- 1Medical College, Nanchang University, Nanchang, China
- 2Office of Clinical Trials Administration, The Fourth Affiliated Hospital of Nanchang University, Nanchang, China
- 3Department of Pathology, The Fourth Affiliated Hospital of Nanchang University, Nanchang, China
- 4Tumor Immunology Institute, Nanchang University, Nanchang, China
- 5Department of General Medicine, The Second Affiliated Hospital of Nanchang University, Nanchang, China
Cancer receives enduring international attention due to its extremely high morbidity and mortality. Immunotherapy, which is generally expected to overcome the limits of traditional treatments, serves as a promising direction for patients with recurrent or metastatic malignancies. Bacteria-based vectors such as Listeria monocytogenes take advantage of their unique characteristics, including preferential infection of host antigen presenting cells, intracellular growth within immune cells, and intercellular dissemination, to further improve the efficacy and minimize off-target effects of tailed immune treatments. Listeria monocytogenes can reshape the tumor microenvironment to bolster the anti-tumor effects both through the enhancement of T cells activity and a decrease in the frequency and population of immunosuppressive cells. Modified Listeria monocytogenes has been employed as a tool to elicit immune responses against different tumor cells. Currently, Listeria monocytogenes vaccine alone is insufficient to treat all patients effectively, which can be addressed if combined with other treatments, such as immune checkpoint inhibitors, reactivated adoptive cell therapy, and radiotherapy. This review summarizes the recent advances in the molecular mechanisms underlying the involvement of Listeria monocytogenes vaccine in anti-tumor immunity, and discusses the most concerned issues for future research.
1 Introduction
Cancer constitutes a significant factor for the worldwide mortality rate. In 2023, the United States will experience 1,958,310 new cancer cases and 609,820 deaths (1). Conventional treatments such as surgery, radiotherapy, and chemotherapy still suffered from recurrence, metastasis, and drug resistance, leading to an urgent need for novel therapeutic strategies (2). Therefore, tumor immunotherapy has emerged (3), with the aim to destroy tumor cells selectively via activating or reactivating host cellular immunity mainly mediated by T cells (4). Immunomodulatory drugs can also work against cancer cells through increasing the concentration of tumor-specific antibodies, natural killer (NK) cells, dendritic cells (DCs), macrophages and cytokines. Recent studies support tumor immunotherapy as an effective strategy, which can surpass the constraints of conventional treatments and improve the prognosis of patients with different malignancies (3).
However, the efficacy of immunotherapy is greatly affected by the tumor microenvironment (5). Clinically, some patients show low immune responses and limited benefits. Thus, immunotherapy based on different vectors is expected to reshape TME. Bacteria are regarded as suitable carriers due to their immunogenicity and tropism for hypoxic tissue. Bacteria-based immunotherapy can colonize TME to effectively activate the immune system of patients who do not response to conventional treatments (6).
Listeria monocytogenes (Lm), a Gram-positive bacterium, is facultatively anaerobic. Lm-infected host exhibits mild to severe gastroenteritis, bacterial sepsis, and even bacterial meningitis (7). The virulence of Lm increases in direct proportion to its colonization and dissemination ability (8). Pore-forming toxin listeriolysin-O (LLO) facilitates the escape of Lm from the phagosome of phagocytic and nonphagocytic cells (9). Thus, Lm can be found both in the cytoplasm and endosomal compartments (10, 11). Besides, Lm has a unique life cycle and capabilities to induce a robust cytotoxic immune cell response (12). The Lm surface proteins, internalin A (inlA) and internalin B (inlB), interact with surface receptors E-cadherin and C-Met to facilitate the entrance of Lm into nonphagocytic cells (13, 14). After internalization, Lm is enclosed in the host phagosome. Through secreting phospholipases (plcA and plcB) and LLO, Lm perforates phagosomes and enters the cytoplasm to escape phagolysosome killing (15, 16). The tumor-associated antigens (TAAs) secreted by Lm undergo degradation by proteasomes, subsequently stimulating specific CD8+ T cells via MHC class I molecules (17). Owing to hypoxia, suppressive TME, and the ability to grow intracellularly, Lm is easier to escape the immune surveillance and infiltrate into TME compared to other bacteria. The apparent synergy between the expansion of effector T cells and a sharp immunosuppressive cells reduction can enhance innate immunity and restructure TME to improve the efficacy of immunotherapies. Many studies have shown significant interest in Lm and tried to make it a vector to promote tumor immunotherapy. Nevertheless, using Lm-based therapy alone still faces the problem of poor therapeutic effects and complications because of its potential pathogenicity. The combination of Lm-based therapy with other treatments has been proposed as a possible candidate to overcome the limitations. This review will focus on how Lm-based modulates immune pathways to hold a promising anti-tumor response and current progresses in Lm-based tumor immunotherapy.
2 Mechanisms of Lm-specific immunotherapy
2.1 An antigen vector
Cancer vaccines usually consist of TAAs and paired adjuvants. Bacteria-delivered TAAs activate specific cytotoxic T lymphocyte (CTLs) against tumor cells and induce memory T cells to prevent relapse. Both the pathway of Lm invasion and the ability to survive in macrophages are critical for delivering target antigens and stimulating immune responses (18, 19). Taken by oral administration or intravenous injection, Lm vaccines preferentially infect host antigen presenting cells (APCs) (7, 20). Internalized Lm can secrete LLO to enhance the permeability of the phagosome, leading to the translocation of TAAs to the cytoplasm (21). TAAs are presented by the MHC-I complex to the cell surface and activate CD8+ T cells (22). Moreover, Lm replicates and secretes actin assembly-inducing protein (actA) to polymerize host actin and facilitate its spread among cells (23, 24). These characteristics make Lm an attractive candidate as an antigen vector for immunotherapy.
A number of Lm strains expressing different antigens have been created. Lm can deliver prostate specific antigen (PSA) to increase CD8+ T cell number in spleens and tumors and inhibit regulatory T cell (Treg) allocation (25). Pancreatic ductal adenocarcinoma (PDAC) associated antigen Annexin A2 (ANXA2)-expressing Lm could activate CD8+ T cells in TME and improve the survival of the rodent models of PDAC (26). Additionally, a fusion of Lm antigens and TAAs enhances anti-tumor responses. Given the ability to perforate phagosomes and help Lm spread from cell to cell, modified LLO and actA are mostly employed as fusion partners. Although the human papillomavirus (HPV) antigen E7-expressing strain alone has almost no impact on tumor growth, the combination of Lm-derived E7 and truncated version LLO (tLLO) results in tumor regression in up to 75% of patients (27). Similar to tLLO, the fusion of truncated actA with E7 also shows enhanced anti-tumor effects (28).
2.2 Effects on immune cells in TME
One of the main reasons why many immunotherapies fail to provide therapeutic benefit is that the immunosuppressive microenvironment impedes anti-tumor responses. Tregs and myeloid-derived suppressor cells (MDSCs) in TME are primarily responsible for T cell inhibition and rapid depletion of tumor-specific T cells (29–31). The innate immune responses to Lm, including the effects of NK cells, tumor-associated macrophages (TAMs), mast cells, and neutrophils, contribute to TME remodeling (Figure 1).
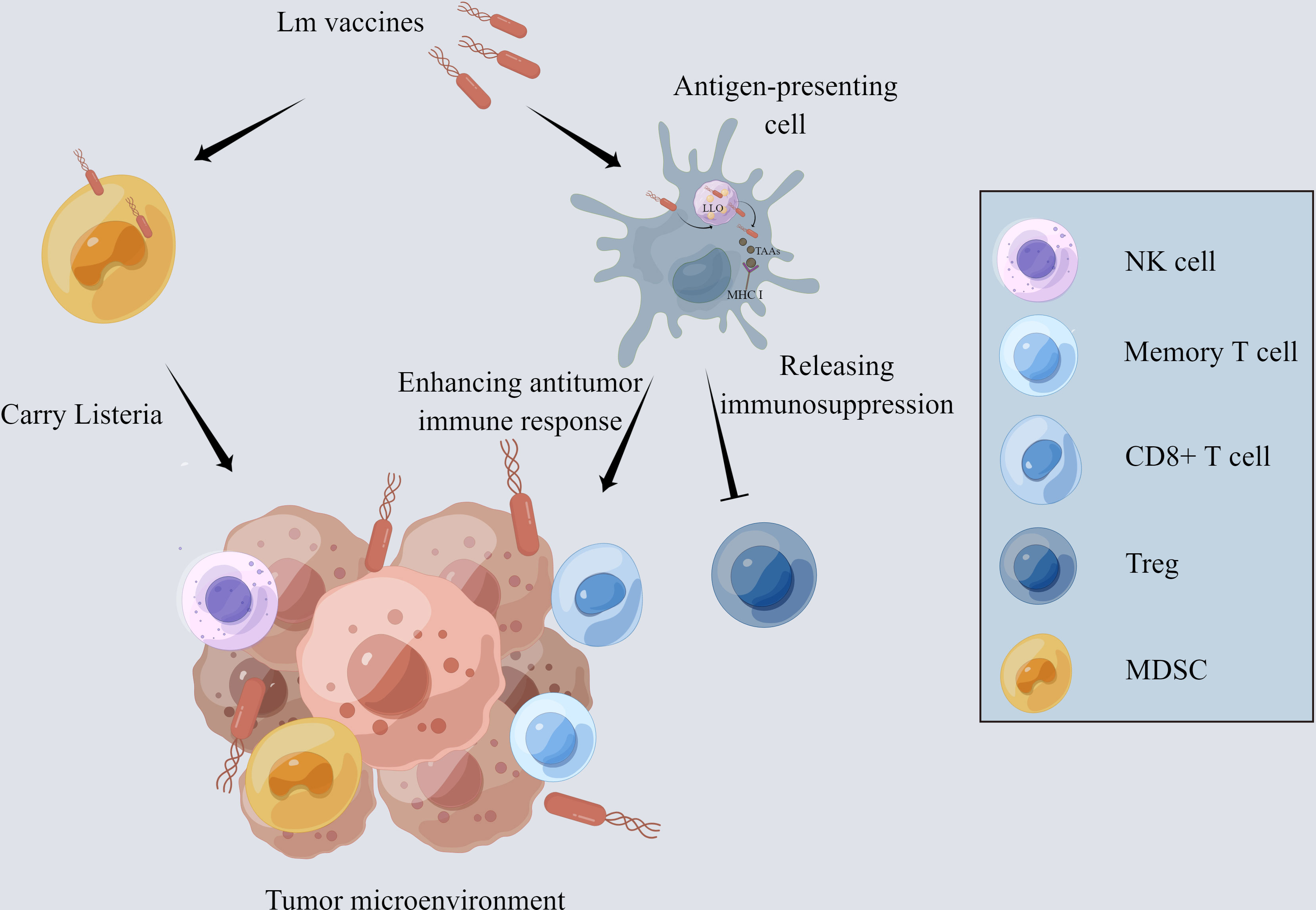
Figure 1 The mechanism of Lm-based vaccine affecting tumor microenvironment. The Lm-based vaccine is phagocytosed by antigen-presenting cells and escapes the phagosome by releasing the pore-forming toxin listeriolysin-O. MHCI takes up the tumor-associated antigens delivered by Lm-based vaccine and presents it on the cell surface, which activates the anti-tumor immune response of CD8+ T cells. In addition, the number and activity of memory T cells and NK cells are increased while decreasing the number and activity of Treg cells and reducing the immunosuppression in tumor microenvironment. Lm-based vaccine also directly infects MDSCs and spread to the TME with the aid of MDSCs.
