- 1Department of Microbiology and Immunology, Geisel School of Medicine at Dartmouth, Hanover, NH, United States
- 2Department of Medicine, Geisel School of Medicine at Dartmouth, Hanover, NH, United States
- 3Center for Digestive Health, Dartmouth Health, Lebanon, NH, United States
- 4Dartmouth Cancer Center, Lebanon, NH, United States
Nearly 50 ATP-binding cassette (ABC) transporters are encoded by mammalian genomes. These transporters are characterized by conserved nucleotide-binding and hydrolysis (i.e., ATPase) domains, and power directional transport of diverse substrate classes – ions, small molecule metabolites, xenobiotics, hydrophobic drugs, and even polypeptides – into or out of cells or subcellular organelles. Although immunological functions of ABC transporters are only beginning to be unraveled, emerging literature suggests these proteins have under-appreciated roles in the development and function of T lymphocytes, including many of the key effector, memory and regulatory subsets that arise during responses to infection, inflammation or cancers. One transporter in particular, MDR1 (Multidrug resistance-1; encoded by the ABCB1 locus in humans), has taken center stage as a novel player in immune regulation. Although MDR1 remains widely viewed as a simple drug efflux pump in tumor cells, recent evidence suggests that this transporter fills key endogenous roles in enforcing metabolic fitness of activated CD4 and CD8 T cells. Here, we summarize current understanding of the physiological functions of ABC transporters in immune regulation, with a focus on the anti-oxidant functions of MDR1 that may shape both the magnitude and repertoires of antigen-specific effector and memory T cell compartments. While much remains to be learned about the functions of ABC transporters in immunobiology, it is already clear that they represent fertile new ground, both for the definition of novel immunometabolic pathways, and for the discovery of new drug targets that could be leveraged to optimize immune responses to vaccines and cancer immunotherapies.
1 Introduction
The field of immunometabolism has exploded over the past two decades. Among many notable discoveries, it is now understood that the activation and differentiation of immune cells – including T cells – involves rapid and profound metabolic reprogramming. On one hand, antigen- and TCR-mediated increases in aerobic glycolysis and mitochondrial respiration allows primed T cells to meet the bioenergetic and biosynthetic demands of cell growth and proliferation. On the other, differences in the magnitude and type of metabolic activities an antigen-primed T cell undertakes can play instructive roles in the commitment of emergent effector, memory and/or regulatory T cell subsets (1–5). CD8 effector T cells and CD4 Th17 and Th1 cells, for example, rely mainly on glycolysis to support rapid proliferation and potent effector functions, whereas CD8 memory cells and CD4 T regulatory (Treg) cells utilize mitochondrial respiration and fatty acid oxidation (FAO) to establish long-term persistence in nutrient-sparse tissues and execute immunosuppressive functions, respectively (3). It is thus not surprising that genetic or environmental perturbations to metabolic pathways have profound influence over the type and quality of T cell responses; further elucidation of the underlying mechanisms holds promises of revealing new preventative or treatment strategies for human infectious or malignant diseases.
Flux through growth-supporting metabolic pathways requires active transport of numerous organic and inorganic molecules across biological membranes. Adenosine triphosphate (ATP)-binding cassette (ABC) transporters constitute one of the largest super-families of transmembrane proteins encoded by the human genome. These transporters utilize ATP hydrolysis to power directional translocation of diverse substrates, against chemical gradients, and across lipid membranes, including the plasma membrane and membranes of intracellular organelles (6). ABC transporters are evolutionarily conserved and present throughout all kingdoms of life, from prokaryotes to humans (7), with major functions centering on either the direct promotion of cellular (e.g., lipid, heme) metabolism, or facilitating cellular detoxification via transport of potentially toxic metabolic byproducts or xenobiotic compounds (8–11). Indeed, loss of function polymorphisms in human ABC transporter loci are now linked to many human diseases, including anemia, obesity, atherosclerosis (AS), congenital cholestasis, peroxisome disorders, cystic fibrosis (CF) and Tangier disease (TD) (8, 9, 12).
More recent studies have begun to highlight direct, important and endogenous functions of ABC transporters in adaptive immune regulation generally, and development and function of T cells specifically (13–25). Here, we discuss the current state of understanding of the ABC transporters in regulating T cell differentiation and function, while also providing forward-looking perspectives as to how transport-dependent cellular metabolic pathways may intersect with antigen receptor signaling to shape T cell lineage commitment, and even the clonality of emergent effector and memory T cell pools.
2 Classification and structure of ABC transporters
48 human genes encode ABC transport proteins, most of which have direct orthologs in mice and lower vertebrates. In the early 2000’s, ABC transporters were renamed and reclassified into seven sub-families (ABCA to ABCG), based on phylogenetic analysis and sequence/structural similarity (7, 26–28). Members of the ABCE and ABCF sub-families are notable in that they do not appear to function as transporters per se, but rather participate in translational regulation and mRNA surveillance (29–32).
X-ray crystallographic studies of several ABC transporters have provided atomic-level resolution of the canonical structure of ABC transporters, and specifically the organization of four main functional domains — two nucleotide binding domains (NBD1, NBD2) and two transmembrane domains (TMD1, TMD2). Most eukaryotic ABC transporters are expressed as either a single polypeptide containing all four functional domains, or as half-transporters capable of homo- or hetero-dimerization (33). The NBD contain several conserved functional motifs, whereas the TMDs are more variable and contain 6-11 membrane-spanning α-helices which form the transmembrane pore and mediate substrate binding (33). Diversity amongst TMDs allow the various ABC transporters to bind, and subsequently transport, diverse substrate classes (e.g., heme, lipids, xenobiotics, etc.), and also underlies the phylogenetic relationships between sub-family members. In all cases, ABC transport activity involves ATP-dependent NBD dimerization, which induces conformational changes in the TMDs that exposes the inner region of the pore to the outside and allows for unidirectional transport against chemical gradients (34).
