- 1Department of Dermatology, West China Hospital, Sichuan University, Chengdu, China
- 2Laboratory of Dermatology, Clinical Institute of Inflammation and Immunology, Frontiers Science Center for Disease-Related Molecular Network and State Key Laboratory of Biotherapy, West China Hospital, Sichuan University, Chengdu, China
- 3Radiation Sciences Research Center, Laser Research Center in Medical Sciences, AJA University of Medical Sciences, Tehran, Iran
- 4International Network for Photo Medicine and Photo Dynamic Therapy (INPMPDT), Universal Scientific Education and Research Network (USERN), Tehran, Iran
Antimicrobial photodynamic therapy (aPDT) has become a potent contender in the fight against microbial infections, especially in the context of the rising antibiotic resistance crisis. Recently, there has been significant interest in polyphenolic natural products as potential photosensitizers (PSs) in aPDT, given their unique chemical structures and inherent antimicrobial properties. Polyphenolic natural products, abundant and readily obtainable from natural sources, are generally regarded as safe and highly compatible with the human body. This comprehensive review focuses on the latest developments and future implications of using natural polyphenols as PSs in aPDT. Paramount polyphenolic compounds, including curcumin, hypericin, quercetin, hypocrellin, celastrol, riboflavin, resveratrol, gallic acid, and aloe emodin, are elaborated upon with respect to their structural characteristics, absorption properties, and antimicrobial effects. Furthermore, the aPDT mechanism, specifically its targeted action on microbial cells and biofilms, is also discussed. Polyphenolic natural products demonstrate immense potential as PSs in aPDT, representing a promising alternate approach to counteract antibiotic-resistant bacteria and biofilm-related infections.
1 Introduction
The pervasive phenomenon of antimicrobial resistance (AMR) in a broad array of pathogenic microorganisms presents a grave and pressing concern for global health and developmental progress (1). Multidrug resistance (MDR) in bacteria culminates in hundreds of thousands of deaths annually (2), underscoring AMR’s role as a profound international health concern (3). Concurrently, the limited availability of effective drugs for fungal infections and the rising resistance to these