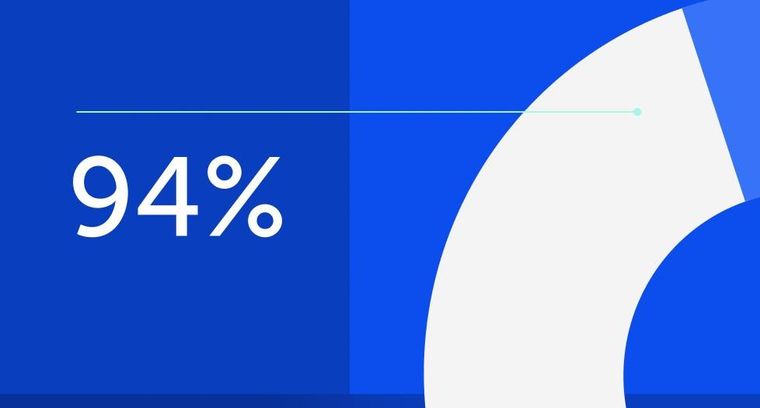
94% of researchers rate our articles as excellent or good
Learn more about the work of our research integrity team to safeguard the quality of each article we publish.
Find out more
REVIEW article
Front. Immunol., 24 November 2023
Sec. Cancer Immunity and Immunotherapy
Volume 14 - 2023 | https://doi.org/10.3389/fimmu.2023.1298891
This article is part of the Research TopicImmune Infiltration of Gastrointestinal Tract Cancers: Mechanisms and Clinical ImplicationsView all 5 articles
Gastrointestinal (GI) cancers remain a significant global health burden, accounting for a substantial number of cases and deaths. Regrettably, the inadequacy of dependable biomarkers hinders the precise forecasting of patient prognosis and the selection of appropriate therapeutic sequencing for individuals with GI cancers, leading to suboptimal outcomes for numerous patients. The intricate interplay between tumor-infiltrating lymphocytes (TILs) and the tumor immune microenvironment (TIME) has been shown to be a pivotal determinant of response to anti-cancer therapy and consequential clinical outcomes across a multitude of cancer types. Therefore, the assessment of TILs has garnered global interest as a promising prognostic biomarker in oncology, with the potential to improve clinical decision-making substantially. Moreover, recent discoveries in immunotherapy have progressively changed the landscape of cancer treatment and significantly prolonged the survival of patients with advanced cancers. Nonetheless, the response rate remains constrained within solid tumor sufferers, even when TIL landscapes appear comparable, which calls for the development of our understanding of cellular and molecular cross-talk between TIME and tumor. Hence, this comprehensive review encapsulates the extant literature elucidating the TILs’ underlying molecular pathogenesis, prognostic significance, and their relevance in the realm of immunotherapy for patients afflicted by GI tract cancers. Within this review, we demonstrate that the type, density, and spatial distribution of distinct TIL subpopulations carries pivotal implications for the prediction of anti-cancer treatment responses and patient survival. Furthermore, this review underscores the indispensable role of TILs in modulating therapeutic responses within distinct molecular subtypes, such as those characterized by microsatellite stability or programmed cell death ligand-1 expression in GI tract cancers. The review concludes by outlining future directions in TIL-based personalized medicine, including integrating TIL-based approaches into existing treatment regimens and developing novel therapeutic strategies that exploit the unique properties of TILs and their potential as a promising avenue for personalized cancer treatment.
Gastrointestinal (GI) cancers are responsible for a significant portion of the global cancer burden, accounting for 26% of all new cases and 35.4% of all cancer-related deaths. Notably, six types of GI cancers, including liver, stomach, colon, esophagus, pancreas, and rectum cancers, rank among the top ten list of tumor-related mortality rates worldwide (1).
The American Joint Committee on Cancer (AJCC) and Union for International Cancer Control (UICC) established the tumor-node-metastasis classification system (TNM) to assess the histopathological parameters of tumor invasion and predict the prognosis and chemotherapy response of individuals with GI cancers (2, 3). However, despite receiving equivalent treatment, patients with similar TNM stages may experience varying clinical outcomes due to the genetic and epigenetic heterogeneity of the cancers, as evidenced by several studies (4–6). The advent of anticancer therapy, particularly the remarkable advancements in immunotherapy, has significantly transformed the treatment of cancers. Nevertheless, only a subset of GI cancer patients exhibits a favorable response to these treatments, and the underlying causes of treatment failure in other patients remain poorly understood (7, 8). Therefore, gaining a comprehensive understanding of the molecular mechanisms that drive cancer progression is critical to facilitating the development of effective prognostic and predictive biomarkers for clinical research and practice (9).
Cancer cells require a conducive microenvironment to thrive and proliferate. The tumor microenvironment (TME) comprises a dynamic and complex network of factors, including immune cells, cancer-associated fibroblasts, stroma, and blood vessels, which create a favorable milieu for tumor growth, i.e., a low oxygen concentration, an acidic pH, and immunosuppression, which are known to promote tumor development and progression (10, 11).
Tumor-infiltrating lymphocytes (TILs) are a crucial component of the TME, playing a critical role in modulating the immune response to cancer cells. TILs are thought to exert both pro-tumor and anti-tumoral effects (12), and their presence and abundance in the TME have been implicated in cancer prognosis and response to therapy in various malignancies, including breast, lung, and ovarian cancers (13–15). Considering the pivotal role of TILs in cancer development and their impact on the efficacy of various anticancer therapies, this review conducts a comprehensive assessment of the molecular mechanisms of TILs in GI cancers. Furthermore, this review evaluates the responsiveness of different therapeutic strategies currently in clinical development to TILs, providing crucial insights into their clinical utility.
The authors conducted an extensive literature search to gather relevant studies on the subject. The investigation was conducted using PubMed and several high-impact journals, with diverse search terms such as “Tumor-infiltrating lymphocytes”, “T cells”, “CD8+”, “CD4+”, “CD3+”, “CD45RO+”, “FOXP3+”, “B cell”, “Immunoscore”, “Immunotherapy”, “Checkpoint inhibitor”, “Chemotherapy”, “Microsatellite*”, “Esophageal cancer”, “Stomach cancer”, “Gastric cancer”, “Pancreatic cancer”, “Hepatocellular cancer”, “Colorectal cancer”, “ Prognosis”, and “Survival”. The authors prioritized studies published from 2018 to 2023, and when no studies were available, older literature was included. The comprehensive search strategy ensured that the study’s findings were based on the most up-to-date and relevant available evidence.
The research included studies investigating immune infiltrates in non-metastatic and metastatic GI cancer patients. The studies analyzed various oncological outcomes, including response rate and survival, while also considering patients’ clinicopathological features and molecular assessments, such as the MMR status of microsatellite stability or instability. To ensure the quality of the evidence, the researchers excluded preclinical studies, narrative reviews, editorials, letters, opinions, non-English language publications, and conference abstracts that lacked sufficient methodological details. By applying these strict inclusion and exclusion criteria, the authors aimed to provide a comprehensive analysis of high-quality studies to support their findings.
The tumor immune microenvironment (TIME) is an integral component of the TME. It comprises a complex and dynamic network of immune cells and their associated molecules that engage in intricate interactions with tumor cells. The TIME is composed of a diverse array of immune cell types, including tumor-associated macrophages, myeloid-derived suppressor cells (MDSCs), tumor-associated neutrophils, mast cells, natural killer (NK) cells, dendritic cells (DCs), and T and B lymphocytes, that infiltrate cancerous tissues (16). The functions of these immune cells in the TIME are tightly linked to their inherent properties and the molecules they express, such as cytokines or inhibitory ligands, and play a crucial role in tumor evolution and growth (17, 18). Hence, the primary focus of recent translational clinical research is to enhance patients’ immune systems to enhance their ability to combat and eliminate cancer cells effectively. However, it is essential to note that, similar to the two-faced ancient Roman god Janus, immune system cells can exhibit dual roles, either mounting a protective antitumor response or inadvertently facilitating cancer progression. This duality largely depends on the composition of immune cell infiltrates within the tumor and the intricate communication between these cells and the tumor cells. Figure 1 illustrates an overall view of the intricate workings of the TME and multifaceted interactions with tumor cells.
Figure 1 Orchestra of the Tumor microenvironment (TME): The tumor microenvironment is the environment surrounding a tumor inside the body. It includes immune cells, the extracellular matrix, blood vessels, and other cells, like fibroblasts; different TME cells and components are mentioned in the figure (Created with BioRender.com).
The presence of tumor-associated immune cells within the TME poses a critical question that remains one of the foremost challenges in oncology: How do cancer cells evade the immune system? To address this question, it is essential to trace the progression from a physiologically anti-tumor microenvironment in the early stages of cancer development to an immune-suppressive microenvironment that promotes the survival and growth of cancer cells, a phenomenon commonly referred to as immune evasion (19).
Typically, During the early stages of tumor development, anti-tumor immune cells can recognize and eliminate cancer cells. CD8+ T-cells are identified as the predominant anti-tumor cells. Once they are stimulated and activated by Antigen-presenting cells (APCs), CD8+ T-cells undergo differentiation into cytotoxic T lymphocytes (CTLs). These CTLs execute a potent anti-tumoral response by releasing granules containing perforin and granzyme, which directly destroy cancer cells (20–22). Moreover, activation of CD4+ T-cells results in the release of cytokines such as interferon-gamma (IFN-γ) (which increases expression of MHC-I, increases expression of major histocompatibility complex [MHC] molecules on APCs, promotes antigen presentation, promotes differentiation of CD4+ T-cells into a T helper-1 [Th1]) and interleukin-2 (IL-2) (which is a proliferative cytokine that activates other CD4 + and promotes CD8+ T-cell maturation into CTLs) (23) (Figure 2).
Figure 2 (A) Early stage of cancer development: Cytotoxic T lymphocytes (CTL), which are differentiated from CD8+ T-cells via IL-2, are able to destroy tumor cells by releasing perforin and granzymes. IL-2 plus IFN-γ is released by CD4+ cells after activation through MHC presented by APC cells. IFN-γ increases the expression of represented MHC by APCs to CD8+ T-cells and also enhances the differentiation of CD4+ cells into T helper-1(Th1). (B)Immune evasion mechanisms: 1) Genomic instability, immune evasion, angiogenesis, and metastatic dissemination are among the elements that lead to tumor progression. One factor that leads to the stimulation of cases is said to be chronic inflammation, which plays a vital role in the development of cancer. 2) various factors, including Tregs, suppress or activate effector immune cells. Inhibiting the immune system using suppressive molecules or different surface receptors, inhibiting the function of dendritic cells (DC), and releasing inflammatory cytokines are among the parts of Treg in this system. Also, inhibition of Teff activation in 2 ways by limiting TCR ligand binding and using granzyme and metabolic disorders are other functions of Tregs. 3) Furthermore, CTLs fail to recognize tumor cells, and this is due to the reduction of MHC-I presented by APCs to CD8+ T-cells. 4) Tumor cells use FAS ligand (FasL) proteins to suppress CTLs, leading to their apoptosis, and finally induce inhibition of immune response (Created with BioRender.com).
Nevertheless, many types of human tumors can suppress the immune system to enhance their survival (24, 25). Inflammation is thought to play a significant role in establishing an immune-suppressive TME. The significance of inflammation in cancer progression is not a recent hypothesis; Rudolph Virchow first proposed it in 1863. In his study, Virchow observed leukocytes surrounding cancer cells and suggested that cancer might arise from chronic inflammation (26). Inflammation is a host immune response that aims to limit infections or repair damaged tissue. While it can be beneficial for healing, persistent chronic inflammation can lead to cell and tissue alteration, increasing cancer risk (27).
In the present understanding of cancer, chronic inflammation is widely acknowledged as a crucial characteristic, with potential causes including persistent microbial infections, autoimmune disorders, and immune dysregulation. An illustrative example of this relationship is the increased risk of gastric cancer associated with chronic infection by Helicobacter pylori (28). Similarly, immune dysregulation observed in inflammatory bowel disease contributes to a higher incidence of colorectal cancer (29).
Long-term inflammation can also increase the risk of cancer development by releasing reactive oxygen species (ROS) and reactive nitrogen species (RNS), which can cause harm to the cellular components, including DNA. As a result, it may lead to genetic mutations that influence genes responsible for cell growth and division, triggering abnormal cell growth and tumor formation (30, 31).
As the tumor grows and the tumor TME undergoes alterations, excessive tumor-associated antigens are produced during tumorigenesis. Consequently, the immune system’s ability to prime new repertoires of T cells and direct them toward the tumor is affected, leading to changes in the effectiveness of tumor containment. In parallel, cancer cells and the TME employ mechanisms to suppress the anti-tumor function of the immune system, often through the recruitment of regulatory CD4+ T-cells (Tregs) (32). Tregs play a critical role in the priming, activation, and cytotoxicity of other effector immune cells, including CTLs, Th1 CD4+ T-cells, NK cells, macrophages, and neutrophils (33, 34).
Moreover, some tumor cells escape immune detection by decreasing the expression of specific antigen-presenting proteins (for example, MHC-I) at their surface, rendering them invisible to CTL (35, 36). But more often, tumors secrete proteins that inhibit CTLs to suppress immune responses. For example, Fas ligand (FasL) has been reported to be expressed by the tumor cells, MDSCs, and vascular endothelium in many human solid tumors (37, 38). Consequently, the presence of FasL triggers apoptosis in the CTLs (39).
Tumor cells employ various factors, such as Cyclooxygenase-2 (COX-2), Prostaglandin E2 (PGE2), IL-6, Granulocyte-macrophage colony-stimulating factor (GM-CSF), S100 proteins, and Snail, to recruit and expand MDSCs within the TME. MDSCs, in turn, exert immunosuppressive effects on T-cell activation through multiple mechanisms. These include the depletion of essential amino acids like L-arginine and cysteine from the TME, the production of ROS and peroxynitrite, and the downregulation of CD62L and T-cell activation. MDSCs also contribute to the induction of Tregs via the production of IL-10 and transforming growth factor-beta (TGF-β). Additionally, MDSC expansion and IL-10 production inhibit DC antigen presentation, further contributing to immune suppression within the TME (40).
The secretion of certain growth factors, including TGF-β and epidermal growth factor (EGF), can also lead to increased cell proliferation and decreased responsiveness to signals that usually trigger programmed cancer-cell death (41, 42). Over time, this unregulated growth can lead to the emergence of cancer (Figure 2).
Amidst the diverse immune cell types that infiltrate cancerous tissues within the TIME, tumor-infiltrating lymphocytes are gaining recognition as a critical component due to their versatile functions and clinical potential (43). The formation of TILs from circulating lymphocytes is a complex process that begins with the migration of immune cells across the tumor endothelial barrier to reach the tumor site. However, the tumor endothelium is often disrupted and can directly suppress T cell function, impeding TIL infiltration. Notably, vessels carrying circulating lymphocytes are predominantly absent from the tumor core, instead localizing in the surrounding stroma and/or invasive margin, suggesting a directional aspect to TIL infiltration. These findings highlight the pivotal role of the tumor endothelium in regulating T-cell migration and infiltration and underscore the importance of the stroma in tumor development (44). Histopathological analyses of tumor samples have revealed distinct TIL distribution patterns across tumor types, with different immune cell types found in specific locations around and within the tumor. Interestingly, TIL distribution was observed to be non-random and organized in particular areas (45).
TILs generally encompass a diverse assortment of immune cell types, such as clusters of T-cells and B-cells (46, 47).
In the realm of cancer research, T-cells have received significant attention due to their prominence as the second most prevalent immune cell type found in human tumors across diverse cancer types. In the early stages of tumor development, if a sufficient number of immunogenic antigens are generated, naïve T cells undergo priming within the draining lymph nodes and/or bloodstream. This priming event is accompanied by their activation and subsequent migration to the tumor microenvironment (TME). Among the various types of T cells, the most widely recognized and extensively studied are the CD8+ T-cells (cytotoxic T-cells, or killer T-cells) and CD4+ T-cells (helper T-cells) (48).
