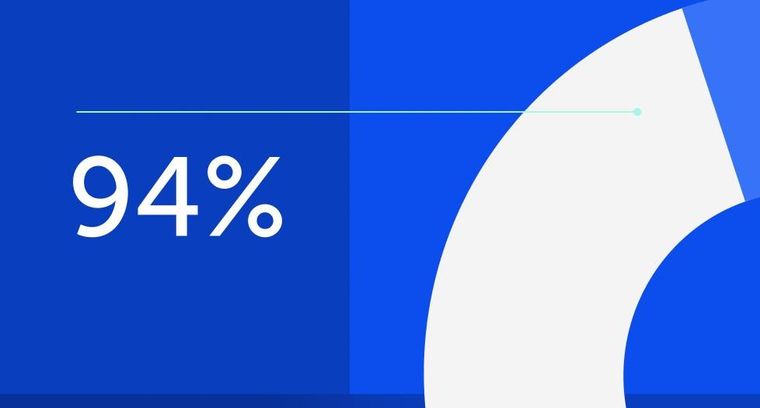
94% of researchers rate our articles as excellent or good
Learn more about the work of our research integrity team to safeguard the quality of each article we publish.
Find out more
REVIEW article
Front. Immunol., 18 December 2023
Sec. Inflammation
Volume 14 - 2023 | https://doi.org/10.3389/fimmu.2023.1296687
This article is part of the Research TopicPolarization of cellular immune response in the context of inflammation and cancerView all 8 articles
Macrophages are highly heterogeneous and plastic, and have two main polarized phenotypes that are determined by their microenvironment, namely pro- and anti-inflammatory macrophages. Activation of pro-inflammatory macrophages is closely associated with metabolic reprogramming, especially that of aerobic glycolysis. Mitochondrial pyruvate dehydrogenase kinase (PDK) negatively regulates pyruvate dehydrogenase complex activity through reversible phosphorylation and further links glycolysis to the tricarboxylic acid cycle and ATP production. PDK is commonly associated with the metabolism and polarization of macrophages in metabolic and inflammatory diseases. This review examines the relationship between PDK and macrophage metabolism and discusses the mechanisms by which PDK regulates macrophage polarization, migration, and inflammatory cytokine secretion in metabolic and inflammatory diseases. Elucidating the relationships between the metabolism and polarization of macrophages under physiological and pathological conditions, as well as the regulatory pathways involved, may provide valuable insights into the etiology and treatment of macrophage-mediated inflammatory diseases.
Macrophages, the main effector cells of the innate immune response, are highly plastic and polarize into two phenotypes based on their microenvironment, namely classically activated (M1) and alternatively activated (M2) macrophages (1–3). The M1 phenotype primarily exerts inflammatory responses by secreting inflammatory factors, such as tumor necrosis factor (TNF)-α, interleukin (IL)-1β, IL-6, nitric oxide (NO), and reactive oxygen intermediates, to remove antigenic foreign substances from the host (4). The M2 phenotype performs inflammatory clearance and tissue repair by secreting IL-10, IL-13, transforming growth factor-β, and other anti-inflammatory molecules (5). Metabolites, oxygen tension, cytokines, inflammatory signals, and other factors of the tissue microenvironment play definitive roles in macrophage metabolic reprogramming, phenotype, and immune function. Moreover, the metabolic state of macrophages may directly or indirectly influence their phenotype and function (6, 7).
The pyruvate dehydrogenase complex (PDC) is one of the main regulators of mammalian metabolic activity; in mitochondria, it consists of pyruvate dehydrogenase (E1), dihydrolipoic acid acetyltransferase (E2), dihydrolipoic acid dehydrogenase (E3), and E3-binding protein (E3BP) (8, 9). The PDC participates in the aerobic oxidation of glucose by catalyzing the oxidative decarboxylation of pyruvate to generate acetyl-CoA and NADH. PDC activity is primarily modulated by the four isozymes of PDK (PDK1–4) (10, 11). PDK1–4 inhibit PDC activity and downstream energy metabolism by phosphorylating specific serine residues (Ser293, Ser300, and Ser232) of the PDC E1α subunit; the PDC E1α subunit can be activated by dephosphorylation, which is catalyzed by pyruvate dehydrogenase phosphatase (10, 11). PDK1–4 sequences are up to 70% homologous and show differences only in their tissue distribution. Although PDK1 is abundant in the heart, skeletal muscle, and pancreatic islets, its expression levels are low in other tissues. PDK2 is relatively widespread and is highly expressed in the heart, kidney, liver, and skeletal muscle but shows lower expression in other tissues. PDK3 is only weakly expressed in some tissues, including the lung, kidney, testis, and brain; PDK4 is highly expressed in the heart, skeletal muscle, liver, kidney, and pancreatic islets (8, 12, 13). Thus, it is established that these four enzymes are distributed differently in various tissues (Figure 1).
Figure 1 Mechanism of PDK-mediated regulation of PDC and the expression of PDKs. (A) Mitochondrial PDKs negatively regulate PDC activity by reversible phosphorylation and further link glycolysis to the tricarboxylic acid cycle. PDC activity is governed by reversible phosphorylation and dephosphorylation of its E1α subunit. The process of PDC inactivation is mediated by PDK phosphorylation of three E1α serine residues (Ser293, Ser300, and Ser232). (B) The expression of PDKs is shown in various tissues. PDK, pyruvate dehydrogenase kinase; PDP, pyruvate dehydrogenase phosphatase; PDC, pyruvate dehydrogenase complex.
Pyruvate decarboxylation is a critical step in cellular metabolism. Under normal physiological conditions, pyruvate dehydrogenase phosphatase activates the PDC, which subsequently catalyzes pyruvate in the tricarboxylic acid cycle to generate large quantities of ATP to satisfy the energy demands of the body. However, when the body is in a starved state, the PDC is deactivated by PDK phosphorylation, resulting in pyruvate decarboxylation disorders and accumulation of pyruvate in the cytoplasm. During starvation, pyruvate can be used as a precursor for glucose synthesis via the gluconeogenic pathway and can participate in the maintenance of blood glucose levels. Compared with that in normal tissues, the expression of PDK isoforms can be differentially upregulated in different tumor tissues under the induction of various factors, and the inhibition of PDC activity causes enhanced glycolysis in tumor cells (14–17). Under certain pathological conditions, the PDC undergoes phosphorylation, followed by inactivation, due to the action of PDK, and pyruvate cannot be completely oxidized or translated into fatty acids. In contrast, the malignant transformation of cells or alteration of metabolic pathways can continually activate PDK (18, 19) and cause disorders affecting glucose energy metabolism; this leads to tumors, diabetes, heart disease, and other related diseases. Thus, regulating the metabolic state of cells by inhibiting PDK activity can be an attractive strategy for the treatment of tumors, diabetes, heart disease, and other related diseases.
