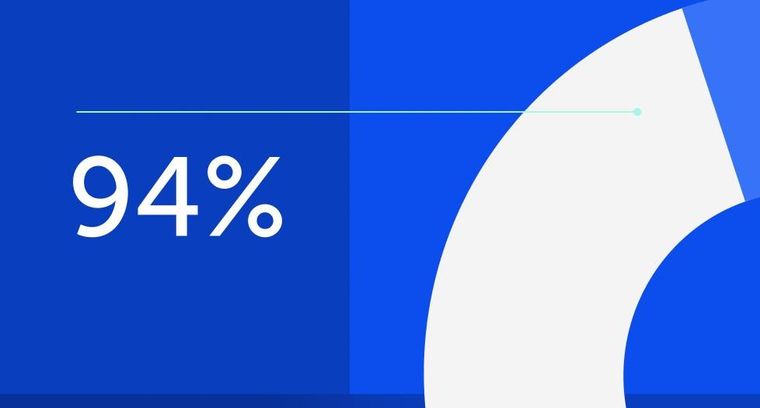
94% of researchers rate our articles as excellent or good
Learn more about the work of our research integrity team to safeguard the quality of each article we publish.
Find out more
REVIEW article
Front. Immunol., 24 November 2023
Sec. Cancer Immunity and Immunotherapy
Volume 14 - 2023 | https://doi.org/10.3389/fimmu.2023.1285801
This article is part of the Research TopicRecent advances in gene modified immune cells and oncolytic virus for cancer immunotherapyView all 8 articles
γδ T cells, a specialized subset of T lymphocytes, have garnered significant attention within the realm of cancer immunotherapy. Operating at the nexus between adaptive and innate immunological paradigms, these cells showcase a profound tumor discernment repertoire, hinting at novel immunotherapeutic strategies. Significantly, these cells possess the capability to directly identify and eliminate tumor cells without reliance on HLA-antigen presentation. Furthermore, γδ T cells have the faculty to present tumor antigens to αβ T cells, amplifying their anti-tumoral efficacy.Within the diverse and heterogeneous subpopulations of γδ T cells, distinct immune functionalities emerge, manifesting either anti-tumor or pro-tumor roles within the tumor microenvironment. Grasping and strategically harnessing these heterogeneous γδ T cell cohorts is pivotal to their integration in tumor-specific immunotherapeutic modalities. The aim of this review is to describe the heterogeneity of the γδ T cell lineage and the functional plasticity it generates in the treatment of malignant tumors. This review endeavors to elucidate the intricate heterogeneity inherent to the γδ T cell lineage, the consequential functional dynamics in combating malignancies, the latest advancements from clinical trials, and the evolving landscape of γδ T cell-based oncological interventions, while addressing the challenges impeding the field.
There is a distinct and conserved population of T lymphocytes called γδ T cells, named for the γ and δ chains making up the T cell receptor (TCR) that sets them apart from the classical T cells (CD4+ and CD8+) that contain αβ TCRs. They represent a distinctive subset of T cells that exist in a transitional state between the adaptive and innate immune systems (1–3). Functionally, there is compelling evidence to suggest that their antigen receptors exhibit greater specificity and diversity compared to the surface antigen receptors found in αβ T cells or B cells (4). γδ T cells play an important role as the first line of defense of the immune system while also participating in the adaptive immune response. Serving as a conduit between innate and adaptive immunity to elicit potent reactions (5), γδ T cells are viewed as promising immunotherapeutic agents within the realm of cancer treatment, offering a fresh perspective in the field of anti-tumor immunity (6, 7).
In many mammalian species, γδ T cells emerge as the primary lymphocyte subset during fetal development (8, 9). Their receptor, composed of a γ and a δ chain, is formed through somatic variable-diversity-joining (V(D)J) recombination, similar to the segments of α- and β-chains in αβ TCRs (10). There are numerous configurations for the T cell receptor’s γδ variable region (Vγ) and delta chain variable region (Vδ), and the fusion of these two regions allows for the formation of a sizable collection of roughly 1020 TCR clonotypes (11), providing significant diversity to γδ T cell subsets.Human γδ T cells are traditionally classified into three primary subgroups: Vδ1, Vδ2, and Vδ3, determined by the Vδ chain usage (12–14). Among the three main γδ T cell subsets in humans, Vδ1 T cells predominantly pair with the Vγ I family, which includes (Vγ2/3/4/5/8), and the Vδ2 subset predominantly binds Vγ II (Vγ9), typically Vγ9Vδ2 T cells (15). Unique tissue localization, activation, and function are displayed by various γδ T cell subsets and their distribution within the human body can be distinguished clearly (16–19). Vδ1 T cells are predominantly located in epithelial tissues, including the intestines and skin, as well as organs like the spleen and liver. These cells have a vital function in safeguarding the preservation of epithelial tissue integrity (20). Vδ2 T cells, mainly the Vγ9Vδ2T cell subset, account for approximately 60-95% of peripheral γδ T cells in the circulation (21). These cells make up around 2-5% of the circulating CD3+ T cell population and play a dual role as both effector cells and antigen-presenting cells (APCs) (22). Vδ3 T cells, which are infrequently observed in circulatory systems, are notably prevalent in hepatic tissues, particularly in individuals with infections or malignancies.
The tumor microenvironment (TME) significantly influences the activity of γδ T cells across various cancers. In the complex TME, γδ T cells are recruited or activated toward the tumor site. However, there also exists a synergistic or pleiotropic effect of tumor cells and multiple factors in the TME, where infiltrating γδ T cells are activated or depleted, or polarized to a tumor-promoting phenotype, thus supporting cancer progression (18).
Some investigators have analyzed the effect of the TME on γδ T cell recruitment in a preclinical transplantable B16 melanoma model, where human Vδ1 T cells use the CCR2/CCL2 pathway to migrate toward the tumor, where they exert critical non-redundant anti-tumor functions (23). Consistent with this study, Vδ1 T cell infiltration was abundant in breast and primary prostate cancers with significantly upregulated CCL2 expression (24, 25). Furthermore, in cases of hepatocellular carcinoma (HCC), tumor cells harness the CCL4/CCL5 chemokine pathway, interacting with the CCR1/CCR5 receptors, thereby orchestrating the mobilization of γδ T cells either from the peripheral blood or peritumor region to the tumor region (26). In the TME of breast cancer, breast cancer cells secrete IP-10, which mediates the transport and migration of γδ1 T cells to the tumor site via IP-10/CXCR3 (27, 28). It has also been claimed that the CCR4/CCR8-CCL17/CCL22 pathway also significantly induces Vδ1 T cell migration. Meanwhile, high levels of CCL17 and CCL22 were detected in a variety of tumors, such as lung cancer, gastric cancer, B-cell non-Hodgkin’s lymphoma, Hodgkin’s lymphoma, and peripheral T-cell lymphoma. In lymphomas, CCL17 was specifically expressed in classical Hodgkin’s lymphoma, whereas CCL22 was expressed in nodular lymphocyte-predominant Hodgkin’s lymphoma and B-cell non-Hodgkin’s lymphoma (29).
Both in vivo and in vitro studies have revealed the multifaceted roles of various γδ T cell subtypes in modulating tumor cell proliferation, underscoring their intricate contribution to the dynamics of cancer progression. Flow cytometry and transcriptome analyses revealed that tumor-infiltrating lymphocytes contained an average of 4% γδ T cells, most of which expressed Vδ1. Among γδ T cells in the TME, the Vδ1 T cell subset highly expresses CXCR1 and weakly expresses CCR5, whereas Vγ9Vδ2 T cells show only strong expression of CCR5 (30). Moreover, Vγ9Vδ2 T cells concurrently expressed CCR3 and CXCR3, enabling them to initiate anti-tumoral responses in peripheral tissues, especially during the metastatic processes (18).
Vγ9Vδ2 T lymphocytes have been identified to demonstrate cytotoxic properties against breast cancer cells, enhancing apoptotic pathways and attenuating angiogenic signaling processes (31). Accumulated γδ1 T cells in the breast TME are termed γδ1 Tregs (32, 33), and these breast tumor-derived γδ Tregs suppress innate and adaptive immunity by inducing immune senescence and preventing dendritic cell maturation and activity (24, 34).
In the study of γδ T cells in the TME of colorectal cancer (CRC), the results showed that γδ T cells were mainly detected in paracancerous tissues but rarely in intra-tumoral tissues, and there was no significant increase in the number of T cell subpopulations of Vδ1 and Vδ2 in the CRC-infiltrating γδ T cells, but the main subpopulation was Vδ1 T cells (35, 36). The shifted balance between these subpopulations might hold implications for the progression of colon cancer (37).
Transcriptomic analysis of the peripheral blood of leukemia patients showed the presence of many tumor-infiltrating Vγ9Vδ2 cells, which positively correlated with the survival of these patients (18, 38). But then a new finding emerged that patients with chronic lymphocytic leukemia (CLL) had an increased percentage of Vδ1 cells, which replaced Vγ9Vδ2 cells as the predominant γδ T-cell subtype in the peripheral blood (39). And the study noted that a higher percentage of Vγ9Vδ2 cells was associated with a poor prognosis in patients with untreated CLL, as these lymphocytes exhibited signs of functional failure with reduced NKG2D expression (40, 41).
Infusion of large numbers of γδ T cells (Vδ1 and Vδ2 T cells) into high-risk leukemia patients by allogeneic hematopoietic stem cell transplantation (HSCT) contributes to the rapid control of infections and leukemia relapse. In HSCT recipients, Vδ2 and Vδ1 T cells were found to be cytotoxic to primary acute leukemia cells, whereas newly generated Vδ1 and Vδ3 cells in the TME underwent an adaptive response driven by cytomegalovirus (CMV) reactivation (42).
Despite accounting for a relatively small proportion of total T cells, γδ T cells have a complex and crucial role in the onset and progression of cancer. The function of γδ T cells in the TME can be altered by several circumstances to become either support tumor growth or combat it. Subsets of γδ T cells indirectly achieve anti-tumor immunity by producing specific factors to promote Th1, Th2, or Th17 differentiation (43–45) or cross-transmitting signals with B cells (46, 47), natural killer (NK) cells (45), and dendritic cells (48) in TME (49). There are also specific subpopulations of γδ T cells secrete a quantity of IL-17, which can directly act on epithelial cells to promote the progression of cancer, and γδ T can affect αβ T cells through immune checkpoints, supporting the creation of an immunosuppressive microenvironment that promotes tumorigenesis (2, 50). This dual role may be attributed to the inherent plasticity of γδ T cells, which includes the recruitment or residence of specific γδ T cell subsets at the tumor site and the ability to differentiate into different functional cell subsets based on the TME (51, 52).