drugs have led to a distressingly high mortality rate (4–6). Additionally, the unprecedented emergence of SARS-CoV-2 has introduced a global threat to human life and health. The severe implications of antimicrobial resistance on human health and economic systems necessitate the accelerated development of innovative strategies to counteract this formidable issue effectively (7, 8). As shown in Figure 1, in response to this, there has been a surge of research interest dedicated to developing alternative solutions to combat antimicrobial resistance, such as cationic polymers, peptidoglycans, metal nanoparticles, nanocarriers, photodynamic therapy (PDT), and photothermal therapy (PTT) (9, 10).
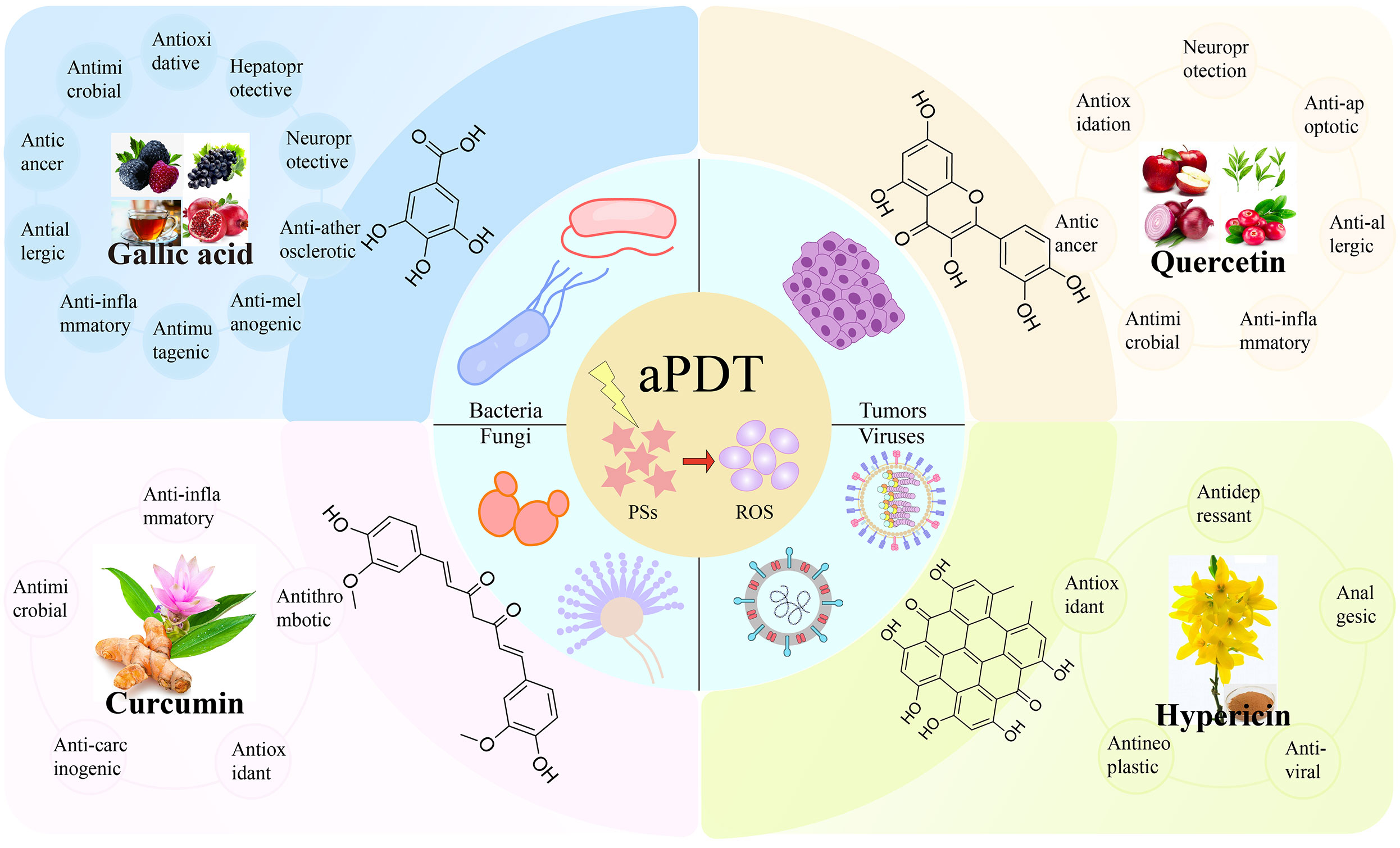
Figure 1 The representative polyphenolic natural products as photosensitizers for antimicrobial photodynamic therapy.
PDT is a therapeutic modality that employs low-energy light to activate photosensitizers (PSs) for both diagnostic and therapeutic purposes. Antimicrobial photodynamic therapy (aPDT), a specific application of PDT, serves as a chemical treatment method to control infections caused by bacteria, fungi, and viruses. As a potent and promising alternative, aPDT strives to mitigate the proliferation of pathogenic microorganisms, encompassing both gram-positive and gram-negative bacteria, fungi, viruses, and parasites. This is achieved by curtailing microbial growth, preventing biofilm formation, and potentially resolving antibiotic resistance issues (11, 12). One notable advantage of aPDT is its noninvasive or minimally invasive nature, which enables a targeted approach primarily against the microorganisms, sparing animal tissue cells from unnecessary damage. This relatively simple and selective approach ensures effective pathogen elimination while minimizing harm to the host (13). The fundamental components of aPDT include the light, PSs, and ambient oxygen. Independently, these elements are benign, but their amalgamation can render a potent antimicrobial effect. This process entails the use of a PS, which, when activated by a particular wavelength of light in the presence of oxygen, generates a copious amount of reactive oxygen species (ROS). These ROS, in turn, interact with multiple targets within microbial cells, inducing the oxidation of biomolecules and ultimately causing cell death.
