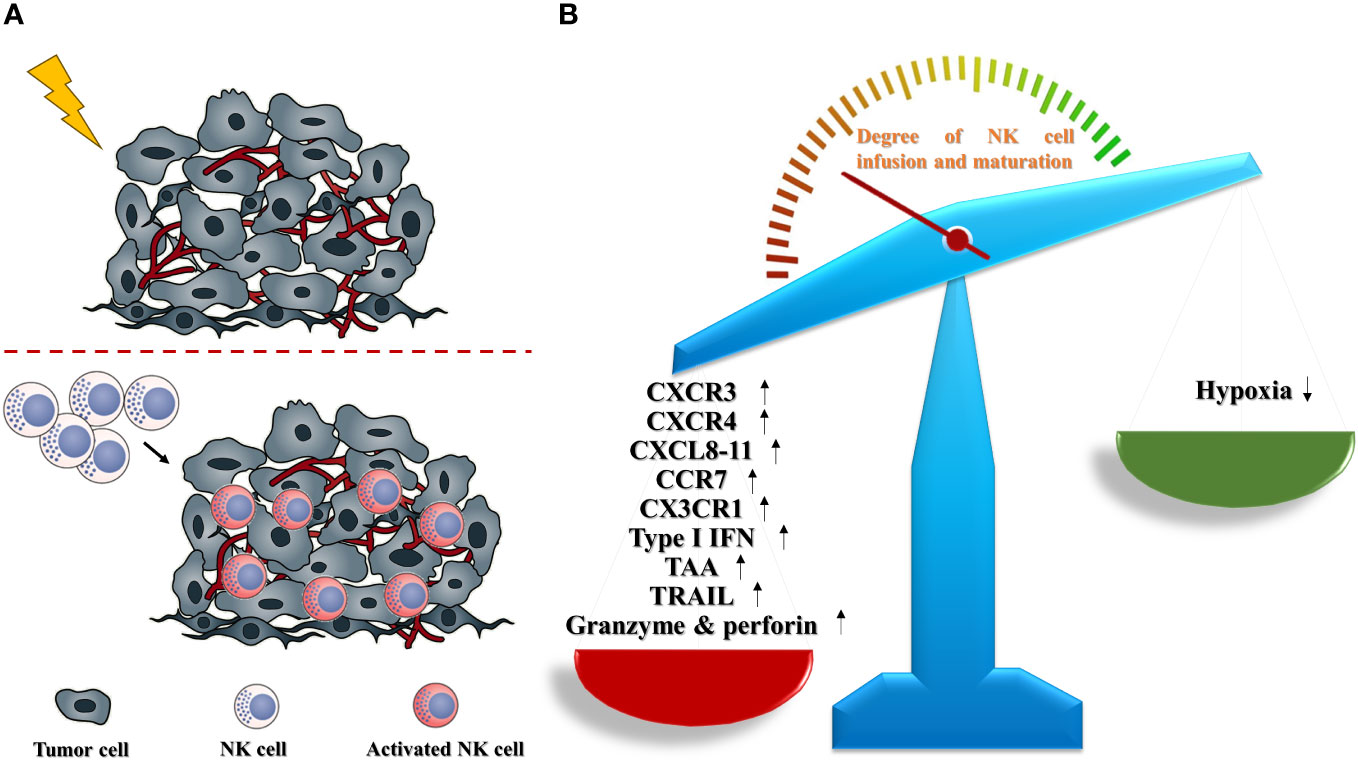
Figure 1 Radiotherapy promotes the infiltration and activation of NK cells in solid tumors (schematic). (A) In solid tumors, radiotherapy contributes to a boost trafficking of NK cells into TME, and accelerates the functional maturation of infused NK cells. (B) Radiotherapy could remodel the TME to make a NK-favored microenvironment via upregulating NK-promoted parameters including CXCR3, CXCR4, CXCL8-11, CCR7, CX3CR1, type I IFN, TAA, TRAIL, granzyme and perforin, as well as abating NK-suppressed obstacle, especially hypoxia.
3 NK cells amplify radiotherapy-induced anticancer damage
Radiotherapy has been applied against almost all types of solid tumors for decades. The efficacy of radiotherapy in curing some malignancies can be suboptimal. The radioresistance of these tumors can be the reason for this poor response to radiotherapy. Various signaling pathways, such as DNA damage response (122, 123) and autophagic apoptosis (124, 125) are involved in the radioresistance of tumors. Most radiosensitizers targeting these mechanisms have been under preclinical study for years, but few have been approved for clinical use. During mechanistic studies, researchers observed a link between microenvironmental NK cells and tumor radioresistance. In general, NK cells can improve the tumor response to radiotherapy via two pathways: (i) cytokines (or cytotoxic granules) secretion (2); molecular signal transduction.
3.1 Secretion of cytokines or cytotoxic granules enhance tumor radiosensitivity
Stimulated NK cells can produce cytotoxic granules (e.g., perforin, granzymes) as well as multiple cytokines (e.g., IFN-γ, TNF-α, IL-13) (14, 126). NK cells kill tumor cells directly or increase the immunosurveillance of other immune cells (e.g., CD8+ T cells) indirectly via secretion of these effectors, but biological processes in cancer cells (e.g., ferroptosis, autophagy, repair of DNA damage) may also be influenced by them, which will influence the radioresistance of tumors.
Among NK cell-derived cytokines, IFN-γ is the most fundamental factor involved in the crosstalk of NK cells with almost all immune-active cells, such as CD8+ T cells, helper CD4+ T cells, antigen-presenting cells, and macrophages (127). The effects of IFN-γ on various biological processes related to radiosensitization in tumor cells are beginning to be identified. In a model of prostate cancer in mice, NK cells secreting IFN-γ increased ferroptosis in cancer cells which, in turn, enhanced NK-cell function (including IFN-γ production) (128). Peng and colleagues suggested IFN-γ signaling to be a natural ferroptosis-promoting mechanism in tumors involving the metabolism of C16 and C18 acyl chain-containing phospholipids (129). Both of those works did not study the continuous impact on the radioresistance of tumors, but the role of enhanced ferroptosis in sensitizing various types of neoplasm to radiation is accepted. Numerous preclinical studies have disclosed a relatively low level of ferroptosis in malignancies with a weak response to radiotherapy, and that pharmaceutical induction of ferroptosis resensitized these tumors to radiation significantly (130–132). Hence, by triggering ferroptosis, IFN-γ should overcome the radioresistance of tumors and enhance radiation-induced damage. Rao and colleagues suggested that all-trans retinoic acid can overwhelm the radioresistance of solid tumors by inducing inflammatory macrophage-derived IFN-γ (133). Hence, apart from ferroptosis or fatty-acid metabolism, more pathways may participate in the IFN-γ-mediated radiosensitization of solid tumors.
TNF-α is synthesized and secreted mainly by NK cells. In common with other TNF family members, TNF-α is involved in the maintenance and homeostasis of the immune system, inflammation, and defense against malignancy (134). Apart from these immunosurveillance functions, a unique influence of TNF-α upon radiosensitization in solid tumors was reported decades earlier. In a model of prostate cancer, when cells were irradiated 24 h after exposure to TNF-α, increased cell death was observed. In contrast, radiation delivered 24 h before TNF-α exposure did not lead to more cell death than after TNF-α alone (135). This finding suggested that TNF-α sensitized tumors to radiotherapy. A similar result was observed in a model of B-cell lymphoma treated with radiation plus ceramide inhibition (136). Kimura and colleagues believed that TNF-α sensitized tumors to radiation by increasing intracellular ceramide production.
