- Department of Microbiology and Cell Biology, Indian Institute of Science, Bangalore, India
Ovarian cancer (OC) is the third most common gynecological cancer and alone has an emergence rate of approximately 308,069 cases worldwide (2020) with dire survival rates. To put it into perspective, the mortality rate of OC is three times higher than that of breast cancer and it is predicted to only increase significantly by 2040. The primary reasons for such a high rate are that the physical symptoms of OC are detectable only during the advanced phase of the disease when resistance to chemotherapies is high and around 80% of the patients that do indeed respond to chemotherapy initially, show a poor prognosis subsequently. This highlights a pressing need to develop new and effective therapies to tackle advanced OC to improve prognosis and patient survival. A major advance in this direction is the emergence of combination immunotherapeutic methods to boost CD8+ T cell function to tackle OC. In this perspective, we discuss our view of the current state of some of the combination immunotherapies in the treatment of advanced OC, their limitations, and potential approaches toward a safer and more effective response.
Introduction
Cancer immunotherapy has revolutionized treatment for several malignancies (1). Tumor development involves numerous immune evasion strategies of the tumor cells including the establishment of the immunosuppressive microenvironment that inhibits the anti-tumor function of the immune system (2, 3). The immunosuppressive pathways include inhibition of antigen presentation processes and cells (4), poor infiltration and activation of cytotoxic lymphocytes (5), and metabolic reprogramming of the tumor as well as immune cells reinforcing the immunosuppressive niche within the tumor (5). Immunotherapies leverage the immune system, particularly the CD8+ T cells, to counteract the immune evasion and immunosuppressive mechanisms in cancer. In the case of OC, the complex OC-tumor microenvironment (TME) poses a potent suppression program against the host immune system allowing tumor immune evasion and limiting the efficacy of the immunotherapeutic approaches (6).
There are at least two TMEs in OC reinforcing a multilayered immunosuppressive niche: the primary solid tumor and the liquid microenvironment surrounding it called malignant ascites that accumulates with the progression of the disease. Malignant ascites accumulation is one of the hallmarks of OC and it is associated with greater tumor burden, enhanced metastasis to distant organs, and more frequent recurrence of the disease (7). OC cells that are shed from the primary tumor spend most of their lifetime in the ascites microenvironment. OC conditioning of the malignant ascites further provides growth and survival advantages to the cancer cells (8, 9). Though the ascites microenvironment and peritoneal space in general is replete with numerous supposedly anti-tumor immune cells like macrophages, neutrophils, lymphocytes, NK cells, etc. (8, 10, 11), these cells are often dysfunctional and are unable to execute their anti-tumor activity (12–14) (Figure 1A).
Immunosuppression in OC
Various factors in the ovarian tumor microenvironment contribute to the creation of an immunosuppressive milieu. These include both soluble factors as well as factors presented on the cells within the tumor microenvironment. Out of the soluble factors, IL10 is an important anti-inflammatory cytokine that is markedly increased in ascites from OC patients and helps in cancer cell migration and proliferation. In addition to inhibiting CD8+ T cells directly, IL10 activates STAT3 signaling in tumor cells, which promotes cell proliferation correlating with enhanced OC growth and chemoresistance (15–17). The Vascular Endothelial Growth factors (VEGFs) secreted in the ascites, particularly VEGF-A and VEGF-C, have received significant attention as the drivers of immunosuppression. Their levels are markedly elevated in malignant ascites compared to ascites of non-malignant origin. Higher VEGF levels enhance vascular permeability, secretion of matrix metalloproteinases, and angiogenesis, thereby promoting metastasis (18). Damage-Associated Molecular Patterns (DAMPs) are yet other acellular factors mediating immunosuppression. The release of DAMPs activates TME neutrophils and promotes metastasis of OC, although the cellular mechanisms enabling this effect are not fully understood. One potential mechanism is via mitochondrial DAMPs (mtDAMPs) that activate neutrophils triggering the generation of extracellular traps (NETs). Nets can subsequently cause cancer-associated thrombosis and enhanced metastasis. The mtDAMPS also serve as an important prognostic biomarker in OC associated with poor survival rates (19).
At a molecular level, the expression of immune checkpoint receptors (PD-1, CTLA4, LAG3) on T cells and their ligands (PD-L1, CD80/86, FGL1) in cellular TME leads to T cell exhaustion facilitating tumor immune evasion (20) (Figure 1B). Immunosuppression is further reinforced by the network of immunosuppressive cells such as the regulatory T cells (T-regs), tumor-associated macrophages (TAMs), tumor-entrained neutrophils, and myeloid–derived suppressor cells (MDSCs)- all of which suppress T cell responses (Figure 2; Table 1). In advanced cancers, there is also a ‘myeloid bias’ that skews the differentiation of immature myeloid progenitors towards granulocyte-like neutrophils (10). Classically, the Tumor-associated neutrophils (TAN) can exhibit functional polarization either into N1 (anti-tumorigenic) or N2 (pro-tumorigenic) neutrophils in response to cytokine stimulation (21, 22), and the mature circulating neutrophils are not intrinsically suppressive. However, in the case of OC, the mature circulating neutrophils acquire a suppressive phenotype once they are recruited in the ascites microenvironment, and suppress CD8+ T cells by mechanisms distinct from the classical pro-tumor N2 TANs (10). The malignant ascites-entrained suppressive neutrophils in OC although inhibit the proliferation and expansion of CTLs, they do not induce exhaustion of the CTLs. Their suppressive effect is partly mediated by the complement pathway, as inhibition of the C3 component of the complement pathway abrogates the suppressive phenotype (10, 23). Indeed, malignant ascites contains elevated amounts of the C3a component of the complement pathway (24). In addition, the formation of NETs contributes to the establishment of the pre-metastatic niche and subsequent colonization of NET-bound OC cells in the omentum (25), indicating a crucial pro-tumor role of these neutrophils in OC.
