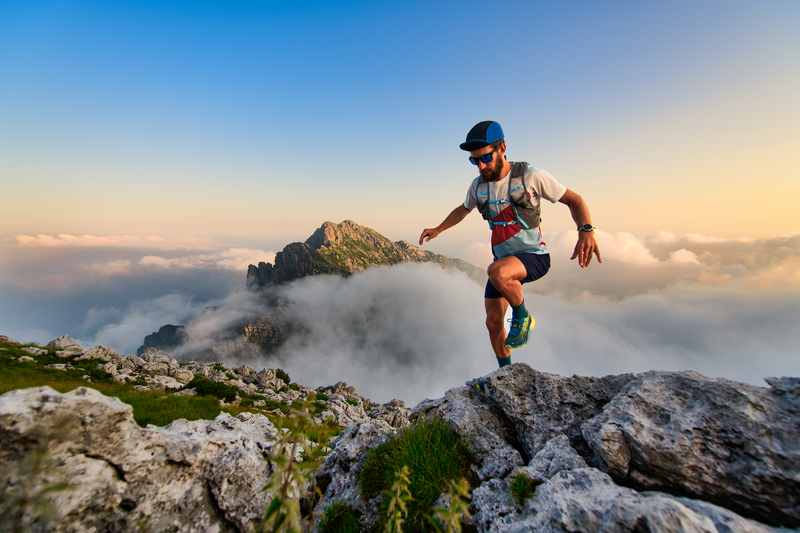
95% of researchers rate our articles as excellent or good
Learn more about the work of our research integrity team to safeguard the quality of each article we publish.
Find out more
REVIEW article
Front. Immunol. , 29 November 2023
Sec. Multiple Sclerosis and Neuroimmunology
Volume 14 - 2023 | https://doi.org/10.3389/fimmu.2023.1290666
Disease-modifying therapies for relapsing multiple sclerosis reduce relapse rates by suppressing peripheral immune cells but have limited efficacy in progressive forms of the disease where cells in the central nervous system play a critical role. To our knowledge, alemtuzumab, fumarates (dimethyl, diroximel, and monomethyl), glatiramer acetates, interferons, mitoxantrone, natalizumab, ocrelizumab, ofatumumab, and teriflunomide are either limited to the periphery or insufficiently studied to confirm direct central nervous system effects in participants with multiple sclerosis. In contrast, cladribine and sphingosine 1-phosphate receptor modulators (fingolimod, ozanimod, ponesimod, and siponimod) are central nervous system-penetrant and could have beneficial direct central nervous system properties.
As of 2020, approximately 2.8 million people were recorded to have multiple sclerosis (MS) worldwide (global prevalence: 35.9 [95% confidence interval, 35.87–35.95] per 100,000 persons), with women being twice as likely as men to develop MS (1), highlighting the need for effective disease-modifying therapies (DMTs). The typical progression of MS is shown in Figure 1 (2). Current treatment strategies aim at reducing relapse risk, decreasing the formation of new brain lesions, and slowing disability progression (3, 4). Most currently available DMTs are immunomodulatory or immunosuppressive agents that act predominantly on peripheral immune responses (3, 4). While immunotherapies reduce relapse activity, most therapies for relapsing MS (RMS) have limited efficacy in treating progressive forms of MS, and targeting peripheral inflammation is not sufficient to slow disease progression (5).
Figure 1 Typical clinical course of MS. The clinical course of MS is heterogenous, but RRMS is the most common subtype characterized by an initial episode of neurological symptoms (CIS) followed by periods of remissions and relapses. Some patients have an initial diagnosis of primary progressive MS, which is associated with progressive decline. Over time, improvement during remissions wane, and disability accumulates, with most patients developing secondary progressive MS. In secondary progressive MS, neurodegeneration is accompanied with a decline in brain volume. CIS, clinically isolated syndrome; MS, multiple sclerosis; RRMS, relapsing-remitting multiple sclerosis. Source: Adapted (colors revised, text edited/moved, and content removed) from Håkansson et al. (2). under Creative Commons Attribution NonCommercial 4.0 International License (https://creativecommons.org/licenses/by-nc/4.0/).
As MS progresses, resident central nervous system (CNS) cells play a more dominant, critical role in MS pathogenesis; thus, the ability of DMTs to cross the blood-brain barrier (BBB) and directly target sites of inflammation, demyelination, and neuroaxonal damage could have additional benefits over therapies that are limited to the periphery (6, 7). For DMTs to be effective in progressive MS, they must also target chronic CNS inflammation (5). The purpose of this narrative review is to provide an overview of therapies currently approved by the US Food and Drug Administration (FDA) and European Medicines Agency (EMA) for MS treatment that penetrate the CNS and to elaborate on future perspectives in the MS therapeutic landscape.
DMTs approved by the FDA and EMA as of February 2023 were included in this review. Pivotal phase 3 clinical trials in adults with RMS or progressive MS subtypes were assessed for alemtuzumab (CARE-MS I and CARE-MS II) (8, 9), dimethyl fumarate (CONFIRM and DEFINE) (10, 11), glatiramer acetate (Copolymer 1 Multiple Sclerosis Study Group) (12), interferons (IFN) (IFNB Multiple Sclerosis Study Group, Multiple Sclerosis Collaborative Research Group, European Study Group on IFN β-1b in Secondary Progressive MS, CHAMPS, EVIDENCE, BENEFIT, and ADVANCE) (13–20), mitoxantrone (MIMS) (21), natalizumab (AFFIRM) (22), ocrelizumab (OPERA I, OPERA II, and ORATORIO) (23, 24), ofatumumab (ASCLEPIOS I and ASCLEPIOS II) (25), teriflunomide (TEMSO and TOWER) (26, 27), cladribine (CLARITY) (28), fingolimod (FREEDOMS, FREEDOMS II, and TRANSFORMS) (29–31), ozanimod (SUNBEAM and RADIANCE) (32, 33), ponesimod (OPTIMUM) (34), and siponimod (EXPAND) (35).
Given the relatively recent FDA and EMA approvals of a subset of DMTs assessed here (e.g., ofatumumab, ozanimod, ponesimod), additional phase 3 clinical trials, post hoc analyses of phase 3 clinical trials, or open-label extension trials that enrolled phase 3 participants were included if relevant data were not reported in the pivotal phase 3 clinical trial publications or there were no significant differences in any measures of brain volume, cognition, or disability against a comparator in ≥1 pivotal study. Other relevant references were retrieved from October 2021 to July 2022 by searching PubMed for the generic name of each DMT combined with each of the following search terms individually: astrocyte, brain volume/atrophy, evoked potential latency, magnetic transfer ratio, microglia, multiple sclerosis, myelination, myelin water fraction, neuron, oligodendrocyte, oligodendrocyte progenitor/precursor cells, phase 3, remyelination, slowly expanding lesions (SELs), and smoldering lesions. All references were included at the authors’ discretion.
DMTs were categorized as direct CNS agents based on mechanism of action, ability to traverse the BBB, and compilation of data from the literature search (positive phase 3 clinical trial data for ≥2 measures of neurodegeneration that a direct-acting DMT would be expected to affect [brain volume, cognition, and/or disability]). Assessments of remyelination, most notably magnetic transfer ratio (MTR)/myelin water fraction (MWF) (36), were also evaluated. Ultimately, a DMT was considered limited to the periphery based on data suggesting limited ability or inability to cross the BBB, unavailable or nonsignificant phase 3 clinical trial data for the clinical outcomes assessed during the development of this narrative review, and the authors’ expert opinion.
The BBB normally controls immune cell infiltrates in the CNS by limiting cell entry to only cell subsets required for immune surveillance (37). During periods of neuroinflammation, the BBB is impaired and immune cell entry into the CNS becomes less regulated (37). Early RMS pathophysiology is characterized by BBB injury, influx of peripheral immune cells into the CNS, and the development of focal inflammatory demyelinating lesions in grey and white matter [Figure 2A (38)] (4, 39). Breakdown of the BBB in patients with MS may therefore facilitate proinflammatory cell migration into the CNS (40). During relapses, which are most prominent early in MS, peripheral innate and adaptive immune cells, such as CD4+ and CD8+ T cells, B cells, and myeloid cells, accumulate in the perivascular space and infiltrate the CNS parenchyma (4, 41–44).
Figure 2 Pathophysiology of multiple sclerosis. (A) In the early stages of MS, relapses coincide CNS inflammation and demyelination that are typically discernible as white matter lesions via MRI. Peripheral immune cell infiltration can occur from meningeal blood vessels (across the BBB), the subarachnoid space, or the choroid plexus (across the blood-CSF barrier). These infiltrates may then accumulate in perivascular spaces and enter the CNS parenchyma, and along with activated CNS-resident microglia and astrocytes, promote demyelination and oligodendrocyte and neuroaxonal injury through direct contact-dependent mechanisms or via soluble inflammatory and neurotoxic mediators. (B) In the later stages of MS, progressive neurological decline is accompanied by CNS atrophy. Immune cell infiltration is dampened, but CNS inflammation persists. Meningeal tertiary lymphoid-like structures can also contribute to late-stage inflammation in SPMS. Irrespective of MS subtype, CNS-resident innate immune cells may contribute to chronic inflammation. Astrocytes produce ligands and factors that can promote microglial recruitment and activation while also preventing remyelination at the sites of neuroaxonal injury by inhibiting progenitor cell development into mature oligodendrocytes. APC, antigen-presenting cell; BBB, blood-brain barrier; CCL, chemokine ligand; CNS, central nervous system; CSF, cerebrospinal fluid; FDC, follicular dendritic cell; GM, grey matter; IFN-y, interferon gamma; IL, interleukin; MAIT, mucosal-associated invariant T cell; MRI, magnetic resonance imaging; MS, multiple sclerosis; NO, nitric oxide; RNS, reactive nitrogen species; ROS, reactive oxygen species; SPMS, secondary progressive multiple sclerosis; TH, T helper. Adapted from Dendrou et al. (38).
