- 1Sanquan College of Xinxiang Medical University, Xinxiang, China
- 2Department of Radiation Oncology, Hubei Cancer Hospital, Tongji Medical College, Huazhong University of Science and Technology, Wuhan, China
- 3Zhejiang Provincial Clinical Research Center for Obstetrics and Gynecology, Hangzhou, China
- 4Department of Gynecology, The Second Affiliated Hospital, Zhejiang University School of Medicine, Hangzhou, China
Ovarian cancer remains a challenging disease with limited treatment options and poor prognosis. The tumor microenvironment (TME) plays a crucial role in tumor growth, progression, and therapy response. One characteristic feature of the TME is the abnormal tumor vasculature, which is associated with inadequate blood perfusion, hypoxia, and immune evasion. Vascular normalization, a therapeutic strategy aiming to rectify the abnormal tumor vasculature, has emerged as a promising approach to reshape the TME, enhance antitumor immunity, and synergize with immunotherapy in ovarian cancer. This review paper provides a comprehensive overview of vascular normalization and its potential implications in ovarian cancer. In this review, we summarize the intricate interplay between anti-angiogenesis and immune modulation, as well as ICI combined with anti-angiogenesis therapy in ovarian cancer. The compelling evidence discussed in this review contributes to the growing body of knowledge supporting the utilization of combination therapy as a promising treatment paradigm for ovarian cancer, paving the way for further clinical development and optimization of this therapeutic approach.
1 Background
According to comprehensive global cancer statistics, ovarian cancer remains a significant health concern for women worldwide. Annually, over 230,000 females receive a distressing diagnosis of ovarian cancer, accounting for approximately 3.6% of newly reported cancer cases. Tragically, the disease claims the lives of more than 150,000 women each year, representing 4.3% of all cancer-related fatalities. Thus, ovarian cancer stands as the second leading cause of death among gynecological malignancies, second only to cervical cancer (1). Ovarian cancer possesses distinctive characteristics, including delayed detection, early metastasis, drug resistance, and challenging treatment protocols, all of which contribute to a poor prognosis (2–4). Within the hierarchy of fatal gynecological tumors, epithelial ovarian cancer claims the highest mortality rate (5–7). Alarming statistics are observed within China, where more than 52,000 women succumb to epithelial ovarian cancer annually, accompanied by a concerning escalation in both incidence and mortality rates (8). However, there is a glimmer of hope in the form of targeted therapies, which have demonstrated promising outcomes as maintenance treatments (9–11). These innovative drugs not only aid in disease control but also offer the potential to delay disease progression and improve the overall prognosis for ovarian cancer patients (12–18).
Among these novel targeted strategies, anti-angiogenesis therapy has become a key issue in the field of cancer therapeutics (19–21). Angiogenesis plays a crucial role in the progression of ovarian cancer, contributing to tumor development and distant metastasis (22). The humanized monoclonal antibody targeting vascular endothelial growth factor (VEGF) bevacizumab, has been extensively investigated in epithelial ovarian cancer (23). The effectiveness of bevacizumab has been confirmed in multiple clinical studies, even in refractory ovarian cancer (24–26). Actually, anti-angiogenesis therapy not only disrupts blood vessels crucial for cancer growth and metastasis but also has a significant impact on reshaping the tumor microenvironment (TME) (27). Preclinical and clinical studies have consistently shown that the combination of anti-angiogenesis with immune checkpoint blockade (ICB) therapy surpasses the efficacy of monotherapy (28–35). In mouse models, the combination therapy demonstrates a remarkable ability to decrease the ratio of pro-tumor to anti-tumor immune cells and effectively downregulate the levels of immune checkpoints, surpassing the effects observed with PD-1 blockade alone (36–39). These findings have generated considerable excitement, leading to the initiation of numerous clinical trials aimed at investigating the synergistic effects of this combination therapy, with encouraging outcomes being observed (40–43). This review provides a comprehensive summary of the most recent understanding surrounding ICB plus anti-angiogenesis strategy, highlighting the significant advances made in relevant clinical trials.
2 Anti-angiogenesis therapy for ovarian cancer
2.1 Tumor angiogenesis
Angiogenesis, the formation of new blood vessels, was first described by Scottish surgeon John Hunter. It plays a vital role in both normal physiological processes and pathological conditions (44). Physiological angiogenesis occurs during embryogenesis, tissue repair, and regeneration, and is a short-term, controlled process. In contrast, pathological angiogenesis is associated with diseases and involves uncontrolled endothelial cell proliferation, migration, and degradation of the extracellular matrix (45). Then, a groundbreaking study by Folkman revealed the significant relationship between angiogenesis and tumor growth. He introduced the concept that tumor growth depends on neovascularization, marking a milestone in research (46). Tumor angiogenesis primarily occurs through the budding of new blood vessels from existing microvascular beds. These newly formed vessels provide nutrients and oxygen for tumor growth while also serving as a pathway for distant metastasis (47–53). Folkman’s work also led to the emergence of “anti-angiogenesis therapy” as a new approach to cancer treatment and opened up exciting avenues for biomedical research (54–56).
Angiogenesis in tumor neovascularization is regulated by a complex interplay of pro-angiogenic and anti-angiogenesis factors. Pro-angiogenic factors promote angiogenesis, while endogenous inhibitory angiogenic factors prevent excessive vessel formation (57). Examples of pro-angiogenic factors include vascular endothelial growth factor (VEGF), angiopoietin (ANGPT), platelet-derived growth factor (PDGF), fibroblast growth factor (FGF), and other cytokines (58–61). On the other hand, endostatin, angiostatin, platelet factor 4 (PF4), interferon alpha (IFN-α), and other factors act as inhibitory angiogenic factors (62–65). The balance between these factors determines the local angiogenesis of a tumor, with an increase in pro-angiogenic factors or a decrease in anti-angiogenesis factors tipping the balance towards angiogenesis (66).
The VEGF family, consisting of VEGFA, VEGFB, VEGFC, VEGFD, VEGFE, and placental growth factor (PIGF), is a central player in angiogenesis (67). VEGF receptors (VEGFRs), including VEGFR1 (Flt-1), VEGFR2 (KDR/Flk-1), and VEGFR3 (Flt-4), are tyrosine kinase receptors involved in signal transduction (68). Different isoforms of VEGFA exhibit varying levels of activity and localization in the body. VEGFA primarily activates and binds to VEGFR-2, triggering a cascade of intracellular signaling pathways that stimulate endothelial cell proliferation, increase vascular permeability, and promote neo-angiogenesis (69). Another important component in angiogenesis, independent of VEGF/VEGFR, is the ANGPT/TIE system (70). The ANGPT family includes ANGPT1, ANGPT2, ANGPT3, and ANGPT4, with ANGPT1 and ANGPT2 being extensively studied (71). ANGPT1 is mainly expressed in perivascular cells such as pericytes and smooth muscle cells. It interacts with Tie2 receptors in a paracrine manner, promoting endothelial cell viability and maintaining vascular integrity. ANGPT2, predominantly expressed in vascular endothelial cells, competitively blocks the effects of ANGPT1, leading to increased vascular permeability and disruption of the resting vascular system (72–74). ANGPT2’s interaction with endothelial progenitor cells may serve as an initiating factor in neovascularization (75–77). Understanding the mechanisms and factors involved in angiogenesis has significant implications for therapeutic interventions and targeting tumor growth. Anti-angiogenesis strategies aim to inhibit or disrupt the formation of new blood vessels, thereby starving tumors of the necessary nutrients and oxygen for their growth (78). These approaches include the use of anti- angiogenesis drugs that target specific factors or receptors involved in angiogenesis. Generally, angiogenesis is a complex process crucial for normal physiological functions and pathological conditions. It is determined by the delicate balance between anti-angiogenesis and pro-angiogenesis regulators. The VEGF/VEGFR and ANGPT/TIE systems play essential roles in angiogenesis, with VEGFA and ANGPT1 being key players. Intriguingly, nuclear PD-L1 is reported to be implicated in angiogenesis, elucidating the intricate biological signaling that orchestrates the interaction between immune responses and vascularization (79). So far, multiple anti-angiogenesis agents have been approved for cancer treatment Figure 1; Table 1 (80).