In the realm of oncology, γδ T cells serve as a robustly positive prognostic indicator in most malignancies (47, 53, 54). Pan-cancer analysis based on the TCGA database in 2015 showed that γδ T cells were the best predictor of the prognosis within a range of solid tumors (50). γδ T cells are crucial for cancer immune surveillance and indeed studies have found that the incidence of cancers in mice lacking γδ T cells increases (55). Notably, γδ T cells accumulate in tumor-associated lymphoid tissues (38, 56) and can penetrate solid tumor tissues (57, 58). They can naturally infiltrate into the tissues of the whole body, including the lung, liver, and intestinal tract, which can be difficult malignancies to penetrate therapeutically.
The γδ TCR of Vγ9Vδ2 T cells is highly sensitive to tumor perception. During the course of tumorigenesis, the intracellular accumulation of phosphoantigens (pAgs) such as isoprenyl diphosphate (IPP) and dimethylallyl diphosphate (DMAPP) can weakly activate these cells. Meanwhile, the exogenous pAg (E)-4-hydroxy-3-methyl-but-2-enyl pyrophosphate (HMB-PP) can be co-locked intracellularly by transmembrane chymotrypsin 3A1 (butyrophilin 3A1, BTN3A1) and BTN2A1, and extracellularly detached and bound to the γδ TCR, resulting in efficient activation of γδ T cells (59, 60).
γδ T cells have characteristics of both the innate and adaptive immune systems and can act directly or indirectly on tumor cells (Figure 1; Table 1). To directly attack cells, γδ T cells rapidly migrate into the local tumor microenvironment by recognizing NK cell receptors on the cell surface. γδ 1T cells and γδ 2 T cells are both capable of ex vivo lysing of tumor cells and express chemokine receptors that enhance tumor homing (4). Activated γδ T cells can release granzyme and perforin to kill tumor cells directly (78). In addition, different γδ T cell subsets attach to tumor cells through the death receptors TNF-related apoptosis-inducing ligand receptor (TRAILR), CD95 (also known as FAS), and TRAIL and lyse cancer cells (65, 79, 80). The cell surface receptors NKG2D (81) and CD16 (48) also mediate the direct killing of γδ T cells based on antibody-dependent cytotoxicity and effector responses (48, 82). Complementing their cytotoxic capabilities, γδ T cells can also secrete cytokines IFN-γ and TNF-α, jointly suppressing tumor-associated angiogenesis (83).In some hematologic tumors, γδ T cells have been found to be capable of immunosurveillance by NK-like mechanisms (81, 84). Remarkably, around 80% of quiescent circulating γδ T cells express NK receptors. Most of these cytotoxic Vγ9Vδ2 T cell clones express HLA class I inhibitory NK cell receptors, such as CD94/NKG2A, KIR2DL1, KIR2DL2, KIR3DL1, or KIR3DL2 (85). Intriguingly, the majority of the clones express several different receptors, which help them to recognize different types of tumors (86). In breast cancer, Vδ1T cells residing at the tumor site recognize the tumor through innate stimuli including NKG2D (87). Within the TMEs, γδ T cells exert intermediate anti-tumor effects by interacting with B cells, dendritic cells, αβ T cells, and NK cells. γδ T cells can be used as antigen-presenting cell to activate αβ T cells (68). They can increase the amount of IFN-γ secreted by αβ T to regulate the TME by inducing recruitment of CTL, NK cells, and Th1, inducing M1-type polarization of macrophages (12), activating dendritic cells to induce their maturation (88), upregulating the expression of MHC class I in tumor cells to improve anti-tumor immune response (89) and preventing pro-tumor T helper cells from functioning (Treg, Th17 and/or Th2). Additionally, epithelial Vγ5 T cells induce B cell transformation and secretion of large amounts of Ig E, CCR5-expressing Vγ9Vδ2 T cell subsets promote antibody production and class switching (90), leading to the development of an immediate adaptive immune response in skin malignancies brought on by chemicals (91).
Figure 1 The anti-tumor function of γδ T cells. γδ T cells elicit antitumor immune responses through multiple pathways (1) Direct killing effect; (2) Secretion of IFN-γ and TNF-α; (3) Induced B cell transformation to secrete large amounts of Ig E and produce adaptive immune responses; (4) Eliciting CD8+ T cell responses. TNF, tumor necrosis factor; TRAIL, TNF-related apoptosis-inducing ligand; ADCC, antibody-dependent cell-mediated cytotoxicity; CTL, cytotoxic T lymphocyte; NK, natural killer cell; DC, dendritic cells.
Specific γδ T cells within the tumor microenvironment are known to secrete IL-17 (92), which promotes the emergence of autoimmune and inflammatory disorders (93, 94). At the same time, IL-17-producing γδ T cells promote the growth of tumors in a variety of ways. Recent studies have elucidated five key characteristics that underscore the tumor-promoting roles of these γδ T cells (Figure 2; Table 1). Firstly, γδ T cells have been shown to have a pro-angiogenic effect (95). Vascular endothelial growth factor (VEGF) and angiopoietin-2 (ANG-2) are angiogenic factors that γδ T cells can produce to promote angiogenesis (92, 96). Moreover, Margarida Rei et al. discovered that small peritoneal macrophages (SPM) were activated by IL-17-secreting Vγ6 γδ T cells, which accelerated the progression of ovarian cancer. Migration inhibitory factor (MIF) and IL-6 are two of the many tumor-promoting mediators that SPM can generate. They may promote the development of a variety of pro-inflammatory and pro-angiogenic molecules, while also protecting tumor cells from death (96). Secondly, these cells can prevent immune cells from performing their anti-tumor immunological functions. Specifically, IL-17 production from γδ T cells can directly suppress the anti-tumor activities of CTL and Th1 cells. Additionally, a significant proportion of γδ1 Treg cells can be found in the human breast tumor microenvironment, and they exert potent inhibitory effects on the proliferation of CD4+, CD8+, and Vγ9Vδ2 T cells by inducing senescence in responding immune cells and impairing the maturation and function of DCs (24, 27, 32). Elevated BMP2 in Acute Myeloid Leukemia (AML) patients induces the production of CD25+CD127lowVδ2+ T cells (named Reg-Vδ2). Reg-Vδ2 cells produce a number of regulatory cytokines rather than inflammatory cytokines, and the anti-AML activity of effector Vδ2 cells is significantly inhibited by Reg-Vδ2 cells (97). Furthermore, Vγ1 γδ T cells secrete IL-4 and decrease the NKG2D, perforin, and interferon expression levels in Vγ4 γδ T cells (76).
Figure 2 Pro-tumor functions of γδ T cells. (1) Secretion of IL-17 induces tumor cells to express pro-angiogenic factors, as well as mobilizing SPM to promote inflammatory response and angiogenesis. (2) Inhibition of anti-tumor immune response; (3) Construction of an immunosuppressive microenvironment; (4) Secretion of IL-17 to upregulate AM and endothelial cell permeability, as well as production of IL-22 and AREG to directly induce tumor cell proliferation. VEGFA, vascular endothelial growth factor A; ANG2, angiopoietin-2; SPM, small peritoneal macrophages; MDSC, myeloid-derived suppressor cells; TAN, tumor-associated neutrophils; G-CSF, granulocyte colony-stimulating factor; NE, neutrophil elastase; MMPs, matrix metalloproteinases; AM, adhesion molecule.
Thirdly, γδ T cells can directly construct a tumor immunosuppressive microenvironment. In human colorectal cancer, γδ T cells are polarized by microorganisms present due to disruption of the tumor epithelial barrier and inflammatory dendritic cells (Inf-DCs) in the TME to produce cytokines such as TNF-α, GM-CSF, IL-17 and IL-8. These cytokines recruit myeloid derived suppressor cells (MDSC) into the TME, regulate the development of tumor cells as well as induce Treg differentiation (73). In addition, these cells encourage G-CSF-mediated tumor-associated neutrophils (TAN) proliferation and accumulation in the TME. These TAN, in turn, release a variety of cancer-promoting factors, such as growth factors, neutrophil elastase (NE) and metalloproteinases (MMPs), and produce reactive oxygen species (ROS). These actions promote development of an immunosuppressive tumor microenvironment, inducing the depletion of CD8+ T cells and supporting tumor metastasis, tumor growth and invasion (31). Zhang et al. demonstrated that the “γδT cell-IL17A-Neutrophil” axis in the breast cancer tumor microenvironment promotes immunosuppression as well as enhancing the breast cancer’s tolerance to high-dose anti-VEGFR2 therapy (98).
In addition to this, there are two important protumor mechanisms, IL-17 secreted by γδ T cells modulates adhesion molecules and upregulates endothelial cell permeability to promote tumor metastasis (99). γδ T cells also produce IL-22 and amphiregulin (AREG), which directly induced tumor cell proliferation (100).
γδ T cells can directly identify and kill tumor cells therefore, adoptive and in vivo-induced γδ T cell expansion therapies are promising avenues to explore for anti-cancer immunotherapy purposes (101, 102). γδ T cells may be more favourable for use in adoptive cell immunotherapy compared to αβ T cells as they react more quickly to targets to produce effector factors, and they are found in a range of organs (103). In the hypoxic tumor microenvironment, γδ T cells, particularly the Vδ 1 subset, exhibit greater tissue tendency and greater invasiveness compared to αβ T cells (104). Moreover, graft versus host disease (GvHD) and allogeneic response risks can be decreased by using γδ T cells’ MHC-independent identification of target cells (105). Currently, various strategies are being used to activate and target γδ T cells, including drugs, antibodies, and genetic engineering. These strategies aim to enhance the anti-tumor response of γδ T cells and use them to combat hematological or solid tumors, such as B-cell malignancies (106).
CAR-T cell therapy is a type of immunotherapy that uses the patient’s own immune cells to fight cancer. In this approach,T cells are collected from the patient’s blood and genetically modified in the laboratory to express chimeric antigen receptors (CARs) on their surface. These CARs are designed to recognize specific proteins, called antigens, on the surface of cancer cells. Once the T cells have been modified, they are grown in large numbers and infused back into the patient’s body. The CAR-T cells can then seek out and destroy cancer cells that express the target antigen (107).