Recent findings indicate that Lm vaccines promote the infiltration of IFN-γ-producing effector CD8+ T cells and a remarkable decrease in the frequency of FoxP3+ Tregs, transforming the immunosuppressive TME into inflamed and leading to the inhibition of tumor development (32, 33). Additionally, interferon-stimulated gene 15 (ISG15) exerts a key function in innate immunity. Lm- LLO-ISG15 induces the production of interferon-γ, attracting effector T cells to TME (34). Besides, Lm-based vaccines can also induce tumor-associated macrophage polarization from M2 to M1 with anti-tumor phenotype (35). Lm infection activates neutrophils and mast cells, which, in turn, secret IL-6 and IL-13 to enhance the host immune response (36, 37).
Moreover, Lm vaccines can increase the number and activity of NK cells which perform strong anti-tumor action through targeting and lysing tumor cells (32, 33, 38). Lm vaccine can also reduce the number of MDSCs through direct infection both in the peripheral blood and TME (39, 40). Infected MDSCs can transform into immune-stimulating phenotype and secrete IL-12 to activate the CD8+ T cells and immune responses (41).
In addition, Lm vaccine can target tumor-associated blood vessels through the surface antigens of endothelial cells (42). Endoglin (CD105), a necessary glycoprotein of the TGF-β receptor complex, has been proposed as an available marker for tumor-related angiogenesis and neovascularization. Accumulating evidence supports the high efficacy of CD105-targeted Lm vaccine in breast cancer models through stimulating anti-angiogenic and anti-tumor immune responses (43). CD105 is expressed on both tumor cells and endothelial cells in renal cell carcinoma (RCC) (44, 45). Lm-LLO-CD105A encodes a CD105 antigen fragment that targets RCC tumor cells as well as tumor-related blood vessels. The vaccine can reduce the progression of RCC in subcutaneous and orthotopic models by increasing the infiltration of polyfunctional CD8+ and CD4+ T cells (46).
Although the Lm vaccine has shown significant effects on remodeling TME and increasing the anti-tumor effects of specific T cells, there are still a series of concerns that need to be addressed before its clinical practice. Despite the utilization of attenuated strains, components of Lm still can elicit the body immunity and stimulate macrophages to phagocytize the bacteria. In addition, most bacteria-based vaccines are inevitably cleared by the reticuloendothelial system before landing on the tumor, ultimately with a lower-than-expected anti-tumor efficacy.
3 Development of attenuated Lm strains
The pathogenicity of Lm constitutes a significant constraint for clinical applications. Therefore, the delicate balance between the safety and immunotherapeutic efficacy of the candidates is a fundamental aspect of Lm-based strategies.
Selectively deleting virulence genes of wild type Lm, including inlB, actA, alanine racemase (dal), and D-amino acid aminotransferase (dat), has been widely used to develop an ideal vector. InlB directly mediates the infection of nonphagocytic cells in vitro, while actA promotes a cell-to-cell spread pattern (23, 47). The actA (ΔactA) and inlB (ΔinlB) double-deleted Lm strain cannot be transmitted between cells and exhibit a reduced potential to infect hepatocytes directly or indirectly, but the ability to stimulate innate immunity is intact (48). Meanwhile, the eradication of ΔactA/ΔinlB Lm strain from the liver and spleen is much faster than that of a single mutant strain (49). Moreover, attenuated ΔactA/ΔinlB Lm strain shifts the phenotype of TAMs from an inhibitory (M2) to a stimulatory state (M1), leading to good prognosis in mouse model with aggressive ovarian carcinoma (48). D-alanine is an essential component for the synthesis of the mucopeptide in Lm cell walls, which is controlled by two important genes, dal and dat (50). After inactivating both genes, the replication of Lm must be dependent on exogenous D-alanine (50). The dual deficient strain acquires dal and dat genes from Bacillus subtilis to restart the replication within a limited degree without serious organ damage (33).
Developing killed but metabolically active (KBMA) bacteria also represent a promising pathway. Brockstedt et al. created a nucleotide excision repair gene-deleted Lm vaccine (KBMA Lm), which is highly sensitive to photochemical inactivation induced by psoralen and long-wave UV (38). The anti-tumor KBMA Lm stimulates effective CD4+ and CD8+ T cell responses and an increase in the number of mature DCs in colon cancer model without significantly side effects (51, 52).
Lm recombinase-induced intracellular death (Lm-RIID) is a novel vaccine platform. By inducing Cre recombinase in host cell cytosol to delete essential genes for bacterial viability, Lm-RIID commits suicide intracellularly. As a result, Lm-RIID can elicit potent anti-tumor effects without normal tissue injury. Besides, Lm-RIID exhibits a higher clearance rate than double-deleted Lm strains. The development of Lm-RIID marks a significant advancement in the progress of Lm-based vaccine (53).
ISG15 is considered as a new TAA and therapeutic target for colorectal cancer. Lm-LLO-ISG15 mediates CD4+ T and CD8+ T cell responses through a greater ratio of effector to regulatory T cells in TME (54). Moreover, manganese ions (Mn2+) have been found to promote the activation and infiltration of CD8+ T cells to kill tumor cells in vivo, while calcium ions (Ca2+) can regulate autophagy to facilitate the cross-presentation of antigens (55, 56). Calcium-doped manganese carbonate microspheres and LLO (Ca@MnCO (3)/LLO) are used to form a manganese-containing multimode vaccine delivery system, which facilitates antigen cross-presentation, induces proliferation of CD8+ T cells, and ultimately yields significant anti-tumor effects (57).
Various methods have been employed to control the virulence of Lm vaccine and ensure its safety. The levels of biological materials or drugs bound to the bacterial surface do not increase with the replication of vaccine, resulting in the dilution of effective concentration. Further studies could focus on the interactions between the bacterial vector and therapeutic drugs. In addition, with regard to the risk of triggering bacteremia in the host, it would be remarkably cautious to the complications of Lm. In conclusion, how to ensure the maximum efficacy of Lm vaccines at a safe dose is still the core issue in current vaccine development.
4 Synergy with other therapies
It has been established that carcinogenesis is largely the result of mutations in multiple genes with strong individual differences. Although Lm-based vaccines have shown regulatory effects on cancer immunity, not all patients can benefit from a single cancer vaccine. Thus, Lm-based vaccines are also used in combination with immune checkpoint inhibitors, reactivated adoptive cell therapy, and radiotherapy to achieve greater efficacy with lower side effects (Figure 2) (Table 1).
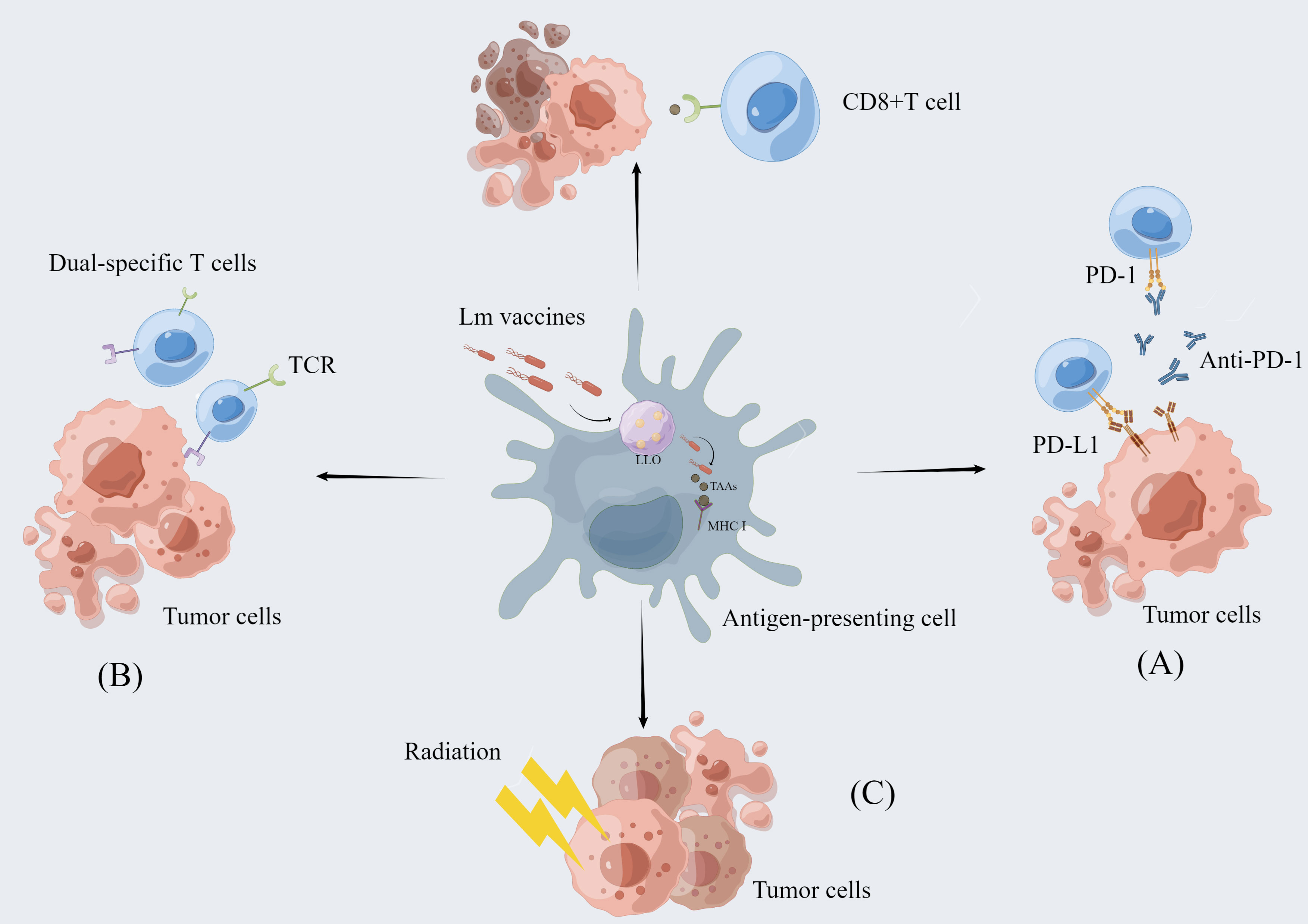
Figure 2 The combination of Lm-based vaccine and other therapies. Lm-based vaccines can be combined with multiple therapies to realize more potent anti-tumor effects. (A) Combination with immune checkpoint inhibitor therapy: Immune checkpoint inhibitors enhance the anti-tumor response of T cells by blocking the binding of checkpoint proteins to partner proteins, such as blocking the binding of PD-1 to PD-L1. Lm-based vaccines reduce immunosuppression by remodeling the tumor microenvironment while activating CD8+ T-cell immune responses to exert synergistic antitumor effects with ICI. (B) Re-energized Adoptive Cell Therapy: The ability of Lm-based vaccines to reduce the immunosuppressive environment in combination with the dual-specific T cells are tumor-reactive CD8+ T cells enhances tumor infiltration and function of CD8+ T cells. (C) Combined application with radiotherapy: Radiotherapy can directly cause tumor cell death and Lm-based vaccine can retard tumor growth. The combination of the two therapies exerts synergistic anti-tumor effect.