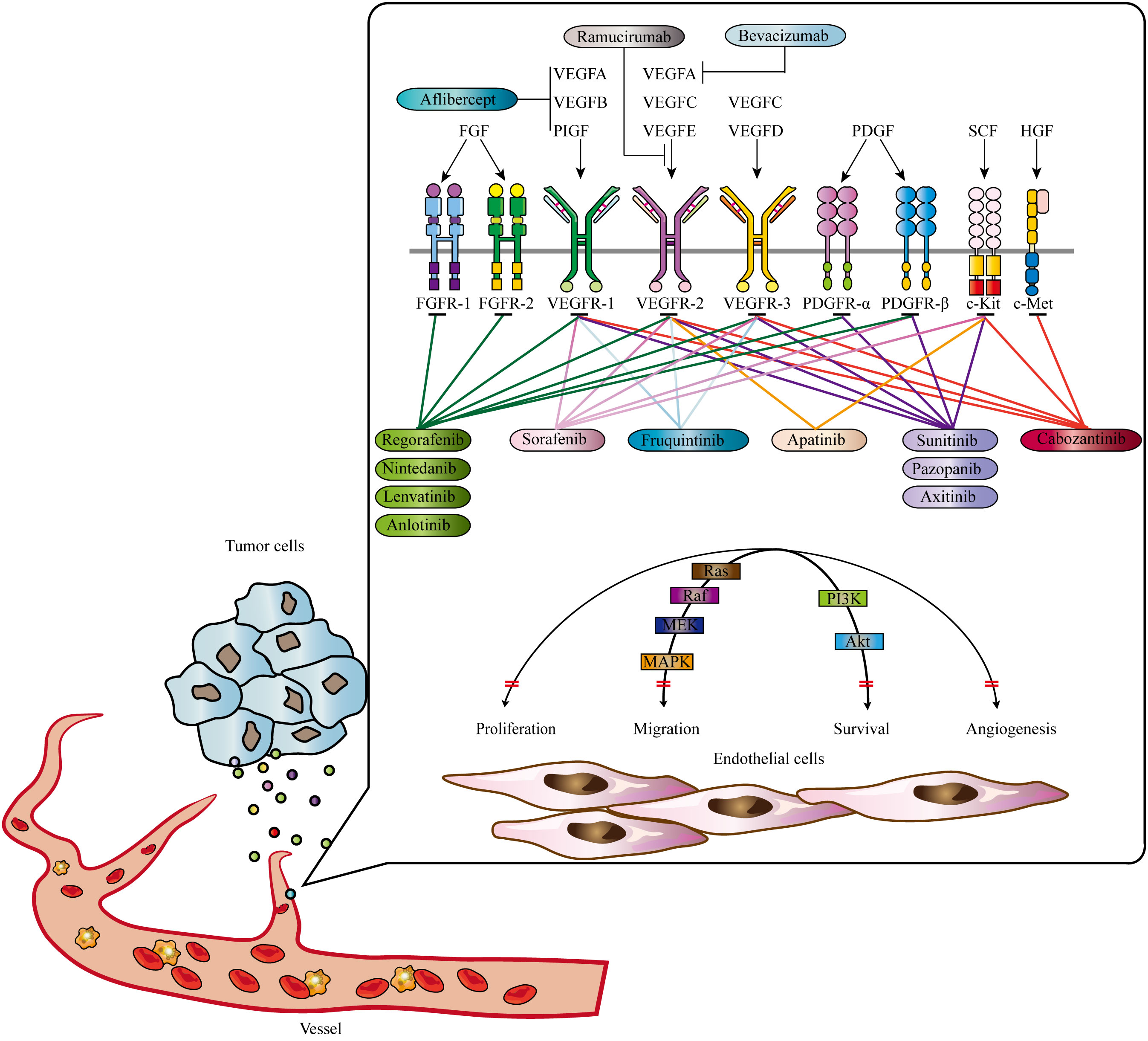
Figure 1 Signaling pathways contributing to tumor angiogenesis and approved anti-tumor angiogenesis drugs. Mutiple signas have been validated to promote tumor angiogenesis, including EGF, FGF, PDGF, HGF, and SCF signaling. Abbrevations: VEGF, Vascular Endothelial Growth Factor; PDGF, Platelet-Derived Growth Factor; FGF, Fibroblast Growth Factor; HGF, Hepatocyte Growth Factor; SCF, Stem Cell Factor. Adapted from Qin et al., 2019 (22).
2.2 Anti-angiogenesis therapy for ovarian cancer
In the microenvironment of ovarian cancer, VEGF signaling is highly activated and strongly correlated with poor differentiation grade and prognosis (81, 82). Therefore, targeting pro-angiogenic signaling pathways, particularly the VEGF pathway, shows promise as an effective strategy for ovarian cancer. Bevacizumab, the first recombinant humanized anti-VEGF-A antibody, is the most widely used anti-angiogenesis agent (83–85). By inhibiting the binding of VEGF-A to VEGFR, bevacizumab impedes the formation of new blood vessels, interferes pre-existing blood vessels, and downregulates intratumoral microvessel density (86, 87). It has received FDA approval for platinum-sensitive refractory ovarian cancer (88). Besides, it has been also approved by European Commission for advanced epithelial ovarian cancer (89). Bevacizumab has demonstrated clinical efficacy in advanced and recurrent ovarian cancer, resulting in delayed tumor development and serving as a maintenance therapy (90, 91). Combining bevacizumab with chemotherapy has been explored for the treatment of ovarian cancer (92–95). However, recent meta-analysis demonstrates a more pronounced improvement in median progression-free survival (PFS) achieved by bevacizumab alone or in combination with other inhibitors, yet it does not indicate any overall survival benefit, even detrimental to post-progression survival (96). The result highlights the need to identify predictive biomarkers that can discriminate the patients potentially benefited from bevacizumab. Although, circulating Tie2, ascites formation, BRCA1/2, homologous recombination deficiency and CD31 status can somewhat influence the efficacy, the identification of predictive biomarkers remains challenging and elusive (97, 98).
Moreover, cediranib, an inhibitor of VEGFR tyrosine kinase (VEGFR1-3), has exhibited limited efficacy and increased toxicity in various cancers, including ovarian cancer (99). Nonetheless, promising results have been observed in cediranib treatment for ovarian cancer patients, particularly regarding PFS when used in combination with chemotherapy and maintenance treatment (99). The combination of Poly ADP-ribose polymerase inhibitors and cediranib has demonstrated enhanced efficacy in inhibiting tumor proliferation and promoting the immune response within the tumor microenvironment, irrespective of HDR status, in a patient-derived xenograft model of ovarian cancer (100). The study conducted by Zhou et al. demonstrated that the plasma concentration of Tie2 serves as a reliable predictive biomarker for generic VEGF signaling inhibitor, including cediranib in ovarian cancer (101). Another murine in vivo investigation revealed that the TME intrinsically characterized with higher level of IL6 and JAK/STAT signaling counteracted the activity of cediranib (102). Besides, pazopanib (selective multi-targeted receptor tyrosine kinase inhibitor targeting VEGFR, FGFR, PDGFR, c-KIT, and c-Fms) effectively inhibits tumor growth and angiogenesis (103, 104). Although pazopanib is associated with adverse events such as neutropenia, it has demonstrated advantages in the treatment of refractory and platinum-resistant ovarian cancer, as well as in platinum-sensitive maintenance therapy (105–107). Additionally, nintedanib, another inhibitor targeting various tyrosine kinases, including PDGFR, FGFR, FLT3, and VEGFR, competitively suppresses non-receptor tyrosine kinases (108, 109). Nintedanib is known to cause common adverse events such as diarrhea (110). Nevertheless, phase 3 trials have exhibited remarkable therapeutic effects when nintedanib is combined with chemotherapy such as carboplatin and paclitaxel in ovarian cancer patients, although serious adverse gastrointestinal events accompany these positive effects (111–113).
In addition to VEGF signaling, there are alternative pathways targeted by angiogenesis inhibitors. ANGPT1/2 bind to the Tie-2 receptor and promote endothelial cell proliferation, survival, and motility. Trebananib, a non-VEGF-dependent anti-angiogenesis agent, binds to ANGPT1/2 and counteracts their action on the Tie-2 receptor, thereby effectively suppressing angiogenesis (114). Trebananib, when combined with paclitaxel, effectively improves the PFS of patients with recurrent ovarian cancer (115). In summary, targeting angiogenesis through VEGF/VEGFR signaling has shown promising results in ovarian cancer. Bevacizumab, cediranib, pazopanib, and nintedanib are among the agents that have been investigated in this context. However, challenges persist in terms of optimizing therapeutic responses, managing side effects, and identifying predictive biomarkers to facilitate better patient selection (116). Further research and clinical investigations are needed to improve the efficacies of anti-angiogenesis agents in ovarian cancer.