Cytotoxic CD8+ T-cells (CTLs) of the adaptive immune system are the most potent effectors in the anti-cancer immune response. Activation of CTL is an antigen-specific process requiring the interaction of the T-cell receptor (TCR) complex with a processed tumor antigen–derived peptide bound to a MHC class I molecule presented by APCs or tumor cells (49). There are two mechanisms through which the CTLs carry out their function of combating cancer: granule exocytosis and the death ligand/death receptor system. The secretory granules comprising perforin and granzymes fuse with the plasma membrane and release their content, eliminating tumor cells. During death ligand/death receptor-mediated apoptosis, when CTLs are activated, FasL and TNF-related apoptosis-inducing ligands (TRAIL) are expressed on their surface. These ligands can kill susceptible cancer cells by interacting with their respective death receptors (50–52) (Figure 3A).
Figure 3 Immune cell differentiation in tissue. (A) Tumor cells are killed by two mechanisms when confronting cytotoxic T lymphocytes (CTL) activated by antigen-presenting cells (APC): first, cytolysis by releasing perforin and granzyme from CTLs. Second, the expression of death ligands FasL and TNF-related apoptosis-inducing ligands (TRAIL) on the surface of CTLs, whose interaction with the relevant death receptors leads to cancer cell apoptosis. (B) After penetrating the tissue and being activated by APCs, Naive CD4+ T cells differentiate into three different forms and have different functions: 1) Th1, secretion of IFN-g, TNF-a, and IL-2 to increase the toxicity and antitumor activity of CTLs, macrophages, and NK cells. 2) Th 2, by secreting different cytokines, promotes tumor cells to develop cancer. 3) Treg suppresses the cytotoxic activity of CTLs by using suppressor molecules. (C) After the penetration of naive B cells into the tissue, they are distinguished in three ways with specific functions: 1) Tumor-infiltrating B cells cause Th1 polarization and toxicity of cells by secreting IFN-γ and IL-12. 2) Plasma cells secrete tumor-specific antibodies and cause phagocytosis of tumor cells and stimulation of complement cascade. 3) Bregs, secretion of immune regulatory cytokines to Th2 polarization and inhibit the activity of CTLs (Created with BioRender.com).
CD4+ T lymphocytes play a crucial role in regulating the immune response and, in turn, are subdivided into at least Th1, Th2, Th9, Th17, and T regulatory (Treg) groups based on cytokine profiles and immune functions (53, 54). CD4+ T-cells interact with antigens in the context of MHC class II. This interaction causes the secretion of cytokines from the CD4+ cells to regulate various immune cells (55).
CD4+ Th1 cells, characterized by their secretion of high levels of proinflammatory cytokines such as IL-2, tumor necrosis factor-α (TNF-α), and IFN-γ, play a crucial role in the anti-tumoral response. Their activities include promoting T-cell priming and activation, enhancing the cytotoxicity of CTLs, boosting the anti-tumoral activity of macrophages and NK cells, and increasing the presentation of tumor antigens (24, 56) (Figure 3B).
CD4+ Th2 cells exhibit the secretion of a distinctive array of cytokines, including IL-4, IL-5, IL-10, IL-13, and IL-17. However, it is noteworthy that not all of these cytokines confer beneficial effects in the context of cancer, as some have been implicated in promoting tumor progression within the Th2 subtype (57). Specifically, IL-4, IL-5, and IL-13 have been extensively demonstrated to contribute to cancer growth and metastasis (57) actively.
Tregs are identified by their expression of the transcription factor Forkhead box p3 (FOXP3) along with a specific combination of cell surface markers, including CD4+, CD25+, and CD127low/−. Among these markers, FOXP3 is considered the most reliable and specific cell marker for identifying Tregs. Its presence indicates the suppressive and regulatory functions associated with Tregs in immune responses (58). FOXP3+ Tregs are responsible for maintaining immune tolerance, which can prevent allergic and other kinds of autoimmune diseases as well as inhibit the anti-tumor immune responses (59, 60). Tregs exert their immunomodulatory effects by utilizing several suppressive molecules and mechanisms. They employ programmed death-1 (PD-1), cytotoxic T lymphocyte-associated antigen (CTLA-4), CD39, and diverse surface receptors to suppress immune responses. Tregs inhibit the function of DCs, which are crucial for initiating immune responses. Additionally, Tregs secrete anti-inflammatory cytokines such as IL-10, TGF-β, and IL-35, which dampen immune activation and promote an immunosuppressive environment. Furthermore, Tregs directly inhibit CTLs by eliciting cytolysis through the release of granzyme and by inducing metabolic disruptions (61, 62).
The balance between these CD4+ T-cell subsets is critical for maintaining immune homeostasis and preventing immune dysfunction in the TME (63).
B lymphocytes are the main cellular components of the humoral compartment of adaptive immunity (64). CD20 is a transmembrane protein found on the surface of B-cells. CD20 is a transmembrane protein found on the surface of B-cells. It is a marker of B-cells and is involved in B-cell development, differentiation, and B-cell receptor signaling (65).
CD20+ B-cells can exert both pro-tumor and anti-tumor effects on cancer growth (66). The balance between these opposing functions is influenced by various factors, including the interplay between B-cells and other immune cells, as well as the TME.
Evidence indicates that B-cells play several roles in promoting tumor progression (67). They can release IL-10, IL-35, and TGF-β that support Treg expansion and Th2 polarization while suppressing effector T-cell activity, which is potentialized by B-cell PD-L1 expression (68) (Figure 3C). Vascular endothelial growth factor (VEGF)-producing B-cells may also promote tumor progression through neoangiogenesis (67, 69).
B-cells can also contribute to suppressing tumor progression through various mechanisms. B-cells have the ability to present tumor-derived antigens to T-cells, facilitating their activation and the subsequent immune response against tumors. Furthermore, B-cells secrete cytokines such as IFN-γ and IL-12, which support the polarization of CD4+ T-cells towards a Th1 phenotype and enhance the cytotoxicity of CD8+ T-cells. Tumor-specific antibodies produced by plasma cells, a differentiated form of B-cells, play a crucial role in tumor immunity. These antibodies can trigger the complement cascade, leading to the destruction of tumor cells. They can also mediate the phagocytosis of tumor cells by immune cells and facilitate antibody-dependent cell cytotoxicity (ADCC) mediated by natural killer (NK) cells.
Moreover, activated B-cells can directly eliminate tumor cells by secretion molecules such as TNF-related apoptosis-inducing ligand (TRAIL) and granzyme B. These effector molecules induce apoptosis in tumor cells, contributing to their elimination (67, 70, 71).
More than two centuries ago, Rosenberg et al. (72) conducted a study that indisputably proved that TILs can enhance the condition of more than 50% of colon adenocarcinoma-bearing mice with hepatic or pulmonary metastasis when combined with cyclophosphamide and IL-2 (72, 73). Despite this conclusive discovery, the use of TILs in cancer treatment is still not prevalent in clinical settings. This unequivocally emphasizes the dire need for further research and adoption of TILs as a potential solution in GI cancer treatment. Here, we present a comprehensive overview of recent scientific advancements in the role of TILs in Esophageal cancer (Table 1), Pancreatic cancer (Table 2), Gastric cancer (Table 3), Colorectal cancer (Table 4), and Hepatocellular cancer (Table 5). Specifically, we summarize these developments in the context of cancer prognosis, treatment strategies, and the associated challenges and opportunities for further advancement.
Based on recent advances in cancer treatment, immunotherapy is crucial in the therapeutic strategy for various cancers, including esophageal cancer (EC) (112). Despite the advances, immunotherapy is not a highly effective treatment for EC patients as the overall response rate is less than 30%, and despite being initially treated with immunotherapy, most patients tend to develop acquired resistance over time (113). Due to the heterogenicity of the TIME in EC, the cause of resistance to immune therapy is still unclear (114). TILs, as extrinsic factors, can participate in cancer development and response to immunotherapy (79). The presence and number of TILs in the tumor environment might predict patients’ prognosis and cancer outcome (115).
Haddad R et al. (77) explored the association between neoadjuvant treatment, TILs, and survival in patients with EC who underwent esophagectomy. Their investigation of Forty-three specimens of EC showed higher abundant of CD8+, CD4+, CD3+, and CD45R0+ cells was positively correlated with praising pathological response to neoadjuvant chemotherapy, disease-free survival (DFS), and overall survival (OS) (77). Findings from the multivariate analysis also showed that CD8+ in the stroma is an independent factor in the prognosis of EC patients (77) (Table 1). In another study, the expressions of CD8+, CD4+, FOXP3+, Immunoglobulin G4 (IgG4), and IL-10 and clinical information of 118 patients with esophageal squamous cell carcinoma (ESCC) were assessed with hematoxylin and eosin (H&E), immunohistochemistry (IHC) staining and multi-color Immunofluorescence (81). The higher expression of CD4+ (OS, hazard ratio [HR]= 0.395, P-value= 0.007) and CD4+ plus CD8+ (OS, HR= 0.478, P-value= 0.013) was positively correlated with better prognosis and survival, and also CD4+ (OS, HR= 0.317, P-value= 0.008) was an independent protective factor for ESCC patients. Higher IgG4 in serum was shown to be associated with poorer prognosis. Additionally, CD4+ regulates IgG4-positive B lymphocytes in TME by producing IL-10 (81).
Within developing and inspiring immunotherapies, immune checkpoint inhibitors (ICBs) targeting the programmed cell death 1/programmed cell death ligand 1 (PD1/PDL1) axis have garnered notable recognition, including the prestigious Nobel Prize in 2018 (116). These inhibitors have been granted approval for application across various solid tumors. Upon encountering the MHCs, T-cells release IFN-γ, thereby augmenting the efficiency of tumor eradication. In response to IFN-γ released by CD8+ T-cells, the expression of PDL1 is upregulated on tumor cells. Concurrently, TCR signaling triggers the upregulation of PD1 on the surface of T-cells. The interaction between PD1 and PDL1 imposes negative regulatory effects, attenuating the anti-tumor function of T-cells (117, 118) (Figure 4). T-cells previously rendered inactive can be revitalized by targeting the PD1/PDL1 interaction through immunotherapy, reinstating their potent anti-tumor capabilities. The PD1/PDL1 blockade is emerging as a hopeful treatment option for cancer, showing impressive antitumor reactions while causing only minor side effects (119).
Figure 4 Mechanism of inhibition of PD1/PDL1 axis by tumor cells: After activation of CD 8+ T-Cell by MHC-I, IFN is secreted and activates the transcription factor IRF in the nucleus and, finally, the expression of PDL 1 in tumor cells. On the other hand, TCR signaling leads to an increase in the PD1 expression on the surface of T cells and the activation of the PD 1/PDL 1 axis, and the interactions between these two ultimately reduce the antitumor effects of T cells. Anti-PD1/PDL1 antibodies, as an effective treatment method, block the PD1/PDL1 function and increase immune cells’ antitumor activity (Created with BioRender.com).
Despite recognizing anti-PD1/PDL1 therapy as a significant breakthrough, clinical data have revealed limited response rates. Studies have shown that a substantial proportion of patients exhibit primary resistance, failing to respond to PD1/PDL1 blockade, while some other initial responders eventually develop acquired resistance (120). The association between the expression of PDL1 and PD1 and its impact on cancer prognosis continues to be a subject of ongoing scientific debate (121). Furthermore, the underlying mechanisms contributing to primary and acquired resistance to PD1/PDL1 therapy remain largely elusive, presenting a significant challenge in the field (122). Researchers are actively investigating the intricate association between PD1/PDL1 expression and TIL composition to unravel the underlying complexities and understand these interactions more deeply. Advancements in this area promise to improve the efficacy of PD1/PDL1-targeted therapies and ultimately optimize clinical outcomes (121).
In a large cohort study involving 433 patients who underwent curative resection for EC, the expression of PD-1 on TILs and cancer cells was meticulously evaluated (79). The study assessed the relationship between PD-1 expression and OS in patients with and without preoperative treatment. Notably, high PD-1 expression on TILs was found to be associated with poorer OS, specifically in individuals who did not receive preoperative therapies. Conversely, no significant difference in OS was observed in patients who underwent preoperative treatments. Furthermore, the researchers implemented a classification system that categorized patients into three distinct groups based on the expression levels of PD-1 and PD-L1. Group 1 encompassed individuals with high expression of both PD-1 and PD-L1, Group 2 consisted of patients with high PD-1 expression but low PD-L1 expression (or vice versa), and Group 3 consisted of individuals with low expression of both PD-1 and PD-L1. Notably, a significant disparity in OS was observed among these three groups, as evidenced by a log-rank P-value of 0.0017. Specifically, the 3-year OS rates were 58% in Group 1, 65% in Group 2, and 74% in Group 3 (79).
Additionally, Soeratram et al. (78) explored immune landscape patterns in the TME before and after neoadjuvant chemoradiation (nCRT). They were applying a comprehensive image analysis of digital image whole slides.; they revealed that the high mean density of combined CD8+, FOXP3+, and PD-1+ TILs in tumor epithelium (in tumor nest contact with tumor cells; distance < 20μm) and stroma (in tumor stroma not contact with tumor cells; distance > 20μm) of biopsies was associated with the better histopathological response (tumor regression grade) in 188 post‐nCRT resected specimens, and only CD8+ was associated with outcome (78). With the aim of evaluating the changes in immune markers after nCRT and the prognostic significance in esophageal squamous cell carcinoma (ESCC), Zhou et al. (74) analyzed indoleamine 2,3-dioxygenase 1 (IDO1), CD8+, and PD-L1 expression in 138 patients with ESCC who underwent nCRT and esophagectomy without achieving complete pathologic response were included for analysis. They demonstrated that the expression levels of IDO1[an immune inhibitor that suppresses T-cell function (123, 124)], PD-L1, and CD8+ density increased significantly after nCRT (P-value < 0.01 for all). Patients with high IDO1 expression after nCRT had poorer OS (P-value = 0.001). High post-CRT CD8+ density was significantly correlated with more favorable OS (P-value = 0.01) and relapse-free survival (RFS) (P-value = 0.008). However, neither pre- nor post-CRT PD-L1 expression was an independent prognostic factor for survival (74).
Interestingly, infiltration of TILs can be divergent in different types of EC, in which a study in 2021 by Conroy et al. showed that expression of CD8+ and, CD45RO+, and FOXP3+ are higher in squamous cell carcinoma (SCC) in comparison to esophageal adenocarcinoma (EAC) (76). However, this study also claims that there is no association between the expression of CD3+, CD8+, and CD45RO+ in the tumoral and stromal regions of EAC and SCC with OS (76). Despite all, a recent meta-analysis of 30 articles comprising 5,122 patients for the role of TILs in the prognosis of EC patients revealed that increased levels of generalized TILs (HR= 0.67, 95% CI= 0.47–0.95, P-value = 0.02), CD8+[HR= 0.68, 95% confidence interval (95% CI)= 0.60–0.78, P-value <0.001], and CD4+(HR= 0.70, 95% CI= 0.57–0.85, P-value <0.001) were associated with better OS and is not correlated with DFS. However, high levels of CD3+ and FOXP3+ were not correlated with OS and DFS of EC patients (125).
Pancreatic cancer (PC) is one of the poor prognosis cancers with a survival rate of 5 years below 5% (126). Poor perfusion environment, which leads to a reduction in the distribution of treatment agents and immune cells into the tumor, and also low TILs infiltration in the core of the tumor, which is called “cold tumor,” are the main reasons for treatment challenges and progression of PC cancer (127, 128)
Tahkola et al. (84) assessed the density of CD8+ and CD3+ in tumor area and invasive margin (IM) in 79 pancreatic ductal adenocarcinomas (PDAC) patients after surgery by digital image analyses and introduced immune cell score (ICS) (84). The patients were divided into three groups with a range of ICS 0 (low CD3+ and CD8+ densities) to ICS 4 (high CD3+ and CD8+ densities) in both regions. The results revealed that higher ICS is associated with better OS and prognosis (84). They also compared two techniques for evaluating TILs in tumoral tissues. They found that the correlation of TIL density in the whole-section technique with survival is higher than in the hotspot technique (84) (Table 2).
The study evaluated the density of TILs in 155 surgically resected PDAC tissues using H&E staining. The tissues were divided into two groups based on their TIL density: stromal TIL-positive and stromal TIL-negative. Results showed that stromal TIL-negative status was an independent predictor of both worse OS (HR=2.80, 95% CI= 1.75-4.48, P-value <0.01) and liver metastasis (HR=2.7, 95% CI= 1.80-4.06, P-value <0.01) (82). Interestingly, this study claimed that TILs can hinder cancer progression through the secretion of TNF-alpha on tumoral cells (82). Previous studies propose that TNF-alpha can induce apoptosis in cancer cells, and its role in different cancers, such as pancreatic and colorectal cancer, has been proved (129–131).