3 ABC transporters in T cells
The first described and arguably most famous ABC transporters within the immune system are the transporters associated with antigen processing (TAP1/2), which transport cytosolic peptides into the endoplasmic reticulum (ER) for loading onto MHC Class I molecules (35). Another immunologically notable ABC transporter is ABCC7 [a.k.a., cystic fibrosis transmembrane conductance regulator (CFTR)], which functions as an apical chloride channel on lung epithelium, and whose loss-of-function leads to chronic bacterial infections of the lung (36). However, numerous ABC transporters are now recognized for filling essential functions within the adaptive immune system generally, and immunometabolism specifically. The obligate mitochondrial transporters ABCB7 and ABCB10, for example, are considered heme transporters based on their roles in erythropoiesis, and are necessary for the development of B cells and CD4 memory T cells, respectively (23, 24). In addition, lipid and multi-drug transporters have recently emerged as key regulators of T cell metabolism and function (Table 1).
3.1 Lipid transporters
As many as 20 human ABC transporters are thought to transport discrete lipids or lipid metabolites. Among these, ABCA1 and ABCG1 are perhaps the best characterized for the roles they play in cholesterol transport and homeostasis, and for regulating TCR signaling intensity (13, 17, 25, 37). These transporters maintain structural organization and integrity of lipid bilayers, at least in part by regulating the number and density of cholesterol-rich lipid rafts (13, 17, 25). Given the key and ubiquitous roles of lipid rafts in signal transduction generally, and in immune cell signaling specifically, it is not surprising that these cholesterol and lipid transporters exert powerful influences over the development and function of T cells (39).
Abcg1-/- mice display larger thymuses than wild-type controls due to thymocyte hyperproliferation and an increase in total thymic cellularity (13). ABCG1 also affects thymic and peripheral development of Foxp3+ Treg cells (17). An increase in thymic (i.e., ‘natural’) Treg cells is observed when Abcg1 is conditionally ablated in T cells using Lck-Cre (17). In the periphery, preferential differentiation of naïve CD4 T cells into Tregs is evoked in the absence of ABCG1 by an increase in cellular cholesterol levels, which inhibits mammalian target of rapamycin (mTOR), triggers the phosphorylation and activation of signal transducer and activator of transcription (STAT)-5, and promotes the induction and stability of Foxp3 expression (Figure 1) (17).
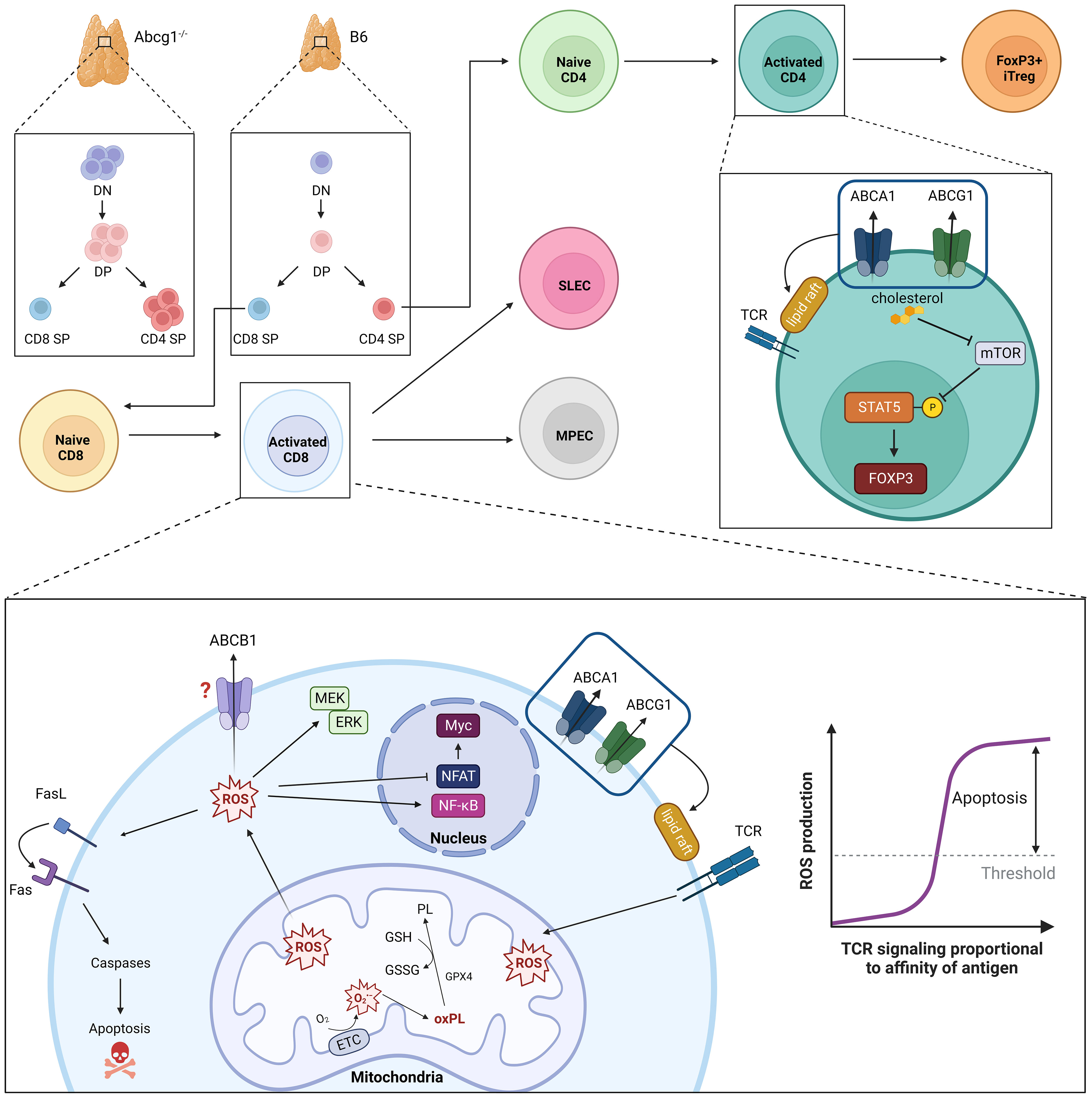
Figure 1 ABC transporters in regulation of T cell development, TCR intensity and oxidative stress. Loss of ABCG1 transport activity results in increased thymic cellularity and frequency of double negative (DN), double positive (DP) and CD4 single positive (SP) cells. Intensity of TCR signaling is modulated by ABCA1- and ABCG1-mediated phospholipid transport; loss of these transporters is associated with increased numbers and densities of cholesterol-rich lipid rafts. Parallel accumulation of intracellular cholesterol also promotes peripheral development of CD4+Foxp3+ induced (i)Treg cells. TCR signaling strength and duration is proportional to the amount of intracellular ROS produced. Moderate ROS levels (i.e., during T cell responses to low- or intermediate-affinity antigens) facilitate T cell proliferation, differentiation and survival through MEK-ERK1/2 and NF-κB