3 Anti-angiogenesis therapy reshapes the TME and enhances ICB efficacy
3.1 Angiogenesis and anti-tumor immunity
The antitumor immunity and ICB efficacy are regulated by the presence and status of tumor-infiltrating lymphocytes (TILs) as well as the process of angiogenesis (117–121). TILs are critical components of the immune response against tumors, and their presence is essential for successful tumor regression with ICB therapy, particularly with interventions targeting the PD-1/PD-L1 pathway. Angiogenesis plays a crucial role in the status of TILs and the response to ICI therapy. In the cancer-immunity cycle, the presentation of neoantigens initiates the formation of tumor-specific T cell clones, which then traffic to and infiltrate the tumor (122). TILs recognize these neoantigens and eliminate tumor cells in an immunosupportive TME (122). However, hyperactive angiogenesis leads to an immunosuppressive TME by affecting multiple processes of the immune response (123–125).
Abnormal angiogenesis negatively impacts the abundance and function of TILs (29). It creates physical barriers that impede T cell infiltration, such as leaky vessels with high interstitial fluid pressure and reduced expression of adhesion molecules like VCAM-1 (126). Additionally, the lack of adequate vasculature compromises the oxygen supply to the tumor, leading to hypoxia. Hypoxia, in turn, upregulates immunoinhibitory signals, including IL-6, IL-10, PD-L1, and IDO (19). Moreover, circulating VEGF hinders the maturation and activity of dendritic cells, facilitating immune evasion of tumor cells (127, 128). On the contrary, hyperactive angiogenesis promotes the expansion of immunohibitory lymphocytes. Tumor hypoxia resulting from abnormal blood vessels upregulates the expression of chemokines that recruit regulatory T cells (Tregs) into the tumor (129, 130). The hypoxic TME also induces the polarization of tumor-associated macrophages (TAMs) toward an M2-like phenotype, which further supports tumor growth and immune evasion (131). Additionally, Fas ligand expressed by tumor endothelial barriers selectively kills cytotoxic CD8+ T cells, while sparing Tregs due to increased cellular FLICE-inhibitory protein on Tregs (132). Angiogenesis exerts a multifaceted influence on tumor growth and immune evasion. Abnormal angiogenesis hampers TIL infiltration and function while promoting the recruitment and activity of pro-tumor lymphocytes.
3.2 The effects of anti-angiogenesis therapy on the TME
Anti-angiogenesis therapy aims to inhibit tumor angiogenesis and disrupt the abnormal tumor vasculature. Initially, these agents were developed to starve tumors by interfering with neo-vascularization (133). However, monotherapy with anti-angiogenic agents did not yield satisfactory results, as tumors developed mechanisms to tolerate hypoxia, leading to invasiveness and metastasis (134, 135). Nevertheless, anti-angiogenesis therapy has shown promise as a sensitizer when combined with other therapies. The concept of vessel normalization, describes the transient state of tumor vessels undergoing anti-angiogenesis treatment (133). In this state, tumor vessels undergo structural and functional changes, including enhanced perfusion, improved pericyte coverage, and relieved hypoxia. The duration and extent of vessel normalization depend on the treatment schedule and dose (136). Anti-angiogenesis therapy has also been shown to reprogram the TME from an immunoinhibitory to an immunosupportive state (137). Normalized tumor vasculature alleviates hypoxia, which can promote the polarization of TAM to an M1-like phenotype (136). Additionally, vessel normalization reduces the recruitment of Treg and myeloid-derived suppressor cells (MDSC) (138). Improved perfusion resulting from vessel normalization also downregulates hypoxia-mediated immunoinhibitory molecules, such as PD-L1 (139).
Preclinical studies have demonstrated the potential synergistic effect of combining ICB with anti-angiogenesis therapy (140–142). The crosstalk between the TME and angiogenesis suggests that anti-angiogenesis therapy may enhance the therapeutic effects of ICB. Various mechanisms have been proposed to explain this synergy. For example, anti-VEGF treatment can abrogate VEGF-induced immune checkpoint expression on intratumoral T cells (143). Furthermore, the induction of high endothelial venule (HEV) formation through combination therapy has been shown to promote T cell infiltration into tumors (39). Combining anti-angiogenesis therapy with ICI has shown promising results in preclinical studies, suggesting a potential strategy for improving the efficacy of immunotherapy. Further investigations are warranted to fully understand the mechanisms underlying the synergistic effects and to optimize the combination therapy approach.
4 Anti-angiogenesis combined with ICB therapy for ovarian cancer patients
In the phase 1 dose-escalation study NCT02298959, anti-angiogenesis agent ziv-aflibercept (recombinant fusion protein containing VEGF-binding domains developed based on VEGFR1/2) combined with anti-PD-1 antibody pembrolizumab exhibited potent antitumor activity in solid tumors (including ovarian cancer, renal cell carcinoma, colorectal cancer, and melanoma) with manageable safety profile (144). The median overall survival reached 12.5 months (90% CI 3.8 to 13.6) in the ovarian cancer subgroup (144). Besides, in the phase 1b trial NCT04236362, 34 platinum-resistant or refractory ovarian cancer patients were enrolled (145). In this clinical study, anti-angiogenesis agent anlotinib combined with anti-PD-1 antibody TQB2450 also showed encouraging antitumor activity (median PFS: 7.8 months; disease control rate: 97.1%; and objective response rate: 47.1%) (145).
Besides, in the phase 1b study NCT01633970, patients with platinum-resistant ovarian cancer benefited from atezolizumab and bevacizumab treatment (146). This combination therapy achieved a response rate of 15%, and all three patients with partial response had a long duration (11.3-18.9 months) (146). Furthermore, in the single-arm phase 2 study NCT02873962, nivolumab combined with bevacizumab showed promising antitumor potential for relapsed ovarian cancer (overall response rate: 28.9%; 95%CI 15.4%-45.9%), especially for platinum-sensitive patients (overall response rate: 40.0%, 95%CI 19.1%-64.0%) (147). Howbeit, combination treatment might predispose patients to cumulative adverse effects. Therefore, optimizing sequencing of administration of ICB and anti-angiogenesis agents is of importance. Intriguingly, due to the specific mechanism that anti-angiogenesis normalizes the tumor vasculature, a lower dosage of ICB can achieve the same efficacy as before (148). Generally, anti-angiogenesis combined with ICB therapy is a promising strategy for ovarian cancer, and more clinical studies are ongoing to further confirm the efficacy of this combination therapy.
5 Perspective
Angiogenesis, the formation of new blood vessels, plays a crucial role in tumor growth and metastasis. The VEGF/VEGFR and ANGPT/TIE systems are key players in the regulation of angiogenesis. Anti-angiogenesis therapy aims to disrupt tumor angiogenesis by targeting specific factors or receptors involved in this process. Bevacizumab, cediranib, pazopanib, and nintedanib are among the agents that have been investigated in the context of ovarian cancer treatment. While these agents have shown clinical efficacy, challenges remain in terms of optimizing therapeutic responses, managing side effects, and identifying predictive biomarkers. The combination of anti-angiogenesis therapy with ICB has shown promising synergistic effects in preclinical and clinical studies. This combination therapy has demonstrated the ability to increase the ratio of anti-tumor to pro-tumor immune cells, downregulate the expression of multiple immune checkpoints, and reshape the TME. The TME plays a critical role in response to ICB therapy, and angiogenesis has been found to have a significant impact on the status of TILs and the efficacy of ICB. Abnormal angiogenesis creates an immunosuppressive TME, hindering TIL infiltration and function while promoting the abundance of pro-tumor lymphocytes. Anti-angiogenesis therapy can normalize tumor vasculature, alleviate hypoxia, and reprogram the TME to an immunosupportive state.
Clinical trials investigating the combination of ICB and anti-angiogenesis therapy in ovarian cancer have shown encouraging outcomes. The combination has demonstrated improved PFS and overall survival compared to monotherapy. However, challenges remain in terms of patient selection, optimizing treatment regimens, and managing potential side effects. Further research is needed to identify predictive biomarkers that can guide treatment decisions and enhance therapeutic responses. Additionally, efforts should be made to develop more potent and specific anti-angiogenesis agents and explore novel targets in the angiogenesis pathway.
6 Conclusion
Ovarian cancer remains a significant health concern for women worldwide, and novel treatment strategies are urgently needed to improve patient outcomes. The combination of immune checkpoint blockade and anti-angiogenesis therapy has emerged as a promising approach for the treatment of ovarian cancer. Anti-angiogenesis therapy disrupts tumor angiogenesis and reshapes the tumor microenvironment, leading to improved infiltration and function of tumor-infiltrating lymphocytes. The combination therapy has demonstrated promising results in preclinical and clinical studies, with improved PFS and overall survival. Further research and clinical investigations are needed to address these challenges and unlock the full potential of the combination of ICB and anti-angiogenesis therapy in ovarian cancer. Efforts should be made to develop more potent and specific anti-angiogenesis agents and explore novel targets in the angiogenesis pathways. With continued advancements in understanding the complex interplay between angiogenesis and the immune response, this combination therapy holds promise in revolutionizing the treatment landscape for ovarian cancer.