IL-13 is a pleiotropic immunoregulatory cytokine produced by Th2 cells, NK cells, or other cells of the innate immune system (137). In an ex vivo experiment, isorhamnetin treatment increased the radiosensitivity of non-small-cell lung cancer cells, and genetic knockdown of IL-13 expression eliminated isorhamnetin-mediated radiosensitivity in cells (138). This result reflected a role of IL-13 in overcoming resistance to radiotherapy in solid tumors. In another study, “protumor” M2-polarized macrophages in the microenvironment of breast cancer were augmented after IL-13 administration, which further mediated tumor radioresistance; however, inhibition of IL-13-mediated M2 polarization of macrophages by PM37 could prevent radioresistance (139). This contradictory IL-13-mediated removal or maintenance of radioresistance may be derived from the difference between in vitro and in vivo experimental models. In a simple model of cancer cells, IL-13-related pathways can sensitize cells to radiation without consideration of the effects on other types of immune cell but, in tumor-bearing mouse model or humans, this effect is inevitable. Accordingly, if taking advantage of IL-13 derived from mature NK cells for reducing tumor radioresistance, the ability of IL-13 to promote groups of protumor immune cells must be considered.
One mechanism of NK cell-mediated tumor killing involves granzymes that fragment nuclear DNA and leads to cell death (85). The biologic/pathologic functions of most granzymes are not known, but GzmB contributes to DNA fragmentation and results in cell death directly. However, studies on GzmB-induced radiosensitization in lung cancer and prostate cancer, respectively, are interesting. Researchers found that administration of resveratrol or AuNPs-si-SP1 could increase radiosensitivity markedly by upregulating the expression and synthesis of GzmB in cancer cells (140, 141). GzmB secreted by NK cells or other immune cells and GzmB synthesized by tumor cells are not identical. Those two studies were the first to focus on the regulatory role of GzmB on tumor radioresistance rather than its direct apoptosis-inducing ability, which expand our knowledge on the biological functions of GzmB.
3.2 Molecules from NK cell-derived exosomes activate radiosensitization pathways
Exosomes are nanovesicles secreted actively by almost all cell types. Exosomes deliver certain intracellular molecules (e.g., nucleic acids, proteins, lipids) to target cells (142). Studies have focused mainly on exosomes released from cancer cells, but the functions and characteristics of exosomes derived from NK cells have been explored in recent years. Reports have suggested that NK cell-derived exosomes could be assimilated by tumors in a time-dependent manner, and such uptake improved the response to anti-cancer treatments such as cisplatin in melanoma and ovarian carcinoma (143, 144). The mechanisms by which NK cell-released exosomes exert this sensitization function have not been elucidated. However, small RNA-sequencing of exosomes secreted by NK cells identified a specific repertoire of NK exosome-associated microRNAs (mIRs) (145). Interestingly, miR-10b-5p (146), miR-92a-3p (147), miR-146a-5p (148), and miR-99a-5p (149) specifically abated tumor radioresistance involving multiple molecular pathways such as ATM/ATR serine/threonine kinase, Janus Kinase, and protein kinase B/mTOR. Apparently, miRNA transduction into tumor cells by NK-derived exosomes may increase the radiosensitivity of multiple malignancy types.
In summary, the data stated above suggest an indirect sensitization role rather than a direct death-inducing function of NK cell-derived cytotoxic granules, cytokines or exosomes in anti-tumoral radiotherapy (Figure 2).
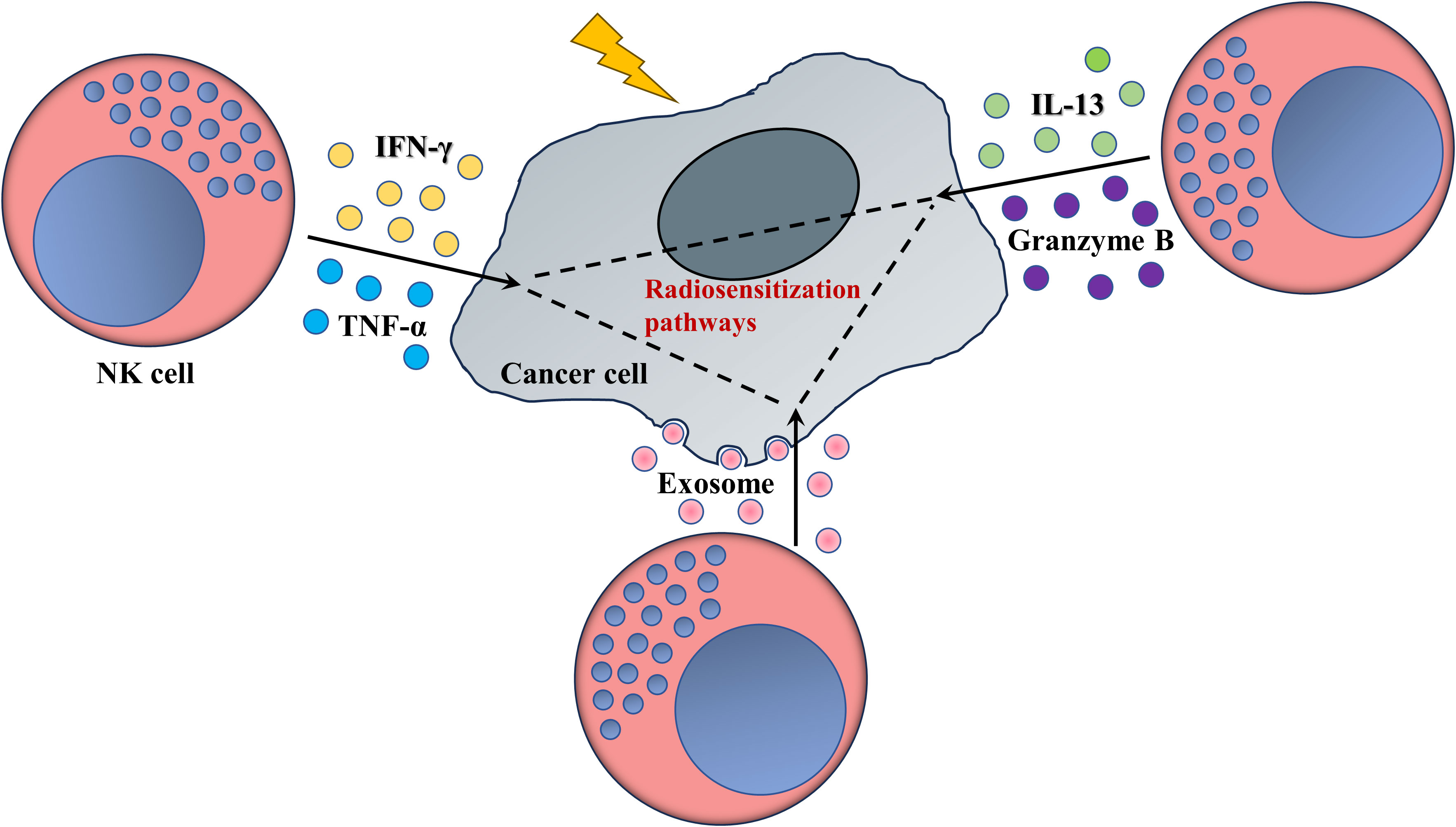
Figure 2 NK cells enhance the response of tumors to radiotherapy (schematic). Mature NK cells secrete various cytokines or cytotoxic granules, as well as plenty of exosomes, whose interactions with radiosensitivity-related pathways or biological process, as ferroptosis, fatty acid metabolism or miRNAs networks, access valid ability in pruning radioresistance of cancer cells.