activation. Conversely, elevated ROS levels (i.e., produced during T cell responses to high-affinity ligands) impair NFAT and Myc expression and promote activation-induced cell death (AICD) via Fas-Fas ligand (FasL). To maintain functional, but not toxic, ROS levels, activated T cells leverage endogenous anti-oxidant systems (e.g., glutathione peroxidase, GPX4) to reduce oxidized phospholipids (oxPL) within mitochondrial membranes using reduced glutathione (GSH) as a cofactor. MDR1 also suppresses oxidative stress in activated T cells, though underlying mechanisms remain ill-defined.
ABCA1 and ABCG1 are necessary for the maintenance of peripheral T cell homeostasis (13, 17, 25, 37). Expression of Abca1 and Abcg1 decreases following naïve T cell activation, which in turn promotes increased membrane cholesterol content to support cell growth and proliferation (38, 40). Intriguingly, ABCA1 and ABCG1 appear to work synergistically in T cells, as ablation of both Abca1 and Abcg1 in a T-cell specific manner enhances TCR signaling in CD4 and CD8 T cells (25, 37). This enhancement of TCR signaling has been attributed to an increase in lipid raft formation (13). On the other hand, upregulation of Abcg1 expression in T cells by the nuclear receptor, liver X receptor (LXR), reduces T cell proliferation (38). The need for tightly regulated lipid transport to support TCR signaling is not limited to conventional T cells; ABCG1, as well as another lipid transporter, ABCA7, are both essential for thymic maturation of invariant natural killer T (iNKT) cells (14, 20). Hence, regulation of cholesterol efflux by ABCA1, ABCG1 and ABCA7 each appear to participate in the regulation of signal transduction, and in establishing a full complement of peripheral lymphocytes.
3.2 Multi-drug transporters
Another class of ABC transporters recently implicated in immune regulation are the so-called multi-drug transporters. This historical classification derives from a combination of functional studies in eukaryotic cells and examination of homologs in bacterial model systems, which together show that multi-drug transporters are capable of effluxing a variety of structurally-unrelated cytostatic drugs from tumor cells (18). However, considering the repeated and high-profile failures of drugs designed to block multi-drug transporters in clinical cancer studies (41), and the emerging endogenous functions of at least some of these transporters, this semantic classification may require updating. Indeed, ‘multi-drug’ transporters – including multidrug resistance protein 1 (MDR1/ABCB1), multidrug resistance protein 4 (MRP4/ABCC4) and breast cancer resistance protein (BCRP1/ABCG2) – each display considerable evolutionary conservation and thus may also be considered ‘orphan’ transporters, as endogenous transport substrates have not yet been described. An important and common theme among these multi-drug transporters is their incredibly broad substrate specificity; MDR1, for example, has been proposed to efflux a variety of synthetic drugs, antiretroviral drugs, glucocorticoids, lipids, fluorescent dyes and even peptide hormones (in yeast) (42–45). Yet many of these same transporters display endogenous antioxidant functions in several cell types and tissues during normal physiology, including in T cells. Thus, it is worth considering that the core evolutionary function of ‘multi-drug’ transporters centers on the regulation of oxidation-reduction (redox) metabolism, which have become masked in recent decades by their proclivity to efflux 20th century medicines.
