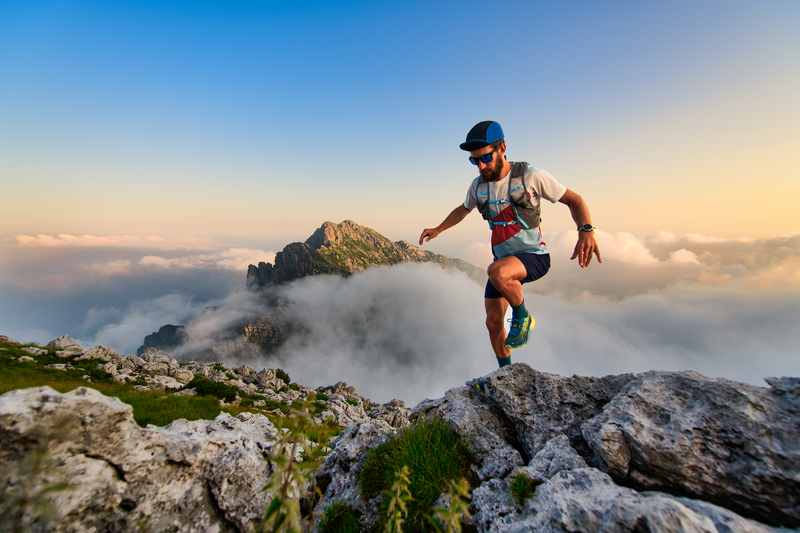
95% of researchers rate our articles as excellent or good
Learn more about the work of our research integrity team to safeguard the quality of each article we publish.
Find out more
REVIEW article
Front. Immunol. , 14 December 2023
Sec. Autoimmune and Autoinflammatory Disorders : Autoimmune Disorders
Volume 14 - 2023 | https://doi.org/10.3389/fimmu.2023.1281567
This article is part of the Research Topic Advances in Organ-Specific Autoimmune Response: from Basics to Clinics View all 7 articles
miRNAs are involved in various vital processes, including cell growth, development, apoptosis, cellular differentiation, and pathological cellular activities. Circulating miRNAs can be detected in various body fluids including serum, plasma, saliva, and urine. It is worth mentioning that miRNAs remain stable in the circulation in biological fluids and are released from membrane-bound vesicles called exosomes, which protect them from RNase activity. It has been shown that miRNAs regulate blood-brain barrier integrity by targeting both tight junction and adherens junction molecules and can also influence the expression of inflammatory cytokines. Some recent studies have examined the impact of certain commonly used drugs in Multiple Sclerosis on miRNA levels. In this review, we will focus on the recent findings on the role of miRNAs in multiple sclerosis, including their role in the cause of MS and molecular mechanisms of the disease, utilizing miRNAs as diagnostic and clinical biomarkers, using miRNAs as a therapeutic modality or target for Multiple Sclerosis and drug responses in patients, elucidating their importance as prognosticators of disease progression, and highlighting their potential as a future treatment for MS.
The American National MS Association of America describes multiple sclerosis as a disease characterized by inflammation that affects myelin sheaths on nerve cells in the spinal cord and brain. Approximately 70% of patients have severe and painful spasms, especially in the legs. Ninety percent of patients with MS complain of sexual problems, which can be caused by the disease or because of the side effects of drugs prescribed to treat this disease (1).
The signs of MS usually occur in two different patterns: A) Periods of sudden exacerbation: these symptoms last from several days to several weeks and recurrence or deterioration in the course of the disease is called an attack or sudden exacerbation, and then the disease goes into remission. This pattern comprises 85% of cases. B) A gradual worsening (progressive form) over time without recovery periods, which comprise 10-15% of cases. In some cases, a combination of these patterns may occur or people may experience periods of relapse and recovery, which later become the progressive form. Loss of BBB integrity leads to the entry of plasma proteins, toxins, and immune cells from the bloodstream into the brain. This influx triggers encephalitis, subsequently contributing to the onset or exacerbation of various neurological disorders and cerebral vascular diseases, including reperfusion/ischemia stroke, hypertension, amyotrophic lateral sclerosis, cerebral cavernous malformation, depression, and multiple sclerosis (2–5).
MS may affect short-term memory rather than long-term memory, attention and concentration, verbal ability, and information processing power. The characteristics of MS are Uhthoff’s phenomenon, which is the severity of the symptoms of the disease when the body temperature rises during physical activity, fever, or hot weather, and Lhermitte’s sign, which is an electric shock-like sensation that occurs on flexion of the neck (1).
Clinical symptoms include:
1. Sensory disorders: numbness of the limbs and local burning feeling in the limbs.
2. Movement disorders: swallowing and speech disorders, weakness and fatigue or muscle spasms, and paralysis of the limbs on one or two sides.
3. Visual disorders: blurred vision, visible field defects, diplopia, and nystagmus (clinical symptoms are primarily unilateral and occur in 60% of cases).
4. Cerebellar disorders: imbalance and tremors of eyes and limbs and dizziness.
5. Involvement of the urinary system: urination disorders and a degree of impotence.
6. Psychological symptoms, cognitive disorders, and depression.
Altered expression of some miRNAs is associated with pathological conditions; the amount of miRNA in the blood or brain of MS patients correlates with the stage of the disease and the progression of the disability. It may also provide timely diagnosis and effective treatment in the future.
There is evidence that apoptosis is an essential homeostatic process responsible for the termination of imbalanced controlled immune responses in MS patients. Several studies have shown that peripheral immune cells of MS patients are relatively resistant to apoptosis under various experimental conditions in comparison with healthy individuals (6, 7).
These and other findings suggest that the induction of peripheral immune cell apoptosis is a potential therapeutic target in MS (8). Gniadek et al. have investigated and found that the immunoregulatory activity of interferon-beta (IFN-β), an important drug for inducing remission in MS, is associated with increased apoptosis.
It has been reported that the most significant changes in miRNA and mRNA expressions in IFN-β-1b occur 1 month after starting treatment. It has been observed that the induction of IFN-β-responsive genes is associated with parallel down-regulation of miRNA expression. It has been shown that the regulation of miRNAs plays a role in the function of molecular mechanisms of IFN-β responses and the treatment of MS.
Studies have shown that most interactions between the expression of hsa-miR-16-5p and hsa-miR-532-5p are associated with IFN-response genes and high levels of the hsa-miR-16-5p expression are in the different blood cell types, especially monocytes. However, the complex interaction between TFs, miRNAs, and genes is still a big challenge. Functionally, some miRNAs (e.g., miR-29 family members) are related to apoptosis and IFN signaling feedback loops. In order to monitor the biological response to treatment and predict individual disease activity and progression, miRNA expression in blood cells may provide a biomarker. It may also be helpful to better comprehend the pathogenic mechanisms and optimize MS treatment (9).
Seidi et al. have shown that apoptosis is disturbed and dysregulated in T cells of MS patients; also, the expression level of FLIP and Bcl-2 proteins increases compared to the control group, making T cells resistant to apoptosis and remain self-reactive (10). The presence of miRNA profiles in naive T cells has been reported. Investigation of T cell sub-types revealed miRNAs via mechanisms induce their differentiation into pro-inflammatory phenotype in MS patients (11).
There is growing evidence that T and B lymphocytes play central core roles in the immunopathology of MS. During treatment with IFN-β, one of the first lines of disease-modifying drugs used to treat relapsing-remitting MS, researchers have seen a significant reduction in the circulating B-cell CD27 sub-population. Memory T and B cells are considered a subset of cells that are important to MS disease and have been targeted to be eliminated (12). IFN-β treatment reduces the expression of the LMP2A gene of EBV in RRMS patients. Since IFN-β targets the reservoirs of EBV infection, memory B cells indirectly reduce the level of EBV gene expression in MS patients. In addition, in terms of etiopathogenesis of MS, IFN-β induced a decrease in the memory T cell, leading to a reduction in the expression of the EBV2A latent membrane protein gene in treated patients (13).
Rizzo et al. found that IFN-β therapy in vivo specifically and significantly induced apoptosis in memory B cells, a substantial increase in apoptotic markers such as Caspase 3 and Annexin-V, through a mechanism requiring FAS-receptor/TACI signaling (Transmembrane activator and CAML interactor) (14–17).
It has also been shown that the glatiramer acetate induces a significant increase in apoptosis in CD4 + T cells in vitro. Maddalena Ruggieri et al. have expressed that Glatiramer Acetate induces pro-apoptotic mechanisms through Cyt-c, Bax, and Bcl-2 in the peripheral lymphocytes of MS patients (18). The critical side effects of the drugs include flu-like symptoms, headache, depression, leukopenia, liver problems, thyroid dysfunction, and injection site reactions (19). The expression level of miRNAs such as miR-21 during the response to traumatic brain injury (TBI) suggests that they may affect the pathophysiological process in brain injury. Ge et al. have reported that miR-21 induces angiogenesis and inhibits apoptosis. Studies showed the essential role of T cells and autoantibodies in the pathogenesis of MS, and these autoantibodies have been suggested as diagnostic biomarkers (20). Differential pathogenesis patterns are defined as a result of demyelination in MS lesions:
Type I: It caused the destruction of myelin by T cells, especially TH17, and the activation of macrophages.
Type II: Particular antibodies and complement activation cause demyelination.
Type III: It is related to distal oligodendropathy, and the degenerative changes occur distally, followed by apoptosis.
Type IV: After this, secondary demyelination occurs and leads to primary oligodendrocyte damage (21).
Small non-coding RNAs are 18-25 nucleotides in size and play an important role in various biological processes (22–24). It has been proposed that miRNAs regulate the expression of one-third of human genes (25). The regulation of several mRNAs by one miRNA has high-potential sufficiency because of a deficiency in complete pairing between the miRNA and the 3’UTR-end sequence of the target mRNA. Recent research has shown that coupling six to seven nucleotides of a miRNA to the 3’ region (UTR) of the target mRNA is necessary to detect the target mRNA. In MS, the profile of miRNAs of CNS lesions and the immune system is altered and can affect gene expression in many cell types involved in MS. The investigation of miRNA dysregulation has provided insight into MS pathophysiology and led to alternative methods for earlier diagnosis and treatment of the disease (26). miRNAs affect the development and regulation of the acquired immune system and T and B biological activities (27).
So far, several studies have been developed on the expression of miRNA in CNS patients’ tissue, animal models, inflammatory lesions of other autoimmune diseases, and paraneoplastic diseases. The analysis of miRNAs’ expression in the active, inactive, and regular white matter lesions CNS’ tissue provided a pattern of 50 miRNAs up-regulated and 30 miRNAs down-regulated in MS compared to healthy subjects (28). Laser capture micro-dissection of active lesions showed that most of the increased expression of miRNAs is expressed by B cells, T cells, astrocytes, and macrophages (28).
