- 1Department of Immunology, School of Medicine, Tehran University of Medical Sciences, Tehran, Iran
- 2Department of Immunology, School of Medicine, Iran University of Medical Sciences, Tehran, Iran
- 3Department of Immunology, School of Medicine, Shahid Beheshti University of Medical Sciences, Tehran, Iran
- 4Infectious Diseases Research Center, Birjand University of Medical Sciences, Birjand, Iran
- 5Department of Biochemistry, School of Medicine, Shiraz University of Medical Sciences, Shiraz, Iran
- 6Department of Immunology, Faculty of Medical Sciences, Tarbiat Modares University, Tehran, Iran
Malignancies contain a relatively small number of Mesenchymal stem/stromal cells (MSCs), constituting a crucial tumor microenvironment (TME) component. These cells comprise approximately 0.01–5% of the total TME cell population. MSC differentiation potential and their interaction with the tumor environment enable these cells to affect tumor cells’ growth, immune evasion, metastasis, drug resistance, and angiogenesis. This type of MSC, known as cancer-associated mesenchymal stem/stromal cells (CA-MSCs (interacts with tumor/non-tumor cells in the TME and affects their function by producing cytokines, chemokines, and various growth factors to facilitate tumor cell migration, survival, proliferation, and tumor progression. Considering that the effect of different cells on each other in the TME is a multi-faceted relationship, it is essential to discover the role of these relationships for targeting in tumor therapy. Due to the immunomodulatory role and the tissue repair characteristic of MSCs, these cells can help tumor growth from different aspects. CA-MSCs indirectly suppress antitumor immune response through several mechanisms, including decreasing dendritic cells (DCs) antigen presentation potential, disrupting natural killer (NK) cell differentiation, inducing immunoinhibitory subsets like tumor-associated macrophages (TAMs) and Treg cells, and immune checkpoint expression to reduce effector T cell antitumor responses. Therefore, if these cells can be targeted for treatment so that their population decreases, we can hope for the treatment and improvement of the tumor conditions. Also, various studies show that CA-MSCs in the TME can affect other vital aspects of a tumor, including cell proliferation, drug resistance, angiogenesis, and tumor cell invasion and metastasis. In this review article, we will discuss in detail some of the mechanisms by which CA-MSCs suppress the innate and adaptive immune systems and other mechanisms related to tumor progression.
1 Introduction
Tumors are formed by complex environmental components, including various cells such as fibroblasts, mesenchymal stromal/stem cells (MSCs), endothelial cells, immune cells, and factors involved in intercellular communication, including extracellular vesicles (EVs), cytokines, and extracellular matrix (1). These components together and coordinate with each other to form the tumor microenvironment (TME) that helps tumor growth. Also, these cells, in relation to the environment, affect the physicochemical conditions of the tumor site, including fibrosis, hypoxia, extracellular pH, and increased interstitial fluid pressure, and in this way, they contribute to tumor growth (2). Tumor-initiating cells (TICs), also known as cancer stem cells (CSCs) (3), are subpopulations of tumor cells in TME that can start tumors and trigger relapses (4, 5). It is believed that CSCs originate from differentiated cells that have undergone mutations or from stem cells that are resident in adult tissues (6–8). Several biomarkers are used to identify CSCs and have been correlated with diagnosis, therapy, and prognosis (9). Despite having specific biomarkers, CSCs are regarded as highly plastic, leading to changes in their phenotype and function over time due to this plasticity (6). It is known that CSCs are capable of forming their microenvironment in favor of tumor growth through the recruitment and activation of specific cell types, including MSCs, which are referred to as cancer-associated mesenchymal stromal/stem cells (CA-MSCs) (9, 10). MSCs usually exist in various mesenchymal tissues such as bone marrow, adipose tissue, cartilage, dental pulp, umbilical cord, and umbilical cord blood, and they can be isolated from these tissues (11). These cells have a high ability of self-renewal and differentiation and can differentiate into a variety of different types, including osteocytic, adipocytic, and chondrocytes. Also, due to the increased expression of chemokine receptors related to inflammation, these cells can migrate to the site of inflammation and induce their actions there. One type of chronic inflammatory site in the body of patients is tumor tissue. Therefore, MSCs can migrate to the tumor tissue and perform various actions there under the influence of the tumor environment (12). MSCs can play their role by producing multiple cytokines, growth factors, and extracellular vesicles, including exosomes (13, 14). MSCs and their exosomes play a role in the treatment of many diseases, such as orthopedic diseases (15), inflammatory diseases, infectious diseases (16, 17), etc. However, it seems that the presence of these cells (CA-MSCs) in tumor tissue can lead to tumor progression.
The CA-MSCs have the potential to modify the stroma and establish an optimal microenvironment for the restoration of CSCs and the progression of tumors. Crosstalk between cancer cells and MSCs within a microenvironment has also altered the CSC phenotype in different cancers. The current evidence suggests that the primary source of CA-MSCs employed by cancer cells derives from both distantly recruited MSCs and resident MSCs, a principal origin of the cells (6, 18, 19). It is typical for tumor and non-tumor cells in the TME to influence the phenotypical and functional transition of naive MSCs into CA-MSCs (20). Multiple mechanisms are involved in crosstalk between CSCs/cancer cells and CA-MSCs, including cell-to-cell interactions (21), secretion of exosomes (22), and paracrine secretion of inflammatory mediators, cytokines, and growth factors (23–25). Considering CA-MSCs and their mediators that play a unique role in the TME, more studies are required to elucidate how CA-MSCs/CSCs interact to overcome tumor immunity.
2 Regulation of immune responses
It has been observed that CA-MSCs interact with tumor cells and recruit various immune cells, especially macrophages and neutrophils, as well as myeloid-derived suppressor cells (MDSCs), then skew their phenotype in favor of tumor cells, immune response suppression, and tumor development, as it is summarized in Table 1.
2.1 Regulation of innate immune responses
2.1.1 Regulation of macrophage functions
Tumor-associated macrophages (TAMs) are divided into two distinct subsets, M1, stimulated by lipopolysaccharide (LPS) alone or combined with Th1 cytokines, and M2, activated by Th2-related cytokines. Producing tumor necrosis factor (TNF), reactive oxygen species (ROS), and facilitating antibody-dependent cellular cytotoxicity, M1-type macrophages have an anticancer function in the TME (52). While extracellular matrix (ECM) remodeling, tumor angiogenesis, immune suppression, and metastasis are all factors that M2-type macrophages use to promote tumor growth (53).
CA-MSCs can also switch macrophages from a pro-inflammatory M1 phenotype to an anti-inflammatory M2 phenotype, which enhances macrophage immunosuppressive effect. For instance, the results of a study showed lower production levels of pro-inflammatory cytokines, such as TNF-α, IP10 (IFN-γ inducible Protein 10kDa), RANTES, and macrophage inflammatory protein-1 alpha (MIP-1α), when macrophages were co-cultured with CA-MSCs derived from gastric cancer compared to normal MSCs (26). The result of in vitro studies have provided more detailed insight into the role of the interaction between MSCs and macrophages in the development of tumors and their metastasis. Researchers have discovered that CA-MSCs express high levels of CCR2, which binds to CCL2, CCL7, and CCL12 (34). CCR2 is also highly expressed in macrophages and regulates myeloid cell recruitment to tumor sites (35). After macrophages were specifically eradicated from melanoma, lymphoma, and breast cancer, Ren G et al. found that the tumor-promoting function of CA-MSC cells was also abolished once macrophages were specifically eliminated from these cancers (34). It is also accompanied by evidence that the tumor-promoting properties of CA-MSCs have been attenuated in CCR2-deficient mice (34, 54).
Additionally, Cascio S’s research on CA-MSCs in ovarian cancer has declared that these cells, which express CX3CL1, CCL2, and TGF-β, lead to the migration of CCR2+ monocytes and M2 TAMs to the TME and the complete elimination of responses to immune checkpoint inhibitor therapy (36). TNF-α and the TME hypoxic condition stimulate MSCs to express CCR2, followed by the production of CCL2, CCL7, and CCL12, and macrophage recruitment (34, 37). According to a comprehensive study on the role of MSCs and macrophages in breast cancer, a triple communication between tumor cells, macrophages, and MSCs contributed to the progression of breast cancer by interacting with chemokines and chemokine receptors. This interaction is conducted by two signaling loops regulated by the hypoxia-induced factor-1α (HIF-1α) (37). A signaling loop occurs when hypoxic CA-MSCs secrete CXCL10, which binds to the CXCR3 of hypoxic breast cancer cells and results in CXCL16 secretion (37). Upon binding to CXCR6 on hypoxic CA-MSCs, CXCL16 promotes the expression of CXCL10, resulting in further MSC recruitment to the tumor site due to more CXCL16 expression (37). Following this, another signaling loop is activated as well: CA-MSCs secrete CCL5, which binds to CCR5 on the surface of cancer cells and triggers the expression of the chemokine colony stimulating factor-1 (CSF-1) on those cancer cells, which then induces macrophages and MDSCs migration (37). Further investigation revealed that not only are CSF-1 and CCR5 targets of HIF-1α, but human samples confirmed that these loops exist as well (37).
2.1.2 Regulation of neutrophils functions
TANs, or tumor-associated neutrophils, phenotypically are diverse and have various functions (55, 56). These cells are an essential component of the TME. Neutrophils, like TAMs (M1 and M2), can be anti-tumorigenic (N1) or pro-tumorigenic (N2), depending on whether they have been activated by TGF-β (57, 58). However, unlike TAMs, the distinction between TAN N1 and N2 phenotypes is made by the activation level as opposed to by various polarizing chemicals (59). CA-MSCs also alter neutrophil functions in favor of tumor growth. For example, when CA-MSCs are stimulated by TNF-α in a mouse breast cancer model with the 4T1 cell line, lung metastasis is increased (38). This phenomenon can be attributed to increased neutrophil recruitment to tumor primary sites. CXCR2 ligands, including CXCL5, CXCL2, and CXCL1, produced by TNF-α activated MSCs account for the deposition of neutrophils at tumor primary sites and contribute to the metastatic microenvironment formation (38).
According to Zhu et al., gastric cancer-derived MSCs (GC-MSCs) interact in 2 directions with neutrophils (41). Firstly, by STAT3-ERK1/2 IL-6-mediated axis, GC-MSCs can upregulate the neutrophil’s activation and chemotaxis, resulting in their survival. The second function of activated TANs is facilitating the differentiation of MSCs into cancer-associated fibroblasts (CAFs) (39).
2.1.3 Regulation of natural killer cell functions
NK cells naturally respond to tumor cells (60, 61), but NK cells’ activity is determined by the engagement of their stimulatory or inhibitory receptor (62). The NK cell receptors include NK group 2D (NKG2D), DNAX accessory molecule 1 (DNAM-1), NKp46, NKp44, and NKp30, which serve as stimulatory receptors. In contrast, killer immunoglobulin-like receptors (KIRs) and CD94/NK group 2A (NKG2A) are inhibitory. TMEs in solid tumors contain different cells and soluble inhibitory factors, such as MSCs, which impair the function of NK cells infiltrating the tumor (63, 64). TGF-β is crucial in tumors’ CA-MSC and NK cell interactions. Evidence suggests that CA-MSCs secreted TGF-β inhibits IFN-γ production and NKG2D activation on cell surfaces. For example, through miR-183 activation, TGF-β inhibits DAP12 transcription and NKp30 and NKG2D expression, effectively suppressing NK cells (45). Additionally, TGF-β, after activating the SMAD2/3 pathway, downregulates NKG2D, DNAM-1, NKp30, and NKp46 expression in the in vitro condition (46).
2.1.4 Regulation of dendritic cells functions
Dendritic cells that infiltrated to tumor tissue (TiDCs), as a heterogeneous group of DCs, express a high level of MHC class I and class II complexes, costimulatory and adhesion-related molecules. These cells are essential for initiating and controlling innate and acquired (or adaptive) immune responses (43). According to recent research, CA-MSCs can suppress the processing and presentation of antigens by DCs and suppress the adaptive immune response (naïve T cell activation), which can help cancer cells evade the immune system (65). As a result of IL-6-mediated STAT3 pathway activation, normal DCs are recruited and transdifferentiated into regulatory DCs (rDCs), which have no antigen presentation but release suppressive mediators, including IDO (47). These mediators (such as IDO) limit T cell-mediated immunity by inducing T cell anergy and Treg cell proliferation (66).
2.1.5 Regulation of mast cells
Mast cells (MCs) are corporate in both tumor progression and suppression, depending on the cancer type, MCs localization, and degree of tumor progression (67–69). One aspect of MCs’ function as cancer promoters is to stimulate angiogenesis, lymphangiogenesis, and degradation of ECM by releasing a wide range of pro-angiogenic molecules [vascular endothelial growth factors (VEGFs), histamine, heparin, and stem cell factor (SCF)] (70–74), lymphangiogenic molecules (75), proteases, and matrix metalloproteinase-9 (MMP-9) (76–78). Meanwhile, MCs increase antitumor inflammation, induce tumor cell apoptosis, and reduce cancer cell invasion and metastasis due to their antitumor effector production, including TNF-α, chondroitin sulfate, tryptase, and IL-1 (69). CA-MSC immunosuppressive effects through MCs have received only minimal comprehensive research so far. Researchers recently found that MCs and CAFs contributed to the morphological transformation of benign epithelial cells into cancerous ones in a prostate cancer micro-tissue model (79).
2.2 Regulation of adaptive immune response
T lymphocytes, such as cytotoxic T lymphocytes (CTLs), Treg cells, and effector helper T (Th) cells, are the prominent soldiers of the immune system in driving and modulating adaptive immune responses (80). There is mounting evidence to support that CA-MSCs modulate T-cell activities and functions (Table 2).
2.2.1 Regulation of T lymphocytes
There are five major types of Th cells: Th22, Th17, Th9, Th2, and Th1 (95), all derived from naive T CD4+ cells. Th1 and Th2 cells, involved in cellular and humoral immunity, respectively, by releasing various particular cytokines (96). Different studies showed that MSCs can decrease the differentiation of naïve T cells into inflammatory subsets, including Th1 and Th17 (11). MSCs that are recruited to the tumor site have an essential role in modulating the responses of T cells. The results of studies show that CA-MSCs, by producing TGF-β, can lead to the induction of regulatory T cell differentiation from naïve T cells (97). Also, they increase the differentiation of IL10-producing Tr1 cells by producing prostaglandine E2 (PGE2) (98). This result was demonstrated in mice infected with Helicobacter pylori and receiving BM-MSCs 10 months after infection. It was shown that this treatment skews the immune response to an immunosuppressive state by inducing Tr1 cells and Treg cells, possibly contributing to an immune microenvironment that tolerates H. pylori-mediated gastric cancer progression. In vitro studies also suggested that Treg cells induced by TGF-β suppress the cytolytic potency of CD8+ T cells and NK cells against T47D breast cancer (99). Also, as mentioned in the previous sections, CA-MSCs can affect the TCD4+ cells’ function by affecting innate immune cells such as DCs and macrophages (100).