MDR1 is the most extensively characterized multi-drug transporter within the ABC family for its role as a regulator of oxidative stress. MDR1 is endogenously expressed in a variety of normal cell types and tissues, with highest levels seen in the liver, intestines, brain, kidney and adrenal glands (46–48). In the hematopoietic system, comprehensive profiling of a fluorescent Abcb1a-ametrine reporter allele in mice showed that MDR1 is expressed in a number of mature innate and adaptive lymphocytes, but is notably absent in most hematopoietic stem and progenitor cells, early thymocytes, and also mature granulocyte and B lymphocyte lineages (22). In CD4 T cells, MDR1 expression is absent in naïve cells, low in Foxp3+ Treg cells, but increased markedly in pro-inflammatory effector subsets, namely IFNγ-secreting Th1 and IL-17-producing Th17 cells, in particular following infiltration of small intestine lamina propria (15). In contrast to this conditional expression in CD4 T cells, MDR1 expression is both constitutive and developmentally regulated in cytotoxic lymphocyte lineages (e.g., NK cells, iNKT cells, CD8 T cells) where the expression of MDR1 is at least partly controlled by Runx family transcription factors (22). Expression of MDR1 (and ABCG2) are also characteristic of tissue-resident memory (Trm) CD8 T cells, as well as of human mucosal associated invariant T (MAIT) cells (16, 19, 49). In line with constitutively high MDR1 expression, mouse CD8 T cells null for MDR1 transport activity (Abcb1a/b-/-) are incapable of becoming productively activated, of accumulating efficiently in response to acute viral or bacterial infections, and of forming functional memory cells (22). By contrast, naïve CD8 T cells do not require MDR1 function for steady-state persistence (22), which suggests that MDR1 transport activity in CD8 T cells is primarily called upon after TCR-stimulation. Indeed, the inability of Abcb1a/b-/- CD8 T cells to accumulate following TCR-stimulation is due to increased cell death, not reduced proliferation, and coincides with a failure of these cells to suppress oxidative stress and maintain functional mitochondria (Figure 1) (22). Others have shown that Th17 cells require both MDR1 and ABCC4 to suppress oxidative stress during states of hypoxia (21). Further, high MDR1 expression in Th1 and Th17 cells in the distal small intestine (i.e., ileum) counters oxidative stress induced by naturally circulating bile acids, an abundant class of liver-derived lipid-emulsifying metabolites that are also potent oxidizing agents (18, 50). Even outside of the immune system, MDR1 has been shown to suppress oxidative stress and safeguard mitochondrial integrity in colonic epithelial cells (51). Collectively, these findings support a broader and more fundamental role for the MDR1 transporter in suppressing oxidative stress upon T cell exposure to intra- or extra-cellular oxidizing agents (e.g., toxic metabolic byproducts, bile acids, etc.). Such transport-dependent antioxidant functions of MDR1 raise intriguing new concepts in the convergence of TCR signaling, metabolic reprogramming, oxidative stress, and even clonal selection in T cells.
4 Determinants of peripheral T cell responses: TCR signaling and ROS
T cell proliferation, lineage commitment, and the execution of effector or regulatory functions are all markedly influenced by the strength and duration of TCR/peptide-MHC engagement (52–54). Upon infection, the size of the antigen-specific CD8 T cell compartment correlates directly with the duration of antigen exposure (55). Strength and duration of TCR signaling also shapes both CD4 and CD8 memory T cell compartments, as highlighted by the preferential skewing of the memory pool towards clones with higher-affinity TCRs (55–57). It is increasingly clear that TCR signaling thresholds also regulate functional outcomes of individual T cell clones (e.g., proliferative capacity, differentiation, etc.); pathways that regulate successful integration of TCR, co-stimulatory and cytokine signaling pathways thus determine the magnitude, type and antigen-specificities of T cell responses.
Proximal TCR signaling induces rapid Ca2+ release into the cytosol from reserves in the endoplasmic reticulum (ER), which upon being emptied, activate extracellular Ca2+ influx across the plasma membrane (58, 59). Besides serving as a potent signal to activate early transcription factors, such as the NFATs, cytosolic Ca2+ is also taken up in mass by mitochondria, which facilitates ATP synthesis and promotes corresponding increases in levels of reactive oxygen species (ROS) (60). This increase in intracellular ROS occurs within minutes of TCR activation, with the majority of ROS production in activated T cells owing to mitochondrial superoxide (O2•−) that “leak” from complexes I and III of the mitochondrial electron transport chain (ETC) (61).
ROS function as key secondary messengers in T cells and are required, at moderate levels, to promote proliferation, differentiation and survival. The activation of extracellular signal-regulated kinase (ERK) 1/2, for example, is highly ROS-dependent, and promotes activation and translocation of several transcription factors, including AP-1 family members, that are necessary for T cell growth and proliferation (62, 63). ROS production has also been implicated in promoting IL-2 production in activated T cells through NF-κB activation (64). At the same time, inappropriately elevated ROS levels are highly cytotoxic, and can stimulate apoptosis by covalently modifying and damaging proteins, nucleic acids, and lipids (65). Oxidative stress also promotes increased expression of Fas ligand (FasL), which on binding to Fas initiates the recruitment and activation of caspases causing activation induced cell death (AICD) (Figure 1) (62, 66, 67).
Considering the dual nature of intracellular ROS – where ROS is both required for T cell activation, but also toxic at increased levels – activated T cells upregulate a host of anti-oxidant enzymes (superoxide dismutases (SODs), catalases, glutathione peroxidases (GPXs), glutathione reductases, etc.) to convert ROS into less reactive products, or to increase production of ROS scavenging molecules, such as glutathione, ascorbate, pyruvate, α-ketoglutarate, and oxaloacetate (65). Disruptions in these anti-oxidant systems result in elevated ROS production and affect metabolic reprogramming in developing T cells. Inhibition of glutathione production, which is important for ROS buffering, negatively affects TCR-induced Myc expression and NFAT activation, which are essential for activated CD8 T cells to switch to glycolytic metabolism (68). Similarly, loss of Gpx4 impairs CD8 T cell responses to viral infections due to accumulation of lipid peroxides, leading to death by ferroptosis (69). Thus, productive immune responses demand that T cells tightly regulate both the production and scavenging of intracellular ROS. Discrete roles for ABC transporters in T cell redox regulation and metabolism remain unclear, but stand to provide exciting new molecular insights into the formation and regulation of effector and memory T cells.