Author contributions
TL: Conceptualization, Supervision, Writing – review & editing, Project administration. PY: Investigation, Writing – original draft. YW: Investigation, Writing – review & editing. DY: Investigation, Writing – review & editing. YS: Investigation, Writing – review & editing. SQ: Conceptualization, Project administration, Supervision, Writing – review & editing.
Funding
The author(s) declare financial support was received for the research, authorship, and/or publication of this article. This project is supported by Nature Science Foundation of Hubei Province (Grant number: 2022CFB988).
Conflict of interest
The authors declare that the research was conducted in the absence of any commercial or financial relationships that could be construed as a potential conflict of interest.
The reviewer LZ declared a shared parent affiliation with the author TL to the handling editor at the time of review.
Publisher’s note
All claims expressed in this article are solely those of the authors and do not necessarily represent those of their affiliated organizations, or those of the publisher, the editors and the reviewers. Any product that may be evaluated in this article, or claim that may be made by its manufacturer, is not guaranteed or endorsed by the publisher.
Abbreviations
VEGF, vascular endothelial growth factor; ANGPT, angiopoietin; ICB, immune checkpoint blockade; PDGF, platelet-derived growth factor; FGF, fibroblast growth factor; VEGFR, VEGF receptor; PFS, Progression free survival; TAM, tumor-associated macrophage; MDSC, myeloid-derived suppressor cell; TIL, tumor-infiltrating lymphocyte; TME, tumor microenvironment.
References
1. Lheureux S, Braunstein M, Oza AM. Epithelial ovarian cancer: Evolution of management in the era of precision medicine. CA Cancer J Clin (2019) 69:280–304. doi: 10.3322/caac.21559
2. Cortez AJ, Tudrej P, Kujawa KA, Lisowska KM. Advances in ovarian cancer therapy. Cancer Chemother Pharmacol (2018) 81:17–38. doi: 10.1007/s00280-017-3501-8
3. Morand S, Devanaboyina M, Staats H, Stanbery L, Nemunaitis J. Ovarian cancer immunotherapy and personalized medicine. Int J Mol Sci (2021) 22(12):6532. doi: 10.3390/ijms22126532
4. Orr B, Edwards RP. Diagnosis and treatment of ovarian cancer. Hematol Oncol Clin North Am (2018) 32:943–64. doi: 10.1016/j.hoc.2018.07.010
5. Jayson GC, Kohn EC, Kitchener HC, Ledermann JA. Ovarian cancer. Lancet (2014) 384:1376–88. doi: 10.1016/s0140-6736(13)62146-7
6. Lheureux S, Gourley C, Vergote I, Oza AM. Epithelial ovarian cancer. Lancet (2019) 393:1240–53. doi: 10.1016/s0140-6736(18)32552-2
7. Yi M, Li T, Niu M, Luo S, Chu Q, Wu K. Epidemiological trends of women's cancers from 1990 to 2019 at the global, regional, and national levels: a population-based study. biomark Res (2021) 9:55. doi: 10.1186/s40364-021-00310-y
8. Chen W, Zheng R, Baade PD, Zhang S, Zeng H, Bray F, et al. Cancer statistics in China, 2015. CA Cancer J Clin (2016) 66:115–32. doi: 10.3322/caac.21338
9. Khalique S, Hook JM, Ledermann JA. Maintenance therapy in ovarian cancer. Curr Opin Oncol (2014) 26:521–8. doi: 10.1097/cco.0000000000000110
10. Guan LY, Lu Y. New developments in molecular targeted therapy of ovarian cancer. Discovery Med (2018) 26:219–29.
11. Kuroki L, Guntupalli SR. Treatment of epithelial ovarian cancer. Bmj (2020) 371:m3773. doi: 10.1136/bmj.m3773
12. Yi M, Dong B, Qin S, Chu Q, Wu K, Luo S. Advances and perspectives of PARP inhibitors. Exp Hematol Oncol (2019) 8:29. doi: 10.1186/s40164-019-0154-9
13. Li T, Wang X, Qin S, Chen B, Yi M, Zhou J. Targeting PARP for the optimal immunotherapy efficiency in gynecologic Malignancies. BioMed Pharmacother (2023) 162:114712. doi: 10.1016/j.biopha.2023.114712
14. Yang C, Xia BR, Zhang ZC, Zhang YJ, Lou G, Jin WL. Immunotherapy for ovarian cancer: adjuvant, combination, and neoadjuvant. Front Immunol (2020) 11:577869. doi: 10.3389/fimmu.2020.577869
15. Grunewald T, Ledermann JA. Targeted therapies for ovarian cancer. Best Pract Res Clin Obstet Gynaecol (2017) 41:139–52. doi: 10.1016/j.bpobgyn.2016.12.001
16. Gupta S, Nag S, Aggarwal S, Rauthan A, Warrier N. Maintenance therapy for recurrent epithelial ovarian cancer: current therapies and future perspectives - a review. J Ovarian Res (2019) 12:103. doi: 10.1186/s13048-019-0579-0
17. O'Malley DM. New therapies for ovarian cancer. J Natl Compr Canc Netw (2019) 17:619–21. doi: 10.6004/jnccn.2019.5018
18. Kurnit KC, Fleming GF, Lengyel E. Updates and new options in advanced epithelial ovarian cancer treatment. Obstet Gynecol (2021) 137:108–21. doi: 10.1097/aog.0000000000004173
19. Ramjiawan RR, Griffioen AW, Duda DG. Anti-angiogenesis for cancer revisited: Is there a role for combinations with immunotherapy? Angiogenesis (2017) 20:185–204. doi: 10.1007/s10456-017-9552-y
20. Chambers A, Kundranda M, Rao S, Mahmoud F, Niu J. Anti-angiogenesis revisited: combination with immunotherapy in solid tumors. Curr Oncol Rep (2021) 23:100. doi: 10.1007/s11912-021-01099-7
21. Niu M, Yi M, Li N, Wu K, Wu K. Advances of targeted therapy for hepatocellular carcinoma. Front Oncol (2021) 11:719896. doi: 10.3389/fonc.2021.719896
22. Qin S, Li A, Yi M, Yu S, Zhang M, Wu K. Recent advances on anti-angiogenesis receptor tyrosine kinase inhibitors in cancer therapy. J Hematol Oncol (2019) 12:27. doi: 10.1186/s13045-019-0718-5
23. Garcia J, Hurwitz HI, Sandler AB, Miles D, Coleman RL, Deurloo R, et al. Bevacizumab (Avastin®) in cancer treatment: A review of 15 years of clinical experience and future outlook. Cancer Treat Rev (2020) 86:102017. doi: 10.1016/j.ctrv.2020.102017
24. Haunschild CE, Tewari KS. Bevacizumab use in the frontline, maintenance and recurrent settings for ovarian cancer. Future Oncol (2020) 16:225–46. doi: 10.2217/fon-2019-0042
25. Nakai H, Matsumura N. The roles and limitations of bevacizumab in the treatment of ovarian cancer. Int J Clin Oncol (2022) 27:1120–6. doi: 10.1007/s10147-022-02169-x
26. Pignata S, Du Bois A, Harter P, Heitz F. Treatment of recurrent ovarian cancer. Ann Oncol (2017) 28:viii51–6. doi: 10.1093/annonc/mdx441. S, C. C.