4.1 Immune checkpoint inhibitors
As one of the most important components of the immune system, immune checkpoints can prevent healthy cells from excessive immune responses. Meanwhile, immune checkpoints can limit the potency of activated T cells through the interactions between T cells and partner proteins on target cells such as tumor cells (74). The most studied immune checkpoint molecules include cytotoxic T-lymphocyte–associated antigen 4 (CTLA-4) and programmed cell death protein-1 (PD-1) (75–78). Cancer cells express PD-L1, the ligand of PD-1, enabling the tumor to evade assault from effector T cells (79). Immune checkpoint inhibitors (ICI) refer to the utilization of blocking antibodies targeting the checkpoint molecules PD-1/PD-L1 and CTLA-4. ICI can restore the recognition and killing efficacy of immune cells and prevent the immune evasion of tumor cells (80, 81). However, separate use of ICI may not be effective for some types of cancer such as PDAC (26). The combination of blockading checkpoint protein with Lm-based immunotherapy has shown positive clinical effectiveness in many types of cancer, including melanoma and hepatocellular carcinoma (35, 81).
Lm-based vaccines can reinforce immune checkpoint blockade function to fight against cancer, mainly via ameliorating the immunosuppressive TME and inducing anti-tumor immune responses. Lm-LLO-E7 vaccine, formed by HPV16-E7 and truncated LLO, can enhance the efficacy of anti-PD-1 antibodies by reducing the number of Tregs and MDSCs in the spleen and TME (27, 39). ANXA2 favors the metastasis of PDAC and induces antibody responses (82). Compared with the single treatment, a combination of Lm-ANXA2 and anti-PD-1 antibody significantly prolonged survival time in PDAC model. Moreover, when Lm-ANXA2 is administered prior to the use of anti-PD-1 antibody, cure rates in implanted PDAC models are increased (26). A Lm-based hepatocellular carcinoma (HCC) vaccine, ΔdalΔdat Lm-multiple peptides fusing genes (MPFG), with the ability to secrete HCC-related TAAs fragments, activates the TAMs through the NF-κB pathway and shifts the cytokine profiles within TME towards an antitumor response. This alteration reinstates the T cell reactivity towards the anti-PD-1 blockade (35, 73). The Lm-GP61 vaccine developed using the CD4+ T cell epitopes can impede tumor progression by inducing TH1 and CTL responses, synergizing PD-L1 blockade to mediate tumor suppression (83). In a mouse melanoma model, ICI synergized with an Lm-based melanoma vaccine to induce protective primary and memory T-cell responses through antigen-specific CD8+ T cells (62).
Nevertheless, the clinical utility of ICI remains restricted due to unresolved obstacles. Owing to tumor heterogeneity, a part of patients with responsive forms of cancer even exhibit no response to current ICI (84). Moreover, in spite of the strong association between immune checkpoint molecule expression and tumor progression, the lack of predictive biomarkers narrows down the benefit population. And it is controversial to use immune checkpoint molecule as the only predictive biomarkers for tumor immunotherapy (85). Hence, in view of this problem, some studies found other factors such as a T cell inflamed gene-expression profile (GEP) and tumor mutational burden (TMB) that can now be used as biomarkers to predict the responsiveness of ICI therapy (86).
4.2 Reenergized adoptive cell therapy
Adoptive cell therapy (ACT) using naturally or engineered immune cells has unprecedented success in oncotherapy, such as hematopoietic malignancies and melanoma (87–89). ACT is dependent on an adequate quantity of anti-tumor T cells with the requisite capabilities to cause cancer regression (90). Thus, the immunosuppressive TME may become an obstacle to progress in enhancing the efficacy of ACT (91). Considering the ability of Lm-based vaccines to infect and deplete MDSCs in TME, it may be the viable option that overcomes the limitations and enhances the efficacy of ACT (92).
Xin et al. developed a new strategy called Reenergized ACT (ReACT) which combines ACT with Lm-based vaccine, integrating the advantages of two methods (64). The bacteria/tumor-(dual) specific T cell is tumor-reactive CD8+ T cell that has been assembled in vitro with an extra T-cell receptor against a bacterial antigen. And the successful eradication of tumors relies on the substantial T cells within the tumor. The use of Lm-based vaccine leads to a significant reduction of MDSCs in TME to increase the intensity of specific T cell action, which makes the dual- specific T cells expand and migrate to the tumor area (64). The study in rodents demonstrated that ReACT enhanced the infiltration and function of CD8+ T cells in tumors, as well as reduced the expression of immune checkpoint molecules (93). In preclinical cancer models, ReACT has exhibited a fascinating efficacy in eradicating the primary tumor and reducing recurrences in the long term (93).
Despite the powerful effects, ReACT still faces some limitations. Local injection, which is used to ensure access to tumor tissues, may restrict the clinical application. Meanwhile, ReACT-induced immune responses may target self-antigens, resulting in normal cell and tissue injury. These concerns may become an important area of experimental and clinical investigation in the years to come.
4.3 Radiotherapy
Radiotherapy (RT) was shown to increase susceptibility of tumor cells to active vaccine therapy. Sublethal irradiation promoted the recruitment of infiltrating T cells in the treatment sites. The phenotype of tumor cells can be changed and more sensitive to Lm-based therapy (94, 95). Given the anti-tumor effects of radiation and Lm-based vaccine, these two methods can better control tumor development (63). Different immune responses elicited by different treatments have a synergistic effect. Combination of RT and chicken ovalbumin (OVA)-expressing ΔactA/ΔinlB Lm (Lm-OVA) increased the number of activated T cells in tumor tissues compared with either alone (63). Similarly, the combined administration of RT and Lm-based prostate cancer vaccine (ADXS31-142) accelerates tumor regression through enhanced specific immune responses in the prostate (68). Through coupling 188Rhenium and attenuated (at) Lm (Listeriaat), Quispe-Tintaya et al. created a unique radioactive Listeriaat (RL) (96). RL delivered a high level of radioactive payload to metastatic colonies causing malignant cell death without normal tissue damage, holding great promise for controlling metastases. However, further clinical trials are still needed to determine their efficacy.
5 Application of Lm-based vaccine in solid tumors
Lm-based vaccines have been tested in many preclinical and clinical trials for different tumors, including cervical cancer, melanoma, pancreatic cancer, breast cancer, prostate cancer, and malignant pleural mesothelioma (Tables 1, 2).
5.1 HPV-associated cervical cancer
Chronic infection with HPV, especially type 16, is the main risk factor for the development of cervical cancer, the fourth most common cancer in women (100). The efficacy of current therapeutic interventions still acquires extensive substantiation (101). Due to the poor prognosis, there remains a lack of consensus regarding the second-line alternatives (102).
Axalimogen filolisbac (ADXS11-001), a new vaccine based on living attenuated Lm, is consisted with LLO and HPV-16 E7 antigen. ADXS11-001 can activate special immune responses to the E7-expressing malignant cells (58). It also increases the number of tumor infiltrating lymphocyte (TIL) and alleviates the immunosuppression status of TME (103). The results obtained from phase I/II/III trials encourage future perspectives for cervical cancer patients (58).
Accumulating evidence confirms that the combination of two different recombinant Lm strains exhibits a more satisfactory anti-tumor potential than using them alone. Treating HPV-infected mice with LMΔactAplcB-E6E7 (LMΔ-E6E7) and LIΔactAplcB-E6E7 (LIΔ-E6E7) can significantly overcome anti-vector immunity and accelerate the regression of tumor than the effect of LIΔ−E6E7 (11). In addition, some studies demonstrated that the optimization of codon usage contributes to the improvement of host immunity against TAAs (104, 105). The codon-optimized LM4Δhly::E7-1 induced stronger Th1-biased immunity, lymphocyte proliferation, and specific CTL activity compared with LM4Δhly::E7. Moreover, LM4Δhly::E7 exhibited a significant improvement in the efficacy of tumor establishment treatment (59).
5.2 Melanoma
Melanoma has been proposed as the most aggressive type of skin cancer originating from melanocytes. Attenuated ΔactA/ΔinlB Lm, capable of expressingmelanoma inhibitory activity (MIA), can inhibit the growth of melanoma through the downregulation of blood vessel density (106). The human high molecular weight melanoma-associated antigen (HMW-MAA)-expressing Lm (Lm-LLO-HMW-MAA-C) acquires the ability to induce cell-mediated immune responses against HMW-MAA, targeting both tumor cells and pericytes in the tumor vascular system (60).
Non-targeting Lm still demonstrates the ability to induce melanoma cell death without specific target antigen. Lmat-LLO, which produces ROS and causes a wide range of melanoma cell apoptosis, significantly reduced the size, volume, and metastatic burden of melanoma in Braf/Pten genetically engineered mice (61). The OVA-expressing Lm with the deletion of actA and phospholipase C stimulated strong CD8+ T cell responses including activation of both primary and memory T cells, resulting in protection against melanoma in mouse model transplanted with B16F10 cell line (62). Moreover, in combination with ICI treatment or RT, Lm vaccine increases the infiltration of antigen-specific CD8+ T cell and NK cells and shows better effects on reducing tumor size (62, 63).
5.3 Pancreatic cancer
Despite the recent advances in diagnosis and treatment, PDAC is still the fourth contributor to deaths from cancer (107). Gemcitabine (GEM) and erlotinib add up to six months to the median survival of patients with advanced PDAC (108, 109). It is difficult for many therapeutics to reach the tumor site due to the stromal barrier of PDAC (110, 111). With the ability to use MDSC as a vector, Lm could penetrate the primary tumor, which is considered to be a more efficient anti-tumor strategy (96).