Pyruvate dehydrogenase kinase (PDK) is an important enzyme that regulates glucose metabolism. Recent research has shown that PDK controls the modulation of macrophage polarization and affects the pro-inflammatory and anti-inflammatory functions of macrophages. PDK inhibitors can enhance anti-inflammatory responses and effectively improve various chronic inflammatory diseases (20). Herein, we review the regulation of macrophage phenotypes and polarization by PDK and the potential underlying molecular mechanisms.
Macrophages are innate immune cells with strong phagocytic and antigen-presenting functions; they are the most well-known members of the innate immune system and play a critical role in inflammation, tissue repair, tumor surveillance, and systemic metabolism (21–23). Macrophages are differentiated monocytes and are highly plastic. When unpolarized, in the resting state, they are identified as the inactive (M0) phenotype. Upon activation by different stimuli, macrophages can be transformed into two active phenotypes, namely classically activated (M1) and alternatively activated (M2). When stimulated by exogenous lipopolysaccharide (LPS), cytokine interferon (IFN)-γ, viruses, and exogenous DNA, macrophages tend to transition toward the pro-inflammatory phenotype and polarize into the M1 type, exerting pro-inflammatory and microbicidal effects by producing massive amounts of TNF-α, IL-1, NO, and other pro-inflammatory factors (1, 4, 24). Macrophages can be polarized to the M2 type after activation by IL-13 or IL-4, which are produced by immune cells (25, 26). Recent evidence suggests that M2 macrophages can be further subdivided into more subtypes (M2a–d), owing to differences in their activation conditions (27–29). The overall cellular functions of M2 macrophages involve regression of inflammation and wound healing. Thus, macrophage polarization directly affects the pathogenesis of related diseases.
Factors in the local tissue microenvironment, such as cytokines, inflammatory signals, and metabolites, determine the metabolic state of macrophages and their functional plasticity (30). Macrophage plasticity and immune function are closely related to alterations in their metabolism. For example, the “Warburg effect” refers to the theory that tumor cells undergo substantial metabolic reprogramming, consume large amounts of glucose, and produce lactate, even if glycolysis is still ongoing at sufficient oxygen levels (31, 32). A metabolic state similar to that of the “Warburg effect” also exists in activated M1 macrophages, which manifests as a reduction in oxidative phosphorylation (OXPHOS) and ATP synthesis and increased glucose consumption and lactate production. This state is accompanied by high expression of key enzymes involved in glycolysis, such as hexokinase and glucose-6-phosphate dehydrogenase, and high production of reactive oxygen species (33, 34). Accumulating evidence suggests that activated M1 macrophages can provide the energy required by cells for rapid pathogen eradication through the aerobic glycolytic pathway. However, activated M2 macrophages lack the metabolic phenotype of aerobic glycolysis; in M2 macrophages, pyruvate directly enters the tricarboxylic acid cycle and undergoes OXPHOS (30, 35).
Clearly, the switch in glycolytic metabolism can directly affect macrophage polarization and, in turn, their immune functions. Thus, interventions in macrophage metabolism are a promising direction for the amelioration of various inflammatory diseases by modulation of macrophage polarization.
As precursors for macrophages and dendritic cells, monocytes are differentiated from hematopoietic stem cells and develop in the bone marrow. After monocytes exude from blood vessels and migrate to different tissues and organs, they differentiate into macrophages. The PDC/PDK axis controls hypo-responsiveness in monocytes by regulating anabolic and catabolic energetics. In monocytes of septic mice and humans, high expression of PDK1 inactivates the PDC, leading to the formation of hypo-responsiveness in monocytes (36). In septic mice, PDK inhibition with dichloroacetic acid (DCA) leads to subsequent PDC activation, which enhances the mouse immune response, accelerates bacterial clearance, and improves survival. In addition, the production of mitochondrial oxidative bioenergy in isolated hepatocytes and splenocytes also increases (36). Unbiased metabolomics was applied to a severe acute inflammatory monocyte culture model simulating sepsis reprogramming, which showed that DCA reversed the inhibition of PDC activity. Consequently, inflammatory monocytes restored energy anabolism, tricarboxylic acid (TCA) cycle intermediates increased, and itaconate levels decreased (36, 37). However, only primary monocytes were examined in the study, and these new concepts have not been extended to studies on animal models or human inflammation-linked diseases, such as endotoxic shock. Further research is needed to prove that PDC/PDK linked to itaconate-induced tolerance and energy transformation is applicable to macrophages, dendritic cells, adaptive immune cells, or failing organs in humans.
Another study showed that inhibiting PDK expression using mitochondrial SIRT1 enhanced mitochondrial energy metabolism and terminated immune tolerance in monocytes via a previously unknown feedback mechanism (38). These findings indicate that PDK plays a crucial and currently unproven function in the regulation of mitochondrial bioenergetics in inflammatory stress, which may be improved to a certain extent by regulating PDK.
PDK1 restricts the conversion of pyruvate to acetyl-CoA, which enters the TCA cycle and produces NADH, and blocks OXPHOS in mitochondria (39, 40). Recent findings provide substantial evidence of the function of PDK1 in macrophages. For example, Zheng et al. found that PDK1 promotes M1 macrophage polarization and suppresses M2 macrophage activation (41). After PDK1 deletion, LPS-induced aerobic glycolysis was attenuated in macrophages, and the number of M1 macrophages decreased. In addition, the production of M1 macrophage-associated inflammatory mediators IL-6, IL-12, IL-1β, and iNOS was markedly reduced. In comparison, it was observed that the activation of M2 macrophages was enhanced and accompanied by increased mitochondrial respiration during early activation. Furthermore, the expression levels of the M2 macrophage markers Arg1, YM-1, FIZZ-1, and MRC1 increased in response to IL-4-induced activation of macrophages after PDK1 knockdown. However, the level of STAT6 phosphorylation in macrophages did not change. Evidence suggests that PDK1 deletion attenuates glucose oxidation and does not affect the STAT6-mediated intracellular signaling pathway.
Macrophage activation and migration are two key functions contributing to the inflammatory process (42, 43). Recent studies have suggested potential correlations between hypoxia-induced metabolic switch and macrophage migration capacity. In hypoxia, PDK1 expression is increased in a hypoxia inducible factor (HIF)-α-dependent manner (44, 45). When the macrophage-derived cell line, RAW264.7, is subjected to mild hypoxia, the HIF-1α-PDK1 axis induces glycolytic reprogramming by converting pyruvate to lactate. As the HIF-1α-PDK1 axis is blocked by DCA, a chemical inhibitor of PDK, macrophage migration is markedly inhibited under hypoxic conditions; this may be attributed to the association of glycolysis with cytoskeletal actin remodeling. Cells migrate by rapidly depleting ATP in the cytoplasm to remodel cytoskeletal actin filaments (46). The infiltration of inflammatory immune cells, such as macrophages, into the inflamed microenvironment plays an important role in the pathogenesis of sepsis, atherosclerosis, and arthritis. Thus, influencing macrophage migration by regulating macrophage metabolism may be a novel therapeutic strategy for ameliorating inflammatory diseases.