CTLs, or CD8+ T cells, mediate cytotoxic activities by inducing tumor cell apoptosis (101, 102). Several inflammatory cytokines secreted by effector T cells, such as IFN-γ and TNF-α, stimulate CA-MSCs to produce TGF-β, CXC chemokines such as CXCL10, CXCL9, and CXCL11, as well as the large amounts of inducing nitric oxide synthetase (iNOS) (103) and IDO (104). All of the mentioned substants suppress effector T lymphocytes in humans, including TCD4+ and CTLs. As Sandra Cascio demonstrates, CA-MSCs have a role by removing TCD8+ cells surrounding tumors and creating “immune tumor exclusions” that prevent TCD8+ cells from interacting with cancer cells, causing resistance to immune checkpoint inhibitor (ICIs) cancer therapy (36). It has been discovered in another study that the injection of B16 melanoma cells into allogeneic mice can result in tumor formation only when it is combined with BM-MSCs (105). In addition, CA-MSCs derived from cervical cancer patients were also shown to prevent antigen-specific CD8+ T lymphocytes from destroying CaSki cells in vitro, a human cervical cancer cell line (106).
2.2.2 Regulation of B lymphocyte functions
A tumor immune microenvironment also contains B cells that can act as potentiators or inhibitors of antitumor immunity and regulate cancer progression (107). A lot of study has been shown recently on how CA-MSCs affect T lymphocytes, but very few studies have looked at how MSCs affect B cells. However, there is evidence that BM-MSCs can cause B cells to enter the G0/G1 phase of the cell cycle and decrease B cell growth and antibody synthesis (108, 109). Despite these results, more study is required to comprehend the consequences of B cell and MSC interaction during tumor formation. According to current studies, CXCL13 is the only chemokine secreted by CAFs that enhances B cell recruitment (110). Therefore, the interaction of CA-MSCs and CAFs with B lymphocytes to suppress antitumor responses needs further investigation (Figure 1).
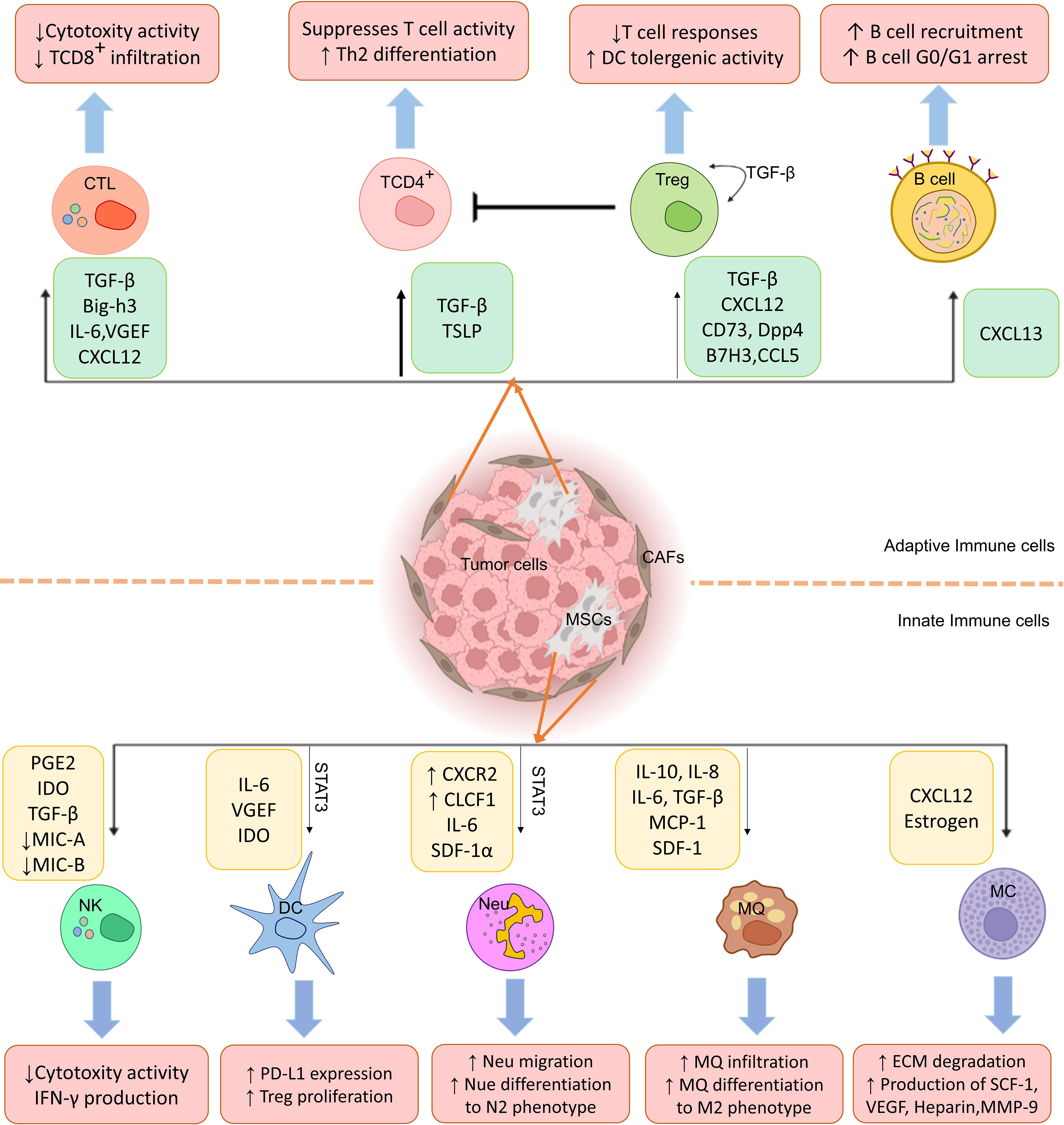
Figure 1 Cancer-associated mesenchymal stem cells (CA-MSCs) affect innate and adaptive immune system cells. As shown in the figure, MSCs disrupt immune system cells’ functions in the tumor environment by producing various mediators and leading immune responses to the expansion of M2 macrophages, regulatory T cells, and N2 neutrophils and suppression of CTL and NK cell responses.
3 Role of CA-MSCs in promoting cancer growth
In the last decade, many studies have been conducted that have shown that MSCs cause the growth and proliferation of tumor cells through the effect on signaling pathways (111). From another aspect, it has been shown that these cells can impede the growth of cancer cells (112), so this issue is being discussed. Interestingly, the anti-tumor or tumor growth-supporting effects of MSCs depend on the source and type of MSCs, so BM-MSCs and adipose tissue derived MSCs (AT-MSCs) have the capacity to promote tumor growth, but UCB-MSCs inhibit tumor growth (113, 114). MSCs increase the growth and malignancy of cancer cells in several different ways, including 1) production of cytokines and chemokines. 2) Phenotypic and metabolic characteristics of MSCs. 3) Immune cell modulation and immunosuppressive effects. 4) Effect on TME. 5) Effect on non-coding RNAs such as miRNAs. In the following, we briefly describe these factors. Studies supporting the function of MSCs to favor tumor growth are summarized in Table 3.
MSCs properties and functions differ based on the activated receptor type. One of the most important of these receptors is Toll-like receptors (TLRs). Among these TLRs, if the TLR-3 receptor is active on the MSC, it is called MSC2 (TLR3-primed MSCs) (140). These cells (MSC2) have the property of suppressing immune cells by secreting anti-inflammatory cytokines such as IL1-RA and IL10. Subsequently, it promotes cancer cells. On the other hand, in the presence and activity of TLR-4 receptors on MSCs, they are called MSC1 (TLR4-primed MSCs). These cells (MSC1) secreted pro-inflammatory and pro-apoptotic factors such as IL17, GM-CSF, and TRAIL. MSC1 reduces the proliferation and inhibits the invasion of tumor cells (141).
It’s interesting to note that the TLR agonist exposure influences MSC function and aids MSCs in switching between MSC1 and MSC2 (anti-tumor or tumor growth promoter). In addition, MSCs can stimulate the growth of cancer cells and angiogenesis in different ways. For example, in prostate and breast tumors, MSCs increased pro-angiogenic factors such as VEGF MIP-2, IL-6, and TGF-β. These factors directly induce the proliferation of tumor cells and angiogenesis and thus increase the growth rate of solid tumors in vitro and in vivo (134). In addition, in a study on hepatocellular carcinoma (HCC), researchers discovered that the mRNA level of TGF-β1 was significantly increased. However, in the MSC-treated group, Smad7 mRNA expression was suppressed. Their research shows that MSCs may promote growth and angiogenesis via the TGF-1/Smad pathway (142). By producing chemokines, CA-MSCs increase the development and cell proliferation in cancer cells; for example, increasing the expression of CCL5 chemokine by BM-MSCs increases proliferation, migration, metastasis, and malignant behaviors in cancer cells (132). It has been found that CA-MSC forms a niche of cancer stem cells, which increases the ability of proliferation in cancer cells (143).
Regarding lymphoblastic leukemia, it has been found that PGE2 produced from MSCs activates the cAMP-PKA signaling pathway in tumor stem cells and inhibits the cancer suppressor function of p53, thereby increasing leukemogenesis (120). Also, it has been shown when MSCs are exposed to TME-like mediated oxidative stress, they can produce lactate, which will be absorbed by cancer cells, and result in producing ATP that will increase the growth and migration of cancer cells (144). Under TME circumstances, MSCs have been seen to differentiate into CAFs, stimulating cancer cell heterogeneity and playing an essential role in cancer progression. Another factor secreted by MSCs that directly affects the growth of tumor cells is neuregulin 1 (NRG1). NRG1 controls cell proliferation and differentiation via binding to EGFRs (145). In vitro, it has been determined that NRG1 produced by BM-MSCs activates the HER2/HER3-dependent PI3K-AKT signaling pathway in CRC and increases cell growth (123).
Other studies have reported that direct cell-cell interaction and co-culture between human UC-MSCs and MDA-MB-231 breast cancer cells can significantly increase the proliferation of tumor cells in mouse models (146), but its molecular mechanisms were not investigated. In a study, it has been determined that hepatocyte growth factor (HGF) secreted from BM-MSCs increases the expression of glucose-6-phosphate dehydrogenase (G6PD) in gastric cells and, subsequently, by affecting the G6PD/NF-κB/HGF axis in gastric cancer cells, increases glycolysis, proliferation, and metastasis of gastric cancer by upregulating c-Myc/HK2 signaling pathway (127). It has been suggested that another supportive function in tumor growth by MSCs is to increase cancer stem cell frequencies. This increase in ovarian cancer cells is due to the increased expression of bone morphogenetic protein (BMP)2, BMP4, and BMP6 factors on MSCs (128). Another method MSCs perform to support the growth of tumor cells is mammosphere formation. It has been shown in a study that when CA-MSCs are co-cultured with breast cancer cells, CA-MSCs induce mammosphere formation in these cancer cells using the EGF/EGFR/Akt pathway. As a result, it increases the growth of cancer cells (129).
Another tumor proliferation-enhancing effect of CA-MSCs is attributed to their role in protecting cancer cells from the immune system through the modulation of regulatory T cells and inhibition of NK cells, macrophages, and CTL functions (97). It has been found that gastric cancer-associated MSCs, by secreting IL-6 and IL-8 cytokines, as well as the JAK2/STAT3 signaling pathway, have caused the polarization of macrophages towards M2 macrophages, which promotes tumor growth (26). CA-MSCs in syngeneic melanoma mice (B16F10), by blocking cysteine in DCs via IL-10-STAT3 signaling, inhibited the proliferation of naive T cells and thus increased the growth and development of melanoma cancer cells (65). Also, by producing TGF-β1, CA-MSCs induce Tregs cells, which suppress immune responses in the breast tumor microenvironment, increasing the proliferation of these cancer cells (97). It has been found that the proximity of CA-MSCs isolated from ovarian cancer with active immune cells and the presence of ICP inhibitors destroy the response to treatment. This effect is caused by CCL2, CX3CL1, and TGF-β1 expression from CA-MSCs and the recruitment of CCR2+ monocytes and M2 macrophages in the TME, inhibiting TCD8+ cells (36). IDO is another substance secreted from CA-MSCs that suppresses anti-tumor immune responses. It has been reported that the cells in the head and neck squamous tumor area have decreased the proliferation and functions of CD4+ and CD8+ T cells against the tumor and increased the growth and invasion of these cancer cells (117).
MSCs decrease the ability of NK cells to secret IFN-γ, thus weakening their anti-cancer role and causing cancer cell growth (115). In addition, MSCs reduce the maturation of DCs and other APCs through PGE2 signaling; thus, T cells cannot be activated, and cancer cells continue to grow (147). Co-culture of MSCs with CD11b/Ly6G-positive neutrophils results in extensive inhibition of T cells in vitro and enhances breast carcinoma growth in vivo (148). It has also been reported that MSCs present in gastric cancer, by producing IL-6 through the STAT3-ERK1/2 signaling pathway, promote neutrophils and their shift towards the supportive phenotype of cancer cells (119). As a result, CA-MSCs secrete immune cell suppressor molecules and chemokines such as ICAM-I, PD-L1, VCAM, HLA-G, COX-2, IDO, TGF-β, PGE2, CXCL11, CXCL8, CXCL9, CXCL6, CXCL10, CXCL1, and promote cancer cells viability and increase their growth.
According to growing research, non-coding RNAs are involved in carcinogenesis, metastasis, and treatment resistance (149). Researchers demonstrated that human UC-MSCs strongly stimulate the growth of lung adenocarcinoma (LUAD) cells in a xenograft tumor model by transfecting miR-410 (150). A mouse cancer model showed that gastric cancer-derived MSCs could significantly increase the migration and growth of HGC-27 through increased miR-221 expression, which may act as a new biomarker in stomach cancer increase (151). Another study found that MSCs accelerated the development of gastric cancer by secreting TGF-β1, which triggered the SMAD2/3 pathway and the lncRNA MACC1-AS1/miR-145-5p/fatty acid oxidation (FAO) axis in cancer cells (121). In addition, in triple-negative breast cancer, MSCs strongly induce the regulation of RNA LINC01133 in adjacent tumor cells; this induction increases the spread of cancer stem cell-like phenotypic features and strengthens cancer cell growth (152). Also, it has been determined that in HCC-associated MSCs, a high expression level of lncRNA-MUF is observed; this lncRNA binds with Annexin A2 (ANXA2) and activates Wnt/b-catenin and causes their signaling and overexpression of miR-34a increases hence hepatocarcinogenesis (122). Microarray studies have shown that the expression of S100A4 on CA-MSCs is increased in liver cancer, and it has been determined that this molecule increases the growth of liver cancer cells by increasing the expression of mir-155 and finally by activating the STAT3 pathway and becomes proliferation of these cancer cells (124). In a study, it has been reported that when BM-MSCs enter the glioblastoma tumor environment, they become CA-MSCs, secrete mir-1587 into their exosomes in a specialized manner, and are absorbed by their cancer cells. mir-1587 reduces the level of NCOR1 in cancer cells and thus increases growth and proliferation in these cells (125). It has also been found that increasing the expression of DNM3OS lncRNA in CA-MSCs through the DNM3OS/KDM6B/TIAM1 pathway and interaction increases invasion, growth, and metastasis in hepatocarcinoma cancer cells (126). In breast cancer, it has been found that MSCs inhibit FoxP2 by increasing the expression of mir-214 and mir-199 in these cancer cells, thus increasing the growth, metastasis, and staying in the phenotypic state of cancer stem cells (153). Researchers have reported that high levels of miR-222 and miR-30a were found in the hCC-MSC secreted exosomes. These miRNAs, by targeting and reducing the expression of MIA3, increase the stimulation of growth, proliferation, and metastasis of colon cancer cells (130). On the other hand, it has been found that the increased expression of miR 221 in the exosomes obtained from CA-MCS cells of gastric cancer has increased the power of migration and tumorigenesis in these cancer cells (154). Additionally, it has been demonstrated that miR-142-3p, which is highly expressed in exosomes made from BM-MSCs, stimulates the Notch signaling pathway by decreasing the expression of Numb in cancer cells, which promotes the development and expansion of colon cancer stem cells (131).