5 Conclusions and future perspectives
Considering that both the developmental trajectories and functions of individual T cell clones involve unique TCR signaling dynamics, and thus discrete redox demands, it becomes increasingly important to decipher whether and how ABC transporters not only regulate the magnitude of monoclonal (i.e., TCR transgenic) T cell responses, but also the selection of T cell clones specific for high- vs. low-affinity antigens. Given the preponderance of available evidence, it is reasonable to expect that MDR1, and perhaps other lipid and multidrug transporters, preferentially regulate T cell responses to abundant and high-affinity (i.e., immunodominant) antigens, in which the highest levels of intracellular ROS are generated. If true, these pathways (and ABC transporters) may prove vital for advancing the next generation of medicines that can improve T cell-mediated immunity to infectious diseases and cancers, where the survival of T cells specific for immunodominant antigens are paramount for efficacy.
Despite being one of the largest families of human transmembrane proteins, a comprehensive understanding of endogenous ABC transporter functions remains lacking. Historically, this has been due to the inherent difficulty of working with membrane proteins and transporters, as well as a dearth of contemporary research reagents (e.g., engineered mouse alleles, antibodies, etc.). While significant strides have been made in recent decades with regard to the structural organization of these transporters, meaningful insights into the physiological and immunological functions of these transporters have lagged. Mechanistic understanding of these transporters remains largely based on data from pharmacological and in vitro studies, but it is becoming increasingly apparent that these transporters have important and context-dependent functions that need to be evaluated in vivo, during both normal- and patho-physiology. This is especially true for transporters, like MDR1, which remain classified as ‘multi-drug’ efflux pumps, despite continued elaboration of new and potent endogenous functions in in vivo mouse and ex vivo human systems. For these, the key unanswered question is why would an ABC transporter be conserved throughout evolution unless it serves a core endogenous function? The continued pursuit of basic science, and the advancement of next-generation medicines, await answers.
Author contributions
MS: Conceptualization, Funding acquisition, Supervision, Writing – review & editing. AB: Conceptualization, Writing – original draft, Writing – review & editing.
Funding
The author(s) declare financial support was received for the research, authorship, and/or publication of this article. This work was supported by startup funds provided to the Sundrud Lab by the Dartmouth Cancer Center.
Acknowledgments
All figures were created using BioRender.com.
Conflict of interest
The authors declare that the research was conducted in the absence of any commercial or financial relationships that could be construed as a potential conflict of interest.
Publisher’s note
All claims expressed in this article are solely those of the authors and do not necessarily represent those of their affiliated organizations, or those of the publisher, the editors and the reviewers. Any product that may be evaluated in this article, or claim that may be made by its manufacturer, is not guaranteed or endorsed by the publisher.
References
1. van der Windt GJ, Everts B, Chang CH, Curtis JD, Freitas TC, Amiel E, et al. Mitochondrial respiratory capacity is a critical regulator of CD8+ T cell memory development. Immunity (2012) 36(1):68–78. doi: 10.1016/j.immuni.2011.12.007
2. Michalek RD, Gerriets VA, Jacobs SR, Macintyre AN, MacIver NJ, Mason EF, et al. Cutting edge: distinct glycolytic and lipid oxidative metabolic programs are essential for effector and regulatory CD4+ T cell subsets. J Immunol (2011) 186(6):3299–303. doi: 10.4049/jimmunol.1003613
3. Geltink RIK, Kyle RL, Pearce EL. Unraveling the complex interplay between T cell metabolism and function. Annu Rev Immunol (2018) 36:461–88. doi: 10.1146/annurev-immunol-042617-053019
4. MacIver NJ, Michalek RD, Rathmell JC. Metabolic regulation of T lymphocytes. Annu Rev Immunol (2013) 31:259–83. doi: 10.1146/annurev-immunol-032712-095956