The significant role of miRNA in disorders is determined by the exchange of disease-related miRNA levels and individual profiles in their immune cell subsets. In vivo, using miRNA mimics and inhibitors has been shown to cause miR-21 overexpression related to an increase in the number of TH17 cells and disease severity in the experimental autoimmune disease encephalomyelitis (EAE). Also, the reduction of miR-21 expression and fewer Th17 cells are directly related to mild EAE disease (29). Irregular miRNAs’ expression is associated with CNS disorders, and in particular, some miRNAs are essential for neurogenesis regulation, neuron growth, oligodendrocyte differentiation, and myelin maintenance (30).
In summary, dysregulation of miRNAs in MS lesions contributes significantly to the mechanisms of MS inflammation. It is also clear that modifications in the pattern of miRNAs reflect both the demyelination process and an effort to remyelinate degenerated axons in the CNS. The profile of miRNA expression of blood cells and CNS lesions in MS patients showed its dysregulation, which is related to the pathological disease aspect. Then the disruption of miRNA expression is the background of biochemical modifications in MS. Whether this abnormal expression of miRNAs leads to the onset of this disease is not yet clear. The dysregulation of miRNAs may have a crucial role in various biological processes, including proliferation, development, differentiation, metabolism, apoptosis, angiogenesis, inflammation, and immunity (31, 32).
Multiple sclerosis (MS) is an autoimmune disease where T cells react to self-antigens found in the brain, particularly myelin antigen, which plays a significant role. Regev et al. showed that serum miRNAs could be used as potential biomarkers to diagnose and investigate disease status in MS (33). MRI is often used to demonstrate and investigate MS. It is essential to find blood biomarkers in MS that can aid in diagnosing the disease, determining disease stages, and establishing connections to disability accumulation. Besides cells, miRNAs have been found in various body fluids that are highly stable due to their resistance to ribonucleases. Circulating miRNAs remain stable even after prolonged storage, freeze-thaw cycles, and exposure to extreme pH conditions. Because of their stability and the development of sensitive detection methods for circulating miRNAs, they are excellent candidates for biomarkers. Researchers have observed changes in circulating miRNAs in several diseases (34, 35).
miRNAs are stable in human tissue and blood samples. Therefore, they can play a role as diagnostic or prognostic biomarkers (36). The reaction between miRNA and target's mRNAs is influenced by miRNA expression levels and affected by the presence of SNPs in miRNA genes and miRNA binding sites, which are generally present in UTR of mRNAs-′3 and create miRNA: mRNA pairs. miRNA binding to mRNA is essential to regulate the amount of mRNA and protein expression. Nucleotides 2-7 from the “seed” region are important for the relative complementarity of miRNA and the detection of the target mRNA to identify the mRNA target site. The presence of SNPs in these locations may disrupt or weaken a miRNA’s target or produce an entirely new sequence in the seed region of miRNA that does not bind to any specific mRNA. The diversity of SNPs can increase or decrease the binding strength of miRNAs effective in protein translation; for example, SNPs associated with miRNAs may cause cancer due to inhibiting the function of tumor suppressor miRNAs (37).
Several studies have been conducted on the profile of miRNAs in two groups of MS patients and control subjects in whole blood, peripheral blood mononuclear cells (PBMC), and brain lesions. All studies have shown that the expression profile of miRNAs is different in MS patients compared to healthy and control subjects. Studies have shown that the expression of miR-155 and miR-326 significantly increased in active brain lesions of MS patients compared to healthy individuals (38). Table 1 shows the variation of miRNAs’ pattern in Multiple Sclerosis patients.
Table 1 Difference of miRNAs pattern in Multiple Sclerosis patients(**UpregulatedmiRNAs,*Down regulated miRNAs).
miRNAs regulate both innate and adaptive immune responses. miRNAs are expressed with a specific pattern in the immune system cells and play an essential role in their expansion, mutation, and activity (19, 63). In several respects, miRNAs control the activity and development of immune system cells and the differentiation and activation of B and T lymphocytes (64, 65). They also play a role in the pathogenesis of MS. The study by Du et al. on the role of miRNAs in T cells and MS disease showed that miR-326 is related to the helper (CD4+ cells) Th17-producing interleukin-17 (IL-17), which plays a remarkable role in autoimmune diseases such as MS (66). They showed that miR-326 has increased in Th-17 cells of RRMS patients related to the extent of the disease, the differentiation of Th-17 cells, and the inhibition of Ets-1, which is a negative regulator of the differentiation of Th-17 cells (29).
Real-time PCR analysis showed increased expression of miR-145 in PBMC of MS patients (67). In addition, miR-326, miR-155, miR-146a, and miR-142-3p are elevated in the PBMC of MS patients. Another study showed that miR-146a, miR-21, miR-326, and miR-146b increased in PBMC of RRMS patients compared to control subjects (44).
An investigation of T cell subtypes has shown that miRNAs induce their differentiation to pro-inflammatory phenotype in MS patients by several mechanisms (11). It has been shown that miR-16-1 and miR-15a increased in CD4+ T cells in the peripheral blood of RRMS patients. miR-128, miR-27b, and miR-340 have also increased expression in blood T cells of MS patients (68, 69).
miRNA plays a crucial role in the regulation of stem cell self-renewal and differentiation by suppressing specific mRNA in stem cells, leading to their differentiation into daughter cells. This function has been observed in various somatic tissue stem cells, germline stem cells, and embryonic stem cells (70).
Another important miRNA in MS is miR.27a.3p, which is highly effective in distinguishing between different types of multiple sclerosis (RRMS, SPMS, and PPMS). It is associated with the T cell receptor signaling pathway and neurotrophin signaling pathway. miR-27a-3p targets several proteins involved in intracellular signaling networks that regulate the activity of mitogen-activated protein kinases (MAPKs) and nuclear factor-kB. It promotes the differentiation of Th17 and Th1 cells while increasing the accumulation of Tr1 and Treg cells. In MS patients, miR-27a-3p expression has been found to be increased in active brain lesions but decreased in the cerebrospinal fluid of patients with Alzheimer’s disease (71).
miR155 is essential for DC maturation and the ability of DCs to increase the activation of CD4+ T cell-specific antigens by inhibiting the expression of c-Fos, which can reduce the secretion of IL- 6, IFN-β, IL-12p70, TNF-α, and IL-12p40 through mature DCs. In mice with miR155 expression, the overexpression of Arg2 in DCs led to a severe depletion of arginine, which can prevent the reaction of T cells (72).
Overexpression of miR-132 in CD4+T cells in the EAE mouse model reduces the proliferation of IL-17 and IFN-γ secreting T cells. It has been observed that miR-26a decreased Th17 cell expression and increased Treg cell function by targeting IL-6. In EAE, inhibition of miR26a led to high levels of Th17 cytokines and exacerbation of EAE clinical symptoms. In contrast, Treg cell-specific transcription factors, Foxp3, were identified to be positively correlated with miR26a expression. Treatment of wild-type mice with anti-miR-21 oligonucleotide affected the disappearance of clinical EAE severity and the reduction of Th17 cells (73).
It has been reported that miR-20b, like a negative regulator of EAE, stops Th17 differentiation in vitro and showed that miR-20b is involved in the mechanisms of EAE through Th17. In addition, miR-30a, miR-326, and miR-23b interfere in the development of EAE by Th17 and through targeting Ets-1. Therefore, it seems that circulating miRNAs can be used as clinical biomarkers because they can reflect the severity and activity of the disease in MS patients. It has been reported that miR-155 is a new negative regulator of BBB function through modulating the regulator effects of the cell-to-brain endothelial cell (BEC) and cell-to-matrix, and it has led to impaired function of BBB in MS (73).
Overexpression of miR-125a-5p in brain endothelial cells reduces the expression of ICAM-1 and the migration of monocytes through brain endothelial cell barriers. Therefore, increasing miR-125a can cause normal function of the brain nerve fibers in neurological diseases induced by endothelial cells, especially in MS (74).
miR-214 increased in oligodendrocytes during differentiation and overexpression of miR-23a increases the differentiation of oligodendrocytes through reduction of lamin B. The increase in the level of miR-23a and miR-214 in MS lesions can reflect myelin regeneration (75). It has been reported that miR-124 is expressed in the M2 phenotype of macrophages and microglia because its overexpression results in the decrease in M1 markers such as TNF-α, IL-6, and iNOS and the increase in M2 phenotype-dependent proteins such as arginase-1, TGF-β, and FIZZI, which are necessary to stop EAE.
miR-181a is one of the first miRNAs that play an important role in the expansion and development of immune cells in thymus cells and has a low expression in the heart, bone marrow, and lymph nodes. The expression level of miR-181a is decreased in the expansion and differentiation of B cells from pro-B cells to the pre-B stage. For instance, miR-223 plays an essential role in the regulation and differentiation of granulocytic cells. Also, miR-150 is critical in differentiating B cells (76). Therefore, miRNAs play a vital role in differentiating different stages of immune cells.
Also, miR-155 plays an important role in the mammalian immune system, especially in regulating and differentiating T lymphocytes, germinal center reactions, and generating appropriate T cell-dependent responses. miR-155 can control mechanisms by regulating the production of cytokines. Many types of special Th cells, including Th9, Th17, Th1, Th2, Treg, and follicular helper T, differentiate in diverse pathways, but they can even have plasticity under certain conditions and become effector types. Recent studies have shown the role of miRNAs in the biogenesis of Treg and the prevention of autoimmune responses. Deficiency of miR-155 in Treg cells results in decreased expression of suppressor of cytokine signaling 1 (SOCS1) and activation of transcription factor (STAT5) in response to a limited amount of IL2. Forkhead box P3-(Foxp3-), dependent on miR-155 regulation, plays a role in Treg maintenance by targeting SOCS1 (77).
The production of B lymphocytes that express high-affinity receptors in the two stages of antigen-dependent selection in secondary lymphoid organs and selection in the bone marrow has been shown to be related to the dynamic setting out of miRNAs. In addition to antigenic selection, miRNAs play a role in expanding transcription factors and differentiation in the early stages of B cells. The continuous expression of miR-150, which is increased in the early stages of the stage of immature B cells and B cell differentiation, leads to the blocking of the expansion and development of B cells and the transition from pro-B to pre-B cells. miR-150 most likely reduces mRNAs that are involved in the activity and formation of pre- and pro-B cells (78).
In recent years, several studies have been conducted on the role of miRNAs in the development of MS, and these have shown that miRNAs are regulated and controlled by different pathways. Du et al. studied IL-17-producing Th17 cells and miR-326 expression in Th17 cells. Silencing miR-326 in vivo caused fewer Th17 cells and moderate EAE phenotype, while its overexpression caused more Th17 cells and aggravated EAE. This report shows the importance of the role of miRNA-326 in the differentiation of Th17 cells and the onset of MS (29).
Junker et al. discovered that several miRNAs, including miRNA-155, miRNA-34a, and miRNA-326, are present in active lesions of MS (28). They specifically identified astrocytes as a source of miR-155 expression and found dysregulated target sites for these miRNAs in the 3-UTR region of the CD47 transcript. Their model suggests that dysregulated miRNAs cause a decrease in CD47 levels, resulting in the loss of inhibitory control over brain cells and an increase in myelin sheath phagocytosis (28). Furthermore, Otaegui et al. discovered that miR-599 and miR-18b are associated with the regression state, while miR-96 is linked to remission (39).