As an important regulator of glucose metabolism, PDK2 is associated with many diseases, such as tumor metabolic reprogramming, hepatic steatosis, and type 2 diabetes, and is closely correlated with immune cell dysregulation and inflammatory responses (47–49). The involvement of PDK2 in hepatic steatosis and cancer, via the regulation of glucose metabolism pathways, has been reported, and the upregulation of PDK2 expression in tumor cells is usually considered an important driver of metabolic rewiring (15, 50).
Macrophages play different roles in pathogen-induced immune responses, tissue repair, and maintenance of homeostasis. Thus, regulating PDK2 to alter macrophage metabolism and influence their activation or polarization has considerable therapeutic potential in cancer and inflammatory diseases (37, 51–53). The B7 family co-stimulatory molecule V-set and immunoglobulin domain-containing 4 (VSIG4) are complementary receptors of the immunoglobulin superfamily that are macrophage-specific surface molecules. VSIG4 mediates pathogen clearance by binding C3b or iC3b of the degradation component of complement C3, and previous studies have found that VSIG4 inhibits macrophage activation and M1 polarization by activating the PI3K/Akt-STAT3 pathway and upregulates PDK2 expression. Meanwhile, the downregulation of PDK2 expression enhances macrophage activation (54). Relevant experimental evidence shows that in PDK2 knocked down RAW264.7 cells or PDK2-/–BMDMs, LPS-induced mitochondrial oxidation and IL-6 and TNF-α levels are increased with high expression of CD40. Simultaneously, RAW264.7 cells with lentiviral overexpression of PDK2 exhibited the opposite effects. Moreover, pharmacological inhibition of PDK2 in vivo has been reported to markedly decrease the mortality of LPS-treated mice, alleviate liver and lung tissue damage, and downregulate pro-inflammatory factor expression. PDK2 regulates macrophage activation and pro-inflammatory factor production in vitro via TLR4-MAPK signaling cascades, which affects the occurrence and development of endotoxic shock (55). The above studies confirmed that PDK2 regulates macrophage polarization via different mechanisms by knocking down the PDK2 gene or modulating PDK2 expression using a lentivirus, which in turn affects the development of inflammation. The experimental protocols and reagents used in these studies may differ, which might explain their differing conclusions. It is worth noting that it may be more beneficial to research the role of PDK2 in activating the TLR4-MAPK signaling pathway in macrophages and inflammatory diseases by using PDK2 gene knockout mice, rather than using PDK2 inhibitors. In addition, the expression of PDK2 increased more than that of other PDK isoenzymes during osteoclast differentiation, and in ovariectomized PDK2-deficient mice, bone loss decreased and the number of osteoclasts reduced. Osteoclast differentiation is inhibited in PDK2-deficient bone marrow-derived monocyte/macrophage lineage cells, and inhibition of PDK2 prevents ovariectomization-induced bone loss (56). Currently, the roles of PDK2 in regulating the activation, proliferation, differentiation, and function of immune cells, including macrophages, dendritic cells, and T and B lymphocytes need further investigation.
As a member of the PDK isoenzyme family, PDK4 regulates mitochondrial pyruvate metabolism (57–59) and is closely associated with the regulation of macrophage activation. HIF-1α is the key factor that regulates the cell response to hypoxia; it is activated under low oxygen conditions and can regulate various physiological activities, such as oxygen metabolism and glycolysis (60). High glucose upregulates the transcriptional activity and protein expression of HIF-1α (61). In atherosclerosis, anaerobic metabolism and pro-inflammatory activation of macrophages is dependent on HIF-1α (62, 63), and a change in HIF-1α protein levels directly affects the expressional level of PDK. Gene silencing of HIF-1α with siRNA decreases PDK4 expression; however, HIF-1α expression is unaffected by PDK4 (64). PDK4 expression is upregulated after HIF-1α directly binds to the promoter region of PDK4. Macrophages stimulated by advanced glycation end-products (AGEs) accelerate the pathological development of atherosclerosis. AGE-bovine serum albumin promotes M1 phenotype polarization by the HIF-1α/PDK4 pathway (65, 66). As a feedback regulator of pyruvate dehydrogenase, PDK4 plays a key function in glucose energy metabolism by participating in different signal transduction cascades (10, 21, 67, 68). Accumulating evidence shows that microRNAs (miRNAs) mediate macrophage activation in some diseases (69–72), and PDK4 plays a role in the regulation of macrophages by miRNAs.
As small and effective post-transcriptional regulators of correlated gene expression, miRNAs regulate the entire genetic network and are involved in multiple cellular processes. MiRNA dysregulation has been implicated in various human diseases (73, 74). MiR-33 affects cellular metabolism by regulating genes related to cholesterol transport, fatty acid metabolism, and insulin signaling (75–78). Cholesterol efflux by macrophages is controlled by ATP production in mitochondria, and the suppression of endogenous miR-33 promotes mitochondrial metabolism, resulting in cholesterol efflux from macrophages and the alleviation of atherosclerosis by targeting PDK4 and other genes (79). MiR-15a-5p plays crucial functions in multiple diseases including endometriosis, sepsis, and acute myocardial infarction (80, 81). Zhu et al. (82) established an animal model of sepsis by cecal ligation perforation. They confirmed the binding of miR-152-3p to PDK4, which resulted in the downregulation of PDK4 expression. MiR-152-3p suppressed LPS/IFN-γ-mediated mitochondrial autophagy of macrophages by directly targeting PDK4, alleviating inflammatory responses (83, 84).
The above studies show that PDK2 or PDK4 alone regulates macrophage activation by affecting cellular metabolism. Recently, the combined effects of PDK2 and PDK4 on macrophage activation and polarization have been investigated. Jha et al. carried out a series of experiments and their findings support that PDK2/PDK4 promotes M1 polarization after activation and that macrophage polarization influences the occurrence and development of chronic inflammatory hyperalgesia (85). Similarly, Min et al. reported that either PDK2/PDK4 gene combined deletion or inhibition of PDK2/PDK4 by small-molecule inhibitors suppresses the inflammatory response of macrophages after inflammatory stimulation (LPS and IFN-γ), downregulates the expression of M1 macrophage markers, affects bactericidal activity, adhesion, and migration of macrophages, alleviates insulin resistance induced by obesity, and improves adipose tissue inflammation (86). Macrophage infiltration induced by hyperglycemia and the release of pro-inflammatory cytokines in the dorsal root ganglia are considerably attenuated in PDK2/PDK4 gene-deficient mice (87). Because macrophages are the main cell population that infiltrate into inflammatory sites, PDK2-/PDK4-regulated macrophage polarization toward the pro-inflammatory M1 type provides a novel mechanism for the interaction between inflammation, chronic pain, and metabolism.