PSs play an instrumental role in aPDT because they are responsible for absorbing light energy. Various synthetic compounds such as tetrapyrrole macrocycles (porphyrins, phthalocyanines), heterocyclic compounds (methylene blue, toluidine blue O), indocyanine green, and psoralens have been extensively studied for their antibacterial potency in aPDT (14–16). In contrast to synthetic compounds, natural products are generally imbued with more complex chemical structures, granting them unique capabilities in moderating physiological processes and contending with external threats. Derived from natural sources such as plants, animals, and microorganisms, these products acquire unique chemical structures through prolonged evolutionary processes. These structures can engage with molecular entities within organisms, thus intervening in and regulating numerous physiological processes. Among these natural products, polyphenols represent a noteworthy class of compounds found abundantly in various plant-based products, such as vegetables, fruits, seeds, and legumes. Characterized by a series of molecules bearing one or more phenolic rings (17, 18), polyphenols frequently exhibit a diverse array of biological activities, including antioxidant, anticancer, antibacterial, antiviral, and anti-inflammatory properties, which render them potent candidates for the treatment of infections and other diseases (19–21). The significance of polyphenolic natural products in aPDT is underscored by their traditional role as a source for modern drug discovery, offering potential drug leads due to their unique structures, diverse chemical and biological properties, and antimicrobial and anti-inflammatory characteristics (22, 23). Consequently, polyphenolic natural products as PSs have gained considerable attention in the field.
This review focuses on recent advances and future prospects of aPDT for treating microbial infections, with a specific emphasis on the application of polyphenolic natural product PSs (Scheme 1). The unique properties and promising potential of these compounds in combating infections warrant further exploration and development to identify effective therapeutic interventions.
2 Polyphenolic natural PSs
2.1 Curcumin
Curcumin (CUR), a natural polyphenol extracted from the dried rhizomes of the ginger plant turmeric (Curcuma longa L.), has a long history of culinary, traditional medicinal, cosmetic, and herbal supplement use (24). Chemically, curcumin is a diarylheptanoid, a polyphenol, with beta-diketone and enone functionalities, and its structure is related to a dimer of ferulic acid (25). Natural curcumin consists of three distinct curcuminoids: curcumin, demethoxycurcumin (DMC), and bisdemethoxycurcumin (BDMC) (21). The compound demonstrates a broad spectrum of pharmacological effects, including anti-inflammatory, antimicrobial, anticarcinogenic, antioxidant, and antithrombotic activities (26, 27). Curcumin is known for its safety, efficacy, and environmentally friendly characteristics (28). It has also been extensively investigated as a highly effective PS in the field of photodynamic therapy due to its broad absorption range between 300 and 500 nm and its nontoxicity in cell culture models and animal studies (29). Due to its favorable properties, curcumin has been extensively researched for its therapeutic potential and supportive care in clinical conditions such as breast cancer, multiple myeloma, non-small cell lung cancer, and depression (30–33).
As a natural compound, curcumin has been widely investigated as a PS in aPDT. For instance, Li et al. demonstrated the effective eradication of Bacillus subtilis (B. subtilis) through curcumin-mediated PDT by inducing an imbalance in the cellular redox state, causing DNA damage and disrupting membrane structures (13). Wang et al. demonstrated that curcumin (25 μM)-mediated aPDT could inhibit 5 log CFU/ml of Staphylococcus saprophyticus (S. saprophyticus) with the irradiation parameters (430-470 nm, 4.32 J/cm2 10 min) in food production (34). Abdulrahman et al. concluded that curcumin-mediated aPDT inhibits the biofilm formation by 70% of Pseudomonas aeruginosa (P. aeruginosa) with 10 J/cm2 laser light and 6.75 mM of curcumin (35). However, the use of curcumin in aPDT is currently limited to local applications on superficial wounds, such as the skin and oral cavity, primarily due to its absorption of blue light within the light spectrum (300-500 nm), which has restricted tissue penetration capabilities. Muniz et al. demonstrated that curcumin (100 µg), as a PS being activated ex vivo by LED (450 nm, 13.5 J/cm2), effectively controlled Staphylococcus aureus (S. aureus) infections in mice with type 1 diabetes mellitus (36). Méndez et al. found that curcumin-mediated aPDT effectively reduced the viability of microbial cells and compromised the vitality of intact biofilms of infected dentin caries microcosms (37). Moreover, curcumin-mediated aPDT has shown efficacy against various pathogens, including Escherichia coli (E. coli, inactivated up to 3 log CFU/mL), Listeria innocua (L. innocua, inactivated more than 5 log CFU/mL) in food systems (38), Propionibacterium acnes (P. acnes, inhibition ratio was 100%) associated with acne vulgaris (39), significantly decreased planktonic Streptococcus mutans (S. mutans) and S. mutans biofilm (2 log10 CFU/mL reductions) in dental caries (40, 41), complete kill of Aggregatibacter actinomycetemcomitans (A. actinomycetemcomitans) (42), methicillin-resistant S. aureus biofilm (2.03 log10 CFU/mL reductions) (24), and fungi such as Candida albicans (C. albicans, 1 log reductions) and other stains of the Candida spp. (43) Table 1 for a detailed description of the application of polyphenols as PSs in aPDT.