Lm-expressing mesothelin (CRS-207) is of great clinical importance for Lm-based PDAC treatment. The administration of low-dose cyclophosphamides (Cy) prior to GM-CSF-secreting allogeneic pancreatic tumor cells (GVAX) (Cy/GVAX) followed by CRS-207 leads to a significant improvement in outcomes in a randomized multicenter phase II study with manageable toxicity (98, 99, 112). However, this strategy (Cy/GVAX + CRS-207) failed to improve the overall survival in a randomized phase IIB study compared to chemotherapy (113). This apparent paradox might be explained by the fact that nontargeted immunosuppressive vaccines are quite difficult to obtain ideal anti-tumor effects. Thus, current clinical trials focus on the combination of the prime-boost vaccination strategy and immune checkpoint inhibitors. Although these issues are a matter of debate, the current study using PD-1 inhibitor (nivolumab) + Cy/GVAX + CRS-207 yielded valuable insights into this field (71, 72).
Immunogenic tetanus toxoid protein (TT(856–1313)) can be delivered directly into PDAC through Lm vaccine and kill infected tumor cells via reactivating previously existing TT-specific memory T cells. Through intraperitoneal injection, Lm directly infects MDSCs which will migrate into TME, leading to the dissemination of Lm. Moreover, Listeria-TT regulates the number and function of macrophages and MDSCs, thus enhancing the sensitivity of tumor to GEM (40). At the same time, it avoids the side effects accompanying the use of high-dose GEM due to reduced sensitivity (40).
Selvanesan et al. developed an attenuated non-toxic and non-pathogenic Listeria-32P as a novel delivery platform, which kills tumor cells by 32P-induced ionizing radiation and Lm-induced ROS (114). The treatment causes a reduction in the growth of PDAC in KPC (conditionally express endogenous Kras-G12D, p53-R172H and pdx1-Cre mutant alleles) mice. Meanwhile, the radioactive Lm can precisely pinpoint the metastatic lesions in distant organs with minimal side effects to the adjacent normal tissue (114).
Besides, the combination of Lm-based immunotherapy with other treatments, such as radiotherapy, shows a promising anti-tumor effect and has a clinical future to prevent recurrence and metastasis of pancreatic cancer (96).
5.4 Breast cancer
Breast cancer (BC) is one of the most common malignancies in women. More than 20% of patients died of metastatic lesions and intervention resistance (115, 116). First-line strategy for metastatic cancer so far is surgery followed by chemotherapy or radiation (117). Despite the recent advances in BC therapy, the elimination of metastatic or primary tumor cells after initial treatment is often incomplete (118). More aggressive strategies are required, but few options are available, resulting in an urgent need for other effective measures. Immunotherapy has shown a promising perspective and can be served as an important candidate for BC patients.
The administration of Mage-b cDNA-expressing Lm (LM-LLO-Mage-b/2nd) before the establishment of tumor shows a more effective function of eliminating metastases than that of Lm-LLO in 4T1 BC model (65). The TAA Mage-b-expressing Lm (Lm-Mage-b) is combined with some immunologic adjuvants to promote a strong immune response. Lm-Mage-b can synergize with α-galactosylceramide and contribute to the increase of NK T cells in the spleen and the elimination of metastatic colonies without cellular toxicity (66). Triple-negative BC (TNBC) represents an extremely aggressive subtype that lacks estrogen receptor, progesterone receptor, and human epidermal growth factor receptor 2 (HER-2) (119, 120). Curcumin inhibits the production of MDSCs-derived IL-6 and enhances the effects of Lm-Mage-b through strong CD8+ T cell responses, resulting in a higher level of efficacy against metastases (121). Listeriaat can decrease the number of infected MDSCs in blood and primary tumors. IL-12 is one of the signals to stimulate clonal expansion of CD8+ T cells (122).
5.5 Prostate cancer
It is estimated that 288,300 new cases of prostate cancer and 34,700 death cases will occur in 2023 (1). Up to 20% of men diagnosed with prostate cancer in the United States have regional or metastatic disease (123). Most of these patients who are treated with 1-3 years androgen-deprivation therapy will finally develop metastatic castration-resistant prostate cancer (124).
PSA can be detected in the majority of prostate cancer cases and has been identified as the target antigen (25). ADXS31-142, a live attenuated Lm-based immunotherapy, is bioengineered to secrete a fusion protein composed of truncated fragments of Lm lysozyme toxin (tLLO) and PSA, termed tLLO-PSA (67). A study demonstrated that the combination of ADXS31-142 and pembrolizumab is safe and well tolerated in patients with metastatic castration-resistant prostate cancer (67).
JNJ-64041809 (JNJ-809), a newly developed immunotherapy based on ΔactA/ΔinlB Lm, targets four prostate cancer antigens, including prostatic acid phosphatase (125), prostate-specific membrane antigen (126), synovial sarcoma X breakpoint 2 (127), and homeobox protein NKX3.1 (128). After the evaluation, the safety of JNJ-809 is manageable and the interventions implemented at an early stage may induce a more intense response. However, the observable antigen-specific immune response is limited and did not translate into an objective clinical response (69).
5.6 Malignant pleural mesothelioma
Exposure to asbestos or other small carcinogenic fibers is most likely responsible for malignant pleural mesothelioma (MPM), a rare malignancy with a poor prognosis (129). Although the combination of pemetrexed and cisplatin has been applied as standard initial treatment for patients with unresectable MPM (130), the high mortality has been promoting the search of alternative treatments (70). Accumulating evidence suggests that immunotherapeutic approaches can be used as promising treatments for MPM (131–134).
Almost all epithelial MPMs overexpress mesothelin (120, 135–137). When combined with chemotherapy, CRS-207 increases infiltration of dendritic NK cells and T cells, accompanied by a transition of macrophage from immunosuppressive M2 to proinflammatory M1 (70). In a phase Ib study in MPM, CRS-207 in combination with pemetrexed/cisplatin increases CD8+ T cell ratio and the infiltration of DCs and NK cells. The therapy leads to a notable reduction in tumor size without serious treatment-associated side effects (70). Besides, cytoreduction surgery could reduce the immunosuppression to restore the mesothelin-expressing Lm vaccine efficacy (138).
6 Future perspectives
By overcoming the immunosuppressive microenvironment and enhancing targeted anti-tumor immune responses, Lm vaccines have shown promising performance in the treatment of both primary and metastatic tumors.
However, the side effects of Lm vaccines are similar to other classical immunotherapies, including potential systemic immune response, hypertension, and fatigue. As a pathogen, Lm has raised concerns about its safety risks, even bacteremia. Several studies have explored the delicate balance between the efficacy and safety of Lm-based vaccines (69, 97, 99, 139, 140). At the same time, a lack of technical facilities limits the ability to test whether a sufficient number of vaccines can reach the tumor site.
Therefore, future research will focus on the development of less toxic and more potent Lm strains. At present, this target is mainly achieved by deleting virulence factors or developing KBMA and Lm-RIID strain. More effective evaluation methods are generally expected to select the best candidates among developed strains. In addition, several studies have explored the combined use of Lm-based vaccines with ICI, ReACT cells, radiotherapy, and other therapies, achieving remarkable results. The ability of Lm-based vaccine to reshape TME and enhance the immune response of CD8+ T cells endows it to exert synergistic anti-tumor effects. Meanwhile, how the Lm-based vaccine helps other approaches to regulate the immune status of TME may become an important area of clinical investigation in years to come.
The differences of immune system between human and experimental species remain a big obstacle to progress in developing Lm-based vaccines. Robust CD8+ T cell immunity can be elicited by Lm-based vaccine to specifically target a tumor antigen in mouse model. However, this result has not been confirmed in human so far. Substantial evidence suggests the possibility that the differences of γδ T cells between mouse and human may contribute to this phenomenon. According to the expression of γδ receptors, T cells can be divided into two subtypes, including γδ T cells (γδ+) and αβ T cells (γδ-) (141). Vγ9Vδ2 T cells represent the majority of human γδ T cells while murine γδ T cells are largely Vγ5Vδ1+ (142, 143). Through upregulation of cholesterol metabolism, Lm-infected human dendritic cells are able to activate Vγ9Vδ2 T cells. And in contrast to mouse, Lm infection in humans induces a distinct proliferation of Vγ9Vδ2 T cells (144). Besides, colorectal cancer antigen guanylyl cyclase C (Lm-GUCY2C)-expressing Lm-based vaccine can induce strong Lm-specific immunity, rather than anti-GUCY2C response. This result indicates that the competition with immunodominant Lm-derived CD8+ T-cell epitope may be involved. Some weak antigens, such as GUCY2C, may exhibit susceptibility to the competition from Lm-derived peptides (145). Despite recent advances, the underlying mechanisms of Lm-derived peptides may explain the unresolved problem in the setting of different species. Further research is significant to explore the synergy between existed vaccines and other treatments.
Author contributions
YD: Formal Analysis, Project administration, Writing – original draft, Writing – review & editing. LS: Investigation, Writing – review & editing. RH: Writing – review & editing. KC: Writing – review & editing. YD: Writing – review & editing. ZZ: Writing – review & editing. YX: Funding acquisition, Resources, Supervision, Validation, Writing – review & editing. HD: Funding acquisition, Resources, Supervision, Validation, Writing – review & editing.
Funding
The author(s) declare financial support was received for the research, authorship, and/or publication of this article. This work was supported by the National Science Foundation of China, No.82160546; the Science Foundation of Jiangxi Province, No. 20202BBG73027, 20202ACBL206017, and 20192BAB205015; the Foundation of Jiangxi Province for Distinguished Scholars No. JXSQ2023201020.
Acknowledgments
The authors would like to give special thanks to the Figdraw platform for providing vector drawing materials, which greatly improved the quality and efficiency of our work. The ID of figures by Figdraw are SUUT7d58d and OUSWT7a4b4.
Conflict of interest
The authors declare that the research was conducted in the absence of any commercial or financial relationships that could be construed as a potential conflict of interest.
Publisher’s note
All claims expressed in this article are solely those of the authors and do not necessarily represent those of their affiliated organizations, or those of the publisher, the editors and the reviewers. Any product that may be evaluated in this article, or claim that may be made by its manufacturer, is not guaranteed or endorsed by the publisher.