In the context of CAR-γδ T cells, diverse extracellular and intracellular domains can be fashioned based on the target antigen, the required co-stimulatory signal, and the signaling partner (108). Some examples of CAR designs for γδ T cells are: CD19-CAR, GD2-CAR (109), CD20-CAR (110), NKG2D-CAR (111), CCR (chimeric co-stimulatory receptor) (112), and NSCAR (non-signaling CAR) (113). To generate CAR γδ T cells, different methods of delivering genes can be used, for example, retrovirus (114), lentivirus (115), transposon (116), or mRNA electroporation (117). Traditional CAR-αβ T cell therapy has produced good clinical data in leukemia and other hematological malignancies, but it has not achieved the same success in solid cancer. In this regard, CAR-γδ T cells might offer a more promising avenue, as they have innate cytotoxicity capabilities, can recognize multiple antigens (118) and acquire the phenotypic and functional properties of antigen-presenting cells (APCs) (59, 119). In preclinical studies, CAR γδ T cells have exhibited potential against a diverse range of hematological and solid tumors, including B-cell lymphoma (110), glioblastoma (120), melanoma (121), colorectal cancer, and ovarian cancer (111). Nevertheless, several challenges persist in the development and application of CAR γδ T cell therapy (122), such as reduced tumor-toxicity, homing, in vivo persistence, heterogeneity, inter-donor variability, tumor microenvironment adaptation, etc.
Adoptive transfer of γδ T cells is a form of cancer treatment that involves the infusion of a patient’s own γδ T cells that have been expanded and activated outside the body. Nevertheless, previous clinical trials utilizing autologous γδ T cells sourced from cancer patients have only demonstrated limited clinical efficacy (123). Hence, current research is increasingly focusing on adoptive transfer therapies with allogeneic Vγ9Vδ2 T cells (124), which have been shown to enhance immune function, including CD4+ T cell, CD8+ T cell, and NK cell counts in cancer patients, even leading to total remission of recurrent hepatocellular carcinoma in one notable case (125). Another study investigated the use of adoptive cell therapy with IL-15-induced γδT cells in a patient-derived renal cell carcinoma xenograft model. The study concluded that IL-15-induced γδ T cells effectively suppressed tumor growth in vivo and prolonged the survival time of RCC-bearing patient−derived xenograft (PDX) mice (126).
In vivo expansion of γδ T-cells stands out as a unique approach to cancer immunotherapy. Unlike the ex vivo expansion seen in adoptive transfer, this method seeks to stimulate γδ T-cells directly within the patient’s body. This approach aims to enhance the anti-tumor activity of γδ T cells by using agents such as zoledronate, phosphoantigens, or specific cytokines such as IL-15 or IL-2 (124). In vivo expansion of γδ T cells has been shown to induce tumor regression and prolong survival in some animal models and clinical trials. A pilot study evaluated the adoptive transfer and in vivo expansion of haploidentical γδ T cells in patients with advanced hematological malignancies ineligible for allogeneic transplantation. Patients received peripheral blood mononuclear cells from half-matched family donors, followed by zoledronate and IL-2 to stimulate donor γδ T cells in vivo. This resulted in significant expansion of donor γδ T cells, NK cells, and double-negative αβ T cells. Impressively, three out of four patients achieved complete remission despite prior refractoriness (127).
At present, many preclinical studies have been conducted by researchers that suggest that γδ T cell therapy works well in multiple tumor models. While treating advanced ovarian cancer, γδ T cells function in the patient’s ascites and tumor by innate and adaptive immunological methods, respectively. This may make γδ T cells a viable treatment option for advanced ovarian cancer (128). For hematological malignancies, researchers have explored various ways to treat tumors using γδ T therapy. Ganesan et al. created a Vγ9/CD123 bispecific antibody that specifically triggers Vγ9+ γδ T cells and causes cytotoxicity to the tumor in vitro. This antibody efficiently induces Vγ9+ γδ T cells to engage with tumor cells. In patients with AML, these cells possess a variety of strategies for mounting an efficient immune response against overloaded tumor cells (129). γδ T cells can identify cancer antigens other than peptides, so extending the pool of possible targets for tumor cell eradication. Combining this feature, Xu et al. proposed a new TCR-T platform. They designed the AbTCR with non-MHC-restricted targets like CD19, which allows for the management of cytokine-related toxicity beyond existing anti-CD19 CAR-T therapies and provides comparable tumor suppression (115). Contrary to conventional CD19 CAR-αβ T, CAR-γδ T cells may still be able to target leukemia cells that lack the CD19 antigen and as such are useful for cases in which the antigen has been lost (130).
Early clinical results have established the promising vista of γδ T cells therapies in leukemia and other hematological tumors and solid tumors such as lung, gastric, and liver cancers. A landmark study led by Zhinan Yin’s team monitored patients with advanced liver and lung cancers over three years post-reception of allogeneic γδ T cell therapy. The team used allogeneic Vγ9Vδ2 γδ T cells from healthy human sources. By treating 132 patients with advanced lung and liver cancer tumors with a total of 414 cell transfusions, their study found that there was not a single case of serious side effects from the allogeneic γδ T cell transfusions and only some patients developed transient, mild clinical reactions (125, 131). Furthermore, the results highlighted a significant extension in survival among eight liver cancer patients and ten lung cancer patients who received ≥5 cell infusions (130). During this decade, dozens of clinical trials have been approved and several products have emerged as well (132). Numerous biotechnological enterprises are channeling significant investments into this burgeoning domain (Table 2). The γδ T treatment proposed by French biologics company ImCheck Therapeutics comprises a novel human-derived anti-BTN3A antibody, ICT01. ICT01 is a monoclonal antibody that specifically promotes Vγ9Vδ2 T cells targeting of BTN3A, which is extensively expressed in diverse solid and hematologic malignancies. ICT01 has been shown to have antitumor activity in vitro and in vivo tumor models against a range of cancers. The study published preliminary data from the first phase 1/2a clinical study on ICT01, revealing the value of the potential clinical application of ICT01 in the care of people with developing malignancies (133).
The Dutch biotech startup Lava Therapeutics have described a humanized bispecific γδ T cell binding antibody (γδ bsTCE). γδ bsTCE directly induces the effective killing of tumor cells through its unique targeting of Vγ9Vδ2 T cells and tumor-associated antigens (TAA). Two of its company’s projects, LAVA-051, and LAVA-1207, have entered clinical phase 2 trials. Multiple myeloma, chronic lymphocytic leukemia, and acute myeloid leukemia all include the antigen CD1d, which is recruited by LAVA-051 to γδ T cells (137). LAVA-051 has been given orphan drug status by the FDA for the treatment of (CLL) based on preliminary data from the Phase 1/2a clinical study and has a satisfactory safety and tolerability profile. Meanwhile, LAVA-1207 was designed to be a γδ bsTCE targeting prostate-specific membrane antigen (PSMA), with its clinical study focusing on metastatic castration-resistant prostate cancer.US-based biotech company IN8bio has also updated positive data from its ongoing phase 1 clinical trial of the allogeneic γδ T cell therapy INB-100 in high-risk AML patients who have previously undergone haploidentical hematopoietic stem cell transplantation (HSCT). From the data, all three patients treated with INB-100 received at least 12 months of follow-up which showed all three were in complete remission (CR). Remarkably, 100% of evaluable-dose patients remained on study and were in CR, with one patient having a progression-free disease course of more than 3 years (NCT03533816).Another ongoing project, INB-200, uses genetically modified autologous γδ T cell immunotherapy for the treatment of glioblastoma (GBM). Data according to the Phase 1 clinical trial of INB-200 for GBM showed that 100% of the six treated patients exceeded the median and expected progression-free survival (PFS). Two of the patients had exceeded the expected overall survival (OS), and the medication was generally well-tolerated and robust.
Innovative developments in CAR-γδ T-cell therapy is also advancing at a rapid pace. The UK company TC Biopharm is developing a new CAR-T therapy that takes advantage of the inherent specificity of γδT cells for phosphorylated antigens expressed only by cancerous and infected cells to develop the ImmuniCAR. OmnImmune, is being tested in a Phase 2b/3 clinical trial, following a 50% CR in Phase 1b/2a clinical data for this therapeutic candidate for AML. Concurrently, Adicet Bio announced clinical data for its allogeneic CAR-γδ T cell therapy ADI-001 for relapsed or refractory B-cell non-Hodgkin lymphoma (NHL). Data from the study showed ADI-001 demonstrated a 75% overall remission rate (ORR) and CR in eight patients who had received multiple prior therapies, including those who relapsed after using CAR-αβ T therapy. Gadeta, a Dutch company, has also innovated in CAR-γδ T-cell therapy, designed to use αβ T cells to carry the T-cell receptor for γδ T cells. The company’s TEGs technology enables the efficient expression of γδ TCR in αβ T cells, mediates tumor-specific proliferation of αβ T cells, and extensively infiltrates CD8+ effector T cells and CD4+ helper αβ T cells into tumors while not affecting normal organs.
Beyond the aforementioned therapeutic strategies, innovative γδ T cell-based treatments for diverse cancers are continually emerging. Induced pluripotent stem cells (iPSCs) termed T-iPSCs were formed by Watanabe et al. by rearranging the TCR γ chain (Vγ9) and TCR δ chain (Vδ2) gene regions (γδ T-iPSCs). Notably, these γδ T-iPSCs can differentiate into hematopoietic progenitor cells, which could theoretically provide a more potent collection of cells for new cancer research and a nearly infinite source of regenerating cells (138). Similarly, Zeng et al. successfully reprogrammed the γδ T-iPSC line of Vγ9Vδ2 T cells and these cells were modified into NK-like γδ T cells, termed “γδ natural killer T” (γδ NKT) cells (139).