Additionally, Zhang et al. found that certain miRNAs, including miR-22, miR-614, miR-1979, miR-648, miR-1826, miR-422a, and miR-572, exhibited different expression levels in the plasma samples of MS patients compared to the control group. These studies demonstrate that various biological samples contain miRNAs that have the potential to distinguish MS from other neurological diseases and differentiate between different types of MS (79).
Chapman et al. also demonstrated an increase in miR-17-5p in CD4+ cells of MS patients, which is associated with changes in the expression of its target genes such as phosphatase and PI3KR1. The relationship between miRNA expression and T-cell activation was supported by the elevated response of miR-17-5p in CD4+ cells in vitro. Other studies have provided new evidence regarding the role of miR-17-92 in autoimmunity (80).
Guraudi-Arellano et al. demonstrated that miRNAs such as miR-27b and miR-128 were elevated in miR-340 and T cells were present in the memory of CD4+ T cells in MS patients. These miRNAs played a role in the differentiation of pathogenic T cells. The altered expression of these miRNAs led to the differentiation of pathogenic Th1 cells in both human cells and mice suggesting their potential as therapeutic targets for regulating T-cell phenotypes in MS (81).
Additionally, Murugayan et al. found increased expression of miR-155 in CD4+ T cells from an EAE model. Mice lacking miR-155 showed reduced inflammation in the CNS and less severe disease symptoms. This study also revealed that miR-155 regulated Th1 cells producing IFN-γ, which contributes to inflammatory reactions in MS patients.
It is essential to develop novel, precise biomarkers that can clearly show the extent of disease improvement and treatment response in MS patients. MRI can identify plaques in the brain of MS patients, but these lesions may be related to other diseases although demyelination can be one of the systematic features of diseases such as lupus erythematosus, Behcet’s disease (BD), Sjogren’s syndrome (SS), Systemic Sclerosis tissue (SSc), or other very rare systemic autoimmune diseases. Some miRNAs showed a specific expression pattern in different diseases or disease stages. For example, Keller et al. have found that miR-145 is present in the blood of all MS patients.
In addition, Ridolfit et al. showed that miR-23a and miR-223 could play an essential role in the development of MS through the NF-kb pathway and FGF gene. They also found that rs1044165 SNP of miR-223 acts as a protective factor, and rs3745453 SNP of miR-23a is a risk factor for MS (80).
Recently, miRNAs have been considered as particular targets for treating autoimmune diseases. Murugaiyan et al. showed that inhibiting miR-21 by locked nucleic acid (LNA)-anti-miR decreases the expression of cytokines in TH17 cells, especially IL-17F, IL-17A, IL-21, and IL-22, and it can effectively prevent disease progression in the EAE model. They also reported that the expression of miR-21 increased in miR-21, and TH17 cell expression-deficient mice are resistant to EAE (82).
Guinea-Viniegra et al. reported that using antagonizing miR-21 improved psoriasis in psoriasis-like mouse and mouse xenotransplantation models. Further studies showed that miR-21 promotes TH17 responses through SMAD-7, a negative regulator of the TGF-β signaling pathway. Also, miR-21 increases the activity of SMAD-2/3, suppresses the expression of IL-2, expands the TGF-β signaling pathway, and finally increases TH17 differentiation (83).
miRNA-based therapies can include the use of specific miRNA mimics to reduce target gene expression or the use of antisense oligonucleotides to block specific proteins. In particular, the anti-miRNA therapeutic strategy is preferred to the antisense mRNA therapeutic strategy because a miRNA affects clusters of genes involved in a disease. A promising strategy is using LNAs (locked nucleic acids), a bicyclic RNA conformational analog with a high affinity to its complementary RNA molecule and remarkable stability in blood and tissue in vivo.
Recent in vivo studies on the potential application of LNA-modified oligonucleotides on the function of miRNAs have led to the expansion of their use for therapeutic purposes. The LNA-modified miR-122 inhibitor was used for treating hepatitis C infection in phase one clinical trials (84, 85). Recent studies have shown that miR-340, miR-128, and miR-27b increase CD4+ T cells of MS patients and cause a shift in the production of TH2 to TH1 cytokines. In vitro, using miRNA inhibitory oligonucleotides, including miR-340, miR-27b, and miR-128, has increased TH2 responses. Therefore, the results indicate that the inhibition of these miRNAs in vivo can be used as a therapeutic target for MS disease (86).
Changing the pattern of miRNAs can reflect both the demyelination process and the attempt to regenerate degenerated axon myelin in the CNS. It is controversial whether a disrupted expression of miRNAs leads to MS. The disruption of miRNAs may play an essential role in many biological progressions such as reproduction, differentiation, development, metabolism, angiogenesis, inflammation, immunity, and apoptosis (Figure 1).
Figure 1 Different expressions of miRNA in pathological processes of MS. Dysregulation of miRNAs in active lesions alters the BBB, compromising the brain’s immune privilege. This results in BBB dysfunction and the exposure of neuronal antigens to the peripheral immune system, leading to inflammation. Subsequently, this provokes the release of pro-inflammatory cytokines, further exacerbating the inflammatory response in the brain and contributing to CNS injury.
Fenoglio et al. have shown significantly increased expression levels of miR-150, miR-21, miR-155, and miR-146a in RRMS patients compared to healthy control subjects (63). Statistical investigations and analyses on real-time PCR showed that miRNA-142-3p, miRNA-21, miRNA-146b, miRNA-146a, and miRNA-155 are increased in spinal cords in the EAE mouse model (63). Also, the expression of miRNA-155 was significantly increased in the lymph nodes, spleen, and brain of EAE mice (63).
In particular, Junker et al. have shown that miR-155, miR-34a, and miR-326 have increased expression in active lesions of MS patients compared to healthy individuals. The increased expression of these miRNAs in active MS lesions leads to decreased CD47 expression and failed inhibitory control of macrophages, resulting in myelin phagocytosis (28, 87). Analysis of the researchers’ data showed that miR-142-3p, miR-21, miR-146a, miR-326, miR-155, and miR-146b increased in the brain white matter of MS patients with MS and EAE mice (88).
Investigators have conducted microarray analysis on more than 900 miRNA transcripts collected from plasma samples of MS patients. Six of 900 miRNAs (miR-614, miR-648, miR-572, miR-1826, miR-22, and miR-422a) significantly increased, and miR-1979 significantly decreased in MS patients. It has been shown that miR-223 plays an essential role in granulopoiesis by targeting mef-2c and modulating the NF-κb pathway associated with modulating immune inflammatory responses (46).
The results revealed that miR-145 has considerably increased expression in PBMC of MS patients, and expression of miR-7-1-3p, miR-20a-5p, miR-15b, miR-223, and miR-23a is decreased in the blood of MS patients. miR-142-3p, miR-21, miR-146a, miR-146b, miR-326, and miR-155 expression significantly increased in PBMC and white matter of brain lesions of MS patients compared to healthy controls (26, 89), which is suggested to be used as a diagnostic and differential marker for MS in patients.
The presence of the BBB creates a controlled microenvironment by regulating the exchange of ions and molecules between the bloodstream and brain tissue (90). Several investigations have uncovered the physiological functions of the BBB, which include protecting the brain. In addition to physically preventing potentially harmful substances from entering, the BBB also serves multiple roles in maintaining homeostasis, facilitating the transport of essential molecules, and regulating inflammation (91, 92). The BBB sustains brain homeostasis by controlling specific ion channels and transporters (93, 94).
As a highly dynamic and intricate structure, the BBB consists of pericytes, astrocytes, endothelial cells, and the basement membrane. It modulates the movement of substances between the central nervous system and the bloodstream (94, 95). It is known that the function of the BBB is tightly regulated by specific tight junction proteins. Notably, claudin-5, the most prominently expressed tight junction protein at the BBB, is closely associated with the pathology of ischemic stroke (96).
Previous studies have reported that many miRNAs are now thought of as MS biomarkers due to the pathology of MS being related to the abnormal expression of miRNAs in CNS lesions. To develop effective strategies for promoting remyelination, it is imperative to comprehend the role that miRNAs play in the regulation of oligodendrocyte development and myelination (2, 97).
A comprehensive understanding of the advantages and disadvantages of various strategies aimed at overcoming the challenges associated with efficient miRNA delivery is critical in the development of safe and efficacious therapeutic approaches for preserving BBB integrity in a variety of CNS diseases (2, 98).
The previous findings confirm that the development of novel therapeutic approaches, specifically targeting brain parenchyma, is imperative, as most existing therapies struggle to efficiently penetrate the blood-brain barrier (BBB). The preservation of BBB’s integrity is vital for maintaining brain homeostasis and normal neurological function (2, 98).
In the context of MS and related diseases, the endothelial barrier is compromised, contributing to the development and progression of these conditions (99).
Numerous investigations have primarily concentrated on the pivotal tight junction components of the BBB, specifically claudins, ZO, and occludin proteins. A reduction in the expression of ZO-1 and claudin-5 within brain endothelial cells has been associated with several neurological disorders (75, 99). Claudin-5, as the most prominently expressed tight junction protein in the BBB, exhibits a close association with ischemic stroke pathology (96).
Recent research has recognized the direct targeting of these junction molecules by microRNAs, leading to alterations in BBB integrity (74). These findings strongly indicate that the repair of tight junctions may serve as a therapeutic target for restoring BBB integrity and improving disease management. To date, there are no known molecules capable of directly enhancing the expression of tight junction components to restore BBB integrity (100).
Previous studies have reported that in vivo administration of locked nucleic acid-modified antisense oligonucleotides, targeting miR-501-3p, resulted in the restoration of ZO-1 gene expression and amelioration of BBB disorder in mice afflicted with vascular cognitive impairment, leading to a significant improvement in cognitive function. The BBB, a highly intricate and dynamic structure, rigorously regulates substance exchange through the complex interplay of tight junction proteins among endothelial cells (96, 101).
Utilizing in silico analysis and subsequent confirmatory laboratory experiments, several miRNAs have been identified as regulators of specific diseases, with some of them being related to tight junctions. A fundamental feature of the BBB is the presence of exceptionally robust tight junctions between brain endothelial cells, controlled by an array of junction molecules, covering adherens junction proteins and tight junction (96).
Remarkably, a notable reduction in miR-125a-5p expression was observed in the inflamed blood vessels of multiple sclerosis patients compared to non-inflamed blood vessels, suggesting a positive correlation between BBB integrity and miR-125a-5p during inflammation (74). Upon treatment of brain endothelial cells with the proinflammatory mediator tumor necrosis factor-alpha/interferon-gamma, a significant alteration was noted in 107 miRNAs, including the downregulation of miR-125a-5p (74, 102). Conversely, miR-155 was found to negatively impact BBB integrity during inflammation (102). miR-101 indirectly downregulates claudin-5, ultimately increasing BBB permeability (103). miR-373, miR-27a, and miR-210 are involved in modulating the integrity of the BBB by affecting proteins such as VE-cadherin, occludin, and ZO-1 in various brain disorders (99).
miR-96 indirectly suppresses ZO-1 mRNA expression, thereby promoting monocyte migration across the BBB (104). Also, miR-424-5p binds to the 3’ untranslated region of endophilin-1 mRNA, upregulating endophilin-1 mRNA levels (103, 105). The overexpression of endophilin-1 leads to the inhibition of the EGFR-Jun-N-terminal kinase and EGFR-ERK signaling pathways, resulting in the downregulation of occludin proteins and ZO-1 in vascular endothelial cells (103, 105).