However, the excellent biological and pharmacological activities of curcumin are hindered by its inherent physicochemical properties, including low solubility, rapid metabolization, instability, and the presence of a negative charge state, which hampers effective contact and adhesion to the surfaces of bacteria with negative charge (72). Extensive research has been conducted to address these challenges, particularly through the exploration of an ideal nanocarrier for curcumin (73–75). Additionally, optimizing the formulation and delivery methods of curcumin-based aPDT is crucial to overcome limitations related to tissue penetration. Further research is necessary to improve the bioavailability and absorption of curcumin, maximizing its efficacy in medical and health applications.
2.2 Hypericin
Hypericin (HYP), a naturally occurring pigment isolated from hypericum plants of the genus Hypericum perforatum (commonly referred to as Saint John’s Wort), is well-known for its antidepressant, antioxidant, antineoplastic, potential antiviral and analgesic activities. It has recently been recognized as an effective and promising PS agent found in nature (44, 45). HYP, an anthraquinone derivative exhibits a high quantum yield for the generation of ROS and a slow rate of photobleaching (49, 76). It can also be synthesized from emodin, another anthraquinone derivative (77). The optical properties of HYP enable its absorbance of electromagnetic radiation within the visible spectrum range of 500-620 nm, with a peak absorbance at 595 nm. Upon light exposure, it displays strong red fluorescence, typically emitted at approximately 603 nm, contributing to its intense red fluorescence characteristics (78). HYP exhibits high lipophilicity and poor water solubility, displaying multiple absorption peaks in organic solvents within its visible spectrum, notably at 550 nm and 588 nm in ethanol. Additionally, emodin in ethanol exhibits fluorescence emission at approximately 600 nm. However, when dissolved in aqueous solutions, HYP tends to form nonfluorescent high-molecular-weight aggregates (79, 80).
Recently, there has been increasing interest in investigating the pharmaceutical potential of HYP as a PS in aPDT. Barroso et al. demonstrated effective antimicrobial activity of aPDT using HYP as a PS against P. acnes biofilms and highlighted its potential for clinical treatment of acne vulgaris (44). Kashef et al. investigated the high phototoxicity of HYP against S. aureus, Enterococcus faecalis (E. faecalis), and E. coli at extremely low drug concentrations. While observing minimal cytotoxic effects on cultured human fibroblast cells (46). Aponiene et al. showed efficient elimination of food-borne pathogen Bacillus cereus (B. cereus) through hypericin-based photosensitization in both in vitro experiments and on the surfaces of fruits and vegetables (45). Paz-Cristobal et al. confirmed the greater efficacy of HYP at lower concentrations against azole-resistant C. albicans (47). In a study by Alam et al., the effectiveness of PDT against P. aeruginosa, a gram-negative bacterium with limited PS penetration, was enhanced by combining HYP with ampicillin. This combination acted as a permeabilizing agent, disrupting the bacterial cell wall and increasing cell permeability, thereby maximizing the efficacy of PDT (48). Additionally, Kashef et al. demonstrated the efficacy of combining HYP with acetylcysteine in reducing biofilm formation and disrupting mature biofilms across various bacterial strains, notably, against S. aureus, a prominent pathogen (49).
Despite its desirable properties such as a high quantum yield of singlet oxygen generation, low dark toxicity, a high extinction coefficient near 600 nm, and significant inhibition of gram-positive bacterial growth, the utilization of HYP in biological applications is limited by its high lipophilicity and water insolubility in its natural form. Consequently, its potential in biopharmaceuticals is constrained, and its clinical implementation faces substantial hurdles. Therefore, the development of a delivery system is crucial to overcome these limitations. Various delivery systems, including polymeric nanoparticles and liposomes, have been extensively explored for HYP, showing promising results (76, 81–83).