Peripheral immune cells, resident activated microglia, and astrocytes likely contribute to oligodendrocyte injury, demyelination, and neuroaxonal injury mediated by the secretion of soluble factors and cell contact–dependent mechanisms (4). While the formation of MS lesions is associated with acute axonal injury, subsequent lesion-related Wallerian, retrograde, and neuroaxonal degeneration contribute to the ongoing loss of brain tissue (45, 46).
As the disease progresses, compartmentalized inflammatory mechanisms that predominantly involve microglia, B cells, and other innate cells of the CNS become more prominent, leading to neurodegeneration, CNS atrophy, and disability worsening [Figure 2B (38, 47–51)]. The influx of immune cells into the CNS is dampened, but CNS inflammation persists from resident innate immune cells contributing to a chronic, localized inflammatory response (38). Other mechanisms that contribute to progressive tissue injury and the symptoms associated with progressive forms of the disease may include neurodegeneration resulting from neuroaxonal, astrocyte, and oligodendrocyte damage (4, 52). This tissue injury is evident at the edge of SELs, where “slow-burning” inflammation contributes to increased lesion burden, brain atrophy, cognition, and disability worsening (53, 54). In progressive MS, the BBB is an essential barrier for DMTs to overcome target sites of CNS-compartmentalized inflammation, demyelination, and neuroaxonal damage (6, 7). Clinical efficacy on measures of cognition, brain volume, and disability may therefore indicate direct CNS effects (55–57).
Although small, lipophilic molecules cross the BBB through passive diffusion, large molecules require membrane transport proteins to cross the BBB (58). For large molecule MS therapies that are unable to cross the BBB, it is proposed that effects on peripheral immune cells indirectly affect CNS-compartmentalized processes, potentially through changing the function or circulating concentration of immune cells that would typically access the CNS (59); thus, depending on target cells and the size/lipophilic nature of the compound, MS therapies may have CNS and peripheral effects.
The evidence presented in Supplementary Table 1 for alemtuzumab; dimethyl, diroximel, and monomethyl fumarate; interferons; glatiramer acetate; mitoxantrone; natalizumab; ocrelizumab; ofatumumab; and teriflunomide suggests that the function of these DMTs may be limited to the periphery or that they were insufficiently studied to confirm direct CNS effects. Evidence for cladribine, fingolimod, ozanimod, ponesimod, and siponimod suggests their direct CNS effects (Supplementary Table 2); therefore, these DMTs are the focus of this review.
Cladribine is indicated for the treatment of adults with RMS, including relapsing-remitting MS (RRMS) and active secondary progressive MS (SPMS) in the US and for adults with highly active RMS in the European Union (EU) (60, 61). Cladribine is an adenosine analog prodrug thought to function by causing cytotoxic effects on B and T cells through the impairment of DNA synthesis resulting in lymphocyte depletion (60). Intermittent therapy with cladribine can induce long-term remission of MS that is sustained without ongoing treatment (28). During treatment-free periods, the immune system repopulates and regains the ability to respond to infections without commensurate return of MS disease activity; thus, cladribine is considered an immune reconstitution therapy (62, 63).
As a small molecule, cladribine crosses the BBB in humans (64, 65), with penetration of the CNS observed in children with acute leukemia (64). An in vitro study found cladribine treatment to significantly reduce the granularity, phagocytotic ability, and motility of lipopolysaccharide-stimulated microglia at concentrations that putatively overlap with those found in the cerebrospinal fluid (CSF) of humans (0.1 µM–1 µM) (66). Other preclinical studies confirmed that cladribine inhibits microglial proliferation and proinflammatory cytokine release and induces microglia apoptosis (67, 68).
In a phase 3, randomized, placebo-controlled trial, cladribine-treated participants with RMS had a lower risk of clinical relapse and disability progression and had suppression of magnetic resonance imaging (MRI) brain lesions compared with placebo over 96 weeks (28). A post hoc analysis found that cladribine treatment resulted in less annualized brain atrophy over 2 years compared with placebo-treated participants (69). This reduction in brain atrophy was closely associated with a lower risk of disability progression, suggesting that treatment with cladribine can target neurodegeneration in patients with RMS (69). An observational study also found that cladribine suppresses the intrathecal humoral response, as observed by the disappearance of oligoclonal banding from the CSF of participants with RMS, an effect that was associated with milder neurological disability after 10 years of follow-up (70). To our knowledge, phase 3 clinical trials assessing cognition and studies utilizing MTR/MWF techniques are not published for cladribine.
Fingolimod is indicated for the treatment of patients 10 years of age and older with RMS, including clinically isolated syndrome (CIS), RRMS, and active SPMS, in the US and for patients 10 years of age and older with highly active RMS in patients with active disease despite treatment with at least one other DMT or rapidly evolving severe RMS in the EU (71, 72). Fingolimod is a prodrug metabolized by sphingosine kinase to the active metabolite fingolimod-phosphate, which is a sphingosine-1 phosphate (S1P) receptor modulator that binds with high affinity to S1P1,3,4,5 and blocks the capacity of lymphocytes to egress from lymph nodes, reducing the number of lymphocytes in peripheral blood (71). The full mechanism by which fingolimod exerts therapeutic effects in MS is unknown but may involve lymphocyte sequestration within lymphatic tissue thereby limiting lymphocyte migration into the CNS (71).
The lipophilic properties of fingolimod allow for CNS penetration (73). In experimental autoimmune encephalomyelitis (EAE) rodent models, fingolimod crossed the BBB, accumulated in the white matter of the CNS along the myelin sheath, and reduced S1P1 signaling from astrocytes (73, 74). Following intravenous injection in humans, a radiolabeled fingolimod analog entered the brain, with uptake increasing up to 26 hours after administration (75). An in vivo study found fingolimod improves neurological functional recovery in an EAE mouse model and promotes oligodendrocyte precursor cell proliferation and differentiation (76). Additional in vitro studies observed ameliorated pathological effectors associated with the activation of microglia, ultimately leading to increased morphological markers of remyelination and direct neuronal effects (77, 78).
In phase 3, randomized, RMS clinical trials, treatment with fingolimod reduced annualized relapse rate (ARR), MRI brain lesion activity, and brain volume loss compared with placebo at 12 and 24 months or IFN β-1a at 12 months (29–31). Post hoc analysis of data pooled from the phase 3 FREEDOMS and FREEDOMS II trials found that early fingolimod treatment may offer long-term cognitive benefits in participants with RRMS, as determined via improvements in Paced Auditory Serial Addition Test 3 (PASAT-3) scores from baseline compared with placebo (79). Fingolimod also reduced the risk of 3- and 6-month confirmed disability progression (CDP) over a 24-month study period, with Expanded Disability Status Scale (EDSS) and Multiple Sclerosis Functional Composite (MSFC) z scores remaining stable or improving slightly at month 24 compared with placebo in participants with RMS (29); however, when compared with IFN β-1a treatment, there were no significant differences in time to disability progression or the proportion of participants with confirmed progression at 12 months (31). Subsequently, a post hoc analysis of data from TRANSFORMS, FREEDOMS, FREEDOMS II, and their extensions, found that participants taking continuous fingolimod were more likely to experience confirmed or sustained disability improvement over 8 years than those switching from IFN β-1a and were more likely to experience confirmed disability improvement than those switching from placebo, suggesting long-term benefit (80).
Fingolimod also enhanced tissue damage recovery assessed by MTR in lesions after 6 months and in normal-appearing white matter and grey matter after 2 years (81). In an investigator-driven, randomized, phase 2 trial, fingolimod treatment improved recovery from unilateral optic neuritis in participants with MS or CIS compared with IFN β-1b, further supporting effects on remyelination; however, these results require confirmation in multicenter phase 3 clinical trials (82).
Ozanimod is indicated for the treatment of adults with RMS, including CIS, RRMS, and active SPMS in the US and for RRMS with active disease defined by clinical or imaging features in the EU, as well as ulcerative colitis in the US and EU (83, 84). Ozanimod is a selective S1P receptor modulator that binds with high affinity to S1P1 and S1P5 receptors. Ozanimod blocks lymphocyte egress from lymph nodes and reduces the number of lymphocytes in peripheral blood. Although its full mechanisms of action are unknown, ozanimod is proposed to affect MS through lymphocyte sequestration, thereby limiting lymphocyte migration into the CNS (84).
The ability of ozanimod to cross the BBB was observed preclinically, where the brain:blood ratio was 10:1 in mice following a single oral dose of ozanimod and 16:1 in rats following 5 days of once-daily dosing (85). Ex vivo treatment of EAE corticostriatal slices with ozanimod increased the mRNA expression of a marker of microglia activation while decreasing the expression of other inflammatory markers, and in vitro ozanimod treatment elicited potent protein kinase B (AKT) and extracellular-regulated kinase phosphorylation in human astrocytes (86, 87). In murine EAE, ozanimod reduced clinical scores at a dose that did not induce lymphopenia and elicited neuroprotective effects by reducing axonal breaks and improving functional capabilities following cuprizone-induced demyelination, each of these processes suggesting direct CNS effects (85, 88).
In phase 3, randomized, active comparator–controlled clinical trials, treatment with ozanimod 0.92 mg for up to 24 months reduced ARR, MRI brain lesions, and brain volume loss compared with IFN β-1a (32, 33). In addition, ozanimod treatment improved cognitive processing speed, as observed with greater Symbol Digit Modalities Test (SDMT) z scores, at month 12 compared with IFN β-1a in participants with RMS (32). Mean change in MSFC scores were similar across treatment groups from baseline to month 12 but were improved at month 24 with the ozanimod 0.46 mg dose compared with IFN β-1a (32, 33). Although the proportions of participants with CDP at 3 and 6 months were not significantly different between treatment groups in the phase 3 clinical trials (32, 33), interim analysis of a long-term, open-label extension trial demonstrated sustained control of disability progression with continuous ozanimod use (89). To our knowledge, studies utilizing MTR/MWF to assess the effects of ozanimod on demyelination are not published.