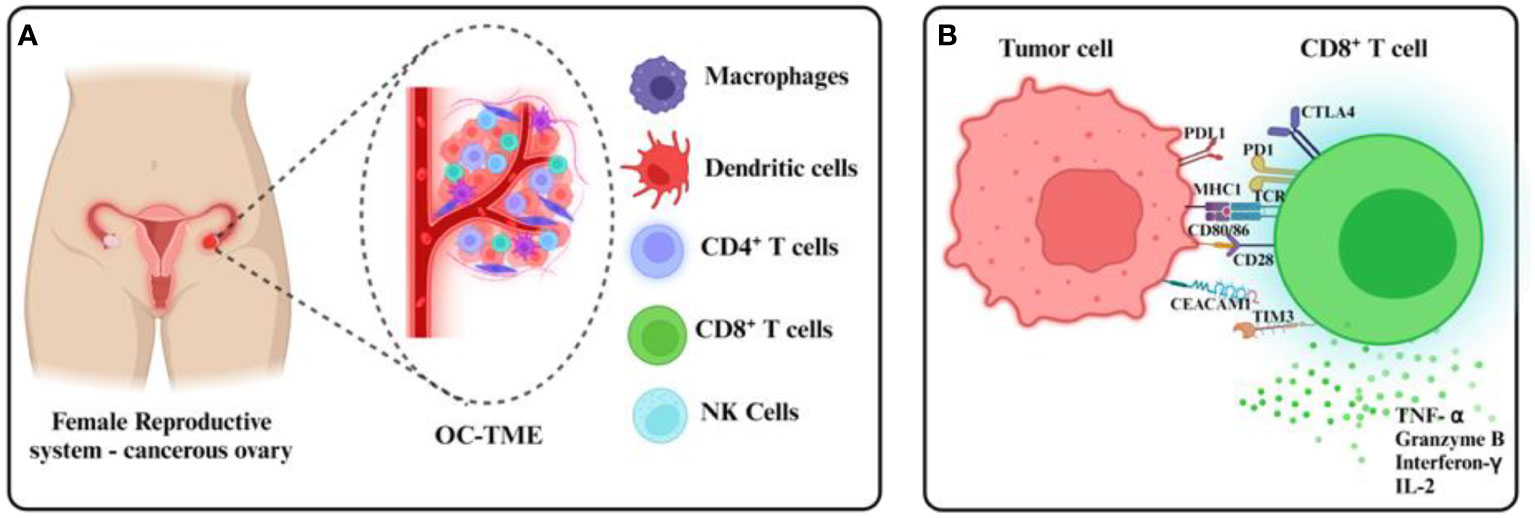
Figure 1 Ovarian cancer TMEs and tumor CD8+ T cell evasion. (A) The ovarian cancer TME comprises immune cellular components within and around the primary tumor in malignant ascites, found usually in dysfunctional states. (B) Immunosuppressive mechanisms including the expression of checkpoint receptors at the CD8+ T cell surface inhibit tumor recognition and cytotoxicity usually mediated by the release of cytolytic granules and effector cytokines.
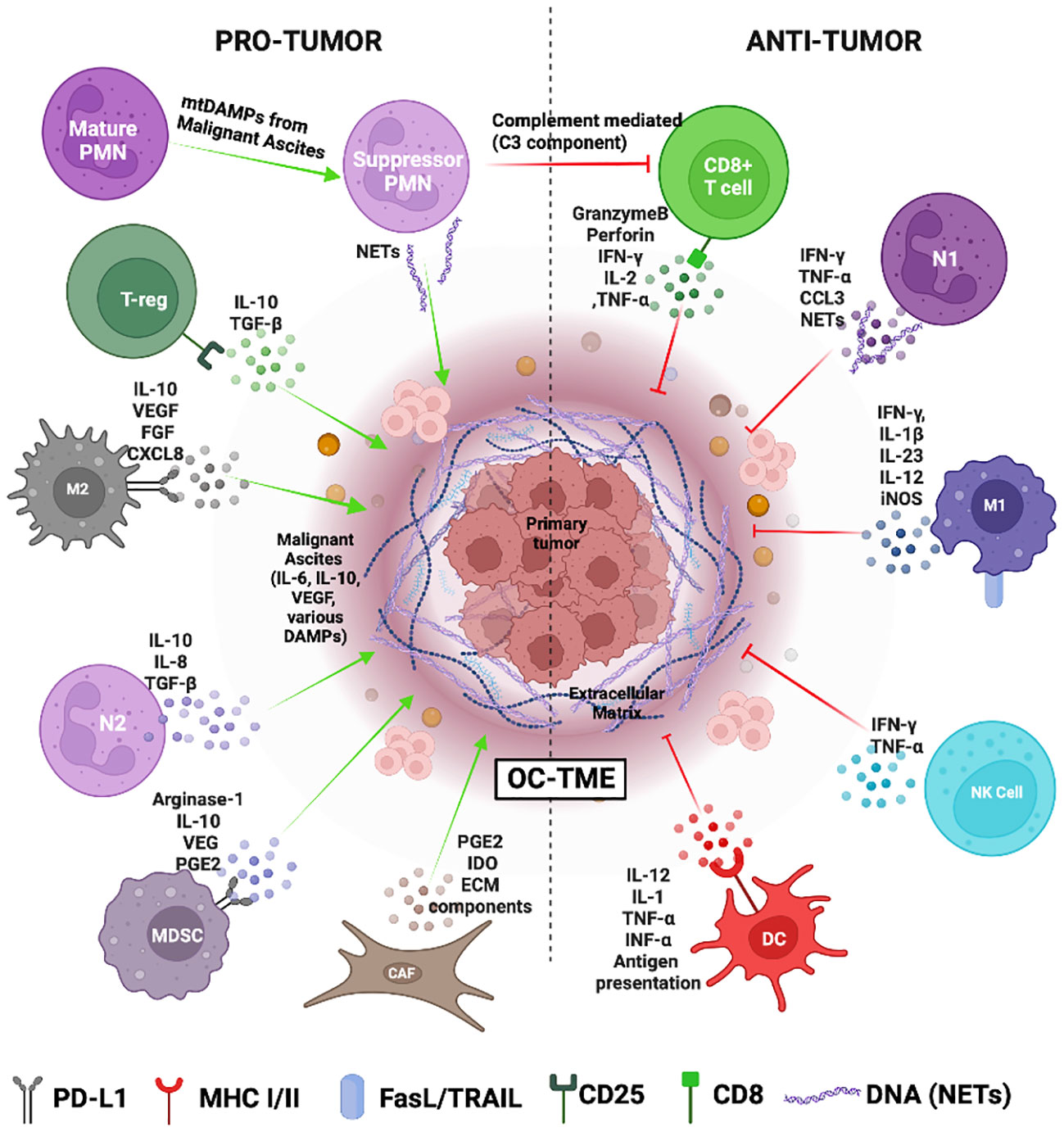
Figure 2 The Ovarian cancer tumor microenvironment (OC-TME). The pro- and anti-tumor function of the immune system in cancer is mediated by diverse immune cell populations that form a complex regulatory network in and around the primary tumor.
The abundance of Tregs in the OC-TME could also contribute to tumor immune privilege and reduced patient survival in OC. Tregs constitute 10 to 17% of T cells in the OC ascites microenvironment and they suppress the anti-tumor CTL effector responses (26). Tumor cells and TAMs recruit CCR4+ Tregs to the TME via secretion of chemokine CCL22. The CCR8-CCL1 and CCR8-CCL18 axis also plays a major role in the migration and infiltration of CD4+CCR8+ Tregs into ovarian tumor tissues that significantly overexpress CCL1 and CCL18 ligands (S. 27). Blocking these receptors on Tregs can potentially impede Treg cell infiltration into the TME and thus help augment CTL functions.
Targeting the aforementioned suppressive cells of OC TME to ameliorate immunosuppression has been a focus of numerous studies (Table 1).
Immunotherapies in OC
Various immunotherapies including checkpoint blockade, CAR-based therapies, and tumor vaccines are currently being tested for OC. However, the success has been mixed thus far as described below:
Several clinical trials on the use of immune checkpoint inhibitors (ICIs) in the treatment of ovarian carcinoma are underway. The PD-1/PD-L1 axis diminishes T-cell activity (28), making PD-1 a promising target for immunotherapy in several cancers (29, 30). In addition, immunosuppressive cells such as Tregs express high levels of PD-1 contributing to the suppressive TME (J.-H. 31, 32). However, anti-PD-1/PD-L1 monotherapy in OC has only shown a modest overall response rate of 9-22% (33). An objective response rate study, KEYNOTE 100, evaluated Pembrolizumab (anti-PD1 antibody) as a single treatment agent in recurrent ovarian carcinoma, eventually reporting a modest response (34). Another trial, JAVELIN Ovarian 100, evaluated Avelumab (anti-PD-L1) in combination with and/or following platinum-based chemotherapy in advanced ovarian carcinoma and reported a marginally promising response, concluding the failure of Avelumab as an individual treatment entity (35). Unfortunately, 95% of the patients in the Ipilimumab monotherapy (NCT 01611558) failed to complete the phase II trial due to toxicity or death (36). A non-randomized interventional clinical trial with a small subset of patients is ongoing which is investigating the efficacy of anti-PD-1, anti-CTLA4, and LAG3 antibodies in metastatic OC patients (37).