In addition, CA-MSCs are able to differentiate into other tumor growth-supporting lineages. It has been shown that the treatment of BM-MSC with PC-3 prostate cancer cells supernatant increases their differentiation into the osteoblastic cell lineage. This process is done through the secretion of FGF-9 by PC-3 cells with the positive regulation of pro-osteoblastic factors, including fibronectin, integrin α5/β1, and osteoprotegerin compared to control environments, then support the growth of cancer cells (155). Finally, we can say that the mechanism of CA-MSCs is probably causing tumor cell proliferation by different factors, including angiogenic factors (βFGF, HIF-1α, and VEGF) (152); Chemokines (CCL5, CCL2, CXCL12, and CCL22) (156); growth factors (PDGF, SCF, HGF, IGF-1E, and GF) (157) and inflammatory cytokines (TGF-β, TNFα, IL-8, and IL-1β).
4 The role of CA-MSCs in tumor metastasis and invasion
As cancer cells alter their morphology, getting metastatic and invasive, they detach from the primary site and localize into a secondary organ far from the origin during metastasis (158). The interactions between cancer cells and other existing cells in the TME are necessary to generate a metastatic TME. One of these cells is CA-MSC, which migrates to tumor sites during inflammation, like the incidents during wound healing (159). The interaction between tumor cells and MSCs can be bidirectional. It has been demonstrated that carcinoma cells-derived IL-1 stimulates the production of PGE2, which in turn, in an autocrine manner with the cooperation of IL-1, induces the release of cytokines like IL-8 and IL-6 by MSCs, leading to the activation of Wnt/β-catenin signaling and stemness properties of cancer cells to enable tumor progression (25). In a model of breast cancer, it has been detected that cancer cells can stimulate the recruitment of BM-MSCs to tumor sites through the SDF-1α/CXCR4 axis. In turn, breast cancer cells metastasize to the bone marrow via SDF-1α, which belongs to the chemokine family and is known as a chemo-attractant mediator (160). In another study, it has been reported that the incubation of breast cancer cells with MSCs-derived exosomes elevates the migratory potential of cancer cells by inducing Wnt/β-catenin signaling. Indeed, the expression of Wnt/β-catenin targeted genes such as Axin2 and Dkk1 increases in exosome-treated cancer cells (161).
The active molecules produced by MSCs can contribute to generating an appropriate microenvironment for tumor metastasis. The role of IL-6 and IL-8 secreted by MSCs as inflammatory chemokines on tumor progression has been demonstrated in some cancer models (162, 163). Some tumor model studies have shown the role of inflammatory mediators derived from MSCs like CXCL1, CXCL2, or CXCL12 on metastatic and invasive properties of cancer cells through activating their specific receptors like CXCR2 and CXCR4 (164, 165). The MSCs-derived extrinsic factors can also induce the proliferation of cancer cells, potentially leading to distant metastasis. It is described that the released CXCL12 and IGF1 produced by MSCs activate the PI3K/AKT signaling pathway in breast cancer cells and then shift the cancer cell population towards more bone metastatic ones (166). The tumor-derived factors can induce the secretion of inflammatory chemokine and cytokines from CA-MSCs. For instance, it is revealed that osteopontin as a tumor-derived inflammatory cytokine promotes CA-MSCs to the secret high level of CCL5, which binds to its receptor (CCR5) on cancer cells to enhance cancer cells’ metastatic potential and MSCs’ ability to migrate to metastasis site (132, 167, 168). In prostate cancer, it is shown that CCL5-derived from MSCs and cancer cells, through suppressing the nuclear translocation of androgen receptors, increases the metastatic potential of cancer cells due to inhibiting androgen receptor signaling (169). It is indicated that blocking these receptors with neutralizing antibodies can repress tumor metastasis in a mouse model of breast cancer (170). Indeed, it is identified that CA-MSCs secret high levels of asporin into tumor stroma, promoting metastatic tumor development and restricting MSCs differentiation through binding to BMP-4 (171).
Furthermore, in a model of breast cancer, it is documented that MSCs induce the metastases and invasive behavior of breast tumors by altering the cancer cells gene expression profiles by upregulating the oncogenic pathways like Wnt and TGF-β and thereby, enhancing the expression of genes related to the cell membrane and matrix-associated proteins (172). Another study showed that MSCs, via upregulating the miR-199 and miR-214, inhibit the expression of the FOXP2 transcription factor in cancer cells to stimulate breast cancer metastasis (153). Moreover, in human MSCs-treated hepatocellular tissues, TNF-α, IL-6, and α5 integrin expression were elevated to promote tumor growth and metastasis in HCC (173). Enhancement in the migratory and invasive ability of glioblastoma cells has also been correlated to TGF-β secreted by MSCs (174). TGF-β, as a growth factor, is implicated in generating invasive and metastatic properties of cancer cells by inducing epithelial-to-mesenchymal transition (EMT) (175). In a co-cultured model of breast cancer cells with MSCs, it has been reported that the migratory capacity of cancer cells increases through the ER-SDF-1/CXCR4 pathway (164). In another study, the role of BM-MSCs in activating the migratory capacity of breast cancer cells has been attributed to the CXCR2 receptor (165). In a mice model of gastric cancer, CA-MSCs enhances the survival and migration of cancer cells by upregulating the expression miR-221 (151). It has been documented that MSCs could transmit mitochondria to glioblastoma stem cells and breast cancer cells to enhance the proliferative and invasive potency of cancer cells through increasing oxidative phosphorylation (OXPHOS) and adenosine triphosphate (ATP) production (176–178).
Several studies declare the crucial role of MSCs in EMT, a process by which cancer cells obtain the stem cell phenotype to migrate to other sites and metastasis. A study on pancreatic cancer has indicated that human BM-MSCs promote EMT and cancer-initiating stem cell-like characteristics in cancer cells via the Notch signaling pathway (179). AT-MSCs have been verified to upregulate the expression of EMT-related genes in invasive breast cancer cells through TGF-β and expressing BMP (180).
Some studies describe the role of MSCs in accelerating the metastasis of tumors by altering the expression of enzymes related to metastasis. It has been shown that Lysyl oxidase is upregulated in breast cancer by recruiting MSCs to modulate breast cancer invasion, metastasis, and EMT (181). Moreover, exosomes released from AT-MSCs have been stated to enhance the expression of homosapien collagen beta galactosyl transferase 2 (COLGALT2), which is a crucial enzyme for collagen glycosylation, to promote metastasis and tumor proliferation in osteosarcoma cells (182). Also, it has been demonstrated that MSCs-derived exosomes enhance the tumorigenic features of ovarian and breast cancer cells through upregulating MMP-2 and MSCs-related markers such as CD73 and CD90 (183). MMPs are crucial for the degradation of ECM (184), and MSCs, by secreting MMPs, play an important role in inducing a pro-metastatic environment (185). Some MSCs-secreted factors, such as TGF-β, can also stimulate tumor fibrosis (186), which can cause the retention of chemokines and growth factors in the fibrotic environment to accelerate metastatic growth (187). In addition, MSCs can cooperate in downregulating or degrading E-cadherin in tumor cells; E-cadherin acts as an adhesion protein to inhibit cancer cell dissociation (188).
The role of MSCs in preparing pre-metastatic sites for circulating cancer cells is shown in some evidence (189–191). It is determined that CA-MSCs express receptors for VEGF, which not only enhance the migration of MSCs to tumor site (192) but also, with high levels of CXCL12 and deposited fibronectin, increases the migration and adherence of lung carcinoma and melanoma cells to pre-metastatic niche (193). Cancer-educated BM-MSCs, as a TME component, induce lung cancer cells’ survival at primary and metastatic sites by extending BM-PMN-MDSCs during the metastasis (194).
By contrast, the inverse role of MSCs on tumors has also been reported in various studies (195–197). In an HCC model, human MSCs suppressed the metastatic potential of cancer cells via downregulating TGF-β despite enhancing tumor growth (198). Recently, it has been indicated that MSCs-derived exosomes convey miR-3940-5p, which reduces the metastasis of colorectal cancer cells by targeting the α6 integrin family and then inactivating TGF-β1 (199).
The recruited MSCs in the TME can get the CAFs-phenotype to contribute to TME in cancer progression and chemoresistance (200). The initial in vitro evidence in this field has found that treatment of BM-MSCs with conditioned media of human breast cancer, pancreatic cancer, and glioma lead to the differentiation and expression of CAFs markers like α-SMA, fibroblast associated protein (FAP), fibroblast-specific protein 1 (FSP1) and vimentin (201). The CA-MSCs express lower amounts of vimentin and FSP1 in comparison with CAFs (202). The excessive capacity of CA-MSCs to differentiate into CAFs versus typical MSCs has been demonstrated in multiple studies (12, 203). An animal model of inflammation-promoted gastric cancer has discovered that more than 20% of CAFs originate from BM-MSCs, which can amplify during tumor progression and induce the probability of malignancy (202). Numerous secreted factors to the TME trigger the signaling pathways to stimulate the CA-MSCs differentiation to CAFs. It is indicated that the secreted factors by tumors activate the TGF-β/Smad signaling pathways in CA-MSCs to facilitate their differentiation to the CAF phenotype (204, 205). In gastric cancer, it is observed that the migrated MSCs to the inflammation site of tumor stroma generate CAFs through activating TGF-β and SDF-1α pathways by TGF-β and CXCL12 isolated from cancer cells and the TME (202) or by tumor derived exosomes (206). It seems that TGF-β plays a significant role in attracting and recruiting MSCs to tumor stroma.
On the other hand, TGF-β secreted by cancer cells and tumor stroma is crucial in the transition of MSCs into CAFs-like cells, which in turn promotes tumor progression (204). Monitoring the fate of MSCs during tumor progression would help better distinguish the heterogeneity of MSCs in the TME and consider its role in therapeutic strategies. Additionally, MSCs are involved in the progression of cancer cells by releasing active molecules such as SDF-1, CCL5/CCR5, CCR2, TNF-α, TGF-β, etc. (Table 4). Therefore, novel therapeutic approaches are needed to suppress or mediate the interaction between cancer cells and MSCs.
5 The role of CA-MSCs in tumor chemoresistance
CA-MSCs are one of the crucial actors in chemotherapy resistance, and they point to potential targets to improve patients’ response to chemotherapy. According to the research of Jeanine M.L. Rood Hart, endogenous MSCs become activated when treated with platinum analogs and release substances that protect tumor cells from a variety of chemotherapeutic agents. Two different polyunsaturated fatty acids caused by platinum (PIFAs), 12-oxo-5,8,10 hepta decatrienoic acid (KHT) and hexadeca 4,7,10,13 tetraenoic acid [16:4(n3)] were discovered using a metabolomics approach. These PIFAs are minute amounts that can result in resistance to various chemotherapeutic drugs. Surprisingly, MSC-induced resistance can be prevented by inhibiting the key enzymes [thromboxane synthase and cyclooxygenase-1(COX-1)] that produce these PIFAs (209). The study examined this possibility to understand the underlying molecular mechanism and determine if exosomes produced from MSCs mediate gastric cancer chemotherapy resistance. They discovered that MSCs-derived exosomes dramatically induced 5-fluorouracil (5-FU) resistance to gastric cancer cells. MSCs-derived exosomes increased the expression of multi-drug resistance-related proteins, such as MDR, MRP, and LRP, and inhibited the 5-FU-induced apoptosis. In gastric cancer cells, MSCs derived exosomes functioned mechanistically to activate the Raf/MEK/ERK kinase cascade and calcium/calmodulin-dependent protein kinases (CaM-Ks). The boosting function of MSCs-derived exosomes in chemoresistance was decreased by hampering the CaM-Ks/Raf/MEK/ERK pathway. Inducing drug resistance in gastric cancer cells through activating the CaM-Ks/Raf/MEK/ERK pathway is one potential effect of MSCs-derived exosomes. Chemotherapy for gastric cancer may be more effective if it focuses on how MSCs-derived exosomes interact with cancer cells (210).
HCC has an important trait of inflammation. A few inflammatory cytokines released in the TME alter how MSCs operate. In both in vivo and in vitro settings, they noticed that MSCs pretreated with the cocktail of IFN-γ and TNF-α promoted resistance to treatment in HCC cell lines. HCC cell line cells experienced autophagy after exposure to MSCs pre-treated with IFN-γ and TNF-α. The HCC cells use this process as a defensive mechanism to tolerate the cell toxicity of chemotherapy medicines. Treatment of the HCC cells with an autophagy inhibitor successfully decreased the MSCs-induced resistance to chemotherapy in these cells. TNF-α and IFN-γ-induced stimulation in MSCs induced TGF-β expressions (211). Another pathway by which CA-MSCs can cause drug resistance in tumor cells is the WNT signaling pathway (212). Studies show that the co-culture of acute lymphoblastic leukemia (ALL) cells and MSCs can lead to the reduction of apoptosis caused by cytarabine in tumor cells (213). The use of Wnt signaling inhibitor agent increased the sensitivity of ALL cells to chemotherapy and compensated for the inhibition of apoptosis induced by MSCs (213). In addition, it has been shown that CA-MSCs can activate the expression level of sphingosine-1-phosphate receptor 1 (S1PR1) in neuroblastoma-related tumor cells and the downstream signaling pathway that functions through JAK2 and STAT3 (214). S1PR1 overexpression protects cancer cells from chemotherapy-induced apoptosis by activating JAK-STAT3 signaling (215). Co-culture of MSCs by oral squamous cell carcinoma (OSCC) also leads to the drug resistance of cancer cells to cisplatin through the activation of the signaling pathway related to PDGFR-α/AKT (216, 217). Studies conducted by Bing Tu et al. show that the co-culture of MSCs with osteosarcoma cells leads to increased resistance to doxorubicin or cisplatin in tumor cells through a STAT3-dependent pathway (218). It has also been determined that pretreatment of MSCs with IL-6 increases their ability to induce STAT3 signaling pathway and induce drug resistance (218).
Another way MSCs contribute to the drug resistance of tumor cells is the acceptance of damaged mitochondria from tumor cells by tunneling nanotubules (TNTs) (219). Inhibiting the formation of TNTs by cytochalasin D leads to a decrease in the transfer of damaged mitochondria from tumor cells to MSCs and leads to a reduction in cancer cells’ drug resistance (178, 220). It also seems that MSCs can transfer healthy mitochondria to tumor cells through these TNTs and induce drug resistance in them.
Aldehyde dehydrogenase (ALDH) activity has a direct relationship with the drug resistance of cancer cells (221). Different studies have shown that MSCs increase the ALDH activity in cancer cells by the production of TGF-β through the p38-dependent signaling pathway. Inhibiting or reducing the production of TGF-β from MSCs decreases their ability to induce drug resistance in tumor cells (222).
Glioma-associated MSCs consist of two distinguishable populations characterized by the level of CD90 expression (223). CA-MSCs that express CD90 at a high level play a role in the proliferation, differentiation, adhesion, and migration of glioma tumor cells and have no effect on drug resistance induced in tumor cells. However, CA-MSCs CD90low are of great importance in the drug resistance of tumor cells. These cells, by increasing the expression of FOXS1 in glioma cells, lead to a decrease in the sensitivity of tumor cells to the drug temozolomide and apoptosis in these cells (224).