In macrophages, the NLRP3 inflammasome plays a crucial role in the defense against pathogenic invasions (88, 89) Abnormal activation of the NLRP3 inflammasome promotes cardiovascular dysfunction, type 2 diabetes, septicemia, novel coronavirus infection, and other inflammatory diseases (90–95). In septic mice, pharmacological inhibition of PDK blocks the activation of the NLRP3 inflammasome and subsequent caspase-1 activation, resulting in decreased production of IL-1β and other cytokines in macrophages. Furthermore, inhibition of PDK improves mitochondrial oxidative damage and dysfunction caused by the activation of the macrophage NLRP3 inflammasome (96). The data provide novel insights and directions for the application of PDK in the treatment of inflammatory diseases, including sepsis. However, in this study, different inhibitors were used to examine the regulation of metabolic pathways in the activation of the NLRP3 inflammasome; therefore, the interference of non-specific inhibitor effects cannot be excluded from the experiment. Since a pathway-specific inhibitor was used to block each targeted pathway and detect the effect on the NLRP3 inflammasome, there might be a combined effect of multiple pathways on PDK-mediated NLRP3 inflammasome activation.
Other studies suggested that double deletion of PDK2 and PDK4 led to the inhibition of metabolic pathways related to citrate and succinate accumulation (6, 97, 98) and that the intermediates of the mitochondrial TCA cycle, succinate (98, 99), itaconate (100–102), and fumarate (103), have different regulatory effects on the activation of the NLRP3 inflammasome. Owing to the complex regulatory mechanisms of PDK on the NLRP3 inflammasome, the influence of PDK on disease development via the regulation of the macrophage NLRP3 inflammasome needs to be further elucidated.
In cells, metabolic reprogramming is essential for the activation, polarization, and function of macrophages. M1 macrophage energy metabolism is dominated by glycolysis of glucose to lactate, whereas M2 macrophages primarily rely on OXPHOS metabolic pathways. The two distinct macrophage phenotypes have different metabolic pathways and functions in inflammation-related diseases. This implies that targeting macrophage metabolism may be an effective way to combat inflammation-related diseases. PDKs regulate metabolic flexibility and contribute to energy homeostasis by negatively moderating PDC activity. In addition, PDK affects the polarization and immune response of macrophages by regulating the metabolic transformation from OXPHOS to aerobic glycolysis, and therefore plays a pivotal role in metabolic and inflammatory diseases (Figure 2 and Table 1). Furthermore, small-molecule inhibitors of PDK have been identified, some of which are currently being investigated in animal models of metabolic and inflammation-related diseases. Although M1 and M2 macrophages have different ways of metabolizing energy, it remains unclear how these pathways contribute to the development of inflammatory diseases. Thus, further research is essential for demonstrating the relationship between PDK and macrophages and their potential as therapeutic targets for the treatment of metabolic and inflammatory diseases.
Figure 2 Schematic illustration of the mechanism by which PDK participates in regulating the polarization of macrophages. The expression of PDK is upregulated under the induction of different inflammatory factors; PDC activity and oxidative phosphorylation are inhibited and aerobic glycolysis increases. Macrophages polarize into the M1 phenotype and multiple pro-inflammatory genes are activated, thereby promoting the occurrence and development of metabolic and inflammatory diseases. PDK, pyruvate dehydrogenase kinase; PDC, pyruvate dehydrogenase complex; LPS, lipopolysaccharide; CFA, Complete Freund’s adjuvant; HIF-1α, hypoxia-inducible factor-1α; TCA, tricarboxylic acid; OXPHOS, oxidative phosphorylation; IL, interleukin; TGF, transforming growth factor.
CYL: Writing – original draft. CBL: Visualization, Writing – original draft. JZ: Funding acquisition, Supervision, Writing – review & editing. YL: Investigation, Writing – original draft. BJ: Investigation, Writing – original draft. HX: Writing – review & editing. CXL: Funding acquisition, Writing – review & editing.
The author(s) declare financial support was received for the research, authorship, and/or publication of this article. This work was supported by the Research Fund for Lin He’s Academician Workstation of New Medicine and Clinical Translation in Jining Medical University (grant no. JYHL2021MS06, JYHL2022ZD02).
We thank Editage (www.editage.com) for English language editing.
The authors declare that the research was conducted in the absence of any commercial or financial relationships that could be construed as a potential conflict of interest.
All claims expressed in this article are solely those of the authors and do not necessarily represent those of their affiliated organizations, or those of the publisher, the editors and the reviewers. Any product that may be evaluated in this article, or claim that may be made by its manufacturer, is not guaranteed or endorsed by the publisher.
1. Benoit M, Desnues B, Mege JL. Macrophage polarization in bacterial infections. J Immunol (2008) 181(6):3733–9. doi: 10.4049/jimmunol.181.6.3733
2. Gordon S. Alternative activation of macrophages. Nat Rev Immunol (2003) 3(1):23–35. doi: 10.1038/nri978
3. Murray PJ, Wynn TA. Protective and pathogenic functions of macrophage subsets. Nat Rev Immunol (2011) 11(11):723–37. doi: 10.1038/nri3073