It should be emphasized that γδ T-cell therapy still has some issues that need to be addressed. Firstly, the scarcity and low efficiency of in vitro expansion remains a serious limitation to entry of γδ T cells into the clinical pipeline. Expanding a considerable number of cell products through in vitro methods is crucial for the success of γδ T cell adoptive cell therapy. However, the effectiveness of this approach is limited by the inherent differences between donors (140). Recent research has shown that the level of physical activity in a donor can be used as a gauge for determining the in vitro expansion potential of their γδ T cells (124). The dominant subtype of γδ T cells in the peripheral blood of humans and other primates is Vγ9Vδ2 T cells, which account for only 1-10% of circulating lymphocytes in peripheral blood (141, 142). Currently, γδ T cells are largely obtained from peripheral blood mononuclear cells (PBMC) or umbilical cord blood isolated from healthy donors, followed by in vitro stimulation and expansion using synthetic PAgs or bisphosphonates (143–148). Gene modification and iPSCs techniques to produce specific γδ T cells in large quantities are major approaches of pharmaceutical companies to improve production and create a more clinically viable option (149). Efforts are underway to identify strategies that amplify the potency of γδ T cells in antitumor activities. For instance, IL-15 which can render a more active phenotype, greater proliferative capacity, and greater cytotoxicity in γδ T cells, is being investigated Combining IL-15 and γδ T cell immunotherapy may be able to enhance antitumor immunotherapy (150). In this regard, more research is warranted to examine the impact of diverse settings on the expansion of γδ T cells in vitro and to identify measures to promote the toxicity of Vγ9Vδ2 T cells, including candidates IL-2, IL-15, vitamin C, and TGF-β (126, 151, 152).
Another significant hurdle in advancing γδ T cell therapies pertains to the engineering of γδ T cells (153). For immune cell engineering, the most common method is to use lentivirus or retrovirus transfection. However, compared with ordinary αβ T cells, due to the natural antiviral properties of γδ T cells, viral transfection of γδ T cells is extremely difficult. It is also prone to the loss of CAR genes in cells during culture (115).
The broad spectrum of γδ T cells has to be taken into account when talking about the potential of γδT cell therapy. The heterogeneity of γδ T cells we described previously includes different subpopulations that mediate opposite immune responses to tumors. These subgroups are widely distributed throughout the body (12, 16). In addition to the Vδ2 T cell subpopulation, which is primarily present in peripheral blood and has been developed for antitumor therapy, the Vδ1 T cell subpopulation, which is present in tissues, has demonstrated strong cytotoxic potential against tumors when isolated from a variety of human solid tumors, which may partially address the limitations of current CAR-T therapies against solid tumors (37, 122). Combining contemporary high-throughput technologies to grasp the different subsets of γδ T cells at the single-cell level, such as Vδ1T cells (16), with manipulations such as gene editing techniques to enhance the immunological anti-tumor function of γδ T cells, may increase their potential application.It’s imperative to recognize that the tumor microenvironment is replete with various inhibitory immune cell populations. Immunosuppressive cytokines released by these cells can cause γδ T cells to become pro-tumor oriented and secrete IL-17, which drives cancer progression. In certain instances, the leukemic microenvironment adopts strategies to evade the anti-tumor response of these lymphocytes, leading to their exhaustion or polarization into a tumor-promoting phenotype (18). Confronted with these challenges, targeted screening of anti-tumor subsets, exclusion of pro-tumor subsets, determination of how to prevent the initial tumor killer cells from metamorphosis to promote tumor progression cells, or effective depletion of specific pro-tumor γδ T cell subsets, will be the focus of future research.Much work remains, particularly with regards to dissecting the multitude of subsets present in the body and determining how best to promote their anti-tumor activity. Current production bottlenecks further restrict their clinical application. Nevertheless, with ongoing research, it is anticipated that γδ T cells will cement their place as a cornerstone of cancer immunotherapy in the coming years.
WY: Writing – original draft. LD: Writing – review & editing. NL: Writing – review & editing. YHW: Writing – review & editing. YFW: Supervision, Writing – review & editing. PW: Funding acquisition, Supervision, Writing – review & editing.
The author(s) declare financial support was received for the research, authorship, and/or publication of this article. This project was supported by the National Natural Science Foundation of China (81872486, PW), the National Key Research and Development Program of China (2019YFC1316101, PW), and the Natural Science Foundation of Henan Province for Excellent Young Scholars (202300410360, PW).
The authors declare that the research was conducted in the absence of any commercial or financial relationships that could be construed as a potential conflict of interest.
All claims expressed in this article are solely those of the authors and do not necessarily represent those of their affiliated organizations, or those of the publisher, the editors and the reviewers. Any product that may be evaluated in this article, or claim that may be made by its manufacturer, is not guaranteed or endorsed by the publisher.
CAR-T cell, Chimeric antigen receptor-T cell; MHC, major histocompatibility complex; IPP, isopentenyl diphosphate; DMAPP, dimethylallyl diphosphate; HMBPP, (E)-4-hydroxy-3-methyl-but-2-enyl pyrophosphate; HIV, human immunodeficiency virus; NAFLD, nonalcoholic fatty liver disease; TCGA database, The Cancer Genome Atla database; HLA class I, human leukocyte antigen; NKRs, natural killer cell receptors; TNF-α, tumor necrosis factor α; TRAIL, Tumor necrosis factor‐related apoptosis‐inducing ligand; NKG2D, Natural killer group 2, member D; VEGF, Vascular endothelial growth factor; ANG-2, angiopoietin-2; SPM, small peritoneal macrophages; MIF, Migration inhibitory factor; CTL, cytotoxic T lymphocytes; GM-CSF, granulocyte-macrophage colony-stimulating factor; MDSC, myeloid derived suppressor cells; TAN, tumor-associated neutrophils; NE, neutrophil elastase; MMPs, metalloproteinases; ROS, reactive oxygen species; VEGFR2, vascular endothelial growth factor receptor 2; AREG, amphiregulin; GvHD, graft versus host disease; APCs, antigen-presenting cells; AML, Acute Myeloid Leukemia; BTN3A, Butyrophilin 3 A; TAA, tumor-associated antigens; PSMA, prostate-specific membrane antigen; HSCT, hematopoietic stem cell transplantation; CR, complete remission; GBM, glioblastoma; PFS, progression-free survival; OS, overall survival; NHL, non-Hodgkin lymphoma; ORR, overall remission rate; iPSCs, pluripotent stem cells; PBMC, peripheral blood mononuclear cells; PAgs, phosphate antigen; TCR, T cell receptor.
1. Scheper W, Sebestyen Z, Kuball J. Cancer immunotherapy using gammadeltaT cells: dealing with diversity. Front Immunol (2014) 5:601. doi: 10.3389/fimmu.2014.00601
2. Silva-Santos B, Serre K, Norell H. gammadelta T cells in cancer. Nat Rev Immunol (2015) 15(11):683–91. doi: 10.1038/nri3904
3. Mao C, Mou X, Zhou Y, Yuan G, Xu C, Liu H, et al. Tumor-activated TCRgammadelta(+) T cells from gastric cancer patients induce the antitumor immune response of TCRalphabeta(+) T cells via their antigen-presenting cell-like effects. J Immunol Res (2014) 2014:593562. doi: 10.1155/2014/593562
4. Bonneville M, O'Brien RL, Born WK. Gammadelta T cell effector functions: a blend of innate programming and acquired plasticity. Nat Rev Immunol (2010) 10(7):467–78. doi: 10.1038/nri2781
5. Nicol AJ, Tokuyama H, Mattarollo SR, Hagi T, Suzuki K, Yokokawa K, et al. Clinical evaluation of autologous gamma delta T cell-based immunotherapy for metastatic solid tumours. Br J Cancer (2011) 105(6):778–86. doi: 10.1038/bjc.2011.293
6. Conejo-Garcia JR, Innamarato P. gammadelta T cells share the spotlight in cancer. Nat Cancer (2022) 3(6):657–8. doi: 10.1038/s43018-022-00396-9
7. Gao Z, Bai Y, Lin A, Jiang A, Zhou C, Cheng Q, et al. Gamma delta T-cell-based immune checkpoint therapy: attractive candidate for antitumor treatment. Mol Cancer (2023) 22(1):31. doi: 10.1186/s12943-023-01722-0
8. Carding SR, Kyes S, Jenkinson EJ, Kingston R, Bottomly K, Owen JJ, et al. Developmentally regulated fetal thymic and extrathymic T-cell receptor gamma delta gene expression. Genes Dev (1990) 4(8):1304–15. doi: 10.1101/gad.4.8.1304
9. McVay LD, Carding SR. Extrathymic origin of human gamma delta T cells during fetal development. J Immunol (1996) 157(7):2873–82. doi: 10.4049/jimmunol.157.7.2873
10. Dolgin E. Unconventional gammadelta T cells 'the new black' in cancer therapy. Nat Biotechnol (2022) 40(6):805–8. doi: 10.1038/s41587-022-01363-6
11. Pang DJ, Neves JF, Sumaria N, Pennington DJ. Understanding the complexity of gammadelta T-cell subsets in mouse and human. Immunology (2012) 136(3):283–90. doi: 10.1111/j.1365-2567.2012.03582.x
12. Raverdeau M, Cunningham SP, Harmon C, Lynch L. gammadelta T cells in cancer: a small population of lymphocytes with big implications. Clin Transl Immunol (2019) 8(10):e01080. doi: 10.1002/cti2.1080
13. Karunathilaka A, Halstrom S, Price P, Holt M, Lutzky VP, Doolan DL, et al. CD161 expression defines new human gammadelta T cell subsets. Immun Ageing (2022) 19(1):11. doi: 10.1186/s12979-022-00269-w
14. Christopoulos P, Bukatz D, Kock S, Malkovsky M, Finke J, Fisch P. Improved analysis of TCRgammadelta variable region expression in humans. J Immunol Methods (2016) 434:66–72. doi: 10.1016/j.jim.2016.04.009
15. Perriman L, Tavakolinia N, Jalali S, Li S, Hickey PF, Amann-Zalcenstein D, et al. A three-stage developmental pathway for human Vgamma9Vdelta2 T cells within the postnatal thymus. Sci Immunol (2023) 8(85):eabo4365. doi: 10.1126/sciimmunolabo4365
16. Song Z, Henze L, Casar C, Schwinge D, Schramm C, Fuss J, et al. Human γδ T cell identification from single-cell RNA sequencing datasets by modular TCR expression. J Leukoc Biol (2023) qiad069. doi: 10.1093/jleuko/qiad069