7 The role of CA-MSCs in tumor angiogenesis
Carcinoma-associated mesenchymal stromal/stem cells in the TME can affect the progression of ovarian tumors by promoting angiogenesis at the tumor site, which speeds up tumor growth. It has been shown that endothelial cells, CA-MSCs, and ovarian adenocarcinoma cells secrete pro-angiogenic cytokines, including IL-6, IL-8, and VEGF, at higher levels when they are in contact with a particular cell type (225). CA-MSCscan also induces macrophage differentiation to an M2 phenotype and activates them, causing them to release a large amount of pro-angiogenic cytokines that are helpful for the advancement of all related ovarian cancer cells that have been studied (226). According to research by W-H Huang et al., the angiogenesis and tumor growth rate are both accelerated when different CRCs are combined with non-tumorigenic MSCs. IL-6 released from MSCs stimulates the release of endothelin-1 (ET-1) in cancer cells, which in turn triggers the activation of Akt and ERK in endothelial cells, improving their ability to attract other cells to the tumor and promoting angiogenesis (227). An anti-IL-6 antibody or lentiviral-mediated RNAi against IL-6 in MSCs, the inhibition or knockdown of ET-1 in cancer cells, or the suppression of ERK and Akt in host endothelium cells can all be used to target the IL-6/ET-1/Akt or ERK pathway of tumor-stroma interaction. These show that efforts to stop the interaction between MSCs and cancer cells aid in preventing angiogenesis and reducing tumor growth. These findings indicate that targeting the interaction between the proangiogenic factors secreted by cancer cells and the tumor microenvironment, specifically the IL-6 released by MSCs, may result in novel therapeutic and preventive approaches (225).
MSCs transplanted into mice support tumor angiogenesis in vivo through the expression and production of VEGF and lead to an increase in the density of CD31+ vessels after MSC transfer. The use of siRNAs that interfere with VEGF expression reduces the ability of MSCs to induce angiogenesis at the tumor site (228). It has also been shown that MSCs bind to blood vessel endothelial cells after intratumoral injection and express procytic markers such as αSMA, NG2, and PDGFRβ. Pericytes promote tumor growth and tumor-related angiogenesis through the production of various proangiogenic soluble factors (229).
7 Conclusion and future perspective
As mentioned in this article, intercellular communication in TME can affect most aspects of tumor development (Figure 2). One of the most important cells that affect tumor cells is MSCs, which can also differentiate into fibroblasts (CAFs). Due to their characteristics, these cells can increase the expansion and production of cancer stem cells and help the stability of the tumor.
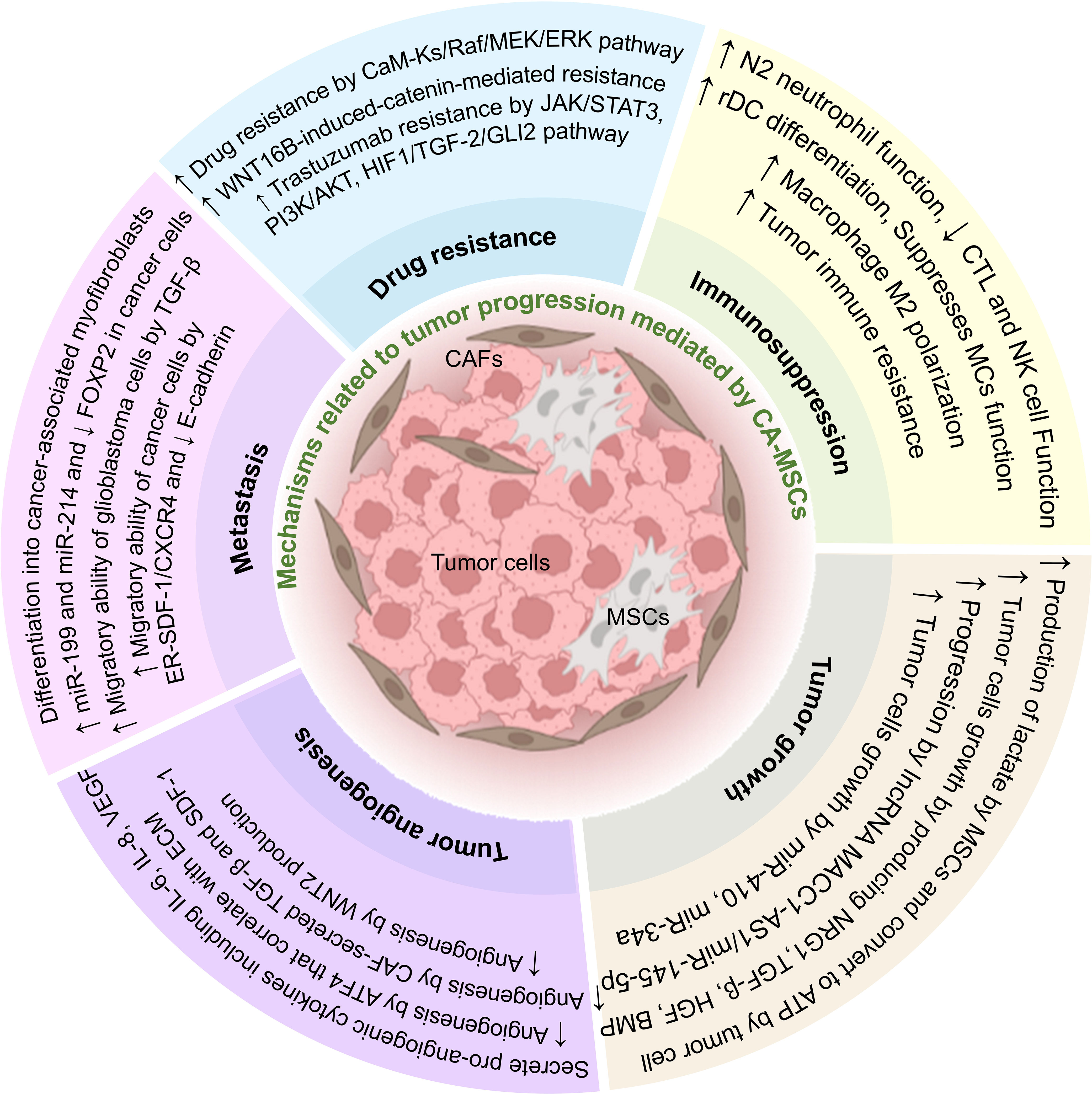
Figure 2 The effect of CA-MSCs on tumor progression. CA-MSCs contribute to tumor growth by affecting the main mechanisms of tumor expansion, including development, angiogenesis, invasion, metastasis, and drug resistance. Also, these cells contribute to the expansion of the tumor by suppressing the responses related to the immune system in the tumor microenvironment.
Also, CA-MSCs can contribute to tumor progression by suppressing the immune system, increasing metastasis, invasion, angiogenesis, tumor growth, and survival of tumor cells, and also by increasing drug resistance in tumor cells. In addition, MSCs can differentiate in the tumor environment and change the conditions of the TME in favor of the tumor. Intercellular communication in TME is very complex, and CA-MSCs usually exert their effect on tumor cells by using three mechanisms, including producing extracellular vesicles, soluble mediators, and direct cell-to-cell contact. According to the mentioned studies in this article, it seems that CA-MSCs are one of the main culprits of tumor expansion. So, it can be concluded that preventing the spread of CA-MSCs can also prevent the spread of tumors. It is very important to know this point because cancer stem cells are troublesome in many cancers and can lead to tumor recurrence after treatment. Also, preventing the spread of CA-MSCs can partially prevent metastasis, invasion, and drug resistance induced by these cells in tumor treatment.
On the other hand, inhibiting the expansion and function of CA-MSCs can lead to removing the suppression of the immune system against tumor cells and decrease the tumor’s power in immune evasion so that functional immune responses against tumor expansion are formed. As mentioned, MSCs, by expressing chemokine receptors related to migration to the site of inflammation, in clinical uses, they may migrate to the site of chronic inflammation induced by the tumor. According to the authors, they suggest the use of engineered MSCs that do not express these chemokine receptors (230). However, the lack of chemokine receptor expression can affect MSCs’ therapeutic potential and their migration to the target site. This issue can be solved through local injection of MSCs. Until now (September 2023), this approach has not been used in any of the pre-clinical or clinical studies. For this reason, the authors of this article, considering the importance of the presence of CA-MSCs in the tumor tissue and their role, encourage researchers to investigate this matter. However, as mentioned, TME is very complex, and proving the role and therapeutic importance of removing or preventing the spread of CA-MSCs requires more studies.
Author contributions
AH: Conceptualization, Methodology, Supervision, Visualization, Writing – original draft, Writing – review & editing. KM: Investigation, Writing – original draft, Writing – review & editing. ZM: Writing – original draft, Writing – review & editing. AK: Writing – original draft, Writing – review & editing. NR: Writing – original draft, Writing – review & editing. NH: Writing – original draft, Writing – review & editing. AA: Writing – original draft, Writing – review & editing. SS: Conceptualization, Supervision, Writing – review & editing.
Funding
The author(s) declare that no financial support was received for the research, authorship, and/or publication of this article.
Conflict of interest
The authors declare that the research was conducted in the absence of any commercial or financial relationships that could be construed as a potential conflict of interest.
Publisher’s note
All claims expressed in this article are solely those of the authors and do not necessarily represent those of their affiliated organizations, or those of the publisher, the editors and the reviewers. Any product that may be evaluated in this article, or claim that may be made by its manufacturer, is not guaranteed or endorsed by the publisher.
Glossary
References
1. Wei R, Liu S, Zhang S, Min L, Zhu S. Cellular and extracellular components in tumor microenvironment and their application in early diagnosis of cancers. Analytical Cell Pathol (2020) 2020. doi: 10.1155/2020/6283796
2. Quail DF, Joyce JA. Microenvironmental regulation of tumor progression and metastasis. Nat Med (2013) 19(11):1423–37. doi: 10.1038/nm.3394
3. Nokhodchi A, Chavan S, Ghafourian TJP. In vitro dissolution and Permeability Testing of Inhalation Products: Challenges and Advances, Vol. 15. (2023). p. 983.
4. Capp J-P. Cancer stem cells: From historical roots to a new perspective. J Oncol (2019) 2019:. doi: 10.1155/2019/5189232
5. Spillane JB, Henderson MA. Cancer stem cells: a review. ANZ J Surg (2007) 77(6):464–8. doi: 10.1111/j.1445-2197.2007.04096.x
6. Hanahan D. and R.A.J.c. Weinberg. Hallmarks cancer: next generation. (2011) 144(5):646–74. doi: 10.1016/j.cell.2011.02.013
8. Blackadar CB. Historical review of the causes of cancer. WJCO (2016) 7(1):54. doi: 10.5306/wjco.v7.i1.54
9. Walcher L, Kistenmacher A-K, Suo H, Kitte R, Dluczek S, Strauß A, et al. Cancer stem cells—origins and biomarkers: perspectives for targeted personalized therapies, Vol. 11. (2020). p. 1280.
10. Shi Y, Du L, Lin L, Wang Y. Tumour-associated mesenchymal stem/stromal cells: emerging therapeutic targets, Vol. 16. (2017). pp. 35–52.
11. Hazrati A, Malekpour K, Soudi S, Hashemi SM. Mesenchymal stromal/stem cells and their extracellular vesicles application in acute and chronic inflammatory liver diseases: emphasizing on the anti-fibrotic and immunomodulatory mechanisms. Front Immunol (2022) 13:865888. doi: 10.3389/fimmu.2022.865888
12. Ridge SM, Sullivan FJ, Glynn SA. Mesenchymal stem cells: key players in cancer progression. Mol Cancer (2017) 16(1):1–10. doi: 10.1186/s12943-017-0597-8
13. Hazrati A, Soudi S, Hashemi SM. Wharton's jelly mesenchymal stem cells-derived exosomes and imipenem in combination reduce apoptosis and inflammatory responses in E. Coli-infected HepG2 cells. Iranian J Allergy Asthma Immunol (2022) 21(3):273–86. doi: 10.18502/ijaai.v21i3.9801
14. Malekpour K, Hazrati A, Soudi S, Roshangar L, Pourfathollah AA, Ahmadi M. Combinational administration of mesenchymal stem cell-derived exosomes and metformin reduces inflammatory responses in an in vitro model of insulin resistance in HepG2 cells. Heliyon (2023) 9(5). doi: 10.1016/j.heliyon.2023.e15489
15. Malekpour K, Hazrati A, Zahar M, Markov A, Zekiy AO, Navashenaq JG, et al. The potential use of mesenchymal stem cells and their derived exosomes for orthopedic diseases treatment. Stem Cell Rev Rep (2022) 18(3):933–51. doi: 10.1007/s12015-021-10185-z
16. Chenari A, Hazrati A, Hosseini AZ, Motiee M, Soudi S. The effect of mesenchymal stem cell-derived supernatant nasal administration on lung inflammation and immune response in BCG-vaccinated BALB/c mice. Life Sci (2023) 317:121465. doi: 10.1016/j.lfs.2023.121465
17. Motallebnezhad M, Omraninava M, Ghaleh HEG, Jonaidi-Jafari N, Hazrati A, Malekpour K, et al. Potential therapeutic applications of extracellular vesicles in the immunopathogenesis of COVID-19. Pathology-Research Pract (2022) p:154280. doi: 10.1016/j.prp.2022.154280
18. Uccelli A, Moretta L, Pistoia V. Mesenchymal stem cells in health and disease. Nat Rev Immunol (2008) 8(9):726–36. doi: 10.1038/nri2395
19. Waite KA, Eng C. From developmental disorder to heritable cancer: it's all in the BMP/TGF-β family. N.R.G (2003) 4(10):763–73. doi: 10.1038/nrg1178