Ponesimod is indicated for the treatment of adults with RMS, including CIS, RRMS, and active SPMS in the US and EU (90, 91). Ponesimod is an S1P receptor modulator that binds with high affinity exclusively to S1P1 and blocks the capacity of lymphocytes to egress from lymph nodes, reducing the number of lymphocytes in peripheral blood (90). Like the other S1P receptor modulators, ponesimod is proposed to act through lymphocyte sequestration and reduction of lymphocyte migration into the CNS (90).
Preclinical studies of ponesimod demonstrated dampened neuroinflammation through the attenuation of glial activation in murine EAE and neuroinflammatory responses in primary human astrocytes (92, 93). In addition, ponesimod prevented cuprizone-induced demyelination in the cingulum of an EAE mouse model, supporting protective and likely selective effects against demyelination in the brain (93).
In a phase 3, randomized, active comparator-controlled clinical trial, ARR, number of MRI brain lesions, and brain volume loss at week 108 was lower with ponesimod compared with teriflunomide in participants with RMS (34). Cognitive processing speed and auditory processing speed were assessed with the SDMT and PASAT-3, respectively. Mean changes in SDMT and PASAT-3 scores from baseline to week 108 were numerically higher at most visits through week 108 in ponesimod-treated participants versus those treated with teriflunomide (94). Although ponesimod treatment did not impact time to 12- or 24-week confirmed disability accumulation compared with teriflunomide in participants with RMS, change from baseline to week 108 in MSFC z-scores favored ponesimod (34, 94). To our knowledge, studies utilizing MTR/MWF to assess the effects of ponesimod on remyelination are not published.
Siponimod is indicated for the treatment of adults with RMS, including CIS, RRMS, and active SPMS in the US and for adults with active SPMS in the EU (95). Siponimod is an S1P receptor modulator that binds with high affinity to S1P1 and S1P5 and blocks the capacity of lymphocytes to egress from lymph nodes, reducing the number of lymphocytes in the peripheral blood (95).
Siponimod is lipophilic and crosses the BBB, reaching dose-proportional steady-state levels in blood, concomitant with 6- to 8-fold higher levels in mouse brain homogenates after 10 days of treatment (96, 97). In preclinical studies, siponimod decreased oligodendrocyte cell death and axon demyelination, stimulated remyelination, prevented neurons from astrocyte-induced degeneration, attenuated astrogliosis and microgliosis, and induced proregenerative microglia (96, 98–101). In addition, siponimod provided neuroprotective benefits in murine EAE, independent of peripheral immune effects, suggesting direct CNS effects (96, 102).
In a phase 3, randomized clinical trial in SPMS, siponimod slowed disability progression, decreased ARR, reduced the accumulation of MRI brain lesions, and reduced the rate of brain atrophy compared with placebo (35). Of the five participants with SPMS treated with siponimod from the phase 3 study who consented to CSF sampling, all had siponimod (low nM range) in their CSF (97). Post hoc analyses demonstrated positive effects on cognition, as siponimod improved SDMT scores from baseline at months 12 and 24 compared with placebo (103). Most notably, siponimod had a significant and consistent impact on MTR decrease in normal-appearing white matter and cortical grey matter over time compared with placebo, while improving MTR recovery in newly formed lesions, consistent with possible remyelination (104). In addition, siponimod treatment improved brain tissue integrity/myelination within newly formed normalized MTR lesions across brain tissues compared with placebo (105).
Pivotal active-comparator phase 3 clinical trials established greater efficacy in measures of brain volume, cognition, and/or disability for DMTs acting directly on the CNS (e.g., fingolimod, ozanimod, and ponesimod) than those that may be limited to the periphery (e.g., teriflunomide and IFN β-1a) in participants with MS (31–34). Fingolimod treatment, however, did not slow disease progression compared with placebo in a phase 3 clinical trial of participants with primary progressive MS, suggesting that this anti-inflammatory therapy for relapse-onset MS is less likely to be effective in primary progressive disease, and other treatment approaches may be needed for these patients (106). In addition, other putative remyelination-promoting therapies, including opicinumab, MD-1003, and elezanumab failed to meet their study endpoints in MS clinical trials; lack of efficacy may have been due to the studied dose, time of treatment initiation, proof of concept study endpoints, or patient selection (107–110). The impact of centrally acting DMTs on brain volume, cognition, and/or disability contrasts with the lack of observed efficacy of remyelinating therapies and warrants further investigation.
The MS treatment landscape is continuously evolving, and ublituximab (an anti-CD20 monoclonal antibody) was recently approved by the FDA (111). Other therapies that target novel pathways are also in development (e.g., autologous hematopoietic stem cell transplantation [aHSCT], intrathecal stem cells, Bruton’s tyrosine kinase [BTK] inhibitors, clemastine fumarate, Epstein Barr virus [EBV]-targeted T cell immunotherapy, and temelimab) (112–122); preliminary data for these novel therapies are suggestive of CNS effects, as summarized in more detail below.
An observational, multicenter study provided Class IV evidence for aHSCT as an immunosuppressant MS therapy, because treatment induced durable disease remission for over 10 years in most participants with RRMS (including extremely aggressive forms of MS) (121). A randomized, phase 3 clinical trial (NCT04047628) is underway to determine the efficacy of aHSCT in participants with RMS, RRMS, or SPMS (123). Similarly, a pilot study of intrathecal mesenchymal stem cells in participants with RRMS or SPMS found that treatment stalled the disease progression of SPMS, and a phase 1/2 study (NCT04749667) is recruiting to determine efficacy in participants with progressive MS (112, 124).
BTK inhibitors primarily prevent B cell activation and maturation and are considered a promising therapeutic treatment option for MS that could target CNS-compartmentalized B cells, macrophages, and microglia (125). In a murine EAE model, the BTK inhibitors evobrutinib and tolebrutinib crossed the BBB (126, 127), with other preclinical studies showing BTK inhibitors to target CNS-inflammatory processes, including the reduction of microglial activation and inflammatory signaling (128–130). In addition, BTK inhibition has a positive effect on remyelination by targeting microglia, as demonstrated in ex vivo and in vivo models (131). A phase 1 trial found that evobrutinib was well tolerated in healthy volunteers, and a phase 2 trial found that treatment reduced the total number of GdE lesions compared with placebo but did not have an effect on relapse rate or disability progression in participants with RMS (118, 122). In a phase 1 tolebrutinib clinical trial, the CSF:plasma ratio of free tolebrutinib was 2.25 and treatment was considered well tolerated in healthy volunteers (116). A phase 2b trial in participants with RMS found that tolebrutinib treatment reduced the total number of GdE lesions compared with placebo (51). Additional phase 2 and phase 3 clinical trials are planned/underway for evobrutinib (NCT02975349, NCT04338022, and NCT04338061) and tolebrutinib (NCT04411641, NCT04410991, NCT04410978, and NCT04458051), as well as other BTK inhibitors, including fenebrutinib (NCT05119569, NCT04586010, NCT04586023, and NCT04544449), orelabrutinib (NCT04711148), remibrutinib (NCT05147220 and NCT05156281), and BIIB061 (NCT04079088) for relapsing and progressive forms of MS (118, 132–145).
Clemastine fumarate, originally developed as a first-generation antihistamine, is a muscarinic receptor antagonist that crosses the BBB and may promote remyelination (146). In the randomized, placebo-controlled crossover ReBUILD study, clemastine fumarate reduced visual-evoked potential latency delay in participants with RMS and chronic demyelinating optic neuropathy (120) and is considered the first randomized, controlled MS trial to demonstrate efficacy of a remyelinating agent (120). Phase 1 and 2 trials are planned/underway to assess the effects of clemastine fumarate as a monotherapy or in combination with metformin on remyelination in participants with relapsing or progressive forms of MS (147, 148).
EBV-targeted T cell immunotherapies have the potential to target cells associated with MS pathophysiology and may have a role in the CNS, as T cells access all compartments within the CNS (114). A case report of a patient with secondary progressive MS treated with an EBV-targeted T cell immunotherapy showed clinical improvement with reduced disease activity via MRI and decreased intrathecal immunoglobulin production (115). An open-label phase 1 trial of 10 participants with primary progressive multiple sclerosis and SPMS found EBV-targeted T cell treatment to be associated with clinical improvement in 7 participants, with 3 having improved EDSS score (114). Retrospective analysis of participants with progressive MS in part 1 of EMBOLD (NCT03283826), a single-arm phase 1 study with an open-label extension, found long-term disability improvement during ATA188 treatment (investigational EBV-targeted T cell immunotherapy) to be related to brain volume change and MTR in T2 lesions, suggesting the potential for remyelination over time (117). Part 2 of EMBOLD is a double-blind, randomized, placebo-controlled trial that is currently underway (149).
Early data for the immunoglobulin G4 monoclonal antibody temelimab suggest CNS effects on MRI measures of neurodegeneration (119). Although a randomized, placebo-controlled phase 2b study of temelimab failed to show an effect on acute inflammation, radiologic signs of possible neural protective effects suggest the potential use of temelimab in progressive MS (119).
In future clinical trials, applying appropriate endpoints that distinguish between peripheral and direct CNS effects may guide the therapeutic landscape for MS. A molecule is commonly considered to penetrate the BBB if the brain:plasma ratio is >0.04 using nonperfused brain tissue; however, this single parameter does not guarantee that adequate brain penetration is achieved for ligand-target interaction for a specific compound and should be accompanied by assessment of therapeutic response (150). Assessing the therapeutic response in the CNS may be accomplished with biomarkers (e.g., neurofilament light chain and glial fibrillary acidic protein), monitoring SELs and/or paramagnetic rim lesions, myelin measurements (e.g., MTR/MWF and ultrashort echo time MRI), P100 latency using multifocal or full-field visual evoked potential, optical coherence tomography, or in vivo imaging techniques (e.g., positron emission tomography imaging of microglia). The reliability of these techniques for determining direct effects within the CNS remains under investigation (110, 151–157). Well-designed clinical trials that consider these approaches are needed to substantiate new treatment modalities for MS that may include combining BBB-penetrating DMTs with peripherally acting monoclonal antibodies to limit disease progression and improve treatment outcomes through promoting immunomodulation, remyelination, and neuroprotection (158, 159).