27. Trédan O, Lacroix-Triki M, Guiu S, Mouret-Reynier MA, Barrière J, Bidard FC, et al. Angiogenesis and tumor microenvironment: bevacizumab in the breast cancer model. Target Oncol (2015) 10:189–98. doi: 10.1007/s11523-014-0334-9
28. Ren S, Xiong X, You H, Shen J, Zhou P. The combination of immune checkpoint blockade and angiogenesis inhibitors in the treatment of advanced non-small cell lung cancer. Front Immunol (2021) 12:689132. doi: 10.3389/fimmu.2021.689132
29. Yi M, Jiao D, Qin S, Chu Q, Wu K, Li A. Synergistic effect of immune checkpoint blockade and anti-angiogenesis in cancer treatment. Mol Cancer (2019) 18:60. doi: 10.1186/s12943-019-0974-6
30. Li SQ, Yang Y, Ye LS. Angiogenesis and immune checkpoint dual blockade: Opportunities and challenges for hepatocellular carcinoma therapy. World J Gastroenterol (2022) 28:6034–44. doi: 10.3748/wjg.v28.i42.6034
31. Song Y, Fu Y, Xie Q, Zhu B, Wang J, Zhang B. Anti-angiogenic agents in combination with immune checkpoint inhibitors: A promising strategy for cancer treatment. Front Immunol (2020) 11:1956. doi: 10.3389/fimmu.2020.01956
32. Yuan J, Khilnani A, Brody J, Andtbacka RHI, Hu-Lieskovan S, Luke JJ. Current strategies for intratumoural immunotherapy - Beyond immune checkpoint inhibition. Eur J Cancer (2021) 157:493–510. doi: 10.1016/j.ejca.2021.08.004
33. Sheng X, Yan X, Chi Z, Si L, Cui C, Tang B, et al. Axitinib in combination with toripalimab, a humanized immunoglobulin G(4) monoclonal antibody against programmed cell death-1, in patients with metastatic mucosal melanoma: an open-label phase IB trial. J Clin Oncol (2019) 37:2987–99. doi: 10.1200/jco.19.00210
34. Kawazoe A, Fukuoka S, Nakamura Y, Kuboki Y, Wakabayashi M, Nomura S, et al. Lenvatinib plus pembrolizumab in patients with advanced gastric cancer in the first-line or second-line setting (EPOC1706): an open-label, single-arm, phase 2 trial. Lancet Oncol (2020) 21:1057–65. doi: 10.1016/s1470-2045(20)30271-0
35. Wilky BA, Trucco MM, Subhawong TK, Florou V, Park W, Kwon D, et al. Axitinib plus pembrolizumab in patients with advanced sarcomas including alveolar soft-part sarcoma: a single-centre, single-arm, phase 2 trial. Lancet Oncol (2019) 20:837–48. doi: 10.1016/s1470-2045(19)30153-6
36. Cui X, Jia H, Xin H, Zhang L, Chen S, Xia S, et al. A novel bispecific antibody targeting PD-L1 and VEGF with combined anti-tumor activities. Front Immunol (2021) 12:778978. doi: 10.3389/fimmu.2021.778978
37. Wu J, Zhao X, Sun Q, Jiang Y, Zhang W, Luo J, et al. Synergic effect of PD-1 blockade and endostar on the PI3K/AKT/mTOR-mediated autophagy and angiogenesis in Lewis lung carcinoma mouse model. BioMed Pharmacother (2020) 125:109746. doi: 10.1016/j.biopha.2019.109746
38. Kato Y, Tabata K, Kimura T, Yachie-Kinoshita A, Ozawa Y, Yamada K, et al. Lenvatinib plus anti-PD-1 antibody combination treatment activates CD8+ T cells through reduction of tumor-associated macrophage and activation of the interferon pathway. PloS One (2019) 14:e0212513. doi: 10.1371/journal.pone.0212513
39. Allen E, Jabouille A, Rivera LB, Lodewijckx I, Missiaen R, Steri V, et al. Combined antiangiogenic and anti-PD-L1 therapy stimulates tumor immunity through HEV formation. Sci Transl Med (2017) 9(385):eaak9679. doi: 10.1126/scitranslmed.aak9679
40. Herbst RS, Arkenau HT, Santana-Davila R, Calvo E, Paz-Ares L, Cassier PA, et al. Ramucirumab plus pembrolizumab in patients with previously treated advanced non-small-cell lung cancer, gastro-oesophageal cancer, or urothelial carcinomas (JVDF): a multicohort, non-randomised, open-label, phase 1a/b trial. Lancet Oncol (2019) 20:1109–23. doi: 10.1016/s1470-2045(19)30458-9
41. Cheng H, Zong L, Kong Y, Wang X, Gu Y, Cang W, et al. Camrelizumab plus apatinib in patients with high-risk chemorefractory or relapsed gestational trophoblastic neoplasia (CAP 01): a single-arm, open-label, phase 2 trial. Lancet Oncol (2021) 22:1609–17. doi: 10.1016/s1470-2045(21)00460-5
42. Atkins MB, Plimack ER, Puzanov I, Fishman MN, McDermott DF, Cho DC, et al. Axitinib in combination with pembrolizumab in patients with advanced renal cell cancer: a non-randomised, open-label, dose-finding, and dose-expansion phase 1b trial. Lancet Oncol (2018) 19:405–15. doi: 10.1016/s1470-2045(18)30081-0
43. Saba NF, Steuer CE, Ekpenyong A, McCook-Veal A, Magliocca K, Patel M, et al. Pembrolizumab and cabozantinib in recurrent metastatic head and neck squamous cell carcinoma: a phase 2 trial. Nat Med (2023) 29:880–7. doi: 10.1038/s41591-023-02275-x
44. Viallard C, Larrivée B. Tumor angiogenesis and vascular normalization: alternative therapeutic targets. Angiogenesis (2017) 20:409–26. doi: 10.1007/s10456-017-9562-9
45. Folkman J. Angiogenesis in cancer, vascular, rheumatoid and other disease. Nat Med (1995) 1:27–31. doi: 10.1038/nm0195-27
46. Folkman J. Tumor angiogenesis: therapeutic implications. N Engl J Med (1971) 285:1182–6. doi: 10.1056/nejm197111182852108
47. Li S, Xu HX, Wu CT, Wang WQ, Jin W, Gao HL, et al. Angiogenesis in pancreatic cancer: current research status and clinical implications. Angiogenesis (2019) 22:15–36. doi: 10.1007/s10456-018-9645-2
48. Yang Y, Sun M, Wang L, Jiao B. HIFs, angiogenesis, and cancer. J Cell Biochem (2013) 114:967–74. doi: 10.1002/jcb.24438
49. Pellegata NS, Berriel Diaz M, Rohm M, Herzig S. Obesity and cancer-extracellular matrix, angiogenesis, and adrenergic signaling as unusual suspects linking the two diseases. Cancer Metastasis Rev (2022) 41:517–47. doi: 10.1007/s10555-022-10058-y
50. Zhou Z, Yao H, Hu H. Disrupting tumor angiogenesis and "the hunger games" for breast cancer. Adv Exp Med Biol (2017) 1026:171–95. doi: 10.1007/978-981-10-6020-5_8
51. Folkman J. Role of angiogenesis in tumor growth and metastasis. Semin Oncol (2002) 29:15–8. doi: 10.1053/sonc.2002.37263
52. Liotta LA, Steeg PS, Stetler-Stevenson WG. Cancer metastasis and angiogenesis: an imbalance of positive and negative regulation. Cell (1991) 64:327–36. doi: 10.1016/0092-8674(91)90642-c
53. Li CY, Shan S, Cao Y, Dewhirst MW. Role of incipient angiogenesis in cancer metastasis. Cancer Metastasis Rev (2000) 19:7–11. doi: 10.1023/a:1026538601947
54. Liu Y, Huang N, Liao S, Rothzerg E, Yao F, Li Y, et al. Current research progress in targeted anti-angiogenesis therapy for osteosarcoma. Cell Prolif (2021) 54:e13102. doi: 10.1111/cpr.13102
55. Munir S, Shah AA, Shahid M, Ahmed MS, Shahid A, Rajoka MSR, et al. Anti-angiogenesis potential of phytochemicals for the therapeutic management of tumors. Curr Pharm Des (2020) 26:265–78. doi: 10.2174/1381612826666191230142638
56. Teleanu RI, Chircov C, Grumezescu AM, Teleanu DM. Tumor angiogenesis and anti-angiogenic strategies for cancer treatment. J Clin Med (2019) 9(1):84. doi: 10.3390/jcm9010084
57. Ribatti D, Nico B, Crivellato E, Roccaro AM, Vacca A. The history of the angiogenic switch concept. Leukemia (2007) 21:44–52. doi: 10.1038/sj.leu.2404402
58. Ferrara N, Gerber HP, LeCouter J. The biology of VEGF and its receptors. Nat Med (2003) 9:669–76. doi: 10.1038/nm0603-669
59. Fagiani E, Christofori G. Angiopoietins in angiogenesis. Cancer Lett (2013) 328:18–26. doi: 10.1016/j.canlet.2012.08.018
60. Seki T, Hosaka K, Lim S, Fischer C, Honek J, Yang Y, et al. Endothelial PDGF-CC regulates angiogenesis-dependent thermogenesis in beige fat. Nat Commun (2016) 7:12152. doi: 10.1038/ncomms12152
61. Matsuki M, Hoshi T, Yamamoto Y, Ikemori-Kawada M, Minoshima Y, Funahashi Y, et al. Lenvatinib inhibits angiogenesis and tumor fibroblast growth factor signaling pathways in human hepatocellular carcinoma models. Cancer Med (2018) 7:2641–53. doi: 10.1002/cam4.1517
62. Folkman J. Antiangiogenesis in cancer therapy–endostatin and its mechanisms of action. Exp Cell Res (2006) 312:594–607. doi: 10.1016/j.yexcr.2005.11.015
63. O'Reilly MS, Holmgren L, Shing Y, Chen C, Rosenthal RA, Moses M, et al. Angiostatin: a novel angiogenesis inhibitor that mediates the suppression of metastases by a Lewis lung carcinoma. Cell (1994) 79:315–28. doi: 10.1016/0092-8674(94)90200-3
64. Bikfalvi A. Platelet factor 4: an inhibitor of angiogenesis. Semin Thromb Hemost (2004) 30:379–85. doi: 10.1055/s-2004-831051
65. Sidky YA, Borden EC. Inhibition of angiogenesis by interferons: effects on tumor- and lymphocyte-induced vascular responses. Cancer Res (1987) 47:5155–61.