4. Orecchioni M, Ghosheh Y, Pramod AB, Ley K. Macrophage polarization: different gene signatures in M1(Lps+) vs. Classically and M2(Lps-) vs. Alternatively activated macrophages. Front Immunol (2019) 10:1084. doi: 10.3389/fimmu.2019.01084
5. Ross EA, Devitt A, Johnson JR. Macrophages: the good, the bad, and the gluttony. Front Immunol (2021) 12:708186. doi: 10.3389/fimmu.2021.708186
6. Pearce EL, Pearce EJ. Metabolic pathways in immune cell activation and quiescence. Immunity (2013) 38(4):633–43. doi: 10.1016/j.immuni.2013.04.005
7. McGettrick AF, O’Neill LA. How metabolism generates signals during innate immunity and inflammation. J Biol Chem (2013) 288(32):22893–8. doi: 10.1074/jbc.R113.486464
8. Klyuyeva A, Tuganova A, Kedishvili N, Popov KM. Tissue-specific kinase expression and activity regulate flux through the pyruvate dehydrogenase complex. J Biol Chem (2019) 294(3):838–51. doi: 10.1074/jbc.RA118.006433
9. Zeng Z, Huang Q, Mao L, Wu J, An S, Chen Z, et al. The pyruvate dehydrogenase complex in sepsis: metabolic regulation and targeted therapy. Front Nutr (2021) 8:783164. doi: 10.3389/fnut.2021.783164
10. Pin F, Novinger LJ, Huot JR, Harris RA, Couch ME, O’Connell TM, et al. PDK4 drives metabolic alterations and muscle atrophy in cancer cachexia. FASEB J (2019) 33(6):7778–90. doi: 10.1096/fj.201802799R
11. Woolbright BL, Rajendran G, Harris RA, Taylor JA 3rd. Metabolic flexibility in cancer: targeting the pyruvate dehydrogenase kinase : pyruvate dehydrogenase axis. Mol Cancer Ther (2019) 18(10):1673–81. doi: 10.1158/1535-7163.MCT-19-0079
12. Atas E, Oberhuber M, Kenner L. The implications of PDK1-4 on tumor energy metabolism, aggressiveness and therapy resistance. Front Oncol (2020) 10:583217. doi: 10.3389/fonc.2020.583217
13. Bowker-Kinley MM, Davis WI, Wu P, Harris RA, Popov KM. Evidence for existence of tissue-specific regulation of the mammalian pyruvate dehydrogenase complex. Biochem J (1998) 329(Pt 1):191–6. doi: 10.1042/bj3290191
14. Ognibene M, Cangelosi D, Morini M, Segalerba D, Bosco MC, Sementa AR, et al. Immunohistochemical analysis of PDK1, PHD3 and HIF-1alpha expression defines the hypoxic status of neuroblastoma tumors. PloS One (2017) 12(11):e0187206. doi: 10.1371/journal.pone.0187206
15. He Z, Li Z, Zhang X, Yin K, Wang W, Xu Z, et al. MiR-422a regulates cellular metabolism and Malignancy by targeting pyruvate dehydrogenase kinase 2 in gastric cancer. Cell Death Dis (2018) 9(5):505. doi: 10.1038/s41419-018-0564-3
16. Woolbright BL, Choudhary D, Mikhalyuk A, Trammel C, Shanmugam S, Abbott E, et al. The role of pyruvate dehydrogenase kinase-4 (PDK4) in bladder cancer and chemoresistance. Mol Cancer Ther (2018) 17(9):2004–12. doi: 10.1158/1535-7163.MCT-18-0063
17. Golias T, Kery M, Radenkovic S, Papandreou I. Microenvironmental control of glucose metabolism in tumors by regulation of pyruvate dehydrogenase. Int J Cancer (2019) 144(4):674–86. doi: 10.1002/ijc.31812
18. Gray LR, Tompkins SC, Taylor EB. Regulation of pyruvate metabolism and human disease. Cell Mol Life Sci (2014) 71(14):2577–604. doi: 10.1007/s00018-013-1539-2
19. Kolobova E, Tuganova A, Boulatnikov I, Popov KM. Regulation of Pyruvate Dehydrogenase Activity through Phosphorylation at Multiple Sites. Biochem J (2001) 358(Pt 1):69–77. doi: 10.1042/0264-6021:3580069
20. Wang S, Liu R, Yu Q, Dong L, Bi Y, Liu G. Metabolic reprogramming of macrophages during infections and cancer. Cancer Lett (2019) 452:14–22. doi: 10.1016/j.canlet.2019.03.015
21. Sica A, Mantovani A. Macrophage plasticity and polarization: in vivo veritas. J Clin Invest (2012) 122(3):787–95. doi: 10.1172/JCI59643
22. Gordon S, Martinez FO. Alternative activation of macrophages: mechanism and functions. Immunity (2010) 32(5):593–604. doi: 10.1016/j.immuni.2010.05.007
23. Boutilier AJ, Elsawa SF. Macrophage polarization states in the tumor microenvironment. Int J Mol Sci (2021) 22(13):6995. doi: 10.3390/ijms22136995
24. Nathan CF, Murray HW, Wiebe ME, Rubin BY. Identification of interferon-gamma as the lymphokine that activates human macrophage oxidative metabolism and antimicrobial activity. J Exp Med (1983) 158(3):670–89. doi: 10.1084/jem.158.3.670
25. Malyshev I, Malyshev Y. Current concept and update of the macrophage plasticity concept: intracellular mechanisms of reprogramming and M3 macrophage “Switch” Phenotype. BioMed Res Int (2015) 2015:341308. doi: 10.1155/2015/341308
26. Shrivastava R, Shukla N. Attributes of alternatively activated (M2) macrophages. Life Sci (2019) 224:222–31. doi: 10.1016/j.lfs.2019.03.062
27. Mantovani A, Sica A, Sozzani S, Allavena P, Vecchi A, Locati M. The chemokine system in diverse forms of macrophage activation and polarization. Trends Immunol (2004) 25(12):677–86. doi: 10.1016/j.it.2004.09.015
28. Roszer T. Understanding the mysterious M2 macrophage through activation markers and effector mechanisms. Mediators Inflammation (2015) 2015:816460. doi: 10.1155/2015/816460
29. Jinnouchi H, Guo L, Sakamoto A, Torii S, Sato Y, Cornelissen A, et al. Diversity of macrophage phenotypes and responses in atherosclerosis. Cell Mol Life Sci (2020) 77(10):1919–32. doi: 10.1007/s00018-019-03371-3
30. Galvan-Pena S, O’Neill LA. Metabolic reprograming in macrophage polarization. Front Immunol (2014) 5:420. doi: 10.3389/fimmu.2014.00420
31. Vaupel P, Schmidberger H, Mayer A. The warburg effect: essential part of metabolic reprogramming and central contributor to cancer progression. Int J Radiat Biol (2019) 95(7):912–9. doi: 10.1080/09553002.2019.1589653
32. Koppenol WH, Bounds PL, Dang CV. Otto warburg’s contributions to current concepts of cancer metabolism. Nat Rev Cancer (2011) 11(5):325–37. doi: 10.1038/nrc3038