Of the DMTs currently available for the treatment of MS, data for cladribine, fingolimod, ozanimod, ponesimod, and siponimod are suggestive of direct CNS effects. Future MS studies may show a broader therapeutic benefit for DMTs that directly impact the CNS than those that act on the peripheral immune response alone.
H-PH: Writing – review & editing, Conceptualization. BACC: Writing – review & editing, Conceptualization. MB: Writing – review & editing, Conceptualization. SGM: Writing – review & editing, Conceptualization. AB-O: Writing – review & editing, Conceptualization. LS: Writing – review & editing, Conceptualization.
The author(s) declare financial support was received for the research, authorship, and/or publication of this article. The authors declare that this review project received funding from Bristol Myers Squibb (Princeton, NJ) for writing and editorial assistance. The funder was not involved in the design, literature review, interpretation, the writing of this article, or the decision to submit it for publication.
Writing and editorial assistance was provided by Noud van Helmond, MD, PhD, of Peloton Advantage, LLC, an OPEN Health company, and was funded by Bristol Myers Squibb.
Author H-PH: Personal fees for consulting, serving on steering committees, and speaking from Bayer Healthcare, Biogen, Celgene, GeNeuro, Genzyme, MedImmune, Merck, Novartis, Octapharma, Roche, Sanofi, and Teva. Author BACC: Personal compensation for consulting for Alexion, Atara, Autobahn, Avotres, Biogen, Boston Pharma, EMD Serono, Gossamer Bio, Hexal/Sandoz, Horizon, Immunic AG, Neuron23, Novartis, Sanofi, Siemens, TG Therapeutics, and Therini; and received research support from Genentech. Author MB: Institutional funding from Alexion, Biogen, Bristol Myers Squibb, Merck, Novartis, and Roche; personal compensation for speaking from Biogen and Novartis. Author SGM: Honoraria for lecturing and travel expenses for attending meetings from Almirall, Amicus Therapeutics Germany, Bayer Health Care, Biogen, Bristol Myers Squibb/Celgene, Diamed, Genzyme, MedDay Pharmaceuticals, Merck Serono, Novartis, Novo Nordisk, Ono Pharma, Roche, Sanofi-Aventis, Chugai Pharma, QuintilesIMS, and Teva. His research is funded by the German Ministry for Education and Research, Bundesinstitut für Risikobewertung, Deutsche Forschungsgemeinschaft, Else Kröner Fresenius Foundation, Gemeinsamer Bundesausschuss, German Academic Exchange Service, Hertie Foundation, Interdisciplinary Center for Clinical Research Muenster, German Foundation Neurology, and Alexion, Almirall, Amicus Therapeutics Germany, Biogen, Diamed, Fresenius Medical Care, Genzyme, HERZ Burgdorf, Merck Serono, Novartis, Ono Pharma, Roche, and Teva. Author AB-O: Received fees for advisory board participation and/or consulting from Accure, Atara Biotherapeutics, Biogen, BMS/Celgene/Receptos, GlaxoSmithKline, Gossamer, Janssen/Actelion, MedImmune, Merck/EMD Serono, Novartis, Roche/Genentech, and Sanofi-Genzyme; and has received grant support to the University of Pennsylvania from Biogen Idec, Merck/EMD Serono, Novartis, and Roche/Genentech. Author LS: Consulting for AbbVie, Atreca, Bristol Myers Squibb, EMD Serono, Novartis, Teva, Pasithea, 180 Life Sciences, and TG Therapeutics, and research support from Atara and Bristol Myers Squibb.
The author(s) declared that they were an editorial board member of Frontiers, at the time of submission. This had no impact on the peer review process and the final decision.
All claims expressed in this article are solely those of the authors and do not necessarily represent those of their affiliated organizations, or those of the publisher, the editors and the reviewers. Any product that may be evaluated in this article, or claim that may be made by its manufacturer, is not guaranteed or endorsed by the publisher.
The Supplementary Material for this article can be found online at: https://www.frontiersin.org/articles/10.3389/fimmu.2023.1290666/full#supplementary-material
1. Walton C, King R, Rechtman L, Kaye W, Leray E, Marrie RA, et al. Rising prevalence of multiple sclerosis worldwide: insights from the atlas of ms, third edition. Mult Scler (2020) 26(14):1816–21. doi: 10.1177/1352458520970841
2. Håkansson I. Biomarkers and Disease Activity in Multiple Sclerosis : A Cohort Study on Patients with Clinically Isolated Syndrome and Relapsing Remitting Multiple Sclerosis. Linköping, Sweden: Linköping University Electronic Press (2019) p. 1–78. Available at: http://liu.diva-portal.org/smash/get/diva2:1358189/PREVIEW01.png. doi: 10.3384/diss.diva-160762
3. Montalban X, Gold R, Thompson AJ, Otero-Romero S, Amato MP, Chandraratna D, et al. Ectrims/ean guideline on the pharmacological treatment of people with multiple sclerosis. Mult Scler (2018) 24(2):96–120. doi: 10.1177/1352458517751049
4. Attfield KE, Jensen LT, Kaufmann M, Friese MA, Fugger L. The immunology of multiple sclerosis. Nat Rev Immunol (2022) 22(12):734–50. doi: 10.1038/s41577-022-00718-z
5. Cree BAC, Arnold DL, Chataway J, Chitnis T, Fox RJ, Pozo Ramajo A, et al. Secondary progressive multiple sclerosis: new insights. Neurology (2021) 97(8):378–88. doi: 10.1212/wnl.0000000000012323
6. Lassmann H. Targets of therapy in progressive ms. Mult Scler (2017) 23(12):1593–9. doi: 10.1177/1352458517729455
7. Correale J, Halfon MJ, Jack D, Rubstein A, Villa A. Acting centrally or peripherally: A renewed interest in the central nervous system penetration of disease-modifying drugs in multiple sclerosis. Mult Scler Relat Disord (2021) 56:103264. doi: 10.1016/j.msard.2021.103264
8. Cohen JA, Coles AJ, Arnold DL, Confavreux C, Fox EJ, Hartung HP, et al. Alemtuzumab versus interferon beta 1a as first-line treatment for patients with relapsing-remitting multiple sclerosis: A randomised controlled phase 3 trial. Lancet (2012) 380(9856):1819–28. doi: 10.1016/S0140-6736(12)61769-3
9. Coles AJ, Twyman CL, Arnold DL, Cohen JA, Confavreux C, Fox EJ, et al. Alemtuzumab for patients with relapsing multiple sclerosis after disease-modifying therapy: A randomised controlled phase 3 trial. Lancet (2012) 380(9856):1829–39. doi: 10.1016/S0140-6736(12)61768-1
10. Fox RJ, Miller DH, Phillips JT, Hutchinson M, Havrdova E, Kita M, et al. Placebo-controlled phase 3 study of oral bg-12 or glatiramer in multiple sclerosis. N Engl J Med (2012) 367(12):1087–97. doi: 10.1056/NEJMoa1206328
11. Gold R, Kappos L, Arnold DL, Bar-Or A, Giovannoni G, Selmaj K, et al. Placebo-controlled phase 3 study of oral bg-12 for relapsing multiple sclerosis. N Engl J Med (2012) 367(12):1098–107. doi: 10.1056/NEJMoa1114287
12. Johnson KP, Brooks BR, Cohen JA, Ford CC, Goldstein J, Lisak RP, et al. Copolymer 1 reduces relapse rate and improves disability in relapsing-remitting multiple sclerosis: results of a phase iii multicenter, double-blind placebo-controlled trial. The copolymer 1 multiple sclerosis study group. Neurology (1995) 45(7):1268–76. doi: 10.1212/wnl.45.7.1268
13. Jacobs L, O'Malley J, Freeman A, Ekes R. Intrathecal interferon reduces exacerbations of multiple sclerosis. Science (1981) 214(4524):1026–8. doi: 10.1126/science.6171035
14. The IFNB Multiple Sclerosis Study Group. Interferon beta-1b is effective in relapsing-remitting multiple sclerosis. I. Clinical results of a multicenter, randomized, double-blind, placebo-controlled trial. The ifnb multiple sclerosis study group. Neurology (1993) 43(4):655–61. doi: 10.1212/wnl.43.4.655
15. Jacobs LD, Cookfair DL, Rudick RA, Herndon RM, Richert JR, Salazar AM, et al. Intramuscular interferon beta-1a for disease progression in relapsing multiple sclerosis. The multiple sclerosis collaborative research group (Mscrg). Ann Neurol (1996) 39(3):285–94. doi: 10.1002/ana.410390304
16. European Study Group on Interferon beta-1b in Secondary Progressive MS. Placebo-controlled multicentre randomised trial of interferon beta-1b in treatment of secondary progressive multiple sclerosis. European study group on interferon beta-1b in secondary progressive ms. Lancet (1998) 352(9139):1491–7. doi: 10.1212/wnl.43.4.655
17. Jacobs LD, Beck RW, Simon JH, Kinkel RP, Brownscheidle CM, Murray TJ, et al. Intramuscular interferon beta-1a therapy initiated during a first demyelinating event in multiple sclerosis. Champs study group. N Engl J Med (2000) 343(13):898–904. doi: 10.1056/nejm200009283431301
18. Panitch H, Miller A, Paty D, Weinshenker B. Interferon beta-1b in secondary progressive ms: results from a 3-year controlled study. Neurology (2004) 63(10):1788–95. doi: 10.1212/01.wnl.0000146958.77317.3e
19. Kappos L, Polman CH, Freedman MS, Edan G, Hartung HP, Miller DH, et al. Treatment with interferon beta-1b delays conversion to clinically definite and mcdonald ms in patients with clinically isolated syndromes. Neurology (2006) 67(7):1242–9. doi: 10.1212/01.wnl.0000237641.33768.8d
20. Calabresi PA, Kieseier BC, Arnold DL, Balcer LJ, Boyko A, Pelletier J, et al. Pegylated interferon beta-1a for relapsing-remitting multiple sclerosis (Advance): A randomised, phase 3, double-blind study. Lancet Neurol (2014) 13(7):657–65. doi: 10.1016/s1474-4422(14)70068-7
21. Hartung HP, Gonsette R, König N, Kwiecinski H, Guseo A, Morrissey SP, et al. Mitoxantrone in progressive multiple sclerosis: A placebo-controlled, double-blind, randomised, multicentre trial. Lancet (2002) 360(9350):2018–25. doi: 10.1016/s0140-6736(02)12023-x