5. Ma EH, Verway MJ, Johnson RM, Roy DG, Steadman M, Hayes S, et al. Metabolic profiling using stable isotope tracing reveals distinct patterns of glucose utilization by physiologically activated CD8(+) T cells. Immunity (2019) 51(5):856–870 e5. doi: 10.1016/j.immuni.2019.09.003
6. Rees DC, Johnson E, Lewinson O. ABC transporters: the power to change. Nat Rev Mol Cell Biol (2009) 10(3):218–27. doi: 10.1038/nrm2646
7. Higgins CF. ABC transporters: from microorganisms to man. Annu Rev Cell Biol (1992) 8:67–113. doi: 10.1146/annurev.cb.08.110192.000435
8. Yamamoto M, Arimura H, Fukushige T, Minami K, Nishizawa Y, Tanimoto A, et al. Abcb10 role in heme biosynthesis in vivo: Abcb10 knockout in mice causes anemia with protoporphyrin IX and iron accumulation. Mol Cell Biol (2014) 34(6):1077–84. doi: 10.1128/MCB.00865-13
9. Ichikawa Y, Bayeva M, Ghanefar M, Potini V, Sun L, Mutharasan RK, et al. Disruption of ATP-binding cassette B8 in mice leads to cardiomyopathy through a decrease in mitochondrial iron export. Proc Natl Acad Sci USA (2012) 109(11):4152–7. doi: 10.1073/pnas.1119338109
10. Schaedler TA, Thornton JD, Kruse I, Schwarzländer M, Meyer AJ, van Veen HW, et al. A conserved mitochondrial ATP-binding cassette transporter exports glutathione polysulfide for cytosolic metal cofactor assembly. J Biol Chem (2014) 289(34):23264–74. doi: 10.1074/jbc.M114.553438
11. Wang X, Collins HL, Ranalletta M, Fuki IV, Billheimer JT, Rothblat GH, et al. Macrophage ABCA1 and ABCG1, but not SR-BI, promote macrophage reverse cholesterol transport. vivo. J Clin Invest (2007) 117(8):2216–24. doi: 10.1172/JCI32057
12. Fitzgerald ML, Mujawar Z, Tamehiro N. ABC transporters, atherosclerosis and inflammation. Atherosclerosis (2010) 211(2):361–70. doi: 10.1016/j.atherosclerosis.2010.01.011
13. Armstrong AJ, Gebre AK, Parks JS, Hedrick CC. ATP-binding cassette transporter G1 negatively regulates thymocyte and peripheral lymphocyte proliferation. J Immunol (2010) 184(1):173–83. doi: 10.4049/jimmunol.0902372
14. Sag D, Wingender G, Nowyhed H, Wu R, Gebre AK, Parks JS, et al. ATP-binding cassette transporter G1 intrinsically regulates invariant NKT cell development. J Immunol (2012) 189(11):5129–38. doi: 10.4049/jimmunol.1201570
15. Ramesh R, Kozhaya L, McKevitt K, Djuretic IM, Carlson TJ, Quintero MA, et al. Pro-inflammatory human Th17 cells selectively express P-glycoprotein and are refractory to glucocorticoids. J Exp Med (2014) 211(1):89–104. doi: 10.1084/jem.20130301
16. Boddupalli CS, Nair S, Gray SM, Nowyhed HN, Verma R, Gibson JA, et al. ABC transporters and NR4A1 identify a quiescent subset of tissue-resident memory T cells. J Clin Invest (2016) 126(10):3905–16. doi: 10.1172/JCI85329
17. Cheng HY, Gaddis DE, Wu R, McSkimming C, Haynes LD, Taylor AM, et al. Loss of ABCG1 influences regulatory T cell differentiation and atherosclerosis. J Clin Invest (2016) 126(9):3236–46. doi: 10.1172/JCI83136
18. Cao W, Kayama H, Chen ML, Delmas A, Sun A, Kim SY, et al. The xenobiotic transporter mdr1 enforces T cell homeostasis in the presence of intestinal bile acids. Immunity (2017) 47(6):1182–1196.e10. doi: 10.1016/j.immuni.2017.11.012
19. Fergusson JR, Ussher JE, Kurioka A, Klenerman P, Walker LJ. High MDR-1 expression by MAIT cells confers resistance to cytotoxic but not immunosuppressive MDR-1 substrates. Clin Exp Immunol (2018) 194(2):180–91. doi: 10.1111/cei.13165
20. Nowyhed HN, Chandra S, Kiosses W, Marcovecchio P, Andary F, Zhao M, et al. ATP binding cassette transporter ABCA7 regulates NKT cell development and function by controlling CD1d expression and lipid raft content. Sci Rep (2017) 7:40273. doi: 10.1038/srep40273
21. Xie A, Robles RJ, Mukherjee S, Zhang H, Feldbrügge L, Csizmadia E, et al. HIF-1α-induced xenobiotic transporters promote Th17 responses in Crohn’s disease. J Autoimmun (2018) 94:122–33. doi: 10.1016/j.jaut.2018.07.022
22. Chen ML, Sun A, Cao W, Eliason A, Mendez KM, Getzler AJ, et al. Physiological expression and function of the MDR1 transporter in cytotoxic T lymphocytes. J Exp Med (2020) 217(5):e20191388. doi: 10.1084/jem.20191388
23. Lehrke MJ, Shapiro MJ, Rajcula MJ, Kennedy MM, McCue SA, Medina KL, et al. The mitochondrial iron transporter ABCB7 is required for B cell development, proliferation, and class switch recombination in mice. Elife (2021) 10:e69621. doi: 10.7554/eLife.69621
24. Sun W, Jia X, Liesa M, Tantin D, Ward DM. ABCB10 loss reduces CD4+ T cell activation and memory formation. J Immunol (2022) 208(2):328–37. doi: 10.4049/jimmunol.2100514