Zhang and colleagues (83) reported that the location and distribution of TILs in the TME can impact the prognosis of patients with PDAC. They conducted IHC staining on 143 PDAC samples to evaluate TILs in the intraepithelial (lymphocytes in direct contact with tumor cells) and intratumoral (lymphocytes within the tumor tissue) regions. The study found that CD8+ T-cell intraepithelial attack was an independent favorable prognostic factor for OS and negatively correlated with vascular invasion. Conversely, high intratumoral CD8+ T-cell infiltration without CD8+ T-cell intraepithelial attack was a poor prognostic factor, accompanied by T-stage progression. The study highlights the potential importance of intraepithelial immune responses in developing and treating PDAC (83).
Delayre et al. (85) used tissue microarray from 43 patients with left-sided (body and tail) PC specimens that went through IHC of TILs (CD8+, CD 45+, CD3+, CD4+, FOXP3+), CAFs (vimentin, α-smooth muscle actin αSMA), and functional markers (PD-L1 and Ki-67) to examine their association with DFS and OS using computer-assisted quantitative analysis (85). Results proposed that a high CD4+/CD3+ lymphocyte ratio, along with a low αSMA/vimentin ratio, is correlated with poorer survival. Further, a high FOXP3+/CD8+ ratio was also associated with poorly differentiated tumors (85).
CD8+ memory T-cells (like non-circulating tissue-resident memory cells) stay long in tumoral tissue and play a crucial role in immune suppression (132). The members of tissue-resident memory T-cells induce their anti-cancer effects through overexpression of E-cadherin and improve the cytotoxicity of T-cells (133). A study on 81 operated PDAC patients was done to investigate the prognostic role of tissue-resident memory T-cell and TME features (87). Samples from intra-tumoral and peritumoral areas for evaluating tissue-resident memory cells, TILs, CD204+ macrophages, tumor stroma, and PDL1+ have been collected and underwent the staining process. Higher expression of intra-tumoral tissue-resident memory cell counts was associated with better survival (P-value = 0.84) in PDAC patients (87). Decreased survival was observed in tumors with increased CD204+ tumor-associated macrophages, which were immunosuppressive elements of the microenvironment (P-value = 0.29). Also, there was no correlation between the expression of PDL-1+ and survival in 81 operated PDAC tissues (87).
A systematic review and meta-analysis, which included 39 investigations on PDAC, was done by Orhan et al. (134) in 2020. They revealed that upregulation of CD8+ and CD3+ are associated with better OS (HR= 0.58, 95% CI= 0.50-0.68) and (HR= 0.58, 95% CI= 0.50-0.68) respectively, but increased levels of FOXP3+ is correlates with worse OS (HR= 1.48, 95% CI= 1.20-1.83). Interestingly, the role of CD4+ in OS is ambiguous (134). Also, there was no significant difference in the location of immune cell infiltration in the tumoral tissue (134).
Microsatellites are short, repetitive DNA sequences that are susceptible to errors when DNA replicates. Microsatellite instability (MSI) arises from DNA mismatch repair system errors, causing the accumulation of mutations in microsatellites. There are three MSI categories: MSI-high (MSI-H), MSI-low (MSI-L), and microsatellite stable (MSS) (135, 136). Notably, MSI-H/deficient MMR (dMMR) tumors have a 20-fold higher mutation rate compared to MSS tumors, and over 80% of MSI-High tumors exhibit a high tumor mutation burden (TMB) exceeding 20 mutations per megabase (Mb). TMB positively correlates with the number of neoantigens in various cancer types (137). TMB is recognized as a predictive marker for the response to cancer immunotherapy (138, 139). High TMB can generate numerous neoantigens that stimulate an anti-tumor immune response, potentially leading to improved responses to immunotherapy (140). The elevated mutational burden and frequent frameshift mutations in MSI-H/dMMR tumors result in the production of numerous neoantigens recognized by the immune system, which can trigger lymphocytic infiltrates (135, 141) (Figure 5).
Figure 5 MSI status leads to a potent immune response. Neoantigens are produced by tumor cells due to genomic mutations, such as mutations in the DNA mismatch repair system (MMR) that lead to microsatellite instability (MSI). These antigens interact with T cell receptors (TCR) to increase the production of TILs. TILs recognize these antigens and induce a robust immune response (Created with BioRender.com).
To study the heterogeneity of MSI status in PC, a tissue microarray containing 597 tumors was examined through IHC using MutL protein homolog 1 (MLH1), postmeiotic segregation increased-2 (PMS2), MutS homolog 2 (MSH2), and MSH6 antibodies to detect MMR proteins and automated digital image analysis of CD8+. The results demonstrated markedly higher CD8 + in tumors “with” than “without” MMR deficiency (P-value < 0.0001), suggesting a role of MSI in the immune response. The significantly higher CD8 density in MMR deficient compared with MMR intact PCs may thus provide an additional hint towards a potential utility of ICI in the PCs (86). In a study involving 108 patients with PDAC, Tahkola et al. (142) assessed the Immune Cell Score (ICS) by quantifying the number of immune cells (CD3+ and CD8+) within the tumor core and invasive margin. The evaluation involved the utilization of tissue microarrays, IHC, and digital analysis, along with the application of MLH1 immunostaining to identify tumor tissues with MSI. The study found a significant correlation between a high ICS and improved disease-specific survival (DSS) and OS in PDAC patients. Notably, there was no observed connection between MSI status and ICS or the survival outcomes of individuals with PC (142). Still, more efforts must be made to investigate the association of MSI and TIL infiltration in patients’ prognosis and clinical characterization.
Advanced gastric cancer (GC) continues to pose a significant challenge, with a relatively low median survival of approximately 12-15 months (143, 144). It has become evident that relying solely on the TNM staging system is insufficient for accurately predicting prognosis and determining the appropriate benefits of adjuvant chemotherapy for patients with stage II and III GC following surgical intervention. As a result, researchers are actively investigating additional factors that can complement the TNM staging system in order to improve prognostic accuracy and guide treatment decisions for these patients.
Early in 1922, McCarty was the first one who brought up the concept of TILs, and considered the infiltration of lymphocytes into tumor tissue as an antitumor activity of the immune system and suspected that this could be a positive factor for the post-operative life of GC patients (145). Considering the role of the immune system in cancer development, in recent years, many efforts have been made to develop a comprehensive immune system to provide more accurate prognoses for GC patients.
A study investigated an Immunoscore system that assessed the density of CD3+, CD8+, and PD-L1 in the epithelial and stromal compartments of the tumor center (CT) and IM in 153 patients with MSI-H GC. Combining the analysis of PD-L1 expression and Immunoscore, the patients were classified into four distinct subgroups, demonstrating a significant difference in OS. The PD-L1 (+)/Immunoscore Low group exhibited the worst prognosis, while the PD-L1 (+)/Immunoscore High group showed the best prognosis. Multivariate analysis identified the combined status of PD-L1 expression and Immunoscore as an independent and significant prognostic factor for OS in patients with MSI-H GC (88) (Table 3). In a subsequent study by Jiang et al. (89) involving 879 gastric cancer (GC) patients, it was demonstrated that a higher Immunoscore group was associated with a significant survival advantage in terms of overall survival (OS). The study employed the least absolute shrinkage and selection operator (LASSO) Cox regression model to construct a classifier. The Immunoscore was calculated using a specific formula incorporating the densities of four distinct immune cell types (CD3+, CD8+, CD45RO+, and CD66b) from both the CT and IM regions (89).
Zou et al. (93) conducted a retrospective analysis of 101 GC patients (stage II-III) who underwent gastrectomy followed by chemoradiotherapy. The study aimed to assess the prognostic value of IS, which was determined by IHC staining of CD3+ and CD8+ T-cell counts in both the CT and IM regions. Based on the IS levels, patients were categorized into three groups. The results revealed that GC patients with higher IS levels exhibited significantly improved DFS (P-value < 0.001) and OS (P-value < 0.001). The IS demonstrated superior predictive ability compared to the traditional pathological TNM (pTNM) staging system (Area Under the ROC Curve [AUC]: 0.801 vs. 0.677 and 0.800 vs. 0.660 for DFS and OS, respectively) (93).
In a study by Zhang et al. (146), a scoring system incorporated intratumoral and stromal TILs in a cohort of 833 patients with stage I-III GC. The analysis revealed a significant association between TILs and various clinicopathological parameters, including tumor size, histological grade, lymph node metastasis, nerve invasion, tumor thrombus, pathological TNM stage, and World Health Organization subtypes. Moreover, high levels of TILs (hi-TIL) were identified as a positive and significant predictor of OS using Kaplan-Meier survival analysis (P-value < 0.001) and multivariate Cox regression analysis (HR = 0.431, 95% CI: 0.347-0.534, P-value < 0.001). Additionally, patients with high-TIL tumors demonstrated improved DFS and OS following curative surgery compared to those with low-TIL tumors (146).
Studies have shown that B-cells in the TME have a dual role in promoting or inhibiting tumor growth (147). Consistent with the potential role of B-cells in anti-tumor immunity, Ni et al. (94) conducted a study involving 584 GC patients who underwent radical gastrectomy and found that increased infiltration of CD20+ B-cells in GC was independently associated with significantly improved OS and DFS. The study also revealed high CD20+ B-cell infiltration levels correlated with lower lymph node metastasis rates and lower pathological TNM stage. Furthermore, both univariate and multivariate Cox regression analyses demonstrated that CD20+ B-cell infiltration served as an independent protective factor for prognosis (94).
In a meta-analysis conducted in 2020, it was observed that elevated infiltration of CD3+, CD8+, and CD4+ T-cells within the TME of GC was significantly associated with improved OS outcomes. While it has been postulated that FOXP3+ Tregs may induce immune suppression within the TME and consequently exacerbate GC prognosis, the meta-analysis revealed that FOXP3+ Treg infiltration did not exhibit a definitive association with clinical outcomes. These findings suggest a potential complex interplay between T-cell subsets and Tregs in the context of GC immunobiology. This necessitates further investigations to elucidate their precise roles and the mechanisms underlying their impact on disease progression (148).
The Klintrup–Mäkinen grade classifies tumor inflammatory cell infiltrates (including the number of lymphoid cells, neutrophilic and eosinophilic granulocytes) at the tumor IM using H&E-stained slides (149). Several studies suggest an association between high Klintrup–Mäkinen grade and good prognosis in colorectal cancer (149, 150). In 2020, Kemi et al. (91) conducted a study to assess and compare the prognostic significance of Immunocore (based on CD3+ and CD8+ lymphocyte densities at the tumor CT and IM) and Klintrup–Mäkinen grades and examine the consistency of Klintrup–Mäkinen grade assessment in GC (91). The study revealed that a high Klintrup–Mäkinen grade independently predicted a longer 5-year overall survival (adjusted HR = 0.59, 95% CI: 0.45–0.77) in both the intestinal (adjusted HR = 0.61, 95% CI = 0.44–0.85) and diffuse subgroups (adjusted HR = 0.52, 95% CI = 0.31–0.86). Both Immunocore and Klintrup–Mäkinen grades emerged as prognostic factors in gastric adenocarcinoma. Moreover, the Spearman correlation coefficient between the three-tiered Immunoscore and the two-tiered Klintrup–Mäkinen grade was found to be 0.425 (91). Recently, Yun et al. (92) established an IS based on the densities of CD3+, CD8+, and Foxp3+ T-lymphocytes in CT and IM regions of 389 patients who underwent surgical resection for stage II/III GC and received adjuvant chemotherapy with 5-FU. They examined the impact of this IS on patient survival. The study found that individuals with a high IS experienced significantly longer DFS (P-value <0.001). Moreover, the IS was consistent between patients with MSI-H and Microsatellite Stable (MSS)/MSI-Low status (83.3% and 80.5%, respectively). Further subgroup analysis based on MSI status revealed that patients with a high IS experienced substantial DFS and OS benefits in both the MSS/MSI-Low group (DFS: HR= 0.527, P-value= 0.004; OS: HR= 0.528, P-value= 0.007) and the MSI-H group (DFS: HR= 0.166, P-value= 0.028; OS: HR = 0.177, P-value = 0.035) (92).
In the study conducted by Yuan et al. (151), the research focus was on establishing connections between blood markers such as lymphocytes, monocytes, platelets, and neutrophils and the primary TME. In order to achieve this, the researchers employed multiplexed IHC to quantitatively assess proteins within the tumor environment at a sub-cellular level in a cohort of 80 GC patients. The study’s findings revealed a significant correlation between a higher lymphocyte-to-monocyte ratio (LMR) at the initial assessment and improved immune-related progression-free survival (PFS), as well as a tendency toward enhanced immune-related OS. Conversely, a higher neutrophil-to-lymphocyte ratio (NLR) was linked to poorer immune-related OS (151).
The relationship between TILs and colorectal cancer (CRC) patient prognosis was first reported in 1998. Specifically, the study demonstrated that the infiltration of CD8+ T-cells within cancer cell nests was associated with patient prognosis in the human CRC (152). This finding has since been corroborated by subsequent research and underscores the importance of TILs in progressing and managing CRC (153–155). In a recent study, Xin et al. (106) determined that more CD8+ lymphocyte infiltration in either primary tumors or paired distant metastases predicted an excellent prognosis (P-value =0.036 and 0.031, respectively). In multivariate analysis, CD8+ TIL density in primary tumors was an independent predictive factor for OS (HR= 0.28, 95% CI= 0.09-0.93, P-value =0.038) (106).
Elomaa et al. (105) conducted a study to assess the prognostic significance of the spatial distribution of T-cells in CRC. The study involved using IHC and digital image analysis to identify CD3+ and CD8+ cells and tumor cells in a total of 1229 CRC samples. The authors introduced the T-cell proximity score as a novel prognostic parameter based on evaluating the co-localization of tumor cells with T-cells. The study’s findings demonstrated that a high T-cell proximity score was significantly correlated with favorable outcomes in CRC. Importantly, this association remained significant even after accounting for potential confounding factors such as disease stage, MMR status, and T-cell density score (105) (Table 4). These results suggest that the spatial arrangement and proximity between T-cells and tumor cells could be a valuable prognostic factor in CRC.
Immunotherapy with ICIs has demonstrated clinical benefits in colon cancer patients, particularly those with microsatellite MSI-H status. In a clinical study conducted in 2020, pembrolizumab, an anti-PD-1 agent, exhibited significant improvements in PFS (16.5 vs. 8.2 months) compared to standard treatments as a first-line therapy for metastatic MSI-H colon cancer patients (156). Although MSI-H has shown promise as a predictive biomarker for immunotherapy across pan-cancer, its clinical applicability in colorectal cancer is limited by its relatively low prevalence among CRC patients. Additionally, some colon cancer patients with MSI-H status may still exhibit intrinsic or acquired resistance to immunotherapy (157). Therefore, the efficacy of MSI status as a biomarker for immunotherapy in colon cancer patients may be limited, and alternative or complementary biomarkers should be explored. Extensive lymphocytic infiltration is more frequently observed in MSI tumors than in MSS tumors. The relationship between TILs and MSI status can provide further insight into differentiating CRC patients with better prognostic outcomes. Understanding this relationship may help identify additional factors for predicting prognosis and response to immunotherapy in colon cancer patients.
In line with the concept mentioned above, Williams et al. (96) developed a TIL/MMR-based classification system to stratify the prognosis of CRC subtypes in patients with stage II/III tumors. Interestingly, the study found that even in the presence of MSI, a TIL-low status was associated with a clinically aggressive phenotype (96). This suggests that TIL status can provide additional prognostic information beyond MSI status alone and may help identify patients with poorer outcomes despite having MSI-positive tumors.
Indeed, immunotherapies have demonstrated limited efficacy in the treatment of MSS and MMR-proficient (pMMR) metastatic colorectal cancer (mCRC) patients (158). However, within the subset of MSS mCRC patients, evidence indicates that a higher baseline density of CD8+ TILs is associated with an increased likelihood of benefiting from immunotherapy. Specifically, a study involving durvalumab (anti-PD-1) in combination with trametinib (a mitogen-activated protein kinase inhibitor) demonstrated that MSS mCRC patients with higher levels of CD8+ TILs at baseline were more likely to experience positive treatment responses or clinical benefits (103).