66. Kazerounian S, Lawler J. Integration of pro- and anti-angiogenic signals by endothelial cells. J Cell Commun Signal (2018) 12:171–9. doi: 10.1007/s12079-017-0433-3
67. Apte RS, Chen DS, Ferrara N. VEGF in signaling and disease: beyond discovery and development. Cell (2019) 176:1248–64. doi: 10.1016/j.cell.2019.01.021
68. Hicklin DJ, Ellis LM. Role of the vascular endothelial growth factor pathway in tumor growth and angiogenesis. J Clin Oncol (2005) 23:1011–27. doi: 10.1200/jco.2005.06.081
69. Skouras VS, Maragkos C, Grapsa D, Syrigos KN. Targeting neovasculature with multitargeted antiangiogenesis tyrosine kinase inhibitors in non-small cell lung cancer. BioDrugs (2016) 30:421–39. doi: 10.1007/s40259-016-0194-1
70. Akwii RG, Sajib MS, Zahra FT, Mikelis CM. Role of angiopoietin-2 in vascular physiology and pathophysiology. Cells (2019) 8(5):471. doi: 10.3390/cells8050471
71. Saharinen P, Eklund L, Alitalo K. Therapeutic targeting of the angiopoietin-TIE pathway. Nat Rev Drug Discovery (2017) 16:635–61. doi: 10.1038/nrd.2016.278
72. Vanderborght B, Lefere S, Vlierberghe HV, Devisscher L. The angiopoietin/tie2 pathway in hepatocellular carcinoma. Cells (2020) 9(11):2382. doi: 10.3390/cells9112382
73. Hussain RM, Neiweem AE, Kansara V, Harris A, Ciulla TA. Tie-2/Angiopoietin pathway modulation as a therapeutic strategy for retinal disease. Expert Opin Investig Drugs (2019) 28:861–9. doi: 10.1080/13543784.2019.1667333
74. Gillen J, Richardson D, Moore K. Angiopoietin-1 and angiopoietin-2 inhibitors: clinical development. Curr Oncol Rep (2019) 21:22. doi: 10.1007/s11912-019-0771-9
75. Scholz A, Plate KH, Reiss Y. Angiopoietin-2: a multifaceted cytokine that functions in both angiogenesis and inflammation. Ann N Y Acad Sci (2015) 1347:45–51. doi: 10.1111/nyas.12726
76. Nicolini G, Forini F, Kusmic C, Iervasi G, Balzan S. Angiopoietin 2 signal complexity in cardiovascular disease and cancer. Life Sci (2019) 239:117080. doi: 10.1016/j.lfs.2019.117080
77. Parmar D, Apte M. Angiopoietin inhibitors: A review on targeting tumor angiogenesis. Eur J Pharmacol (2021) 899:174021. doi: 10.1016/j.ejphar.2021.174021
78. Mitchell DC, Bryan BA. Anti-angiogenic therapy: adapting strategies to overcome resistant tumors. J Cell Biochem (2010) 111:543–53. doi: 10.1002/jcb.22764
79. Yu J, Zhuang A, Gu X, Hua Y, Yang L, Ge S, et al. Nuclear PD-L1 promotes EGR1-mediated angiogenesis and accelerates tumorigenesis. Cell Discovery (2023) 9:33. doi: 10.1038/s41421-023-00521-7
80. Yetkin-Arik B, Kastelein AW, Klaassen I, Jansen CHJR, Latul YP, Vittori M, et al. Angiogenesis in gynecological cancers and the options for anti-angiogenesis therapy. Biochim Biophys Acta Rev Cancer (2021) 1875:188446. doi: 10.1016/j.bbcan.2020.188446
81. Boocock CA, Charnock-Jones DS, Sharkey AM, McLaren J, Barker PJ, Wright KA, et al. Expression of vascular endothelial growth factor and its receptors flt and KDR in ovarian carcinoma. J Natl Cancer Inst (1995) 87:506–16. doi: 10.1093/jnci/87.7.506
82. Wang JY, Sun T, Zhao XL, Zhang SW, Zhang DF, Gu Q, et al. Functional significance of VEGF-a in human ovarian carcinoma: role in vasculogenic mimicry. Cancer Biol Ther (2008) 7:758–66. doi: 10.4161/cbt.7.5.5765
83. Adams GP, Weiner LM. Monoclonal antibody therapy of cancer. Nat Biotechnol (2005) 23:1147–57. doi: 10.1038/nbt1137
84. Assoun S, Brosseau S, Steinmetz C, Gounant V, Zalcman G. Bevacizumab in advanced lung cancer: state of the art. Future Oncol (2017) 13:2515–35. doi: 10.2217/fon-2017-0302
85. Marquina G, Manzano A, Casado A. Targeted agents in cervical cancer: beyond bevacizumab. Curr Oncol Rep (2018) 20:40. doi: 10.1007/s11912-018-0680-3
86. Markowska A, Sajdak S, Markowska J, Huczyński A. Angiogenesis and cancer stem cells: New perspectives on therapy of ovarian cancer. Eur J Med Chem (2017) 142:87–94. doi: 10.1016/j.ejmech.2017.06.030
87. Reinthaller A. Antiangiogenic therapies in ovarian cancer. Memo (2016) 9:139–43. doi: 10.1007/s12254-016-0282-4
88. Monk BJ, Choi DC, Pugmire G, Burger RA. Activity of bevacizumab (rhuMAB VEGF) in advanced refractory epithelial ovarian cancer. Gynecol Oncol (2005) 96:902–5. doi: 10.1016/j.ygyno.2004.12.001
89. Wang CW, Chang CC, Khalil MA, Lin YJ, Liou YA, Hsu PC, et al. Histopathological whole slide image dataset for classification of treatment effectiveness to ovarian cancer. Sci Data (2022) 9:25. doi: 10.1038/s41597-022-01127-6
90. Monk BJ, Han E, Josephs-Cowan CA, Pugmire G, Burger RA. Salvage bevacizumab (rhuMAB VEGF)-based therapy after multiple prior cytotoxic regimens in advanced refractory epithelial ovarian cancer. Gynecol Oncol (2006) 102:140–4. doi: 10.1016/j.ygyno.2006.05.006
91. Burger RA, Sill MW, Monk BJ, Greer BE, Sorosky JI. Phase II trial of bevacizumab in persistent or recurrent epithelial ovarian cancer or primary peritoneal cancer: a Gynecologic Oncology Group Study. J Clin Oncol (2007) 25:5165–71. doi: 10.1200/jco.2007.11.5345
92. Oza AM, Cook AD, Pfisterer J, Embleton A, Ledermann JA, Pujade-Lauraine E, et al. Standard chemotherapy with or without bevacizumab for women with newly diagnosed ovarian cancer (ICON7): overall survival results of a phase 3 randomised trial. Lancet Oncol (2015) 16:928–36. doi: 10.1016/s1470-2045(15)00086-8
93. Aghajanian C, Blank SV, Goff BA, Judson PL, Teneriello MG, Husain A, et al. OCEANS: a randomized, double-blind, placebo-controlled phase III trial of chemotherapy with or without bevacizumab in patients with platinum-sensitive recurrent epithelial ovarian, primary peritoneal, or fallopian tube cancer. J Clin Oncol (2012) 30:2039–45. doi: 10.1200/jco.2012.42.0505
94. Hardesty MM, Krivak TC, Wright GS, Hamilton E, Fleming EL, Belotte J, et al. OVARIO phase II trial of combination niraparib plus bevacizumab maintenance therapy in advanced ovarian cancer following first-line platinum-based chemotherapy with bevacizumab. Gynecol Oncol (2022) 166:219–29. doi: 10.1016/j.ygyno.2022.05.020
95. Coleman RL, Brady MF, Herzog TJ, Sabbatini P, Armstrong DK, Walker JL, et al. Bevacizumab and paclitaxel-carboplatin chemotherapy and secondary cytoreduction in recurrent, platinum-sensitive ovarian cancer (NRG Oncology/Gynecologic Oncology Group study GOG-0213): a multicentre, open-label, randomised, phase 3 trial. Lancet Oncol (2017) 18:779–91. doi: 10.1016/s1470-2045(17)30279-6
96. Takamatsu S, Nakai H, Yamaguchi K, Hamanishi J, Mandai M, Matsumura N. Time-dependent changes in risk of progression during use of bevacizumab for ovarian cancer. JAMA Netw Open (2023) 6:e2326834. doi: 10.1001/jamanetworkopen.2023.26834