22. Polman CH, O'Connor PW, Havrdova E, Hutchinson M, Kappos L, Miller DH, et al. A randomized, placebo-controlled trial of natalizumab for relapsing multiple sclerosis. N Engl J Med (2006) 354(9):899–910. doi: 10.1056/NEJMoa044397
23. Hauser SL, Bar-Or A, Comi G, Giovannoni G, Hartung HP, Hemmer B, et al. Ocrelizumab versus interferon beta-1a in relapsing multiple sclerosis. N Engl J Med (2017) 376(3):221–34. doi: 10.1056/NEJMoa1601277
24. Montalban X, Hauser SL, Kappos L, Arnold DL, Bar-Or A, Comi G, et al. Ocrelizumab versus placebo in primary progressive multiple sclerosis. N Engl J Med (2017) 376(3):209–20. doi: 10.1056/NEJMoa1606468
25. Hauser SL, Bar-Or A, Cohen JA, Comi G, Correale J, Coyle PK, et al. Ofatumumab versus teriflunomide in multiple sclerosis. N Engl J Med (2020) 383(6):546–57. doi: 10.1056/NEJMoa1917246
26. O'Connor P, Wolinsky JS, Confavreux C, Comi G, Kappos L, Olsson TP, et al. Randomized trial of oral teriflunomide for relapsing multiple sclerosis. N Engl J Med (2011) 365(14):1293–303. doi: 10.1056/NEJMoa1014656
27. Confavreux C, O'Connor P, Comi G, Freedman MS, Miller AE, Olsson TP, et al. Oral teriflunomide for patients with relapsing multiple sclerosis (Tower): A randomised, double-blind, placebo-controlled, phase 3 trial. Lancet Neurol (2014) 13(3):247–56. doi: 10.1016/s1474-4422(13)70308-9
28. Giovannoni G, Comi G, Cook S, Rammohan K, Rieckmann P, Soelberg Sørensen P, et al. A placebo-controlled trial of oral cladribine for relapsing multiple sclerosis. N Engl J Med (2010) 362(5):416–26. doi: 10.1056/NEJMoa0902533
29. Kappos L, Radue EW, O'Connor P, Polman C, Hohlfeld R, Calabresi P, et al. A placebo-controlled trial of oral fingolimod in relapsing multiple sclerosis. N Engl J Med (2010) 362(5):387–401. doi: 10.1056/NEJMoa0909494
30. Calabresi PA, Radue EW, Goodin D, Jeffery D, Rammohan KW, Reder AT, et al. Safety and efficacy of fingolimod in patients with relapsing-remitting multiple sclerosis (Freedoms ii): A double-blind, randomised, placebo-controlled, phase 3 trial. Lancet Neurol (2014) 13(6):545–56. doi: 10.1016/s1474-4422(14)70049-3
31. Cohen JA, Barkhof F, Comi G, Hartung HP, Khatri BO, Montalban X, et al. Oral fingolimod or intramuscular interferon for relapsing multiple sclerosis. N Engl J Med (2010) 362(5):402–15. doi: 10.1056/NEJMoa0907839
32. Comi G, Kappos L, Selmaj KW, Bar-Or A, Arnold DL, Steinman L, et al. Safety and efficacy of ozanimod versus interferon beta-1a in relapsing multiple sclerosis (Sunbeam): A multicentre, randomised, minimum 12-month, phase 3 trial. Lancet Neurol (2019) 18(11):1009–20. doi: 10.1016/S1474-4422(19)30239-X
33. Cohen JA, Comi G, Selmaj KW, Bar-Or A, Arnold DL, Steinman L, et al. Safety and efficacy of ozanimod versus interferon beta-1a in relapsing multiple sclerosis (Radiance): A multicentre, randomised, 24-month, phase 3 trial. Lancet Neurol (2019) 18(11):1021–33. doi: 10.1016/S1474-4422(19)30238-8
34. Kappos L, Fox RJ, Burcklen M, Freedman MS, Havrdová EK, Hennessy B, et al. Ponesimod compared with teriflunomide in patients with relapsing multiplesclerosis in the active-comparator phase 3 optimum study: A randomized clinical trial. JAMA Neurol (2021) 78(5):558–67. doi: 10.1001/jamaneurol.2021.0405
35. Kappos L, Bar-Or A, Cree BAC, Fox RJ, Giovannoni G, Gold R, et al. Siponimod versus placebo in secondary progressive multiple sclerosis (Expand): A double-blind, randomised, phase 3 study. Lancet (2018) 391(10127):1263–73. doi: 10.1016/s0140-6736(18)30475-6
36. Cunniffe N, Coles A. Promoting remyelination in multiple sclerosis. J Neurol (2021) 268(1):30–44. doi: 10.1007/s00415-019-09421-x
37. Marchetti L, Engelhardt B. Immune cell trafficking across the blood-brain barrier in the absence and presence of neuroinflammation. Vasc Biol (Bristol England) (2020) 2(1):H1–h18. doi: 10.1530/vb-19-0033
38. Dendrou CA, Fugger L, Friese MA. Immunopathology of multiple sclerosis. Nat Rev Immunol (2015) 15(9):545–58. doi: 10.1038/nri3871
39. Hochmeister S, Grundtner R, Bauer J, Engelhardt B, Lyck R, Gordon G, et al. Dysferlin is a new marker for leaky brain blood vessels in multiple sclerosis. J Neuropathol Exp Neurol (2006) 65(9):855–65. doi: 10.1097/01.jnen.0000235119.52311.16
40. Filippi M, Bar-Or A, Piehl F, Preziosa P, Solari A, Vukusic S, et al. Multiple sclerosis. Nat Rev Dis Primers (2018) 4(1):43. doi: 10.1038/s41572-018-0041-4
41. Babbe H, Roers A, Waisman A, Lassmann H, Goebels N, Hohlfeld R, et al. Clonal expansions of cd8(+) T cells dominate the T cell infiltrate in active multiple sclerosis lesions as shown by micromanipulation and single cell polymerase chain reaction. J Exp Med (2000) 192(3):393–404. doi: 10.1084/jem.192.3.393
42. Kuenz B, Lutterotti A, Ehling R, Gneiss C, Haemmerle M, Rainer C, et al. Cerebrospinal fluid B cells correlate with early brain inflammation in multiple sclerosis. PloS One (2008) 3(7):e2559. doi: 10.1371/journal.pone.0002559
43. Park C, Ponath G, Levine-Ritterman M, Bull E, Swanson EC, De Jager PL, et al. The landscape of myeloid and astrocyte phenotypes in acute multiple sclerosis lesions. Acta Neuropathol Commun (2019) 7(1):130. doi: 10.1186/s40478-019-0779-2
44. Ineichen BV, Okar SV, Proulx ST, Engelhardt B, Lassmann H, Reich DS. Perivascular spaces and their role in neuroinflammation. Neuron (2022) 110(21):3566–81. doi: 10.1016/j.neuron.2022.10.024
45. Kuhlmann T, Lingfeld G, Bitsch A, Schuchardt J, Brück W. Acute axonal damage in multiple sclerosis is most extensive in early disease stages and decreases over time. Brain (2002) 125(Pt 10):2202–12. doi: 10.1093/brain/awf235
46. Lassmann H. Pathogenic mechanisms associated with different clinical courses of multiple sclerosis. Front Immunol (2018) 9:3116. doi: 10.3389/fimmu.2018.03116
47. Magliozzi R, Howell O, Vora A, Serafini B, Nicholas R, Puopolo M, et al. Meningeal B-cell follicles in secondary progressive multiple sclerosis associate with early onset of disease and severe cortical pathology. Brain (2007) 130(Pt 4):1089–104. doi: 10.1093/brain/awm038
48. MaChado-Santos J, Saji E, Tröscher AR, Paunovic M, Liblau R, Gabriely G, et al. The compartmentalized inflammatory response in the multiple sclerosis brain is composed of tissue-resident cd8+ T lymphocytes and B cells. Brain (2018) 141(7):2066–82. doi: 10.1093/brain/awy151
49. Bhargava P, Hartung HP, Calabresi PA. Contribution of B cells to cortical damage in multiple sclerosis. Brain (2022) 145(10):3363–73. doi: 10.1093/brain/awac233
50. Jain RW, Yong VW. B cells in central nervous system disease: diversity, locations and pathophysiology. Nat Rev Immunol (2022) 22(8):513–24. doi: 10.1038/s41577-021-00652-6
51. Yong VW. Microglia in multiple sclerosis: protectors turn destroyers. Neuron (2022) 110(21):3534–48. doi: 10.1016/j.neuron.2022.06.023
52. Kuhlmann T, Moccia M, Coetzee T, Cohen JA, Correale J, Graves J, et al. Multiple sclerosis progression: time for a new mechanism-driven framework. Lancet Neurol (2022) 22(1):78–88. doi: 10.1016/s1474-4422(22)00289-7
53. Preziosa P, Pagani E, Meani A, Moiola L, Rodegher M, Filippi M, et al. Slowly expanding lesions predict 9-year multiple sclerosis disease progression. Neurol Neuroimmunol Neuroinflamm (2022) 9(2):e1139. doi: 10.1212/nxi.0000000000001139
54. Calvi A, Carrasco FP, Tur C, Chard DT, Stutters J, De Angelis F, et al. Association of slowly expanding lesions on mri with disability in people with aecondary progressive multiple sclerosis. Neurology (2022) 98(17):e1783–e93. doi: 10.1212/wnl.0000000000200144
55. Giedraitiene N, Drukteiniene E, Kizlaitiene R, Cimbalas A, Asoklis R, Kaubrys G. Cognitive decline in multiple sclerosis is related to the progression of retinal atrophy and presence of oligoclonal bands: A 5-year follow-up study. Front Neurol (2021) 12:678735. doi: 10.3389/fneur.2021.678735
56. Wang C, Barnett MH, Yiannikas C, Barton J, Parratt J, You Y, et al. Lesion activity and chronic demyelination are the major determinants of brain atrophy in ms. Neurol Neuroimmunol Neuroinflamm (2019) 6(5):e593. doi: 10.1212/nxi.0000000000000593
57. Pulizzi A, Rovaris M, Judica E, Sormani MP, Martinelli V, Comi G, et al. Determinants of disability in multiple sclerosis at various disease stages: A multiparametric magnetic resonance study. Arch Neurol (2007) 64(8):1163–8. doi: 10.1001/archneur.64.8.1163
58. Wong AD, Ye M, Levy AF, Rothstein JD, Bergles DE, Searson PC. The blood-brain barrier: an engineering perspective. Front Neuroeng (2013) 6:7. doi: 10.3389/fneng.2013.00007
59. Antel JP, Miron VE. Central nervous system effects of current and emerging multiple sclerosis-directed immuno-therapies. Clin Neurol Neurosurg (2008) 110(9):951–7. doi: 10.1016/j.clineuro.2008.03.021
62. Giovannoni G, Mathews J. Cladribine tablets for relapsing-remitting multiple sclerosis: A clinician's review. Neurol Ther (2022) 11(2):571–95. doi: 10.1007/s40120-022-00339-7
63. Meuth SG, Bayas A, Kallmann B, Linker R, Rieckmann P, Wattjes MP, et al. Long-term management of multiple sclerosis patients treated with cladribine tablets beyond year 4. Expert Opin Pharmacother (2022) 23(13):1503–10. doi: 10.1080/14656566.2022.2106783
64. Kearns CM, Blakley RL, Santana VM, Crom WR. Pharmacokinetics of cladribine (2-chlorodeoxyadenosine) in children with acute leukemia. Cancer Res (1994) 54(5):1235–9.