2.3 Quercetin
Quercetin (QCT), a natural polyphenol, belongs to the subclass of flavonols, one of the six subclasses of flavonoid compounds (84). It is abundantly found in various fruits and vegetables such as apples, grapes, onions, and tomatoes, as well as beverages such as tea and red wine, nuts and honey, from different plant sources (50, 84). As a secondary metabolite, QCT exhibits a diverse array of pharmacological activities, including neuroprotection, antioxidation, antimicrobial, anticancer, anti-inflammatory, and anti-allergic and anti-apoptotic effects (50, 51). QCT demonstrates distinct absorption peaks at 380 and 258 nm (85), and its biological efficacy is significantly enhanced at micromolar concentrations when activated by light within the range of 405 ± 10 nm (51).
Despite limited research on the application of QCT as a PS in aPDT, some studies have explored its correlation and potential. One study demonstrated that QCT-mediated aPDT significantly reduced the growth of E. coli and Listeria monocytogenes (L. monocytogenes) in a buffer solution, indicating its potential as an antimicrobial agent against these bacteria (52). Pourhajibagher et al. utilized QCT with a light-emitting diode to effectively reduce the growth of A. baumannii biofilms and downregulate genes involved in the biofilm formation (53). Condat et al. developed synthetic photoactivable glycerol-based coatings incorporating QCT, which demonstrated a remarkable 99% inhibition of S. aureus proliferation after 2 and 6 hours of incubation under light activation (50). Another study conducted by Pourhajibagher et al. demonstrated that the synergistic combination of blue laser and low-concentration nanoquercetin can disrupt the microbial biofilm of S. mutans and reduce its metabolic activity (51). However, further research is necessary to evaluate the antibacterial pharmacological activity of QCT and determine its potential value in clinical applications.
2.4 Hypocrellins
Hypocrellins, primarily composed of hypocrellin A and B, which are perylenoquinone derivatives, are obtained from the fruiting bodies of the traditional Chinese medicine fungi Hypocrella bambusae and Shiraia bambusae (86, 87). Hypocrellins, structurally related to HYP, are predominantly lipophilic, although a few hydrophobic hypocrellin derivatives have been synthesized, with limited studies on their properties (86, 88). Structurally, hypocrellin A (HA) and hypocrellin B (HB) exhibit a high degree of similarity, differing only by the presence of a single hydroxyl group (59, 89). Hypocrellins exhibit several advantageous characteristics, including a notable quantum yield for singlet oxygen (1O2) generation, strong generation of anionic free radicals in deoxygenated environments, rapid clearance from normal tissues, minimal dark toxicity, and existence in a pure monomeric form. These exceptional attributes have led to the extensive utilization of hypocrellin as a PS in photodynamic therapy (89). In ethanol, HA exhibits three distinct absorption peaks at 581 nm, 542 nm, and 463 nm, within the visible light spectrum range of 400 - 700 nm (54). The absorption wavelength of HB ranges from 450 nm to 550 nm (90).
Hypocrellins have been extensively studied for their potential applications in treating various dermatological conditions, and viral infections, including human immunodeficiency virus (HIV), and even cancer (91). Due to their unique characteristics, such as ease of preparation and purification, high photoreactivity with low dark toxicity (92), and rapid tissue clearance, hypocrellins have garnered significant attention as novel therapeutic agents and/or diagnostic tools (87, 91). In PDT, HA plays a crucial role in anticancer treatment (93). However, research on the antimicrobial photodynamic activity of HA is limited and primarily focused on C. albicans (55), Candida auris (C. auris) (56, 57), and methicillin-resistant S. aureus (58). Nonetheless, the efficacy of HA is limited by certain characteristics, including poor water solubility, tendency to aggregate under physiological conditions, and limited absorption within the phototherapeutic window, which restricts its clinical application in PDT. To overcome these limitations, Guo and colleagues developed a self-assembled amphiphilic micelle that is sensitive to lipase, enabling efficient delivery of HA. The micelles composed of mPEG-PCL/HA demonstrated promising antimicrobial activity against methicillin-resistant Staphylococcus aureus (MRSA) (58). In another study, Liu et al. prepared a recyclable and light-triggered nanofibrous membrane of polylactic acid conjugated with HA and modified porous organic cages with HA for targeting C. auris and multidrug-resistant Candida species, respectively (56, 57). Similarly, research on HB primarily revolves around its antitumor and antiviral properties. Studies have revealed that HB demonstrates potent photodynamic effects against malignant tumors, human immunodeficiency virus type I (HIV-I), and herpetic stomatitis (90). In their in vitro experiments, Hu et al. demonstrated that HB-LED PDT triggers apoptosis in human keloid fibroblasts through the mitochondrial apoptotic pathway (89). Moreover, Hashimoto et al. found that HB-mediated aPDT exhibits promise as a viable alternative treatment for P. aeruginosa-infected burns, as it effectively reduces P. aeruginosa at the infection site, delays bacteremia, maintains lower bacterial levels in the bloodstream compared to untreated groups, and significantly increases the lifespan of mice (60). The Jan group investigated the photodynamic inactivation effects of HB on both azole-sensitive and azole-resistant strains of C. albicans in vitro. HB exhibited negligible dark toxicity and efficiently deactivated C. albicans cells in a light-dose and PS concentration-dependent manner (59). Recently, Law et al. proposed HB as a potential PS for PDT in the treatment of SARS-CoV-2 (94). These innovative approaches hold great potential for enhancing therapeutic outcomes in the treatment of microbial infections.