97. Zhou C, Clamp A, Backen A, Berzuini C, Renehan A, Banks RE, et al. Systematic analysis of circulating soluble angiogenesis-associated proteins in ICON7 identifies Tie2 as a biomarker of vascular progression on bevacizumab. Br J Cancer (2016) 115:228–35. doi: 10.1038/bjc.2016.194
98. Tewari KS, Burger RA, Enserro D, Norquist BM, Swisher EM, Brady MF, et al. Final overall survival of a randomized trial of bevacizumab for primary treatment of ovarian cancer. J Clin Oncol (2019) 37:2317–28. doi: 10.1200/jco.19.01009
99. Orbegoso C, Marquina G, George A, Banerjee S. The role of Cediranib in ovarian cancer. Expert Opin Pharmacother (2017) 18:1637–48. doi: 10.1080/14656566.2017.1383384
100. Bizzaro F, Fuso Nerini I, Taylor MA, Anastasia A, Russo M, Damia G, et al. VEGF pathway inhibition potentiates PARP inhibitor efficacy in ovarian cancer independent of BRCA status. J Hematol Oncol (2021) 14:186. doi: 10.1186/s13045-021-01196-x
101. Zhou C, O'Connor J, Backen A, Valle JW, Bridgewater J, Dive C, et al. Plasma Tie2 trajectories identify vascular response criteria for VEGF inhibitors across advanced biliary tract, colorectal and ovarian cancers. ESMO Open (2022) 7:100417. doi: 10.1016/j.esmoop.2022.100417
102. Gopinathan G, Berlato C, Lakhani A, Szabova L, Pegrum C, Pedrosa AR, et al. Immune mechanisms of resistance to cediranib in ovarian cancer. Mol Cancer Ther (2022) 21:1030–43. doi: 10.1158/1535-7163.Mct-21-0689
103. Miyamoto S, Kakutani S, Sato Y, Hanashi A, Kinoshita Y, Ishikawa A. Drug review: pazopanib. Jpn J Clin Oncol (2018) 48:503–13. doi: 10.1093/jjco/hyy053
104. Limvorasak S, Posadas EM. Pazopanib: therapeutic developments. Expert Opin Pharmacother (2009) 10:3091–102. doi: 10.1517/14656560903436493
105. Pignata S, Lorusso D, Scambia G, Sambataro D, Tamberi S, Cinieri S, et al. Pazopanib plus weekly paclitaxel versus weekly paclitaxel alone for platinum-resistant or platinum-refractory advanced ovarian cancer (MITO 11): a randomised, open-label, phase 2 trial. Lancet Oncol (2015) 16:561–8. doi: 10.1016/s1470-2045(15)70115-4
106. du Bois A, Floquet A, Kim JW, Rau J, del Campo JM, Friedlander M, et al. Incorporation of pazopanib in maintenance therapy of ovarian cancer. J Clin Oncol (2014) 32:3374–82. doi: 10.1200/jco.2014.55.7348
107. Floquet A, Vergote I, Colombo N, Fiane B, Monk BJ, Reinthaller A, et al. Progression-free survival by local investigator versus independent central review: comparative analysis of the AGO-OVAR16 Trial. Gynecol Oncol (2015) 136:37–42. doi: 10.1016/j.ygyno.2014.11.074
108. Lamb YN. Nintedanib: A review in fibrotic interstitial lung diseases. Drugs (2021) 81:575–86. doi: 10.1007/s40265-021-01487-0
109. Khalique S, Banerjee S. Nintedanib in ovarian cancer. Expert Opin Investig Drugs (2017) 26:1073–81. doi: 10.1080/13543784.2017.1353599
110. Hughes G, Toellner H, Morris H, Leonard C, Chaudhuri N. Real world experiences: pirfenidone and nintedanib are effective and well tolerated treatments for idiopathic pulmonary fibrosis. J Clin Med (2016) 5(9):78. doi: 10.3390/jcm5090078
111. du Bois A, Kristensen G, Ray-Coquard I, Reuss A, Pignata S, Colombo N, et al. Standard first-line chemotherapy with or without nintedanib for advanced ovarian cancer (AGO-OVAR 12): a randomised, double-blind, placebo-controlled phase 3 trial. Lancet Oncol (2016) 17:78–89. doi: 10.1016/s1470-2045(15)00366-6
112. Ray-Coquard I, Cibula D, Mirza MR, Reuss A, Ricci C, Colombo N, et al. Final results from GCIG/ENGOT/AGO-OVAR 12, a randomised placebo-controlled phase III trial of nintedanib combined with chemotherapy for newly diagnosed advanced ovarian cancer. Int J Cancer (2020) 146:439–48. doi: 10.1002/ijc.32606
113. Ferron G, De Rauglaudre G, Becourt S, Delanoy N, Joly F, Lortholary A, et al. Neoadjuvant chemotherapy with or without nintedanib for advanced epithelial ovarian cancer: Lessons from the GINECO double-blind randomized phase II CHIVA trial. Gynecol Oncol (2023) 170:186–94. doi: 10.1016/j.ygyno.2023.01.008
114. Eroglu Z, Stein CA, Pal SK. Targeting angiopoietin-2 signaling in cancer therapy. Expert Opin Investig Drugs (2013) 22:813–25. doi: 10.1517/13543784.2013.793306
115. Monk BJ, Poveda A, Vergote I, Raspagliesi F, Fujiwara K, Bae DS, et al. Anti-angiopoietin therapy with trebananib for recurrent ovarian cancer (TRINOVA-1): a randomised, multicentre, double-blind, placebo-controlled phase 3 trial. Lancet Oncol (2014) 15:799–808. doi: 10.1016/s1470-2045(14)70244-x
116. Lim D, Do Y, Kwon BS, Chang W, Lee MS, Kim J, et al. Angiogenesis and vasculogenic mimicry as therapeutic targets in ovarian cancer. BMB Rep (2020) 53:291–8. doi: 10.5483/BMBRep.2020.53.6.060
117. Yi M, Zhang J, Li A, Niu M, Yan Y, Jiao Y, et al. The construction, expression, and enhanced anti-tumor activity of YM101: a bispecific antibody simultaneously targeting TGF-β and PD-L1. J Hematol Oncol (2021) 14:27. doi: 10.1186/s13045-021-01045-x
118. Yi M, Niu M, Zhang J, Li S, Zhu S, Yan Y, et al. Combine and conquer: manganese synergizing anti-TGF-β/PD-L1 bispecific antibody YM101 to overcome immunotherapy resistance in non-inflamed cancers. J Hematol Oncol (2021) 14:146. doi: 10.1186/s13045-021-01155-6
119. Cristescu R, Mogg R, Ayers M, Albright A, Murphy E, Yearley J, et al. Pan-tumor genomic biomarkers for PD-1 checkpoint blockade-based immunotherapy. Science (2018) 362(6411):eaar3593. doi: 10.1126/science.aar3593
120. Yi M, Niu M, Wu Y, Ge H, Jiao D, Zhu S, et al. Combination of oral STING agonist MSA-2 and anti-TGF-β/PD-L1 bispecific antibody YM101: a novel immune cocktail therapy for non-inflamed tumors. J Hematol Oncol (2022) 15:142. doi: 10.1186/s13045-022-01363-8
121. Yi M, Wu Y, Niu M, Zhu S, Zhang J, Yan Y, et al. Anti-TGF-β/PD-L1 bispecific antibody promotes T cell infiltration and exhibits enhanced antitumor activity in triple-negative breast cancer. J Immunother Cancer (2022) 10(12):e005543. doi: 10.1136/jitc-2022-005543
122. Chen DS, Mellman I. Oncology meets immunology: the cancer-immunity cycle. Immunity (2013) 39:1–10. doi: 10.1016/j.immuni.2013.07.012
123. Lanitis E, Irving M, Coukos G. Targeting the tumor vasculature to enhance T cell activity. Curr Opin Immunol (2015) 33:55–63. doi: 10.1016/j.coi.2015.01.011
124. Rahma OE, Hodi FS. The intersection between tumor angiogenesis and immune suppression. Clin Cancer Res (2019) 25:5449–57. doi: 10.1158/1078-0432.Ccr-18-1543
125. Li C, Liu T, Bazhin AV, Yang Y. The sabotaging role of myeloid cells in anti-angiogenic therapy: coordination of angiogenesis and immune suppression by hypoxia. J Cell Physiol (2017) 232:2312–22. doi: 10.1002/jcp.25726