33. Hard GC. Some biochemical aspects of the immune macrophage. Br J Exp Pathol (1970) 51(1):97–105.
34. Newsholme P, Gordon S, Newsholme EA. Rates of utilization and fates of glucose, glutamine, pyruvate, fatty acids and ketone bodies by mouse macrophages. Biochem J (1987) 242(3):631–6. doi: 10.1042/bj2420631
35. Ganeshan K, Chawla A. Metabolic regulation of immune responses. Annu Rev Immunol (2014) 32:609–34. doi: 10.1146/annurev-immunol-032713-120236
36. McCall CE, Zabalawi M, Liu T, Martin A, Long DL, Buechler NL, et al. Pyruvate dehydrogenase complex stimulation promotes immunometabolic homeostasis and sepsis survival. JCI Insight (2018) 3(15):e99292. doi: 10.1172/jci.insight.99292
37. Zhu X, Long D, Zabalawi M, Ingram B, Yoza BK, Stacpoole PW, et al. Stimulating pyruvate dehydrogenase complex reduces itaconate levels and enhances tca cycle anabolic bioenergetics in acutely inflamed monocytes. J Leukoc Biol (2020) 107(3):467–84. doi: 10.1002/JLB.3A1119-236R
38. Vachharajani VT, Liu T, Brown CM, Wang X, Buechler NL, Wells JD, et al. SIRT1 inhibition during the hypoinflammatory phenotype of sepsis enhances immunity and improves outcome. J Leukoc Biol (2014) 96(5):785–96. doi: 10.1189/jlb.3MA0114-034RR
39. Roche TE, Hiromasa Y. Pyruvate dehydrogenase kinase regulatory mechanisms and inhibition in treating diabetes, heart ischemia, and cancer. Cell Mol Life Sci (2007) 64(7-8):830–49. doi: 10.1007/s00018-007-6380-z
40. Kaplon J, Zheng L, Meissl K, Chaneton B, Selivanov VA, Mackay G, et al. A key role for mitochondrial gatekeeper pyruvate dehydrogenase in oncogene-induced senescence. Nature (2013) 498(7452):109–12. doi: 10.1038/nature12154
41. Tan Z, Xie N, Cui H, Moellering DR, Abraham E, Thannickal VJ, et al. Pyruvate dehydrogenase kinase 1 participates in macrophage polarization via regulating glucose metabolism. J Immunol (2015) 194(12):6082–9. doi: 10.4049/jimmunol.1402469
42. Peyssonnaux C, Cejudo-Martin P, Doedens A, Zinkernagel AS, Johnson RS, Nizet V. Cutting edge: essential role of hypoxia inducible factor-1alpha in development of lipopolysaccharide-induced sepsis. J Immunol (2007) 178(12):7516–9. doi: 10.4049/jimmunol.178.12.7516
43. MacMicking JD, Nathan C, Hom G, Chartrain N, Fletcher DS, Trumbauer M, et al. Altered responses to bacterial infection and endotoxic shock in mice lacking inducible nitric oxide synthase. Cell (1995) 81(4):641–50. doi: 10.1016/0092-8674(95)90085-3
44. Kim JW, Tchernyshyov I, Semenza GL, Dang CV. HIF-1-mediated expression of pyruvate dehydrogenase kinase: A metabolic switch required for cellular adaptation to hypoxia. Cell Metab (2006) 3(3):177–85. doi: 10.1016/j.cmet.2006.02.002
45. Papandreou I, Cairns RA, Fontana L, Lim AL, Denko NC. HIF-1 mediates adaptation to hypoxia by actively downregulating mitochondrial oxygen consumption. Cell Metab (2006) 3(3):187–97. doi: 10.1016/j.cmet.2006.01.012
46. Semba H, Takeda N, Isagawa T, Sugiura Y, Honda K, Wake M, et al. HIF-1alpha-PDK1 axis-induced active glycolysis plays an essential role in macrophage migratory capacity. Nat Commun (2016) 7:11635. doi: 10.1038/ncomms11635
47. Go Y, Jeong JY, Jeoung NH, Jeon JH, Park BY, Kang HJ, et al. Inhibition of Pyruvate Dehydrogenase Kinase 2 Protects against Hepatic Steatosis through Modulation of Tricarboxylic Acid Cycle Anaplerosis and Ketogenesis. Diabetes (2016) 65(10):2876–87. doi: 10.2337/db16-0223
48. Wu CY, Tso SC, Chuang JL, Gui WJ, Lou M, Sharma G, et al. Targeting hepatic pyruvate dehydrogenase kinases restores insulin signaling and mitigates chrebp-mediated lipogenesis in diet-induced obese mice. Mol Metab (2018) 12:12–24. doi: 10.1016/j.molmet.2018.03.014
49. Bajotto G, Murakami T, Nagasaki M, Qin B, Matsuo Y, Maeda K, et al. Increased expression of hepatic pyruvate dehydrogenase kinases 2 and 4 in young and middle-aged otsuka long-evans tokushima fatty rats: induction by elevated levels of free fatty acids. Metabolism (2006) 55(3):317–23. doi: 10.1016/j.metabol.2005.09.014
50. Sun H, Zhu A, Zhou X, Wang F. Suppression of pyruvate dehydrogenase kinase-2 re-sensitizes paclitaxel-resistant human lung cancer cells to paclitaxel. Oncotarget (2017) 8(32):52642–50. doi: 10.18632/oncotarget.16991
51. Sun WH, Chen YH, Lee HH, Tang YW, Sun KH. PDK1- and PDK2-mediated metabolic reprogramming contributes to the TGFβ1-promoted stem-like properties in head and neck cancer. Cancer Metab (2022) 10(1):23. doi: 10.1186/s40170-022-00300-0
52. Nunes-Xavier CE, Mingo J, Emaldi M, Flem-Karlsen K, Maelandsmo GM, Fodstad O, et al. Heterogeneous expression and subcellular localization of pyruvate dehydrogenase complex in prostate cancer. Front Oncol (2022) 12:873516. doi: 10.3389/fonc.2022.873516
53. Cui L, Cheng Z, Liu Y, Dai Y, Pang Y, Jiao Y, et al. Overexpression of PDK2 and PDK3 reflects poor prognosis in acute myeloid leukemia. Cancer Gene Ther (2020) 27(1-2):15–21. doi: 10.1038/s41417-018-0071-9
54. Li J, Diao B, Guo S, Huang X, Yang C, Feng Z, et al. VSIG4 inhibits proinflammatory macrophage activation by reprogramming mitochondrial pyruvate metabolism. Nat Commun (2017) 8(1):1322. doi: 10.1038/s41467-017-01327-4
55. Li C, Dai J, Liu C, Dong G, Zhang X, Zhang J, et al. Pyruvate dehydrogenase kinase 2 accelerates endotoxin shock by promoting mitogen-activated protein kinase activation. Inflammation (2023) 46(1):418–31. doi: 10.1007/s10753-022-01744-8
56. Lee JM, Kim MJ, Lee SJ, Kim BG, Choi JY, Lee SM, et al. PDK2 deficiency prevents ovariectomy-induced bone loss in mice by regulating the RANKL-NFATc1 pathway during osteoclastogenesis. J Bone Miner Res (2021) 36(3):553–66. doi: 10.1002/jbmr.4202
57. Liu TF, Vachharajani VT, Yoza BK, McCall CE. NAD+-dependent sirtuin 1 and 6 proteins coordinate a switch from glucose to fatty acid oxidation during the acute inflammatory response. J Biol Chem (2012) 287(31):25758–69. doi: 10.1074/jbc.M112.362343