17. Wu D, Wu P, Qiu F, Wei Q, Huang J. Human gammadeltaT-cell subsets and their involvement in tumor immunity. Cell Mol Immunol (2017) 14(3):245–53. doi: 10.1038/cmi.2016.55
18. Barros MS, de Araujo ND, Magalhaes-Gama F, Pereira Ribeiro TL, Alves Hanna FS, Tarrago AM, et al. gammadelta T cells for leukemia immunotherapy: new and expanding trends. Front Immunol (2021) 12:729085. doi: 10.3389/fimmu.2021.729085
19. Fichtner AS, Ravens S, Prinz I. Human gammadelta TCR repertoires in health and disease. Cells (2020) 9(4). doi: 10.3390/cells9040800
20. Wu Y, Biswas D, Usaite I, Angelova M, Boeing S, Karasaki T, et al. A local human Vdelta1 T cell population is associated with survival in nonsmall-cell lung cancer. Nat Cancer (2022) 3(6):696–709. doi: 10.1038/s43018-022-00376-z
21. Mensurado S, Blanco-Dominguez R, Silva-Santos B. The emerging roles of gammadelta T cells in cancer immunotherapy. Nat Rev Clin Oncol (2023) 20(3):178–91. doi: 10.1038/s41571-022-00722-1
22. Fisher JP, Yan M, Heuijerjans J, Carter L, Abolhassani A, Frosch J, et al. Neuroblastoma killing properties of Vdelta2 and Vdelta2-negative gammadeltaT cells following expansion by artificial antigen-presenting cells. Clin Cancer Res (2014) 20(22):5720–32. doi: 10.1158/1078-0432.CCR-13-3464
23. Lanca T, Costa MF, Goncalves-Sousa N, Rei M, Grosso AR, Penido C, et al. Protective role of the inflammatory CCR2/CCL2 chemokine pathway through recruitment of type 1 cytotoxic gammadelta T lymphocytes to tumor beds. J Immunol (2013) 190(12):6673–80. doi: 10.4049/jimmunol.1300434
24. Peng G, Wang HY, Peng W, Kiniwa Y, Seo KH, Wang RF. Tumor-infiltrating gammadelta T cells suppress T and dendritic cell function via mechanisms controlled by a unique toll-like receptor signaling pathway. Immunity (2007) 27(2):334–48. doi: 10.1016/j.immuni.2007.05.020
25. Nouri Y, Weinkove R, Perret R. T-cell intrinsic Toll-like receptor signaling: implications for cancer immunotherapy and CAR T-cells. J Immunother Cancer (2021) 9(11):e003065. doi: 10.1136/jitc-2021-003065
26. Zhao N, Dang H, Ma L, Martin SP, Forgues M, Ylaya K, et al. Intratumoral gammadelta T-cell infiltrates, chemokine (C-C motif) ligand 4/chemokine (C-C motif) ligand 5 protein expression and survival in patients with hepatocellular carcinoma. Hepatology (2021) 73(3):1045–60. doi: 10.1002/hep.31412
27. Ye J, Ma C, Wang F, Hsueh EC, Toth K, Huang Y, et al. Specific recruitment of gammadelta regulatory T cells in human breast cancer. Cancer Res (2013) 73(20):6137–48. doi: 10.1158/0008-5472.CAN-13-0348
28. Chen X, Shang W, Xu R, Wu M, Zhang X, Huang P, et al. Distribution and functions of gammadelta T cells infiltrated in the ovarian cancer microenvironment. J Transl Med (2019) 17(1):144. doi: 10.1186/s12967-019-1897-0
29. Zhou J, Kang N, Cui L, Ba D, He W. Anti-gammadelta TCR antibody-expanded gammadelta T cells: a better choice for the adoptive immunotherapy of lymphoid Malignancies. Cell Mol Immunol (2012) 9(1):34–44. doi: 10.1038/cmi.2011.16
30. Lee M, Park C, Woo J, Kim J, Kho I, Nam DH, et al. Preferential infiltration of unique vgamma9Jgamma2-vdelta2 T cells into glioblastoma multiforme. Front Immunol (2019) 10:555. doi: 10.3389/fimmu.2019.00555
31. Coffelt SB, Kersten K, Doornebal CW, Weiden J, Vrijland K, Hau CS, et al. IL-17-producing gammadelta T cells and neutrophils conspire to promote breast cancer metastasis. Nature (2015) 522(7556):345–8. doi: 10.1038/nature14282
32. Ye J, Ma C, Hsueh EC, Eickhoff CS, Zhang Y, Varvares MA, et al. Tumor-derived gammadelta regulatory T cells suppress innate and adaptive immunity through the induction of immunosenescence. J Immunol (2013) 190(5):2403–14. doi: 10.4049/jimmunol.1202369
33. Liu X, Hoft DF, Peng G. Senescent T cells within suppressive tumor microenvironments: emerging target for tumor immunotherapy. J Clin Invest (2020) 130(3):1073–83. doi: 10.1172/JCI133679
34. Weimer P, Wellbrock J, Sturmheit T, Oliveira-Ferrer L, Ding Y, Menzel S, et al. Tissue-specific expression of TIGIT, PD-1, TIM-3, and CD39 by gammadelta T cells in ovarian cancer. Cells (2022) 11(6). doi: 10.3390/cells11060964
35. Rong L, Li K, Li R, Liu HM, Sun R, Liu XY. Analysis of tumor-infiltrating gamma delta T cells in rectal cancer. World J Gastroenterol (2016) 22(13):3573–80. doi: 10.3748/wjg.v22.i13.3573
36. Yu L, Wang Z, Hu Y, Wang Y, Lu N, Zhang C. Tumor-infiltrating gamma delta T-cells reveal exhausted subsets with remarkable heterogeneity in colorectal cancer. Int J Cancer (2023) 153(9):1684–97. doi: 10.1002/ijc.34669
37. Corsale AM, Di Simone M, Lo Presti E, Dieli F, Meraviglia S. gammadelta T cells and their clinical application in colon cancer. Front Immunol (2023) 14:1098847. doi: 10.3389/fimmu.2023.1098847
38. Tosolini M, Pont F, Poupot M, Vergez F, Nicolau-Travers ML, Vermijlen D, et al. Assessment of tumor-infiltrating TCRVgamma9Vdelta2 gammadelta lymphocyte abundance by deconvolution of human cancers microarrays. Oncoimmunology (2017) 6(3):e1284723. doi: 10.1080/2162402x.2017.1284723
39. Simoes C, Silva I, Carvalho A, Silva S, Santos S, Marques G, et al. Quantification and phenotypic characterization of peripheral blood Vdelta1 + T cells in chronic lymphocytic leukemia and monoclonal B cell lymphocytosis. Cytometry B Clin Cytom (2019) 96(2):164–8. doi: 10.1002/cyto.b.21645
40. Coscia M, Vitale C, Peola S, Foglietta M, Rigoni M, Griggio V, et al. Dysfunctional Vgamma9Vdelta2 T cells are negative prognosticators and markers of dysregulated mevalonate pathway activity in chronic lymphocytic leukemia cells. Blood (2012) 120(16):3271–9. doi: 10.1182/blood-2012-03-417519
41. Allegra A, Casciaro M, Lo Presti E, Musolino C, Gangemi S. Harnessing unconventional T cells and innate lymphoid cells to prevent and treat hematological Malignancies: prospects for new immunotherapy. Biomolecules (2022) 12(6). doi: 10.3390/biom12060754
42. Pistoia V, Tumino N, Vacca P, Veneziani I, Moretta A, Locatelli F, et al. Human gammadelta T-cells: from surface receptors to the therapy of high-risk leukemias. Front Immunol (2018) 9:984. doi: 10.3389/fimmu.2018.00984
43. Caccamo N, La Mendola C, Orlando V, Meraviglia S, Todaro M, Stassi G, et al. Differentiation, phenotype, and function of interleukin-17-producing human Vgamma9Vdelta2 T cells. Blood (2011) 118(1):129–38. doi: 10.1182/blood-2011-01-331298
44. Meraviglia S, Caccamo N, Salerno A, Sireci G, Dieli F. Partial and ineffective activation of V gamma 9V delta 2 T cells by Mycobacterium tuberculosis-infected dendritic cells. J Immunol (2010) 185(3):1770–6. doi: 10.4049/jimmunol.1000966
45. Liu M, Hu Y, Yuan Y, Tian Z, Zhang C. gammadeltaT cells suppress liver fibrosis via strong cytolysis and enhanced NK cell-mediated cytotoxicity against hepatic stellate cells. Front Immunol (2019) 10:477. doi: 10.3389/fimmu.2019.00477
46. Daley D, Zambirinis CP, Seifert L, Akkad N, Mohan N, Werba G, et al. gammadelta T cells support pancreatic oncogenesis by restraining alphabeta T cell activation. Cell (2020) 183(4):1134–6. doi: 10.1016/j.cell.2020.10.041
47. Rampoldi F, Ullrich L, Prinz I. Revisiting the interaction of gammadelta T-cells and B-cells. Cells (2020) 9(3). doi: 10.3390/cells9030743
48. Silva-Santos B, Mensurado S, Coffelt SB. gammadelta T cells: pleiotropic immune effectors with therapeutic potential in cancer. Nat Rev Cancer (2019) 19(7):392–404. doi: 10.1038/s41568-019-0153-5
49. Chan KF, Duarte JDG, Ostrouska S, Behren A. gammadelta T cells in the tumor microenvironment-interactions with other immune cells. Front Immunol (2022) 13:894315. doi: 10.3389/fimmu.2022.894315
50. Gentles AJ, Newman AM, Liu CL, Bratman SV, Feng W, Kim D, et al. The prognostic landscape of genes and infiltrating immune cells across human cancers. Nat Med (2015) 21(8):938–45. doi: 10.1038/nm.3909
51. Lo Presti E, Toia F, Oieni S, Buccheri S, Turdo A, Mangiapane LR, et al. Squamous Cell Tumors Recruit gammadelta T Cells Producing either IL17 or IFNgamma Depending on the Tumor Stage. Cancer Immunol Res (2017) 5(5):397–407. doi: 10.1158/2326-6066.CIR-16-0348
52. Peters C, Meyer A, Kouakanou L, Feder J, Schricker T, Lettau M, et al. TGF-beta enhances the cytotoxic activity of Vdelta2 T cells. Oncoimmunology (2019) 8(1):e1522471. doi: 10.1080/2162402x.2018.1522471
53. Pawlik-Gwozdecka D, Zielinski M, Sakowska J, Adamkiewicz-Drozynska E, Trzonkowski P, Niedzwiecki M. CD8+ gammadelta T cells correlate with favorable prognostic factors in childhood acute lymphoblastic leukemia. Arch Med Sci (2021) 17(2):561–3. doi: 10.5114/aoms/132316
54. Le Floch AC, Rouviere MS, Salem N, Ben Amara A, Orlanducci F, Vey N, et al. Prognostic immune effector signature in adult acute lymphoblastic leukemia patients is dominated by gammadelta T cells. Cells (2023) 12(13). doi: 10.3390/cells12131693
55. He W, Hao J, Dong S, Gao Y, Tao J, Chi H, et al. Naturally activated V gamma 4 gamma delta T cells play a protective role in tumor immunity through expression of eomesodermin. J Immunol (2010) 185(1):126–33. doi: 10.4049/jimmunol.0903767
56. Viey E, Lucas C, Romagne F, Escudier B, Chouaib S, Caignard A. Chemokine receptors expression and migration potential of tumor-infiltrating and peripheral-expanded Vgamma9Vdelta2 T cells from renal cell carcinoma patients. J Immunother (2008) 31(3):313–23. doi: 10.1097/CJI.0b013e3181609988
57. Wu D, Wu P, Wu X, Ye J, Wang Z, Zhao S, et al. Ex vivo expanded human circulating Vdelta1 gammadeltaT cells exhibit favorable therapeutic potential for colon cancer. Oncoimmunology (2015) 4(3):e992749. doi: 10.4161/2162402x.2014.992749
58. Chabab G, Boissiere-Michot F, Mollevi C, Ramos J, Lopez-Crapez E, Colombo PE, et al. Diversity of tumor-infiltrating, gammadelta T-cell abundance in solid cancers. Cells (2020) 9(6). doi: 10.3390/cells9061537
59. Yuan L, Ma X, Yang Y, Qu Y, Li X, Zhu X, et al. Phosphoantigens glue butyrophilin 3A1 and 2A1 to activate Vgamma9Vdelta2 T cells. Nature (2023) 621(7980):840–8. doi: 10.1038/s41586-023-06525-3
60. Yang Y, Li L, Yuan L, Zhou X, Duan J, Xiao H, et al. A structural change in butyrophilin upon phosphoantigen binding underlies phosphoantigen-mediated vgamma9Vdelta2 T cell activation. Immunity (2019) 50(4):1043–53 e5. doi: 10.1016/j.immuni.2019.02.016
61. Knight A, Mackinnon S, Lowdell MW. Human Vdelta1 gamma-delta t cells exert potent specific cytotoxicity against primary multiple myeloma cells. Cytotherapy (2012) 14(9):1110–8.