20. Kudo-Saito C. Cancer-associated mesenchymal stem cells aggravate tumor progression, Vol. 3. (2015). p. 23.
21. Ye J, Wu D, Wu P, Chen Z, Huang JJTB. The cancer stem cell niche: cross talk between cancer stem cells and their microenvironment. Tumor Biol (2014) 35:3945–51. doi: 10.1007/s13277-013-1561-x
22. Lindoso RS, Collino F, Camussi G. Extracellular vesicles derived from renal cancer stem cells induce a pro-tumorigenic phenotype in mesenchymal stromal cells. Oncotarget (2015) 6(10):7959. doi: 10.18632/oncotarget.3503
23. Nishimura K, Semba S, Aoyagi K, Sasaki H, Yokozaki HJP. Mesenchymal stem cells provide an advantageous tumor microenvironment for the restoration of cancer stem cells. Pathobiology (2012) 79(6):290–306. doi: 10.1159/000337296
24. Alcolea S, Antón R, Camacho M, Soler M, Alfranca A, Avilés-Jurado F-X, et al. Interaction between head and neck squamous cell carcinoma cells and fibroblasts in the biosynthesis of PGE2. J Lipid Res (2012) 53(4):630–42. doi: 10.1194/jlr.M019695
25. Li H-J, Reinhardt F, Herschman HR, Weinberg RAJCd. Cancer-stimulated mesenchymal stem cells create a carcinoma stem cell niche via prostaglandin E2 signalingPGE2 and the MSC-derived cancer stem cell niche. Cancer Discov (2012) 2(9):840–55. doi: 10.1158/2159-8290.CD-12-0101
26. Li W, Zhang X, Wu F, Zhou Y, Bao Z, Li H, et al. Gastric cancer-derived mesenchymal stromal cells trigger M2 macrophage polarization that promotes metastasis and EMT in gastric cancer. Cell Death Dis (2019) 10(12):918. doi: 10.1038/s41419-019-2131-y
27. Nagarsheth N, Wicha MS, Zou W. Chemokines in the cancer microenvironment and their relevance in cancer immunotherapy. Nat Rev Immunol (2017) 17(9):559–72. doi: 10.1038/nri.2017.49
28. Zhang R, Qi F, Zhao F, Li G, Shao S, Zhang X, et al. Cancer-associated fibroblasts enhance tumor-associated macrophages enrichment and suppress NK cells function in colorectal cancer. Cell Death Dis (2019) 10(4):273. doi: 10.1038/s41419-019-1435-2
29. Zhang J, Chen L, Xiao M, Wang C, Qin ZJTA. FSP1+ fibroblasts promote skin carcinogenesis by maintaining MCP-1-mediated macrophage infiltration and chronic inflammation. Am J Pathol (2011) 178(1):382–90. doi: 10.1016/j.ajpath.2010.11.017
30. Cohen N, Shani O, Raz Y, Sharon Y, Hoffman D, Abramovitz L, et al. Fibroblasts drive an immunosuppressive and growth-promoting microenvironment in breast cancer via secretion of Chitinase 3-like 1. Oncogene (2017) 36(31):4457–68. doi: 10.1038/onc.2017.65
31. Ksiazkiewicz M, Gottfried E, Kreutz M, Mack M, Hofstaedter F, Kunz-Schughart LAJI. Importance of CCL2-CCR2A/2B signaling for monocyte migration into spheroids of breast cancer-derived fibroblasts. Immunobiology (2010) 215(9-10):737–47. doi: 10.1016/j.imbio.2010.05.019
32. Comito G, Giannoni E, Segura C, Barcellos-de-Souza P, Raspollini M, Baroni G, et al. Cancer-associated fibroblasts and M2-polarized macrophages synergize during prostate carcinoma progression. Oncogene (2014) 33(19):2423–31. doi: 10.1038/onc.2013.191
33. Taddei ML, Cavallini L, Comito G, Giannoni E, Folini M, Marini A, et al. Senescent stroma promotes prostate cancer progression: the role of miR-210. Mol Oncol (2014) 8(8):1729–46. doi: 10.1016/j.molonc.2014.07.009
34. Ren G, Zhao X, Wang Y, Zhang X, Chen X, Xu C, et al. CCR2-dependent recruitment of macrophages by tumor-educated mesenchymal stromal cells promotes tumor development and is mimicked by TNFα. Cell Stem Cell (2012) 11(6):812–24. doi: 10.1016/j.stem.2012.08.013
35. Bonecchi R, Galliera E, Borroni EM, Corsi MM, Locati M, Mantovani A. Chemokines and chemokine receptors: an overview. Front Biosci-Landmark (2009) 14(2):540–51. doi: 10.2741/3261
36. Cascio S, Chandler C, Zhang L, Sinno S, Gao B, Onkar S, et al. Cancer-associated MSC drive tumor immune exclusion and resistance to immunotherapy, which can be overcome by Hedgehog inhibition. Sci Adv (2021) 7(46):eabi5790. doi: 10.1126/sciadv.abi5790
37. Chaturvedi P, Gilkes DM, Takano N, Semenza GL. Hypoxia-inducible factor-dependent signaling between triple-negative breast cancer cells and mesenchymal stem cells promotes macrophage recruitment. Proc Natl Acad Sci (2014) 111(20):E2120–9. doi: 10.1073/pnas.1406655111
38. Liao D, Luo Y, Markowitz D, Xiang R, Reisfeld RA. Cancer associated fibroblasts promote tumor growth and metastasis by modulating the tumor immune microenvironment in a 4T1 murine breast cancer model. PloS One (2009) 4(11):e7965. doi: 10.1371/journal.pone.0007965
39. Song M, He J, Pan QZ, Yang J, Zhao J, Zhang YJ, et al, et al. Cancer-associated fibroblast-mediated cellular crosstalk supports hepatocellular carcinoma progression. Hepatology (2021) 73(5):1717–35. doi: 10.1002/hep.31792
40. Cheng Y, Li H, Deng Y, Tai Y, Zeng K, Zhang Y, et al. Cancer-associated fibroblasts induce PDL1+ neutrophils through the IL6-STAT3 pathway that foster immune suppression in hepatocellular carcinoma. Cell Death Dis (2018) 9(4):422. doi: 10.1038/s41419-018-0458-4
41. Zhu Q, Zhang X, Zhang L, Li W, Wu H, Yuan X, et al. The IL-6-STAT3 axis mediates a reciprocal crosstalk between cancer-derived mesenchymal stem cells and neutrophils to synergistically prompt gastric cancer progression. Cell Death Dis (2014) 5(6):e1295. doi: 10.1038/cddis.2014.263
42. James AW, Pang S, Askarinam A, Corselli M, Zara JN, Goyal R, et al. Additive effects of sonic hedgehog and Nell-1 signaling in osteogenic versus adipogenic differentiation of human adipose-derived stromal cells. Stem Cells Dev (2012) 21(12):2170–8. doi: 10.1089/scd.2011.0461
43. Katopodi T, Petanidis S, Charalampidis C, Chatziprodromidou I, Eskitzis P, Tsavlis D, et al. Tumor-infiltrating dendritic cells: decisive roles in cancer immunosurveillance, immunoediting, and tumor T cell tolerance. Cells (2022) 11(20):3183. doi: 10.3390/cells11203183
44. Çakır U. MELANOMA-ASSOCIATED FIBROBLASTS INCREASE INTERLEUKIN-10 PRODUCTION OF MACROPHAGES IN A CYCLOOXIGENASE-DEPENDENT MANNER.
45. Donatelli SS, Zhou J-M, Gilvary DL, Eksioglu EA, Chen X, Cress WD, et al. TGF-β–inducible microRNA-183 silences tumor-associated natural killer cells. (2014) 111(11):4203–8. doi: 10.1073/pnas.1319269111
46. Viel S, Marçais A, Guimaraes FS-F, Loftus R, Rabilloud J, Grau M, et al. TGF-β inhibits the activation and functions of NK cells by repressing the mTOR pathway. Sci Signal (2016) 9(415):ra19–9. doi: 10.1126/scisignal.aad1884
47. Cheng J, Deng Y, Yi H, Wang G, Fu B, Chen W, et al. Hepatic carcinoma-associated fibroblasts induce IDO-producing regulatory dendritic cells through IL-6-mediated STAT3 activation. Oncogenesis (2016) 5(2):e198–8. doi: 10.1038/oncsis.2016.7
48. Oyama T, Ran S, Ishida T, Nadaf S, Kerr L, Carbone DP, et al. Vascular endothelial growth factor affects dendritic cell maturation through the inhibition of nuclear factor-κB activation in hemopoietic progenitor cells. J Immunol (1998) 160(3):1224–32. doi: 10.1158/1078-0432.ccr-18-1543
49. Rahma OE, Hodi F.S.J.C.C.R. The intersection between tumor angiogenesis and immune suppressionAntiangiogenesis and immunotherapy. Clin Cancer Res (2019) 25(18):5449–57. doi: 10.1158/1078-0432.ccr-18-1543
50. Curiel TJ, Wei S, Dong H, Alvarez X, Cheng P, Mottram P, et al. Blockade of B7-H1 improves myeloid dendritic cell–mediated antitumor immunity. Nat Med (2003) 9(5):562–7. doi: 10.1038/nm863
51. Yang X, Lin Y, Shi Y, Li B, Liu W, Yin W, et al. FAP promotes immunosuppression by cancer-associated fibroblasts in the tumor microenvironment via STAT3-CCL2 signaling. Cancer Res (2016) 76(14):4124–35. doi: 10.1158/0008-5472.CAN-15-2973
52. Shapouri-Moghaddam A, Mohammadian S, Vazini H, Taghadosi M, Esmaeili SA, Mardani F, et al. Macrophage plasticity, polarization, and function in health and disease. J Cell Physiol (2018) 233(9):6425–40. doi: 10.1002/jcp.26429
53. Murray PJ. Macrophage polarization. Annu Rev Physiol (2017) 79:541–66. doi: 10.1146/annurev-physiol-022516-034339
54. Guilloton F, Caron G, Ménard C, Pangault C, Amé-Thomas P, Dulong J, et al. Mesenchymal stromal cells orchestrate follicular lymphoma cell niche through the CCL2-dependent recruitment and polarization of monocytes. Blood (2012) 119(11):2556–67. doi: 10.1182/blood-2011-08-370908
55. Wu L, Saxena S, Awaji M, Singh RKJC. Tumor-associated neutrophils in cancer: going pro. Cancers (2019) 11(4):564. doi: 10.3390/cancers11040564
56. Que H, Fu Q, Lan T, Tian X, Wei X. Tumor-associated neutrophils and neutrophil-targeted cancer therapies. Biochim Biophys Acta (BBA) Rev Cancer (2022) 1877:188762. doi: 10.1016/j.bbcan.2022.188762
57. Fridlender ZG, Sun J, Kim S, Kapoor V, Cheng G, Ling L, et al. Polarization of tumor-associated neutrophil phenotype by TGF-beta: "N1" versus "N2" TAN. Cancer Cell (2009) 16(3):183–94. doi: 10.1016/j.ccr.2009.06.017
58. Jablonska J, Leschner S, Westphal K, Lienenklaus S, Weiss S. Neutrophils responsive to endogenous IFN-beta regulate tumor angiogenesis and growth in a mouse tumor model. J Clin Invest (2010) 120(4):1151–64. doi: 10.1172/JCI37223
59. Piccard H, Muschel RJ, Opdenakker G. On the dual roles and polarized phenotypes of neutrophils in tumor development and progression. Crit Rev Oncol Hematol (2012) 82(3):296–309. doi: 10.1016/j.critrevonc.2011.06.004
60. Yu J, Caligiuri MA. Viral-and tumor-reactive natural killer cells. In: Seminars in Immunology. Elsevier (2023).
61. Laskowski TJ, Biederstädt A, Rezvani K.J.N.R.C. Natural killer cells in antitumour adoptive cell immunotherapy. Nat Rev Cancer (2022) 22(10):557–75. doi: 10.1038/s41568-022-00491-0
62. Wolf NK, Kissiov DU, Raulet DH. Roles of natural killer cells in immunity to cancer, and applications to immunotherapy. NRI (2023) 23(2):90–105. doi: 10.1038/s41577-022-00732-1
63. Stojanovic A, Cerwenka A. Natural killer cells and solid tumors. J Innate Immun (2011) 3(4):355–64. doi: 10.1159/000325465
64. Habif G, Crinier A, André P, Vivier E, Narni-Mancinelli E. Targeting natural killer cells in solid tumors. Cell Mol Immunol (2019) 16(5):415–22. doi: 10.1038/s41423-019-0224-2
65. Ghosh T, Barik S, Bhuniya A, Dhar J, Dasgupta S, Ghosh S, et al. Tumor-associated mesenchymal stem cells inhibit naïve T cell expansion by blocking cysteine export from dendritic cells. Int J Cancer (2016) 139(9):2068–81. doi: 10.1002/ijc.30265
66. Foca NS, Berloco PB, Cortesini R. Tolerogenic dendritic cells in cancer, transplantation, and autoimmune diseases. Hum Immunol (2009) 70(5):277–80. doi: 10.1016/j.humimm.2009.03.003
67. Aponte-López A, Muñoz-Cruz S. Mast cells in the tumor microenvironment. Cancer Immunol Immunother (2020), 159–73. doi: 10.1007/978-3-030-49270-0_9
68. Dalton DK, Noelle RJ. The roles of mast cells in anticancer immunity. (2012) 61:1511–20. doi: 10.1007/s00262-012-1246-0
69. Derakhshani A, Vahidian F, Alihasanzadeh M, Mokhtarzadeh A, Nezhad PL, Baradaran BJIl. Mast cells: A double-edged sword in cancer. (2019) 209:28–35. doi: 10.1016/j.imlet.2019.03.011
70. Kolset SO, Pejler G. Serglycin: a structural and functional chameleon with wide impact on immune cells. (2011) 187(10):4927–33. doi: 10.4049/jimmunol.1100806
71. Beer TW, Ng LB, Murray K. Mast cells have prognostic value in Merkel cell carcinoma. Immunol Lett (2008) 30(1):27–30. doi: 10.1097/DAD.0b013e31815c932a
72. Medina V, Cricco G, Nuñez M, Martín G, Mohamad N, Correa-Fiz F, et al. Histamine-mediated signaling processes in human Malignant mammary cells. Cancer Biol Ther (2006) 5(11):1462–71. doi: 10.4161/cbt.5.11.3273
73. Vizio B, Biasi F, Scirelli T, Novarino A, Prati A, Ciuffreda L, et al. Pancreatic-carcinoma-cell-derived pro-angiogenic factors can induce endothelial-cell differentiation of a subset of circulating CD34+ progenitors. J Transl Med (2013) 11:314. doi: 10.1186/1479-5876-11-314
74. Carmeliet P, Jain RKJN. Molecular mechanisms and clinical applications of angiogenesis. Nature (2011) 473(7347):298–307. doi: 10.1038/nature10144
75. Detoraki A, Staiano RI, Granata F, Giannattasio G, Prevete N, de Paulis A, et al. Vascular endothelial growth factors synthesized by human lung mast cells exert angiogenic effects. Mechanisms of Allergy and Clinical Immunology (2009) 123(5):1142–9.e5. doi: 10.1016/j.jaci.2009.01.044
76. Messex JK, Liou G-Y. Impact of immune cells in the tumor microenvironment of prostate cancer metastasis. (2023) 13(2):333. doi: 10.3390/life13020333
77. Baram D, Vaday GG, Salamon P, Drucker I, Hershkoviz R, Mekori YA. Human mast cells release metalloproteinase-9 on contact with activated T cells: juxtacrine regulation by TNF-alpha. J Immunol (2001) 167(7):4008–16. doi: 10.4049/jimmunol.167.7.4008
78. Huang B, Lei Z, Zhang GM, Li D, Song C, Li B, et al. SCF-mediated mast cell infiltration and activation exacerbate the inflammation and immunosuppression in tumor microenvironment. Blood (2008) 112(4):1269–79. doi: 10.1182/blood-2008-03-147033