58. Jeoung NH. Pyruvate dehydrogenase kinases: therapeutic targets for diabetes and cancers. Diabetes Metab J (2015) 39(3):188–97. doi: 10.4093/dmj.2015.39.3.188
59. Sugden MC, Holness MJ. Mechanisms underlying regulation of the expression and activities of the mammalian pyruvate dehydrogenase kinases. Arch Physiol Biochem (2006) 112(3):139–49. doi: 10.1080/13813450600935263
60. Semenza GL. Hypoxia-inducible factor 1 and cardiovascular disease. Annu Rev Physiol (2014) 76:39–56. doi: 10.1146/annurev-physiol-021113-170322
61. Yan J, Zhang Z, Shi H. HIF-1 is involved in high glucose-induced paracellular permeability of brain endothelial cells. Cell Mol Life Sci (2012) 69(1):115–28. doi: 10.1007/s00018-011-0731-5
62. Tawakol A, Singh P, Mojena M, Pimentel-Santillana M, Emami H, MacNabb M, et al. HIF-1α and PFKFB3 mediate a tight relationship between proinflammatory activation and anerobic metabolism in atherosclerotic macrophages. Arterioscler Thromb Vasc Biol (2015) 35(6):1463–71. doi: 10.1161/ATVBAHA.115.305551
63. Wang T, Liu H, Lian G, Zhang SY, Wang X, Jiang C. HIF1α-induced glycolysis metabolism is essential to the activation of inflammatory macrophages. Mediators Inflammation (2017) 2017:9029327. doi: 10.1155/2017/9029327
64. Li J, Yang YL, Li LZ, Zhang L, Liu Q, Liu K, et al. Succinate accumulation impairs cardiac pyruvate dehydrogenase activity through GRP91-dependent and independent signaling pathways: therapeutic effects of ginsenoside rb1. Biochim Biophys Acta Mol Basis Dis (2017) 1863(11):2835–47. doi: 10.1016/j.bbadis.2017.07.017
65. Jha MK, Song GJ, Lee MG, Jeoung NH, Go Y, Harris RA, et al. Metabolic connection of inflammatory pain: pivotal role of a pyruvate dehydrogenase kinase-pyruvate dehydrogenase-lactic acid axis. J Neurosci (2015) 35(42):14353–69. doi: 10.1523/JNEUROSCI.1910-15.2015
66. Han X, Ma W, Zhu Y, Sun X, Liu N. Advanced glycation end products enhance macrophage polarization to the M1 phenotype via the HIF-1α/PDK4 pathway. Mol Cell Endocrinol (2020) 514:110878. doi: 10.1016/j.mce.2020.110878
67. Pilegaard H, Neufer PD. Transcriptional regulation of pyruvate dehydrogenase kinase 4 in skeletal muscle during and after exercise. Proc Nutr Soc (2004) 63(2):221–6. doi: 10.1079/pns2004345
68. Park H, Jeoung NH. Inflammation increases pyruvate dehydrogenase kinase 4 (PDK4) expression via the jun N-terminal kinase (JNK) pathway in C2C12 cells. Biochem Biophys Res Commun (2016) 469(4):1049–54. doi: 10.1016/j.bbrc.2015.12.113
69. Ahangari F, Price NL, Malik S, Chioccioli M, Barnthaler T, Adams TS, et al. MicroRNA-33 deficiency in macrophages enhances autophagy, improves mitochondrial homeostasis, and protects against lung fibrosis. JCI Insight (2023) 8(4):e158100. doi: 10.1172/jci.insight.158100
70. Cao Y, Tu Y, Xiong J, Tan S, Luo L, Wu A, et al. MicroRNA-15b-5p encapsulated by M2 macrophage-derived extracellular vesicles promotes gastric cancer metastasis by targeting BRMS1 and suppressing DAPK1 transcription. J Exp Clin Cancer Res (2022) 41(1):152. doi: 10.1186/s13046-022-02356-8
71. Virga F, Cappellesso F, Stijlemans B, Henze AT, Trotta R, Van Audenaerde J, et al. Macrophage miR-210 induction and metabolic reprogramming in response to pathogen interaction boost life-threatening inflammation. Sci Adv (2021) 7(19):eabf0466. doi: 10.1126/sciadv.abf0466
72. Dang CP, Leelahavanichkul A. Over-expression of miR-223 induces M2 macrophage through glycolysis alteration and attenuates LPS-induced sepsis mouse model, the cell-based therapy in sepsis. PloS One (2020) 15(7):e0236038. doi: 10.1371/journal.pone.0236038
73. Garofalo M, Croce CM. MicroRNAs: master regulators as potential therapeutics in cancer. Annu Rev Pharmacol Toxicol (2011) 51:25–43. doi: 10.1146/annurev-pharmtox-010510-100517
74. Gebert LFR, MacRae IJ. Regulation of microRNA function in animals. Nat Rev Mol Cell Biol (2019) 20(1):21–37. doi: 10.1038/s41580-018-0045-7
75. Rayner KJ, Suarez Y, Davalos A, Parathath S, Fitzgerald ML, Tamehiro N, et al. MiR-33 contributes to the regulation of cholesterol homeostasis. Science (2010) 328(5985):1570–3. doi: 10.1126/science.1189862
76. Davalos A, Goedeke L, Smibert P, Ramirez CM, Warrier NP, Andreo U, et al. MiR-33a/b contribute to the regulation of fatty acid metabolism and insulin signaling. Proc Natl Acad Sci U.S.A. (2011) 108(22):9232–7. doi: 10.1073/pnas.1102281108
77. Rayner KJ, Sheedy FJ, Esau CC, Hussain FN, Temel RE, Parathath S, et al. Antagonism of mir-33 in mice promotes reverse cholesterol transport and regression of atherosclerosis. J Clin Invest (2011) 121(7):2921–31. doi: 10.1172/JCI57275
78. Horie T, Baba O, Kuwabara Y, Chujo Y, Watanabe S, Kinoshita M, et al. MicroRNA-33 deficiency reduces the progression of atherosclerotic plaque in apoe-/- mice. J Am Heart Assoc (2012) 1(6):e003376. doi: 10.1161/JAHA.112.003376
79. Karunakaran D, Thrush AB, Nguyen MA, Richards L, Geoffrion M, Singaravelu R, et al. Macrophage mitochondrial energy status regulates cholesterol efflux and is enhanced by anti-miR33 in atherosclerosis. Circ Res (2015) 117(3):266–78. doi: 10.1161/CIRCRESAHA.117.305624
80. Hong X, Li S, Wang J, Zhao Z, Feng Z. Circular RNA circFADS2 is overexpressed in sepsis and suppresses LPS-induced lung cell apoptosis by inhibiting the maturation of miR-15a-5p. BMC Immunol (2021) 22(1):29. doi: 10.1186/s12865-021-00419-7
81. Fan K, Huang W, Qi H, Song C, He C, Liu Y, et al. The egr-1/miR15a-5p/GPX4 axis regulates ferroptosis in acute myocardial infarction. Eur J Pharmacol (2021) 909:174403. doi: 10.1016/j.ejphar.2021.174403
82. Zhu QJ, Wang J, Li Y, Bai ZJ, Guo XB, Pan T. PRKCA promotes mitophagy through the miR-15a-5p/PDK4 axis to relieve sepsis-induced acute lung injury. Infect Immun (2023) 91(1):e0046522. doi: 10.1128/iai.00465-22
83. Zhu L, Shi D, Cao J, Song L. LncRNA CASC2 alleviates sepsis-induced acute lung injury by regulating the miR-152-3p/PDK4 axis. Immunol Invest (2022) 51(5):1257–71. doi: 10.1080/08820139.2021.1928693