Abundant miRNAs have been identified as direct or indirect regulators of specific barrier proteins. In a prior study, it was demonstrated that miR-27a targets VE-cadherin to disrupt vascular integrity (106). Consequently, a potential therapeutic approach to enhance VE-cadherin expression may thereby resolve abnormal vascular integrity (107). Therefore, miR-27a emerges as a potential common regulator of vascular integrity, particularly within the cerebral vascular system (106, 107).
The most critical tight junction (TJ) proteins linked to the BBB include claudin-5, occludin, and ZO-1 (108). miR-96 indirectly inhibits the expression of ZO-1 mRNA, leading to increased migration of monocytes across the BBB (104). MiR-424-5p binds to the 3’ untranslated region (UTR) of endophilin-1 mRNA and upregulates endophilin-1 mRNA (103, 105). Overexpression of endophilin-1 results in the inhibition of the EGFR-Jun-N-terminal kinase and EGFR-ERK signaling pathways, subsequently downregulating occludin proteins and ZO-1 in vascular endothelial cells (103, 105). Also, miR-101 indirectly downregulates claudin-5, resulting in increased BBB permeability (103).
The loss of myelin is a pathological hallmark in MS, and various factors influence the processes of developmental myelination and remyelination within the central nervous system (CNS). Many of these factors impact the exceedingly regulated transition from an immature, proliferating oligodendrocyte progenitor cell (OPC) to a mature, functional, myelinating oligodendrocyte (OL) (75).
microRNAs upregulated in active MS lesions, including miR-326, miR-155, and miR-34a target the 3’-untranslated region of CD47 in reporter assays. This targeting reduces CD47 levels in brain resident cells, shifting macrophages from inhibitory control (28). Furthermore, miR-23b has been found to suppress auto-inflammatory responses associated with IL-17 in systemic lupus erythematosus (SLE) and mouse models of MS (109).
In individuals with MS, there is a notable reduction in the levels of miR-223, miR-15b, and miR-23a in their serum (110). Specifically, miR-23a/b can be regarded as a distinctive astrocytic marker, and its expression is influenced by stimuli promoting astrocyte differentiation (111). miR-23a, linked with certain long non-coding RNAs (lncRNAs), participates in a complex regulatory network that modulates critical genes for myelin formation and maintenance. miR-23a appears to play a role in advancing less-differentiated oligodendrocyte progenitor cells (OPCs) toward myelination and mature oligodendrocytes (OLs), likely by enhancing the expression of genes enriched in mature OLs. This regulatory mechanism may also comprise the suppression of genes enriched in neurons and, to a lesser extent, astrocytes (112, 112). Astrocytes, a type of glial cell in the central nervous system, assume diverse responsibilities in maintaining a healthy neural environment and the integrity of the BBB (113).
The elevated levels of miR-23a, a microRNA that promotes myelin production, in MS brain tissue may be attributed to the ongoing remyelination process in MS through OLs. However, it is worth noting that peripheral immune cells in MS patients also exhibit increased miR-23a expression. Therefore, the heightened miR-23a levels in the MS brain may stem from increased infiltration of immune cells (112). Taken together, the contrasting regulation of miR-23a in OPCs and astrocytes suggests a complex interplay of molecular events during MS. Future studies on the current issue are therefore required to comprehensively understand the specific targets and functions of miR-23a in these cell types in the context of MS.
Under normal physiological conditions, there is a minimal level of immune cell trafficking across the BBB as a component of the brain’s immunosurveillance, which is essential for effective immune response within the brain and clearance of infectious pathogens (114, 115). In the presence of inflammation, a disrupted BBB compromises the brain’s immune privilege, exposing neuronal antigens to peripheral inflammatory molecules. This exposure further stimulates the inflammatory response within the brain, ultimately accelerating the development of neurological diseases (2).
Inflammatory cytokines, including interleukin-1β, TGF-β, TNF-α, and IL-6, have been shown to be upregulated during pathological processes that compromise BBB function (2, 116, 117). Several lines of evidence from animal studies also support the role of IL-1β in BBB dysfunction during neuroinflammation. Treatment with IL-1β receptor antagonists or genetic deletion of the IL-1 receptor attenuates neuroinflammation-induced BBB hyperpermeability in mice (2, 118).
microRNAs can also utilize their influence on markers or inflammatory cytokines in order to regulate the integrity of the BBB. For instance, miR-21-5p modulated the BBB through the targeting of pro-inflammatory cytokines such as TNF-α, NF-kB, and IL-6 signaling pathway (119). Furthermore, the enhanced expression of let-7g and miR-98 has been found to diminish neuro-inflammation prompted by BBB permeability (120).
Moreover, it has been reported that several miRNAs such as miR-125-5p and miR-320a have shown reduced expression in brain/spinal cord tissues or blood samples from patients with multiple sclerosis, as well as in in vitro BBB models exposed to pro-inflammatory cytokine. These downregulated miRNAs contain miR-126, miR-125-5p, miR-126, and miR-320a as reported in various studies (121, 122).
In cases of brain tumors, regions with an intact BBB hinder the delivery of drugs to tumor cells at therapeutic concentrations. In the context of BBB penetration, exosomes can transport endogenous peptides and proteins from one side of the BBB to the other. This indicates that the paracellular pathway is less critical than previously believed for delivering therapeutics to tumors (123). Exosomes are extracellular vesicles and small membrane-bound carriers released by cells to facilitate communication with distant or neighboring cells. Exosomes possess unique properties, including specific surface proteins, which guide them to specific recipient cells for binding and uptake (124, 125).
Remarkably, exosomes have emerged as potential contributors to the preservation of BBB integrity, such as exosomes derived from neurons that carry miR-132. These specialized exosomes facilitate communication between brain endothelial cells and neurons, ultimately playing a significant role in upholding the integrity of the BBB (2).
To ensure inherent compatibility with human cells, extracellular vesicles (EVs) efficiently transport cellular membranes and do not encounter various challenges related to drug delivery, including issues like RNA degradation, accumulation in endosomes, susceptibility to phagocytosis, resistance to multiple drugs, potential cytotoxicity, and immunogenic responses (126, 127). It is recommended that further research should be undertaken using the following strategies and utilizing exosomes for miRNA and mRNA delivery in the treatment of various diseases such as MS that has been provided (127).
Some recent studies have investigated the effect of some usual drugs used in MS on miRNA levels. Ingwersen et al. found that miR-29a, miR-103, miR-18, and miR-20b were increased after natalizumab treatment, and miR-20b and miR-326 play an essential role in regulating Th17 responses and blood-brain barrier damage in patients. miR-29a was also involved in the differentiation and expansion of Th1 responses. Petrocca et al. reported that natalizumab increased miR-106b and decreased the expression of miR-17, which are miRNAs involved in TGF-𝛽 signaling and the expansion of CD4+ T cell responses by targeting TGFBR2.
Also, the reduction of miR-17 expression has been associated with the elevation of pro-apoptotic proteins, including the BIM, Bcl-2 family, the cyclin-dependent kinase inhibitor 1, p21, and E2F1. These targets, which are altered by natalizumab, can modify cellular responses in the EAE model and correlate with disease severity inversely. Therefore, the effect of natalizumab on miRNA expression can help to comprehend its impact on the process of pathogenesis and disease progression. It has been shown that INF-𝛽 treatment increased the expression of miR-26a-5p in MS patients. It has been reported that miR-26a-5p is associated with the reduction of postsynaptic density protein 95 (DLG4), which plays a crucial role in neuron signaling after 3 months of INF-𝛽 treatment (128).
This evidence suggests that these markers may be used as novel biomarkers to investigate the therapeutic effect of IFN-𝛽 and develop new treatments for MS patients. Treatment with Glatiramer acetate (GA) can reduce miR-142-3p and miR-146a expression in PBMC of MS patients, in which miR-142-3p can affect the expansion of Treg responses. Reducing miR-146a expression can shift Th1 responses to Th2 and improve MS patients by inhibiting monocyte response (129) (Table 2). Understanding the complexity of the miRNA network can open a new window for finding individual biomarkers in clinical diagnosis and monitoring the effectiveness of treatment. These findings showed that miRNAs may support and evaluate therapeutic effects.
The development of disease-modifying drugs for the treatment of MS has recently received much attention. Interferon-β (IFN-β) and glatiramer acetate reduce the risk of patients returning to the level of RR-MS; approximately one-third of RR-MS patients had an adverse (inadequate) response to drugs. It is one of the important principles that have been the focus of researchers who use miRNAs as new molecular drugs for MS. However, similar problems have been observed with other drugs, such as toxicity, side effects, and stability of miRNAs in target cells, which must all be resolved.
Also, miRNA selection as a drug for a pathological condition should be based on target detection and confirmation. The role of genes in disease is another factor in adopting miRNA as a drug. Vandenbroek et al. discovered that SOCS1 is a crucial regulator of cytokinin signaling. It has been identified that IFN-β performed modulatory effects on the immune system through the induction of SOCS3 and SOCS1 in various types of inflammatory sub-cells, thereby preventing inflammatory reactions in cells. It has been reported that SOCS1 is a therapeutic tool for the EAE mouse model of multiple sclerosis. Therefore, SOCS1-targeting miRNAs may be good candidates for influencing immune responses to cytokinins by regulating SOCS1 expression.
Although no miRNA drug candidates have reached phase 3 trials according to the “Clintrials.gov” database, there are ongoing phase trials for drugs like miR-122/Miravirsen and MRX34. miR-122/Miravirsen is an anti-miR drug composed of locked nucleic acid (LNAs) ribonucleotides that are currently being tested for the treatment of HCV infections (130). MRX34 is a liposomal formulation of miR-34a, which is a tumor suppressor that is often lost or expressed at reduced levels in various types of tumors. It is being investigated as a potential first-in-class miRNA mimic cancer therapy (131, 132). MRX34 has been registered in the “Clintrials.gov” database for a Phase 1 study on microRNA-based cancer therapy (133).