62. Viey E, Fromont G, Escudier B, Morel Y, Da Rocha S, Chouaib S, et al. Phosphostim-activated gamma delta t cells kill autologous metastatic renal cell carcinoma. J Immunol (2005) 174(3):1338–47.
63. Alexander AA, Maniar A, Cummings JS, Hebbeler AM, Schulze DH, Gastman BR, et al. Isopentenyl pyrophosphate-activated CD56+ gammadelta t lymphocytes display potent antitumor activity toward human squamous cell carcinoma. Clin Cancer Res (2008) 14(13):4232–40.
64. Capietto AH, Martinet L, Fournie JJ. Stimulated gammadelta t cells increase the in vivo efficacy of trastuzumab in HER-2+ breast cancer. J Immunol (2011) 187(2):1031–8.
65. Tawfik D, Groth C, Gundlach JP, Peipp M, Kabelitz D, Becker T, et al. TRAIL-receptor 4 modulates gammadelta T cell-cytotoxicity toward cancer cells. Front Immunol (2019) 10:2044. doi: 10.3389/fimmu.2019.02044
66. Dokouhaki P, Schuh NW, Joe B, Allen CA, Der SD, Tsao MS, et al. NKG2D regulates production of soluble TRAIL by ex vivo expanded human gammadelta t cells. Eur J Immunol (2013) 43(12):3175–82.
67. Chen HC, Joalland N, Bridgeman JS, Alchami FS, Jarry U, Khan MWA, et al. Synergistic targeting of breast cancer stem-like cells by human gammadelta t cells and CD8(+) t cells. Immunol Cell Biol (2017) 95(7):620–9.
68. Holmen Olofsson G, Idorn M, Carnaz Simoes AM, Aehnlich P, Skadborg SK, Noessner E, et al. Vgamma9Vdelta2 T cells concurrently kill cancer cells and cross-present tumor antigens. Front Immunol (2021) 12:645131. doi: 10.3389/fimmu.2021.645131
69. McCarthy NE, Bashir Z, Vossenkamper A, Hedin CR, Giles EM, Bhattacharjee S, et al. Proinflammatory Vdelta2+ t cells populate the human intestinal mucosa and enhance IFN-gamma production by colonic alphabeta t cells. J Immunol (2013) 191(5):2752–63.
70. Maniar A, Zhang X, Lin W, Gastman BR, Pauza CD, Strome SE, et al. Human gammadelta t lymphocytes induce robust NK cell-mediated antitumor cytotoxicity through CD137 engagement. Blood (2010) 116(10):1726–33.
71. Patil RS, Shah SU, Shrikhande SV, Goel M, Dikshit RP, Chiplunkar SV. IL17 producing gammadeltaT cells induce angiogenesis and are associated with poor survival in gallbladder cancer patients. Int J Cancer. (2016) 139(4):869–81.
72. Hu Z, Luo D, Wang D, Ma L, Zhao Y, Li L. IL-17 activates the IL-6/STAT3 signal pathway in the proliferation of hepatitis b virus-related hepatocellular carcinoma. Cell Physiol Biochem (2017) 43(6):2379–90.
73. Wu P, Wu D, Ni C, Ye J, Chen W, Hu G, et al. gammadeltaT17 cells promote the accumulation and expansion of myeloid-derived suppressor cells in human colorectal cancer. Immunity (2014) 40(5):785–800. doi: 10.1016/j.immuni.2014.03.013
74. Ma S, Cheng Q, Cai Y, Gong H, Wu Y, Yu X, et al. IL-17A produced by gammadelta t cells promotes tumor growth in hepatocellular carcinoma. Cancer Res (2014) 74(7):1969–82.
75. Daley D, Zambirinis CP, Seifert L, Akkad N, Mohan N, Werba G, et al. Gammadelta t cells support pancreatic oncogenesis by restraining alphabeta t cell activation. Cell (2016) 166(6):1485–99 e15.
76. Hao J, Dong S, Xia S, He W, Jia H, Zhang S, et al. Regulatory role of Vgamma1 gammadelta T cells in tumor immunity through IL-4 production. J Immunol (2011) 187(10):4979–86. doi: 10.4049/jimmunol.1101389
77. Mao Y, Yin S, Zhang J, Hu Y, Huang B, Cui L, et al. A new effect of IL-4 on human gammadelta t cells: promoting regulatory Vdelta1 t cells via IL-10 production and inhibiting function of Vdelta2 t cells. Cell Mol Immunol (2016) 13(2):217–28.
78. Mohamadzadeh M, McGuire MJ, Smith DJ, Gaspari AA, Bergstresser PR, Takashima A. Functional roles for granzymes in murine epidermal gamma(delta) T-cell-mediated killing of tumor targets. J Invest Dermatol (1996) 107(5):738–42. doi: 10.1111/1523-1747.ep12365634
79. Chitadze G, Oberg HH, Wesch D, Kabelitz D. The ambiguous role of gammadelta T lymphocytes in antitumor immunity. Trends Immunol (2017) 38(9):668–78. doi: 10.1016/j.it.2017.06.004
80. Strid J, Roberts SJ, Filler RB, Lewis JM, Kwong BY, Schpero W, et al. Acute upregulation of an NKG2D ligand promotes rapid reorganization of a local immune compartment with pleiotropic effects on carcinogenesis. Nat Immunol (2008) 9(2):146–54. doi: 10.1038/ni1556
81. Simoes AE, Di Lorenzo B, Silva-Santos B. Molecular determinants of target cell recognition by human gammadelta T cells. Front Immunol (2018) 9:929. doi: 10.3389/fimmu.2018.00929
82. Hoeres T, Pretscher D, Holzmann E, Smetak M, Birkmann J, Triebel J, et al. Improving immunotherapy against B-cell Malignancies using gammadelta T-cell-specific stimulation and therapeutic monoclonal antibodies. J Immunother (2019) 42(9):331–44. doi: 10.1097/CJI.0000000000000289
83. Gao Y, Yang W, Pan M, Scully E, Girardi M, Augenlicht LH, et al. Gamma delta T cells provide an early source of interferon gamma in tumor immunity. J Exp Med (2003) 198(3):433–42. doi: 10.1084/jem.20030584
84. Poccia F, Gougeon ML, Bonneville M, Lopez-Botet M, Moretta A, Battistini L, et al. Innate T-cell immunity to nonpeptidic antigens. Immunol Today (1998) 19(6):253–6. doi: 10.1016/S0167-5699(98)01266-3
85. D'Ombrain MC, Hansen DS, Simpson KM, Schofield L. gammadelta-T cells expressing NK receptors predominate over NK cells and conventional T cells in the innate IFN-gamma response to Plasmodium falciparum malaria. Eur J Immunol (2007) 37(7):1864–73. doi: 10.1002/eji.200636889
86. Pennington DJ, Vermijlen D, Wise EL, Clarke SL, Tigelaar RE, Hayday AC. The integration of conventional and unconventional T cells that characterizes cell-mediated responses. Adv Immunol (2005) 87:27–59. doi: 10.1016/S0065-2776(05)87002-6
87. Almeida AR, Correia DV, Fernandes-Platzgummer A, da Silva CL, da Silva MG, Anjos DR, et al. Delta one T cells for immunotherapy of chronic lymphocytic leukemia: clinical-grade expansion/differentiation and preclinical proof of concept. Clin Cancer Res (2016) 22(23):5795–804. doi: 10.1158/1078-0432.CCR-16-0597
88. Ismaili J, Olislagers V, Poupot R, Fournie JJ, Goldman M. Human gamma delta T cells induce dendritic cell maturation. Clin Immunol (2002) 103(3 Pt 1):296–302. doi: 10.1006/clim.2002.5218
89. Riond J, Rodriguez S, Nicolau ML, al Saati T, Gairin JE. In vivo major histocompatibility complex class I (MHCI) expression on MHCIlow tumor cells is regulated by gammadelta T and NK cells during the early steps of tumor growth. Cancer Immun (2009) 9:10.