65. Liliemark J. The clinical pharmacokinetics of cladribine. Clin Pharmacokinet (1997) 32(2):120–31. doi: 10.2165/00003088-199732020-00003
66. Jørgensen L, Hyrlov KH, Elkjaer ML, Weber AB, Pedersen AE, Svenningsen ÅF, et al. Cladribine modifies functional properties of microglia. Clin Exp Immunol (2020) 201(3):328–40. doi: 10.1111/cei.13473
67. Singh V, Voss EV, Bénardais K, Stangel M. Effects of 2-chlorodeoxyadenosine (Cladribine) on primary rat microglia. J Neuroimmune Pharmacol (2012) 7(4):939–50. doi: 10.1007/s11481-012-9387-7
68. Aybar F, Julia Perez M, Silvina Marcora M, Eugenia Samman M, Marrodan M, María Pasquini J, et al. 2-chlorodeoxyadenosine (Cladribine) preferentially inhibits the biological activity of microglial cells. Int Immunopharmacol (2022) 105:108571. doi: 10.1016/j.intimp.2022.108571
69. De Stefano N, Giorgio A, Battaglini M, De Leucio A, Hicking C, Dangond F, et al. Reduced brain atrophy rates are associated with lower risk of disability progression in patients with relapsing multiple sclerosis treated with cladribine tablets. Mult Scler (2018) 24(2):222–6. doi: 10.1177/1352458517690269
70. Rejdak K, Stelmasiak Z, Grieb P. Cladribine induces long lasting oligoclonal bands disappearance in relapsing multiple sclerosis patients: 10-year observational study. Mult Scler Relat Disord (2019) 27:117–20. doi: 10.1016/j.msard.2018.10.006
73. Foster CA, Howard LM, Schweitzer A, Persohn E, Hiestand PC, Balatoni B, et al. Brain penetration of the oral immunomodulatory drug fty720 and its phosphorylation in the central nervous system during experimental autoimmune encephalomyelitis: consequences for mode of action in multiple sclerosis. J Pharmacol Exp Ther (2007) 323(2):469–75. doi: 10.1124/jpet.107.127183
74. Choi JW, Gardell SE, Herr DR, Rivera R, Lee CW, Noguchi K, et al. Fty720 (Fingolimod) efficacy in an animal model of multiple sclerosis requires astrocyte sphingosine 1-phosphate receptor 1 (S1p1) modulation. Proc Natl Acad Sci USA (2011) 108(2):751–6. doi: 10.1073/pnas.1014154108
75. Tamagnan G, Tavares A, Barret O, Alagille D, Seibyl J, Marek K, et al. Brain distribution of bzm055, an analog of fingolimod (Fty720), in human. Mult Scler (2012) 18(suppl 4):379.
76. Zhang J, Zhang ZG, Li Y, Ding X, Shang X, Lu M, et al. Fingolimod treatment promotes proliferation and differentiation of oligodendrocyte progenitor cells in mice with experimental autoimmune encephalomyelitis. Neurobiol Dis (2015) 76:57–66. doi: 10.1016/j.nbd.2015.01.006
77. Jackson SJ, Giovannoni G, Baker D. Fingolimod modulates microglial activation to augment markers of remyelination. J Neuroinflamm (2011) 8:76. doi: 10.1186/1742-2094-8-76
78. Doi Y, Takeuchi H, Horiuchi H, Hanyu T, Kawanokuchi J, Jin S, et al. Fingolimod phosphate attenuates oligomeric amyloid B-induced neurotoxicity via increased brain-derived neurotrophic factor expression in neurons. PloS One (2013) 8(4):e61988. doi: 10.1371/journal.pone.0061988
79. Langdon DW, Tomic D, Penner IK, Calabrese P, Cutter G, Häring DA, et al. Baseline characteristics and effects of fingolimod on cognitive performance in patients with relapsing-remitting multiple sclerosis. Eur J Neurol (2021) 28(12):4135–45. doi: 10.1111/ene.15081
80. Cree BA, Cohen JA, Reder AT, Tomic D, Silva D, Piani Meier D, et al. Disability improvement as a clinically relevant outcome in clinical trials of relapsing forms of multiple sclerosis. Mult Scler (2021) 27(14):2219–31. doi: 10.1177/13524585211000280
81. Preziosa P, Storelli L, Meani A, Moiola L, Rodegher M, Filippi M, et al. Effects of fingolimod and natalizumab on brain T1-/T2-weighted and magnetization transfer ratios: A 2-year study. Neurotherapeutics (2021) 18(2):878–88. doi: 10.1007/s13311-020-00997-1
82. Albert C, Mikolajczak J, Liekfeld A, Piper SK, Scheel M, Zimmermann HG, et al. Fingolimod after a first unilateral episode of acute optic neuritis (Moving) - preliminary results from a randomized, rater-blind, active-controlled, phase 2 trial. BMC Neurol (2020) 20(1):75. doi: 10.1186/s12883-020-01645-z
85. Scott FL, Clemons B, Brooks J, Brahmachary E, Powell R, Dedman H, et al. Ozanimod (Rpc1063) is a potent sphingosine-1-phosphate receptor-1 (S1p1 ) and receptor-5 (S1p5 ) agonist with autoimmune disease-modifying activity. Br J Pharmacol (2016) 173(11):1778–92. doi: 10.1111/bph.13476
86. Musella A, Gentile A, Guadalupi L, Rizzo FR, De Vito F, Fresegna D, et al. Central modulation of selective sphingosine-1-phosphate receptor 1 ameliorates experimental multiple sclerosis. Cells (2020) 9(5):1290. doi: 10.3390/cells9051290
87. Selkirk JV, Yan YG, Ching N, Paget K, Hargreaves R. In vitro assessment of the binding and functional reponses of ozanimod and its plasma metabolites across human sphingosine 1-phosphate receptors. Eur J Pharmacol (2022) 941:175442. doi: 10.1016/j.ejphar.2022.175442
88. Taylor Meadows KR, Selkirk JV, Akhtar MW, Hutton C, Opiteck GJ, Scott FL. Ozanimod (Rpc1063) is potentially neuroprotective through direct cns effects. Mult Scler (2017) 23(suppl 3):624.
89. Cree BA, Selmaj KW, Steinman L, Comi G, Bar-Or A, Arnold DL, et al. Long-term safety and efficacy of ozanimod in relapsing multiple sclerosis: up to 5 Years of follow-up in the daybreak open-label extension trial. Mult Scler (2022) 28(12):1944–62. doi: 10.1177/13524585221102584
92. Fourgeaud L, Le M, Needham A, Ait-Tihyaty M, Lair LL, Breu V, et al. A central effect of ponesimod on neuroinflammation in a pre-clinical model of multiple sclerosis. Mult Scler (2021) 27(1 suppl):105. doi: 10.1177/13524585211015908
93. Kihara Y, Jonnalagadda D, Zhu Y, Ray M, Ngo T, Palmer C, et al. Ponesimod inhibits astrocyte-mediated neuroinflammation and protects against cingulum demyelination via S1p(1) -selective modulation. FASEB J (2022) 36(2):e22132. doi: 10.1096/fj.202101531R
94. Fox R, Kappos L, Burcklen M, Freedman M, Havrdova E, Hennessy B, et al. Effect on disability measures and msfc in patients with relapsing multiple sclerosis from the phase 3 ponesimod versus teriflunomide optimum study. Mult Scler (2020) 26(suppl 3):216–7.
96. Gentile A, Musella A, Bullitta S, Fresegna D, De Vito F, Fantozzi R, et al. Siponimod (Baf312) prevents synaptic neurodegeneration in experimental multiple sclerosis. J Neuroinflamm (2016) 13(1):207. doi: 10.1186/s12974-016-0686-4
97. Bigaud M, Rudolph B, Briard E, Beerli C, Schubart A, Gardin A. Siponimod penetrates, distributes and acts on the central nervous system: translational insights. Neurology (2020) 94(15 suppl):3973.