2.5 Resveratrol
Resveratrol, also known as trans-3,4,5-trihydroxystilbene, is a naturally derived polyphenolic compound and phytoalexin. It is synthesized in response to various stressors, including plant damage or microbial infections caused by bacteria or fungi (95). Resveratrol is commonly found in a variety of dietary substances, such as grapes, berries (cranberries), red wine, nuts (peanuts) and other foods (96–98). Chemically, it belongs to the stilbene family and acts as a fundamental precursor for the synthesis of other stilbenes, such as piceatannol and pterostilbene (specifically trans-3,5-dimethoxy-4’-hydroxystilbene) (96). Resveratrol presents a diverse array of biological activities, encompassing antimicrobial, antiviral, antioxidant, anti-aging, anti-inflammatory, and anticancer properties. Moreover, it has been recognized for its cardioprotective and neuroprotective attributes (99). These notable biological functions can be attributed to its unique molecular structure, which enables effective interactions with various biomolecules. Resveratrol displays a wide absorption spectrum ranging from 290 nm to 360 nm, with a peak wavelength observed at approximately 320 nm (100).
The antimicrobial activity of resveratrol has been studied extensively. Klančnik et al. reported a minimum inhibitory concentration (MIC) of 0.313 mg/ml for resveratrol against Campylobacter jejuni (101). In contrast, Duracka et al. found no significant bactericidal activity of resveratrol against Enterococcus faecalis in rabbit ejaculates (102). Li et al. discovered that resveratrol, at a concentration of 800 µg/mL, significantly inhibits the growth of S. mutans (96). Furthermore, Kugaji et al. demonstrated remarkable antibacterial and anti-biofilm activity of resveratrol against Porphyromonas gingivalis (P. gingivalis), a bacterium associated with gum disease (99). Dos Santos et al. were the first to establish a connection between aPDT and resveratrol, highlighting its effective inhibition of S. aureus when used as a PS (61). Resveratrol as a natural polyphenol compound, exhibits therapeutic potential. However, it is pertinent to acknowledge that the stability of the resveratrol can be influenced by factors such as UV radiation, pH, and temperature (103).
2.6 Gallic acid
Gallic acid (GA) (3,4,5-trihydroxybenzoic acid), a natural polyphenolic compound, is abundant in various plants, including trees, herbs, fruits, and nuts, as well as processed beverages such as red wine and green tea (104). Recognized for its inherent and potent biological activities, GA exhibits a diverse range of effects, encompassing antioxidative, antimicrobial, antiallergic, anticancer, anti-inflammatory, antimutagenic, anti-melanogenic, anti-atherosclerotic, neuroprotective, and hepatoprotective properties (105, 106). Its versatile applications span multiple fields, such as medicine, chemical research, pharmaceuticals, cosmetics, and the food industry (107). The polyphenolic functional groups present in GA contribute to its remarkable ability to scavenge oxygen-derived free radicals (108). Moreover, GA is commonly employed as a standard compound for quantifying phenol content using the Folin-Ciocalteu method (109). Derived from protocatechuic acid, GA serves as an intermediate in the secondary metabolism of plants (108). Structurally, GA is a phenolic acid consisting of benzene ring with a carboxyl group and three hydroxyl groups attached to it. Its formation can be obtained through the acid hydrolysis of hydrolysable tannins (110). It has the capability to absorb ultraviolet (UV) irradiation and light in the visible spectrum (111).