126. Teng MW, Ngiow SF, Ribas A, Smyth MJ. Classifying cancers based on T-cell infiltration and PD-L1. Cancer Res (2015) 75:2139–45. doi: 10.1158/0008-5472.Can-15-0255
127. Gabrilovich DI, Chen HL, Girgis KR, Cunningham HT, Meny GM, Nadaf S, et al. Production of vascular endothelial growth factor by human tumors inhibits the functional maturation of dendritic cells. Nat Med (1996) 2:1096–103. doi: 10.1038/nm1096-1096
128. Huang Y, Chen X, Dikov MM, Novitskiy SV, Mosse CA, Yang L, et al. Distinct roles of VEGFR-1 and VEGFR-2 in the aberrant hematopoiesis associated with elevated levels of VEGF. Blood (2007) 110:624–31. doi: 10.1182/blood-2007-01-065714
129. Curiel TJ, Coukos G, Zou L, Alvarez X, Cheng P, Mottram P, et al. Specific recruitment of regulatory T cells in ovarian carcinoma fosters immune privilege and predicts reduced survival. Nat Med (2004) 10:942–9. doi: 10.1038/nm1093
130. Facciabene A, Peng X, Hagemann IS, Balint K, Barchetti A, Wang LP, et al. Tumour hypoxia promotes tolerance and angiogenesis. via CCL28 T(reg) Cells Nat (2011) 475:226–30. doi: 10.1038/nature10169
131. Movahedi K, Laoui D, Gysemans C, Baeten M, Stangé G, Van den Bossche J, et al. Different tumor microenvironments contain functionally distinct subsets of macrophages derived from Ly6C(high) monocytes. Cancer Res (2010) 70:5728–39. doi: 10.1158/0008-5472.Can-09-4672
132. Motz GT, Santoro SP, Wang LP, Garrabrant T, Lastra RR, Hagemann IS, et al. Tumor endothelium FasL establishes a selective immune barrier promoting tolerance in tumors. Nat Med (2014) 20:607–15. doi: 10.1038/nm.3541
133. Jain RK. Normalization of tumor vasculature: an emerging concept in antiangiogenic therapy. Science (2005) 307:58–62. doi: 10.1126/science.1104819
134. Ebos JM, Lee CR, Cruz-Munoz W, Bjarnason GA, Christensen JG, Kerbel RS. Accelerated metastasis after short-term treatment with a potent inhibitor of tumor angiogenesis. Cancer Cell (2009) 15:232–9. doi: 10.1016/j.ccr.2009.01.021
135. Pàez-Ribes M, Allen E, Hudock J, Takeda T, Okuyama H, Viñals F, et al. Antiangiogenic therapy elicits Malignant progression of tumors to increased local invasion and distant metastasis. Cancer Cell (2009) 15:220–31. doi: 10.1016/j.ccr.2009.01.027
136. Huang Y, Yuan J, Righi E, Kamoun WS, Ancukiewicz M, Nezivar J, et al. Vascular normalizing doses of antiangiogenic treatment reprogram the immunosuppressive tumor microenvironment and enhance immunotherapy. Proc Natl Acad Sci U.S.A. (2012) 109:17561–6. doi: 10.1073/pnas.1215397109
137. Fukumura D, Kloepper J, Amoozgar Z, Duda DG, Jain RK. Enhancing cancer immunotherapy using antiangiogenics: opportunities and challenges. Nat Rev Clin Oncol (2018) 15:325–40. doi: 10.1038/nrclinonc.2018.29
138. Du Four S, Maenhout SK, Niclou SP, Thielemans K, Neyns B, Aerts JL. Combined VEGFR and CTLA-4 blockade increases the antigen-presenting function of intratumoral DCs and reduces the suppressive capacity of intratumoral MDSCs. Am J Cancer Res (2016) 6:2514–31.
139. Noman MZ, Desantis G, Janji B, Hasmim M, Karray S, Dessen P, et al. PD-L1 is a novel direct target of HIF-1α, and its blockade under hypoxia enhanced MDSC-mediated T cell activation. J Exp Med (2014) 211:781–90. doi: 10.1084/jem.20131916
140. Wang Q, Gao J, Di W, Wu X. Anti-angiogenesis therapy overcomes the innate resistance to PD-1/PD-L1 blockade in VEGFA-overexpressed mouse tumor models. Cancer Immunol Immunother (2020) 69:1781–99. doi: 10.1007/s00262-020-02576-x
141. Chen JL, Pan CK, Huang YS, Tsai CY, Wang CW, Lin YL, et al. Evaluation of antitumor immunity by a combination treatment of high-dose irradiation, anti-PDL1, and anti-angiogenic therapy in murine lung tumors. Cancer Immunol Immunother (2021) 70:391–404. doi: 10.1007/s00262-020-02690-w
142. Qi X, Yang M, Ma L, Sauer M, Avella D, Kaifi JT, et al. Synergizing sunitinib and radiofrequency ablation to treat hepatocellular cancer by triggering the antitumor immune response. J Immunother Cancer (2020) 8(2):e001038. doi: 10.1136/jitc-2020-001038
143. Meder L, Schuldt P, Thelen M, Schmitt A, Dietlein F, Klein S, et al. Combined VEGF and PD-L1 blockade displays synergistic treatment effects in an autochthonous mouse model of small cell lung cancer. Cancer Res (2018) 78:4270–81. doi: 10.1158/0008-5472.Can-17-2176
144. Rahma OE, Tyan K, Giobbie-Hurder A, Brohl AS, Bedard PL, Renouf DJ, et al. Phase IB study of ziv-aflibercept plus pembrolizumab in patients with advanced solid tumors. J Immunother Cancer (2022) 10(3):e003569. doi: 10.1136/jitc-2021-003569
145. Lan CY, Zhao J, Yang F, Xiong Y, Li R, Huang Y, et al. Anlotinib combined with TQB2450 in patients with platinum-resistant or -refractory ovarian cancer: A multi-center, single-arm, phase 1b trial. Cell Rep Med (2022) 3:100689. doi: 10.1016/j.xcrm.2022.100689
146. Moroney JW, Powderly J, Lieu CH, Bendell JC, Eckhardt SG, Chang CW, et al. Safety and clinical activity of atezolizumab plus bevacizumab in patients with ovarian cancer: A phase ib study. Clin Cancer Res (2020) 26:5631–7. doi: 10.1158/1078-0432.Ccr-20-0477
147. Liu JF, Herold C, Gray KP, Penson RT, Horowitz N, Konstantinopoulos PA, et al. Assessment of combined nivolumab and bevacizumab in relapsed ovarian cancer: A phase 2 clinical trial. JAMA Oncol (2019) 5:1731–8. doi: 10.1001/jamaoncol.2019.3343
Keywords: ovarian cancer, vascular normalization, tumor microenvironment, antitumor immunity, immune infiltration, immunotherapy
Citation: Yu P, Wang Y, Yuan D, Sun Y, Qin S and Li T (2023) Vascular normalization: reshaping the tumor microenvironment and augmenting antitumor immunity for ovarian cancer. Front. Immunol. 14:1276694. doi: 10.3389/fimmu.2023.1276694
Received: 12 August 2023; Accepted: 09 October 2023;
Published: 23 October 2023.
Edited by:
Yujiao Deng, The Second Affiliated Hospital of Xi’an Jiaotong University, ChinaReviewed by:
Linghui Zhou, Zhejiang University, ChinaShiyu Li, The Chinese University of Hong Kong, China
Copyright © 2023 Yu, Wang, Yuan, Sun, Qin and Li. This is an open-access article distributed under the terms of the Creative Commons Attribution License (CC BY). The use, distribution or reproduction in other forums is permitted, provided the original author(s) and the copyright owner(s) are credited and that the original publication in this journal is cited, in accordance with accepted academic practice. No use, distribution or reproduction is permitted which does not comply with these terms.
*Correspondence: Shuang Qin, UWluc19vbmNvQDE2My5jb20=; Tianye Li, aGxqbHR5MTk5M0AxNjMuY29t