The use of combination therapy of Ipilimumab (anti-CTLA4), Nivolumab (anti-PD-1), and Avelumab along with carboplatin/doxorubicin respectively still showed low improvement in progression-free survival (35, 50, 51). Together, while these results uncovered the potential of combination immunotherapies in OC, it is apparent that deeper research into both the choice of checkpoint therapy targets as well as the non-immune targets for more effective chemo-immunotherapies is warranted.
Multicomponent therapies targeting both cancer-derived factors as well as T-cell inhibitory receptors hold great promise in OC. For example, PARP inhibitors worked well in an HR-deficient background by exhibiting synthetic lethality and selective toxicity in OC (52, 53). The combination of PARP inhibitor (Niraparib) with anti-PD-1 (Pembrolizumab) showed a 73% disease control rate in pre-clinical study models of high-grade serous OC (54) An active phase III trial involving the use of Rucaparib (PARP inhibitor) and Nivolumab is currently ongoing (55) and results are awaited. In addition, VEGF-A is an interesting target because not only it is pro-angiogenic but is also known to increase the expression of PD-1 on T-cells and induce proliferation of Tregs, all of which add to the immunosuppressive TME. A combination of anti-PD-1 with Bevacizumab (anti-VEGF) reported a clinical benefit of about 95% in recurrent OC patients with a progression-free survival (PFS) of over 12 months (56). A phase III interventional trial involving 1404 patients administered with Bevacizumab, PARP inhibitor, and anti-PD-1 is ongoing which could lead to promising treatment options for advanced-stage OC patients (57). Some of the other immunotherapy trials involving anti-VEGF treatments are listed in Table 2.
Adoptive immunotherapy has emerged as one of the most popular immunotherapeutic approaches for advanced cancers. Several studies utilizing lymphocytes expressing chimeric antigen receptors (CARs) specific to overexpressed antigens in OC are currently underway. The administration of autologous anti-mesothelin CAR-T cells showed an increased immune response in recurrent OC patients (58). Trials are also ongoing for folate receptor and MUC-16-based CAR-T cell therapy (59–61). In addition to CAR-T, the research into NK-CAR therapies is also gaining momentum for OC immunotherapy. The immunosuppressive microenvironment of OC including the malignant ascites suppresses the expression of NK cell receptor NKp30 which leads to reduced IFN-γ production and NK cell activation. Patient-derived experimental models have reported reduced tumor migration and increased survival benefits in the presence of NK cells (48, 62). Trials targeting the NKG2A inhibitory ligand- commonly expressed on both NK and CD8+ T-cells-using monalizumab in OC, unfortunately, showed a non-significant clinical effect (63). A clinical trial based on the NK-CARs targeting mesothelin in OC is currently underway (64).
Dendritic cells (DC) present tumor antigens to T-cells. DC-based vaccine strategy holds considerable promise in OC. There are about 126 registered OC vaccine trials, of which 24 are based on DC vaccines (65, 66). A small phase I trial with 11 patients who received DC loaded with HER-2 derived peptide reported an overall 3-year survival rate of 90% (67). Furthermore, a DC vaccine with folate receptor-α peptide in advanced OC demonstrated a PFS of 39% without any grade 3 toxicity (68). DC-based therapeutic strategies hold great promise in OC because the OC TME shows increased DC infiltration. Although the infiltrated DC possess weak antigen-presenting ability due to decreased expression of MHC-II and co-stimulatory molecules (69–71), they still offer attractive potential for improved immunotherapies for alleviating the suppressive effect of TME on antigen presentation pathways (Table 2).
Challenges in effective immunotherapy response
Multiple features of OC-TME limit successful immunotherapy response by reinforcing the immunosuppressive TME. The diverse cellular and soluble factors of the primary TME and the malignant ascites pose a significant challenge to designing immunotherapeutic strategies for OC. Understanding the intricate super-and sub-cellular networks that generate and maintain the immunosuppressive microenvironment in both TMEs is crucial for developing targeted immunotherapies with higher efficacy and safety. For example, CCL22 and TGF- β produced by the cancer cells and cancer-associated fibroblasts could recruit Tregs which in turn produce TGF-β and induce the conversion of naïve CD4+ T cells into Tregs (39, 72). Furthermore, the highly glycolytic OC-TME contains lactic acid that drives the metabolism and proliferation of T-regs contributing to a highly immunosuppressive microenvironment (40, 73). The suppressive cells such as MDSCs and TANs are found not just in the primary tumor but also in the peripheral blood and ascites of OC patients and are significantly associated with a shorter survival rate (42). MDSCs secrete arginase-1 which lowers the levels of arginine, an essential amino acid required for effector T-cell differentiation and NK cell survival. Additionally, nitric acid produced by MDSCs activates STAT-1 and downregulates the IFN-γ expression in NK and cytotoxic T-cells. MDSCs also upregulate the expression of PD-L1 leading up to T cell exhaustion (74). The cancer cells secrete M2-type cytokines such as IL-10, VEGF, PDGF, CXCL12, CCL2, and CCL3 that mediate the recruitment of monocytes and M0 macrophages and drive them towards an M2 pro-tumor phenotype observed often in OC tissues (41, 47). M2 macrophages or tumor-associated macrophages (TAMs) secrete CCL22 and draw Tregs and MDSCs into the TME strengthening an immunosuppressive milieu. This is further aided by mature granulocytic neutrophils that acquire an inhibitory phenotype on getting recruited to the ascites microenvironment and suppress the immune cells via complement mediated pathway (10). The OC tumor cells also express checkpoint ligands such as PD-L1 and B7-H4 which drives the inactivation of cytotoxic T cells (26). In addition, OC is considered to be a cold tumor with low median tumor mutational burden at 3.6 mutations/million bases (75). The relatively low mutational burden hinders the identification of neoantigens by immune cells impeding the intended anti-tumor immune response (1, 76).
OC metastasis involves the generation and shedding of malignant tumor spheroids in the peritoneal cavity. These spheroids are unique to OC and are highly specialized entities that pose an additional barrier to successful immunotherapies owing to their distinct metabolic reprogramming compared to the primary tumor and lack of vascularization. The spheroids also play a role in ECM remodeling, the release of immunosuppressive cytokines (IL-6, IL-8)(8), growth factors (VEGF)(77), and immunosuppressive molecules (CA125, Galectins, LPA) that limit effector immune cell infiltration and function (8, 78, 79). Characterizing and targeting the spheroid metabolic vulnerabilities along with enhanced drug delivery strategies to compensate for the lack of vascular supply as well as overcoming the ECM barrier around the spheroids should be integrated into immunotherapies to efficiently break immune suppression and clear tumor burden.