In a study of neoadjuvant immunotherapy (nivolumab [anti-PD-1] plus ipilimumab [anti-CTLA-4]) for stage I-III colon cancer patients, CD8+PD-1+ T-cell infiltration was a predictive biomarker of response in pMMR patients (97).
In a single-arm phase 2 clinical trial conducted by Kuang et al. (104), the researchers investigated the potential of concurrent treatment with the DNA methyltransferase inhibitor azacitidine to enhance the antitumor activity of pembrolizumab in mCRC patients. The trial enrolled 30 patients who were refractory to chemotherapy, and the participants received a median of three cycles of the combined therapy. Notably, the density of CD8+ TILs increased during the treatment compared to the levels observed before treatment initiation. Furthermore, a higher baseline CD8+ TIL density was associated with a greater likelihood of benefiting from the treatment. In addition, the study also observed a correlation between tumor demethylation during treatment and tumor CD8+ TIL density increases (104). This suggests that the demethylation process may play a role in modulating the immune microenvironment by influencing the density of CD8+ TILs.
In an open-label, single-center pilot trial, combination therapy of perioperative durvalumab and tremelimumab (an anti-cytotoxic T lymphocyte-associated antigen 4 antibody) was investigated as a potential treatment for patients with resectable liver metastasis CRC—the trial aimed to assess whether this combination therapy could enhance immune responses in this disease setting. The study enrolled 24 patients, and the findings showed that four of them achieved a complete pathological response. Among them, two had dMMR status, and the other two had POLE (DNA polymerase ϵ) mutations. Pre- and post-treatment tumor tissue analysis indicated comparable levels of T-cell infiltration, but there was evidence of CD8+ and CD4+ activation after treatment. Also, post-treatment samples from patients with prolonged RFS showed increased B-cell transcriptome signature and B-cell density (102).
Changes in the epigenetic makeup of TILs have been linked to how cancer patients respond to immunotherapy. More precisely, modifications in DNA methylation and histone structure can impact the activation and functioning of T-cells, potentially affecting how well TILs can identify and eradicate cancer cells (159). Zou et al. (101) devised a DNA methylation signature tailored for CD8+ TILs to assess immune response and prognosis in CRC. Using Illumina EPIC methylation arrays, they identified specific DNA methylation patterns in CD8+ T-cells and created a signature score. Their study revealed that a low CD8+ MeTIL score, which signifies an abundance of CD8+ TILs, was linked to MSI-H tumors and predicted improved survival among CRC cohorts. These findings suggest that the CD8+ MeTIL signature score could be a valuable prognostic biomarker for CRC, highlighting the potential of epigenetic signatures in assessing immune response and prognosis in cancer (101).
Consensus molecular subtypes (CMS) is a classification system that stratifies CRC into four distinct subtypes based on gene expression profiling, providing insights into the tumors’ underlying biology and clinical behavior (160). The CMS classification system has been shown to have prognostic and predictive value and may help guide treatment decisions and improve outcomes for patients with colorectal cancer (161). In a recent study, Hu et al. (162) conducted a comprehensive analysis of CMS subtypes’ molecular characteristics and immunotherapy responses using multiple bioinformatics databases. Their findings suggest that CMS1 patients are more likely to respond positively to immunotherapy than the other CMS subtypes. This is attributed to the presence of immune infiltration and activation, which is significantly higher in the CMS1 subtype than in the other subtypes. In particular, TILs were found to be significantly more abundant in the CMS1 subtype (163). These results provide important insights into the potential use of CMS subtyping to predict immunotherapy response in colorectal cancer patients.
ImmunoScore® (IS®) is a novel diagnostic tool that has gained attention in recent years to predict the risk of cancer recurrence in patients with CRC. Developed by the Society for Immunotherapy of Cancer, IS® measures the density of immune cells in tumor tissue, providing valuable information about the patient’s immune response to the cancer. The use of IS® has been shown to be an effective means of predicting the risk of recurrence in CRC patients, making it a promising tool in the fight against this deadly disease (164). IS® quantified the density of two types of immune cells, CD3+ and CD8+ T-cells, in the CT and IM for each case, using a standardized IHC staining protocol. The stained tissue samples are then scanned and analyzed using digital pathology software to generate a score reflecting the immune cells’ density. Next, the means of four percentiles (two markers and two regions) are calculated and converted into the IS®. In a three-category IS® analysis, TIL densities between 0-25%, 25-70%, and 70-100% are scored as “low,” “intermediate,” and “high,” respectively. In a two-category analysis, TIL densities between 0-25% are scored as “low,” while densities between 25-100% are scored as “intermediate-high” (165) (Figure 6).
Figure 6 Schematic Illustration of the Immunoscore (IS) determination. In the top left image, digital pathology software is used to automatically identify tumor (CT) tissue, invasive margin (IM), and normal tissue in colon cancer samples. In the top right images, the software also automatically detects the numbers of CD3+ and CD8+ T-cells. The bottom chart illustrates the calculation of the IS for colon cancer. The method involves converting the densities of CD3+ and CD8+ T-cells in both the CT and IM into percentile values. Then, the means of four percentiles are calculated for the IS. In a three-category IS analysis, the mean densities within the ranges of 0-25%, 25-70%, and 70-100% are categorized as “low,” “intermediate,” and “high,” respectively. In a two-category analysis, the mean densities between 0-25% are labeled as “low,” while densities ranging from 25-100% are classified as “intermediate-high.”
Several studies have reported the validity of IS®as a prognostic marker in patients with CRC, regardless of the type of chemotherapy regimen used in the treatment (166, 167). In 2018, the Society for Immunotherapy of Cancer aimed to validate the Consensus IS®’s accuracy and prognostic value in classifying CRC patients. The study enrolled 2,681 stage I-III colon cancer patients from 14 different international centers. The IS® was determined by quantifying the density of CD3+ and CD8+ T-cells in the tumor core and IM using a standardized protocol. The study found that the patients with a high IS® had the lowest risk of recurrence at five years vs. patients with a low IS® (HR= 0.20, 95% CI= 0.10-0.38; P-value <0·0001) (164). Two years later, the Society for Immunotherapy of Cancer conducted another multicenter study to explore the association between the IS® and the effect of chemotherapy on time to recurrence (TTR) in patients with stage III colon cancer. As expected, the study found that patients with a high IS® had a significantly prolonged TTR, OS, and DFS (all P-values < 0.001). Among patients with MSS tumors, the high IS® was significantly associated with prolonged TTR (HR= 0.36; 95% CI= 0.21-0.62; P-value = 0.0003). Although, in the high-IS® group, chemotherapy was significantly associated with survival for both low-risk [HR (chemotherapy vs. no chemotherapy)= 0.42; 95% CI= 0.25-0.71; P-value = 0.0011] and high-risk [HR (chemotherapy vs. no chemotherapy)= 0.5; 95% CI= 0.33-0.77; P-value = 0.0015] patients; this association was not observed for the low-IS® group (P-value > 0.12) (99). These results support the implementation of the consensus IS® as a new component of a TNM-Immune cancer classification.
In a study by Pagès et al. (98), the three-category IS® was used to investigate its efficacy in predicting response to oxaliplatin-based adjuvant chemotherapy in stage III CRC patients. The study found that patients with a low IS® were at a higher risk of relapse or death compared to those with an intermediate-high IS® (HR= 1.54; 95% CI= 1.24-1.93, P-value = 0.0001). The IS® remained significantly associated with DFS in multivariable analysis when adjusted for gender, histological grade, T/N stage, and MSI (P-value = 0.003). In patients treated with mFOLFOX6 [leucovorin calcium (folinic acid), fluorouracil, and oxaliplatin], a statistically significant interaction was observed between IS® and treatment duration (3 vs. 6 months) in terms of predicting DFS (P-value = 0.057) (98).
Collectively, these findings suggest that the IS® may be a valuable prognostic biomarker for predicting patient outcomes and guiding treatment decisions in colon cancer. In addition, these studies provide strong evidence for implementing the consensus IS® as a new component of a TNM-Immune classification of cancer. The standardized protocol used in the studies may also pave the way for further international collaborations and standardization of immune biomarkers in cancer research.
Hepatocellular carcinoma (HCC) represents a significant global health burden, accounting for the third-highest cancer-related mortality worldwide (1). Recent advances in managing HCC introduced new therapeutic drugs, such as Lenvatinib, as a first-line therapeutic agent in unresectable HCC patients (168). However, the prognosis of HCC cases is poor, and the rate of recurrence and metastasis is still high (169, 170). Anticipation of discovering new predictor biomarkers in survival and treatment response of HCC patients and generating personalized treatment strategies will be increased in the near future.
Several recent studies have assessed the role of CD8+ T-cells as a significant tumor-infiltrating lymphocyte in the prognosis of HCC. The result was controversial; Sun et al. showed that high levels of CD8+ are associated with better prognosis (171) (Table 5). However, two other studies found no relation or even worse association between CD8+ density and the survival of patients (172, 173). Stulpinas et al. hypothesized that the region of assessment of CD8+ density in HCC samples can affect the results. Infiltration of lymphocytes in tumor microenvironment and non-tumoral nearby parenchyma can independently manage the survival of patients (110). In this study, the samples of 106 patients with HCC underwent H&E staining and hexagonal grid-based digital image analysis. The density of CD8+ T-cells was measured in malignant and non-malignant regions of the samples. Outcomes revealed that an increase in the standard deviation of CD8+ density in tumors is positively associated with better OS (HR= 0.41, P-value= 0.0026). In apposition, higher mean CD8+ density in non-tumoral parenchyma is an independent factor in worsening OS (110). Interestingly, in the next step, the authors create a new score for the prognosis of HCC patients by measuring another parameter. The combined OS risk score consists of 5 items. A higher score is correlated with intravascular invasion of the tumor, long duration of surgery, blood Basophil count > 0.055 × 109/L, and aspartate transaminase (AST) level > 135 U/L, which causes worsened OS. Also, stage pT1 of HCC, bigger tumor size, higher mean CD8+ density in the non-malignant region, wider tumor-free margin, and shorter time of surgery are associated with shorter regression‐free survival (110).
In another theory, the controversial effect of CD8+ T-cells in the prognosis of cancer can result from regulatory cells in the tumor microenvironment, which can affect the function of CD8+ T-cells (174). Exhausted CD8+ T-cells lose their effective cytotoxic capacity. Also, cytokine secretion and proliferative ability of the T-cell is decreased (175). Exhaustion in CD8+ T-cell causes expression of inhibitory receptors such as PD1 and T-cell immunoglobulin and mucin-domain-containing-3 (TIM3) (176). Despite advances in PD-1 blocked therapy in different cancers, the optimal response still depends on T-cell infiltration and function in the tumor environment (177). As a result, discovering the proteins and cells that can affect the CD8+ T-cell function can help to increase the quality of PD-1 blocked therapy.
In a recent study, Wang et al. assessed the expression level of thymocyte selection-associated high mobility group box protein (TOX) in 40 specimens of HCC (107). The role of TOX in T-cell differentiation has been proved in studies (107, 178). Wang et al. divided the HCC samples into three groups for evaluating the CD8+ T-cell, including good effector function (PD-1-TIM3-), moderate exhaustion (PD-1int TIM3+) and severe exhaustion (PD-1hi TIM3+). Under transcriptome sequencing analysis, the expression of TOX was higher in the group of PD-1hi TIM3+ CD8+ T-cells (107). Additionally, the knockdown of the TOX gene is associated with better anti-tumor function of CD8+ T-cells. In fact, TOX promotes PD-1 recycling on CD8+ T-cells through inhibiting RAS and PI3K-Akt pathways, which hinder the lysosomal digestion of PD-1 on CD8+ T-cells (107). In addition, lower levels of TOX on CD8+ T-cells in the periphery are associated with better prognosis and lower TNM stages in HCC patients (107). These findings open new insights into improving the PD-1 blocked therapy by downregulating TOX expression, which enhances CD8+ T-cells from exhaustion level.
Another way to increase the quality of PD-1 blocked therapy is to select co-stimulatory receptors, which enhance T-cell differentiation and cytotoxic functions (179). Kim et al. showed that CD137L is one of the most co-stimulatory receptors expressed in HCC. This study was performed on 79 patients with HCC and demonstrated that CD137L expression is higher in PD-1high CD8+ T-cells (108). To evaluate the effect of CD137L expression on PD-1high CD8+ T-cells function, the amount of CD39+CD103+ CD8+ TIL subsets was measured and demonstrated that an increased level of CD39+CD103+ is observed in CD137L positive cells, which is associated with activation of T-cells and tumor responsiveness. Besides, the CD137L expression on PD-1high CD8+ T-cells renovates the potency and proliferation of T-cells (108). In vitro assay of CD137L agonistic antibody effect on CD8+ TILs demonstrated a notable increase in proliferation of CD8+ T-cells and production of IFN-γ and TNF-α. Kim et al. proved that combination therapy of T-cells with 4-1BB co-stimulatory agonists and PD-1 blocked can improve the strength of T-cells in HCC (108).
Altogether, finding new pathways of markers that affect the T-cell Exhaustion and regulate their expression besides PD-1 blocked therapy can provide new promising immunotherapeutic strategies in managing HCC.
Advances in immune checkpoint inhibitor therapy, such as PD-1 blockade and targeted molecular therapy, have unveiled novel systematic approaches to anti-cancer treatment for HCC patients. Finn et al. proved that combination therapy with Atezolizumab (anti-PD-L1) plus Bevacizumab (anti-VEGF monoclonal antibody) increases OS and PFS of HCC patients and replaced as the primary chemotherapy line in advanced HCC patients (180). Despite this, HCC is still a poorly controlled cancer, and new personalized therapeutic strategies are needed. Kuwano et al. measured CD8+ T-cell as a predictive marker for progression-free survival in patients who underwent Atezolizumab plus Bevacizumab and Lenvatinib alone treatments. Lenvatinib is a molecular inhibitor of multiple receptor tyrosine kinases such as fibroblast growth factor (FGF), VEGF, and platelet-derived growth factor (PDGF) receptors. It is one of the first-line choices in treating unresectable HCC (181). Computed tomography (CT) or magnetic resonance imaging (MRI) were tools used in this study to evaluate the response to treatment every 6 to 12 weeks. Immunohistochemistry of CD8+ T-cells of HCC biopsy in 24 patients before treatment with atezolizumab plus bevacizumab demonstrated that higher CD8+ T-cells are associated with better progression-free survival. However, no correlation has been found between high CD8+ T-cell density in tumoral biopsy and Lenvatinib alone treatment (111). This study suggests a personal therapeutic strategy in HCC patients, though tumor liver biopsy is invasive. Nevertheless, new non-invasive strategies are needed to find markers for predicting treatment response in HCC patients.
The existence of TILs in the tumor microenvironment of the cancer is an essential component for the response and activation of immunotherapeutic agents (182)
Gao et al. showed that evaluating the prognosis of overall immune markers of TILs can bring newer approaches in HCC management and eliminate the limitation and biased information of valuing the subpopulations of TILs individually (109). This study was accomplished in two phases. The first phase was estimating the TILs density in 315 samples of HCC patients by H&E staining, which is called WCH set, and the next phase was validating the prognosis of TILs in 370 HCC patients from The Cancer Genome Atlas (TCGA). The tumors were categorized into three groups: high TILs ≧ at 50%, intermediate TILs between 10% and 50%, and low TILs < 10%. The results prove that OS and DFS is better in higher (OS, HR= 0.33 (0.13-0.83), P-value= 0.02, DFS, HR= 0.21 (0.09-0.52), P-value= 7.86 × 10-4) and intermediate (OS, HR= 0.54 (0.34-0.86), P-value= 0.01, DFS, HR= 0.34 (0.21-0.56), P-value= 1.56 × 10-5) TILs groups in WCH set. Also, this data is validated in the TCGA set, confirming that low TIL density was associated with worse OS and DFS. In conclusion, the result of this study suggests a promising approach to using TIL density for determining the prognosis of HCC patients in clinical assessments (109).
There are a considerable number of studies on the function of TILs in GI tract cancers, which fully show an active immune response correlates with survival and provides a rationale for TIL therapy in GI cancers. The intricate interplay between TILs and tumor cells in TIME has been demonstrated to be a key determinant of the response to immunotherapy. Currently, PD-L1 expression, tumor mutation burden (TMB), and MSI-H/dMMR are the sole predictive biomarkers to determine eligibility for treatment with ICI, yet they lack robustness (183).