References
1. Siegel RL, Miller KD, Wagle NS, Jemal A. Cancer statistics, 2023. CA: Cancer J Clin (2023) 73(1):17–48. doi: 10.3322/caac.21763
2. Allemailem KS. Innovative approaches of engineering tumor-targeting bacteria with different therapeutic payloads to fight cancer: A smart strategy of disease management. Int J Nanomed (2021) 16:8159–84. doi: 10.2147/ijn.S338272
3. Esfahani K, Roudaia L, Buhlaiga N, Del Rincon SV, Papneja N, Miller WH Jr. A review of cancer immunotherapy: from the past, to the present, to the future. Curr Oncol (2020) 27(Suppl 2):S87–s97. doi: 10.3747/co.27.5223
4. Lahiri A, Maji A, Potdar PD, Singh N, Parikh P, Bisht B, et al. Lung cancer immunotherapy: progress, pitfalls, and promises. Mol Cancer (2023) 22(1):40. doi: 10.1186/s12943-023-01740-y
5. Spohn G, Guler R, Johansen P, Keller I, Jacobs M, Beck M, et al. A virus-like particle-based vaccine selectively targeting soluble Tnf-Alpha protects from arthritis without inducing reactivation of latent tuberculosis. J Immunol (2007) 178(11):7450–7. doi: 10.4049/jimmunol.178.11.7450
6. Fan JY, Huang Y, Li Y, Muluh TA, Fu SZ, Wu JB. Bacteria in cancer therapy: A new generation of weapons. Cancer Med (2022) . 11(23):4457–68. doi: 10.1002/cam4.4799
7. Radoshevich L, Cossart P. Listeria monocytogenes: towards a complete picture of its physiology and pathogenesis. Nat Rev Microbiol (2018) 16(1):32–46. doi: 10.1038/nrmicro.2017.126
8. Bolhassani A, Naderi N, Soleymani S. Prospects and progress of listeria-based cancer vaccines. Expert Opin Biol Ther (2017) 17(11):1389–400. doi: 10.1080/14712598.2017.1366446
9. Wallecha A, Carroll KD, Maciag PC, Rivera S, Shahabi V, Paterson Y. Multiple effector mechanisms induced by recombinant listeria monocytogenes anticancer immunotherapeutics. Adv Appl Microbiol (2009) 66:1–27. doi: 10.1016/s0065-2164(08)00801-0
10. Portnoy DA, Jacks PS, Hinrichs DJ. Role of hemolysin for the intracellular growth of listeria monocytogenes. J Exp Med (1988) 167(4):1459–71. doi: 10.1084/jem.167.4.1459
11. Su L, Zhang Y, Zhang X, Liu T, Liu S, Li Y, et al. Combination immunotherapy with two attenuated listeria strains carrying shuffled hpv-16 E6e7 protein causes tumor regression in a mouse tumor model. Sci Rep (2021) 11(1):13404. doi: 10.1038/s41598-021-92875-9
12. Oladejo M, Paterson Y, Wood LM. Clinical experience and recent advances in the development of listeria-based tumor immunotherapies. Front Immunol (2021) 12:642316. doi: 10.3389/fimmu.2021.642316
13. Shen Y, Naujokas M, Park M, Ireton K. Inib-dependent internalization of listeria is mediated by the met receptor tyrosine kinase. Cell (2000) 103(3):501–10. doi: 10.1016/s0092-8674(00)00141-0
14. Mengaud J, Ohayon H, Gounon P, Mege RM, Cossart P. E-cadherin is the receptor for internalin, a surface protein required for entry of L. Monocytogenes into epithelial cells. Cell (1996) 84(6):923–32. doi: 10.1016/s0092-8674(00)81070-3
15. Smith GA, Marquis H, Jones S, Johnston NC, Portnoy DA, Goldfine H. The two distinct phospholipases C of listeria monocytogenes have overlapping roles in escape from a vacuole and cell-to-cell spread. Infect Immun (1995) 63(11):4231–7. doi: 10.1128/iai.63.11.4231-4237.1995
16. Hamon MA, Ribet D, Stavru F, Cossart P. Listeriolysin O: the swiss army knife of listeria. Trends Microbiol (2012) 20(8):360–8. doi: 10.1016/j.tim.2012.04.006
17. Flickinger JC Jr., Rodeck U, Snook AE. Listeria monocytogenes as a vector for cancer immunotherapy: current understanding and progress. Vaccines (2018) 6(3):48. doi: 10.3390/vaccines6030048
18. da Silva AJ, Zangirolami TC, Novo-Mansur MT, Giordano Rde C, Martins EA. Live bacterial vaccine vectors: an overview. Braz J Microbiol (2014) 45(4):1117–29. doi: 10.1590/s1517-83822014000400001
19. Singh R, Wallecha A. Cancer immunotherapy using recombinant listeria monocytogenes: transition from bench to clinic. Hum Vaccin (2011) 7(5):497–505. doi: 10.4161/hv.7.5.15132
20. Stavru F, Archambaud C, Cossart P. Cell biology and immunology of listeria monocytogenes infections: novel insights. Immunol Rev (2011) 240(1):160–84. doi: 10.1111/j.1600-065X.2010.00993.x
21. Barry RA, Bouwer HG, Portnoy DA, Hinrichs DJ. Pathogenicity and immunogenicity of listeria monocytogenes small-plaque mutants defective for intracellular growth and cell-to-cell spread. Infect Immun (1992) 60(4):1625–32. doi: 10.1128/iai.60.4.1625-1632.1992
22. Ikonomidis G, Paterson Y, Kos FJ, Portnoy DA. Delivery of a viral antigen to the class I processing and presentation pathway by listeria monocytogenes. J Exp Med (1994) 180(6):2209–18. doi: 10.1084/jem.180.6.2209
23. Kocks C, Gouin E, Tabouret M, Berche P, Ohayon H, Cossart PL. Monocytogenes-induced actin assembly requires the acta gene product, a surface protein. Cell (1992) 68(3):521–31. doi: 10.1016/0092-8674(92)90188-i
24. Tilney LG, Portnoy DA. Actin filaments and the growth, movement, and spread of the intracellular bacterial parasite, listeria monocytogenes. J Cell Biol (1989) 109(4 Pt 1):1597–608. doi: 10.1083/jcb.109.4.1597
25. Shahabi V, Reyes-Reyes M, Wallecha A, Rivera S, Paterson Y, Maciag P. Development of a listeria monocytogenes based vaccine against prostate cancer. Cancer Immunol Immunother: CII (2008) 57(9):1301–13. doi: 10.1007/s00262-008-0463-z
26. Kim VM, Blair AB, Lauer P, Foley K, Che X, Soares K, et al. Anti-pancreatic tumor efficacy of a listeria-based, annexin A2-targeting immunotherapy in combination with anti-Pd-1 antibodies. J Immunother Cancer (2019) 7(1):132. doi: 10.1186/s40425-019-0601-5
27. Gunn GR, Zubair A, Peters C, Pan ZK, Wu TC, Paterson Y. Two listeria monocytogenes vaccine vectors that express different molecular forms of human papilloma virus-16 (Hpv-16) E7 induce qualitatively different T cell immunity that correlates with their ability to induce regression of established tumors immortalized by Hpv-16. J Immunol (2001) 167(11):6471–9. doi: 10.4049/jimmunol.167.11.6471
28. Wood LM, Pan ZK, Shahabi V, Paterson Y. Listeria-derived acta is an effective adjuvant for primary and metastatic tumor immunotherapy. Cancer Immunol Immunother: CII (2010) 59(7):1049–58. doi: 10.1007/s00262-010-0830-4
29. Zhao H, Liao X, Kang Y. Tregs: where we are and what comes next? Front Immunol (2017) 8:1578. doi: 10.3389/fimmu.2017.01578
30. Gabrilovich DI. Myeloid-derived suppressor cells. Cancer Immunol Res (2017) 5(1):3–8. doi: 10.1158/2326-6066.Cir-16-0297
31. Sharma P, Hu-Lieskovan S, Wargo JA, Ribas A. Primary, adaptive, and acquired resistance to cancer immunotherapy. Cell (2017) 168(4):707–23. doi: 10.1016/j.cell.2017.01.017
32. Deng W, Lira V, Hudson TE, Lemmens EE, Hanson WG, Flores R, et al. Recombinant listeria promotes tumor rejection by Cd8(+) T cell-dependent remodeling of the tumor microenvironment. Proc Natl Acad Sci U.S.A. (2018) 115(32):8179–84. doi: 10.1073/pnas.1801910115
33. Yang Y, Hou J, Lin Z, Zhuo H, Chen D, Zhang X, et al. Attenuated listeria monocytogenes as a cancer vaccine vector for the delivery of Cd24, a biomarker for hepatic cancer stem cells. Cell Mol Immunol (2014) 11(2):184–96. doi: 10.1038/cmi.2013.64
34. Nguyen HM, Oladejo M, Paulishak W, Wood LM. A listeria-based vaccine targeting isg15 exerts anti-tumor efficacy in renal cell carcinoma. Cancer Immunol Immunother: CII (2023) 72(9):2889–903. doi: 10.1007/s00262-022-03352-9
35. Xu G, Feng D, Yao Y, Li P, Sun H, Yang H, et al. Listeria-based hepatocellular carcinoma vaccine facilitates anti-Pd-1 therapy by regulating macrophage polarization. Oncogene (2020) 39(7):1429–44. doi: 10.1038/s41388-019-1072-3
36. Soria-Castro R, Alfaro-Doblado ÁR, Rodríguez-López G, Campillo-Navarro M, Meneses-Preza YG, Galán-Salinas A, et al. Tlr2 regulates mast cell Il-6 and Il-13 production during listeria monocytogenes infection. Front Immunol (2021) 12:650779. doi: 10.3389/fimmu.2021.650779
37. Ueno A, Ikawa M, Maeda K, Tai K, Ito T, Shirafuji N, et al. Persistent severe cerebral edema with neutrophil infiltration following listeria meningitis. Intern Med (2022) 61(22):3431–4. doi: 10.2169/internalmedicine.8291-21
38. Hu W, Wang G, Huang D, Sui M, Xu Y. Cancer immunotherapy based on natural killer cells: current progress and new opportunities. Front Immunol (2019) 10:1205. doi: 10.3389/fimmu.2019.01205
39. Mkrtichyan M, Chong N, Abu Eid R, Wallecha A, Singh R, Rothman J, et al. Anti-pd-1 antibody significantly increases therapeutic efficacy of listeria monocytogenes (Lm)-llo immunotherapy. J Immunother Cancer (2013) 1:15. doi: 10.1186/2051-1426-1-15
40. Selvanesan BC, Chandra D, Quispe-Tintaya W, Jahangir A, Patel A, Meena K, et al. Listeria delivers tetanus toxoid protein to pancreatic tumors and induces cancer cell death in mice. Sci Transl Med (2022) 14(637):eabc1600. doi: 10.1126/scitranslmed.abc1600