Discussion
Immunotherapy has emerged as a powerful strategy for the treatment of numerous types of cancers, and its application in OC has yielded encouraging results thus far. However, the results are still far from perfect when compared to other malignancies, and the success rate is rather limited. Of the immunotherapy trials conducted yet, the multi-pronged therapeutic approaches targeting multiple aspects of immunosuppression as well as the tumor-derived factors have generated the most promising results and should be built upon to generate more effective and safer therapies. One overlooked aspect of OC immunotherapy is the mechanical heterogeneity that exists within tumor cells that potently changes the T cell immune response (80, 81). In addition, there is substantial deposition of extracellular matrix as well as soluble glycoproteins produced by the tumor cells that impede optimal immune response in OC (82–84). Interaction of cancer cells with collagen results in inhibition of apoptosis of the cancer cells, promotes epithelial-to-mesenchymal transitions and eventually results in chemoresistance (85, 86). As a result, the cancer cells escape peritoneal immunosurveillance, an evasion process further aided by complex intercellular crosstalk between the tumor and immune cells within the malignant ascites (12). The ECM components also inhibit immune infiltration as well as the effector function of immune cells at the tumor site by directly providing cell adhesion and immunomodulatory ligands. Some of these receptors such as DDR-1, DDR-2, and LIAR-1 have been identified and targeted in this regard, improving survival outcomes (87).
A major challenge in immunotherapies that is not unique to OC is stratifying patients who would benefit from immunotherapy. Biomarker identification can serve as a cornerstone in this regard. The MA is rich in cytokines (IL-6, IL-10, VEGF)(15, 88, 89), DAMPs (damage-associated molecular patterns) (10) and other immune-modulatory factors (complement proteins, fibronectin, α-1 acid) (90) that can be used as predictive tools for selective immunotherapeutic strategies. Another biomarker in OC patients is alterations in extracellular matrix protein collagen. For instance, excessive collagen type XI alpha 1 (COL11A1) secretion in OC is involved in enhancing cell invasiveness and tumor formation by activating matrix metalloproteinases (MMP). siRNA-mediated silencing of COL11A1 decreases tumor formation and lung colonization. Targeting COLL11A1 or its effector MMP3 can thus be a viable treatment option in OC (13). Interaction of cancer cells with collagen also results in the inhibition of apoptosis of the cancer cells, promotes epithelial-to-mesenchymal transitions, and eventually results in chemoresistance (85, 86). Homologous DNA repair deficiency is another important biomarker in OC (91). Poly (ADP-ribose) Polymerase (PARP) recruits the homologous DNA repair machinery and prevents the activation of the non-homologous end-joining pathway (91). PARP has also been an attractive target in the recent OC immunotherapy trials. In addition to previously identified biomarkers for OC, new biomarkers are being identified in the malignant ascites that could also serve not only as a prognostic tool but also as a target for designing novel immunotherapies (92). The CD4+/CD8+ ratio is also used as a prognostic marker for monitoring the effectiveness of immunotherapies in OC, and a high CD4+/CD8+ ratio is associated with poor clinical outcomes in OC patients (93, 94). The combination of existing predictive biomarkers such as expression of PD-L1 (34, 95), increased infiltration of CD8+ T cells (M. 96, 97) and increased IFN-γ levels in serum (98), increased expression of CA125 (99), VEGFR3 (98), TGF-β (100) would greatly help with clinical outcome and thereby help in identification of immunotherapy responder population. Importantly, although most of the current immunotherapy strategies are being assessed in the recurrent OC scenario, careful identification of response-associated predictive signatures in patients in the primary treatment stages itself could be immensely beneficial in tackling recurrent OC.
Overall, multicomponent immunotherapies are shaping a new era of ovarian cancer treatment. A deeper understanding of tumor–immune crosstalk, the discovery of more OC-specific checkpoint mechanisms, more specific strategies to target metastatic spheroids, and advanced technologies for early detection as well as patients’ stratification during therapy would bolster the efficacy and safety of immunotherapeutic approaches leading to more effective outcomes.
Data availability statement
The original contributions presented in the study are included in the article/supplementary material. Further inquiries can be directed to the corresponding author.
Author contributions
SaK: Writing – original draft, Writing – review & editing. SA: Writing – review & editing. MK: Visualization, Writing – original draft. PB: Writing – review & editing. SuK: Conceptualization, Writing – original draft, Writing – review & editing.
Funding
The author(s) declare financial support was received for the research, authorship, and/or publication of this article. This work was supported in part by the Indian Institute of Science Startup fund, DST SERB- POWER grant, Infosys award, and Syngene CSR grant (SK). Both SKS and MK are supported via DST SERB- POWER Grant India. Priyobrata Biswas is supported by a fellowship from UGC-NET.
Acknowledgments
The authors wish to acknowledge IISc Health Centre (Dr. Nirmala) and Kidwai Memorial Institute of Oncology (Dr. Pallavi V. R.) for their intellectual input.
Conflict of interest
The authors declare that the research was conducted in the absence of any commercial or financial relationships that could be construed as a potential conflict of interest.
The author(s) declared that they were an editorial board member of Frontiers, at the time of submission. This had no impact on the peer review process and the final decision.
Publisher’s note
All claims expressed in this article are solely those of the authors and do not necessarily represent those of their affiliated organizations, or those of the publisher, the editors and the reviewers. Any product that may be evaluated in this article, or claim that may be made by its manufacturer, is not guaranteed or endorsed by the publisher.
References
1. Yang C, Xia B-R, Zhang Z-C, Zhang Y-J, Lou G, Jin W-L. Immunotherapy for ovarian cancer: adjuvant, combination, and neoadjuvant. Front Immunol (2020) 11:577869. doi: 10.3389/fimmu.2020.577869
2. Labani-Motlagh A, Ashja-Mahdavi M, Loskog A. The tumor microenvironment: A milieu hindering and obstructing antitumor immune responses. Front Immunol (2020) 11:940. doi: 10.3389/fimmu.2020.00940
3. Kim SK, Cho SW. The evasion mechanisms of cancer immunity and drug intervention in the tumor microenvironment. Front Pharmacol (2022) 13:868695. doi: 10.3389/fphar.2022.868695
4. Liu H, Lin J, Zhou W, Moses R, Dai Z, Kossenkov AV, et al. KDM5A inhibits antitumor immune responses through downregulation of the antigen-presentation pathway in ovarian cancer. Cancer Immunol Res (2022) 10(8):1028–38. doi: 10.1158/2326-6066.CIR-22-0088
5. Mistarz A, Winkler M, Battaglia S, Liu S, Hutson A, Rokita H, et al. Reprogramming the tumor microenvironment leverages CD8+ T cell responses to a shared tumor/self antigen in ovarian cancer. Mol Ther Oncolytics (2023) 28:230–48. doi: 10.1016/j.omto.2023.02.002
6. Preston CC, Goode EL, Hartmann LC, Kalli KR, Knutson KL. Immunity and immune suppression in human ovarian cancer. Immunotherapy (2011) 3(4):539–56. doi: 10.2217/imt.11.20
7. Ford CE, Werner B, Hacker NF, Warton K. The untapped potential of ascites in ovarian cancer research and treatment. Br J Cancer (2020) 123(1). doi: 10.1038/s41416-020-0875-x. Article 1.