98. Tiwari-Woodruff S, Yamate-Morgan H, Sekyi M, Lauderdale K, Hasselmann J, Schubart A. The sphingosine 1-phosphate (S1p) receptor modulator, siponimod decreases oligodendrocyte cell death and axon demyelination in a mouse model of multiple sclerosis. Neurology (2016) 86(16 suppl):I10.011.
99. Mannioui A, Vauzanges Q, Fini JB, Henriet E, Sekizar S, Azoyan L, et al. The xenopus tadpole: an in vivo model to screen drugs favoring remyelination. Mult Scler (2018) 24(11):1421–32. doi: 10.1177/1352458517721355
100. Dietrich M, Hecker C, Martin E, Langui D, Gliem M, Stankoff B, et al. Increased remyelination and proregenerative microglia under siponimod therapy in mechanistic models. Neurol Neuroimmunol Neuroinflamm (2022) 9(3):e1161. doi: 10.1212/nxi.0000000000001161
101. Colombo E, Bassani C, De Angelis A, Ruffini F, Ottoboni L, Comi G, et al. Siponimod (Baf312) activates nrf2 while hampering nfκb in human astrocytes, and protects from astrocyte-induced neurodegeneration. Front Immunol (2020) 11:635. doi: 10.3389/fimmu.2020.00635
102. Hundehege P, Cerina M, Eichler S, Thomas C, Herrmann AM, Göbel K, et al. The next-generation sphingosine-1 receptor modulator baf312 (Siponimod) improves cortical network functionality in focal autoimmune encephalomyelitis. Neural Regener Res (2019) 14(11):1950–60. doi: 10.4103/1673-5374.259622
103. Benedict RHB, Tomic D, Fox R, Cree BAC, Vermersch P, Giovannoni G, et al. (2019). Siponimod improves cognitive processing speed in patients with secondary progressive multiple sclerosis: expand subgroup analyses, in: Annual meeting of the International MS Cognition Society, Amsterdam, Netherlands, June 6-7, 2019.
104. Arnold DL, Vermersch P, Cree BAC, Bar-or A, Giovannoni G, Gold R, et al. Evidence for improved myelination in patients treated with siponimod: results from the phase 3 expand mri substudy. Eur J Neurol (2020) 27(suppl 1):194–5.
105. Arnold DL, Piani-Meier D, Bar-Or A, Benedict RH, Cree BA, Giovannoni G, et al. Effect of siponimod on magnetic resonance imaging measures of neurodegeneration and myelination in secondary progressive multiple sclerosis: gray matter atrophy and magnetization transfer ratio analyses from the expand phase 3 trial. Mult Scler (2022) 28(10):1526–40. doi: 10.1177/13524585221076717
106. Lublin F, Miller DH, Freedman MS, Cree BAC, Wolinsky JS, Weiner H, et al. Oral fingolimod in primary progressive multiple sclerosis (Informs): A phase 3, randomised, double-blind, placebo-controlled trial. Lancet (2016) 387(10023):1075–84. doi: 10.1016/s0140-6736(15)01314-8
107. Cadavid D, Mellion M, Hupperts R, Edwards KR, Calabresi PA, Drulović J, et al. Safety and efficacy of opicinumab in patients with relapsing multiple sclerosis (Synergy): A randomised, placebo-controlled, phase 2 trial. Lancet Neurol (2019) 18(9):845–56. doi: 10.1016/s1474-4422(19)30137-1
108. Cree BAC, Cutter G, Wolinsky JS, Freedman MS, Comi G, Giovannoni G, et al. Safety and efficacy of md1003 (High-dose biotin) in patients with progressive multiple sclerosis (Spi2): A randomised, double-blind, placebo-controlled, phase 3 trial. Lancet Neurol (2020) 19(12):988–97. doi: 10.1016/s1474-4422(20)30347-1
109. Cree B. (2021). Elezanumab did not outperform placebo in progressive and relapsing ms, in: Ectrims 2021 Peer-Reviewed Conference Report, Dusseldorf, Germany. p. 9. Medicom Medical Publishers.
110. Krämer J, Wiendl H. What have failed, interrupted, and withdrawn antibody therapies in multiple sclerosis taught us? Neurotherapeutics (2022) 19(3):785–807. doi: 10.1007/s13311-022-01246-3
111. U.S. Food and Drug Administration. Fda Roundup: December 30, 2022. U.S. Food and Drug Administration (2022). Available at: https://www.fda.gov/news-events/press-announcements/fda-roundup-december-30-2022.
112. Sahraian MA, Mohyeddin Bonab M, Baghbanian SM, Owji M, Naser Moghadasi A. Therapeutic use of intrathecal mesenchymal stem cells in patients with multiple sclerosis: A pilot study with booster injection. Immunol Invest (2019) 48(2):160–8. doi: 10.1080/08820139.2018.1504301
113. Reich DS, Arnold DL, Vermersch P, Bar-Or A, Fox RJ, Matta A, et al. Safety and efficacy of tolebrutinib, an oral brain-penetrant btk inhibitor, in relapsing multiple sclerosis: A phase 2b, randomised, double-blind, placebo-controlled trial. Lancet Neurol (2021) 20(9):729–38. doi: 10.1016/s1474-4422(21)00237-4
114. Pender MP, Csurhes PA, Smith C, Douglas NL, Neller MA, Matthews KK, et al. Epstein-barr virus-specific T cell therapy for progressive multiple sclerosis. JCI Insight (2018) 3(22):e124714. doi: 10.1172/jci.insight.124714
115. Pender MP, Csurhes PA, Smith C, Beagley L, Hooper KD, Raj M, et al. Epstein-barr virus-specific adoptive immunotherapy for progressive multiple sclerosis. Mult Scler (2014) 20(11):1541–4. doi: 10.1177/1352458514521888
116. Owens TD, Smith PF, Redfern A, Xing Y, Shu J, Karr DE, et al. Phase 1 clinical trial evaluating safety, exposure and pharmacodynamics of btk inhibitor tolebrutinib (Prn2246, sar442168). Clin Transl Sci (2022) 15(2):442–50. doi: 10.1111/cts.13162
117. Noteboom S, Arnold D, Bar-Or A, Pender M, Hodgkinson S, Broadley S, et al. Long-term disability improvement during ebv-targeted T-cell immunotherapy ata188 is related to brain volume change and normalised magnetisation transfer ratio in T2 lesions. Mult Scler (2022) 28(3 suppl):1005–6. doi: 10.1177/13524585221126910
118. Montalban X, Arnold DL, Weber MS, Staikov I, Piasecka-Stryczynska K, Willmer J, et al. Placebo-controlled trial of an oral btk inhibitor in multiple sclerosis. N Engl J Med (2019) 380(25):2406–17. doi: 10.1056/NEJMoa1901981
119. Hartung HP, Derfuss T, Cree BA, Sormani MP, Selmaj K, Stutters J, et al. Efficacy and safety of temelimab in multiple sclerosis: results of a randomized phase 2b and extension study. Mult Scler (2021) 28(3):429–40. doi: 10.1177/13524585211024997
120. Green AJ, Gelfand JM, Cree BA, Bevan C, Boscardin WJ, Mei F, et al. Clemastine fumarate as a remyelinating therapy for multiple sclerosis (Rebuild): A randomised, controlled, double-blind, crossover trial. Lancet (2017) 390(10111):2481–9. doi: 10.1016/s0140-6736(17)32346-2
121. Boffa G, Massacesi L, Inglese M, Mariottini A, Capobianco M, Lucia M, et al. Long-term clinical outcomes of hematopoietic stem cell transplantation in multiple sclerosis. Neurology (2021) 96(8):e1215–26. doi: 10.1212/wnl.0000000000011461
122. Becker A, Martin EC, Mitchell DY, Grenningloh R, Bender AT, Laurent J, et al. Safety, tolerability, pharmacokinetics, target occupancy, and concentration-qt analysis of the novel btk inhibitor evobrutinib in healthy volunteers. Clin Transl Sci (2020) 13(2):325–36. doi: 10.1111/cts.12713
123. National Institute of Allergy and Infectious Diseases. Best Available Therapy Versus Autologous Hematopoetic Stem Cell Transplant for Multiple Sclerosis (Beat-Ms) (Beat-Ms). ClinicalTrials.gov (2022). Available at: https://clinicaltrials.gov/ct2/show/NCT04047628.
124. Study of Mesenchymal Autologous Stem Cells as Regenerative Treatment for Multiple Sclerosis (Smart-Ms). ClinicalTrials.gov (2022). Available at: https://clinicaltrials.gov/ct2/show/NCT04749667.
125. Oh J, Bar-Or A. Emerging therapies to target cns pathophysiology in multiple sclerosis. Nat Rev Neurol (2022) 18(8):465–75. doi: 10.1038/s41582-022-00675-0
126. Boschert U, Crandall T, Pereira A, Higginbotham G, Wu Y, Grenningloh R, et al. (2017). T cell mediated experimental cns autoimmunity induced by plp in sjl mice is modulated by evobrutinib (M2951) a novel bruton's tyrosine kinase inhibitor, in: Triennial Joint Meeting of the European and Americas Committees for Treatment and Research in Multiple Sclerosis, Paris, France, October 25-28, 2017.
127. Francesco M, Wong M, LaStant J, Finkle D, Loewenstein N, Macsata R, et al. (2017). Prn2246, a potent and selective blood brain barrier penetrating btk inhibitor, exhibits efficacy in central nervous system immunity, in: Triennial Joint Meeting of the European and Americas Committees for Treatment and Research in Multiple Sclerosis, Paris, France, October 25-28, 2017.
128. Gruber R, Blazier A, Lee L, Ryan S, Cheong A, Havari E, et al. Evaluating the effect of btk inhibitor tolebrutinib in human tri-culture (P1-1.Virtual). Neurology (2022) 98(18 suppl):2594.