A major challenge lies in delivering drugs to the brain. Various methods such as modified micelles, liposomes, nanoparticles, intranasal administration, and other delivery approaches have been tested to overcome the BBB with varying degrees of success (134). One complex approach involves designing lipid-soluble small molecules weighing less than 400 daltons that can successfully cross the blood-brain barrier, while macromolecules struggle to penetrate it effectively. miRNA-based drugs have demonstrated significant effectiveness in treating various health conditions such as hepatitis C, cancer, cardiac abnormalities, and pathological fibrosis kidney disease. Some miRNA candidates have shown promising results in clinical trials, while others have not been successful. Additionally, there is an ongoing Phase 1 trial for MRG 110, an antisense oligonucleotide that uses locked nucleic acid (LNA) to block the function of miR-92 (135). MiRagen has recently announced a Phase 1 trial for MRG 110 as a potential application in wound healing and heart failure (135, 136). MiRagen also has active Phase 1 and Phase 2 trials for miR-155 (Cobomarsen; MRG-106) and miR-29 (MRG-201), respectively, targeting keloid and scar tissue formation and T lymphoma patients. In 2019, Regulus suggested a new potential miRNA drug called RGLS5579, which targets miR-10b and may be used in trials for patients with glioblastoma multiforme (137). Recently, a Phase 1 trial using a newer technology called “TargomiR” showed promising results in patients with non-small cell lung cancer or recurrent malignant pleural mesothelioma (Table 3).
Briefly, TargomiR delivery vehicles contain a miRNA mimic, a small cell derived from bacteria, and a targeting component such as an antibody that recognizes a specific protein in the target cells. In the first human trial of the drug TargomiR called MesomiR-1, the miRNA mimic miR-16 was found to be a tumor suppressor and the target group was lung cancer cells that overexpressed the EGFR antibody (138, 139). These studies provide hope for mesothelioma patients who have low survival rates (136). The first human trial of siRNA took place in 2004 and the first siRNA drug was approved in 2018 (140).
miRNAs play roles in various cellular processes and have been identified as post-transcriptional gene expression regulators. miRNAs play a key role in various vital processes such as cell growth, development, apoptosis, and cell differentiation. miRNAs’ function and regulation of their expression are still largely unknown, and even more complex is the regulatory network between miRNAs and their target genes. Recent studies have revealed a unique miRNA pattern in different body tissues. To date, clinical applications in peripheral tissues have been reported for miRNA mimics (to overexpress transcripts) and miRNA suppressors (to silence the function of transcripts). Dysregulated miRNAs influence the inflammatory response and BBB permeability. miRNA alterations can serve as potential biomarkers to assess treatment efficacy. The altered expression of specific miRNAs has been linked to the development of MS and the differentiation of pathogenic T cells. Furthermore, miRNAs have emerged as promising candidates for distinguishing different types of MS and assessing treatment responses.
As discoveries from the Human Genome Project continue to shape clinical research, emerging miRNA drugs offer potential treatments for health conditions that cannot be addressed by current options. Bioinformatics programs aid in related biological pathways and identifying miRNA binding sites in target genes. In vivo experiments have also played a role in accelerating the translation of miRNAs into clinical medicine. These experiments have paved the way for investigating miRNAs, which are non-coding RNA transcripts that outnumber protein-coding genes. Future human experiments will likely focus on epigenetic targets. MiRNAs could serve as valuable diagnostic tools as their expression patterns in biological samples, such as blood and cerebrospinal fluid, can differentiate MS from other neurological diseases. Additionally, they discuss the challenges of drug delivery to the brain and the potential of miRNA-based therapies, including miRNA mimics and antisense oligonucleotides, as a novel approach to treating MS by regulating the expression of specific target genes. Ongoing clinical trials are investigating the potential of miRNA-based therapies in various diseases, including cancer and MS. Research into miRNA-based therapies is ongoing, offering potential avenues for the development of novel treatments for MS and other diseases. Understanding the complex network of miRNAs in MS provides valuable insights into the disease’s mechanisms and potential therapeutic interventions.
AEN: Conceptualization, Data curation, Writing – original draft. SMMZ: Writing – review & editing. HN: Writing – review & editing. ASA: Methodology, Writing – review & editing. AF: Investigation, Methodology, Writing – review & editing. MA: Investigation, Writing – review & editing. RN: Conceptualization, Writing – review & editing. SS: Writing – review & editing. MM: Conceptualization, Methodology, Supervision, Writing – review & editing.
The author(s) declare financial support was received for the research, authorship, and/or publication of this article.
We would like to express our gratitude to Gordon A. Ferns at Brighton and Sussex Medical School for reviewing and improving the text.
The authors declare that the research was conducted in the absence of any commercial or financial relationships that could be construed as a potential conflict of interest.
All claims expressed in this article are solely those of the authors and do not necessarily represent those of their affiliated organizations, or those of the publisher, the editors and the reviewers. Any product that may be evaluated in this article, or claim that may be made by its manufacturer, is not guaranteed or endorsed by the publisher.
EBV, Epstein-Barr virus; EGFR, Epidermal Growth Factor Receptor; FGF, Fibroblast Growth Factor; FLIP, FLICE-like Inhibitory Protein; HCV, Hepatitis C virus; LMP2A, Latent Membrane Protein 2A; miRNA, microRNA; NF-kB, Nuclear factor-Kb; PBMC, Peripheral blood mononuclear cells; PPMS, Primary-progressive multiple sclerosis; PI3KR, Phosphatidylinositol 3-kinase receptor; RRMS, Relapsing-Remitting MS; SMAD, Small mothers against decapentaplegic; SPMS, Secondary- progressive multiple sclerosis; TGF-β, Transforming growth factor beta; Tregs, Regulatory T cells.
1. Huang WJ, Chen WW, Zhang X. Multiple sclerosis: Pathology, diagnosis and treatments. Exp Ther Med (2017) 13(6):3163–6. doi: 10.3892/etm.2017.4410
2. Li J, Zheng M, Shimoni O, Banks WA, Bush AI, Gamble JR, et al. Development of novel therapeutics targeting the blood-brain barrier: from barrier to carrier. Advanced Sci (Weinheim Baden-Wurttemberg Germany) (2021) 8(16):e2101090. doi: 10.1002/advs.202101090
3. Dudek KA, Dion-Albert L, Lebel M, LeClair K, Labrecque S, Tuck E, et al. Molecular adaptations of the blood-brain barrier promote stress resilience vs. depression. Proc Natl Acad Sci U S A (2020) 117(6):3326–36. doi: 10.1073/pnas.1914655117
4. Sweeney MD, Kisler K, Montagne A, Toga AW, Zlokovic BV. The role of brain vasculature in neurodegenerative disorders. Nat Neurosci (2018) 21(10):1318–31. doi: 10.1038/s41593-018-0234-x
5. Sweeney MD, Montagne A, Sagare AP, Nation DA, Schneider LS, Chui HC, et al. Vascular dysfunction-The disregarded partner of Alzheimer's disease. Alzheimer's dementia (2019) 15(1):158–67. doi: 10.1016/j.jalz.2018.07.222
6. Correale J, Lund B, McMillan M, Ko DY, McCarthy K, Weiner LP. T cell vaccination in secondary progressive multiple sclerosis. J Neuroimmunol (2000) 107(2):130–9. doi: 10.1016/S0165-5728(00)00235-6
7. Segal BM, Cross AH. Fas(t) track to apoptosis in MS: TNF receptors may suppress or potentiate CNS demyelination. Neurology (2000) 55(7):906–7. doi: 10.1212/WNL.55.7.906
8. Sharief MK, Semra YK, Seidi OA, Zoukos Y. Interferon-beta therapy downregulates the anti-apoptosis protein FLIP in T cells from patients with multiple sclerosis. J Neuroimmunol (2001) 120(1-2):199–207. doi: 10.1016/S0165-5728(01)00422-2
9. Hecker M, Thamilarasan M, Koczan D, Schröder I, Flechtner K, Freiesleben S, et al. MicroRNA expression changes during interferon-beta treatment in the peripheral blood of multiple sclerosis patients. Int J Mol Sci (2013) 14(8):16087–110. doi: 10.3390/ijms140816087
10. Raphael I, Webb J, Stuve O, Haskins W, Forsthuber T. Body fluid biomarkers in multiple sclerosis: how far we have come and how they could affect the clinic now and in the future. Expert Rev Clin Immunol (2015) 11(1):69–91. doi: 10.1586/1744666X.2015.991315
11. Guerau-de-Arellano M, Smith KM, Godlewski J, Liu Y, Winger R, Lawler SE, et al. Micro-RNA dysregulation in multiple sclerosis favours pro-inflammatory T-cell-mediated autoimmunity. Brain J Neurol (2011) 134 (Pt. 12):3578–89. doi: 10.1093/brain/awr262
12. Hampe, Christiane S. “B Cell in Autoimmune Diseases.” Scientifica (2012) 2012:215308. doi: 10.6064/2012/215308
13. Bennett JL, O'Connor KC, Bar-Or A, Zamvil SS, Hemmer B, Tedder TF, et al. B lymphocytes in neuromyelitis optica. Neurology(R) neuroimmunology neuroinflammation (2015) 2(3):e104. doi: 10.1212/NXI.0000000000000104
14. Browning JL. B cells move to centre stage: novel opportunities for autoimmune disease treatment. Nat Rev Drug discovery (2006) 5(7):564–76. doi: 10.1038/nrd2085
15. Salomonsson S, Larsson P, Tengnér P, Mellquist E, Hjelmström P, Wahren-Herlenius M. Expression of the B cell-attracting chemokine CXCL13 in the target organ and autoantibody production in ectopic lymphoid tissue in the chronic inflammatory disease Sjögren's syndrome. Scandinavian J Immunol (2002) 55(4):336–42. doi: 10.1046/j.1365-3083.2002.01058.x
16. Groom J, Kalled SL, Cutler AH, Olson C, Woodcock SA, Schneider P, et al. Association of BAFF/BLyS overexpression and altered B cell differentiation with Sjögren's syndrome. J Clin Invest (2002) 109(1):59–68. doi: 10.1172/JCI0214121
17. Fava RA, Kennedy SM, Wood SG, Bolstad AI, Bienkowska J, Papandile A, et al. Lymphotoxin-beta receptor blockade reduces CXCL13 in lacrimal glands and improves corneal integrity in the NOD model of Sjögren's syndrome. Arthritis Res Ther (2011) 13(6):R182. doi: 10.1186/ar3507
18. Ruggieri M, Avolio C, Scacco S, Pica C, Lia A, Zimatore GB, et al. Glatiramer acetate induces pro-apoptotic mechanisms involving Bcl-2, Bax and Cyt-c in peripheral lymphocytes from multiple sclerosis patients. J neurol (2006) 253(2):231–6. doi: 10.1007/s00415-005-0965-y
19. Bartel DP. MicroRNAs: genomics, biogenesis, mechanism, and function. Cell (2004) 116(2):281–97. doi: 10.1016/S0092-8674(04)00045-5
20. Jagot F, Davoust N. Is It worth Considering Circulating microRNAs in Multiple Sclerosis? Front Immunol (2016) 7:129. doi: 10.3389/fimmu.2016.00129
21. Mirshafiey A, Kianiaslani M. Autoantigens and autoantibodies in multiple sclerosis. Iranian J allergy asthma Immunol (2013) 12(4):292–303.