79. Pereira BA, Lister NL, Hashimoto K, Teng L, Flandes-Iparraguirre M, Eder A, et al. Tissue engineered human prostate microtissues reveal key role of mast cell-derived tryptase in potentiating cancer-associated fibroblast (CAF)-induced morphometric transition in vitro. Biomaterials (2019) 197:72–85. doi: 10.1016/j.biomaterials.2018.12.030
80. Kumar BV, Connors TJ, Farber DL. Human T cell development, localization, and function throughout life. Immunity (2018) 48(2):202–13. doi: 10.1016/j.immuni.2018.01.007
81. Gutcher I, Donkor MK, Ma Q, Rudensky AY, Flavell RA, Li MOJI. Autocrine transforming growth factor-β1 promotes in vivo Th17 cell differentiation. Immunity (2011) 34(3):396–408. doi: 10.1016/j.immuni.2011.03.005
82. De Monte L, Reni M, Tassi E, Clavenna D, Papa I, Recalde H, et al. Intratumor T helper type 2 cell infiltrate correlates with cancer-associated fibroblast thymic stromal lymphopoietin production and reduced survival in pancreatic cancer. J Exp Med (2011) 208(3):469–78. doi: 10.1084/jem.20101876
83. Henke E, Nandigama R, Ergün S. Extracellular matrix in the tumor microenvironment and its impact on cancer therapy. Front Mol Biosci (2019) 6:160. doi: 10.3389/fmolb.2019.00160
84. Kato T, Noma K, Ohara T, Kashima H, Katsura Y, Sato H, et al. Cancer-associated fibroblasts affect intratumoral CD8(+) and FoxP3(+) T cells via IL6 in the tumor microenvironment. Clin Cancer Res (2018) 24(19):4820–33. doi: 10.1158/1078-0432.CCR-18-0205
85. Ene-Obong A, Clear AJ, Watt J, Wang J, Fatah R, Riches JC, et al. Activated pancreatic stellate cells sequester CD8+ T cells to reduce their infiltration of the juxtatumoral compartment of pancreatic ductal adenocarcinoma. Gastroenterology (2013) 145(5):1121–32. doi: 10.1053/j.gastro.2013.07.025
86. Kraman M, Bambrough PJ, Arnold JN, Roberts EW, Magiera L, Jones JO, et al. Suppression of antitumor immunity by stromal cells expressing fibroblast activation protein-alpha. Science (2010) 330(6005):827–30. doi: 10.1126/science.1195300
87. Feig C, Jones JO, Kraman M, Wells RJ, Deonarine A, Chan DS, et al. Targeting CXCL12 from FAP-expressing carcinoma-associated fibroblasts synergizes with anti-PD-L1 immunotherapy in pancreatic cancer. Proc Natl Acad Sci U.S.A. (2013) 110(50):20212–7. doi: 10.1073/pnas.1320318110
88. Thomas DA, Massagué J. TGF-beta directly targets cytotoxic T cell functions during tumor evasion of immune surveillance. Cancer Cell (2005) 8(5):369–80. doi: 10.1016/j.ccr.2005.10.012
89. Goehrig D, Nigri J, Samain R, Wu Z, Cappello P, Gabiane G, et al. Stromal protein βig-h3 reprogrammes tumour microenvironment in pancreatic cancer. Gut (2019) 68(4):693–707. doi: 10.1136/gutjnl-2018-317570
90. Zhang B, Wu C, Zhang Z, Yan K, Li C, Li Y, et al. CXCL12 is associated with FoxP3(+) tumor-infiltrating lymphocytes and affects the survival of patients with oral squamous cell carcinoma. Oncol Lett (2019) 18(2):1099–106. doi: 10.3892/ol.2019.10415
91. Jacobs J, Deschoolmeester V, Zwaenepoel K, Flieswasser T, Deben C, Van den Bossche J, et al. Unveiling a CD70-positive subset of cancer-associated fibroblasts marked by pro-migratory activity and thriving regulatory T cell accumulation. Oncoimmunology (2018) 7(7):e1440167. doi: 10.1080/2162402X.2018.1440167
92. Tan W, Zhang W, Strasner A, Grivennikov S, Cheng JQ, Hoffman RM, et al. Tumour-infiltrating regulatory T cells stimulate mammary cancer metastasis through RANKL-RANK signalling. Nature (2011) 470(7335):548–53. doi: 10.1038/nature09707
93. Chen W, Jin W, Hardegen N, Lei KJ, Li L, Marinos N, et al. Conversion of peripheral CD4+CD25- naive T cells to CD4+CD25+ regulatory T cells by TGF-beta induction of transcription factor Foxp3. J Exp Med (2003) 198(12):1875–86. doi: 10.1084/jem.20030152
94. Costa A, Kieffer Y, Scholer-Dahirel A, Pelon F, Bourachot B, Cardon M, et al. Fibroblast heterogeneity and immunosuppressive environment in human breast cancer. Cancer Cell (2018) 33(3):463–79.e10. doi: 10.1016/j.ccell.2018.01.011
95. Saravia J, Chapman NM, Chi HJC. Helper T cell differentiation. Cell Mol Immunol (2019) 16(7):634–43. doi: 10.1038/s41423-019-0220-6
96. Hilligan KL, Ronchese FJC. Antigen presentation by dendritic cells and their instruction of CD4+ T helper cell responses. (2020) 17(6):587–99. doi: 10.1038/s41423-020-0465-0
97. Patel SA, Meyer JR, Greco SJ, Corcoran KE, Bryan M, Rameshwar P. Mesenchymal stem cells protect breast cancer cells through regulatory T cells: role of mesenchymal stem cell-derived TGF-β. J Immunol (2010) 184(10):5885–94. doi: 10.4049/jimmunol.0903143
98. Bernardo ME, Fibbe WE. Mesenchymal stromal cells: sensors and switchers of inflammation. Cell Stem Cell (2013) 13(4):392–402. doi: 10.1016/j.stem.2013.09.006
99. Patel SA, Meyer JR, Greco SJ, Corcoran KE, Bryan M, Rameshwar P. Mesenchymal stem cells protect breast cancer cells through regulatory T cells: role of mesenchymal stem cell-derived TGF-beta. J Immunol (2010) 184(10):5885–94. doi: 10.4049/jimmunol.0903143
100. Li X, Fan Q, Peng X, Yang S, Wei S, Liu J, et al. Mesenchymal/stromal stem cells: necessary factors in tumour progression. Cell Death Discovery (2022) 8(1):333. doi: 10.1038/s41419-021-04424-x
101. Farhood B, Najafi M, Mortezaee K. CD8(+) cytotoxic T lymphocytes in cancer immunotherapy: A review. J Cell Physiol (2019) 234(6):8509–21. doi: 10.1002/jcp.27782
102. Uzhachenko RV, Shanker A. CD8(+) T lymphocyte and NK cell network: circuitry in the cytotoxic domain of immunity. Front Immunol (2019) 10:1906. doi: 10.3389/fimmu.2019.01906
103. Ren G, Zhang L, Zhao X, Xu G, Zhang Y, Roberts AI, et al. Mesenchymal stem cell-mediated immunosuppression occurs via concerted action of chemokines and nitric oxide. Cell Stem Cell (2008) 2(2):141–50. doi: 10.1016/j.stem.2007.11.014
104. Su J, Chen X, Huang Y, Li W, Li J, Cao K, et al. Phylogenetic distinction of iNOS and IDO function in mesenchymal stem cell-mediated immunosuppression in mammalian species. Cell Death Differ (2014) 21(3):388–96. doi: 10.1038/cdd.2013.149
105. Djouad F, Bony C, Apparailly F, Louis-Plence P, Jorgensen C, Noël D. Earlier onset of syngeneic tumors in the presence of mesenchymal stem cells. Transplantation (2006) 82(8):1060–6. doi: 10.1097/01.tp.0000236098.13804.0b
106. Montesinos JJ, Mora-García Mde L, Mayani H, Flores-Figueroa E, García-Rocha R, Fajardo-Orduña GR, et al. In vitro evidence of the presence of mesenchymal stromal cells in cervical cancer and their role in protecting cancer cells from cytotoxic T cell activity. Stem Cells Dev (2013) 22(18):2508–19. doi: 10.1089/scd.2013.0084
107. Downs-Canner SM, Meier J, Vincent BG, Serody JS. B cell function in the tumor microenvironment. Annu Rev Immunol (2022) 40:169–93. doi: 10.1146/annurev-immunol-101220-015603
108. Corcione A, Benvenuto F, Ferretti E, Giunti D, Cappiello V, Cazzanti F, et al. Human mesenchymal stem cells modulate B-cell functions. Blood (2006) 107(1):367–72. doi: 10.1182/blood-2005-07-2657
109. Asari S, Itakura S, Ferreri K, Liu CP, Kuroda Y, Kandeel F, et al. Mesenchymal stem cells suppress B-cell terminal differentiation. Exp Hematol (2009) 37(5):604–15. doi: 10.1016/j.exphem.2009.01.005
110. Harper J, Sainson RC. Regulation of the anti-tumour immune response by cancer-associated fibroblasts. Semin Cancer Biol (2014) 25:69–77. doi: 10.1016/j.semcancer.2013.12.005
111. Wang W, Zhong W, Yuan J, Yan C, Hu S, Tong Y, et al. Involvement of Wnt/β-catenin signaling in the mesenchymal stem cells promote metastatic growth and chemoresistance of cholangiocarcinoma. Oncotarget (2015) 6(39):42276. doi: 10.18632/oncotarget.5514
112. Lin HD, Bongso A, Gauthaman K, Biswas A, Choolani M, Fong C-Y. Human Wharton’s jelly stem cell conditioned medium enhances freeze-thaw survival and expansion of cryopreserved CD34+ cells. Stem Cell Rev Rep (2013) 9:172–83. doi: 10.1007/s12015-013-9426-7
113. Galland S, Stamenkovic I. Mesenchymal stromal cells in cancer: a review of their immunomodulatory functions and dual effects on tumor progression. J Pathol (2020) 250(5):555–72. doi: 10.1002/path.5357
114. Ma Y, Hao X, Zhang S, Zhang J. The in vitro and in vivo effects of human umbilical cord mesenchymal stem cells on the growth of breast cancer cells. Breast Cancer Res Treat (2012) 133:473–85. doi: 10.1007/s10549-011-1774-x
115. Galland S, Vuille J, Martin P, Letovanec I, Caignard A, Fregni G, et al. Tumor-derived mesenchymal stem cells use distinct mechanisms to block the activity of natural killer cell subsets. Cell Rep (2017) 20(12):2891–905. doi: 10.1016/j.celrep.2017.08.089
116. Sun L, Wang Q, Chen B, Zhao Y, Shen B, Wang X, et al. Human gastric cancer mesenchymal stem cell-derived IL15 contributes to tumor cell epithelial-mesenchymal transition via upregulation tregs ratio and PD-1 expression in CD4+ T cell. Stem Cells Dev (2018) 27(17):1203–14. doi: 10.1089/scd.2018.0043
117. Liotta F, Querci V, Mannelli G, Santarlasci V, Maggi L, Capone M, et al. Mesenchymal stem cells are enriched in head neck squamous cell carcinoma, correlates with tumour size and inhibit T-cell proliferation. Br J Cancer (2015) 112(4):745–54. doi: 10.1038/bjc.2015.15
118. Escobar P, Bouclier C, Serret J, Bièche I, Brigitte M, Caicedo A, et al. IL-1β produced by aggressive breast cancer cells is one of the factors that dictate their interactions with mesenchymal stem cells through chemokine production. Oncotarget (2015) 6(30):29034. doi: 10.18632/oncotarget.4732
119. Zhu Q, Zhang X, Zhang L, Li W, Wu H, Yuan X, et al. The IL-6–STAT3 axis mediates a reciprocal crosstalk between cancer-derived mesenchymal stem cells and neutrophils to synergistically prompt gastric cancer progression. Cell Death Dis (2014) 5(6):e1295–5. doi: 10.1038/cddis.2014.263
120. Naderi EH, Skah S, Ugland H, Myklebost O, Sandnes DL, Torgersen ML, et al. Bone marrow stroma-derived PGE2 protects BCP-ALL cells from DNA damage-induced p53 accumulation and cell death. Mol Cancer (2015) 14(1):1–12. doi: 10.1186/s12943-014-0278-9
121. He W, Liang B, Wang C, Li S, Zhao Y, Huang Q, et al. MSC-regulated lncRNA MACC1-AS1 promotes stemness and chemoresistance through fatty acid oxidation in gastric cancer. Oncogene (2019) 38(23):4637–54. doi: 10.1038/s41388-019-0747-0
122. Yan X, Zhang D, Wu W, Wu S, Qian J, Hao Y, et al. Mesenchymal Stem Cells Promote Hepatocarcinogenesis via lncRNA–MUF Interaction with ANXA2 and miR-34aLncRNA–MUF Induced by HCC-MSC Promotes Hepatocarcinogenesis. Cancer Res (2017) 77(23):6704–16. doi: 10.1158/0008-5472.CAN-17-1915
123. De Boeck A, Pauwels P, Hensen K, Rummens J-L, Westbroek W, Hendrix A, et al. Bone marrow-derived mesenchymal stem cells promote colorectal cancer progression through paracrine neuregulin 1/HER3 signalling. Gut (2013) 62(4):550–60. doi: 10.1136/gutjnl-2011-301393
124. Yan XL, Jia YL, Chen L, Zeng Q, Zhou JN, Fu CJ, et al. Hepatocellular carcinoma-associated mesenchymal stem cells promote hepatocarcinoma progression: role of the S100A4-miR155-SOCS1-MMP9 axis. Hepatology (2013) 57(6):2274–86. doi: 10.1002/hep.26257
125. Figueroa J, Phillips LM, Shahar T, Hossain A, Gumin J, Kim H, et al. Exosomes from Glioma-Associated Mesenchymal Stem Cells Increase the Tumorigenicity of Glioma Stem-like Cells via Transfer of miR-1587GA-hMSCs Regulate GSCs via Exosomal miRNA. Cancer Res (2017) 77(21):5808–19. doi: 10.1158/0008-5472.CAN-16-2524
126. Wang W, Wang Q, Huang D-B, Sun Q-K, Wu S-S, Zhao Y-J, et al. Tumor-associated mesenchymal stem cells promote hepatocellular carcinoma metastasis via a DNM3OS/KDM6B/TIAM1 axis. Cancer Lett (2021) 503:19–31. doi: 10.1016/j.canlet.2021.01.011
127. Chen B, Cai T, Huang C, Zang X, Sun L, Guo S, et al. G6PD-NF-κB-HGF signal in gastric cancer-associated mesenchymal stem cells promotes the proliferation and metastasis of gastric cancer cells by upregulating the expression of HK2. Front Oncol (2021) 11:648706. doi: 10.3389/fonc.2021.648706
128. McLean K, Gong Y, Choi Y, Deng N, Yang K, Bai S, et al. Human ovarian carcinoma–associated mesenchymal stem cells regulate cancer stem cells and tumorigenesis via altered BMP production. J Clin Invest (2011) 121(8):3206–19. doi: 10.1172/JCI45273
129. Yan X-l, Fu C-J, Chen L, Qin J-H, Zeng Q, Yuan H-F, et al. Mesenchymal stem cells from primary breast cancer tissue promote cancer proliferation and enhance mammosphere formation partially via EGF/EGFR/Akt pathway. Breast Cancer Res Treat (2012) 132:153–64. doi: 10.1007/s10549-011-1577-0
130. Du Q, Ye X, Lu S-R, Li H, Liu H-Y, Zhai Q, et al. Exosomal miR-30a and miR-222 derived from colon cancer mesenchymal stem cells promote the tumorigenicity of colon cancer through targeting MIA3. J Gastrointestinal Oncol (2021) 12(1):52. doi: 10.21037/jgo-20-513