25. Zhao Y, Zhang L, Liu L, Zhou X, Ding F, Yang Y, et al. Specific loss of ABCA1 (ATP-binding cassette transporter A1) suppresses TCR (T-cell receptor) signaling and provides protection against atherosclerosis. Arterioscler Thromb Vasc Biol (2022) 42(12):e311–26. doi: 10.1161/ATVBAHA.122.318226
26. Dean M, Rzhetsky A, Allikmets R. The human ATP-binding cassette (ABC) transporter superfamily. Genome Res (2001) 11(7):1156–66. doi: 10.1101/gr.184901
27. Borst P, Elferink RO. Mammalian ABC transporters in health and disease. Annu Rev Biochem (2002) 71:537–92. doi: 10.1146/annurev.biochem.71.102301.093055
28. Dean M, Annilo T. Evolution of the ATP-binding cassette (ABC) transporter superfamily in vertebrates. Annu Rev Genomics Hum Genet (2005) 6:123–42. doi: 10.1146/annurev.genom.6.080604.162122
29. Hassel BA, Zhou A, Sotomayor C, Maran A, Silverman RH. A dominant negative mutant of 2-5A-dependent RNase suppresses antiproliferative and antiviral effects of interferon. EMBO J (1993) 12(8):3297–304. doi: 10.1002/j.1460-2075.1993.tb05999.x
30. Bisbal C, Martinand C, Silhol M, Lebleu B, Salehzada T. Cloning and characterization of a RNAse L inhibitor. A New component interferon-regulated 2-5A pathway. J Biol Chem (1995) 270(22):13308–17. doi: 10.1074/jbc.270.22.13308
31. Tyzack JK, Wang X, Belsham GJ, Proud CG. ABC50 interacts with eukaryotic initiation factor 2 and associates with the ribosome in an ATP-dependent manner. J Biol Chem (2000) 275(44):34131–9. doi: 10.1074/jbc.M002868200
32. Zhao Z, Fang LL, Johnsen R, Baillie DL. ATP-binding cassette protein E is involved in gene transcription and translation in Caenorhabditis elegans. Biochem Biophys Res Commun (2004) 323(1):104–11. doi: 10.1016/j.bbrc.2004.08.068
33. Thomas C, Tampé R. Multifaceted structures and mechanisms of ABC transport systems in health and disease. Curr Opin Struct Biol (2018) 51:116–28. doi: 10.1016/j.sbi.2018.03.016
34. Alam A, Locher KP. Structure and mechanism of human ABC transporters. Annu Rev Biophys (2023) 52:275–300. doi: 10.1146/annurev-biophys-111622-091232
35. Ritz U, Seliger B. The transporter associated with antigen processing (TAP): structural integrity, expression, function, and its clinical relevance. Mol Med (2001) 7(3):149–58. doi: 10.1007/BF03401948
36. Corradi V, Vergani P, Tieleman DP. Cystic fibrosis transmembrane conductance regulator (CFTR): CLOSED AND OPEN STATE CHANNEL MODELS. J Biol Chem (2015) 290(38):22891–906. doi: 10.1074/jbc.M115.665125
37. Bazioti V, La Rose AM, Maassen S, Bianchi F, de Boer R, Halmos B, et al. T cell cholesterol efflux suppresses apoptosis and senescence and increases atherosclerosis in middle aged mice. Nat Commun (2022) 13(1):3799. doi: 10.1038/s41467-022-31135-4
38. Bensinger SJ, Bradley MN, Joseph SB, Zelcer N, Janssen EM, Hausner MA, et al. LXR signaling couples sterol metabolism to proliferation in the acquired immune response. Cell (2008) 134(1):97–111. doi: 10.1016/j.cell.2008.04.052
39. Jury EC, Flores-Borja F, Kabouridis PS. Lipid rafts in T cell signalling and disease. Semin Cell Dev Biol (2007) 18(5):608–15. doi: 10.1016/j.semcdb.2007.08.002
40. Yang W, Bai Y, Xiong Y, Zhang J, Chen S, Zheng X, et al. Potentiating the antitumour response of CD8(+) T cells by modulating cholesterol metabolism. Nature (2016) 531(7596):651–5. doi: 10.1038/nature17412
41. Waghray D, Zhang Q. Inhibit or evade multidrug resistance P-glycoprotein in cancer treatment. J Med Chem (2018) 61(12):5108–21. doi: 10.1021/acs.jmedchem.7b01457
42. Young L, Leonhard K, Tatsuta T, Trowsdale J, Langer T. Role of the ABC transporter Mdl1 in peptide export from mitochondria. Science (2001) 291(5511):2135–8. doi: 10.1126/science.1056957
43. Sakaeda T, Nakamura T, Okumura K. MDR1 genotype-related pharmacokinetics and pharmacodynamics. Biol Pharm Bull (2002) 25(11):1391–400. doi: 10.1248/bpb.25.1391
44. Minuesa G, Arimany-Nardi C, Erkizia I, Cedeño S, Moltó J, Clotet B, et al. P-glycoprotein (ABCB1) activity decreases raltegravir disposition in primary CD4+P-gphigh cells and correlates with HIV-1 viral load. J Antimicrob Chemother (2016) 71(10):2782–92. doi: 10.1093/jac/dkw215
45. Whyte-Allman SK, Kaul R, Bendayan R. Regulation of ABC drug efflux transporters in human T-cells exposed to an HIV pseudotype. Front Pharmacol (2021) 12:711999. doi: 10.3389/fphar.2021.711999
46. Sugawara I, Kataoka I, Morishita Y, Hamada H, Tsuruo T, Itoyama S, et al. Tissue distribution of P-glycoprotein encoded by a multidrug-resistant gene as revealed by a monoclonal antibody, MRK 16. Cancer Res (1988) 48(7):1926–9.