90. Bansal RR, Mackay CR, Moser B, Eberl M. IL-21 enhances the potential of human gammadelta T cells to provide B-cell help. Eur J Immunol (2012) 42(1):110–9. doi: 10.1002/eji.201142017
91. Crawford G, Hayes MD, Seoane RC, Ward S, Dalessandri T, Lai C, et al. Epithelial damage and tissue gammadelta T cells promote a unique tumor-protective IgE response. Nat Immunol (2018) 19(8):859–70. doi: 10.1038/s41590-018-0161-8
92. Wakita D, Sumida K, Iwakura Y, Nishikawa H, Ohkuri T, Chamoto K, et al. Tumor-infiltrating IL-17-producing gammadelta T cells support the progression of tumor by promoting angiogenesis. Eur J Immunol (2010) 40(7):1927–37. doi: 10.1002/eji.200940157
93. Petermann F, Rothhammer V, Claussen MC, Haas JD, Blanco LR, Heink S, et al. gammadelta T cells enhance autoimmunity by restraining regulatory T cell responses via an interleukin-23-dependent mechanism. Immunity (2010) 33(3):351–63. doi: 10.1016/j.immuni.2010.08.013
94. Sutton CE, Lalor SJ, Sweeney CM, Brereton CF, Lavelle EC, Mills KH. Interleukin-1 and IL-23 induce innate IL-17 production from gammadelta T cells, amplifying Th17 responses and autoimmunity. Immunity (2009) 31(2):331–41. doi: 10.1016/j.immuni.2009.08.001
95. Silva-Santos B. Promoting angiogenesis within the tumor microenvironment: the secret life of murine lymphoid IL-17-producing gammadelta T cells. Eur J Immunol (2010) 40(7):1873–6. doi: 10.1002/eji.201040707
96. Rei M, Goncalves-Sousa N, Lanca T, Thompson RG, Mensurado S, Balkwill FR, et al. Murine CD27(-) Vgamma6(+) gammadelta T cells producing IL-17A promote ovarian cancer growth via mobilization of protumor small peritoneal macrophages. Proc Natl Acad Sci USA (2014) 111(34):E3562–70. doi: 10.1073/pnas.1403424111
97. Liang S, Dong T, Yue K, Gao H, Wu N, Liu R, et al. Identification of the immunosuppressive effect of gammadelta T cells correlated to bone morphogenetic protein 2 in acute myeloid leukemia. Front Immunol (2022) 13:1009709. doi: 10.3389/fimmu.2022.1009709
98. Zhang Z, Yang C, Li L, Zhu Y, Su K, Zhai L, et al. "gammadeltaT cell-IL17A-neutrophil" Axis drives immunosuppression and confers breast cancer resistance to high-dose anti-VEGFR2 therapy. Front Immunol (2021) 12:699478. doi: 10.3389/fimmu.2021.699478
99. Kulig P, Burkhard S, Mikita-Geoffroy J, Croxford AL, Hovelmeyer N, Gyulveszi G, et al. IL17A-mediated endothelial breach promotes metastasis formation. Cancer Immunol Res (2016) 4(1):26–32. doi: 10.1158/2326-6066.CIR-15-0154
100. Jin C, Lagoudas GK, Zhao C, Bullman S, Bhutkar A, Hu B, et al. Commensal Microbiota Promote Lung Cancer Development via gammadelta T Cells. Cell (2019) 176(5):998–1013 e16. doi: 10.1016/j.carolcarrollell.2018.12.040
101. Buccheri S, Guggino G, Caccamo N, Li Donni P, Dieli F. Efficacy and safety of gammadeltaT cell-based tumor immunotherapy: a meta-analysis. J Biol Regul Homeost Agents (2014) 28(1):81–90.
102. Janssen A, Villacorta Hidalgo J, Beringer DX, van Dooremalen S, Fernando F, van Diest E, et al. gammadelta T-cell receptors derived from breast cancer-infiltrating T lymphocytes mediate antitumor reactivity. Cancer Immunol Res (2020) 8(4):530–43. doi: 10.1158/2326-6066.CIR-19-0513
103. Edwards SC, Sutton CE, Ladell K, Grant EJ, McLaren JE, Roche F, et al. A population of proinflammatory T cells coexpresses alphabeta and gammadelta T cell receptors in mice and humans. J Exp Med (2020) 217(5):e20190834. doi: 10.1084/jem.20190834
104. Morandi F, Yazdanifar M, Cocco C, Bertaina A, Airoldi I. Engineering the bridge between innate and adaptive immunity for cancer immunotherapy: focus on gammadelta T and NK cells. Cells (2020) 9(8). doi: 10.3390/cells9081757
105. Song Y, Zhu Y, Hu B, Liu Y, Lin D, Jin Z, et al. Donor gammadeltaT Cells Promote GVL Effect and Mitigate aGVHD in Allogeneic Hematopoietic Stem Cell Transplantation. Front Immunol (2020) 11:558143. doi: 10.3389/fimmu.2020.558143
106. Rimailho L, Faria C, Domagala M, Laurent C, Bezombes C, Poupot M. gammadelta T cells in immunotherapies for B-cell Malignancies. Front Immunol (2023) 14:1200003. doi: 10.3389/fimmu.2023.1200003
107. Sermer D, Brentjens R. CAR T-cell therapy: Full speed ahead. Hematol Oncol (2019) 37 Suppl 1:95–100. doi: 10.1002/hon.2591
108. Marofi F, Motavalli R, Safonov VA, Thangavelu L, Yumashev AV, Alexander M, et al. CAR T cells in solid tumors: challenges and opportunities. Stem Cell Res Ther (2021) 12(1):81. doi: 10.1186/s13287-020-02128-1
109. Rischer M, Pscherer S, Duwe S, Vormoor J, Jurgens H, Rossig C. Human gammadelta T cells as mediators of chimaeric-receptor redirected anti-tumour immunity. Br J Haematol (2004) 126(4):583–92. doi: 10.1111/j.1365-2141.2004.05077.x
110. Nishimoto KP, Barca T, Azameera A, Makkouk A, Romero JM, Bai L, et al. Allogeneic CD20-targeted gammadelta T cells exhibit innate and adaptive antitumor activities in preclinical B-cell lymphoma models. Clin Transl Immunol (2022) 11(2):e1373. doi: 10.1002/cti2.1373
111. Ng YY, Tay JCK, Li Z, Wang J, Zhu J, Wang S. T cells expressing NKG2D CAR with a DAP12 signaling domain stimulate lower cytokine production while effective in tumor eradication. Mol Ther (2021) 29(1):75–85. doi: 10.1016/j.ymthe.2020.08.016
112. Obajdin J, Davies DM, Maher J. Engineering of chimeric natural killer cell receptors to develop precision adoptive immunotherapies for cancer. Clin Exp Immunol (2020) 202(1):11–27. doi: 10.1111/cei.13478
113. Fleischer LC, Becker SA, Ryan RE, Fedanov A, Doering CB, Spencer HT. Non-signaling chimeric antigen receptors enhance antigen-directed killing by γδ T cells in contrast to αβ T cells. Mol Ther oncolytics (2020) 18:149–60. doi: 10.1016/j.omto.2020.06.003
114. Capsomidis A, Benthall G, Van Acker HH, Fisher J, Kramer AM, Abeln Z, et al. Chimeric antigen receptor-engineered human gamma delta T cells: enhanced cytotoxicity with retention of cross presentation. Mol Ther (2018) 26(2):354–65. doi: 10.1016/j.ymthe.2017.12.001
115. Rozenbaum M, Meir A, Aharony Y, Itzhaki O, Schachter J, Bank I, et al. Gamma-delta CAR-T cells show CAR-directed and independent activity against leukemia. Front Immunol (2020) 11:1347. doi: 10.3389/fimmu.2020.01347
116. Deniger DC, Switzer K, Mi T, Maiti S, Hurton L, Singh H, et al. Bispecific T-cells expressing polyclonal repertoire of endogenous gammadelta T-cell receptors and introduced CD19-specific chimeric antigen receptor. Mol Ther (2013) 21(3):638–47. doi: 10.1038/mt.2012.267
117. Ang WX, Ng YY, Xiao L, Chen C, Li Z, Chi Z, et al. Electroporation of NKG2D RNA CAR improves vgamma9Vdelta2 T cell responses against human solid tumor xenografts. Mol Ther Oncolytics (2020) 17:421–30. doi: 10.1016/j.omto.2020.04.013
118. Brandes M, Willimann K, Bioley G, Levy N, Eberl M, Luo M, et al. Cross-presenting human gammadelta T cells induce robust CD8+ alphabeta T cell responses. Proc Natl Acad Sci USA (2009) 106(7):2307–12. doi: 10.1073/pnas.0810059106
119. Foord E, Arruda LCM, Gaballa A, Klynning C, Uhlin M. Characterization of ascites- and tumor-infiltrating gammadelta T cells reveals distinct repertoires and a beneficial role in ovarian cancer. Sci Transl Med (2021) 13(577):eabb0192. doi: 10.1126/scitranslmed.abb0192
120. Li L, Zhu X, Qian Y, Yuan X, Ding Y, Hu D, et al. Chimeric antigen receptor T-cell therapy in glioblastoma: current and future. Front Immunol (2020) 11:594271. doi: 10.3389/fimmu.2020.594271
121. Harrer DC, Simon B, Fujii SI, Shimizu K, Uslu U, Schuler G, et al. RNA-transfection of gamma/delta T cells with a chimeric antigen receptor or an alpha/beta T-cell receptor: a safer alternative to genetically engineered alpha/beta T cells for the immunotherapy of melanoma. BMC Cancer (2017) 17(1):551. doi: 10.1186/s12885-017-3539-3
122. Ganapathy T, Radhakrishnan R, Sakshi S, Martin S. CAR gammadelta T cells for cancer immunotherapy. Is the field more yellow than green? Cancer Immunol Immunother (2023) 72(2):277–86. doi: 10.1007/s00262-022-03260-y
123. Hoeres T, Smetak M, Pretscher D, Wilhelm M. Improving the efficiency of vgamma9Vdelta2 T-cell immunotherapy in cancer. Front Immunol (2018) 9:800. doi: 10.3389/fimmu.2018.00800
124. Burnham RE, Zoine JT, Story JY, Garimalla SN, Gibson G, Rae A, et al. Characterization of Donor Variability for gammadelta T Cell ex vivo Expansion and Development of an Allogeneic gammadelta T Cell Immunotherapy. Front Med (Lausanne) (2020) 7:588453. doi: 10.3389/fmed.2020.588453