8. Rickard BP, Conrad C, Sorrin AJ, Ruhi MK, Reader JC, Huang SA, et al. Malignant ascites in ovarian cancer: cellular, acellular, and biophysical determinants of molecular characteristics and therapy response. Cancers (2021) 13(17):4318. doi: 10.3390/cancers13174318
9. Nunes M, Ricardo S. Chemoresistance in ovarian cancer: the role of Malignant ascites. In: Lele S, editor. Ovarian Cancer. Exon Publications: Brisbane, Australia (2022). Available at: http://www.ncbi.nlm.nih.gov/books/NBK585987/.
10. Singel KL, Emmons TR, Khan ANH, Mayor PC, Shen S, Wong JT, et al. Mature neutrophils suppress T cell immunity in ovarian cancer microenvironment. JCI Insight (2019) 4(5):e122311. doi: 10.1172/jci.insight.122311
11. Rakina M, Kazakova A, Villert A, Kolomiets L, Larionova I. Spheroid formation and peritoneal metastasis in ovarian cancer: the role of stromal and immune components. Int J Mol Sci (2022) 23(11). doi: 10.3390/ijms23116215. Article 11.
12. Giuntoli RL, Webb TJ, Zoso A, Rogers O, Diaz-Montes TP, Bristow RE, et al. Ovarian cancer-associated ascites demonstrates altered immune environment: implications for antitumor immunity. Anticancer Res (2009) 29(8):2875–84.
13. Wu Y-H, Chang T-H, Huang Y-F, Huang H-D, Chou C-Y. COL11A1 promotes tumor progression and predicts poor clinical outcome in ovarian cancer. Oncogene (2014) 33(26):3432–40. doi: 10.1038/onc.2013.307. Article 26.
14. Kumar P, Ranmale S, Tongaonkar H, Mania-Pramanik J. Immune profile of blood, tissue and peritoneal fluid: A comparative study in high grade serous epithelial ovarian cancer patients at interval debulking surgery. Vaccines (2022) 10(12):2121. doi: 10.3390/vaccines10122121
15. Kolomeyevskaya N, Eng KH, Khan ANH, Grzankowski KS, Singel KL, Moysich K, et al. Cytokine profiling of ascites at primary surgery identifies an interaction of tumor necrosis factor-α and interleukin-6 in predicting reduced progression-free survival in epithelial ovarian cancer. Gynecologic Oncol (2015) 138(2):352–7. doi: 10.1016/j.ygyno.2015.05.009
16. Browning L, Patel MR, Horvath EB, Tawara K, Jorcyk CL. IL-6 and ovarian cancer: Inflammatory cytokines in promotion of metastasis. Cancer Manage Res (2018) 10:6685–93. doi: 10.2147/CMAR.S179189
17. Lane D, Matte I, Garde-Granger P, Bessette P, Piché A. Ascites IL-10 promotes ovarian cancer cell migration. Cancer Microenviron (2018) 11(2–3):115–24. doi: 10.1007/s12307-018-0215-3
18. Masoumi Moghaddam S, Amini A, Morris DL, Pourgholami MH. Significance of vascular endothelial growth factor in growth and peritoneal dissemination of ovarian cancer. Cancer Metastasis Rev (2012) 31(1):143–62. doi: 10.1007/s10555-011-9337-5
19. Singel KL, Grzankowski KS, Khan ANMNH., Grimm MJ, D’Auria AC, Morrell K, et al. Mitochondrial DNA in the tumour microenvironment activates neutrophils and is associated with worse outcomes in patients with advanced epithelial ovarian cancer. Br J Cancer (2019) 120(2):207–17. doi: 10.1038/s41416-018-0339-8
20. Luo X, Xu J, Yu J, Yi P. Shaping immune responses in the tumor microenvironment of ovarian cancer. Front Immunol (2021) 12:692360. doi: 10.3389/fimmu.2021.692360
21. Masucci MT, Minopoli M, Carriero MV. Tumor associated neutrophils. Their role in tumorigenesis, metastasis, prognosis and therapy. Front Oncol (2019) 9:1146. doi: 10.3389/fonc.2019.01146
22. Yang M, Zhang G, Wang Y, He M, Xu Q, Lu J, et al. Tumour-associated neutrophils orchestrate intratumoural IL-8-driven immune evasion through Jagged2 activation in ovarian cancer. Br J Cancer (2020) 123(9):1404–16. doi: 10.1038/s41416-020-1026-0
23. Emmons TR, Giridharan T, Singel KL, Khan ANH, Ricciuti J, Howard K, et al. Mechanisms driving neutrophil-induced T-cell immunoparalysis in ovarian cancer. Cancer Immunol Res (2021) 9(7):790–810. doi: 10.1158/2326-6066.CIR-20-0922
24. Bjørge L, Hakulinen J, Vintermyr OK, Jarva H, Jensen TS, Iversen OE, et al. Ascitic complement system in ovarian cancer. Br J Cancer (2005) 92(5):895–905. doi: 10.1038/sj.bjc.6602334
25. Lee W, Ko SY, Mohamed MS, Kenny HA, Lengyel E, Naora H. Neutrophils facilitate ovarian cancer premetastatic niche formation in the omentum. J Exp Med (2019) 216(1):176–94. doi: 10.1084/jem.20181170
26. Curiel TJ, Coukos G, Zou L, Alvarez X, Cheng P, Mottram P, et al. Specific recruitment of regulatory T cells in ovarian carcinoma fosters immune privilege and predicts reduced survival. Nat Med (2004) 10(9):942–9. doi: 10.1038/nm1093
27. Liu S, Tao Z, Lou J, Li R, Fu X, Xu J, et al. CD4+CCR8+ Tregs in ovarian cancer: A potential effector Tregs for immune regulation. J Trans Med (2023) 21(1):803. doi: 10.1186/s12967-023-04686-3
28. Webb JR, Milne K, Kroeger DR, Nelson BH. PD-L1 expression is associated with tumor-infiltrating T cells and favorable prognosis in high-grade serous ovarian cancer. Gynecologic Oncol (2016) 141(2):293–302. doi: 10.1016/j.ygyno.2016.03.008. Article 2.
29. McDermott DF, Atkins MB. PD-1 as a potential target in cancer therapy. Cancer Med (2013) 2(5):662–73. doi: 10.1002/cam4.106
30. Alsaab HO, Sau S, Alzhrani R, Tatiparti K, Bhise K, Kashaw SK, et al. PD-1 and PD-L1 checkpoint signaling inhibition for cancer immunotherapy: mechanism, combinations, and clinical outcome. Front Pharmacol (2017) 8:561. doi: 10.3389/fphar.2017.00561
31. Ohue Y, Nishikawa H. Regulatory T (Treg) cells in cancer: Can Treg cells be a new therapeutic target? Cancer Sci (2019) 110(7):2080–9. doi: 10.1111/cas.14069
32. Kim J-H, Kim BS, Lee S-K. Regulatory T cells in tumor microenvironment and approach for anticancer immunotherapy. Immune Network (2020) 20(1):e4. doi: 10.4110/in.2020.20.e4
33. Zhang Y, Cui Q, Xu M, Liu D, Yao S, Chen M. Current advances in PD-1/PD-L1 blockade in recurrent epithelial ovarian cancer. Front Immunol (2022) 13:901772. doi: 10.3389/fimmu.2022.901772
34. Matulonis UA, Shapira-Frommer R, Santin AD, Lisyanskaya AS, Pignata S, Vergote I, et al. Antitumor activity and safety of pembrolizumab in patients with advanced recurrent ovarian cancer: Results from the phase II KEYNOTE-100 study. Ann Oncol (2019) 30(7):1080–7. doi: 10.1093/annonc/mdz135
35. Pfizer. A RANDOMIZED, OPEN-LABEL, MULTICENTER, PHASE 3 STUDY TO EVALUATE THE EFFICACY AND SAFETY OF AVELUMAB (MSB0010718C) IN COMBINATION WITH AND/OR FOLLOWING CHEMOTHERAPY IN PATIENTS WITH PREVIOUSLY UNTREATED EPITHELIAL OVARIAN CANCER JAVELIN OVARIAN 100 (Clinical Trial Registration NCT02718417; Issue NCT02718417) (2020). Available at: https://clinicaltrials.gov/ct2/show/NCT02718417.