22. Ruan K, Fang X, Ouyang G. MicroRNAs: novel regulators in the hallmarks of human cancer. Cancer letters (2009) 285(2):116–26. doi: 10.1016/j.canlet.2009.04.031
23. Reddy SD, Gajula RP, Pakala SB, Kumar R. MicroRNAs and cancer therapy: the next wave or here to stay? Cancer Biol Ther (2010) 9(7):479–82. doi: 10.4161/cbt.9.7.11402
24. Seto AG. The road toward microRNA therapeutics. Int J Biochem Cell Biol (2010) 42(8):1298–305. doi: 10.1016/j.biocel.2010.03.003
25. Ardekani AM, Naeini MM. The role of microRNAs in human diseases. Avicenna J Med Biotechnol (2010) 2(4):161–79.
26. Ma X, Zhou J, Zhong Y, Jiang L, Mu P, Li Y, et al. Expression, regulation and function of microRNAs in multiple sclerosis. Int J Med Sci (2014) 11(8):810–8. doi: 10.7150/ijms.8647
27. Qiu S, Lin S, Hu D, Feng Y, Tan Y, Peng Y. Interactions of miR-323/miR-326/miR-329 and miR-130a/miR-155/miR-210 as prognostic indicators for clinical outcome of glioblastoma patients. J Trans Med (2013) 11:10. doi: 10.1186/1479-5876-11-10
28. Junker A, Krumbholz M, Eisele S, Mohan H, Augstein F, Bittner R, et al. MicroRNA profiling of multiple sclerosis lesions identifies modulators of the regulatory protein CD47. Brain J Neurol (2009) 132(Pt 12):3342–52. doi: 10.1093/brain/awp300
29. Du C, Liu C, Kang J, Zhao G, Ye Z, Huang S, et al. MicroRNA miR-326 regulates TH-17 differentiation and is associated with the pathogenesis of multiple sclerosis. Nat Immunol (2009) 10(12):1252–9. doi: 10.1038/ni.1798
30. Huang Y, Zou Q, Wang SP, Tang SM, Zhang GZ, Shen XJ. Construction and detection of expression vectors of microRNA-9a in BmN cells. J Zhejiang Univ Sci B (2011) 12(7):527–33. doi: 10.1631/jzus.B1000296
31. Wu T, Chen G. miRNAs participate in MS pathological processes and its therapeutic response. Mediators inflammation (2016) 2016:4578230. doi: 10.1155/2016/4578230
32. Fawaz CN, Makki IS, Kazan JM, Gebara NY, Andary FS, Itani MM, et al. Neuroproteomics and microRNAs studies in multiple sclerosis: transforming research and clinical knowledge in biomarker research. Expert Rev proteomics (2015) 12(6):637–50. doi: 10.1586/14789450.2015.1099435
33. Regev K, Paul A, Healy B, von Glenn F, Diaz-Cruz C, Gholipour T, et al. Comprehensive evaluation of serum microRNAs as biomarkers in multiple sclerosis. Neurology(R) neuroimmunology neuroinflammation (2016) 3(5):e267. doi: 10.1212/NXI.0000000000000267
34. Wang WT, Chen YQ. Circulating miRNAs in cancer: from detection to therapy. J Hematol Oncol (2014) 7:86. doi: 10.1186/s13045-014-0086-0
35. Zeng L, Cui J, Wu H, Lu Q. The emerging role of circulating microRNAs as biomarkers in autoimmune diseases. Autoimmunity (2014) 47(7):419–29. doi: 10.3109/08916934.2014.929667
36. Sapre N, Macintyre G, Clarkson M, Naeem H, Cmero M, Kowalczyk A, et al. A urinary microRNA signature can predict the presence of bladder urothelial carcinoma in patients undergoing surveillance. Br J cancer (2016) 114(4):454–62. doi: 10.1038/bjc.2015.472
37. Preskill C, Weidhaas JB. SNPs in microRNA binding sites as prognostic and predictive cancer biomarkers. Crit Rev oncogenesis (2013) 18(4):327–40. doi: 10.1615/CritRevOncog.2013007254
38. Chen C, Zhou Y, Wang J, Yan Y, Peng L, Qiu W. Dysregulated microRNA involvement in multiple sclerosis by induction of T helper 17 cell differentiation. Front Immunol (2018) 9:1256. doi: 10.3389/fimmu.2018.01256
39. Otaegui D, Baranzini SE, Armañanzas R, Calvo B, Muñoz-Culla M, Khankhanian P, et al. Differential micro RNA expression in PBMC from multiple sclerosis patients. PLoS One (2009) 4(7):e6309. doi: 10.1371/journal.pone.0006309
40. Keller A, Leidinger P, Lange J, Borries A, Schroers H, Scheffler M, et al. Multiple sclerosis: microRNA expression profiles accurately differentiate patients with relapsing-remitting disease from healthy controls. PloS One (2009) 4(10):e7440. doi: 10.1371/journal.pone.0007440
41. Lindberg RL, Hoffmann F, Mehling M, Kuhle J, Kappos L. Altered expression of miR-17-5p in CD4+ lymphocytes of relapsing-remitting multiple sclerosis patients. Eur J Immunol (2010) 40(3):888–98. doi: 10.1002/eji.200940032
42. De Santis G, Ferracin M, Biondani A, Caniatti L, Rosaria Tola M, Castellazzi M, et al. Altered miRNA expression in t regulatory cells in course of multiple sclerosis. J Neuroimmunol (2010) 226(1-2):165–71. doi: 10.1016/j.jneuroim.2010.06.009
43. Cox MB, Cairns MJ, Gandhi KS, Carroll AP, Moscovis S, Stewart GJ, et al. MicroRNAs miR-17 and miR-20a inhibit t cell activation genes and are under-expressed in MS whole blood. PloS One (2010) 5(8):e12132. doi: 10.1371/journal.pone.0012132
44. Fenoglio C, Cantoni C, De Riz M, Ridolfi E, Cortini F, Serpente M, et al. Expression and genetic analysis of miRNAs involved in CD4+ cell activation in patients with multiple sclerosis. Neurosci letters (2011) 504(1):9–12. doi: 10.1016/j.neulet.2011.08.021
45. Waschbisch A, Atiya M, Linker RA, Potapov S, Schwab S, Derfuss T. Glatiramer acetate treatment normalizes deregulated microRNA expression in relapsing remitting multiple sclerosis. PloS One (2011) 6(9):e24604. doi: 10.1371/journal.pone.0024604
46. Siegel SR, Mackenzie J, Chaplin G, Jablonski NG, Griffiths L. Circulating microRNAs involved in multiple sclerosis. Mol Biol Rep (2012) 39(5):6219–25. doi: 10.1007/s11033-011-1441-7
47. Noorbakhsh F, Ellestad KK, Maingat F, Warren KG, Han MH, Steinman L, et al. Impaired neurosteroid synthesis in multiple sclerosis. Brain (2011) 134(Pt 9):2703–21. doi: 10.1093/brain/awr200
48. Sievers C, Meira M, Hoffmann F, Fontoura P, Kappos L, Lindberg RL. Altered microRNA expression in b lymphocytes in multiple sclerosis: towards a better understanding of treatment effects. Clin Immunol (2012) 144:70–9.
49. Martinelli-Boneschi F, Esposito F, Brambilla P, Lindström E, Lavorgna G, Stankovich J, et al. A genome-wide association study in progressive multiple sclerosis. Mult Scler. (2012) 18(10):1384–94. doi: 10.1177/1352458512439118
50. Haghikia A, Haghikia A, Hellwig K, Baraniskin A, Holzmann A, Décard BF, et al. Regulated microRNAs in the CSF of patients with multiple sclerosis: a case-control study. Neurology (2012) 79(22):2166–70. doi: 10.1212/WNL.0b013e3182759621
51. Yang D, Wang WZ, Zhang XM, Yue H, Li B, Lin L, et al. MicroRNA expression aberration in chinese patients with relapsing remitting multiple sclerosis. J Mol Neurosci (2014) 52(1):131–7. doi: 10.1007/s12031-013-0138-x
52. Gandhi R, Healy B, Gholipour T, Egorova S, Musallam A, Hussain MS, et al. Circulating microRNAs as biomarkers for disease staging in multiple sclerosis. Ann Neurol (2013) 73(6):729–40. doi: 10.1002/ana.23880
53. Søndergaard HB, Hesse D, Krakauer M, Sørensen PS, Sellebjerg F. Differential microRNA expression in blood in multiple sclerosis. Mult Scler. (2013) 19(14):1849–57. doi: 10.1177/1352458513490542
54. Keller A, Leidinger P, Lange J, Borries A, Schroers H, Scheffler M, et al. Multiple sclerosis: microRNA expression profiles accurately differentiate patients with relapsing-remitting disease from healthy controls. PloS One (2009) 4(10):e7440. doi: 10.1371/journal.pone.0007440
55. Kacperska MJ, Jastrzebski K, Tomasik B, Walenczak J, Konarska-Krol M, Glabinski A. Selected extracellular microRNA as potential biomarkers of multiple sclerosis activity–preliminary study. J Mol Neurosci (2015) 56(1):154–63. doi: 10.1007/s12031-014-0476-3
56. Mancuso R, Hernis A, Agostini S, Rovaris M, Caputo D, Clerici M. MicroRNA-572 expression in multiple sclerosis patients with different patterns of clinical progression. J Transl Med (2015) 13:148. doi: 10.1186/s12967-015-0504-2
57. Freiesleben S, Hecker M, Zettl UK, Fuellen G, Taher L. Analysis of microRNA and gene expression profiles in multiple sclerosis: Integrating interaction data to uncover regulatory mechanisms. Sci Rep (2016) 6:34512. doi: 10.1038/srep34512
58. Vistbakka J, Elovaara I, Lehtimäki T, Hagman S. Circulating microRNAs as biomarkers in progressive multiple sclerosis. Mult Scler. (2017) 23(3):403–12. doi: 10.1177/1352458516651141
59. Ebrahimkhani S, Vafaee F, Young PE, Hur SSJ, Hawke S, Devenney E, et al. Exosomal microRNA signatures in multiple sclerosis reflect disease status. Sci Rep (2017) 7(1):14293. doi: 10.1038/s41598-017-14301-3
60. Liguori M, Nuzziello N, Simone M, Amoroso N, Viterbo RG, Tangaro S, et al. Association between miRNAs expression and cognitive performances of pediatric multiple sclerosis patients: A pilot study. Brain Behav (2019) 9(2):e01199. doi: 10.1002/brb3.1199
61. Yang Q, Pan W, Qian L. Identification of the miRNA-mRNA regulatory network in multiple sclerosis. Neurol Res (2017) 39(2):142–51. doi: 10.1080/01616412.2016.1250857
62. Muñoz-San Martín M, Reverter G, Robles-Cedeño R, Buxò M, Ortega FJ, Gómez I, et al. Analysis of miRNA signatures in CSF identifies upregulation of miR-21 and miR-146a/b in patients with multiple sclerosis and active lesions. J Neuroinflammation. (2019) 16(1):220. doi: 10.1186/s12974-019-1590-5