4 Concluding remarks and future perspectives
NK cells have been suggested to be candidates for anti-tumor therapy in recent years, and inspiring data have been documented in individuals with hematologic neoplasms. Nevertheless, application of NK cells in the treatment of solid malignancies is hampered by many obstacles (150, 151). Despite strategies aimed at promoting the infiltration and activation of NK cells in the TME, translation into clinical practice is difficult. In contrast, priming the TME with radiotherapy could “jumpstart” the immune activity of NK cells by providing a permissive microenvironment for them. Moreover, mature NK cells have been reported to improve the response of solid tumors to radiotherapy. This complementary function between radiotherapy and NK cells likely results in a synergistic effect on tumor eradication, which is why a combination of radiotherapy and NK-based ACT is crucial against cancer. This strategy should be valid but direct evidence to prove its efficacy and safety is lacking. We expect more clinical trials focusing on radiotherapy and NK-based ACT but the histology subtype, radiotherapy dose/sequence, and interference by other immune cells in the TME must be taken into consideration.
In a whole, radiotherapy could be one of the keys to unlock the potential of therapy based on the transfer of NK cells against cancer, and conversely, infusion and maturation of NK cells may resolve the long-standing radioresistance in solid tumors.
Author contributions
WZ: Writing – original draft. SL: Investigation, Writing – review & editing. YC: Data curation, Writing – review & editing. CS: Writing – review & editing. XS: Writing – review & editing.
Funding
The author(s) declare financial support was received for the research, authorship, and/or publication of this article. This work was supported by the Jiangsu Provincial Outstanding Postdoctoral Program (no. 2023ZB564).
Conflict of interest
The authors declare that the research was conducted in the absence of any commercial or financial relationships that could be construed as a potential conflict of interest.
Publisher’s note
All claims expressed in this article are solely those of the authors and do not necessarily represent those of their affiliated organizations, or those of the publisher, the editors and the reviewers. Any product that may be evaluated in this article, or claim that may be made by its manufacturer, is not guaranteed or endorsed by the publisher.
Abbreviations
ACT, adoptive cell therapy; CC, C-C chemokine; CD, cluster of differentiation; cGAS, cyclic guanosine monophosphate-adenosine monophosphate synthase; CXC, C-X-C motif chemokine; CX3C, C-X3-C chemokine; DC, dendritic cell; DR, death receptor; Gy, Gray; GzmB, granzyme B; HIF, hypoxia-inducible factor; IFN-γ, interferon-γ; IL, interleukin; MDSC, myeloid-derived suppressor cell; miR, microRNA; M2, alternatively activated macrophage; NK, natural killer; NY-ESO-1, New York esophageal squamous cell carcinoma-1; SBRT, stereotactic body radiotherapy; STING, stimulator of interferon gene; TAA, tumor-associated antigen; TIL, tumor-infiltrating lymphocyte; TME, tumor microenvironment; TNF, tumor necrosis factor; TRAIL, TNF-related apoptosis-inducing ligand.
References
1. Rosenberg SA, Restifo NP. Adoptive cell transfer as personalized immunotherapy for human cancer. Science (2015) 348:62–8. doi: 10.1126/science.aaa4967
2. Rosenberg SA, Packard BS, Aebersold PM, Solomon D, Topalian SL, Toy ST, et al. Use of tumor-infiltrating lymphocytes and interleukin-2 in the immunotherapy of patients with metastatic melanoma. A preliminary report. N Engl J Med (1988) 319:1676–80. doi: 10.1056/NEJM198812223192527
3. Maude SL, Laetsch TW, Buechner J, Rives S, Boyer M, Bittencourt H, et al. Tisagenlecleucel in children and young adults with B-cell lymphoblastic leukemia. N Engl J Med (2018) 378:439–48. doi: 10.1056/NEJMoa1709866
4. Gardner RA, Finney O, Annesley C, Brakke H, Summers C, Leger K, et al. Intent-to-treat leukemia remission by CD19 CAR T cells of defined formulation and dose in children and young adults. Blood (2017) 129:3322–31. doi: 10.1182/blood-2017-02-769208
5. Lee DW, Kochenderfer JN, Stetler-Stevenson M, Cui YK, Delbrook C, Feldman SA, et al. T cells expressing CD19 chimeric antigen receptors for acute lymphoblastic leukaemia in children and young adults: a phase 1 dose-escalation trial. Lancet (2015) 385:517–28. doi: 10.1016/S0140-6736(14)61403-3
6. Park JH, Riviere I, Gonen M, Wang X, Senechal B, Curran KJ, et al. Long-term follow-up of CD19 CAR therapy in acute lymphoblastic leukemia. N Engl J Med (2018) 378:449–59. doi: 10.1056/NEJMoa1709919
7. Gardner R, Wu D, Cherian S, Fang M, Hanafi LA, Finney O, et al. Acquisition of a CD19-negative myeloid phenotype allows immune escape of MLL-rearranged B-ALL from CD19 CAR-T-cell therapy. Blood (2016) 127:2406–10. doi: 10.1182/blood-2015-08-665547
8. Fry TJ, Shah NN, Orentas RJ, Stetler-Stevenson M, Yuan CM, Ramakrishna S, et al. CD22-targeted CAR T cells induce remission in B-ALL that is naive or resistant to CD19-targeted CAR immunotherapy. Nat Med (2018) 24:20–8. doi: 10.1038/nm.4441
9. Jacoby E, Nguyen SM, Fountaine TJ, Welp K, Gryder B, Qin H, et al. CD19 CAR immune pressure induces B-precursor acute lymphoblastic leukaemia lineage switch exposing inherent leukaemic plasticity. Nat Commun (2016) 7:12320. doi: 10.1038/ncomms12320
10. Mueller KT, Maude SL, Porter DL, Frey N, Wood P, Han X, et al. Cellular kinetics of CTL019 in relapsed/refractory B-cell acute lymphoblastic leukemia and chronic lymphocytic leukemia. Blood (2017) 130:2317–25. doi: 10.1182/blood-2017-06-786129
11. Chiossone L, Dumas PY, Vienne M, Vivier E. Natural killer cells and other innate lymphoid cells in cancer. Nat Rev Immunol (2018) 18:671–88. doi: 10.1038/s41577-018-0061-z
12. Luetke-Eversloh M, Cicek BB, Siracusa F, Thom JT, Hamann A, Frischbutter S, et al. NK cells gain higher IFN-gamma competence during terminal differentiation. Eur J Immunol (2014) 44:2074–84. doi: 10.1002/eji.201344072
13. Vivier E, Raulet DH, Moretta A, Caligiuri MA, Zitvogel L, Lanier LL, et al. Innate or adaptive immunity? The example of natural killer cells. Science (2011) 331:44–9. doi: 10.1126/science.1198687
14. Guillerey C, Huntington ND, Smyth MJ. Targeting natural killer cells in cancer immunotherapy. Nat Immunol (2016) 17:1025–36. doi: 10.1038/ni.3518
15. Vivier E, Ugolini S, Blaise D, Chabannon C, Brossay L. Targeting natural killer cells and natural killer T cells in cancer. Nat Rev Immunol (2012) 12:239–52. doi: 10.1038/nri3174
16. Miller JS, Soignier Y, Panoskaltsis-Mortari A, McNearney SA, Yun GH, Fautsch SK, et al. Successful adoptive transfer and in vivo expansion of human haploidentical NK cells in patients with cancer. Blood (2005) 105:3051–57. doi: 10.1182/blood-2004-07-2974