131. Chen D, Sun Y, Yuan Y, Han Z, Zhang P, Zhang J, et al. miR-100 induces epithelial-mesenchymal transition but suppresses tumorigenesis, migration and invasion. PLoS Genet (2014) 10(2):e1004177. doi: 10.1371/journal.pgen.1004177
132. Karnoub AE, Dash AB, Vo AP, Sullivan A, Brooks MW, Bell GW, et al. Mesenchymal stem cells within tumour stroma promote breast cancer metastasis. Nature (2007) 449(7162):557–63. doi: 10.1038/nature06188
133. Miyazaki Y, Oda T, Mori N, Kida YS. Adipose-derived mesenchymal stem cells differentiate into pancreatic cancer-associated fibroblasts in vitro. FEBS Open Bio (2020) 10(11):2268–81. doi: 10.1002/2211-5463.12976
134. Zhang T, Lee YW, Rui YF, Cheng TY, Jiang XH, Li G. Bone marrow-derived mesenchymal stem cells promote growth and angiogenesis of breast and prostate tumors. Stem Cell Res Ther (2013) 4(3):1–15. doi: 10.1186/scrt221
135. Chandler EM, Seo BR, Califano JP, Andresen Eguiluz RC, Lee JS, Yoon CJ, et al. Implanted adipose progenitor cells as physicochemical regulators of breast cancer. Proc Natl Acad Sci (2012) 109(25):9786–91. doi: 10.1073/pnas.1121160109
136. Lee MJ, Heo SC, Shin SH, Kwon YW, Do EK, Suh D-S, et al. Oncostatin M promotes mesenchymal stem cell-stimulated tumor growth through a paracrine mechanism involving periostin and TGFBI. Int J Biochem Cell Biol (2013) 45(8):1869–77. doi: 10.1016/j.biocel.2013.05.027
137. Spaeth EL, Dembinski JL, Sasser AK, Watson K, Klopp A, Hall B, et al. Mesenchymal stem cell transition to tumor-associated fibroblasts contributes to fibrovascular network expansion and tumor progression. PLoS One (2009) 4(4):e4992. doi: 10.1371/journal.pone.0004992
138. Nomoto-Kojima N, Aoki S, Uchihashi K, Matsunobu A, Koike E, Ootani A, et al. Interaction between adipose tissue stromal cells and gastric cancer cells in vitro. Cell Tissue Res (2011) 344(2):287–98. doi: 10.1007/s00441-011-1144-3
139. Ullah M, Akbar A, Ng NN, Concepcion W, Thakor AS. Mesenchymal stem cells confer chemoresistance in breast cancer via a CD9 dependent mechanism. Oncotarget (2019) 10(37):3435. doi: 10.18632/oncotarget.26952
140. Qiu Y, Guo J, Mao R, Chao K, Chen B, He Y, et al. TLR3 preconditioning enhances the therapeutic efficacy of umbilical cord mesenchymal stem cells in TNBS-induced colitis via the TLR3-Jagged-1-Notch-1 pathway. Mucosal Immunol (2017) 10(3):727–42. doi: 10.1038/mi.2016.78
141. Waterman RS, Tomchuck SL, Henkle SL, Betancourt AM. A new mesenchymal stem cell (MSC) paradigm: polarization into a pro-inflammatory MSC1 or an Immunosuppressive MSC2 phenotype. PloS One (2010) 5(4):e10088. doi: 10.1371/journal.pone.0010088
142. Li GC, Zhang HW, Zhao QC, Sun L, Yang JJ, Hong L, et al. Mesenchymal stem cells promote tumor angiogenesis via the action of transforming growth factor β1. Oncol Lett (2016) 11(2):1089–94. doi: 10.3892/ol.2015.3997
143. Ramasamy R, Lam EW, Soeiro I, Tisato V, Bonnet D, Dazzi F. Mesenchymal stem cells inhibit proliferation and apoptosis of tumor cells: impact on in vivo tumor growth. Leukemia (2007) 21(2):304–10. doi: 10.1038/sj.leu.2404489
144. Bonuccelli G, Avnet S, Grisendi G, Salerno M, Granchi D, Dominici M, et al. Role of mesenchymal stem cells in osteosarcoma and metabolic reprogramming of tumor cells. Oncotarget (2014) 5(17):7575. doi: 10.18632/oncotarget.2243
145. Crovello CS, Lai C, Cantley LC, Carraway KL. Differential signaling by the epidermal growth factor-like growth factors neuregulin-1 and neuregulin-2. J Biol Chem (1998) 273(41):26954–61. doi: 10.1074/jbc.273.41.26954
146. Mandel K, Yang Y, Schambach A, Glage S, Otte A, Hass R. Mesenchymal stem cells directly interact with breast cancer cells and promote tumor cell growth in vitro and in vivo. Stem Cells Dev (2013) 22(23):3114–27. doi: 10.1089/scd.2013.0249
147. Parekkadan B, Van Poll D, Suganuma K, Carter EA, Berthiaume F, Tilles AW, et al. Mesenchymal stem cell-derived molecules reverse fulminant hepatic failure. PloS One (2007) 2(9):e941. doi: 10.1371/journal.pone.0000941
148. Hu X, Zhou Y, Dong K, Sun Z, Zhao D, Wang W, et al. Programming of the development of tumor-promoting neutrophils by mesenchymal stromal cells. Cell Physiol Biochem (2014) 33(6):1802–14. doi: 10.1159/000362959
149. Wang Y, He L, Du Y, Zhu P, Huang G, Luo J, et al. The long noncoding RNA lncTCF7 promotes self-renewal of human liver cancer stem cells through activation of Wnt signaling. Cell Stem Cell (2015) 16(4):413–25. doi: 10.1016/j.stem.2015.03.003
150. Dong L, Pu Y, Zhang L, Qi Q, Xu L, Li W, et al. Human umbilical cord mesenchymal stem cell-derived extracellular vesicles promote lung adenocarcinoma growth by transferring miR-410. Cell Death Dis (2018) 9(2):218. doi: 10.1038/s41419-018-0323-5
151. Wang M, Zhao C, Shi H, Zhang B, Zhang L, Zhang X, et al. Deregulated microRNAs in gastric cancer tissue-derived mesenchymal stem cells: novel biomarkers and a mechanism for gastric cancer. Br J Cancer (2014) 110(5):1199–210. doi: 10.1038/bjc.2014.14
152. Matsushita H, Vesely MD, Koboldt DC, Rickert CG, Uppaluri R, Magrini VJ, et al. Cancer exome analysis reveals a T-cell-dependent mechanism of cancer immunoediting. Nature (2012) 482(7385):400–4. doi: 10.1038/nature10755
153. Cuiffo BG, Campagne A, Bell GW, Lembo A, Orso F, Lien EC, et al. MSC-regulated microRNAs converge on the transcription factor FOXP2 and promote breast cancer metastasis. Cell Stem Cell (2014) 15(6):762–74. doi: 10.1016/j.stem.2014.10.001
154. Wang D, Li J, Zhang Y, Zhang M, Chen J, Li X, et al. Umbilical cord mesenchymal stem cell transplantation in active and refractory systemic lupus erythematosus: a multicenter clinical study. Arthritis Res Ther (2014) 16(2):1–14. doi: 10.1186/ar4520
155. Fritz V, Brondello J, Gordeladze J, Reseland J, Bony C, Yssel H, et al. Bone-metastatic prostate carcinoma favors mesenchymal stem cell differentiation toward osteoblasts and reduces their osteoclastogenic potential. J Cell Biochem (2011) 112(11):3234–45. doi: 10.1002/jcb.23258
156. Ponte AL, Marais E, Gallay N, Langonné A, Delorme B, Hérault O, et al. The in vitro migration capacity of human bone marrow mesenchymal stem cells: comparison of chemokine and growth factor chemotactic activities. Stem Cells (2007) 25(7):1737–45. doi: 10.1634/stemcells.2007-0054
157. De Becker A, Van Riet I. Homing and migration of mesenchymal stromal cells: how to improve the efficacy of cell therapy? World J Stem Cells (2016) 8(3):73. doi: 10.4252/wjsc.v8.i3.73
158. Ganesh K, Massagué J. Targeting metastatic cancer. Nat Med (2021) 27(1):34–44. doi: 10.1038/s41591-020-01195-4
159. Loh J-K, Wang M-L, Cheong S-K, Tsai F-T, Huang S-H, Wu J-R, et al. The study of cancer cell in stromal environment through induced pluripotent stem cell–derived mesenchymal stem cells. J Chin Med Assoc (2022) 85(8):821–30. doi: 10.1097/JCMA.0000000000000759
160. Corcoran KE, Trzaska KA, Fernandes H, Bryan M, Taborga M, Srinivas V, et al. Mesenchymal stem cells in early entry of breast cancer into bone marrow. PloS One (2008) 3(6):e2563. doi: 10.1371/journal.pone.0002563
161. Lin R, Wang S, Zhao RC. Exosomes from human adipose-derived mesenchymal stem cells promote migration through Wnt signaling pathway in a breast cancer cell model. Mol Cell Biochem (2013) 383:13–20. doi: 10.1007/s11010-013-1746-z
162. Tsai KS, Yang SH, Lei YP, Tsai CC, Chen HW, Hsu CY, et al. Mesenchymal stem cells promote formation of colorectal tumors in mice. Gastroenterology (2011) 141(3):1046–56. doi: 10.1053/j.gastro.2011.05.045
163. Liu S, Ginestier C, Ou SJ, Clouthier SG, Patel SH, Monville F, et al. Breast cancer stem cells are regulated by mesenchymal stem cells through cytokine networksMSCs regulate breast cancer stem cells. Cancer Res (2011) 71(2):614–24. doi: 10.1158/0008-5472.CAN-10-0538
164. Rhodes LV, Antoon JW, Muir SE, Elliott S, Beckman BS, Burow ME. Effects of human mesenchymal stem cells on ER-positive human breast carcinoma cells mediated through ER-SDF-1/CXCR4 crosstalk. Mol Cancer (2010) 9(1):1–15. doi: 10.1186/1476-4598-9-295
165. Halpern JL, Kilbarger A, Lynch CC. Mesenchymal stem cells promote mammary cancer cell migration in vitro via the CXCR2 receptor. Cancer Lett (2011) 308(1):91–9. doi: 10.1016/j.canlet.2011.04.018
166. Zhang XH-F, Jin X, Malladi S, Zou Y, Wen YH, Brogi E, et al. Selection of bone metastasis seeds by mesenchymal signals in the primary tumor stroma. Cell (2013) 154(5):1060–73. doi: 10.1016/j.cell.2013.07.036
167. Mi Z, Bhattacharya SD, Kim VM, Guo H, Talbot LJ, Kuo PC. Osteopontin promotes CCL5-mesenchymal stromal cell-mediated breast cancer metastasis. Carcinogenesis (2011) 32(4):477–87. doi: 10.1093/carcin/bgr009
168. Nishikawa G, Kawada K, Nakagawa J, Toda K, Ogawa R, Inamoto S, et al. Bone marrow-derived mesenchymal stem cells promote colorectal cancer progression via CCR5. Cell Death Dis (2019) 10(4):264. doi: 10.1038/s41419-019-1508-2
169. Luo J, Ok Lee S, Liang L, Huang C, Li L, Wen S, et al. Infiltrating bone marrow mesenchymal stem cells increase prostate cancer stem cell population and metastatic ability via secreting cytokines to suppress androgen receptor signaling. Oncogene (2014) 33(21):2768–78. doi: 10.1038/onc.2013.233
170. Müller A, Homey B, Soto H, Ge N, Catron D, Buchanan ME, et al. Involvement of chemokine receptors in breast cancer metastasis. nature (2001) 410(6824):50–6. doi: 10.1038/35065016
171. Hughes RM, Simons BW, Khan H, Miller R, Kugler V, Torquato S, et al. Asporin restricts mesenchymal stromal cell differentiation, alters the tumor microenvironment, and drives metastatic progressionAsporin in MSCs and metastasis. Cancer Res (2019) 79(14):3636–50. doi: 10.1158/0008-5472.CAN-18-2931
172. Chen Y-C, Gonzalez ME, Burman B, Zhao X, Anwar T, Tran M, et al. Mesenchymal stem/stromal cell engulfment reveals metastatic advantage in breast cancer. Cell Rep (2019) 27(13):3916–26.e5. doi: 10.1016/j.celrep.2019.05.084
173. Chen J, Ji T, Wu D, Jiang S, Zhao J, Lin H, et al. Human mesenchymal stem cells promote tumor growth via MAPK pathway and metastasis by epithelial mesenchymal transition and integrin α5 in hepatocellular carcinoma. Cell Death Dis (2019) 10(6):425. doi: 10.1038/s41419-019-1622-1
174. Rodini CO, da Silva PBG, Assoni AF, Carvalho VM, Okamoto OK. Mesenchymal stem cells enhance tumorigenic properties of human glioblastoma through independent cell-cell communication mechanisms. Oncotarget (2018) 9(37):24766. doi: 10.18632/oncotarget.25346
175. Hardy SD, Shinde A, Wang W-H, Wendt MK, Geahlen RL. Regulation of epithelial-mesenchymal transition and metastasis by TGF-β, P-bodies, and autophagy. Oncotarget (2017) 8(61):103302. doi: 10.18632/oncotarget.21871
176. Caicedo A, Fritz V, Brondello J-M, Ayala M, Dennemont I, Abdellaoui N, et al. MitoCeption as a new tool to assess the effects of mesenchymal stem/stromal cell mitochondria on cancer cell metabolism and function. Sci Rep (2015) 5(1):9073. doi: 10.1038/srep09073
177. Mombo BN, Gerbal-Chaloin S, Bokus A, Daujat-Chavanieu M, Jorgensen C, Hugnot J-P, et al. MitoCeption: transferring isolated human MSC mitochondria to glioblastoma stem cells. J Visualized Experiments: JoVE (2017) 120. doi: 10.3791/55245
178. Malekpour K, Hazrati A, Soudi S, Hashemi SM. Mechanisms behind therapeutic potentials of mesenchymal stem cell mitochondria transfer/delivery. J Controlled Release (2023) 354:755–69. doi: 10.1016/j.jconrel.2023.01.059
179. Kabashima-Niibe A, Higuchi H, Takaishi H, Masugi Y, Matsuzaki Y, Mabuchi Y, et al. Mesenchymal stem cells regulate epithelial–mesenchymal transition and tumor progression of pancreatic cancer cells. Cancer Sci (2013) 104(2):157–64. doi: 10.1111/cas.12059
180. McAndrews KM, McGrail DJ, Ravikumar N, Dawson MR. Mesenchymal stem cells induce directional migration of invasive breast cancer cells through TGF-β. Sci Rep (2015) 5(1):16941. doi: 10.1038/srep16941
181. El-Haibi CP, Bell GW, Zhang J, Collmann AY, Wood D, Scherber CM, et al. Critical role for lysyl oxidase in mesenchymal stem cell-driven breast cancer Malignancy. Proc Natl Acad Sci (2012) 109(43):17460–5. doi: 10.1073/pnas.1206653109
182. Wang Y, Chu Y, Li K, Zhang G, Guo Z, Wu X, et al. Exosomes secreted by adipose-derived mesenchymal stem cells foster metastasis and osteosarcoma proliferation by increasing COLGALT2 expression. Front Cell Dev Biol (2020) 8:353. doi: 10.3389/fcell.2020.00353
183. Yang Y, Bucan V, Baehre H, Von Der Ohe J, Otte A, Hass R. Acquisition of new tumor cell properties by MSC-derived exosomes. Int J Oncol (2015) 47(1):244–52. doi: 10.3892/ijo.2015.3001