Considering the previously discussed concept, the assessment of TILs can offer valuable insights into tumor immune response and potentially serve as an additional parameter for evaluating treatment outcomes. Considering TILs as a predictive factor for response to neoadjuvant therapy in preoperative prognostication could be a significant advancement toward personalized treatment approaches. In certain cancer types, biopsies have been effectively employed to assess TILs as predictors of treatment response (184, 185). This demonstrates the potential utility of TIL evaluation in the clinical practice of GI cancer patients, allowing for identifying patients who are more likely to respond favorably to specific therapies, thereby enabling tailored treatment strategies.
In the wake of an immunotherapy revolution and given the prognostic promise of the Immunoscore classification system in various cancers. Perhaps the Immunoscore® for colorectal cancer was the most successful one among all human cancers, which has demonstrated significant potential as an adjunct or alternative to the TNM cancer staging system. Therefore, the International Immunoscore Project has attempted to standardize immune measurements in other GI cancers (4). Notwithstanding encouraging evaluation of its efficacy in CRC, some studies addressed some shortfalls of the IS system for other cancers. For instance, in EC, a study utilizing a similar approach as Immunoscore® failed to detect significant associations between patient survival and the expression of CD3+, CD8+, or CD45RO+ (5). IS has been subject to scrutiny in other types of cancer, with several studies raising concerns about its failure in efficacy for not considering other immune cell infiltrates, including macrophages and NK cells, as well as the heterogeneity of T-cell infiltrates within the TIME (6).
Indeed, the individual lymphocyte subsets within the TME are crucial but insufficient for effective tumor immune control. The success of an antitumor immune response depends on various factors, including the proper localization of TILs, clustering, interplay, and costimulation of all lymphocyte subsets. These subsets coordinated, and synergistic action contributes to an effective immune response against tumors. Proper identification of the presence and location of TILs within the tumor tissue and their distribution and proximity to tumor cells can provide valuable information for predicting prognosis and therapy response.
This may suggest that a more extensive IS may be required for other GI cancers compared to what is currently proving successful for CRC. Among others, Wen et al. (7) have proposed a novel four-score system for GC that integrates the expression of CD8+, PD-L1 on tumor cells and immune cells, and PD-1+ on immune cells. This approach exhibits potential for superior prognostic application compared to existing models for GC patients. There is another challenge to incorporating the assessment of TILs into routine clinical practice for GI cancer oncology and pathology reporting due to the existence of several distinct methods for histological quantification of TIL subsets, each with its own specific scoring technique or cut-off. To establish reference values and confirm the validity of such approaches, extensive homogenous comparative analyses are necessary prior to the routine implementation of TIL assessment in the pathology of GI cancers. Moreover, Artificial intelligence (AI) is poised to transform the field of pathology by enabling more efficient and accurate diagnoses, as well as more personalized treatment plans. By leveraging machine learning algorithms to analyze large datasets and identify subtle patterns, AI has the potential to improve the accuracy and speed of pathology diagnoses, ultimately leading to better patient outcomes (186). Automatic digital machine learning presents a promising tool for assessing TILs precisely and consistently. This advanced technology allows for the simultaneous evaluation of complex TIL composition and localization using multiple defining markers while minimizing interobserver variability commonly encountered with manual assessment techniques (187). The use of an automated signature of CD8xPD-L1 has been reported as a predictive marker in non-small-cell lung cancer patients (188). However, further investigation is required to determine the optimal implementation and integration of digital analysis as an independent technology into daily clinical practice to fully leverage its potential benefits (186). A recent report demonstrated the agreement between manual and computational scoring of TILs. Yoo et al. (189) developed an open-source software-based analytic pipeline to quantify TILs from whole-slide images of 578 stage III or high-risk stage II CRC patients, which were stained for CD3+ and CD8+ using IHC. The findings indicated acceptable concordance between the automatic quantification of TILs and visual inspection by a pathologist, supporting the reliability of the computational approach (189). A semi-automated approach may be the most effective to optimize the application of digital analysis findings. In this method, a pathologist first selects the area of interest to ensure that a representative field is evaluated. Subsequently, a computer application or software is utilized to identify and provide an estimation of TILs in the selected area. The recent introduction of an open-source algorithm for the automated evaluation of TILs in melanoma is a notable development that has generated enthusiasm among researchers (190). This innovative tool has the potential to significantly advance the field of digital pathology by streamlining the evaluation process and improving the accuracy and reliability of TIL assessment. Finally, adopting a universally established method and cutoff for evaluating TILs of each cancer could make the quantification process more effective and promote their clinical implementation. This approach would enhance the consistency and reproducibility of TIL assessment across different laboratories and improve their clinical relevance and utility in cancer diagnosis and treatment. Moving on from the role of TILs in forecasting the prognosis into therapeutic utilities, we postulated that combinatorial immunotherapy regimens represent a pivotal approach for optimizing response rates and clinical outcomes in patients undergoing immunotherapy for cancer treatment. Specifically, the concurrent targeting of T-cells and NK cells through ICIs has garnered attention and is currently being translated into clinical practice. Notably, emerging agents such as anti-NKG2A (anti-CD94/NK group 2 member A) and anti-TIGIT (anti-T-cell immunoreceptor with immunoglobulin and ITIM domains) are being investigated in this context (191, 192).
Another future direction for TIL-based personalized medicine is the development of novel therapeutic strategies that exploit the unique properties of TILs. For instance, recently, a novel approach to immunotherapy known as adoptive cellular therapy (ACT) has emerged, offering a potential solution to the problem of drug resistance observed in solid tumors treated with ICI. This innovative approach involves isolating and expanding immune cells, such as T-cells, from a patient’s tumor or blood and their subsequent infusion back into the patient to enhance the immune response against the cancer (193). TIL therapy has been proven to have impressive clinical benefits for patients with various solid tumors such as lung cancer (194), cervical cancer (195), melanoma (196), CRC (197), and cholangiocarcinoma (198); regarding CRC, in phase two clinical trial involving a 50-year-old woman with metastatic colorectal cancer, a single infusion of 1.48 × 1011 TILs was administered. The infusion consisted of approximately 75% CD8+ T-cells specifically engineered to recognize the KRAS G12D mutant. Following treatment, all metastatic lesions in the patient regressed, and a nine-month partial response was achieved (197). Another clinical trial of TIL therapy using CAR (chimeric antigen receptor) cells targeting MAGE-A4 in patients with advanced solid tumors showed that patients with EC experienced an objective partial response in mediastinal and para-esophageal lymph nodes (199). These promising results suggest that TIL therapy may have significant potential as a treatment for solid cancers, and further research in this area is warranted for other GI cancers.
Immunotherapies have made remarkable strides, but there are still challenges to overcome. Limited response rates, unpredictable efficacy, and potential side effects have hindered their clinical application. However, by understanding how cancer cells and TILs interact in a spatial coordinate system, we can gain new insights into cancer progression and improve the efficiency of current immunotherapies. Thanks to new technologies emerging in the field of digital machine learning, with a focus on spatial mapping and quantification, the systematic and comprehensive understanding of in situ crosstalk between immune cells and cancer cells in the TME and their dynamic changes during treatment is within reach. These advancements are sure to propel the clinical success of immunotherapies even further.
MoeP: Supervision, Writing – original draft, Writing – review & editing. YG: Conceptualization, Writing – original draft. MobP: Visualization, Writing – original draft. ES: Writing – review & editing. EN-M: Conceptualization, Project administration, Supervision, Writing – review & editing.
The author(s) declare that no financial support was received for the research, authorship, and/or publication of this article.
We want to thank Biorender.com for providing the high-quality figures used in this manuscript. We also extend our appreciation to the Research Institute for Gastroenterology and Liver Diseases of Shahid Beheshti University of Medical Sciences for their valuable support throughout the course of this research.
The authors declare that the research was conducted in the absence of any commercial or financial relationships that could be construed as a potential conflict of interest.
All claims expressed in this article are solely those of the authors and do not necessarily represent those of their affiliated organizations, or those of the publisher, the editors and the reviewers. Any product that may be evaluated in this article, or claim that may be made by its manufacturer, is not guaranteed or endorsed by the publisher.
1. Sung H, Ferlay J, Siegel RL, Laversanne M, Soerjomataram I, Jemal A, et al. Global cancer statistics 2020: GLOBOCAN estimates of incidence and mortality worldwide for 36 cancers in 185 countries. CA Cancer J Clin (2021) 71(3):209–49. doi: 10.3322/caac.21660
2. Koessler T, Alsina M, Arnold D, Ben-Aharon I, Lutz MP, Obermannova R, et al. Highlights from ASCO-GI 2021 from EORTC Gastrointestinal tract cancer group. Br J Cancer (2021) 125(7):911–9. doi: 10.1038/s41416-021-01474-y
3. Ji X, Bu ZD, Yan Y, Li ZY, Wu AW, Zhang LH, et al. The 8th edition of the American Joint Committee on Cancer tumor-node-metastasis staging system for gastric cancer is superior to the 7th edition: results from a Chinese mono-institutional study of 1663 patients. Gastric Cancer (2018) 21(4):643–52. doi: 10.1007/s10120-017-0779-5
4. Park YH, Lee SJ, Cho EY, Choi Y, Lee JE, Nam SJ, et al. Clinical relevance of TNM staging system according to breast cancer subtypes. Ann Oncol (2011) 22(7):1554–60. doi: 10.1093/annonc/mdq617
5. Noh SH, Park SR, Yang HK, Chung HC, Chung IJ, Kim SW, et al. Adjuvant capecitabine plus oxaliplatin for gastric cancer after D2 gastrectomy (CLASSIC): 5-year follow-up of an open-label, randomised phase 3 trial. Lancet Oncol (2014) 15(12):1389–96. doi: 10.1016/S1470-2045(14)70473-5
6. Lea D, Håland S, Hagland HR, Søreide K. Accuracy of TNM staging in colorectal cancer: a review of current culprits, the modern role of morphology and stepping-stones for improvements in the molecular era. Scand J Gastroenterol (2014) 49(10):1153–63. doi: 10.3109/00365521.2014.950692
7. Vesely MD, Zhang T, Chen L. Resistance mechanisms to anti-PD cancer immunotherapy. Annu Rev Immunol (2022) 40:45–74. doi: 10.1146/annurev-immunol-070621-030155
8. Hatamian S, Etesam S, Mazidimoradi A, Momenimovahed Z, Salehiniya H. The barriers and facilitators of gastric cancer screening: a systematic review. J Gastrointest Cancer (2021) 52(3):839–45. doi: 10.1007/s12029-021-00652-8
9. Koncina E, Haan S, Rauh S, Letellier E. Prognostic and predictive molecular biomarkers for colorectal cancer: updates and challenges. Cancers (2020) 12(2):319. doi: 10.3390/cancers12020319
10. Barkley D, Moncada R, Pour M, Liberman DA, Dryg I, Werba G, et al. Cancer cell states recur across tumor types and form specific interactions with the tumor microenvironment. Nat Genet (2022) 54(8):1192–201. doi: 10.1038/s41588-022-01141-9
11. Kumari S, Advani D, Sharma S, Ambasta RK, Kumar P. Combinatorial therapy in tumor microenvironment: Where do we stand? Biochim Biophys Acta Rev Cancer (2021) 1876(2):188585. doi: 10.1016/j.bbcan.2021.188585
12. Peña-Romero AC, Orenes-Piñero E. Dual effect of immune cells within tumour microenvironment: pro- and anti-tumour effects and their triggers. Cancers (Basel) (2022) 14(7):1681. doi: 10.3390/cancers14071681
13. de Jong VMT, Wang Y, Ter Hoeve ND, Opdam M, Stathonikos N, Jóźwiak K, et al. Prognostic value of stromal tumor-infiltrating lymphocytes in young, node-negative, triple-negative breast cancer patients who did not receive (neo)Adjuvant systemic therapy. J Clin Oncol (2022) 40(21):2361–74. doi: 10.1200/JCO.21.01536
14. Federico L, McGrail DJ, Bentebibel SE, Haymaker C, Ravelli A, Forget MA, et al. Distinct tumor-infiltrating lymphocyte landscapes are associated with clinical outcomes in localized non-small-cell lung cancer. Ann Oncol (2022) 33(1):42–56. doi: 10.1016/j.annonc.2021.09.021
15. Fanale D, Dimino A, Pedone E, Brando C, Corsini LR, Filorizzo C, et al. Prognostic and predictive role of tumor-infiltrating lymphocytes (TILs) in ovarian cancer. Cancers (Basel) (2022) 14(18):4344. doi: 10.3390/cancers14184344
16. Giraldo NA, Sanchez-Salas R, Peske JD, Vano Y, Becht E, Petitprez F, et al. The clinical role of the TME in solid cancer. Br J Cancer (2019) 120(1):45–53. doi: 10.1038/s41416-018-0327-z
17. Candeias SM, Gaipl US. The immune system in cancer prevention, development and therapy. Anticancer Agents Med Chem (2016) 16(1):101–7. doi: 10.2174/1871520615666150824153523
18. Galon J, Bruni D. Tumor immunology and tumor evolution: intertwined histories. Immunity (2020) 52(1):55–81. doi: 10.1016/j.immuni.2019.12.018
19. Borgmann M, Quante M. Impact of the tumor microenvironment for esophageal tumor development-an opportunity for prevention? Cancers (Basel) (2022) 14(9):2246. doi: 10.3390/cancers14092246