17. Bachanova V, Cooley S, Defor TE, Verneris MR, Zhang B, McKenna DH, et al. Clearance of acute myeloid leukemia by haploidentical natural killer cells is improved using IL-2 diphtheria toxin fusion protein. Blood (2014) 123:3855–63. doi: 10.1182/blood-2013-10-532531
18. Romee R, Rosario M, Berrien-Elliott MM, Wagner JA, Jewell BA, Schappe T, et al. Cytokine-induced memory-like natural killer cells exhibit enhanced responses against myeloid leukemia. Sci Transl Med (2016) 8:357ra123. doi: 10.1126/scitranslmed.aaf2341
19. Ruggeri L, Capanni M, Urbani E, Perruccio K, Shlomchik WD, Tosti A, et al. Effectiveness of donor natural killer cell alloreactivity in mismatched hematopoietic transplants. Science (2002) 295:2097–100. doi: 10.1126/science.1068440
20. Fang F, Xiao W, Tian Z. NK cell-based immunotherapy for cancer. Semin Immunol (2017) 31:37–54. doi: 10.1016/j.smim.2017.07.009
21. Yilmaz A, Cui H, Caligiuri MA, Yu J. Chimeric antigen receptor-engineered natural killer cells for cancer immunotherapy. J Hematol Oncol (2020) 13:168. doi: 10.1186/s13045-020-00998-9
22. Tong L, Jimenez-Cortegana C, Tay A, Wickstrom S, Galluzzi L, Lundqvist A. NK cells and solid tumors: therapeutic potential and persisting obstacles. Mol Cancer (2022) 21:206. doi: 10.1186/s12943-022-01672-z
23. Federico SM, McCarville MB, Shulkin BL, Sondel PM, Hank JA, Hutson P, et al. A pilot trial of humanized anti-GD2 monoclonal antibody (hu14.18K322A) with chemotherapy and natural killer cells in children with recurrent/refractory neuroblastoma. Clin Cancer Res (2017) 23:6441–49. doi: 10.1158/1078-0432.CCR-17-0379
24. Modak S, Le Luduec JB, Cheung IY, Goldman DA, Ostrovnaya I, Doubrovina E, et al. Adoptive immunotherapy with haploidentical natural killer cells and Anti-GD2 monoclonal antibody m3F8 for resistant neuroblastoma: Results of a phase I study. Oncoimmunology (2018) 7:e1461305. doi: 10.1080/2162402X.2018.1461305
25. Pitt JM, Marabelle A, Eggermont A, Soria JC, Kroemer G, Zitvogel L. Targeting the tumor microenvironment: removing obstruction to anticancer immune responses and immunotherapy. Ann Oncol (2016) 27:1482–92. doi: 10.1093/annonc/mdw168
26. Zhang S, Liu W, Hu B, Wang P, Lv X, Chen S, et al. Prognostic significance of tumor-infiltrating natural killer cells in solid tumors: A systematic review and meta-analysis. Front Immunol (2020) 11:1242. doi: 10.3389/fimmu.2020.01242
27. Wennerberg E, Kremer V, Childs R, Lundqvist A. CXCL10-induced migration of adoptively transferred human natural killer cells toward solid tumors causes regression of tumor growth in vivo. Cancer Immunol Immunother (2015) 64:225–35. doi: 10.1007/s00262-014-1629-5
28. Yamamoto Y, Miyazato K, Takahashi K, Yoshimura N, Tahara H, Hayakawa Y. Lung-resident natural killer cells control pulmonary tumor growth in mice. Cancer Sci (2018) 109:2670–76. doi: 10.1111/cas.13703
29. Wendel M, Galani IE, Suri-Payer E, Cerwenka A. Natural killer cell accumulation in tumors is dependent on IFN-gamma and CXCR3 ligands. Cancer Res (2008) 68:8437–45. doi: 10.1158/0008-5472.CAN-08-1440
30. Tripp CS, Wolf SF, Unanue ER. Interleukin 12 and tumor necrosis factor alpha are costimulators of interferon gamma production by natural killer cells in severe combined immunodeficiency mice with listeriosis, and interleukin 10 is a physiologic antagonist. Proc Natl Acad Sci U.S.A. (1993) 90:3725–29. doi: 10.1073/pnas.90.8.3725
31. Garces-Lazaro I, Kotzur R, Cerwenka A, Mandelboim O. NK cells under hypoxia: the two faces of vascularization in tumor and pregnancy. Front Immunol (2022) 13:924775. doi: 10.3389/fimmu.2022.924775
32. Muri J, Kopf M. Redox regulation of immunometabolism. Nat Rev Immunol (2021) 21:363–81. doi: 10.1038/s41577-020-00478-8
33. Meazza R, Azzarone B, Orengo AM, Ferrini S. Role of common-gamma chain cytokines in NK cell development and function: perspectives for immunotherapy. J BioMed Biotechnol (2011) 2011:861920. doi: 10.1155/2011/861920
34. Cichocki F, Valamehr B, Bjordahl R, Zhang B, Rezner B, Rogers P, et al. GSK3 inhibition drives maturation of NK cells and enhances their antitumor activity. Cancer Res (2017) 77:5664–75. doi: 10.1158/0008-5472.CAN-17-0799
35. Bryceson YT, March ME, Ljunggren HG, Long EO. Synergy among receptors on resting NK cells for the activation of natural cytotoxicity and cytokine secretion. Blood (2006) 107:159–66. doi: 10.1182/blood-2005-04-1351
36. Rosario M, Liu B, Kong L, Collins LI, Schneider SE, Chen X, et al. The IL-15-based ALT-803 complex enhances fcgammaRIIIa-triggered NK cell responses and in vivo clearance of B cell lymphomas. Clin Cancer Res (2016) 22:596–608. doi: 10.1158/1078-0432.CCR-15-1419
37. Formenti SC, Demaria S. Combining radiotherapy and cancer immunotherapy: a paradigm shift. J Natl Cancer Inst (2013) 105:256–65. doi: 10.1093/jnci/djs629
38. Lugade AA, Moran JP, Gerber SA, Rose RC, Frelinger JG, Lord EM. Local radiation therapy of B16 melanoma tumors increases the generation of tumor antigen-specific effector cells that traffic to the tumor. J Immunol (2005) 174:7516–23. doi: 10.4049/jimmunol.174.12.7516
39. Reits EA, Hodge JW, Herberts CA, Groothuis TA, Chakraborty M, Wansley EK, et al. Radiation modulates the peptide repertoire, enhances MHC class I expression, and induces successful antitumor immunotherapy. J Exp Med (2006) 203:1259–71. doi: 10.1084/jem.20052494
40. Matsumura S, Wang B, Kawashima N, Braunstein S, Badura M, Cameron TO, et al. Radiation-induced CXCL16 release by breast cancer cells attracts effector T cells. J Immunol (2008) 181:3099–107. doi: 10.4049/jimmunol.181.5.3099
41. Wang L, Jiang J, Chen Y, Jia Q, Chu Q. The roles of CC chemokines in response to radiation. Radiat Oncol (2022) 17:63. doi: 10.1186/s13014-022-02038-x
42. Formenti SC, Demaria S. Radiation therapy to convert the tumor into an in situ vaccine. Int J Radiat Oncol Biol Phys (2012) 84:879–80. doi: 10.1016/j.ijrobp.2012.06.020
43. Hintelmann K, Kriegs M, Rothkamm K, Rieckmann T. Improving the efficacy of tumor radiosensitization through combined molecular targeting. Front Oncol (2020) 10:1260. doi: 10.3389/fonc.2020.01260
44. Wang J, Matosevic S. Functional and metabolic targeting of natural killer cells to solid tumors. Cell Oncol (Dordr) (2020) 43:577–600. doi: 10.1007/s13402-020-00523-7
45. Weil S, Memmer S, Lechner A, Huppert V, Giannattasio A, Becker T, et al. Natural killer group 2D ligand depletion reconstitutes natural killer cell immunosurveillance of head and neck squamous cell carcinoma. Front Immunol (2017) 8:387. doi: 10.3389/fimmu.2017.00387
46. Fisher B, Packard BS, Read EJ, Carrasquillo JA, Carter CS, Topalian SL, et al. Tumor localization of adoptively transferred indium-111 labeled tumor infiltrating lymphocytes in patients with metastatic melanoma. J Clin Oncol (1989) 7:250–61. doi: 10.1200/JCO.1989.7.2.250