125. Alnaggar M, Xu Y, Li J, He J, Chen J, Li M, et al. Allogenic Vgamma9Vdelta2 T cell as new potential immunotherapy drug for solid tumor: a case study for cholangiocarcinoma. J Immunother Cancer (2019) 7(1):36. doi: 10.1186/s40425-019-0501-8
126. Zhang B, Li H, Liu W, Tian H, Li L, Gao C, et al. Adoptive cell therapy of patient-derived renal cell carcinoma xenograft model with IL-15-induced gammadeltaT cells. Med Oncol (2021) 38(3):30. doi: 10.1007/s12032-021-01474-1
127. Wilhelm M, Smetak M, Schaefer-Eckart K, Kimmel B, Birkmann J, Einsele H, et al. Successful adoptive transfer and in vivo expansion of haploidentical gammadelta T cells. J Transl Med (2014) 12:45. doi: 10.1186/1479-5876-12-45
128. Ganesan R, Chennupati V, Ramachandran B, Hansen MR, Singh S, Grewal IS. Selective recruitment of gammadelta T cells by a bispecific antibody for the treatment of acute myeloid leukemia. Leukemia (2021) 35(8):2274–84. doi: 10.1038/s41375-021-01122-7
129. Xu Y, Yang Z, Horan LH, Zhang P, Liu L, Zimdahl B, et al. A novel antibody-TCR (AbTCR) platform combines Fab-based antigen recognition with gamma/delta-TCR signaling to facilitate T-cell cytotoxicity with low cytokine release. Cell Discov (2018) 4:62. doi: 10.1038/s41421-018-0066-6
130. Xu Y, Xiang Z, Alnaggar M, Kouakanou L, Li J, He J, et al. Allogeneic Vgamma9Vdelta2 T-cell immunotherapy exhibits promising clinical safety and prolongs the survival of patients with late-stage lung or liver cancer. Cell Mol Immunol (2021) 18(2):427–39. doi: 10.1038/s41423-020-0515-7
131. De Gassart A, Le KS, Brune P, Agaugue S, Sims J, Goubard A, et al. Development of ICT01, a first-in-class, anti-BTN3A antibody for activating Vgamma9Vdelta2 T cell-mediated antitumor immune response. Sci Transl Med (2021) 13(616):eabj0835. doi: 10.1126/scitranslmedabj0835
132. Ma L, Feng Y, Zhou Z. A close look at current gammadelta T-cell immunotherapy. Front Immunol (2023) 14:1140623. doi: 10.3389/fimmu.2023.1140623
133. de Weerdt I, Lameris R, Ruben JM, de Boer R, Kloosterman J, King LA, et al. A bispecific single-domain antibody boosts autologous vgamma9Vdelta2-T cell responses toward CD1d in chronic lymphocytic leukemia. Clin Cancer Res (2021) 27(6):1744–55. doi: 10.1158/1078-0432.CCR-20-4576
134. Marcu-Malina V, Heijhuurs S, van Buuren M, Hartkamp L, Strand S, Sebestyen Z, et al. Redirecting alphabeta T cells against cancer cells by transfer of a broadly tumor-reactive gammadeltaT-cell receptor. Blood (2011) 118(1):50–9.
135. Lamb LS, Pereboeva L, Youngblood S, Gillespie GY, Nabors LB, Markert JM, et al. A combined treatment regimen of MGMT-modified gammadelta T cells and temozolomide chemotherapy is effective against primary high grade gliomas. Sci Rep. (2021) 11(1):21133.
136. Di Lorenzo B, Simoes AE, Caiado F, Tieppo P, Correia DV, Carvalho T, et al. Broad Cytotoxic Targeting of Acute Myeloid Leukemia by Polyclonal Delta One T Cells. Cancer Immunol Res (2019) 7(4):552–8.
137. Watanabe D, Koyanagi-Aoi M, Taniguchi-Ikeda M, Yoshida Y, Azuma T, Aoi T. The generation of human gammadeltaT cell-derived induced pluripotent stem cells from whole peripheral blood mononuclear cell culture. Stem Cells Transl Med (2018) 7(1):34–44. doi: 10.1002/sctm.17-0021
138. Zeng J, Tang SY, Wang S. Derivation of mimetic gammadelta T cells endowed with cancer recognition receptors from reprogrammed gammadelta T cell. PloS One (2019) 14(5):e0216815. doi: 10.1371/journal.Pone.0216815
139. Makkouk A, Yang XC, Barca T, Lucas A, Turkoz M, Wong JTS, et al. Off-the-shelf Vdelta1 gamma delta T cells engineered with glypican-3 (GPC-3)-specific chimeric antigen receptor (CAR) and soluble IL-15 display robust antitumor efficacy against hepatocellular carcinoma. J Immunother Cancer (2021) 9(12):e00344. doi: 10.1136/jitc-2021-003441
140. Khan MW, Curbishley SM, Chen HC, Thomas AD, Pircher H, Mavilio D, et al. Expanded human blood-derived gammadeltaT cells display potent antigen-presentation functions. Front Immunol (2014) 5:344. doi: 10.3389/fimmu.2014.00344
141. Deroost K, Langhorne J. Gamma/delta T cells and their role in protection against malaria. Front Immunol (2018) 9:2973. doi: 10.3389/fimmu.2018.02973
142. Baker FL, Bigley AB, Agha NH, Pedlar CR, O'Connor DP, Bond RA, et al. Systemic beta-Adrenergic Receptor Activation Augments the ex vivo Expansion and Anti-Tumor Activity of Vgamma9Vdelta2 T-Cells. Front Immunol (2019) 10:3082. doi: 10.3389/fimmu.2019.03082
143. Berglund S, Gaballa A, Sawaisorn P, Sundberg B, Uhlin M. Expansion of gammadelta T cells from cord blood: A therapeutical possibility. Stem Cells Int (2018) 2018:8529104. doi: 10.1155/2018/8529104
144. Wang RN, Wen Q, He WT, Yang JH, Zhou CY, Xiong WJ, et al. Optimized protocols for gammadelta T cell expansion and lentiviral transduction. Mol Med Rep (2019) 19(3):1471–80. doi: 10.3892/mmr.2019.9831
145. Zhang H, Chai W, Yang W, Han W, Mou W, Xi Y, et al. The increased IL-17-producing gammadeltaT cells promote tumor cell proliferation and migration in neuroblastoma. Clin Immunol (2020) 211:108343. doi: 10.1016/j.clim.2020.108343
146. Beucke N, Wesch D, Oberg HH, Peters C, Bochem J, Weide B, et al. Pitfalls in the characterization of circulating and tissue-resident human gammadelta T cells. J Leukoc Biol (2020) 107(6):1097–105. doi: 10.1002/JLB.5MA1219-296R
147. Schilbach K, Krickeberg N, Kaisser C, Mingram S, Kind J, Siegers GM, et al. Suppressive activity of Vdelta2(+) gammadelta T cells on alphabeta T cells is licensed by TCR signaling and correlates with signal strength. Cancer Immunol Immunother (2020) 69(4):593–610. doi: 10.1007/s00262-019-02469-8
148. Van Acker HH, Anguille S, Willemen Y, Van den Bergh JM, Berneman ZN, Lion E, et al. Interleukin-15 enhances the proliferation, stimulatory phenotype, and antitumor effector functions of human gamma delta T cells. J Hematol Oncol (2016) 9(1):101. doi: 10.1186/s13045-016-0329-3
149. Kawamoto H, Masuda K, Nagano S. Regeneration of antigen-specific T cells by using induced pluripotent stem cell (iPSC) technology. Int Immunol (2021) 33(12):827–33. doi: 10.1093/intimm/dxab091
150. Aehnlich P, Carnaz Simoes AM, Skadborg SK, Holmen Olofsson G, Thor Straten P. Expansion with IL-15 increases cytotoxicity of vgamma9Vdelta2 T cells and is associated with higher levels of cytotoxic molecules and T-bet. Front Immunol (2020) 11:1868. doi: 10.3389/fimmu.2020.01868
151. Kouakanou L, Xu Y, Peters C, He J, Wu Y, Yin Z, et al. Vitamin C promotes the proliferation and effector functions of human gammadelta T cells. Cell Mol Immunol (2020) 17(5):462–73. doi: 10.1038/s41423-019-0247-8
152. Beatson RE, Parente-Pereira AC, Halim L, Cozzetto D, Hull C, Whilding LM, et al. TGF-beta1 potentiates Vgamma9Vdelta2 T cell adoptive immunotherapy of cancer. Cell Rep Med (2021) 2(12):100473. doi: 10.1016/j.xCRM.2021.100473
Keywords: γδ T cells, T cell subsets, heterogeneity, CAR-γδ T, adoptive cell transfer therapy, cancer immunotherapy
Citation: Yan W, Dunmall LSC, Lemoine NR, Wang Y, Wang Y and Wang P (2023) The capability of heterogeneous γδ T cells in cancer treatment. Front. Immunol. 14:1285801. doi: 10.3389/fimmu.2023.1285801
Received: 30 August 2023; Accepted: 06 November 2023;
Published: 24 November 2023.
Edited by:
Yao Wang, Chinese PLA General Hospital, ChinaReviewed by:
Min Chen, Chongqing Medical University, ChinaCopyright © 2023 Yan, Dunmall, Lemoine, Wang, Wang and Wang. This is an open-access article distributed under the terms of the Creative Commons Attribution License (CC BY). The use, distribution or reproduction in other forums is permitted, provided the original author(s) and the copyright owner(s) are credited and that the original publication in this journal is cited, in accordance with accepted academic practice. No use, distribution or reproduction is permitted which does not comply with these terms.
*Correspondence: Pengju Wang, d2FuZ3BlbmdqdUB6enUuZWR1LmNu; Yafeng Wang, eWZ3YW5nQHp6dS5lZHUuY24=
Disclaimer: All claims expressed in this article are solely those of the authors and do not necessarily represent those of their affiliated organizations, or those of the publisher, the editors and the reviewers. Any product that may be evaluated in this article or claim that may be made by its manufacturer is not guaranteed or endorsed by the publisher.
Research integrity at Frontiers
Learn more about the work of our research integrity team to safeguard the quality of each article we publish.