20. Rastogi I, Jeon D, Moseman JE, Muralidhar A, Potluri HK, McNeel DG. Role of B cells as antigen presenting cells. Front Immunol (2022) 13:954936. doi: 10.3389/fimmu.2022.954936
21. Hay ZLZ, Slansky JE. Granzymes: the molecular executors of immune-mediated cytotoxicity. Int J Mol Sci (2022) 23(3):1833. doi: 10.3390/ijms23031833
22. Zeng Z, Chew HY, Cruz JG, Leggatt GR, Wells JW. Investigating T cell immunity in cancer: achievements and prospects. Int J Mol Sci (2021) 22(6):2907. doi: 10.3390/ijms22062907
23. Zheng K, Gao L, Hao J, Zou X, Hu X. An immunotherapy response prediction model derived from proliferative CD4(+) T cells and antigen-presenting monocytes in ccRCC. Front Immunol (2022) 13:972227. doi: 10.3389/fimmu.2022.972227
24. Gonzalez H, Hagerling C, Werb Z. Roles of the immune system in cancer: from tumor initiation to metastatic progression. Genes Dev (2018) 32(19-20):1267–84. doi: 10.1101/gad.314617.118
25. Elmusrati A, Wang J, Wang C-Y. Tumor microenvironment and immune evasion in head and neck squamous cell carcinoma. Int J Oral Science (2021) 13(1):24. doi: 10.1038/s41368-021-00131-7
26. Balkwill F, Mantovani A. Inflammation and cancer: back to Virchow? Lancet (2001) 357(9255):539–45. doi: 10.1016/S0140-6736(00)04046-0
27. Coussens LM, Werb Z. Inflammation and cancer. Nature (2002) 420(6917):860–7. doi: 10.1038/nature01322
28. Zhu L, Huang Y, Li H, Shao S. Helicobacter pylori promotes gastric cancer progression through the tumor microenvironment. Appl Microbiol Biotechnol (2022) 106(12):4375–85. doi: 10.1007/s00253-022-12011-z
29. Yalchin M, Baker AM, Graham TA, Hart A. Predicting colorectal cancer occurrence in IBD. Cancers (Basel) (2021) 13(12):2908. doi: 10.3390/cancers13122908
30. Yu W, Tu Y, Long Z, Liu J, Kong D, Peng J, et al. Reactive oxygen species bridge the gap between chronic inflammation and tumor development. Oxid Med Cell Longev (2022) 2022:2606928. doi: 10.1155/2022/2606928
31. Zhao H, Wu L, Yan G, Chen Y, Zhou M, Wu Y, et al. Inflammation and tumor progression: signaling pathways and targeted intervention. Signal Transduct Target Ther (2021) 6(1):263. doi: 10.1038/s41392-021-00658-5
32. Zhang Y, Lazarus J, Steele NG, Yan W, Lee HJ, Nwosu ZC, et al. Regulatory T-cell depletion alters the tumor microenvironment and accelerates pancreatic carcinogenesis. Cancer Discovery (2020) 10(3):422–39. doi: 10.1158/2159-8290.CD-19-0958
33. Zhou F, Shayan G, Sun S, Huang X, Chen X, Wang K, et al. Spatial architecture of regulatory T-cells correlates with disease progression in patients with nasopharyngeal cancer. Front Immunol (2022) 13:1015283. doi: 10.3389/fimmu.2022.1015283
34. Ohue Y, Nishikawa H. Regulatory T (Treg) cells in cancer: Can Treg cells be a new therapeutic target? Cancer Sci (2019) 110(7):2080–9. doi: 10.1111/cas.14069
35. Cornel AM, Mimpen IL, Nierkens S. MHC class I downregulation in cancer: underlying mechanisms and potential targets for cancer immunotherapy. Cancers (Basel) (2020) 12(7):1760. doi: 10.3390/cancers12071760
36. Seliger B. Novel insights into the molecular mechanisms of HLA class I abnormalities. Cancer Immunol Immunother. (2012) 61(2):249–54. doi: 10.1007/s00262-011-1153-9
37. Flores-Mendoza G, Rodríguez-Rodríguez N, Rubio RM, Madera-Salcedo IK, Rosetti F, Crispín JC. Fas/fasL signaling regulates CD8 expression during exposure to self-antigens. Front Immunol (2021) 12:635862. doi: 10.3389/fimmu.2021.635862
38. Motz GT, Santoro SP, Wang LP, Garrabrant T, Lastra RR, Hagemann IS, et al. Tumor endothelium FasL establishes a selective immune barrier promoting tolerance in tumors. Nat Med (2014) 20(6):607–15. doi: 10.1038/nm.3541
39. Zhu J, Petit PF, Van den Eynde BJ. Apoptosis of tumor-infiltrating T lymphocytes: a new immune checkpoint mechanism. Cancer Immunol Immunother. (2019) 68(5):835–47. doi: 10.1007/s00262-018-2269-y
40. Dysthe M, Parihar R. Myeloid-derived suppressor cells in the tumor microenvironment. Adv Exp Med Biol (2020) 1224:117–40. doi: 10.1007/978-3-030-35723-8_8
41. Kasza A. IL-1 and EGF regulate expression of genes important in inflammation and cancer. Cytokine (2013) 62(1):22–33. doi: 10.1016/j.cyto.2013.02.007
42. Colak S, Ten Dijke P. Targeting TGF-β Signaling in cancer. Trends Cancer (2017) 3(1):56–71. doi: 10.1016/j.trecan.2016.11.008
43. Lin B, Du L, Li H, Zhu X, Cui L, Li X. Tumor-infiltrating lymphocytes: Warriors fight against tumors powerfully. BioMed Pharmacother. (2020) 132:110873. doi: 10.1016/j.biopha.2020.110873
44. Fridman WH, Pagès F, Sautès-Fridman C, Galon J. The immune contexture in human tumours: impact on clinical outcome. Nat Rev Cancer (2012) 12(4):298–306. doi: 10.1038/nrc3245
45. Paijens ST, Vledder A, de Bruyn M, Nijman HW. Tumor-infiltrating lymphocytes in the immunotherapy era. Cell Mol Immunol (2021) 18(4):842–59. doi: 10.1038/s41423-020-00565-9
46. Pruneri G, Vingiani A, Denkert C. Tumor infiltrating lymphocytes in early breast cancer. Breast (2018) 37:207–14. doi: 10.1016/j.breast.2017.03.010
47. Bruni D, Angell HK, Galon J. The immune contexture and Immunoscore in cancer prognosis and therapeutic efficacy. Nat Rev Cancer (2020) 20(11):662–80. doi: 10.1038/s41568-020-0285-7
48. Xiong Y, Bosselut R. CD4-CD8 differentiation in the thymus: connecting circuits and building memories. Curr Opin Immunol (2012) 24(2):139–45. doi: 10.1016/j.coi.2012.02.002
49. Raskov H, Orhan A, Christensen JP, Gögenur I. Cytotoxic CD8(+) T cells in cancer and cancer immunotherapy. Br J Cancer (2021) 124(2):359–67. doi: 10.1038/s41416-020-01048-4
50. Martínez-Lostao L, Anel A, Pardo J. How do cytotoxic lymphocytes kill cancer cells? Clin Cancer Res (2015) 21(22):5047–56. doi: 10.1158/1078-0432.CCR-15-0685
51. Prokhnevska N, Cardenas MA, Valanparambil RM, Sobierajska E, Barwick BG, Jansen C, et al. CD8(+) T cell activation in cancer comprises an initial activation phase in lymph nodes followed by effector differentiation within the tumor. Immunity (2023) 56(1):107–24.e5. doi: 10.1016/j.immuni.2022.12.002
52. van der Leun AM, Thommen DS, Schumacher TN. CD8(+) T cell states in human cancer: insights from single-cell analysis. Nat Rev Cancer (2020) 20(4):218–32. doi: 10.1038/s41568-019-0235-4
53. Kim HJ, Cantor H. CD4 T-cell subsets and tumor immunity: the helpful and the not-so-helpful. Cancer Immunol Res (2014) 2(2):91–8. doi: 10.1158/2326-6066.CIR-13-0216
54. Wang J, Nan Y, Liu M, Hu K. The role of CD4(+) T cells in the immunotherapy of brain disease by secreting different cytokines. J Neuroimmune Pharmacol (2022) 17(3-4):409–22. doi: 10.1007/s11481-022-10056-5
55. Farhood B, Najafi M, Mortezaee K. CD8(+) cytotoxic T lymphocytes in cancer immunotherapy: A review. J Cell Physiol (2019) 234(6):8509–21. doi: 10.1002/jcp.27782
56. Saravia J, Chapman NM, Chi H. Helper T cell differentiation. Cell Mol Immunol (2019) 16(7):634–43. doi: 10.1038/s41423-019-0220-6
57. Basu A, Ramamoorthi G, Albert G, Gallen C, Beyer A, Snyder C, et al. Differentiation and regulation of T(H) cells: A balancing act for cancer immunotherapy. Front Immunol (2021) 12:669474. doi: 10.3389/fimmu.2021.669474
58. Olguín JE, Medina-Andrade I, Rodríguez T, Rodríguez-Sosa M, Terrazas LI. Relevance of regulatory T cells during colorectal cancer development. Cancers (Basel) (2020) 12(7):1888. doi: 10.3390/cancers12071888
59. Dominguez-Villar M, Hafler DA. Regulatory T cells in autoimmune disease. Nat Immunol (2018) 19(7):665–73. doi: 10.1038/s41590-018-0120-4
60. Togashi Y, Shitara K, Nishikawa H. Regulatory T cells in cancer immunosuppression - implications for anticancer therapy. Nat Rev Clin Oncol (2019) 16(6):356–71. doi: 10.1038/s41571-019-0175-7
61. Xu Z, Jiang X, Dai X, Li B. The dynamic role of FOXP3(+) tregs and their potential therapeutic applications during SARS-coV-2 infection. Front Immunol (2022) 13:916411. doi: 10.3389/fimmu.2022.916411
62. Kempkes RWM, Joosten I, Koenen H, He X. Metabolic pathways involved in regulatory T cell functionality. Front Immunol (2019) 10:2839. doi: 10.3389/fimmu.2019.02839
63. Fu Q, Chen N, Ge C, Li R, Li Z, Zeng B, et al. Prognostic value of tumor-infiltrating lymphocytes in melanoma: a systematic review and meta-analysis. Oncoimmunology (2019) 8(7):1593806. doi: 10.1080/2162402X.2019.1593806
64. Sebina I, Pepper M. Humoral immune responses to infection: common mechanisms and unique strategies to combat pathogen immune evasion tactics. Curr Opin Immunol (2018) 51:46–54. doi: 10.1016/j.coi.2018.02.001
65. Pavlasova G, Mraz M. The regulation and function of CD20: an “enigma” of B-cell biology and targeted therapy. Haematologica (2020) 105(6):1494–506. doi: 10.3324/haematol.2019.243543
66. Kuroda H, Jamiyan T, Yamaguchi R, Kakumoto A, Abe A, Harada O, et al. Tumor-infiltrating B cells and T cells correlate with postoperative prognosis in triple-negative carcinoma of the breast. BMC Cancer (2021) 21(1):286. doi: 10.1186/s12885-021-08009-x
67. Kinker GS, Vitiello GAF, Ferreira WAS, Chaves AS, Cordeiro de Lima VC, Medina TDS. B cell orchestration of anti-tumor immune responses: A matter of cell localization and communication. Front Cell Dev Biol (2021) 9:678127. doi: 10.3389/fcell.2021.678127
68. Lauss M, Donia M, Svane IM, Jönsson G. B cells and tertiary lymphoid structures: friends or foes in cancer immunotherapy? Clin Cancer Res (2022) 28(9):1751–8. doi: 10.1158/1078-0432.CCR-21-1130
69. Liu Y, Liu Z, Yang Y, Cui J, Sun J, Liu Y. The prognostic and biology of tumour-infiltrating lymphocytes in the immunotherapy of cancer. Br J Cancer (2023) 129(7):1041–9. doi: 10.1038/s41416-023-02321-y
70. Sur SY, Lim GH, Park SM, Seo KW, Youn HY. Anti-tumor effect of activated canine B cells with interleukin-21 and anti-B cell receptor. Anticancer Res (2023) 43(9):4007–14. doi: 10.21873/anticanres.16588
71. Rudnicka D, Oszmiana A, Finch DK, Strickland I, Schofield DJ, Lowe DC, et al. Rituximab causes a polarization of B cells that augments its therapeutic function in NK-cell-mediated antibody-dependent cellular cytotoxicity. Blood (2013) 121(23):4694–702. doi: 10.1182/blood-2013-02-482570
72. Rosenberg SA, Spiess P, Lafreniere R. A new approach to the adoptive immunotherapy of cancer with tumor-infiltrating lymphocytes. Science (1986) 233(4770):1318–21. doi: 10.1126/science.3489291
73. Eberlein TJ, Rosenstein M, Rosenberg SA. Regression of a disseminated syngeneic solid tumor by systemic transfer of lymphoid cells expanded in interleukin 2. J Exp Med (1982) 156(2):385–97. doi: 10.1084/jem.156.2.385
74. Zhou S, Yang H, Zhang J, Wang J, Liang Z, Liu S, et al. Changes in indoleamine 2,3-dioxygenase 1 expression and CD8+ Tumor-infiltrating lymphocytes after neoadjuvant chemoradiation therapy and prognostic significance in esophageal squamous cell carcinoma. Int J Radiat Oncol Biol Phys (2020) 108(1):286–94. doi: 10.1016/j.ijrobp.2020.01.020
75. Kovaleva OV, Rashidova MA, Samoilova DV, Podlesnaya PA, Mochalnikova VV, Gratchev A. Immunosuppressive phenotype of esophagus tumors stroma. Anal Cell Pathol (Amst). (2020) 2020:5424780. doi: 10.1155/2020/5424780
76. Conroy MJ, Kennedy SA, Doyle SL, Hayes B, Kavanagh M, van der Stok EP, et al. A study of the immune infiltrate and patient outcomes in esophageal cancer. Carcinogenesis (2021) 42(3):395–404. doi: 10.1093/carcin/bgaa101
77. Haddad R, Zlotnik O, Goshen-Lago T, Levi M, Brook E, Brenner B, et al. Tumor lymphocyte infiltration is correlated with a favorable tumor regression grade after neoadjuvant treatment for esophageal adenocarcinoma. J Pers Med (2022) 12(4):627. doi: 10.3390/jpm12040627
78. Soeratram TT, Creemers A, Meijer SL, de Boer OJ, Vos W, Hooijer GK, et al. Tumor-immune landscape patterns before and after chemoradiation in resectable esophageal adenocarcinomas. J Pathol (2022) 256(3):282–96. doi: 10.1002/path.5832
79. Nomoto D, Baba Y, Okadome K, Yagi T, Kalikawe R, Kiyozumi Y, et al. Prognostic impact of PD-1 on tumor-infiltrating lymphocytes in 433 resected esophageal cancers. Ann Thorac Surg (2022) 113(1):286–94. doi: 10.1016/j.athoracsur.2021.01.013
80. Noma T, Makino T, Ohshima K, Sugimura K, Miyata H, Honma K, et al. Immunoscore signatures in surgical specimens and tumor-infiltrating lymphocytes in pretreatment biopsy predict treatment efficacy and survival in esophageal cancer. Ann Surg (2023) 277(3):e528–e37. doi: 10.1097/SLA.0000000000005104
81. Wang H, Su C, Li Z, Ma C, Hong L, Li Z, et al. Evaluation of multiple immune cells and patient outcomes in esophageal squamous cell carcinoma. Front Immunol (2023) 14:1091098. doi: 10.3389/fimmu.2023.1091098
82. Lianyuan T, Dianrong X, Chunhui Y, Zhaolai M, Bin J. The predictive value and role of stromal tumor-infiltrating lymphocytes in pancreatic ductal adenocarcinoma (PDAC). Cancer Biol Ther (2018) 19(4):296–305. doi: 10.1080/15384047.2017.1416932
83. Zhang J, Wang YF, Wu B, Zhong ZX, Wang KX, Yang LQ, et al. Intraepithelial attack rather than intratumorally infiltration of CD8+T lymphocytes is a favorable prognostic indicator in pancreatic ductal adenocarcinoma. Curr Mol Med (2017) 17(10):689–98. doi: 10.2174/1566524018666180308115705
84. Tahkola K, Leppänen J, Ahtiainen M, Väyrynen J, Haapasaari KM, Karttunen T, et al. Immune cell score in pancreatic cancer-comparison of hotspot and whole-section techniques. Virchows Arch (2019) 474(6):691–9. doi: 10.1007/s00428-019-02549-1
85. Delayre T, Guilbaud T, Resseguier N, Mamessier E, Rubis M, Moutardier V, et al. Prognostic impact of tumour-infiltrating lymphocytes and cancer-associated fibroblasts in patients with pancreatic adenocarcinoma of the body and tail undergoing resection. Br J Surg (2020) 107(6):720–33. doi: 10.1002/bjs.11434
86. Fraune C, Burandt E, Simon R, Hube-Magg C, Makrypidi-Fraune G, Kluth M, et al. MMR deficiency is homogeneous in pancreatic carcinoma and associated with high density of cd8-positive lymphocytes. Ann Surg Oncol (2020) 27(10):3997–4006. doi: 10.1245/s10434-020-08209-y
87. Başoğlu T, Akar KE, Bağcı P, Akgül Babacan N, Öztürk MA, Öztürk FE, et al. Prognostic value of tissue-resident memory T cells and tumor microenvironmental features in resected pancreatic adenocarcinoma. Balkan Med J (2022) 39(1):12–20. doi: 10.5152/balkanmedj.2021.21122
88. Kim K-J, Yang HK, Kim WH, Kang GH. Combined prognostic effect of PD-L1 expression and immunoscore in microsatellite-unstable advanced gastric cancers. Oncotarget (2017) 8(35):58887. doi: 10.18632/oncotarget.19439
89. Jiang Y, Zhang Q, Hu Y, Li T, Yu J, Zhao L, et al. ImmunoScore signature: A prognostic and predictive tool in gastric cancer. Ann Surg (2018) 267(3):504–13. doi: 10.1097/SLA.0000000000002116
90. Zhang D, He W, Wu C, Tan Y, He Y, Xu B, et al. Scoring system for tumor-infiltrating lymphocytes and its prognostic value for gastric cancer. Front Immunol (2019) 10:71. doi: 10.3389/fimmu.2019.00071