47. Sokol CL, Luster AD. The chemokine system in innate immunity. Cold Spring Harb Perspect Biol (2015) 7:a016303. doi: 10.1101/cshperspect.a016303
48. Olson TS, Ley K. Chemokines and chemokine receptors in leukocyte trafficking. Am J Physiol Regul Integr Comp Physiol (2002) 283:R7–28. doi: 10.1152/ajpregu.00738.2001
49. Yao X, Matosevic S. Chemokine networks modulating natural killer cell trafficking to solid tumors. Cytokine Growth Factor Rev (2021) 59:36–45. doi: 10.1016/j.cytogfr.2020.12.003
50. Hayakawa Y, Smyth MJ. CD27 dissects mature NK cells into two subsets with distinct responsiveness and migratory capacity. J Immunol (2006) 176:1517–24. doi: 10.4049/jimmunol.176.3.1517
51. Kim J, Kim JS, Lee HK, Kim HS, Park EJ, Choi JE, et al. CXCR3-deficient natural killer cells fail to migrate to B16F10 melanoma cells. Int Immunopharmacol (2018) 63:66–73. doi: 10.1016/j.intimp.2018.07.026
52. Rezaeifard S, Talei A, Shariat M, Erfani N. Tumor infiltrating NK cell (TINK) subsets and functional molecules in patients with breast cancer. Mol Immunol (2021) 136:161–67. doi: 10.1016/j.molimm.2021.03.003
53. Fitzgerald AA, Wang S, Agarwal V, Marcisak EF, Zuo A, Jablonski SA, et al. DPP inhibition alters the CXCR3 axis and enhances NK and CD8+ T cell infiltration to improve anti-PD1 efficacy in murine models of pancreatic ductal adenocarcinoma. J Immunother Cancer (2021) 9:e002837. doi: 10.1136/jitc-2021-002837
54. Chibaya L, Murphy KC, DeMarco KD, Gopalan S, Liu H, Parikh CN, et al. EZH2 inhibition remodels the inflammatory senescence-associated secretory phenotype to potentiate pancreatic cancer immune surveillance. Nat Cancer (2023) 4:872–92. doi: 10.1038/s43018-023-00553-8
55. Dillon MT, Bergerhoff KF, Pedersen M, Whittock H, Crespo-Rodriguez E, Patin EC, et al. ATR inhibition potentiates the radiation-induced inflammatory tumor microenvironment. Clin Cancer Res (2019) 25:3392–403. doi: 10.1158/1078-0432.CCR-18-1821
56. Zheng Y, Liu Y, Zhang F, Su C, Chen X, Zhang M, et al. Radiation combined with KRAS-MEK inhibitors enhances anticancer immunity in KRAS-mutated tumor models. Transl Res (2023) 252:79–90. doi: 10.1016/j.trsl.2022.08.005
57. Yamazaki T, Kirchmair A, Sato A, Buque A, Rybstein M, Petroni G, et al. Mitochondrial DNA drives abscopal responses to radiation that are inhibited by autophagy. Nat Immunol (2020) 21:1160–71. doi: 10.1038/s41590-020-0751-0
58. Seo YN, Baik JS, Lee SM, Lee JE, Ahn HR, Lim MS, et al. Ionizing Radiation Selectively Increases CXC Ligand 10 Level via the DNA-Damage-Induced p38 MAPK-STAT1 Pathway in Murine J774A.1 Macrophages. Cells (2023) 12:1009. doi: 10.3390/cells12071009
59. Liang T, Chen J, Xu G, Zhang Z, Xue J, Zeng H, et al. STAT1 and CXCL10 involve in M1 macrophage polarization that may affect osteolysis and bone remodeling in extrapulmonary tuberculosis. Gene (2022) 809:146040. doi: 10.1016/j.gene.2021.146040
60. Kawada K, Hosogi H, Sonoshita M, Sakashita H, Manabe T, Shimahara Y, et al. Chemokine receptor CXCR3 promotes colon cancer metastasis to lymph nodes. Oncogene (2007) 26:4679–88. doi: 10.1038/sj.onc.1210267
61. Kawada K, Sonoshita M, Sakashita H, Takabayashi A, Yamaoka Y, Manabe T, et al. Pivotal role of CXCR3 in melanoma cell metastasis to lymph nodes. Cancer Res (2004) 64:4010–17. doi: 10.1158/0008-5472.CAN-03-1757
62. Walser TC, Rifat S, Ma X, Kundu N, Ward C, Goloubeva O, et al. Antagonism of CXCR3 inhibits lung metastasis in a murine model of metastatic breast cancer. Cancer Res (2006) 66:7701–07. doi: 10.1158/0008-5472.CAN-06-0709
63. Liu M, Guo S, Stiles JK. The emerging role of CXCL10 in cancer (Review). Oncol Lett (2011) 2:583–89. doi: 10.3892/ol.2011.300
64. Khan P, Fatima M, Khan MA, Batra SK, Nasser MW. Emerging role of chemokines in small cell lung cancer: Road signs for metastasis, heterogeneity, and immune response. Semin Cancer Biol (2022) 87:117–26. doi: 10.1016/j.semcancer.2022.11.005
65. Wightman SC, Uppal A, Pitroda SP, Ganai S, Burnette B, Stack M, et al. Oncogenic CXCL10 signalling drives metastasis development and poor clinical outcome. Br J Cancer (2015) 113:327–35. doi: 10.1038/bjc.2015.193
66. Mir MA, Maurer MJ, Ziesmer SC, Slager SL, Habermann T, Macon WR, et al. Elevated serum levels of IL-2R, IL-1RA, and CXCL9 are associated with a poor prognosis in follicular lymphoma. Blood (2015) 125:992–98. doi: 10.1182/blood-2014-06-583369
67. Wu Z, Huang X, Han X, Li Z, Zhu Q, Yan J, et al. The chemokine CXCL9 expression is associated with better prognosis for colorectal carcinoma patients. BioMed Pharmacother (2016) 78:8–13. doi: 10.1016/j.biopha.2015.12.021
68. Sato Y, Motoyama S, Nanjo H, Wakita A, Yoshino K, Sasaki T, et al. CXCL10 expression status is prognostic in patients with advanced thoracic esophageal squamous cell carcinoma. Ann Surg Oncol (2016) 23:936–42. doi: 10.1245/s10434-015-4909-1
69. Walser TC, Ma X, Kundu N, Dorsey R, Goloubeva O, Fulton AM. Immune-mediated modulation of breast cancer growth and metastasis by the chemokine Mig (CXCL9) in a murine model. J Immunother (2007) 30:490–98. doi: 10.1097/CJI.0b013e318031b551
70. Liu W, Liu Y, Fu Q, Zhou L, Chang Y, Xu L, et al. Elevated expression of IFN-inducible CXCR3 ligands predicts poor prognosis in patients with non-metastatic clear-cell renal cell carcinoma. Oncotarget (2016) 7:13976–83. doi: 10.18632/oncotarget.7468
71. Xin H, Kikuchi T, Andarini S, Ohkouchi S, Suzuki T, Nukiwa T, et al. Antitumor immune response by CX3CL1 fractalkine gene transfer depends on both NK and T cells. Eur J Immunol (2005) 35:1371–80. doi: 10.1002/eji.200526042
72. Carlsten M, Levy E, Karambelkar A, Li L, Reger R, Berg M, et al. Efficient mRNA-Based Genetic Engineering of Human NK Cells with High-Affinity CD16 and CCR7 Augments Rituximab-Induced ADCC against Lymphoma and Targets NK Cell Migration toward the Lymph Node-Associated Chemokine CCL19. Front Immunol (2016) 7:105. doi: 10.3389/fimmu.2016.00105
73. Somanchi SS, Somanchi A, Cooper LJ, Lee DA. Engineering lymph node homing of ex vivo-expanded human natural killer cells via trogocytosis of the chemokine receptor CCR7. Blood (2012) 119:5164–72. doi: 10.1182/blood-2011-11-389924
74. Muller N, Michen S, Tietze S, Topfer K, Schulte A, Lamszus K, et al. Engineering NK cells modified with an EGFRvIII-specific chimeric antigen receptor to overexpress CXCR4 improves immunotherapy of CXCL12/SDF-1alpha-secreting glioblastoma. J Immunother (2015) 38:197–210. doi: 10.1097/CJI.0000000000000082
75. Klusa D, Lohaus F, Franken A, Baumbach M, Cojoc M, Dowling P, et al. Dynamics of CXCR4 positive circulating tumor cells in prostate cancer patients during radiotherapy. Int J Cancer (2023) 152:2639–54. doi: 10.1002/ijc.34457
76. Tamas K, Domanska UM, van Dijk TH, Timmer-Bosscha H, Havenga K, Karrenbeld A, et al. CXCR4 and CXCL12 expression in rectal tumors of stage IV patients before and after local radiotherapy and systemic neoadjuvant treatment. Curr Pharm Des (2015) 21:2276–83. doi: 10.2174/1381612821666150105155615