36. Bristol-Myers Squibb. A Phase II Safety and Efficacy Study of Ipilimumab Monotherapy in Recurrent Platinum Sensitive Ovarian Cancer Subjects (Clinical Trial Registration NCT01611558; Issue NCT01611558) (2020). Available at: https://clinicaltrials.gov/ct2/show/NCT01611558.
37. Svane IM. T-cell Therapy in Combination With Nivolumab, Relatlimab and Ipilimumab for Patients With Advanced Ovarian-, Fallopian Tube- and Primary Peritoneal Cancer (Clinical Trial Registration NCT04611126; Issue NCT04611126) (2021). Available at: https://clinicaltrials.gov/ct2/show/NCT04611126.
38. Chang D-K, Peterson E, Sun J, Goudie C, Drapkin RI, Liu JF, et al. Anti-CCR4 monoclonal antibody enhances antitumor immunity by modulating tumor-infiltrating Tregs in an ovarian cancer xenograft humanized mouse model. Oncoimmunology (2015) 5(3):e1090075. doi: 10.1080/2162402X.2015.1090075
39. Cassar E, Kartikasari AER, Plebanski M. Regulatory T cells in ovarian carcinogenesis and future therapeutic opportunities. Cancers (2022) 14(22):5488. doi: 10.3390/cancers14225488
40. Kumagai S, Koyama S, Itahashi K, Tanegashima T, Lin Y, Togashi Y, et al. Lactic acid promotes PD-1 expression in regulatory T cells in highly glycolytic tumor microenvironments. Cancer Cell (2022) 40(2):201–18.e9. doi: 10.1016/j.ccell.2022.01.001
41. Truxova I, Cibula D, Spisek R, Fucikova J. Targeting tumor-associated macrophages for successful immunotherapy of ovarian carcinoma. J ImmunoTherapy Cancer (2023) 11(2):e005968. doi: 10.1136/jitc-2022-005968
42. Okła K, Czerwonka A, Wawruszak A, Bobiński M, Bilska M, Tarkowski R, et al. Clinical relevance and immunosuppressive pattern of circulating and infiltrating subsets of myeloid-derived suppressor cells (MDSCs) in epithelial ovarian cancer. Front Immunol (2019) 10:691. doi: 10.3389/fimmu.2019.00691
43. Li T, Liu T, Zhu W, Xie S, Zhao Z, Feng B, et al. Targeting MDSC for immune-checkpoint blockade in cancer immunotherapy: current progress and new prospects. Clin Med Insights Oncol (2021) 15:11795549211035540. doi: 10.1177/11795549211035540
44. Kakarla S, Song X-T, Gottschalk S. Cancer-associated fibroblasts as targets for immunotherapy. Immunotherapy (2012) 4(11):1129–38. doi: 10.2217/imt.12.112
45. Chardin L, Leary A. Immunotherapy in ovarian cancer: thinking beyond PD-1/PD-L1. Front Oncol (2021) 11:795547. doi: 10.3389/fonc.2021.795547
46. Pirš B, Škof E, Smrkolj V, Smrkolj Š. Overview of immune checkpoint inhibitors in gynecological cancer treatment. Cancers (2022) 14(3):631. doi: 10.3390/cancers14030631
47. Ly K, W. As, D. T, W. J, P. W, F. Gn, et al. Intratumoral mediated immunosuppression is prognostic in genetically engineered murine models of glioma and correlates to immunotherapeutic responses. Clin Cancer Research (2010) 16(23):5722–33. doi: 10.1158/1078-0432.CCR-10-1693
48. Sun Y, Yao Z, Zhao Z, Xiao H, Xia M, Zhu X, et al. Natural killer cells inhibit metastasis of ovarian carcinoma cells and show therapeutic effects in a murine model of ovarian cancer. Exp Ther Med (2018) 16(2):1071–8. doi: 10.3892/etm.2018.6342
49. Nersesian S, Glazebrook H, Toulany J, Grantham SR, Boudreau JE. Naturally killing the silent killer: NK cell-based immunotherapy for ovarian cancer. Front Immunol (2019) 10:1782. doi: 10.3389/fimmu.2019.01782
50. AstraZeneca. A Phase Ib Study to Evaluate the Safety and Tolerability of Durvalumab and Tremelimumab in Combination With First-Line Chemotherapy in Patients With Advanced Solid Tumors. (Clinical Trial Registration NCT02658214; Issue NCT02658214) (2020). Available at: https://clinicaltrials.gov/ct2/show/NCT02658214.
51. Pfizer. A PHASE 3, MULTICENTER, RANDOMIZED, OPEN-LABEL STUDY OF AVELUMAB (MSB0010718C) ALONE OR IN COMBINATION WITH PEGYLATED LIPOSOMAL DOXORUBICIN VERSUS PEGYLATED LIPOSOMAL DOXORUBICIN ALONE IN PATIENTS WITH PLATINUM-RESISTANT/REFRACTORY OVARIAN CANCER (Clinical Trial Registration NCT02580058; Issue NCT02580058). clinicaltrials.gov (2022). Available at: https://clinicaltrials.gov/ct2/show/NCT02580058.
52. Miller RE, El-Shakankery KH, Lee J-Y. PARP inhibitors in ovarian cancer: Overcoming resistance with combination strategies. J Gynecologic Oncol (2022) 33(3):e44. doi: 10.3802/jgo.2022.33.e44
53. Hockings H, Miller RE. The role of PARP inhibitor combination therapy in ovarian cancer. Ther Adv Med Oncol (2023) 15:17588359231173184. doi: 10.1177/17588359231173183
54. Konstantinopoulos PA, Waggoner SE, Vidal GA, Mita MM, Fleming GF, Holloway RW, et al. TOPACIO/Keynote-162 (NCT02657889): A phase 1/2 study of niraparib + pembrolizumab in patients (pts) with advanced triple-negative breast cancer or recurrent ovarian cancer (ROC)—Results from ROC cohort. J Clin Oncol (2018) 36(15_suppl):106. doi: 10.1200/JCO.2018.36.15_suppl.106. Article 15_suppl.
55. Clovis Oncology, I. ATHENA (A Multicenter, Randomized, Double-Blind, Placebo- Controlled Phase 3 Study in Ovarian Cancer Patients Evaluating Rucaparib and Nivolumab as Maintenance Treatment Following Response to Front-Line Platinum-Based Chemotherapy) (Clinical Trial Registration NCT03522246; Issue NCT03522246) (2023). Available at: https://clinicaltrials.gov/ct2/show/NCT03522246.