91. Kemi N, Hiltunen N, Väyrynen JP, Pohjanen VM, Helminen O, Junttila A, et al. Immune cell infiltrate and prognosis in gastric cancer. Cancers (Basel) (2020) 12(12):3604. doi: 10.3390/cancers12123604
92. Yun S, Koh J, Nam SK, Kwak Y, Ahn SH, Do Park J, et al. Immunoscore is a strong predictor of survival in the prognosis of stage II/III gastric cancer patients following 5-FU-based adjuvant chemotherapy. Cancer Immunol Immunother. (2021) 70(2):431–41. doi: 10.1007/s00262-020-02694-6
93. Zou W, Zhou ML, Zhang LY, Yang JN, Yang W, Wang YQ, et al. Immune score predicts outcomes of gastric cancer patients treated with adjuvant chemoradiotherapy. J Oncol (2021) 2021:9344124. doi: 10.1155/2021/9344124
94. Ni Z, Xing D, Zhang T, Ding N, Xiang D, Zhao Z, et al. Tumor-infiltrating B cell is associated with the control of progression of gastric cancer. Immunol Res (2021) 69(1):43–52. doi: 10.1007/s12026-020-09167-z
95. Yan H, Chen Y, Yang Z, Li Z, Che X, Xiao J, et al. An immune cell signature is associated with disease-free survival and adjuvant chemosensitivity of patients with resectable gastric cancer. Front Immunol (2020) 11:621623. doi: 10.3389/fimmu.2020.621623
96. Williams DS, Mouradov D, Jorissen RN, Newman MR, Amini E, Nickless DK, et al. Lymphocytic response to tumour and deficient DNA mismatch repair identify subtypes of stage II/III colorectal cancer associated with patient outcomes. Gut (2019) 68(3):465–74. doi: 10.1136/gutjnl-2017-315664
97. Chalabi M, Fanchi LF, Dijkstra KK, Van den Berg JG, Aalbers AG, Sikorska K, et al. Neoadjuvant immunotherapy leads to pathological responses in MMR-proficient and MMR-deficient early-stage colon cancers. Nat Med (2020) 26(4):566–76. doi: 10.1038/s41591-020-0805-8
98. Pagès F, André T, Taieb J, Vernerey D, Henriques J, Borg C, et al. Prognostic and predictive value of the Immunoscore in stage III colon cancer patients treated with oxaliplatin in the prospective IDEA France PRODIGE-GERCOR cohort study. Ann Oncol (2020) 31(7):921–9. doi: 10.1016/j.annonc.2020.03.310
99. Mlecnik B, Bifulco C, Bindea G, Marliot F, Lugli A, Lee JJ, et al. Multicenter international society for immunotherapy of cancer study of the consensus immunoscore for the prediction of survival and response to chemotherapy in stage III colon cancer. J Clin Oncol (2020) 38(31):3638–51. doi: 10.1200/JCO.19.03205
100. Reichling C, Taieb J, Derangere V, Klopfenstein Q, Le Malicot K, Gornet JM, et al. Artificial intelligence-guided tissue analysis combined with immune infiltrate assessment predicts stage III colon cancer outcomes in PETACC08 study. Gut (2020) 69(4):681–90. doi: 10.1136/gutjnl-2019-319292
101. Zou Q, Wang X, Ren D, Hu B, Tang G, Zhang Y, et al. DNA methylation-based signature of CD8+ tumor-infiltrating lymphocytes enables evaluation of immune response and prognosis in colorectal cancer. J Immunother Cancer (2021) 9(9). doi: 10.1136/jitc-2021-002671
102. Kanikarla Marie P, Haymaker C, Parra ER, Kim YU, Lazcano R, Gite S, et al. Pilot clinical trial of perioperative durvalumab and tremelimumab in the treatment of resectable colorectal cancer liver metastases. Clin Cancer Res (2021) 27(11):3039–49. doi: 10.1158/1078-0432.CCR-21-0163
103. Johnson B, Haymaker CL, Parra ER, Soto LMS, Wang X, Thomas JV, et al. Phase II study of durvalumab (anti-PD-L1) and trametinib (MEKi) in microsatellite stable (MSS) metastatic colorectal cancer (mCRC). J Immunother Cancer (2022) 10(8). doi: 10.1136/jitc-2022-005332
104. Kuang C, Park Y, Augustin RC, Lin Y, Hartman DJ, Seigh L, et al. Pembrolizumab plus azacitidine in patients with chemotherapy refractory metastatic colorectal cancer: a single-arm phase 2 trial and correlative biomarker analysis. Clin Epigenetics. (2022) 14(1):3. doi: 10.1186/s13148-021-01226-y
105. Elomaa H, Ahtiainen M, Väyrynen SA, Ogino S, Nowak JA, Friman M, et al. Prognostic significance of spatial and density analysis of T lymphocytes in colorectal cancer. Br J Cancer (2022) 127(3):514–23. doi: 10.1038/s41416-022-01822-6
106. Xin H, Zhou C, Wang G, Zhang J, Liu Y, Li B, et al. Heterogeneity of PD-L1 expression and CD8 lymphocyte infiltration in metastatic colorectal cancer and their prognostic significance. Heliyon (2023) 9(2):e13048. doi: 10.1016/j.heliyon.2023.e13048
107. Wang X, He Q, Shen H, Xia A, Tian W, Yu W, et al. TOX promotes the exhaustion of antitumor CD8(+) T cells by preventing PD1 degradation in hepatocellular carcinoma. J Hepatol (2019) 71(4):731–41. doi: 10.1016/j.jhep.2019.05.015
108. Kim HD, Park S, Jeong S, Lee YJ, Lee H, Kim CG, et al. 4-1BB delineates distinct activation status of exhausted tumor-infiltrating CD8(+) T cells in hepatocellular carcinoma. Hepatology (2020) 71(3):955–71. doi: 10.1002/hep.30881
109. Gao F, Xie K, Xiang Q, Qin Y, Chen P, Wan H, et al. The density of tumor-infiltrating lymphocytes and prognosis in resectable hepatocellular carcinoma: a two-phase study. Aging (Albany NY). (2021) 13(7):9665–78. doi: 10.18632/aging.202710
110. Stulpinas R, Zilenaite-Petrulaitiene D, Rasmusson A, Gulla A, Grigonyte A, Strupas K, et al. Prognostic value of CD8+ Lymphocytes in hepatocellular carcinoma and perineoplastic parenchyma assessed by interface density profiles in liver resection samples. Cancers (Basel) (2023) 15(2):366. doi: 10.3390/cancers15020366
111. Kuwano A, Yada M, Miyazaki Y, Tanaka K, Kurosaka K, Ohishi Y, et al. Tumor−infiltrating CD8(+) T cells as a biomarker for chemotherapy efficacy in unresectable hepatocellular carcinoma. Oncol Lett (2023) 25(6):259. doi: 10.3892/ol.2023.13845
112. Ribas A, Wolchok JD. Cancer immunotherapy using checkpoint blockade. Science (2018) 359(6382):1350–5. doi: 10.1126/science.aar4060
113. Fang P, Zhou J, Liang Z, Yang Y, Luan S, Xiao X, et al. Immunotherapy resistance in esophageal cancer: Possible mechanisms and clinical implications. Front Immunol (2022) 13:975986. doi: 10.3389/fimmu.2022.975986
114. Baba Y, Nomoto D, Okadome K, Ishimoto T, Iwatsuki M, Miyamoto Y, et al. Tumor immune microenvironment and immune checkpoint inhibitors in esophageal squamous cell carcinoma. Cancer Sci (2020) 111(9):3132–41. doi: 10.1111/cas.14541
115. Denkert C, von Minckwitz G, Brase JC, Sinn BV, Gade S, Kronenwett R, et al. Tumor-infiltrating lymphocytes and response to neoadjuvant chemotherapy with or without carboplatin in human epidermal growth factor receptor 2-positive and triple-negative primary breast cancers. J Clin Oncol (2015) 33(9):983–91. doi: 10.1200/JCO.2014.58.1967
116. Huang PW, Chang JW. Immune checkpoint inhibitors win the 2018 Nobel Prize. BioMed J (2019) 42(5):299–306. doi: 10.1016/j.bj.2019.09.002
117. Lei Q, Wang D, Sun K, Wang L, Zhang Y. Resistance mechanisms of anti-PD1/PDL1 therapy in solid tumors. Front Cell Dev Biol (2020) 8:672. doi: 10.3389/fcell.2020.00672
118. Alsaab HO, Sau S, Alzhrani R, Tatiparti K, Bhise K, Kashaw SK, et al. PD-1 and PD-L1 checkpoint signaling inhibition for cancer immunotherapy: mechanism, combinations, and clinical outcome. Front Pharmacol (2017) 8:561. doi: 10.3389/fphar.2017.00561
119. Franzin R, Netti GS, Spadaccino F, Porta C, Gesualdo L, Stallone G, et al. The use of immune checkpoint inhibitors in oncology and the occurrence of AKI: where do we stand? Front Immunol (2020) 11:574271. doi: 10.3389/fimmu.2020.574271
120. Sharma P, Hu-Lieskovan S, Wargo JA, Ribas A. Primary, adaptive, and acquired resistance to cancer immunotherapy. Cell (2017) 168(4):707–23. doi: 10.1016/j.cell.2017.01.017
121. Darb-Esfahani S, Kunze CA, Kulbe H, Sehouli J, Wienert S, Lindner J, et al. Prognostic impact of programmed cell death-1 (PD-1) and PD-ligand 1 (PD-L1) expression in cancer cells and tumor-infiltrating lymphocytes in ovarian high grade serous carcinoma. Oncotarget (2016) 7(2):1486–99. doi: 10.18632/oncotarget.6429
122. Eto S, Yoshikawa K, Nishi M, Higashijima J, Tokunaga T, Nakao T, et al. Programmed cell death protein 1 expression is an independent prognostic factor in gastric cancer after curative resection. Gastric Cancer (2016) 19(2):466–71. doi: 10.1007/s10120-015-0519-7
123. Tang K, Wu YH, Song Y, Yu B. Indoleamine 2,3-dioxygenase 1 (IDO1) inhibitors in clinical trials for cancer immunotherapy. J Hematol Oncol (2021) 14(1):68. doi: 10.1186/s13045-021-01080-8
124. Kiyozumi Y, Baba Y, Okadome K, Yagi T, Ishimoto T, Iwatsuki M, et al. IDO1 expression is associated with immune tolerance and poor prognosis in patients with surgically resected esophageal cancer. Ann Surg (2019) 269(6):1101–8. doi: 10.1097/SLA.0000000000002754
125. Gao Y, Guo W, Geng X, Zhang Y, Zhang G, Qiu B, et al. Prognostic value of tumor-infiltrating lymphocytes in esophageal cancer: an updated meta-analysis of 30 studies with 5,122 patients. Ann Transl Med (2020) 8(13):822. doi: 10.21037/atm-20-151
126. Siegel RL, Miller KD, Jemal A. Cancer statistics, 2019. CA Cancer J Clin (2019) 69(1):7–34. doi: 10.3322/caac.21551
127. Provenzano PP, Cuevas C, Chang AE, Goel VK, Von Hoff DD, Hingorani SR. Enzymatic targeting of the stroma ablates physical barriers to treatment of pancreatic ductal adenocarcinoma. Cancer Cell (2012) 21(3):418–29. doi: 10.1016/j.ccr.2012.01.007
128. Bonaventura P, Shekarian T, Alcazer V, Valladeau-Guilemond J, Valsesia-Wittmann S, Amigorena S, et al. Cold tumors: A therapeutic challenge for immunotherapy. Front Immunol (2019) 10:168. doi: 10.3389/fimmu.2019.00168
129. Benderska N, Ivanovska J, Rau TT, Schulze-Luehrmann J, Mohan S, Chakilam S, et al. DAPK-HSF1 interaction as a positive-feedback mechanism stimulating TNF-induced apoptosis in colorectal cancer cells. J Cell Sci (2014) 127(Pt 24):5273–87. doi: 10.1242/jcs.157024
130. Jamieson NB, Mohamed M, Oien KA, Foulis AK, Dickson EJ, Imrie CW, et al. The relationship between tumor inflammatory cell infiltrate and outcome in patients with pancreatic ductal adenocarcinoma. Ann Surg Oncol (2012) 19(11):3581–90. doi: 10.1245/s10434-012-2370-y
131. Wang LJ, Chiou JT, Lee YC, Chang LS. Docetaxel-triggered SIDT2/NOX4/JNK/HuR signaling axis is associated with TNF-α-mediated apoptosis of cancer cells. Biochem Pharmacol (2022) 195:114865. doi: 10.1016/j.bcp.2021.114865
132. Han J, Khatwani N, Searles TG, Turk MJ, Angeles CV. Memory CD8(+) T cell responses to cancer. Semin Immunol (2020) 49:101435. doi: 10.1016/j.smim.2020.101435
133. Mami-Chouaib F, Blanc C, Corgnac S, Hans S, Malenica I, Granier C, et al. Resident memory T cells, critical components in tumor immunology. J Immunother Cancer (2018) 6(1):87. doi: 10.1186/s40425-018-0399-6
134. Orhan A, Vogelsang RP, Andersen MB, Madsen MT, Hölmich ER, Raskov H, et al. The prognostic value of tumour-infiltrating lymphocytes in pancreatic cancer: a systematic review and meta-analysis. Eur J Cancer (2020) 132:71–84. doi: 10.1016/j.ejca.2020.03.013
135. Li K, Luo H, Huang L, Luo H, Zhu X. Microsatellite instability: a review of what the oncologist should know. Cancer Cell Int (2020) 20(1):16. doi: 10.1186/s12935-019-1091-8
136. De’ Angelis GL, Bottarelli L, Azzoni C, De’ Angelis N, Leandro G, Di Mario F, et al. Microsatellite instability in colorectal cancer. Acta BioMed (2018) 89(9-s):97–101. doi: 10.23750/abm.v89i9-S.7960
137. Picard E, Verschoor CP, Ma GW, Pawelec G. Relationships between immune landscapes, genetic subtypes and responses to immunotherapy in colorectal cancer. Front Immunol (2020) 11:369. doi: 10.3389/fimmu.2020.00369
138. Ruan H, Oike T, Sato H, Ando K, Ohno T. Association between tumor mutational burden, stromal CD8(+) tumor-infiltrating lymphocytes, and clinical factors in cervical cancers treated with radiotherapy. Cancers (Basel) (2023) 15(4):1210. doi: 10.3390/cancers15041210
139. Samstein RM, Lee CH, Shoushtari AN, Hellmann MD, Shen R, Janjigian YY, et al. Tumor mutational load predicts survival after immunotherapy across multiple cancer types. Nat Genet (2019) 51(2):202–6. doi: 10.1038/s41588-018-0312-8
140. Li M, Gao X, Wang X. Identification of tumor mutation burden-associated molecular and clinical features in cancer by analyzing multi-omics data. Front Immunol (2023) 14:1090838. doi: 10.3389/fimmu.2023.1090838
141. Bai Z, Zhou Y, Ye Z, Xiong J, Lan H, Wang F. Tumor-infiltrating lymphocytes in colorectal cancer: the fundamental indication and application on immunotherapy. Front Immunol (2021) 12:808964. doi: 10.3389/fimmu.2021.808964
142. Tahkola K, Mecklin JP, Wirta EV, Ahtiainen M, Helminen O, Böhm J, et al. High immune cell score predicts improved survival in pancreatic cancer. Virchows Arch (2018) 472(4):653–65. doi: 10.1007/s00428-018-2297-1
143. Koizumi W, Narahara H, Hara T, Takagane A, Akiya T, Takagi M, et al. S-1 plus cisplatin versus S-1 alone for first-line treatment of advanced gastric cancer (SPIRITS trial): a phase III trial. Lancet Oncol (2008) 9(3):215–21. doi: 10.1016/S1470-2045(08)70035-4
144. Wilke H, Muro K, Van Cutsem E, Oh SC, Bodoky G, Shimada Y, et al. Ramucirumab plus paclitaxel versus placebo plus paclitaxel in patients with previously treated advanced gastric or gastro-oesophageal junction adenocarcinoma (RAINBOW): a double-blind, randomised phase 3 trial. Lancet Oncol (2014) 15(11):1224–35. doi: 10.1016/S1470-2045(14)70420-6
145. MacCARTY WC. PRINCIPLES OF PROGNOSIS IN CANCER. J Am Med Assoc (1931) 96(1):30–3. doi: 10.1001/jama.1931.02720270032009
146. Jiang X, Li Q, Zhang S, Song C, Zheng P. Long noncoding RNA GIHCG induces cancer progression and chemoresistance and indicates poor prognosis in colorectal cancer. OncoTargets Ther (2019) 12:1059. doi: 10.2147/OTT.S192290
147. Downs-Canner SM, Meier J, Vincent BG, Serody JS. B cell function in the tumor microenvironment. Annu Rev Immunol (2022) 40:169–93. doi: 10.1146/annurev-immunol-101220-015603
148. Zhang N, Cao M, Duan Y, Bai H, Li X, Wang Y. Prognostic role of tumor-infiltrating lymphocytes in gastric cancer: a meta-analysis and experimental validation. Arch Med Sci (2020) 16(5):1092–103. doi: 10.5114/aoms.2019.86101
149. Klintrup K, Mäkinen JM, Kauppila S, Väre PO, Melkko J, Tuominen H, et al. Inflammation and prognosis in colorectal cancer. Eur J Cancer (2005) 41(17):2645–54. doi: 10.1016/j.ejca.2005.07.017
150. Park JH, McMillan DC, Powell AG, Richards CH, Horgan PG, Edwards J, et al. Evaluation of a tumor microenvironment-based prognostic score in primary operable colorectal cancer. Clin Cancer Res (2015) 21(4):882–8. doi: 10.1158/1078-0432.CCR-14-1686
151. Yuan J, Zhao X, Li Y, Yao Q, Jiang L, Feng X, et al. The association between blood indexes and immune cell concentrations in the primary tumor microenvironment predicting survival of immunotherapy in gastric cancer. Cancers (Basel) (2022) 14(15):3608. doi: 10.3390/cancers14153608
152. Naito Y, Saito K, Shiiba K, Ohuchi A, Saigenji K, Nagura H, et al. CD8+ T cells infiltrated within cancer cell nests as a prognostic factor in human colorectal cancer. Cancer Res (1998) 58(16):3491–4.