GA has demonstrated remarkable inhibitory effects on the motility, adhesion, and biofilm formation of S. aureus, S. pyogenes, P. aeruginosa, and L. monocytogenes (112–114). In an insightful study by Cossu et al., GA treatment combined with UV-A irradiation significantly inactivated metabolically active E. coli O157:H7 (62). Furthermore, De Oliveira et al. demonstrated that the synergistic combination of GA with lactic acid (LA) and UV-A was specifically effective against E. coli O157:H7 (63). A study conducted by Nakamura et al. investigated the antibacterial effect of GA (4 mmol/L) on S. aureus under LED light irradiation, resulting in a 99.9% reduction in bacteria. Notably, the authors suggest that the antibacterial action is induced by photooxidation and automatic oxidation of GA, as its individual bactericidal effect is less pronounced (64).
2.7 Aloe emodin
Aloe emodin (AE) is a naturally occurring anthraquinone derivative with structural similarity to HYP. It is extracted from traditional Chinese medicine (TCM) plants such as Aloe vera, Rheum officinale Baill., Rumex patientia Linn., Cassia mimosoides L. and Polygonum multiflorum Thunb (115, 116). AE shares a remarkable chemical structure resemblance to HYP, an extensively studied classical PS, and exhibits light absorption capability in the ultraviolet-visible regions. AE displays three primary absorption bands centered at 250 nm, 284 nm and 430 nm. Light sources within the blue region, including lasers emitting wavelengths of 405 nm, 430 nm, and 473 nm, as well as broadband light using suitable filters, effectively activate AE (65). The maximum absorption band of AE in the blue region makes AE-mediated PDT particularly advantageous for the treating of superficial diseases, including skin cancer, oral disorders, and ocular conditions. The singlet oxygen quantum yield (1O2) of AE was determined to be 0.57 (2) in methanol, which is marginally higher than that of methylene blue (117).
Recently, AE has gained increasing attention due to its potential applications in the treatment of various diseases. Several studies have indicated that aloin, a compound found in aloe vera, possesses various biological properties, including antiviral, antibacterial, anti-inflammatory, and hepatoprotective activities (118–120). Moreover, AE has demonstrated anticancer activity against lung squamous cell carcinoma, neuroectodermal tumors, hepatocellular carcinoma cells, gastric cancer cells, and colon cancer cells (121, 122). However, AE exhibits low solubility in aqueous medium (~19 μM), leading to poor oral absorption and bioavailability (123). Furthermore, long-term administration of AE may result in genotoxicity, including gene lesions and mutations, and pose potential risks such as the occurrence of acute renal failure. These factors constrain the widespread application of AE in the medical field. Consequently, research efforts aimed at enhancing the aqueous solubility of AE assume significant importance as they can substantially improve its bioavailability (124–126).
Nanomaterials are widely recognized as exceptional drug carriers due to their good biodistribution, enhanced bioavailability, and low drug toxicity. Li et al. developed AE-encapsulated nanoliposomes using reverse evaporation to improve the bioavailability of AE against human gastric cancer cells (126). Unfortunately, there have been few studies on nanomaterials for AE-mediated aPDT. AE has emerged as a promising agent for aPDT, garnering considerable attention for the treatment of surface or localized bacterial infections in recent years. Studies conducted by Li and Wang et al. provide evidence that AE-mediated aPDT is highly effective in inactivating in vitro isolates of MDR Acinetobacter baumannii (A. baumannii) and successfully treating infections caused by MDR A. baumannii following thermal burn injuries in mice. In summary, AE, as an exceptionally promising PS, exhibits tremendous potential in the context of managing of superficial infections caused by MDR A. baumannii through aPDT (65, 127). Ma et al. confirmed that AE-aPDT exhibited significant efficacy in the inactivation of C. albicans cells in a concentration-dependent manner by causing damage to the cell wall, cytoplasm, and nuclei (66). Additionally, the research conducted by Ma et al. demonstrated that AE is highly effective in inactivating Trichophyton rubrum (T. rubrum) microconidia in a light dose-dependent manner, exhibiting substantial inhibitory effects on the growth of T. rubrum (67). Cui et al. reported the in vitro photodynamic antimicrobial efficacy of AE on Malassezia furfur (M. furfur), a lipo-dependent yeast fungus frequently found on the skin. The findings revealed that AE-mediated aPDT demonstrated remarkable effectiveness in inactivating the fungal cells in a concentration- and light energy dose-dependent manner (68). These results suggest the potential application of AE-aPDT as a promising therapeutic option for addressing M. furfur-related skin conditions.