41. Chandra D, Jahangir A, Quispe-Tintaya W, Einstein MH, Gravekamp C. Myeloid-derived suppressor cells have a central role in attenuated listeria monocytogenes-based immunotherapy against metastatic breast cancer in young and old mice. Br J Cancer (2013) 108(11):2281–90. doi: 10.1038/bjc.2013.206
42. Dallas NA, Samuel S, Xia L, Fan F, Gray MJ, Lim SJ, et al. Endoglin (Cd105): A marker of tumor vasculature and potential target for therapy. Clin Cancer Res (2008) 14(7):1931–7. doi: 10.1158/1078-0432.Ccr-07-4478
43. Wood LM, Pan ZK, Guirnalda P, Tsai P, Seavey M, Paterson Y. Targeting tumor vasculature with novel listeria-based vaccines directed against cd105. Cancer Immunol Immunother: CII (2011) 60(7):931–42. doi: 10.1007/s00262-011-1002-x
44. Hu J, Guan W, Liu P, Dai J, Tang K, Xiao H, et al. Endoglin is essential for the maintenance of self-renewal and chemoresistance in renal cancer stem cells. Stem Cell Rep (2017) 9(2):464–77. doi: 10.1016/j.stemcr.2017.07.009
45. Saroufim A, Messai Y, Hasmim M, Rioux N, Iacovelli R, Verhoest G, et al. Tumoral cd105 is a novel independent prognostic marker for prognosis in clear-cell renal cell carcinoma. Br J Cancer (2014) 110(7):1778–84. doi: 10.1038/bjc.2014.71
46. Oladejo M, Nguyen HM, Silwal A, Reese B, Paulishak W, Markiewski MM, et al. Listeria-based immunotherapy directed against cd105 exerts anti-angiogenic and anti-tumor efficacy in renal cell carcinoma. Front Immunol (2022) 13:1038807. doi: 10.3389/fimmu.2022.1038807
47. Cossart P, Lecuit M. Interactions of listeria monocytogenes with mammalian cells during entry and actin-based movement: bacterial factors, cellular ligands and signaling. EMBO J (1998) 17(14):3797–806. doi: 10.1093/emboj/17.14.3797
48. Lizotte PH, Baird JR, Stevens CA, Lauer P, Green WR, Brockstedt DG, et al. Attenuated listeria monocytogenes reprograms M2-polarized tumor-associated macrophages in ovarian cancer leading to inos-mediated tumor cell lysis. Oncoimmunology (2014) 3:e28926. doi: 10.4161/onci.28926
49. Brockstedt DG, Giedlin MA, Leong ML, Bahjat KS, Gao Y, Luckett W, et al. Listeria-based cancer vaccines that segregate immunogenicity from toxicity. Proc Natl Acad Sci USA (2004) 101(38):13832–7. doi: 10.1073/pnas.0406035101
50. Thompson RJ, Bouwer HG, Portnoy DA, Frankel FR. Pathogenicity and immunogenicity of a listeria monocytogenes strain that requires D-alanine for growth. Infect Immun (1998) 66(8):3552–61. doi: 10.1128/iai.66.8.3552-3561.1998
51. Brockstedt DG, Bahjat KS, Giedlin MA, Liu W, Leong M, Luckett W, et al. Killed but metabolically active microbes: A new vaccine paradigm for eliciting effector T-cell responses and protective immunity. Nat Med (2005) 11(8):853–60. doi: 10.1038/nm1276
52. Skoberne M, Yewdall A, Bahjat KS, Godefroy E, Lauer P, Lemmens E, et al. Kbma listeria monocytogenes is an effective vector for Dc-mediated induction of antitumor immunity. J Clin Invest (2008) 118(12):3990–4001. doi: 10.1172/jci31350
53. Hanson WG, Benanti EL, Lemmens EE, Liu W, Skoble J, Leong ML, et al. A potent and effective suicidal listeria vaccine platform. Infect Immun (2019) 87(8):e00144–19. doi: 10.1128/iai.00144-19
54. Nguyen HM, Gaikwad S, Oladejo M, Paulishak W, Wood LM. Targeting ubiquitin-like protein, isg15, as a novel tumor associated antigen in colorectal cancer. Cancers (Basel) (2023) 15(4):1237. doi: 10.3390/cancers15041237
55. Hu YX, Han XS, Jing Q. Ca(2+) ion and autophagy. Adv Exp Med Biol (2019) 1206:151–66. doi: 10.1007/978-981-15-0602-4_7
56. Lv M, Chen M, Zhang R, Zhang W, Wang C, Zhang Y, et al. Manganese is critical for antitumor immune responses via cgas-sting and improves the efficacy of clinical immunotherapy. Cell Res (2020) 30(11):966–79. doi: 10.1038/s41422-020-00395-4
57. Huang L, Liao Y, Li C, Ma Z, Liu Z. Multifunctional manganese-containing vaccine delivery system ca@Mnco(3)/llo for tumor immunotherapy. Biomater Adv (2022) 136:212752. doi: 10.1016/j.bioadv.2022.212752
58. Basu P, Mehta A, Jain M, Gupta S, Nagarkar RV, John S, et al. A randomized phase 2 study of adxs11-001 listeria monocytogenes-listeriolysin O immunotherapy with or without cisplatin in treatment of advanced cervical cancer. Int J Gynecol Cancer (2018) 28(4):764–72. doi: 10.1097/igc.0000000000001235
59. Duan F, Chen J, Yao H, Wang Y, Jia Y, Ling Z, et al. Enhanced therapeutic efficacy of listeria-based cancer vaccine with codon-optimized hpv16 E7. Hum Vaccin Immunother (2021) 17(6):1568–77. doi: 10.1080/21645515.2020.1839291
60. Maciag PC, Seavey MM, Pan ZK, Ferrone S, Paterson Y. Cancer immunotherapy targeting the high molecular weight melanoma-associated antigen protein results in a broad antitumor response and reduction of pericytes in the tumor vasculature. Cancer Res (2008) 68(19):8066–75. doi: 10.1158/0008-5472.Can-08-0287
61. Vitiello M, Evangelista M, Di Lascio N, Kusmic C, Massa A, Orso F, et al. Antitumoral effects of attenuated listeria monocytogenes in a genetically engineered mouse model of melanoma. Oncogene (2019) 38(19):3756–62. doi: 10.1038/s41388-019-0681-1
62. Gilley RP, Dube PH. Checkpoint blockade inhibitors enhances the effectiveness of a listeria monocytogenes-based melanoma vaccine. Oncotarget (2020) 11(7):740–54. doi: 10.18632/oncotarget.27490
63. Lim JY, Brockstedt DG, Lord EM, Gerber SA. Radiation therapy combined with listeria monocytogenes-based cancer vaccine synergize to enhance tumor control in the B16 melanoma model. Oncoimmunology (2014) 3:e29028. doi: 10.4161/onci.29028
64. Xin G, Schauder DM, Jing W, Jiang A, Joshi NS, Johnson B, et al. Pathogen boosted adoptive cell transfer immunotherapy to treat solid tumors. Proc Natl Acad Sci U.S.A. (2017) 114(4):740–5. doi: 10.1073/pnas.1614315114
65. Kim SH, Castro F, Gonzalez D, Maciag PC, Paterson Y, Gravekamp C. Mage-B vaccine delivered by recombinant listeria monocytogenes is highly effective against breast cancer metastases. Br J Cancer (2008) 99(5):741–9. doi: 10.1038/sj.bjc.6604526
66. Singh M, Quispe-Tintaya W, Chandra D, Jahangir A, Venkataswamy MM, Ng TW, et al. Direct incorporation of the nkt-cell activator A-galactosylceramide into a recombinant listeria monocytogenes improves breast cancer vaccine efficacy. Br J Cancer (2014) 111(10):1945–54. doi: 10.1038/bjc.2014.486
67. Stein MN, Fong L, Tutrone R, Mega A, Lam ET, Parsi M, et al. Adxs31142 immunotherapy ± Pembrolizumab treatment for metastatic castration-resistant prostate cancer: open-label phase I/Ii keynote-046 study. Oncol (2022) 27(6):453–61. doi: 10.1093/oncolo/oyac048
68. Hannan R, Zhang H, Wallecha A, Singh R, Liu L, Cohen P, et al. Combined immunotherapy with listeria monocytogenes-based Psa vaccine and radiation therapy leads to a therapeutic response in a murine model of prostate cancer. Cancer Immunol Immunother: CII (2012) 61(12):2227–38. doi: 10.1007/s00262-012-1257-x
69. Drake CG, Pachynski RK, Subudhi SK, McNeel DG, Antonarakis ES, Bauer TM, et al. Safety and preliminary immunogenicity of Jnj-64041809, a live-attenuated, double-deleted listeria monocytogenes-based immunotherapy, in metastatic castration-resistant prostate cancer. Prostate Cancer Prostatic Dis (2022) 25(2):219–28. doi: 10.1038/s41391-021-00402-8
70. Hassan R, Alley E, Kindler H, Antonia S, Jahan T, Honarmand S, et al. Clinical response of live-attenuated, listeria monocytogenes expressing mesothelin (Crs-207) with chemotherapy in patients with Malignant pleural mesothelioma. Clin Cancer Res (2019) 25(19):5787–98. doi: 10.1158/1078-0432.Ccr-19-0070
71. Tsujikawa T, Crocenzi T, Durham JN, Sugar EA, Wu AA, Onners B, et al. Evaluation of cyclophosphamide/gvax pancreas followed by listeria-mesothelin (Crs-207) with or without nivolumab in patients with pancreatic cancer. Clin Cancer Res (2020) 26(14):3578–88. doi: 10.1158/1078-0432.Ccr-19-3978
72. Le DT, Crocenzi TS, Uram JN, Lutz ER, Laheru DA, Sugar EA, et al. Randomized phase ii study of the safety, efficacy, and immune response of gvax pancreas vaccine (with cyclophosphamide) and Crs-207 with or without nivolumab in patients with previously treated metastatic pancreatic adenocarcinoma (Stellar). J Clin Oncol (2015) 33(15_suppl):TPS4148–TPS. doi: 10.1200/jco.2015.33.15_suppl.tps4148
73. Chen Y, Yang D, Li S, Gao Y, Jiang R, Deng L, et al. Development of a listeria monocytogenes-based vaccine against hepatocellular carcinoma. Oncogene (2012) 31(17):2140–52. doi: 10.1038/onc.2011.395
74. Wei SC, Anang NAS, Sharma R, Andrews MC, Reuben A, Levine JH, et al. Combination anti-ctla-4 plus anti-Pd-1 checkpoint blockade utilizes cellular mechanisms partially distinct from monotherapies. Proc Natl Acad Sci U.S.A. (2019) 116(45):22699–709. doi: 10.1073/pnas.1821218116
75. Ott PA, Hodi FS, Robert C. Ctla-4 and pd-1/pd-L1 blockade: new immunotherapeutic modalities with durable clinical benefit in melanoma patients. Clin Cancer Res (2013) 19(19):5300–9. doi: 10.1158/1078-0432.Ccr-13-0143