77. Ou DL, Chen CL, Lin SB, Hsu CH, Lin LI. Chemokine receptor expression profiles in nasopharyngeal carcinoma and their association with metastasis and radiotherapy. J Pathol (2006) 210:363–73. doi: 10.1002/path.2053
78. Nardone V, Botta C, Caraglia M, Martino EC, Ambrosio MR, Carfagno T, et al. Tumor infiltrating T lymphocytes expressing FoxP3, CCR7 or PD-1 predict the outcome of prostate cancer patients subjected to salvage radiotherapy after biochemical relapse. Cancer Biol Ther (2016) 17:1213–20. doi: 10.1080/15384047.2016.1235666
79. Whitelaw BS, Tanny S, Johnston CJ, Majewska AK, O’Banion MK, Marples B. In vivo imaging of the microglial landscape after whole brain radiation therapy. Int J Radiat Oncol Biol Phys (2021) 111:1066–71. doi: 10.1016/j.ijrobp.2021.07.038
80. Chen M, Zhao J, Luo C, Pandi SP, Penalva RG, Fitzgerald DC, et al. Para-inflammation-mediated retinal recruitment of bone marrow-derived myeloid cells following whole-body irradiation is CCL2 dependent. Glia (2012) 60:833–42. doi: 10.1002/glia.22315
81. Alghamri MS, Banerjee K, Mujeeb AA, Mauser A, Taher A, Thalla R, et al. Systemic delivery of an adjuvant CXCR4-CXCL12 signaling inhibitor encapsulated in synthetic protein nanoparticles for glioma immunotherapy. ACS Nano (2022) 16:8729–50. doi: 10.1021/acsnano.1c07492
82. Walle T, Kraske JA, Liao B, Lenoir B, Timke C, von Bohlen UHE, et al. Radiotherapy orchestrates natural killer cell dependent antitumor immune responses through CXCL8. Sci Adv (2022) 8:eabh4050. doi: 10.1126/sciadv.abh4050
83. Chen L, Shi V, Wang S, Sun L, Freeman R, Yang J, et al. SCCA1/SERPINB3 suppresses antitumor immunity and blunts therapy-induced T cell responses via STAT-dependent chemokine production. J Clin Invest (2023) 133:e163841. doi: 10.1172/JCI163841
84. Greene S, Robbins Y, Mydlarz WK, Huynh AP, Schmitt NC, Friedman J, et al. Inhibition of MDSC trafficking with SX-682, a CXCR1/2 inhibitor, enhances NK-cell immunotherapy in head and neck cancer models. Clin Cancer Res (2020) 26:1420–31. doi: 10.1158/1078-0432.CCR-19-2625
85. Voskoboinik I, Whisstock JC, Trapani JA. Perforin and granzymes: function, dysfunction and human pathology. Nat Rev Immunol (2015) 15:388–400. doi: 10.1038/nri3839
86. Zamai L, Ahmad M, Bennett IM, Azzoni L, Alnemri ES, PeRussia B. Natural killer (NK) cell-mediated cytotoxicity: differential use of TRAIL and Fas ligand by immature and mature primary human NK cells. J Exp Med (1998) 188:2375–80. doi: 10.1084/jem.188.12.2375
87. Ward-Kavanagh LK, Zhu J, Cooper TK, Schell TD. Whole-body irradiation increases the magnitude and persistence of adoptively transferred T cells associated with tumor regression in a mouse model of prostate cancer. Cancer Immunol Res (2014) 2:777–88. doi: 10.1158/2326-6066.CIR-13-0164
88. Dong C, Dang D, Zhao X, Wang Y, Wang Z, Zhang C. Integrative characterization of the role of IL27 in melanoma using bioinformatics analysis. Front Immunol (2021) 12:713001. doi: 10.3389/fimmu.2021.713001
89. Cheng CC, Ho AS, Peng CL, Chang J, Sie ZL, Wang CL, et al. Sorafenib suppresses radioresistance and synergizes radiotherapy-mediated CD8(+) T cell activation to eradicate hepatocellular carcinoma. Int Immunopharmacol (2022) 112:109110. doi: 10.1016/j.intimp.2022.109110
90. Shen D, Luo J, Chen L, Ma W, Mao X, Zhang Y, et al. PARPi treatment enhances radiotherapy-induced ferroptosis and antitumor immune responses via the cGAS signaling pathway in colorectal cancer. Cancer Lett (2022) 550:215919. doi: 10.1016/j.canlet.2022.215919
91. Merhi M, Raza A, Inchakalody VP, Siveen KS, Kumar D, Sahir F, et al. Persistent anti-NY-ESO-1-specific T cells and expression of differential biomarkers in a patient with metastatic gastric cancer benefiting from combined radioimmunotherapy treatment: a case report. J Immunother Cancer (2020) 8:e001278. doi: 10.1136/jitc-2020-001278
92. Krensky AM. Granulysin: a novel antimicrobial peptide of cytolytic T lymphocytes and natural killer cells. Biochem Pharmacol (2000) 59:317–20. doi: 10.1016/s0006-2952(99)00177-x
93. Russell JH, Ley TJ. Lymphocyte-mediated cytotoxicity. Annu Rev Immunol (2002) 20:323–70. doi: 10.1146/annurev.immunol.20.100201.131730
94. Jiang K, Zhong B, Gilvary DL, Corliss BC, Hong-Geller E, Wei S, et al. Pivotal role of phosphoinositide-3 kinase in regulation of cytotoxicity in natural killer cells. Nat Immunol (2000) 1:419–25. doi: 10.1038/80859
95. Yuan X, Gajan A, Chu Q, Xiong H, Wu K, Wu GS. Developing TRAIL/TRAIL death receptor-based cancer therapies. Cancer Metastasis Rev (2018) 37:733–48. doi: 10.1007/s10555-018-9728-y
96. Dyer MJ, MacFarlane M, Cohen GM. Barriers to effective TRAIL-targeted therapy of Malignancy. J Clin Oncol (2007) 25:4505–06. doi: 10.1200/JCO.2007.13.1011
97. Di Pietro R, Secchiero P, Rana R, Gibellini D, Visani G, Bemis K, et al. Ionizing radiation sensitizes erythroleukemic cells but not normal erythroblasts to tumor necrosis factor-related apoptosis-inducing ligand (TRAIL)–mediated cytotoxicity by selective up-regulation of TRAIL-R1. Blood (2001) 97:2596–603. doi: 10.1182/blood.v97.9.2596
98. Finnberg N, Gruber JJ, Fei P, Rudolph D, Bric A, Kim SH, et al. DR5 knockout mice are compromised in radiation-induced apoptosis. Mol Cell Biol (2005) 25:2000–13. doi: 10.1128/MCB.25.5.2000-2013.2005
99. Zhang X, Cheung RM, Komaki R, Fang B, Chang JY. Radiotherapy sensitization by tumor-specific TRAIL gene targeting improves survival of mice bearing human non-small cell lung cancer. Clin Cancer Res (2005) 11:6657–68. doi: 10.1158/1078-0432.CCR-04-2699
100. Hu S, Zhu L, Song Y, Zhao X, Chen Q, Pan Y, et al. Radiation-induced abscopal reproductive effect is driven by TNF-alpha/p38 MAPK/Rac1 axis in Sertoli cells. Theranostics (2021) 11:5742–58. doi: 10.7150/thno.56853
101. Lovric MM, Hawkins CJ. TRAIL treatment provokes mutations in surviving cells. Oncogene (2010) 29:5048–60. doi: 10.1038/onc.2010.242
102. Wagner JA, Rosario M, Romee R, Berrien-Elliott MM, Schneider SE, Leong JW, et al. CD56bright NK cells exhibit potent antitumor responses following IL-15 priming. J Clin Invest (2017) 127:4042–58. doi: 10.1172/JCI90387
103. Wiedemann GM, Santosa EK, Grassmann S, Sheppard S, Le Luduec JB, Adams NM, et al. Deconvoluting global cytokine signaling networks in natural killer cells. Nat Immunol (2021) 22:627–38. doi: 10.1038/s41590-021-00909-1
104. Raulet DH. Interplay of natural killer cells and their receptors with the adaptive immune response. Nat Immunol (2004) 5:996–1002. doi: 10.1038/ni1114
105. Teitz-Tennenbaum S, Li Q, Rynkiewicz S, Ito F, Davis MA, McGinn CJ, et al. Radiotherapy potentiates the therapeutic efficacy of intratumoral dendritic cell administration. Cancer Res (2003) 63:8466–75.