47. Thiebaut F, Tsuruo T, Hamada H, Gottesman MM, Pastan I, Willingham MC. Cellular localization of the multidrug-resistance gene product P-glycoprotein in normal human tissues. Proc Natl Acad Sci USA (1987) 84(21):7735–8. doi: 10.1073/pnas.84.21.7735
48. Thiebaut F, Tsuruo T, Hamada H, Gottesman MM, Pastan I, Willingham MC. Immunohistochemical localization in normal tissues of different epitopes in the multidrug transport protein P170: evidence for localization in brain capillaries and crossreactivity of one antibody with a muscle protein. J Histochem Cytochem: Off J Histochem Soc (1989) 37(2):159–64. doi: 10.1177/37.2.2463300
49. Dusseaux M, Martin E, Serriari N, Péguillet I, Premel V, Louis D, et al. Human MAIT cells are xenobiotic-resistant, tissue-targeted, CD161hi IL-17-secreting T cells. Blood (2011) 117(4):1250–9. doi: 10.1182/blood-2010-08-303339
50. Bernstein H, Bernstein C, Payne CM, Dvorak K. Bile acids as endogenous etiologic agents in gastrointestinal cancer. World J Gastroenterol: WJG (2009) 15(27):3329–40. doi: 10.3748/wjg.15.3329
51. Ho GT, Aird RE, Liu B, Boyapati RK, Kennedy NA, Dorward DA, et al. MDR1 deficiency impairs mitochondrial homeostasis and promotes intestinal inflammation. Mucosal Immunol (2018) 11(1):120–30. doi: 10.1038/mi.2017.31
52. Constant S, Pfeiffer C, Woodard A, Pasqualini T, Bottomly K. Extent of T cell receptor ligation can determine the functional differentiation of naive CD4+ T cells. J Exp Med (1995) 182(5):1591–6. doi: 10.1084/jem.182.5.1591
53. Fazilleau N, McHeyzer-Williams LJ, Rosen H, McHeyzer-Williams MG. The function of follicular helper T cells is regulated by the strength of T cell antigen receptor binding. Nat Immunol (2009) 10(4):375–84. doi: 10.1038/ni.1704
54. Marchingo JM, Kan A, Sutherland RM, Duffy KR, Wellard CJ, Belz GT, et al. T cell signaling. Antigen affinity, costimulation, and cytokine inputs sum linearly to amplify T cell expansion. Science (2014) 346(6213):1123–7. doi: 10.1126/science.1260044
55. Kretschmer L, Flossdorf M, Mir J, Cho YL, Plambeck M, Treise I, et al. Differential expansion of T central memory precursor and effector subsets is regulated by division speed. Nat Commun (2020) 11(1):113. doi: 10.1038/s41467-019-13788-w
56. Daniels MA, Teixeiro E. TCR signaling in T cell memory. Front Immunol (2015) 6:617. doi: 10.3389/fimmu.2015.00617
57. Akondy RS, Johnson PLF, Nakaya HI, Edupuganti S, Mulligan MJ, Lawson B, et al. Initial viral load determines the magnitude of the human CD8 T cell response to yellow fever vaccination. Proc Natl Acad Sci USA (2015) 112(10):3050–5. doi: 10.1073/pnas.1500475112
58. Weiss A, Imboden J, Shoback D, Stobo J. Role of T3 surface molecules in human T-cell activation: T3-dependent activation results in an increase in cytoplasmic free calcium. Proc Natl Acad Sci USA (1984) 81(13):4169–73. doi: 10.1073/pnas.81.13.4169
59. Trebak M, Kinet JP. Calcium signalling in T cells. Nat Rev Immunol (2019) 19(3):154–69. doi: 10.1038/s41577-018-0110-7
60. Görlach A, Bertram K, Hudecova S, Krizanova O. Calcium and ROS: A mutual interplay. Redox Biol (2015) 6:260–71. doi: 10.1016/j.redox.2015.08.010
61. Kowaltowski AJ, de Souza-Pinto NC, Castilho RF, Vercesi AE. Mitochondria and reactive oxygen species. Free Radical Biol Med (2009) 47(4):333–43. doi: 10.1016/j.freeradbiomed.2009.05.004
62. Devadas S, Zaritskaya L, Rhee SG, Oberley L, Williams MS. Discrete generation of superoxide and hydrogen peroxide by T cell receptor stimulation: selective regulation of mitogen-activated protein kinase activation and fas ligand expression. J Exp Med (2002) 195(1):59–70. doi: 10.1084/jem.20010659
63. Jackson SH, Devadas S, Kwon J, Pinto LA, Williams MS. T cells express a phagocyte-type NADPH oxidase that is activated after T cell receptor stimulation. Nat Immunol (2004) 5(8):818–27. doi: 10.1038/ni1096
64. Kamiński MM, Sauer SW, Kamiński M, Opp S, Ruppert T, Grigaravičius P, et al. T cell activation is driven by an ADP-dependent glucokinase linking enhanced glycolysis with mitochondrial reactive oxygen species generation. Cell Rep (2012) 2(5):1300–15. doi: 10.1016/j.celrep.2012.10.009
65. Belikov AV, Schraven B, Simeoni L. T cells and reactive oxygen species. J Biomed Sci (2015) 22:85. doi: 10.1186/s12929-015-0194-3
66. Kamiński MM, Röth D, Sass S, Sauer SW, Krammer PH, Gülow K. Manganese superoxide dismutase: a regulator of T cell activation-induced oxidative signaling and cell death. Biochim Biophys Acta (2012) 1823(5):1041–52. doi: 10.1016/j.bbamcr.2012.03.003
67. Hildeman DA, Mitchell T, Kappler J, Marrack P. T cell apoptosis and reactive oxygen species. J Clin Invest (2003) 111(5):575–81. doi: 10.1172/JCI200318007
68. Mak TW, Grusdat M, Duncan GS, Dostert C, Nonnenmacher Y, Cox M, et al. Glutathione primes T cell metabolism for inflammation. Immunity (2017) 46(4):675–89. doi: 10.1016/j.immuni.2017.03.019
Keywords: ABC transporters, MDR1, P-glycoprotein, metabolism, reactive oxygen species, TCR signaling, oxidative stress, redox
Citation: Balasubramanian A and Sundrud MS (2023) ATP-dependent transporters: emerging players at the crossroads of immunity and metabolism. Front. Immunol. 14:1286696. doi: 10.3389/fimmu.2023.1286696
Received: 31 August 2023; Accepted: 11 October 2023;
Published: 31 October 2023.
Edited by:
Silvia Monticelli, Institute for Research in Biomedicine (IRB), SwitzerlandReviewed by:
Marco Craveiro, National Institutes of Health (NIH), United StatesCopyright © 2023 Balasubramanian and Sundrud. This is an open-access article distributed under the terms of the Creative Commons Attribution License (CC BY). The use, distribution or reproduction in other forums is permitted, provided the original author(s) and the copyright owner(s) are credited and that the original publication in this journal is cited, in accordance with accepted academic practice. No use, distribution or reproduction is permitted which does not comply with these terms.
*Correspondence: Mark S. Sundrud, bWFyay5zdW5kcnVkQGRhcnRtb3V0aC5lZHU=