63. Fenoglio C, Ridolfi E, Galimberti D, Scarpini E. MicroRNAs as active players in the pathogenesis of multiple sclerosis. Int J Mol Sci (2012) 13(10):13227–39. doi: 10.3390/ijms131013227
64. Bartel DP. MicroRNAs: target recognition and regulatory functions. Cell (2009) 136(2):215–33. doi: 10.1016/j.cell.2009.01.002
65. Liston A, Linterman M, Lu LF. MicroRNA in the adaptive immune system, in sickness and in health. J Clin Immunol (2010) 30(3):339–46. doi: 10.1007/s10875-010-9378-5
66. Tesmer LA, Lundy SK, Sarkar S, Fox DA. Th17 cells in human disease. Immunol Rev (2008) 223:87–113. doi: 10.1111/j.1600-065X.2008.00628.x
67. Akkina S, Becker BN. MicroRNAs in kidney function and disease. Trans Res J Lab Clin Med (2011) 157(4):236–40. doi: 10.1016/j.trsl.2011.01.011
68. Zhang YF, Xu HM, Yu F, Wang M, Li MY, Xu T, et al. Crosstalk between microRNAs and peroxisome proliferator-activated receptors and their emerging regulatory roles in cardiovascular pathophysiology. PPAR Res (2018) 2018:8530371. doi: 10.1155/2018/8530371
69. Lorenzi JC, Brum DG, Zanette DL, de Paula Alves Souza A, Barbuzano FG, Dos Santos AC, et al. miR-15a and 16-1 are downregulated in CD4+ T cells of multiple sclerosis relapsing patients. Int J Neurosci (2012) 122(8):466–71. doi: 10.3109/00207454.2012.678444
70. Gangaraju VK, Lin H. MicroRNAs: key regulators of stem cells. Nat Rev Mol Cell Biol (2009) 10(2):116–25. doi: 10.1038/nrm2621
71. Ahmadian-Elmi M, Bidmeshki Pour A, Naghavian R, Ghaedi K, Tanhaei S, Izadi T, et al. miR-27a and miR-214 exert opposite regulatory roles in Th17 differentiation via mediating different signaling pathways in peripheral blood CD4+ T lymphocytes of patients with relapsing-remitting multiple sclerosis. Immunogenetics (2016) 68(1):43–54. doi: 10.1007/s00251-015-0881-y
72. Smyth LA, Boardman DA, Tung SL, Lechler R, Lombardi G. MicroRNAs affect dendritic cell function and phenotype. Immunology (2015) 144(2):197–205. doi: 10.1111/imm.12390
73. Zhang Z, Xue Z, Liu Y, Liu H, Guo X, Li Y, et al. MicroRNA-181c promotes Th17 cell differentiation and mediates experimental autoimmune encephalomyelitis. Brain behavior immunity. (2018) 70:305–14. doi: 10.1016/j.bbi.2018.03.011
74. Reijerkerk A, Lopez-Ramirez MA, van Het Hof B, Drexhage JA, Kamphuis WW, Kooij G, et al. MicroRNAs regulate human brain endothelial cell-barrier function in inflammation: implications for multiple sclerosis. J Neurosci (2013) 33(16):6857–63. doi: 10.1523/JNEUROSCI.3965-12.2013
75. Galloway DA, Moore CS. miRNAs as emerging regulators of oligodendrocyte development and differentiation. Front Cell Dev Biol (2016) 4:59. doi: 10.3389/fcell.2016.00059
76. Indrieri A, Carrella S, Carotenuto P, Banfi S, Franco B. The pervasive role of the miR-181 family in development, neurodegeneration, and cancer. Int J Mol Sci (2020) 21(6):2092. doi: 10.3390/ijms21062092
77. Wei B, Pei G. microRNAs: critical regulators in Th17 cells and players in diseases. Cell Mol Immunol (2010) 7(3):175–81. doi: 10.1038/cmi.2010.19
78. de Yébenes VG, Bartolomé-Izquierdo N, Ramiro AR. Regulation of B-cell development and function by microRNAs. Immunol Rev (2013) 253(1):25–39. doi: 10.1111/imr.12046
79. Zhang Y, Zhang D, Wang F, Xu D, Guo Y, Cui W. Serum miRNAs panel (miR-16-2*, miR-195, miR-2861, miR-497) as novel non-invasive biomarkers for detection of cervical cancer. Sci Rep (2015) 5:17942. doi: 10.1038/srep17942
80. Chapman CG, Pekow J. The emerging role of miRNAs in inflammatory bowel disease: a review. Ther Adv gastroenterol (2015) 8(1):4–22. doi: 10.1177/1756283X14547360
81. Podshivalova K, Salomon DR. MicroRNA regulation of T-lymphocyte immunity: modulation of molecular networks responsible for T-cell activation, differentiation, and development. Crit Rev Immunol (2013) 33(5):435–76. doi: 10.1615/CritRevImmunol.2013006858
82. Murugaiyan G, da Cunha AP, Ajay AK, Joller N, Garo LP, Kumaradevan S, et al. MicroRNA-21 promotes Th17 differentiation and mediates experimental autoimmune encephalomyelitis. J Clin Invest (2015) 125(3):1069–80. doi: 10.1172/JCI74347
83. Guinea-Viniegra J, Jiménez M, Schonthaler HB, Navarro R, Delgado Y, Concha-Garzón MJ, et al. Targeting miR-21 to treat psoriasis. Sci Trans Med (2014) 6(225):225re1. doi: 10.1126/scitranslmed.3008089
84. Kauppinen S, Vester B, Wengel J. Locked nucleic acid (LNA): High affinity targeting of RNA for diagnostics and therapeutics. Drug Discovery Today Technologies (2005) 2(3):287–90. doi: 10.1016/j.ddtec.2005.08.012
85. Braasch DA, Corey DR. Locked nucleic acid (LNA): fine-tuning the recognition of DNA and RNA. Chem Biol (2001) 8(1):1–7. doi: 10.1016/S1074-5521(00)00058-2
86. Chandan K, Gupta M, Sarwat M. Role of host and pathogen-derived microRNAs in immune regulation during infectious and inflammatory diseases. Front Immunol (2019) 10:3081. doi: 10.3389/fimmu.2019.03081
87. Junker A. Pathophysiology of translational regulation by microRNAs in multiple sclerosis. FEBS letters (2011) 585(23):3738–46. doi: 10.1016/j.febslet.2011.03.052
88. Ma X, Conklin DJ, Li F, Dai Z, Hua X, Li Y, et al. The oncogenic microRNA miR-21 promotes regulated necrosis in mice. Nat Commun (2015) 6:7151. doi: 10.1038/ncomms8151
89. Gandhi R. miRNA in multiple sclerosis: search for novel biomarkers. Multiple sclerosis (Houndmills Basingstoke England) (2015) 21(9):1095–103. doi: 10.1177/1352458515578771
90. Segarra M, Aburto MR, Acker-Palmer A. Blood-brain barrier dynamics to maintain brain homeostasis. Trends neurosciences (2021) 44(5):393–405. doi: 10.1016/j.tins.2020.12.002
91. Bhattacharya A, Kaushik DK, Lozinski BM, Yong VW. Beyond barrier functions: Roles of pericytes in homeostasis and regulation of neuroinflammation. J Neurosci Res (2020) 98(12):2390–405. doi: 10.1002/jnr.24715
92. Fong CW. Permeability of the blood–brain barrier: molecular mechanism of transport of drugs and physiologically important compounds. J Membrane Biol (2015) 248(4):651–69. doi: 10.1007/s00232-015-9778-9
93. Sonar SA, Lal G. Blood–brain barrier and its function during inflammation and autoimmunity. J Leukocyte Biol (2018) 103(5):839–53. doi: 10.1002/JLB.1RU1117-428R
94. Wu D, Chen Q, Chen X, Han F, Chen Z, Wang Y. The blood–brain barrier: structure, regulation, and drug delivery. Signal Transduction Targeted Ther (2023) 8(1):217. doi: 10.1038/s41392-023-01481-w
95. Alahmari A. Blood-brain barrier overview: structural and functional correlation. Neural plasticity (2021) 2021:6564585. doi: 10.1155/2021/6564585
96. Lochhead JJ, Yang J, Ronaldson PT, Davis TP. Structure, function, and regulation of the blood-brain barrier tight junction in central nervous system disorders. Front. Physiol (2020) 11:914. doi: 10.3389/fphys.2020.00914
97. de Faria O Jr., Moore CS, Kennedy TE, Antel JP, Bar-Or A, Dhaunchak AS. MicroRNA dysregulation in multiple sclerosis. Front Genet (2012) 3:311. doi: 10.3389/fgene.2012.00311
98. Figueroa EG, Caballero-Román A, Ticó JR, Miñarro M, Nardi-Ricart A, González-Candia A. miRNA nanoencapsulation to regulate the programming of the blood-brain barrier permeability by hypoxia. Curr Res Pharmacol Drug Discov (2022) 3:100129. doi: 10.1016/j.crphar.2022.100129
99. Bovari-Biri J, Garai K, Banfai K, Csongei V, Pongracz JE. miRNAs as Predictors of Barrier Integrity. Biosensors (2023) 13(4):422. doi: 10.3390/bios13040422
100. Dithmer S, Staat C, Müller C, Ku MC, Pohlmann A, Niendorf T, et al. Claudin peptidomimetics modulate tissue barriers for enhanced drug delivery. Ann New York Acad Sci (2017) 1397(1):169–84. doi: 10.1111/nyas.13359
101. Bauer HC, Krizbai IA, Bauer H, Traweger A. "You Shall Not Pass"-tight junctions of the blood brain barrier. Front Neurosci (2014) 8:392. doi: 10.3389/fnins.2014.00392
102. Lopez-Ramirez MA, Wu D, Pryce G, Simpson JE, Reijerkerk A, King-Robson J, et al. MicroRNA-155 negatively affects blood-brain barrier function during neuroinflammation. FASEB J (2014) 28(6):2551–65. doi: 10.1096/fj.13-248880
103. Zhao X, Zeng H, Lei L, Tong X, Yang L, Yang Y, et al. Tight junctions and their regulation by non-coding RNAs. Int J Biol Sci (2021) 17(3):712–27. doi: 10.7150/ijbs.45885
104. Lazear HM, Daniels BP, Pinto AK, Huang AC, Vick SC, Doyle SE, et al. Interferon-λ restricts West Nile virus neuroinvasion by tightening the blood-brain barrier. Sci Trans Med (2015) 7(284):284ra59. doi: 10.1126/scitranslmed.aaa4304
105. Lv J, Hu W, Yang Z, Li T, Jiang S, Ma Z, et al. Focusing on claudin-5: A promising candidate in the regulation of BBB to treat ischemic stroke. Prog neurobiol (2018) 161:79–96. doi: 10.1016/j.pneurobio.2017.12.001
106. Li J, Zhao Y, Choi J, Ting KK, Coleman P, Chen J, et al. Targeting miR-27a/VE-cadherin interactions rescues cerebral cavernous malformations in mice. PLoS Biol (2020) 18(6):e3000734. doi: 10.1371/journal.pbio.3000734