106. Zheng W, Ranoa D, Huang X, Hou Y, Yang K, Poli EC, et al. RIG-I-like receptor LGP2 is required for tumor control by radiotherapy. Cancer Res (2020) 80:5633–41. doi: 10.1158/0008-5472.CAN-20-2324
107. Deng L, Liang H, Xu M, Yang X, Burnette B, Arina A, et al. STING-dependent cytosolic DNA sensing promotes radiation-induced type I interferon-dependent antitumor immunity in immunogenic tumors. Immunity (2014) 41:843–52. doi: 10.1016/j.immuni.2014.10.019
108. Zhang JY, Tan EM. Autoantibodies to tumor-associated antigens as diagnostic biomarkers in hepatocellular carcinoma and other solid tumors. Expert Rev Mol Diagn (2010) 10:321–28. doi: 10.1586/erm.10.12
109. Demaria O, Gauthier L, Vetizou M, Blanchard AA, Vagne C, Habif G, et al. Antitumor immunity induced by antibody-based natural killer cell engager therapeutics armed with not-alpha IL-2 variant. Cell Rep Med (2022) 3:100783. doi: 10.1016/j.xcrm.2022.100783
110. Liang J, Ding T, Guo ZW, Yu XJ, Hu YZ, Zheng L, et al. Expression pattern of tumour-associated antigens in hepatocellular carcinoma: association with immune infiltration and disease progression. Br J Cancer (2013) 109:1031–39. doi: 10.1038/bjc.2013.390
111. Uribe-Herranz M, Rafail S, Beghi S, Gil-de-Gomez L, Verginadis I, Bittinger K, et al. Gut microbiota modulate dendritic cell antigen presentation and radiotherapy-induced antitumor immune response. J Clin Invest (2020) 130:466–79. doi: 10.1172/JCI124332
112. Wu Y, Kuang DM, Pan WD, Wan YL, Lao XM, Wang D, et al. Monocyte/macrophage-elicited natural killer cell dysfunction in hepatocellular carcinoma is mediated by CD48/2B4 interactions. Hepatology (2013) 57:1107–16. doi: 10.1002/hep.26192
113. Singh AK, Winslow TB, Kermany MH, Goritz V, Heit L, Miller A, et al. A pilot study of stereotactic body radiation therapy combined with cytoreductive nephrectomy for metastatic renal cell carcinoma. Clin Cancer Res (2017) 23:5055–65. doi: 10.1158/1078-0432.CCR-16-2946
114. Shao C, Yang F, Miao S, Liu W, Wang C, Shu Y, et al. Role of hypoxia-induced exosomes in tumor biology. Mol Cancer (2018) 17:120. doi: 10.1186/s12943-018-0869-y
115. Parodi M, Raggi F, Cangelosi D, Manzini C, Balsamo M, Blengio F, et al. Hypoxia modifies the transcriptome of human NK cells, modulates their immunoregulatory profile, and influences NK cell subset migration. Front Immunol (2018) 9:2358. doi: 10.3389/fimmu.2018.02358
116. Balsamo M, Manzini C, Pietra G, Raggi F, Blengio F, Mingari MC, et al. Hypoxia downregulates the expression of activating receptors involved in NK-cell-mediated target cell killing without affecting ADCC. Eur J Immunol (2013) 43:2756–64. doi: 10.1002/eji.201343448
117. DePeaux K, Delgoffe GM. Metabolic barriers to cancer immunotherapy. Nat Rev Immunol (2021) 21:785–97. doi: 10.1038/s41577-021-00541-y
118. Okamoto S, Shiga T, Yasuda K, Watanabe S, Hirata K, Nishijima KI, et al. The reoxygenation of hypoxia and the reduction of glucose metabolism in head and neck cancer by fractionated radiotherapy with intensity-modulated radiation therapy. Eur J Nucl Med Mol Imaging (2016) 43:2147–54. doi: 10.1007/s00259-016-3431-4
119. Kelada OJ, Decker RH, Nath SK, Johung KL, Zheng MQ, Huang Y, et al. High single doses of radiation may induce elevated levels of hypoxia in early-stage non-small cell lung cancer tumors. Int J Radiat Oncol Biol Phys (2018) 102:174–83. doi: 10.1016/j.ijrobp.2018.05.032
120. Inada M, Nishimura Y, Hanaoka K, Nakamatsu K, Doi H, Uehara T, et al. Visualization of tumor hypoxia and re-oxygenation after stereotactic body radiation therapy in early peripheral lung cancer: A prospective study. Radiother Oncol (2023) 180:109491. doi: 10.1016/j.radonc.2023.109491
121. Supiot S, Rousseau C, Dore M, Cheze-Le-Rest C, Kandel-Aznar C, Potiron V, et al. Reoxygenation during radiotherapy in intermediate-risk prostate cancer. Radiother Oncol (2019) 133:16–9. doi: 10.1016/j.radonc.2018.12.022
122. Huang RX, Zhou PK. DNA damage response signaling pathways and targets for radiotherapy sensitization in cancer. Signal Transduct Target Ther (2020) 5:60. doi: 10.1038/s41392-020-0150-x
123. Furgason JM, Bahassi EM. Targeting DNA repair mechanisms in cancer. Pharmacol Ther (2013) 137:298–308. doi: 10.1016/j.pharmthera.2012.10.009
124. Ferreira P, Sousa R, Ferreira J, Militao G, Bezerra DP. Chloroquine and hydroxychloroquine in antitumor therapies based on autophagy-related mechanisms. Pharmacol Res (2021) 168:105582. doi: 10.1016/j.phrs.2021.105582
125. Digomann D, Linge A, Dubrovska A. SLC3A2/CD98hc, autophagy and tumor radioresistance: a link confirmed. Autophagy (2019) 15:1850–51. doi: 10.1080/15548627.2019.1639302
126. Morvan MG, Lanier LL. NK cells and cancer: you can teach innate cells new tricks. Nat Rev Cancer (2016) 16:7–19. doi: 10.1038/nrc.2015.5
127. Alspach E, Lussier DM, Schreiber RD. Interferon gamma and its important roles in promoting and inhibiting spontaneous and therapeutic cancer immunity. Cold Spring Harb Perspect Biol (2019) 11:a028480. doi: 10.1101/cshperspect.a028480
128. Kim KS, Choi B, Choi H, Ko MJ, Kim DH, Kim DH. Enhanced natural killer cell anti-tumor activity with nanoparticles mediated ferroptosis and potential therapeutic application in prostate cancer. J Nanobiotechnol (2022) 20:428. doi: 10.1186/s12951-022-01635-y
129. Liao P, Wang W, Wang W, Kryczek I, Li X, Bian Y, et al. CD8(+) T cells and fatty acids orchestrate tumor ferroptosis and immunity via ACSL4. Cancer Cell (2022) 40:365–78. doi: 10.1016/j.ccell.2022.02.003
130. Zeng L, Ding S, Cao Y, Li C, Zhao B, Ma Z, et al. A MOF-based potent ferroptosis inducer for enhanced radiotherapy of triple negative breast cancer. ACS Nano (2023) 17:13195–210. doi: 10.1021/acsnano.3c00048
131. Yang M, Wu X, Hu J, Wang Y, Wang Y, Zhang L, et al. COMMD10 inhibits HIF1alpha/CP loop to enhance ferroptosis and radiosensitivity by disrupting Cu-Fe balance in hepatocellular carcinoma. J Hepatol (2022) 76:1138–50. doi: 10.1016/j.jhep.2022.01.009
132. Lei G, Zhang Y, Koppula P, Liu X, Zhang J, Lin SH, et al. The role of ferroptosis in ionizing radiation-induced cell death and tumor suppression. Cell Res (2020) 30:146–62. doi: 10.1038/s41422-019-0263-3
133. Rao E, Hou Y, Huang X, Wang L, Wang J, Zheng W, et al. All-trans retinoic acid overcomes solid tumor radioresistance by inducing inflammatory macrophages. Sci Immunol (2021) 6:eaba8426. doi: 10.1126/sciimmunol.aba8426
134. Balkwill F. TNF-alpha in promotion and progression of cancer. Cancer Metastasis Rev (2006) 25:409–16. doi: 10.1007/s10555-006-9005-3
135. Kimura K, Bowen C, Spiegel S, Gelmann EP. Tumor necrosis factor-alpha sensitizes prostate cancer cells to gamma-irradiation-induced apoptosis. Cancer Res (1999) 59:1606–14.