184. Deryugina EI, Quigley JP. Matrix metalloproteinases and tumor metastasis. Cancer metastasis Rev (2006) 25:9–34. doi: 10.1007/s10555-006-7886-9
185. Ries C, Egea V, Karow M, Kolb H, Jochum M, Neth P. MMP-2, MT1-MMP, and TIMP-2 are essential for the invasive capacity of human mesenchymal stem cells: differential regulation by inflammatory cytokines. Blood (2007) 109(9):4055–63. doi: 10.1182/blood-2006-10-051060
186. Mao Y, Keller ET, Garfield DH, Shen K, Wang J. Stromal cells in tumor microenvironment and breast cancer. Cancer Metastasis Rev (2013) 32:303–15. doi: 10.1007/s10555-012-9415-3
187. Hynes RO. The extracellular matrix: not just pretty fibrils. Science (2009) 326(5957):1216–9. doi: 10.1126/science.1176009
188. Dittmer A, Hohlfeld K, Lützkendorf J, Müller LP, Dittmer J. Human mesenchymal stem cells induce E-cadherin degradation in breast carcinoma spheroids by activating ADAM10. Cell Mol Life Sci (2009) 66:3053–65. doi: 10.1007/s00018-009-0089-0
189. Attar-Schneider O, Drucker L, Gottfried M. The effect of mesenchymal stem cells' secretome on lung cancer progression is contingent on their origin: primary or metastatic niche. Lab Invest (2018) 98(12):1549–61. doi: 10.1038/s41374-018-0110-z
190. Valenzuela Alvarez M, Gutierrez LM, Correa A, Lazarowski A, Bolontrade MF. Metastatic niches and the modulatory contribution of mesenchymal stem cells and its exosomes. Int J Mol Sci (2019) 20(8):1946. doi: 10.3390/ijms20081946
191. Shi Y, Du L, Lin L, Wang Y. Tumour-associated mesenchymal stem/stromal cells: emerging therapeutic targets. Nat Rev Drug Discov (2017) 16(1):35–52. doi: 10.1038/nrd.2016.193
192. Ritter E, Perry A, Yu J, Wang T, Tang L, Bieberich E. Breast cancer cell-derived fibroblast growth factor 2 and vascular endothelial growth factor are chemoattractants for bone marrow stromal stem cells. Ann Surg (2008) 247(2):310–4. doi: 10.1097/SLA.0b013e31816401d5
193. Kaplan RN, Riba RD, Zacharoulis S, Bramley AH, Vincent L, Costa C, et al. VEGFR1-positive haematopoietic bone marrow progenitors initiate the pre-metastatic niche. Nature (2005) 438(7069):820–7. doi: 10.1038/nature04186
194. Sai B, Dai Y, Fan S, Wang F, Wang L, Li Z, et al. Cancer-educated mesenchymal stem cells promote the survival of cancer cells at primary and distant metastatic sites via the expansion of bone marrow-derived-PMN-MDSCs. Cell Death Dis (2019) 10(12):941. doi: 10.1038/s41419-019-2149-1
195. Mandal S, Arfuso F, Sethi G, Dharmarajan A, Warrier S. Encapsulated human mesenchymal stem cells (eMSCs) as a novel anti-cancer agent targeting breast cancer stem cells: Development of 3D primed therapeutic MSCs. Int J Biochem Cell Biol (2019) 110:59–69. doi: 10.1016/j.biocel.2019.02.001
196. Kukia NR, Alipanah-Moghadam R, Delirezh N, Mazani M. Mesenchymal stromal stem cell-derived microvesicles enhance tumor lysate pulsed dendritic cell stimulated autologous T lymphocyte cytotoxicity. Asian Pacific J Cancer Prevention: APJCP (2018) 19(7):1895. doi: 10.22034/APJCP.2018.19.7.1895
197. Mohd Ali N, Yeap SK, Ho WY, Boo L, Ky H, Satharasinghe DA, et al. Adipose MSCs suppress MCF7 and MDA-MB-231 breast cancer metastasis and EMT pathways leading to dormancy via exosomal-miRNAs following co-culture interaction. Pharmaceuticals (2020) 14(1):8. doi: 10.3390/ph14010008
198. Li GC, Ye QH, Xue YH, Sun HJ, Zhou HJ, Ren N, et al. Human mesenchymal stem cells inhibit metastasis of a hepatocellular carcinoma model using the MHCC97-H cell line. Cancer Sci (2010) 101(12):2546–53. doi: 10.1111/j.1349-7006.2010.01738.x
199. Li T, Wan Y, Su Z, Li J, Han M, Zhou C. Mesenchymal stem cell-derived exosomal microRNA-3940-5p inhibits colorectal cancer metastasis by targeting integrin α6. Digestive Dis Sci (2021) 66:1916–27. doi: 10.1007/s10620-020-06458-1
200. Paunescu V, Bojin FM, Tatu CA, Gavriliuc OI, Rosca A, Gruia AT, et al. Tumour-associated fibroblasts and mesenchymal stem cells: more similarities than differences. J Cell Mol Med (2011) 15(3):635–46. doi: 10.1111/j.1582-4934.2010.01044.x
201. Mishra PJ, Mishra PJ, Humeniuk R, Medina DJ, Alexe G, Mesirov JP, et al. Carcinoma-associated fibroblast–like differentiation of human mesenchymal stem cells. Cancer Res (2008) 68(11):4331–9. doi: 10.1158/0008-5472.CAN-08-0943
202. Quante M, Tu SP, Tomita H, Gonda T, Wang SS, Takashi S, et al. Bone marrow-derived myofibroblasts contribute to the mesenchymal stem cell niche and promote tumor growth. Cancer Cell (2011) 19(2):257–72. doi: 10.1016/j.ccr.2011.01.020
203. Arena S, Salati M, Sorgentoni G, Barbisan F, Orciani M. Characterization of tumor-derived mesenchymal stem cells potentially differentiating into cancer-associated fibroblasts in lung cancer. Clin Trans Oncol (2018) 20:1582–91. doi: 10.1007/s12094-018-1894-4
204. Barcellos-de-Souza P, Comito G, Pons-Segura C, Taddei ML, Gori V, Becherucci V, et al. Mesenchymal stem cells are recruited and activated into carcinoma-associated fibroblasts by prostate cancer microenvironment-derived TGF-β1. Stem Cells (2016) 34(10):2536–47. doi: 10.1002/stem.2412
205. Shangguan L, Ti X, Krause U, Hai B, Zhao Y, Yang Z, et al. Inhibition of TGF-β/Smad signaling by BAMBI blocks differentiation of human mesenchymal stem cells to carcinoma-associated fibroblasts and abolishes their protumor effects. Stem Cells (2012) 30(12):2810–9. doi: 10.1002/stem.1251
206. Gu J, Qian H, Shen L, Zhang X, Zhu W, Huang L, et al. Gastric cancer exosomes trigger differentiation of umbilical cord derived mesenchymal stem cells to carcinoma-associated fibroblasts through TGF-β/Smad pathway. PloS One (2012) 7(12):e52465. doi: 10.1371/journal.pone.0052465
207. Chaturvedi P, Gilkes DM, Wong CCL, Luo W, Zhang H, Wei H, et al. Hypoxia-inducible factor–dependent breast cancer–mesenchymal stem cell bidirectional signaling promotes metastasis. J Clin Invest (2012) 123(1). doi: 10.1172/JCI64993
208. Walter M, Liang S, Ghosh S, Hornsby P, Li R. Interleukin 6 secreted from adipose stromal cells promotes migration and invasion of breast cancer cells. Oncogene (2009) 28(30):2745–55. doi: 10.1038/onc.2009.130
209. Roodhart JM, Daenen LG, Stigter EC, Prins HJ, Gerrits J, Houthuijzen JM, et al. Mesenchymal stem cells induce resistance to chemotherapy through the release of platinum-induced fatty acids. Cancer Cell (2011) 20(3):370–83. doi: 10.1016/j.ccr.2011.08.010
210. Ji R, Zhang B, Zhang X, Xue J, Yuan X, Yan Y, et al. Exosomes derived from human mesenchymal stem cells confer drug resistance in gastric cancer. Cell Cycle (2015) 14(15):2473–83. doi: 10.1080/15384101.2015.1005530
211. Han Z, Jing Y, Xia Y, Zhang S, Hou J, Meng Y, et al. Mesenchymal stem cells contribute to the chemoresistance of hepatocellular carcinoma cells in inflammatory environment by inducing autophagy. Cell Biosci (2014) 4:22. doi: 10.1186/2045-3701-4-22
212. Huang M, Zhang D, Wu JY, Xing K, Yeo E, Li C, et al. Wnt-mediated endothelial transformation into mesenchymal stem cell–like cells induces chemoresistance in glioblastoma. Sci Trans Med (2020) 12(532):eaay7522. doi: 10.1126/scitranslmed.aay7522
213. Yang Y, Mallampati S, Sun B, Zhang J, Kim S-B, Lee J-S, et al. Wnt pathway contributes to the protection by bone marrow stromal cells of acute lymphoblastic leukemia cells and is a potential therapeutic target. Cancer Lett (2013) 333(1):9–17. doi: 10.1016/j.canlet.2012.11.056
214. Lifshitz V, Priceman SJ, Li W, Cherryholmes G, Lee H, Makovski-Silverstein A, et al. Sphingosine-1-phosphate receptor-1 promotes environment-mediated and acquired chemoresistance. Mol Cancer Ther (2017) 16(11):2516–27. doi: 10.1158/1535-7163.MCT-17-0379
215. Spiegel S, Milstien S. The outs and the ins of sphingosine-1-phosphate in immunity. Nat Rev Immunol (2011) 11(6):403–15. doi: 10.1038/nri2974
216. Wang J, Cui R, Clement CG, Nawgiri R, Powell DW, Pinchuk IV, et al. Activation PDGFR-α/AKT mediated signaling pathways in oral squamous cell carcinoma by mesenchymal stem/stromal cells promotes anti-apoptosis and decreased sensitivity to cisplatin. Front Oncol (2020) 10:552. doi: 10.3389/fonc.2020.00552
217. Zhang H, Bajraszewski N, Wu E, Wang H, Moseman AP, Dabora SL, et al. PDGFRs are critical for PI3K/Akt activation and negatively regulated by mTOR. J Clin Invest (2007) 117(3):730–8. doi: 10.1172/JCI28984
218. Tu B, Zhu J, Liu S, Wang L, Fan Q, Hao Y, et al. Mesenchymal stem cells promote osteosarcoma cell survival and drug resistance through activation of STAT3. Oncotarget (2016) 7(30):48296. doi: 10.18632/oncotarget.10219
219. Wang J, Liu X, Qiu Y, Shi Y, Cai J, Wang B, et al. Cell adhesion-mediated mitochondria transfer contributes to mesenchymal stem cell-induced chemoresistance on T cell acute lymphoblastic leukemia cells. J Hematol Oncol (2018) 11(1):1–13. doi: 10.1186/s13045-018-0554-z
220. Vallabhaneni KC, Haller H, Dumler I. Vascular smooth muscle cells initiate proliferation of mesenchymal stem cells by mitochondrial transfer via tunneling nanotubes. Stem Cells Dev (2012) 21(17):3104–13. doi: 10.1089/scd.2011.0691
221. Januchowski R, Wojtowicz K, Zabel M. The role of aldehyde dehydrogenase (ALDH) in cancer drug resistance. Biomedicine Pharmacotherapy (2013) 67(7):669–80. doi: 10.1016/j.biopha.2013.04.005
222. Yuan B, El Dana F, Ly S, Yan Y, Ruvolo V, Shpall EJ, et al. Bone marrow stromal cells induce an ALDH+ stem cell-like phenotype and enhance therapy resistance in AML through a TGF-β-p38-ALDH2 pathway. PLoS One (2020) 15(11):e0242809. doi: 10.1371/journal.pone.0242809
223. Zhang Q, Yi D-Y, Xue B-Z, Wen W-W, Lu Y-P, Abdelmaksou A, et al. CD90 determined two subpopulations of glioma-associated mesenchymal stem cells with different roles in tumour progression. Cell Death Dis (2018) 9(11):1101. doi: 10.1038/s41419-018-1140-6
224. Xue B-Z, Xiang W, Zhang Q, Wang H-F, Zhou Y-J, Tian H, et al. CD90 low glioma-associated mesenchymal stromal/stem cells promote temozolomide resistance by activating FOXS1-mediated epithelial-mesenchymal transition in glioma cells. Stem Cell Res Ther (2021) 12:1–11. doi: 10.1186/s13287-021-02458-8
225. Huang W, Chang M, Tsai K, Hung M, Chen H, Hung S. Mesenchymal stem cells promote growth and angiogenesis of tumors in mice. Oncogene (2013) 32(37):4343–54. doi: 10.1038/onc.2012.458
226. Castells M, Thibault B, Mery E, Golzio M, Pasquet M, Hennebelle I, et al. Ovarian ascites-derived Hospicells promote angiogenesis via activation of macrophages. Cancer Lett (2012) 326(1):59–68. doi: 10.1016/j.canlet.2012.07.020
227. Hung S-C, Pochampally RR, Chen S-C, Hsu S-C, Prockop DJ. Angiogenic effects of human multipotent stromal cell conditioned medium activate the PI3K-Akt pathway in hypoxic endothelial cells to inhibit apoptosis, increase survival, and stimulate angiogenesis. Stem Cells (2007) 25(9):2363–70. doi: 10.1634/stemcells.2006-0686
228. Beckermann B, Kallifatidis G, Groth A, Frommhold D, Apel A, Mattern J, et al. VEGF expression by mesenchymal stem cells contributes to angiogenesis in pancreatic carcinoma. Br J Cancer (2008) 99(4):622–31. doi: 10.1038/sj.bjc.6604508
229. Bexell D, Gunnarsson S, Tormin A, Darabi A, Gisselsson D, Roybon L, et al. Bone marrow multipotent mesenchymal stroma cells act as pericyte-like migratory vehicles in experimental gliomas. Mol Ther (2009) 17(1):183–90. doi: 10.1038/mt.2008.229
Keywords: MSCs, cancer, immunosuppression, metastasis, tumor growth, angiogenesis
Citation: Hazrati A, Malekpour K, Mirsanei Z, Khosrojerdi A, Rahmani-Kukia N, Heidari N, Abbasi A and Soudi S (2023) Cancer-associated mesenchymal stem/stromal cells: role in progression and potential targets for therapeutic approaches. Front. Immunol. 14:1280601. doi: 10.3389/fimmu.2023.1280601
Received: 20 August 2023; Accepted: 11 October 2023;
Published: 03 November 2023.
Edited by:
Giuseppe Nocentini, University of Perugia, ItalyReviewed by:
Marina Kochetkova, University of South Australia, AustraliaAnn De Becker, Universitair Ziekenhuis Brussel (UZ Brussel), Belgium
Runbi Ji, Jiangsu University Affiliated People’s Hospital, China
Copyright © 2023 Hazrati, Malekpour, Mirsanei, Khosrojerdi, Rahmani-Kukia, Heidari, Abbasi and Soudi. This is an open-access article distributed under the terms of the Creative Commons Attribution License (CC BY). The use, distribution or reproduction in other forums is permitted, provided the original author(s) and the copyright owner(s) are credited and that the original publication in this journal is cited, in accordance with accepted academic practice. No use, distribution or reproduction is permitted which does not comply with these terms.
*Correspondence: Ali Hazrati, YS1oYXpyYXRpaEByYXppLnR1bXMuYWMuaXI=; QWxpaGF6cmF0aTgzM0BnbWFpbC5jb20=; Sara Soudi, c291ZGlAbW9kYXJlcy5hYy5pcg==; c291ZGlzYXJhNzBAZ21haWwuY29t