76. Li S, Zhang S, Liu J, Yang C, Zhang L, Cheng Y. The effect of Pd-L1/Pd-1 immunotherapy in the treatment of squamous non-small-cell lung cancer: A meta-analysis of randomized controlled clinical trials. J Thorac Dis (2019) 11(11):4453–63. doi: 10.21037/jtd.2019.11.12
77. Li K, Tian H. Development of small-molecule immune checkpoint inhibitors of Pd-1/Pd-L1 as a new therapeutic strategy for tumour immunotherapy. J Drug Target (2019) 27(3):244–56. doi: 10.1080/1061186X.2018.1440400
78. Gong J, Chehrazi-Raffle A, Reddi S, Salgia R. Development of pd-1 and Pd-L1 inhibitors as a form of cancer immunotherapy: A comprehensive review of registration trials and future considerations. J Immunother Cancer (2018) 6(1):8. doi: 10.1186/s40425-018-0316-z
79. Nuro-Gyina PK, Rieser EL, Granitto MC, Pei W, Liu Y, Lee PW, et al. Regulation of effector function of cns autoreactive Cd4 T cells through inhibitory receptors and il-7rα. J Neuroinflamm (2016) 13(1):302. doi: 10.1186/s12974-016-0768-3
80. Topalian SL, Hodi FS, Brahmer JR, Gettinger SN, Smith DC, McDermott DF, et al. Safety, activity, and immune correlates of anti-Pd-1 antibody in cancer. N Engl J Med (2012) 366(26):2443–54. doi: 10.1056/NEJMoa1200690
81. Hodi FS, O’Day SJ, McDermott DF, Weber RW, Sosman JA, Haanen JB, et al. Improved survival with ipilimumab in patients with metastatic melanoma. N Engl J Med (2010) 363(8):711–23. doi: 10.1056/NEJMoa1003466
82. Zheng L, Foley K, Huang L, Leubner A, Mo G, Olino K, et al. Tyrosine 23 phosphorylation-dependent cell-surface localization of annexin A2 is required for invasion and metastases of pancreatic cancer. PloS One (2011) 6(4):e19390. doi: 10.1371/journal.pone.0019390
83. Xiao M, Xie L, Cao G, Lei S, Wang P, Wei Z, et al. Cd4(+) T-cell epitope-based heterologous prime-boost vaccination potentiates anti-tumor immunity and Pd-1/Pd-L1 immunotherapy. J Immunother Cancer (2022) 10(5):e004022. doi: 10.1136/jitc-2021-004022
84. Reck M, Rodríguez-Abreu D, Robinson AG, Hui R, Csőszi T, Fülöp A, et al. Pembrolizumab versus chemotherapy for Pd-L1-positive non-small-cell lung cancer. N Engl J Med (2016) 375(19):1823–33. doi: 10.1056/NEJMoa1606774
85. Xia L, Liu Y, Wang Y. Pd-1/Pd-L1 Blockade therapy in advanced non-small-cell lung cancer: current status and future directions. Oncol (2019) 24(Suppl 1):S31–s41. doi: 10.1634/theoncologist.2019-IO-S1-s05
86. Ott PA, Bang YJ, Piha-Paul SA, Razak ARA, Bennouna J, Soria JC, et al. T-cell-inflamed gene-expression profile, programmed death ligand 1 expression, and tumor mutational burden predict efficacy in patients treated with pembrolizumab across 20 cancers: keynote-028. J Clin Oncol (2019) 37(4):318–27. doi: 10.1200/jco.2018.78.2276
87. Bollard CM, Gottschalk S, Torrano V, Diouf O, Ku S, Hazrat Y, et al. Sustained complete responses in patients with lymphoma receiving autologous cytotoxic T lymphocytes targeting epstein-barr virus latent membrane proteins. J Clin Oncol (2014) 32(8):798–808. doi: 10.1200/jco.2013.51.5304
88. Rosenberg SA, Yang JC, Sherry RM, Kammula US, Hughes MS, Phan GQ, et al. Durable complete responses in heavily pretreated patients with metastatic melanoma using T-cell transfer immunotherapy. Clin Cancer Res (2011) 17(13):4550–7. doi: 10.1158/1078-0432.Ccr-11-0116
89. Robbins PF, Morgan RA, Feldman SA, Yang JC, Sherry RM, Dudley ME, et al. Tumor regression in patients with metastatic synovial cell sarcoma and melanoma using genetically engineered lymphocytes reactive with Ny-Eso-1. J Clin Oncol (2011) 29(7):917–24. doi: 10.1200/jco.2010.32.2537
90. Rosenberg SA, Restifo NP. Adoptive cell transfer as personalized immunotherapy for human cancer. Science (2015) 348(6230):62–8. doi: 10.1126/science.aaa4967
91. Labani-Motlagh A, Ashja-Mahdavi M, Loskog A. The tumor microenvironment: A milieu hindering and obstructing antitumor immune responses. Front Immunol (2020) 11:940. doi: 10.3389/fimmu.2020.00940
92. Wallecha A, Singh R, Malinina I. Listeria monocytogenes (Lm)-Llo immunotherapies reduce the immunosuppressive activity of myeloid-derived suppressor cells and regulatory T cells in the tumor microenvironment. J Immunother (2013) 36(9):468–76. doi: 10.1097/cji.0000000000000000
93. Xin G, Khatun A, Topchyan P, Zander R, Volberding PJ, Chen Y, et al. Pathogen-boosted adoptive cell transfer therapy induces endogenous antitumor immunity through antigen spreading. Cancer Immunol Res (2020) 8(1):7–18. doi: 10.1158/2326-6066.Cir-19-0251
94. Garnett CT, Palena C, Chakraborty M, Tsang KY, Schlom J, Hodge JW. Sublethal irradiation of human tumor cells modulates phenotype resulting in enhanced killing by cytotoxic T lymphocytes. Cancer Res (2004) 64(21):7985–94. doi: 10.1158/0008-5472.Can-04-1525
95. Chakraborty M, Abrams SI, Coleman CN, Camphausen K, Schlom J, Hodge JW. External beam radiation of tumors alters phenotype of tumor cells to render them susceptible to vaccine-mediated T-cell killing. Cancer Res (2004) 64(12):4328–37. doi: 10.1158/0008-5472.Can-04-0073
96. Quispe-Tintaya W, Chandra D, Jahangir A, Harris M, Casadevall A, Dadachova E, et al. Nontoxic radioactive listeria(at) is a highly effective therapy against metastatic pancreatic cancer. Proc Natl Acad Sci U.S.A. (2013) 110(21):8668–73. doi: 10.1073/pnas.1211287110
97. Brahmer JR, Johnson ML, Cobo M, Viteri S, Sarto JC, Sukari A, et al. Jnj-64041757 (Jnj-757), a live, attenuated, double-deleted listeria monocytogenes-based immunotherapy in patients with nsclc: results from two phase 1 studies. JTO Clin Res Rep (2021) 2(2):100103. doi: 10.1016/j.jtocrr.2020.100103
98. Le DT, Wang-Gillam A, Vincent Picozzi J, Greten TF, Crocenzi TS, Springett GM, et al. A phase 2, randomized trial of gvax pancreas and Crs-207 immunotherapy versus Gvax alone in patients with metastatic pancreatic adenocarcinoma: updated results. J Clin Oncol (2014) 32(3_suppl):177–. doi: 10.1200/jco.2014.32.3_suppl.177
99. Le DT, Wang-Gillam A, Picozzi V, Greten TF, Crocenzi T, Springett G, et al. Safety and survival with gvax pancreas prime and listeria monocytogenes-expressing mesothelin (Crs-207) boost vaccines for metastatic pancreatic cancer. J Clin Oncol (2015) 33(12):1325–33. doi: 10.1200/jco.2014.57.4244
100. Vu M, Yu J, Awolude OA, Chuang L. Cervical cancer worldwide. Curr Probl Cancer (2018) 42(5):457–65. doi: 10.1016/j.currproblcancer.2018.06.003
101. Dominiecki ME, Beatty GL, Pan ZK, Neeson P, Paterson Y. Tumor sensitivity to ifn-gamma is required for successful antigen-specific immunotherapy of a transplantable mouse tumor model for Hpv-transformed tumors. Cancer Immunol Immunother: CII (2005) 54(5):477–88. doi: 10.1007/s00262-004-0610-0
102. Tewari KS, Java JJ, Gatcliffe TA, Bookman MA, Monk BJ. Chemotherapy-induced neutropenia as a biomarker of survival in advanced ovarian carcinoma: an exploratory study of the gynecologic oncology group. Gynecol Oncol (2014) 133(3):439–45. doi: 10.1016/j.ygyno.2014.03.013
103. Wallecha A, French C, Petit R, Singh R, Amin A, Rothman J. Lm-Llo-based immunotherapies and Hpv-associated disease. J Oncol (2012) 2012:542851. doi: 10.1155/2012/542851
104. Zhao KN, Chen J. Codon usage roles in human papillomavirus. Rev Med Virol (2011) 21(6):397–411. doi: 10.1002/rmv.707
105. Takakura Y, Katayama S, Nagata Y. High-level expression of tamavidin 2 in human cells by codon-usage optimization. Biosci Biotechnol Biochem (2015) 79(4):610–6. doi: 10.1080/09168451.2014.991690
106. Qian Y, Zhang N, Jiang P, Chen S, Chu S, Hamze F, et al. Inhibitory effect of live-attenuated listeria monocytogenes-based vaccines expressing mia gene on Malignant melanoma. J Huazhong Univ Sci Technol Med Sci = Hua zhong ke ji da xue xue bao Yi xue Ying wen ban = Huazhong keji daxue xuebao Yixue Yingdewen ban (2012) 32(4):591–7. doi: 10.1007/s11596-012-1002-x
107. Poruk KE, Firpo MA, Adler DG, Mulvihill SJ. Screening for pancreatic cancer: why, how, and who? Ann Surg (2013) 257(1):17–26. doi: 10.1097/SLA.0b013e31825ffbfb
108. Safdar A, Papadopoulous EB, Armstrong D. Listeriosis in recipients of allogeneic blood and marrow transplantation: thirteen year review of disease characteristics, treatment outcomes and a new association with human cytomegalovirus infection. Bone Marrow Transplant (2002) 29(11):913–6. doi: 10.1038/sj.bmt.1703562
109. Kulke MH, Blaszkowsky LS, Ryan DP, Clark JW, Meyerhardt JA, Zhu AX, et al. Capecitabine plus erlotinib in gemcitabine-refractory advanced pancreatic cancer. J Clin Oncol (2007) 25(30):4787–92. doi: 10.1200/jco.2007.11.8521
110. Dimou A, Syrigos KN, Saif MW. Overcoming the stromal barrier: technologies to optimize drug delivery in pancreatic cancer. Ther Adv Med Oncol (2012) 4(5):271–9. doi: 10.1177/1758834012446008
111. Olive KP, Jacobetz MA, Davidson CJ, Gopinathan A, McIntyre D, Honess D, et al. Inhibition of hedgehog signaling enhances delivery of chemotherapy in a mouse model of pancreatic cancer. Science (2009) 324(5933):1457–61. doi: 10.1126/science.1171362