153. Tiberti S, Catozzi C, Croci O, Ballerini M, Cagnina D, Soriani C, et al. GZMK(high) CD8(+) T effector memory cells are associated with CD15(high) neutrophil abundance in non-metastatic colorectal tumors and predict poor clinical outcome. Nat Commun (2022) 13(1):6752. doi: 10.1038/s41467-022-34467-3
154. Jung M, Lee JA, Yoo SY, Bae JM, Kang GH, Kim JH. Intratumoral spatial heterogeneity of tumor-infiltrating lymphocytes is a significant factor for precisely stratifying prognostic immune subgroups of microsatellite instability-high colorectal carcinomas. Mod Pathol (2022) 35(12):2011–22. doi: 10.1038/s41379-022-01137-0
155. Nazemalhosseini-Mojarad E, Mohammadpour S, Torshizi Esafahani A, Gharib E, Larki P, Moradi A, et al. Intratumoral infiltrating lymphocytes correlate with improved survival in colorectal cancer patients: Independent of oncogenetic features. J Cell Physiol (2019) 234(4):4768–77. doi: 10.1002/jcp.27273
156. André T, Shiu KK, Kim TW, Jensen BV, Jensen LH, Punt C, et al. Pembrolizumab in microsatellite-instability-high advanced colorectal cancer. N Engl J Med (2020) 383(23):2207–18. doi: 10.1056/NEJMoa2017699
157. Buchler T. Microsatellite instability and metastatic colorectal cancer - A clinical perspective. Front Oncol (2022) 12:888181. doi: 10.3389/fonc.2022.888181
158. Baraibar I, Mirallas O, Saoudi N, Ros J, Salvà F, Tabernero J, et al. Combined treatment with immunotherapy-based strategies for MSS metastatic colorectal cancer. Cancers (Basel) (2021) 13(24):6311. doi: 10.3390/cancers13246311
159. Xie Z, Zhou Z, Yang S, Zhang S, Shao B. Epigenetic regulation and therapeutic targets in the tumor microenvironment. Mol Biomed (2023) 4(1):17. doi: 10.1186/s43556-023-00126-2
160. Guinney J, Dienstmann R, Wang X, de Reyniès A, Schlicker A, Soneson C, et al. The consensus molecular subtypes of colorectal cancer. Nat Med (2015) 21(11):1350–6. doi: 10.1038/nm.3967
161. Ros J, Baraibar I, Martini G, Salvà F, Saoudi N, Cuadra-Urteaga JL, et al. The evolving role of consensus molecular subtypes: a step beyond inpatient selection for treatment of colorectal cancer. Curr Treat Options Oncol (2021) 22(12):113. doi: 10.1007/s11864-021-00913-5
162. Sun M, Zhang T, Wang Y, Huang W, Xia L. A novel signature constructed by immune-related lncRNA predicts the immune landscape of colorectal cancer. Front Genet (2021) 12. doi: 10.3389/fgene.2021.695130
163. Hu F, Wang J, Zhang M, Wang S, Zhao L, Yang H, et al. Comprehensive analysis of subtype-specific molecular characteristics of colon cancer: specific genes, driver genes, signaling pathways, and immunotherapy responses. Front Cell Dev Biol (2021) 9:758776. doi: 10.3389/fcell.2021.758776
164. Pagès F, Mlecnik B, Marliot F, Bindea G, Ou FS, Bifulco C, et al. International validation of the consensus Immunoscore for the classification of colon cancer: a prognostic and accuracy study. Lancet (2018) 391(10135):2128–39. doi: 10.1016/S0140-6736(18)30789-X
165. Malka D, Lièvre A, André T, Taïeb J, Ducreux M, Bibeau F. Immune scores in colorectal cancer: Where are we? Eur J Cancer (2020) 140:105–18. doi: 10.1016/j.ejca.2020.08.024
166. Park JH, McMillan DC, Edwards J, Horgan PG, Roxburgh CS. Comparison of the prognostic value of measures of the tumor inflammatory cell infiltrate and tumor-associated stroma in patients with primary operable colorectal cancer. Oncoimmunology (2016) 5(3):e1098801. doi: 10.1080/2162402X.2015.1098801
167. Wirta EV, Seppälä T, Friman M, Väyrynen J, Ahtiainen M, Kautiainen H, et al. Immunoscore in mismatch repair-proficient and -deficient colon cancer. J Pathol Clin Res (2017) 3(3):203–13. doi: 10.1002/cjp2.71
168. Eso Y, Marusawa H. Novel approaches for molecular targeted therapy against hepatocellular carcinoma. Hepatol Res (2018) 48(8):597–607. doi: 10.1111/hepr.13181
169. Zheng X, Jin W, Wang S, Ding H. Progression on the roles and mechanisms of tumor-infiltrating T lymphocytes in patients with hepatocellular carcinoma. Front Immunol (2021) 12:729705. doi: 10.3389/fimmu.2021.729705
170. Forner A, Llovet JM, Bruix J. Hepatocellular carcinoma. Lancet (2012) 379(9822):1245–55. doi: 10.1016/S0140-6736(11)61347-0
171. Sun C, Xu J, Song J, Liu C, Wang J, Weng C, et al. The predictive value of centre tumour CD8+ T cells in patients with hepatocellular carcinoma: comparison with Immunoscore. Oncotarget (2015) 6(34):35602–15. doi: 10.18632/oncotarget.5801
172. Ramzan M, Sturm N, Decaens T, Bioulac-Sage P, Bancel B, Merle P, et al. Liver-infiltrating CD8(+) lymphocytes as prognostic factor for tumour recurrence in hepatitis C virus-related hepatocellular carcinoma. Liver Int (2016) 36(3):434–44. doi: 10.1111/liv.12927
173. Hala S, Asmaa GA, Mervat SS, Nanis SH, Shymaa H. Role of CD8 cytotoxic T lymphocytes in hepatocellular carcinoma: an immunohistochemical study. Med J Cairo Univ (2019) 87(December):4061–9. doi: 10.21608/mjcu.2019.76624
174. Wang WC, Zhang ZQ, Li PP, Ma JY, Chen L, Qian HH, et al. Anti-tumor activity and mechanism of oligoclonal hepatocellular carcinoma tumor-infiltrating lymphocytes in vivo and in vitro. Cancer Biol Ther (2019) 20(9):1187–94. doi: 10.1080/15384047.2019.1599663
175. Dolina JS, Van Braeckel-Budimir N, Thomas GD, Salek-Ardakani S. CD8(+) T cell exhaustion in cancer. Front Immunol (2021) 12:715234. doi: 10.3389/fimmu.2021.715234
176. Hashimoto M, Kamphorst AO, Im SJ, Kissick HT, Pillai RN, Ramalingam SS, et al. CD8 T cell exhaustion in chronic infection and cancer: opportunities for interventions. Annu Rev Med (2018) 69:301–18. doi: 10.1146/annurev-med-012017-043208
177. Wu X, Gu Z, Chen Y, Chen B, Chen W, Weng L, et al. Application of PD-1 blockade in cancer immunotherapy. Comput Struct Biotechnol J (2019) 17:661–74. doi: 10.1016/j.csbj.2019.03.006
178. Khan O, Giles JR, McDonald S, Manne S, Ngiow SF, Patel KP, et al. TOX transcriptionally and epigenetically programs CD8+ T cell exhaustion. Nature (2019) 571(7764):211–8. doi: 10.1038/s41586-019-1325-x
179. Chester C, Sanmamed MF, Wang J, Melero I. Immunotherapy targeting 4-1BB: mechanistic rationale, clinical results, and future strategies. Blood (2018) 131(1):49–57. doi: 10.1182/blood-2017-06-741041
180. Finn RS, Qin S, Ikeda M, Galle PR, Ducreux M, Kim TY, et al. Atezolizumab plus bevacizumab in unresectable hepatocellular carcinoma. N Engl J Med (2020) 382(20):1894–905. doi: 10.1056/NEJMoa1915745
181. Al-Salama ZT, Syed YY, Scott LJ. Lenvatinib: A review in hepatocellular carcinoma. Drugs (2019) 79(6):665–74. doi: 10.1007/s40265-019-01116-x
182. Zamarin D, Holmgaard RB, Subudhi SK, Park JS, Mansour M, Palese P, et al. Localized oncolytic virotherapy overcomes systemic tumor resistance to immune checkpoint blockade immunotherapy. Sci Transl Med (2014) 6(226):226ra32. doi: 10.1126/scitranslmed.3008095
183. Wei J, Ge X, Tang Y, Qian Y, Lu W, Jiang K, et al. An autophagy-related long noncoding RNA signature contributes to poor prognosis in colorectal cancer. J Oncol (2020) 2020. doi: 10.1155/2020/4728947
184. Miyakita H, Sadahiro S, Suzuki T, Chan LF, Ogimi T, Okada K, et al. Tumor-infiltrating lymphocytes in biopsy specimens obtained 7 days after starting chemoradiotherapy for rectal cancer are predictors of the response to chemoradiotherapy. Oncology (2020) 98(12):869–75. doi: 10.1159/000508922
185. Harada Y, Kazama S, Morikawa T, Sonoda H, Ishi H, Emoto S, et al. Clinical significance of CD8(+) and FoxP3(+) tumor-infiltrating lymphocytes and MFG-E8 expression in lower rectal cancer with preoperative chemoradiotherapy. Mol Clin Oncol (2021) 14(5):87. doi: 10.3892/mco.2021.2249
186. Niazi MKK, Parwani AV, Gurcan MN. Digital pathology and artificial intelligence. Lancet Oncol (2019) 20(5):e253–e61. doi: 10.1016/S1470-2045(19)30154-8
187. Wen Z, Wang S, Yang DM, Xie Y, Chen M, Bishop J, et al. Deep learning in digital pathology for personalized treatment plans of cancer patients. Semin Diagn Pathol (2023) 40(2):109–19. doi: 10.1053/j.semdp.2023.02.003
188. Althammer S, Tan TH, Spitzmüller A, Rognoni L, Wiestler T, Herz T, et al. Automated image analysis of NSCLC biopsies to predict response to anti-PD-L1 therapy. J Immunother Cancer (2019) 7(1):121. doi: 10.1186/s40425-019-0589-x
189. Yoo SY, Park HE, Kim JH, Wen X, Jeong S, Cho NY, et al. Whole-slide image analysis reveals quantitative landscape of tumor-immune microenvironment in colorectal cancers. Clin Cancer Res (2020) 26(4):870–81. doi: 10.1158/1078-0432.CCR-19-1159
190. Acs B, Ahmed FS, Gupta S, Wong PF, Gartrell RD, Sarin Pradhan J, et al. An open source automated tumor infiltrating lymphocyte algorithm for prognosis in melanoma. Nat Commun (2019) 10(1):5440. doi: 10.1038/s41467-019-13043-2
191. André P, Denis C, Soulas C, Bourbon-Caillet C, Lopez J, Arnoux T, et al. Anti-NKG2A mAb is a checkpoint inhibitor that promotes anti-tumor immunity by unleashing both T and NK cells. Cell (2018) 175(7):1731–43.e13. doi: 10.1016/j.cell.2018.10.014
192. Zhang Q, Bi J, Zheng X, Chen Y, Wang H, Wu W, et al. Blockade of the checkpoint receptor TIGIT prevents NK cell exhaustion and elicits potent anti-tumor immunity. Nat Immunol (2018) 19(7):723–32. doi: 10.1038/s41590-018-0132-0
193. Amini L, Silbert SK, Maude SL, Nastoupil LJ, Ramos CA, Brentjens RJ, et al. Preparing for CAR T cell therapy: patient selection, bridging therapies and lymphodepletion. Nat Rev Clin Oncol (2022) 19(5):342–55. doi: 10.1038/s41571-022-00607-3
194. Creelan BC, Wang C, Teer JK, Toloza EM, Yao J, Kim S, et al. Tumor-infiltrating lymphocyte treatment for anti-PD-1-resistant metastatic lung cancer: a phase 1 trial. Nat Med (2021) 27(8):1410–8. doi: 10.1038/s41591-021-01462-y
195. Stevanović S, Draper LM, Langhan MM, Campbell TE, Kwong ML, Wunderlich JR, et al. Complete regression of metastatic cervical cancer after treatment with human papillomavirus-targeted tumor-infiltrating T cells. J Clin Oncol (2015) 33(14):1543–50. doi: 10.1200/JCO.2014.58.9093
196. Sarnaik AA, Hamid O, Khushalani NI, Lewis KD, Medina T, Kluger HM, et al. Lifileucel, a tumor-infiltrating lymphocyte therapy, in metastatic melanoma. J Clin Oncol (2021) 39(24):2656–66. doi: 10.1200/JCO.21.00612
197. Tran E, Robbins PF, Lu YC, Prickett TD, Gartner JJ, Jia L, et al. T-cell transfer therapy targeting mutant KRAS in cancer. N Engl J Med (2016) 375(23):2255–62. doi: 10.1056/NEJMoa1609279
198. Tran E, Turcotte S, Gros A, Robbins PF, Lu YC, Dudley ME, et al. Cancer immunotherapy based on mutation-specific CD4+ T cells in a patient with epithelial cancer. Science (2014) 344(6184):641–5. doi: 10.1126/science.1251102
Keywords: gastrointestinal tract cancer, tumor-infiltrating lymphocytes, immunotherapy, immune microenvironment, immune infiltration
Citation: Piroozkhah M, Gholinezhad Y, Piroozkhah M, Shams E and Nazemalhosseini-Mojarad E (2023) The molecular mechanism of actions and clinical utilities of tumor infiltrating lymphocytes in gastrointestinal cancers: a comprehensive review and future prospects toward personalized medicine. Front. Immunol. 14:1298891. doi: 10.3389/fimmu.2023.1298891
Received: 22 September 2023; Accepted: 13 November 2023;
Published: 24 November 2023.
Edited by:
Xinjuan Liu, Capital Medical University, ChinaReviewed by:
Weijian Guo, Fudan University, ChinaCopyright © 2023 Piroozkhah, Gholinezhad, Piroozkhah, Shams and Nazemalhosseini-Mojarad. This is an open-access article distributed under the terms of the Creative Commons Attribution License (CC BY). The use, distribution or reproduction in other forums is permitted, provided the original author(s) and the copyright owner(s) are credited and that the original publication in this journal is cited, in accordance with accepted academic practice. No use, distribution or reproduction is permitted which does not comply with these terms.
*Correspondence: Ehsan Nazemalhosseini-Mojarad, ZWhzYW5tb2phcmFkQGdtYWlsLmNvbQ==
Disclaimer: All claims expressed in this article are solely those of the authors and do not necessarily represent those of their affiliated organizations, or those of the publisher, the editors and the reviewers. Any product that may be evaluated in this article or claim that may be made by its manufacturer is not guaranteed or endorsed by the publisher.
Research integrity at Frontiers
Learn more about the work of our research integrity team to safeguard the quality of each article we publish.