107. Ting KK, Zhao Y, Shen W, Coleman P, Yam M, Chan-Ling T, et al. Therapeutic regulation of VE-cadherin with a novel oligonucleotide drug for diabetic eye complications using retinopathy mouse models. Diabetologia (2019) 62(2):322–34. doi: 10.1007/s00125-018-4770-4
108. Hsieh JT, Rathore APS, Soundarajan G, St John AL. Japanese encephalitis virus neuropenetrance is driven by mast cell chymase. Nat Commun (2019) 10(1):706. doi: 10.1038/s41467-019-08641-z
109. Zhu S, Pan W, Song X, Liu Y, Shao X, Tang Y, et al. The microRNA miR-23b suppresses IL-17-associated autoimmune inflammation by targeting TAB2, TAB3 and IKK-α. Nat Med (2012) 18(7):1077–86. doi: 10.1038/nm.2815
110. Fenoglio C, Ridolfi E, Cantoni C, De Riz M, Bonsi R, Serpente M, et al. Decreased circulating miRNA levels in patients with primary progressive multiple sclerosis. Multiple sclerosis (Houndmills Basingstoke England) (2013) 19(14):1938–42. doi: 10.1177/1352458513485654
111. Gioia U, Di Carlo V, Caramanica P, Toselli C, Cinquino A, Marchioni M, et al. Mir-23a and mir-125b regulate neural stem/progenitor cell proliferation by targeting Musashi1. RNA Biol (2014) 11(9):1105–12. doi: 10.4161/rna.35508
112. Lin ST, Huang Y, Zhang L, Heng MY, Ptácek LJ, Fu YH. MicroRNA-23a promotes myelination in the central nervous system. Proc Natl Acad Sci U S A (2013) 110(43):17468–73. doi: 10.1073/pnas.1317182110
113. Gradisnik L, Velnar T. Astrocytes in the central nervous system and their functions in health and disease: A review. World J Clin cases (2023) 11(15):3385–94. doi: 10.12998/wjcc.v11.i15.3385
114. Matthews PM. Chronic inflammation in multiple sclerosis - seeing what was always there. Nat Rev Neurol (2019) 15(10):582–93. doi: 10.1038/s41582-019-0240-y
115. Senatorov VV Jr., Friedman AR, Milikovsky DZ, Ofer J, Saar-Ashkenazy R, Charbash A, et al. Blood-brain barrier dysfunction in aging induces hyperactivation of TGFβ signaling and chronic yet reversible neural dysfunction. Sci Trans Med (2019) 11(521):eaaw8283. doi: 10.1126/scitranslmed.aaw8283
116. Lanz TV, Ding Z, Ho PP, Luo J, Agrawal AN, Srinagesh H, et al. Angiotensin II sustains brain inflammation in mice via TGF-beta. J Clin Invest (2010) 120(8):2782–94. doi: 10.1172/JCI41709
117. Shen W, Li S, Chung SH, Zhu L, Stayt J, Su T, et al. Tyrosine phosphorylation of VE-cadherin and claudin-5 is associated with TGF-β1-induced permeability of centrally derived vascular endothelium. Eur J Cell Biol (2011) 90(4):323–32. doi: 10.1016/j.ejcb.2010.10.013
118. Menard C, Pfau ML, Hodes GE, Kana V, Wang VX, Bouchard S, et al. Social stress induces neurovascular pathology promoting depression. Nat Neurosci (2017) 20(12):1752–60. doi: 10.1038/s41593-017-0010-3
119. Rinkenbaugh AL, Baldwin AS. The NF-κB pathway and cancer stem cells. Cells (2016) 5(2):16. doi: 10.3390/cells5020016
120. Rom S, Dykstra H, Zuluaga-Ramirez V, Reichenbach NL, Persidsky Y. miR-98 and let-7g* protect the blood-brain barrier under neuroinflammatory conditions. J Cereb Blood Flow Metab (2015) 35(12):1957–65. doi: 10.1038/jcbfm.2015.154
121. Sun P, Hamblin MH, Yin KJ. Non-coding RNAs in the regulation of blood-brain barrier functions in central nervous system disorders. Fluids barriers CNS (2022) 19(1):27. doi: 10.1186/s12987-022-00317-z
122. Duffy CP, McCoy CE. The role of microRNAs in repair processes in multiple sclerosis. Cells (2020) 9(7):1711. doi: 10.3390/cells9071711
123. Sindhwani S, Syed AM, Ngai J, Kingston BR, Maiorino L, Rothschild J, et al. The entry of nanoparticles into solid tumours. Nat materials (2020) 19(5):566–75. doi: 10.1038/s41563-019-0566-2
124. Morad G, Carman CV, Hagedorn EJ, Perlin JR, Zon LI, Mustafaoglu N, et al. Tumor-derived extracellular vesicles breach the intact blood-brain barrier via transcytosis. ACS nano. (2019) 13(12):13853–65. doi: 10.1021/acsnano.9b04397
125. Banks WA, Sharma P, Hansen KM, Ludwig N, Whiteside TL. Characteristics of exosomes and the vascular landscape regulate exosome sequestration by peripheral tissues and brain. Int J Mol Sci (2022) 23(20):12513. doi: 10.3390/ijms232012513
126. Usman WM, Pham TC, Kwok YY, Vu LT, Ma V, Peng B, et al. Efficient RNA drug delivery using red blood cell extracellular vesicles. Nat Commun (2018) 9(1):2359. doi: 10.1038/s41467-018-04791-8
127. Aslan C, Kiaie SH, Zolbanin NM, Lotfinejad P, Ramezani R, Kashanchi F, et al. Exosomes for mRNA delivery: a novel biotherapeutic strategy with hurdles and hope. BMC Biotechnol (2021) 21(1):20. doi: 10.1186/s12896-021-00683-w
128. Meira M, Sievers C, Hoffmann F, Rasenack M, Kuhle J, Derfuss T, et al. Unraveling natalizumab effects on deregulated miR-17 expression in CD4+ T cells of patients with relapsing-remitting multiple sclerosis. J Immunol Res (2014) 2014:897249. doi: 10.1155/2014/897249
129. Piket E, Zheleznyakova GY, Kular L, Jagodic M. Small non-coding RNAs as important players, biomarkers and therapeutic targets in multiple sclerosis: A comprehensive overview. J autoimmunity (2019) 101:17–25. doi: 10.1016/j.jaut.2019.04.002
130. Gebert LF, Rebhan MA, Crivelli SE, Denzler R, Stoffel M, Hall J. Miravirsen (SPC3649) can inhibit the biogenesis of miR-122. Nucleic Acids Res (2014) 42(1):609–21. doi: 10.1093/nar/gkt852
131. Zhao J, Lammers P, Torrance CJ, Bader AG. TP53-independent function of miR-34a via HDAC1 and p21(CIP1/WAF1.). Mol Ther (2013) 21(9):1678–86. doi: 10.1038/mt.2013.148
132. Bader AG. miR-34 - a microRNA replacement therapy is headed to the clinic. Front Genet (2012) 3:120. doi: 10.3389/fgene.2012.00120
133. Daige CL, Wiggins JF, Priddy L, Nelligan-Davis T, Zhao J, Brown D. Systemic delivery of a miR34a mimic as a potential therapeutic for liver cancer. Mol Cancer Ther (2014) 13(10):2352–60. doi: 10.1158/1535-7163.MCT-14-0209
134. Dong L, Wang X, Tan J, Li H, Qian W, Chen J, et al. Decreased expression of microRNA-21 correlates with the imbalance of Th17 and Treg cells in patients with rheumatoid arthritis. J Cell Mol Med (2014) 18(11):2213–24. doi: 10.1111/jcmm.12353
135. Gallant-Behm CL, Piper J, Dickinson BA, Dalby CM, Pestano LA, Jackson AL. A synthetic microRNA-92a inhibitor (MRG-110) accelerates angiogenesis and wound healing in diabetic and nondiabetic wounds. Wound Repair regeneration (2018) 26(4):311–23. doi: 10.1111/wrr.12660
136. Hanna J, Hossain GS, Kocerha J. The potential for microRNA therapeutics and clinical research. Front Genet (2019) 10:478. doi: 10.3389/fgene.2019.00478
137. Ghosh D, Nandi S, Bhattacharjee S. Combination therapy to checkmate Glioblastoma: clinical challenges and advances. Clin Trans Med (2018) 7(1):33. doi: 10.1186/s40169-018-0211-8
138. van Zandwijk N, Pavlakis N, Kao SC, Linton A, Boyer MJ, Clarke S, et al. Safety and activity of microRNA-loaded minicells in patients with recurrent Malignant pleural mesothelioma: a first-in-man, phase 1, open-label, dose-escalation study. Lancet Oncol (2017) 18(10):1386–96. doi: 10.1016/S1470-2045(17)30621-6
139. Reid G, Pel ME, Kirschner MB, Cheng YY, Mugridge N, Weiss J, et al. Restoring expression of miR-16: a novel approach to therapy for Malignant pleural mesothelioma. Ann Oncol (2013) 24(12):3128–35. doi: 10.1093/annonc/mdt412
Keywords: miRNAs, autoimmune, multiple sclerosis, exosomes, therapeutic advances
Citation: Emami Nejad A, Mostafavi Zadeh SM, Nickho H, Sadoogh Abbasian A, Forouzan A, Ahmadlou M, Nedaeinia R, Shaverdi S and Manian M (2023) The role of microRNAs involved in the disorder of blood–brain barrier in the pathogenesis of multiple sclerosis. Front. Immunol. 14:1281567. doi: 10.3389/fimmu.2023.1281567
Received: 22 August 2023; Accepted: 30 October 2023;
Published: 14 December 2023.
Edited by:
Kongyang Ma, Sun Yat-sen University, ChinaReviewed by:
Lixia Zhu, Huazhong University of Science and Technology, ChinaCopyright © 2023 Emami Nejad, Mostafavi Zadeh, Nickho, Sadoogh Abbasian, Forouzan, Ahmadlou, Nedaeinia, Shaverdi and Manian. This is an open-access article distributed under the terms of the Creative Commons Attribution License (CC BY). The use, distribution or reproduction in other forums is permitted, provided the original author(s) and the copyright owner(s) are credited and that the original publication in this journal is cited, in accordance with accepted academic practice. No use, distribution or reproduction is permitted which does not comply with these terms.
*Correspondence: Saham Shaverdi, c2FoYW0uc2hhaHZlcmRpQGdtYWlsLmNvbQ==; Mostafa Manian, TW9zdGFmYW1hbmlhbkBnbWFpbC5jb20=
Disclaimer: All claims expressed in this article are solely those of the authors and do not necessarily represent those of their affiliated organizations, or those of the publisher, the editors and the reviewers. Any product that may be evaluated in this article or claim that may be made by its manufacturer is not guaranteed or endorsed by the publisher.
Research integrity at Frontiers
Learn more about the work of our research integrity team to safeguard the quality of each article we publish.