112. Le DT, Whiting CC, Lutz ER, Nair N, Engstrom A, Lemmens E, et al. Clinical and immune characteristics of rapid dropout and long-term survival in a phase Ii safety and efficacy study of combination Crs-207/Gvax immunotherapy in pancreatic cancer. J Clin Oncol (2016) 34(4_suppl):459–. doi: 10.1200/jco.2016.34.4_suppl.459
113. Le DT, Picozzi VJ, Ko AH, Wainberg ZA, Kindler H, Wang-Gillam A, et al. Results from a phase iib, randomized, multicenter study of Gvax pancreas and crs-207 compared with chemotherapy in adults with previously treated metastatic pancreatic adenocarcinoma (Eclipse study). Clin Cancer Res (2019) 25(18):5493–502. doi: 10.1158/1078-0432.Ccr-18-2992
114. Chandra D, Selvanesan BC, Yuan Z, Libutti SK, Koba W, Beck A, et al. 32-phosphorus selectively delivered by listeria to pancreatic cancer demonstrates a strong therapeutic effect. Oncotarget (2017) 8(13):20729–40. doi: 10.18632/oncotarget.15117
115. Youlden DR, Cramb SM, Dunn NA, Muller JM, Pyke CM, Baade PD. The descriptive epidemiology of female breast cancer: an international comparison of screening, incidence, survival and mortality. Cancer Epidemiol (2012) 36(3):237–48. doi: 10.1016/j.canep.2012.02.007
116. Jewer M, Lee L, Leibovitch M, Zhang G, Liu J, Findlay SD, et al. Translational control of breast cancer plasticity. Nat Commun (2020) 11(1):2498. doi: 10.1038/s41467-020-16352-z
117. Cardoso F, Costa A, Norton L, Cameron D, Cufer T, Fallowfield L, et al. 1st international consensus guidelines for advanced breast cancer (Abc 1). Breast (Edinburgh Scotland) (2012) 21(3):242–52. doi: 10.1016/j.breast.2012.03.003
118. Gonzalez-Angulo AM, Morales-Vasquez F, Hortobagyi GN. Overview of resistance to systemic therapy in patients with breast cancer. Adv Exp Med Biol (2007) 608:1–22. doi: 10.1007/978-0-387-74039-3_1
119. Stead LA, Lash TL, Sobieraj JE, Chi DD, Westrup JL, Charlot M, et al. Triple-negative breast cancers are increased in black women regardless of age or body mass index. Breast Cancer Res: BCR (2009) 11(2):R18. doi: 10.1186/bcr2242
120. Dent R, Trudeau M, Pritchard KI, Hanna WM, Kahn HK, Sawka CA, et al. Triple-negative breast cancer: clinical features and patterns of recurrence. Clin Cancer Res (2007) 13(15 Pt 1):4429–34. doi: 10.1158/1078-0432.Ccr-06-3045
121. Singh M, Ramos I, Asafu-Adjei D, Quispe-Tintaya W, Chandra D, Jahangir A, et al. Curcumin improves the therapeutic efficacy of listeria(at)-mage-B vaccine in correlation with improved T-cell responses in blood of a triple-negative breast cancer model 4t1. Cancer Med (2013) 2(4):571–82. doi: 10.1002/cam4.94
122. Valenzuela J, Schmidt C, Mescher M. The roles of il-12 in providing a third signal for clonal expansion of naive cd8 T cells. J Immunol (2002) 169(12):6842–9. doi: 10.4049/jimmunol.169.12.6842
123. Sinibaldi VJ. Docetaxel treatment in the elderly patient with hormone refractory prostate cancer. Clin Interventions Aging (2007) 2(4):555–60. doi: 10.2147/cia.s1395
124. Chandrasekar T, Yang JC, Gao AC, Evans CP. Mechanisms of resistance in castration-resistant prostate cancer (Crpc). Trans Androl Urol (2015) 4(3):365–80. doi: 10.3978/j.issn.2223-4683.2015.05.02
125. Wei XX, Fong L, Small EJ. Prostate cancer immunotherapy with sipuleucel-T: current standards and future directions. Expert Rev Vaccines (2015) 14(12):1529–41. doi: 10.1586/14760584.2015.1099437
126. Akhtar NH, Pail O, Saran A, Tyrell L, Tagawa ST. Prostate-specific membrane antigen-based therapeutics. Adv Urol (2012) 2012:973820. doi: 10.1155/2012/973820
127. Dubovsky JA, McNeel DG. Inducible expression of a prostate cancer-testis antigen, Ssx-2, following treatment with a DNA methylation inhibitor. Prostate (2007) 67(16):1781–90. doi: 10.1002/pros.20665
128. Bhatia-Gaur R, Donjacour AA, Sciavolino PJ, Kim M, Desai N, Young P, et al. Roles for nkx3.1 in prostate development and cancer. Genes Dev (1999) 13(8):966–77. doi: 10.1101/gad.13.8.966
129. Robinson BW, Lake RA. Advances in Malignant mesothelioma. N Engl J Med (2005) 353(15):1591–603. doi: 10.1056/NEJMra050152
130. Yoshikawa Y, Kuribayashi K, Minami T, Ohmuraya M, Kijima T. Epigenetic alterations and biomarkers for immune checkpoint inhibitors-current standards and future perspectives in Malignant pleural mesothelioma treatment. Front Oncol (2020) 10:554570. doi: 10.3389/fonc.2020.554570
131. Wong RM, Ianculescu I, Sharma S, Gage DL, Olevsky OM, Kotova S, et al. Immunotherapy for Malignant pleural mesothelioma. Current status and future prospects. Am J Respir Cell Mol Biol (2014) 50(5):870–5. doi: 10.1165/rcmb.2013-0472TR
132. Hegmans JP, Veltman JD, Lambers ME, de Vries IJ, Figdor CG, Hendriks RW, et al. Consolidative dendritic cell-based immunotherapy elicits cytotoxicity against Malignant mesothelioma. Am J Respir Crit Care Med (2010) 181(12):1383–90. doi: 10.1164/rccm.200909-1465OC
133. Powell A, Creaney J, Broomfield S, Van Bruggen I, Robinson B. Recombinant gm-csf plus autologous tumor cells as a vaccine for patients with mesothelioma. Lung Cancer (Amsterdam Netherlands) (2006) 52(2):189–97. doi: 10.1016/j.lungcan.2006.01.007
134. Nowak AK, Lake RA, Marzo AL, Scott B, Heath WR, Collins EJ, et al. Induction of tumor cell apoptosis in vivo increases tumor antigen cross-presentation, cross-priming rather than cross-tolerizing host tumor-specific Cd8 T cells. J Immunol (2003) 170(10):4905–13. doi: 10.4049/jimmunol.170.10.4905
135. Hassan R, Laszik ZG, Lerner M, Raffeld M, Postier R, Brackett D. Mesothelin is overexpressed in pancreaticobiliary adenocarcinomas but not in normal pancreas and chronic pancreatitis. Am J Clin Pathol (2005) 124(6):838–45. doi: 10.1309/F1B64CL7H8VJKEAF
136. Hassan R, Kreitman RJ, Pastan I, Willingham MC. Localization of mesothelin in epithelial ovarian cancer. Appl Immunohistochem Mol Morphol (2005) 13(3):243–7. doi: 10.1097/01.pai.00000141545.36485.d6
137. Chang K, Pastan I. Molecular cloning of mesothelin, a differentiation antigen present on mesothelium, mesotheliomas, and ovarian cancers. Proc Natl Acad Sci U.S.A. (1996) 93(1):136–40. doi: 10.1073/pnas.93.1.136
138. Kennedy GT, Judy BF, Bhojnagarwala P, Moon EK, Fridlender ZG, Albelda SM, et al. Surgical cytoreduction restores the antitumor efficacy of a listeria monocytogenes vaccine in Malignant pleural mesothelioma. Immunol Lett (2015) 166(1):28–35. doi: 10.1016/j.imlet.2015.05.009
139. Hochnadel I, Hoenicke L, Petriv N, Neubert L, Reinhard E, Hirsch T, et al. Safety and efficacy of prophylactic and therapeutic vaccine based on live-attenuated listeria monocytogenes in hepatobiliary cancers. Oncogene (2022) 41(14):2039–53. doi: 10.1038/s41388-022-02222-z
140. Musser ML, Berger EP, Tripp CD, Clifford CA, Bergman PJ, Johannes CM. Safety evaluation of the canine osteosarcoma vaccine, live listeria vector. Veterinary Comp Oncol (2021) 19(1):92–8. doi: 10.1111/vco.12642
141. Keestra AM, Godinez I, Xavier MN, Winter MG, Winter SE, Tsolis RM, et al. Early myd88-dependent induction of interleukin-17a expression during salmonella colitis. Infect Immun (2011) 79(8):3131–40. doi: 10.1128/iai.00018-11
142. Chabab G, Barjon C, Bonnefoy N, Lafont V. Pro-tumor Γδ T cells in human cancer: polarization, mechanisms of action, and implications for therapy. Front Immunol (2020) 11:2186. doi: 10.3389/fimmu.2020.02186
143. Pang DJ, Neves JF, Sumaria N, Pennington DJ. Understanding the complexity of Γδ T-cell subsets in mouse and human. Immunology (2012) 136(3):283–90. doi: 10.1111/j.1365-2567.2012.03582.x
144. Alice AF, Kramer G, Bambina S, Bahjat KS, Gough MJ, Crittenden MR. Listeria monocytogenes-infected human monocytic derived dendritic cells activate Vγ9vδ2 T cells independently of hmbpp production. Sci Rep (2021) 11(1):16347. doi: 10.1038/s41598-021-95908-5
Keywords: Listeria, immunotherapy, cancer vaccine, tumor, therapy
Citation: Ding Y-D, Shu L-Z, He R-S, Chen K-Y, Deng Y-J, Zhou Z-B, Xiong Y and Deng H (2023) Listeria monocytogenes: a promising vector for tumor immunotherapy. Front. Immunol. 14:1278011. doi: 10.3389/fimmu.2023.1278011
Received: 15 August 2023; Accepted: 25 September 2023;
Published: 06 October 2023.
Edited by:
Zong Sheng Guo, University at Buffalo, United StatesReviewed by:
Alireza Labani-Motlagh, Hackensack Meridian Health, United StatesThomas Dubensky, Tempest Therapeutics, Inc., United States
Anliang Xia, Nanjing University, China
Copyright © 2023 Ding, Shu, He, Chen, Deng, Zhou, Xiong and Deng. This is an open-access article distributed under the terms of the Creative Commons Attribution License (CC BY). The use, distribution or reproduction in other forums is permitted, provided the original author(s) and the copyright owner(s) are credited and that the original publication in this journal is cited, in accordance with accepted academic practice. No use, distribution or reproduction is permitted which does not comply with these terms.
*Correspondence: Huan Deng, YmVhbmRlbmdAbmN1LmVkdS5jbg==; Ying Xiong, MjQ4NzIzNTdAcXEuY29t