129. Gruber RC, Chretien N, Dufault MR, Proto J, Zhang M, LaMorte M, et al. Central effects of btk inhibition in neuroinflammation. Neurology (2020) 94(15 suppl):808.
130. Weber MS, Harp C, Goodyear A, Yuen TJ, Durk MR, Kappos L. (2021). Fenebrutinib reduces disease activity in a mouse model of inflammatory multiple sclerosis associated with reduced microglial activation, in: Congress of the European Committee for Treatment and Research in Multiple Sclerosis, , October 13-15, 2021.
131. Martin E, Aigrot MS, Grenningloh R, Stankoff B, Lubetzki C, Boschert U, et al. Bruton's tyrosine kinase inhibition promotes myelin repair. Brain plasticity (Amsterdam Netherlands) (2020) 5(2):123–33. doi: 10.3233/bpl-200100
132. A Study of Efficacy and Safety of M2951 in Participants with Relapsing Multiple Sclerosis. ClinicalTrials.gov (2021). Available at: https://clinicaltrials.gov/ct2/show/NCT02975349?term=evobrutinib&cond=multiple+sclerosis&draw=2&rank=5.
133. Study to Evaluate the Efficacy and Safety of Fenebrutinib Compared with Teriflunomide in Relapsing Multiple Sclerosis (Rms) (Fenhance). ClinicalTrials.gov (2021). Available at: https://clinicaltrials.gov/ct2/show/NCT04586023.
134. A Phase 2 Study of Orelabrutinib in Patients with Relapsing-Remitting Multiple Sclerosis. ClinicalTrials.gov (2022). Available at: https://clinicaltrials.gov/ct2/show/NCT04711148.
135. Study of Evobrutinib in Participants with Rms (Evolutionrms 1). ClinicalTrials.gov (2022). Available at: https://clinicaltrials.gov/ct2/show/NCT04338022.
136. Relapsing Forms of Multiple Sclerosis (Rms) Study of Bruton's Tyrosine Kinase (Btk) Inhibitor Tolebrutinib (Sar442168) (Gemini 2). ClinicalTrials.gov (2022). Available at: https://clinicaltrials.gov/ct2/show/NCT04410991.
137. Relapsing Forms of Multiple Sclerosis (Rms) Study of Bruton's Tyrosine Kinase (Btk) Inhibitor Tolebrutinib (Sar442168) (Gemini 1). ClinicalTrials.gov (2022). Available at: https://clinicaltrials.gov/ct2/show/NCT04410978.
138. Primary Progressive Multiple Sclerosis (Ppms) Study of Bruton's Tyrosine Kinase (Btk) Inhibitor Tolebrutinib (Sar442168) (Perseus). ClinicalTrials.gov (2022). Available at: https://clinicaltrials.gov/ct2/show/NCT04458051.
139. A Study to Investigate the Efficacy of Fenebrutinib in Relapsing Multiple Sclerosis (Rms) (Fenopta). ClinicalTrials.gov (2022). Available at: https://clinicaltrials.gov/ct2/show/NCT05119569.
140. A Study to Evaluate the Efficacy and Safety of Fenebrutinib Compared with Teriflunomide in Relapsing Multiple Sclerosis (Rms) (Fenhance). ClinicalTrials.gov (2022). Available at: https://clinicaltrials.gov/ct2/show/NCT04586010.
141. Study of Evobrutinib in Participants with Rms (Evolutionrms 2). ClinicalTrials.gov (2022). Available at: https://clinicaltrials.gov/ct2/show/NCT04338061.
142. A Study to Evaluate the Efficacy and Safety of Fenebrutinib Compared with Ocrelizumab in Adult Participants with Primary Progressive Multiple Sclerosis (Fentrepid). ClinicalTrials.gov (2022). Available at: https://clinicaltrials.gov/ct2/show/NCT04544449.
143. Nonrelapsing Secondary Progressive Multiple Sclerosis (Nrspms) Study of Bruton's Tyrosine Kinase (Btk) Inhibitor Tolebrutinib (Sar442168) (Hercules). ClinicalTrials.gov (2022). Available at: https://clinicaltrials.gov/ct2/show/NCT04411641.
144. Efficacy and Safety of Remibrutinib Compared to Teriflunomide in Participants with Relapsing Multiple Sclerosis. ClinicalTrials.gov (2022). Available at: https://clinicaltrials.gov/ct2/show/NCT05147220?term=remibrutinib&draw=2&rank=2.
145. Study to Evaluate Oral Biib061 Added to Interferon-Beta1 (Ifn-B1) or Glatiramer Acetate in Relapsing Multiple Sclerosis (Rms). ClinicalTrials.gov (2021). Available at: https://clinicaltrials.gov/ct2/show/NCT04079088.
146. Mei F, Fancy SPJ, Shen YA, Niu J, Zhao C, Presley B, et al. Micropillar arrays as a high-throughput screening platform for therapeutics in multiple sclerosis. Nat Med (2014) 20(8):954–60. doi: 10.1038/nm.3618
147. Assessing Changes in Multi-Parametric Mri in Ms Patients Taking Clemastine Fumarate as a Myelin Repair Therapy (Revive). ClinicalTrials.gov (2022). Available at: https://clinicaltrials.gov/ct2/show/NCT05359653.
148. CCMR Two: A Phase Iia, Randomised, Double-Blind, Placebo-Controlled Trial of the Ability of the Combination of Metformin and Clemastine to Promote Remyelination in People with Relapsing-Remitting Multiple Sclerosis Already on Disease-Modifying Therapy. ClinicalTrials.gov (2022). Available at: https://clinicaltrials.gov/ct2/show/NCT05131828.
149. Phase 1/2 Study to Evaluate the Safety and Efficacy of Ata188 in Subjects with Progressive Multiple Sclerosis (Embold). ClinicalTrials.gov (2022). Available at: https://clinicaltrials.gov/ct2/show/NCT03283826.
150. Shaffer CL. Chapter 4 - Defining Neuropharmacokinetic Parameters in Cns Drug Discovery to Determine Cross-Species Pharmacologic Exposure–Response Relationships. In: Macor JE, editor. Annu Rep Med Chem, vol. 45 . Academic Press (2010). p. 55–70.
151. Airas L, Rissanen E, Rinne J. Imaging of microglial activation in ms using pet: research use and potential future clinical application. Mult Scler (2017) 23(4):496–504. doi: 10.1177/1352458516674568
152. Suhara T, Chaki S, Kimura H, Furusawa M, Matsumoto M, Ogura H, et al. Strategies for utilizing neuroimaging biomarkers in cns drug discovery and development: cinp/jsnp working group report. Int J Neuropsychopharmacol (2017) 20(4):285–94. doi: 10.1093/ijnp/pyw111
153. Vargas WS, Monohan E, Pandya S, Raj A, Vartanian T, Nguyen TD, et al. Measuring longitudinal myelin water fraction in new multiple sclerosis lesions. NeuroImage Clin (2015) 9:369–75. doi: 10.1016/j.nicl.2015.09.003
154. Elliott C, Wolinsky JS, Hauser SL, Kappos L, Barkhof F, Bernasconi C, et al. Slowly expanding/evolving lesions as a magnetic resonance imaging marker of chronic active multiple sclerosis lesions. Mult Scler (2019) 25(14):1915–25. doi: 10.1177/1352458518814117
155. Simmons SB, Ontaneda D. Slowly expanding lesions: A new target for progressive multiple sclerosis trials? Neurology (2022) 98(17):699–700. doi: 10.1212/wnl.0000000000200230
156. He Q, Ma Y, Fan S, Shao H, Sheth V, Bydder GM, et al. Direct magnitude and phase imaging of myelin using ultrashort echo time (Ute) pulse sequences: A feasibility study. Magn Reson Imaging (2017) 39:194–9. doi: 10.1016/j.mri.2017.02.009
157. Petzold A, Balcer LJ, Calabresi PA, Costello F, Frohman TC, Frohman EM, et al. Retinal layer segmentation in multiple sclerosis: A systematic review and meta-analysis. Lancet Neurol (2017) 16(10):797–812. doi: 10.1016/s1474-4422(17)30278-8
158. Avasarala J. It's time for combination therapies: in multiple sclerosis. Innov Clin Neurosci (2017) 14(5-6):28–30.
Keywords: sphingosine 1 phosphate receptor modulators, multiple sclerosis, ozanimod, cladribine, ponesimod, siponimod, fingolimod hydrochloride, central nervous system
Citation: Hartung H-P, Cree BAC, Barnett M, Meuth SG, Bar-Or A and Steinman L (2023) Bioavailable central nervous system disease-modifying therapies for multiple sclerosis. Front. Immunol. 14:1290666. doi: 10.3389/fimmu.2023.1290666
Received: 07 September 2023; Accepted: 09 November 2023;
Published: 29 November 2023.
Edited by:
Cris S. Constantinescu, University of Nottingham, United KingdomReviewed by:
Emma C. Tallantyre, Cardiff University, United KingdomCopyright © 2023 Hartung, Cree, Barnett, Meuth, Bar-Or and Steinman. This is an open-access article distributed under the terms of the Creative Commons Attribution License (CC BY). The use, distribution or reproduction in other forums is permitted, provided the original author(s) and the copyright owner(s) are credited and that the original publication in this journal is cited, in accordance with accepted academic practice. No use, distribution or reproduction is permitted which does not comply with these terms.
*Correspondence: Hans-Peter Hartung, aGFucy1wZXRlci5oYXJ0dW5nQHVuaS1kdWVzc2VsZG9yZi5kZQ==
†ORCID: Hans-Peter Hartung, orcid.org/0000-0002-0614-6989
Disclaimer: All claims expressed in this article are solely those of the authors and do not necessarily represent those of their affiliated organizations, or those of the publisher, the editors and the reviewers. Any product that may be evaluated in this article or claim that may be made by its manufacturer is not guaranteed or endorsed by the publisher.
Research integrity at Frontiers
Learn more about the work of our research integrity team to safeguard the quality of each article we publish.