56. Zsiros E, Lynam S, Attwood KM, Wang C, Chilakapati S, Gomez EC, et al. Efficacy and safety of pembrolizumab in combination with bevacizumab and oral metronomic cyclophosphamide in the treatment of recurrent ovarian cancer: A phase 2 nonrandomized clinical trial. JAMA Oncol (2021) 7(1):78–85. doi: 10.1001/jamaoncol.2020.5945. Article 1.
57. AstraZeneca. A Phase III Randomised, Double-Blind, Placebo-Controlled, Multicentre Study of Durvalumab in Combination With Chemotherapy and Bevacizumab, Followed by Maintenance Durvalumab, Bevacizumab and Olaparib in Newly Diagnosed Advanced Ovarian Cancer Patients (DUO-O). (Clinical Trial Registration NCT03737643; Issue NCT03737643) (2023). Available at: https://clinicaltrials.gov/ct2/show/NCT03737643.
58. Chen J, Hu J, Gu L, Ji F, Zhang F, Zhang M, et al. Anti-mesothelin CAR-T immunotherapy in patients with ovarian cancer. Cancer Immunol Immunotherapy (2023) 72(2):409–25. doi: 10.1007/s00262-022-03238-w
59. Kandalaft LE, Powell DJ, Coukos G. A phase I clinical trial of adoptive transfer of folate receptor-alpha redirected autologous T cells for recurrent ovarian cancer. J Trans Med (2012) 10(1). doi: 10.1186/1479-5876-10-157. Article 1.
60. Precigen I. Phase I/Ib Study Evaluating Safety and Efficacy of PRGN-3005 UltraCAR-T® (Autologous CAR T Cells) in Advanced Stage Platinum Resistant Ovarian Cancer Patients (Clinical Trial Registration NCT03907527; Issue NCT03907527). clinicaltrials.gov (2023). Available at: https://clinicaltrials.gov/ct2/show/NCT03907527.
61. Tmunity Therapeutics. A Phase 1 Open-Label, Multi-Center First in Human Study of TnMUC1-Targeted Genetically-Modified Chimeric Antigen Receptor T Cells in Patients With Advanced TnMUC1-Positive Solid Tumors and Multiple Myeloma (Clinical Trial Registration NCT04025216; Issue NCT04025216) (2023). Available at: https://clinicaltrials.gov/ct2/show/NCT04025216.
62. Pesce S, Tabellini G, Cantoni C, Patrizi O, Coltrini D, Rampinelli F, et al. B7-H6-mediated downregulation of NKp30 in NK cells contributes to ovarian carcinoma immune escape. OncoImmunology (2015) 4(4). doi: 10.1080/2162402X.2014.1001224. Article 4.
63. Tinker AV, Hirte HW, Provencher D, Butler M, Ritter H, Tu D, et al. Dose-Ranging and cohort-expansion study of monalizumab (IPH2201) in patients with advanced gynecologic Malignancies: A trial of the Canadian cancer trials group (CCTG): IND221. Clin Cancer Res (2019) 25(20):6052–60. doi: 10.1158/1078-0432.CCR-19-0298. Article 20.
64. Allife Medical Science and Technology Co., Ltd. (2019). Clinical Study on the Safety and Efficacy of Anti-Mesothelin Car NK Cells With Epithelial Ovarian Cancer (Clinical Trial Registration NCT03692637). clinicaltrials.gov. Available at: https://clinicaltrials.gov/ct2/show/NCT03692637
65. Johnson RL, Cummings M, Thangavelu A, Theophilou G, de Jong D, Orsi NM. Barriers to immunotherapy in ovarian cancer: metabolic, genomic, and immune perturbations in the tumour microenvironment. Cancers (2021) 13(24). doi: 10.3390/cancers13246231. Article 24.
66. Caro AA, Deschoemaeker S, Allonsius L, Coosemans A, Laoui D. Dendritic cell vaccines: A promising approach in the fight against ovarian cancer. Cancers (2022) 14(16):4037. doi: 10.3390/cancers14164037
67. Chu CS, Boyer J, Schullery DS, Gimotty PA, Gamerman V, Bender J, et al. Phase I/II randomized trial of dendritic cell vaccination with or without cyclophosphamide for consolidation therapy of advanced ovarian cancer in first or second remission. Cancer Immunology Immunotherapy (2012) 61(5):629–41. doi: 10.1007/s00262-011-1081-8. Article 5.
68. Block MS, Dietz AB, Gustafson MP, Kalli KR, Erskine CL, Youssef B, et al. Th17-inducing autologous dendritic cell vaccination promotes antigen-specific cellular and humoral immunity in ovarian cancer patients. Nat Commun (2020) 11:5173. doi: 10.1038/s41467-020-18962-z
69. Harimoto H, Shimizu M, Nakagawa Y, Nakatsuka K, Wakabayashi A, Sakamoto C, et al. Inactivation of tumor-specific CD8+ CTLs by tumor-infiltrating tolerogenic dendritic cells. Immunol Cell Biol (2013) 91(9):545–55. doi: 10.1038/icb.2013.38
70. Truxova I, Kasikova L, Hensler M, Skapa P, Laco J, Pecen L, et al. Mature dendritic cells correlate with favorable immune infiltrate and improved prognosis in ovarian carcinoma patients. J ImmunoTherapy Cancer (2018) 6(1):139. doi: 10.1186/s40425-018-0446-3
71. Zhang X, He T, Li Y, Chen L, Liu H, Wu Y, et al. Dendritic cell vaccines in ovarian cancer. Front Immunol (2021) 11:613773. doi: 10.3389/fimmu.2020.613773
72. Oh SA, Liu M, Nixon BG, Kang D, Toure A, Bivona M, et al. Foxp3-independent mechanism by which TGF-β controls peripheral T cell tolerance. Proc Natl Acad Sci (2017) 114(36):E7536–44. doi: 10.1073/pnas.1706356114
73. Tondo-Steele K, McLean K. The “Sweet spot” of targeting tumor metabolism in ovarian cancers. Cancers (2022) 14(19):4696. doi: 10.3390/cancers14194696
74. Mabuchi S, Sasano T, Komura N. Targeting myeloid-derived suppressor cells in ovarian cancer. Cells (2021) 10(2). doi: 10.3390/cells10020329. Article 2.