84. Wang HR, Guo XY, Liu XY, Song X. Down-regulation of lncRNA CASC9 aggravates sepsis-induced acute lung injury by regulating miR-195-5p/PDK4 axis. Inflammation Res (2020) 69(6):559–68. doi: 10.1007/s00011-020-01316-2
85. Godai K, Hasegawa-Moriyama M, Kurimoto T, Saito T, Yamada T, Sato T, et al. Peripheral administration of morphine attenuates postincisional pain by regulating macrophage polarization through COX-2-dependent pathway. Mol Pain (2014) 10:36. doi: 10.1186/1744-8069-10-36
86. Min BK, Park S, Kang HJ, Kim DW, Ham HJ, Ha CM, et al. Pyruvate dehydrogenase kinase is a metabolic checkpoint for polarization of macrophages to the M1 phenotype. Front Immunol (2019) 10:944. doi: 10.3389/fimmu.2019.00944
87. Rahman MH, Jha MK, Kim JH, Nam Y, Lee MG, Go Y, et al. Pyruvate dehydrogenase kinase-mediated glycolytic metabolic shift in the dorsal root ganglion drives painful diabetic neuropathy. J Biol Chem (2016) 291(11):6011–25. doi: 10.1074/jbc.M115.699215
88. Broz P, Newton K, Lamkanfi M, Mariathasan S, Dixit VM, Monack DM. Redundant roles for inflammasome receptors NLRP3 and NLRC4 in host defense against salmonella. J Exp Med (2010) 207(8):1745–55. doi: 10.1084/jem.20100257
89. Fang R, Tsuchiya K, Kawamura I, Shen Y, Hara H, Sakai S, et al. Critical roles of ASC inflammasomes in caspase-1 activation and host innate resistance to streptococcus pneumoniae infection. J Immunol (2011) 187(9):4890–9. doi: 10.4049/jimmunol.1100381
90. Wu C, Lu W, Zhang Y, Zhang G, Shi X, Hisada Y, et al. Inflammasome activation triggers blood clotting and host death through pyroptosis. Immunity (2019) 50(6):1401–11 e4. doi: 10.1016/j.immuni.2019.04.003
91. Ferreira AC, Soares VC, de Azevedo-Quintanilha IG, Dias S, Fintelman-Rodrigues N, Sacramento CQ, et al. SARS-coV-2 engages inflammasome and pyroptosis in human primary monocytes. Cell Death Discovery (2021) 7(1):43. doi: 10.1038/s41420-021-00428-w
92. Meyers AK, Zhu X. The NLRP3 inflammasome: metabolic regulation and contribution to inflammaging. Cells (2020) 9(8):1808. doi: 10.3390/cells9081808
93. Junqueira C, Crespo A, Ranjbar S, de Lacerda LB, Lewandrowski M, Ingber J, et al. Fcgammar-mediated SARS-coV-2 infection of monocytes activates inflammation. Nature (2022) 606(7914):576–84. doi: 10.1038/s41586-022-04702-4
94. Pan P, Shen M, Yu Z, Ge W, Chen K, Tian M, et al. SARS-coV-2 N protein promotes NLRP3 inflammasome activation to induce hyperinflammation. Nat Commun (2021) 12(1):4664. doi: 10.1038/s41467-021-25015-6
95. Sefik E, Qu R, Junqueira C, Kaffe E, Mirza H, Zhao J, et al. Inflammasome activation in infected macrophages drives COVID-19 pathology. Nature (2022) 606(7914):585–93. doi: 10.1038/s41586-022-04802-1
96. Meyers AK, Wang Z, Han W, Zhao Q, Zabalawi M, Duan L, et al. Pyruvate dehydrogenase kinase supports macrophage NLRP3 inflammasome activation during acute inflammation. Cell Rep (2023) 42(1):111941. doi: 10.1016/j.celrep.2022.111941
97. Pearce EL, Poffenberger MC, Chang CH, Jones RG. Fueling immunity: insights into metabolism and lymphocyte function. Science (2013) 342(6155):1242454. doi: 10.1126/science.1242454
98. Tannahill GM, Curtis AM, Adamik J, Palsson-McDermott EM, McGettrick AF, Goel G, et al. Succinate is an inflammatory signal that induces IL-1β through HIF-1α. Nature (2013) 496(7444):238–42. doi: 10.1038/nature11986
99. Mills EL, Kelly B, Logan A, Costa ASH, Varma M, Bryant CE, et al. Succinate dehydrogenase supports metabolic repurposing of mitochondria to drive inflammatory macrophages. Cell (2016) 167(2):457–70 e13. doi: 10.1016/j.cell.2016.08.064
100. Bambouskova M, Potuckova L, Paulenda T, Kerndl M, Mogilenko DA, Lizotte K, et al. Itaconate confers tolerance to late NLRP3 inflammasome activation. Cell Rep (2021) 34(10):108756. doi: 10.1016/j.celrep.2021.108756
101. Swain A, Bambouskova M, Kim H, Andhey PS, Duncan D, Auclair K, et al. Comparative evaluation of itaconate and its derivatives reveals divergent inflammasome and type I interferon regulation in macrophages. Nat Metab (2020) 2(7):594–602. doi: 10.1038/s42255-020-0210-0
102. Hooftman A, Angiari S, Hester S, Corcoran SE, Runtsch MC, Ling C, et al. The immunomodulatory metabolite itaconate modifies NLRP3 and inhibits inflammasome activation. Cell Metab (2020) 32(3):468–78 e7. doi: 10.1016/j.cmet.2020.07.016
Keywords: pyruvate dehydrogenase kinase, macrophage, polarization, metabolic reprogramming, inflammation
Citation: Li C, Liu C, Zhang J, Lu Y, Jiang B, Xiong H and Li C (2023) Pyruvate dehydrogenase kinase regulates macrophage polarization in metabolic and inflammatory diseases. Front. Immunol. 14:1296687. doi: 10.3389/fimmu.2023.1296687
Received: 19 September 2023; Accepted: 13 November 2023;
Published: 18 December 2023.
Edited by:
Elisa Wirthgen, University Hospital Rostock, GermanyReviewed by:
Sasa R. Vasilijic, Stanford University, United StatesCopyright © 2023 Li, Liu, Zhang, Lu, Jiang, Xiong and Li. This is an open-access article distributed under the terms of the Creative Commons Attribution License (CC BY). The use, distribution or reproduction in other forums is permitted, provided the original author(s) and the copyright owner(s) are credited and that the original publication in this journal is cited, in accordance with accepted academic practice. No use, distribution or reproduction is permitted which does not comply with these terms.
*Correspondence: Huabao Xiong, eGlvbmdoYmxAeWFob28uY29t; Chunxia Li, eGlhY2h1bjExMTNAbWFpbC5qbm1jLmVkdS5jbg==
Disclaimer: All claims expressed in this article are solely those of the authors and do not necessarily represent those of their affiliated organizations, or those of the publisher, the editors and the reviewers. Any product that may be evaluated in this article or claim that may be made by its manufacturer is not guaranteed or endorsed by the publisher.
Research integrity at Frontiers
Learn more about the work of our research integrity team to safeguard the quality of each article we publish.