136. Chmura SJ, Nodzenski E, Beckett MA, Kufe DW, Quintans J, Weichselbaum RR. Loss of ceramide production confers resistance to radiation-induced apoptosis. Cancer Res (1997) 57:1270–75.
137. Giuffrida P, Caprioli F, Facciotti F, Di Sabatino A. The role of interleukin-13 in chronic inflammatory intestinal disorders. Autoimmun Rev (2019) 18:549–55. doi: 10.1016/j.autrev.2019.03.012
138. Du Y, Jia C, Liu Y, Li Y, Wang J, Sun K. Isorhamnetin enhances the radiosensitivity of A549 cells through interleukin-13 and the NF-kappaB signaling pathway. Front Pharmacol (2020) 11:610772. doi: 10.3389/fphar.2020.610772
139. Rahal OM, Wolfe AR, Mandal PK, Larson R, Tin S, Jimenez C, et al. Blocking interleukin (IL)4- and IL13-mediated phosphorylation of STAT6 (Tyr641) decreases M2 polarization of macrophages and protects against macrophage-mediated radioresistance of inflammatory breast cancer. Int J Radiat Oncol Biol Phys (2018) 100:1034–43. doi: 10.1016/j.ijrobp.2017.11.043
140. Fang Y, Herrick EJ, Nicholl MB. A possible role for perforin and granzyme B in resveratrol-enhanced radiosensitivity of prostate cancer. J Androl (2012) 33:752–60. doi: 10.2164/jandrol.111.015164
141. Zhuang M, Jiang S, Gu A, Chen X, Mingyan E. Radiosensitizing effect of gold nanoparticle loaded with small interfering RNA-SP1 on lung cancer: AuNPs-si-SP1 regulates GZMB for radiosensitivity. Transl Oncol (2021) 14:101210. doi: 10.1016/j.tranon.2021.101210
142. Kalluri R, LeBleu VS. The biology, function, and biomedical applications of exosomes. Science (2020) 367:eaau6977. doi: 10.1126/science.aau6977
143. Zhu L, Kalimuthu S, Gangadaran P, Oh JM, Lee HW, Baek SH, et al. Exosomes derived from natural killer cells exert therapeutic effect in melanoma. Theranostics (2017) 7:2732–45. doi: 10.7150/thno.18752
144. Luo H, Zhou Y, Zhang J, Zhang Y, Long S, Lin X, et al. NK cell-derived exosomes enhance the anti-tumor effects against ovarian cancer by delivering cisplatin and reactivating NK cell functions. Front Immunol (2022) 13:1087689. doi: 10.3389/fimmu.2022.1087689
145. Dosil SG, Lopez-Cobo S, Rodriguez-Galan A, Fernandez-Delgado I, Ramirez-Huesca M, Milan-Rois P, et al. Natural killer (NK) cell-derived extracellular-vesicle shuttled microRNAs control T cell responses. Elife (2022) 11:e76319. doi: 10.7554/eLife.76319
146. Penha R, Pellecchia S, Pacelli R, Pinto L, Fusco A. Ionizing radiation deregulates the microRNA expression profile in differentiated thyroid cells. Thyroid (2018) 28:407–21. doi: 10.1089/thy.2017.0458
147. Wang Z, Liu L, Du Y, Mi Y, Wang L. The HNF1A-AS1/miR-92a-3p axis affects the radiosensitivity of non-small cell lung cancer by competitively regulating the JNK pathway. Cell Biol Toxicol (2021) 37:715–29. doi: 10.1007/s10565-021-09595-z
148. Luo J, Si ZZ, Li T, Li JQ, Zhang ZQ, Chen GS, et al. MicroRNA-146a-5p enhances radiosensitivity in hepatocellular carcinoma through replication protein A3-induced activation of the DNA repair pathway. Am J Physiol Cell Physiol (2019) 316:C299–311. doi: 10.1152/ajpcell.00189.2018
149. Liu Z, Wu K, Gu S, Wang W, Xie S, Lu T, et al. A methyltransferase-like 14/miR-99a-5p/tribble 2 positive feedback circuit promotes cancer stem cell persistence and radioresistance via histone deacetylase 2-mediated epigenetic modulation in esophageal squamous cell carcinoma. Clin Transl Med (2021) 11:e545. doi: 10.1002/ctm2.545
150. Laskowski TJ, Biederstadt A, Rezvani K. Natural killer cells in antitumour adoptive cell immunotherapy. Nat Rev Cancer (2022) 22:557–75. doi: 10.1038/s41568-022-00491-0
Keywords: radiotherapy, natural killer cell, tumor microenvironment, granzyme B, CGAS/STING signaling
Citation: Zheng W, Ling S, Cao Y, Shao C and Sun X (2024) Combined use of NK cells and radiotherapy in the treatment of solid tumors. Front. Immunol. 14:1306534. doi: 10.3389/fimmu.2023.1306534
Received: 04 October 2023; Accepted: 22 December 2023;
Published: 09 January 2024.
Edited by:
Nigel John Waterhouse, The University of Queensland, AustraliaReviewed by:
Elizabeth McDonald, The University of Queensland, AustraliaZhen Zeng, The University of Queensland, Australia
Copyright © 2024 Zheng, Ling, Cao, Shao and Sun. This is an open-access article distributed under the terms of the Creative Commons Attribution License (CC BY). The use, distribution or reproduction in other forums is permitted, provided the original author(s) and the copyright owner(s) are credited and that the original publication in this journal is cited, in accordance with accepted academic practice. No use, distribution or reproduction is permitted which does not comply with these terms.
*Correspondence: Xinchen Sun, c3VueGluY2hlbjIwMTJAMTYzLmNvbQ==