75. Morse CB, Elvin JA, Gay LM, Liao JB. Elevated tumor mutational burden and prolonged clinical response to anti-PD-L1 antibody in platinum-resistant recurrent ovarian cancer. Gynecologic Oncol Rep (2017) 21:78–80. doi: 10.1016/j.gore.2017.06.013
76. Schumacher TN, Schreiber RD. Neoantigens in cancer immunotherapy. Science (2015) 348(6230):69–74. doi: 10.1126/science.aaa4971
77. Horikawa N, Abiko K, Matsumura N, Baba T, Hamanishi J, Yamaguchi K, et al. Anti-VEGF therapy resistance in ovarian cancer is caused by GM-CSF-induced myeloid-derived suppressor cell recruitment. Br J Cancer (2020) 122(6):778–88. doi: 10.1038/s41416-019-0725-x
78. Shimada C, Xu R, Al-Alem L, Stasenko M, Spriggs DR, Rueda BR. Galectins and ovarian cancer. Cancers (2020) 12(6):1421. doi: 10.3390/cancers12061421
79. Chae C-S, Sandoval TA, Hwang S-M, Park ES, Giovanelli P, Awasthi D, et al. Tumor-derived lysophosphatidic acid blunts protective type I interferon responses in ovarian cancer. Cancer Discovery (2022) 12(8):1904–21. doi: 10.1158/2159-8290.CD-21-1181
80. Anderson KG, Stromnes IM, Greenberg PD. Obstacles posed by the tumor microenvironment to T cell activity: A case for synergistic therapies. Cancer Cell (2017) 31(3):311–25. doi: 10.1016/j.ccell.2017.02.008
81. Jiménez-Sánchez A, Memon D, Pourpe S, Veeraraghavan H, Li Y, Vargas HA, et al. Heterogeneous tumor-immune microenvironments among differentially growing metastases in an ovarian cancer patient. Cell (2017) 170(5):927–38.e20. doi: 10.1016/j.cell.2017.07.025
82. Cho A, Howell VM, Colvin EK. The extracellular matrix in epithelial ovarian cancer – A piece of a puzzle. Front Oncol (2015) 5:245. doi: 10.3389/fonc.2015.00245
83. Patankar MS, Gubbels JAA, Felder M, Connor JP. The immunomodulating roles of glycoproteins in epithelial ovarian cancer. Front Biosci (2015) 4.
84. Puttock EH, Tyler EJ, Manni M, Maniati E, Butterworth C, Burger Ramos M, et al. Extracellular matrix educates an immunoregulatory tumor macrophage phenotype found in ovarian cancer metastasis. Nat Commun (2023) 14(1). doi: 10.1038/s41467-023-38093-5. Article 1.
85. Januchowski R, Świerczewska M, Sterzyńska K, Wojtowicz K, Nowicki M, Zabel M. Increased expression of several collagen genes is associated with drug resistance in ovarian cancer cell lines. J Cancer (2016) 7(10):1295. doi: 10.7150/jca.15371
86. Liu M, Zhang X, Long C, Xu H, Cheng X, Chang J, et al. Collagen-based three-dimensional culture microenvironment promotes epithelial to mesenchymal transition and drug resistance of human ovarian cancer in vitro. RSC Adv (2018) 8(16):8910. doi: 10.1039/c7ra13742g
87. Flies DB, Langermann S, Jensen C, Karsdal MA, Willumsen N. Regulation of tumor immunity and immunotherapy by the tumor collagen extracellular matrix. Front Immunol (2023) 14:1199513. doi: 10.3389/fimmu.2023.1199513
88. Lane D, Matte I, Garde-Granger P, Laplante C, Carignan A, Rancourt C, et al. Inflammation-regulating factors in ascites as predictive biomarkers of drug resistance and progression-free survival in serous epithelial ovarian cancers. BMC Cancer (2015) 15(1). doi: 10.1186/s12885-015-1511-7. Article 1.
89. Alain P. IL-6 and VEGF-A, novel prognostic biomarkers for ovarian cancer? J Lab Precis Med (2018) 3:48–8. doi: 10.21037/jlpm.2018.05.01
90. Kolev M, Das M, Gerber M, Baver S, Deschatelets P, Markiewski MM. Inside-out of complement in cancer. Front Immunol (2022) 13:931273. doi: 10.3389/fimmu.2022.931273
91. Konstantinopoulos PA, Lheureux S, Moore KN. PARP inhibitors for ovarian cancer: current indications, future combinations, and novel assets in development to target DNA damage repair. Am Soc Clin Oncol Educ Book (2020) 40:e116–31. doi: 10.1200/EDBK_288015
92. Kuk C, Kulasingam V, Gunawardana CG, Smith CR, Batruch I, Diamandis EP. Mining the Ovarian Cancer Ascites Proteome for Potential Ovarian Cancer Biomarkers*□S. Mol Cell Proteomics (2009) 8(4).661–9
93. Markowska J, Lacki JK, Jaroszewski J, Wiktorowicz K. The usefulness of CD4/CD8 ratio evaluation in monitoring of ovarian cancer patients. Eur J Gynaecological Oncol (1995) 16(1):54–8. doi: 10.2741/405
94. Preston CC, Maurer MJ, Oberg AL, Visscher DW, Kalli KR, Hartmann LC, et al. The ratios of CD8+ T cells to CD4+CD25+ FOXP3+ and FOXP3- T cells correlate with poor clinical outcome in human serous ovarian cancer. PLoS One (2013) 8(11):e80063. doi: 10.1371/journal.pone.0080063
95. Nishio S, Matsumoto K, Takehara K, Kawamura N, Hasegawa K, Takeshima N, et al. Pembrolizumab monotherapy in Japanese patients with advanced ovarian cancer: Subgroup analysis from the KEYNOTE-100. Cancer Sci (2020) 111(4):1324–32. doi: 10.1111/cas.14340
96. Liu M, Silva-Sanchez A, Randall TD, Meza-Perez S. Specialized immune responses in the peritoneal cavity and omentum. J Leukocyte Biol (2021) 109(4):717–29. doi: 10.1002/JLB.5MIR0720-271RR
97. Pujade-Lauraine E, Fujiwara K, Ledermann JA, Oza AM, Kristeleit R, Ray-Coquard I-L, et al. Avelumab alone or in combination with chemotherapy versus chemotherapy alone in platinum-resistant or platinum-refractory ovarian cancer (JAVELIN Ovarian 200): An open-label, three-arm, randomised, phase 3 study. Lancet Oncol (2021) 22(7):1034–46. doi: 10.1016/S1470-2045(21)00216-3
98. Lampert EJ, Zimmer A, Padget M, Cimino-Mathews A, Nair J, Liu Y, et al. Combination of PARP inhibitor olaparib, and PD-L1 inhibitor durvalumab, in recurrent ovarian cancer: A proof-of-concept phase 2 study. Clin Cancer Research (2020) 26(16):4268–79. doi: 10.1158/1078-0432.CCR-20-0056
99. Disis ML, Taylor MH, Kelly K, Beck JT, Gordon M, Moore KM, et al. Efficacy and safety of avelumab for patients with recurrent or refractory ovarian cancer. JAMA Oncol (2019) 5(3):Article 3. doi: 10.1001/jamaoncol.2018.6258
Keywords: ovarian cancer, immunotherapy, immunosuppression, T cells, checkpoint inhibitors
Citation: Kumar S, Acharya S, Karthikeyan M, Biswas P and Kumari S (2024) Limitations and potential of immunotherapy in ovarian cancer. Front. Immunol. 14:1292166. doi: 10.3389/fimmu.2023.1292166
Received: 11 September 2023; Accepted: 15 December 2023;
Published: 09 January 2024.
Edited by:
Ramandeep Rattan, Henry Ford Health System, United StatesReviewed by:
Anm Nazmul Hasan Khan, University at Buffalo, United StatesCopyright © 2024 Kumar, Acharya, Karthikeyan, Biswas and Kumari. This is an open-access article distributed under the terms of the Creative Commons Attribution License (CC BY). The use, distribution or reproduction in other forums is permitted, provided the original author(s) and the copyright owner(s) are credited and that the original publication in this journal is cited, in accordance with accepted academic practice. No use, distribution or reproduction is permitted which does not comply with these terms.
*Correspondence: Sudha Kumari, c3VkaGFrbUBpaXNjLmFjLmlu
†These authors have contributed equally to this work