2.8 Celastrol
Tripterygium wilfordii Hook F. (Tripterygium wilfordii), is an ivylike vine belonging to the Celastraceae family, widely employed as a traditional natural medicine in Chinese traditional medicine (128). The main chemical constituents of Tripterygium wilfordii include diterpenoids, triterpenoids and alkaloids, with triptolide and celastrol being the most studied and clinically applied components (129). Tripterygium wilfordii exhibits a range of pharmacological activities, including anti-inflammatory, immunomodulatory, anticancer, and anti-rheumatic effects. As a result, it finds extensive application in the treatment of autoimmune diseases, encompassing rheumatoid arthritis and systemic lupus erythematosus (130, 131). Furthermore, Tripterygium wilfordii has demonstrated anticancer activity and is currently under investigation as a potential anticancer drug (128). Alam et al. conducted a study exploring the application of a natural PS derived from the medicinal plant Tripterygium wilfordii for aPDT. The ethanolic extract and PS-enriched fraction contained six demethylated chlorophyll derivatives as active compounds. The combined treatment of red light (660 nm) and the natural PS effectively eradicated pathogenic bacteria and fungi, particularly various skin pathogens in vitro. The in vivo efficacy and adverse reactions of aPDT were evaluated using a nematode model infected with S. aureus and Streptococcus pyogenes (69).
Celastrol is a quinone methide triterpenoid natural compound that possesses a broad range of antiviral, anti-inflammatory, and anticancer properties (132). In a previous investigation, titanium dioxide (TiO2) nanofibers conjugated with celastrol were employed for the treatment of HepG2 cancer cells with ultraviolet A (254 nm) (128). Caruso et al. conducted a study investigating the mechanism of action of celastrol at the active site of the main SARS-CoV-2 protease, 3CLpro, employing various techniques. Their findings suggest that celastrol could potentially serve as a PS in photodynamic therapy against SARS-CoV-2 (132, 133).
2.9 Riboflavin
Riboflavin, scientifically termed vitamin B2, is a water-soluble vitamin with inherent photodynamic properties. It can be found in various food sources such as dairy products (milk and cheese), meat, fish, fruits, dark green leafy vegetables, bread, grains, and grain products (134). Chemically, riboflavin comprises an isoalloxazine ring attached to a ribitol side chain and exists in two coenzyme forms: flavin mononucleotide (FMN) and flavin adenine dinucleotide (FAD). These key cofactors play a pivotal role in energy metabolism as indispensable components of oxidation-reduction enzymes, reductases, and dehydrogenases (134, 135). Riboflavin, a potent light-activated free-radical producer, exhibits absorption maxima at 270 nm, 366 nm, and 445 nm, facilitating efficient generation of ROS (135, 136).
Riboflavin plays an indispensable role in maintaining human health and has exhibited the ability to hinder the growth of a diverse spectrum of microorganisms, encompassing bacteria, viruses, fungi, and parasites, suggesting its potential as an effective antimicrobial agent (134). Its biocompatibility, nontoxic characteristics, and ROS generation capacity have attracted significant attention among researchers, particularly in the field of dentistry (135). In aPDT, riboflavin serves as both a photosensitizer and a crosslinking agent. Its multifunctional properties extend beyond reducing inflammation and eradicating microbial biofilms to preserving adhesive strength in orthodontic brackets (135, 136). Studies by Maisch et al. and Mahsa et al. have showcased the safety and effectiveness of riboflavin-based aPDT in eradicating multidrug-resistant bacteria such as S. aureus, E. coli, P. aeruginosa, A. baumannii, and E. faecalis biofilm. Despite the widespread use of riboflavin as a PS in aPDT, its water-soluble nature limits its incorporation rate in diverse biological tissues. Consequently, numerous studies have focused on enhancing its bioavailability by employing riboflavin derivatives or nanodelivery systems. Zhang et al. demonstrated that riboflavin formulated into a nanoemulsion exhibited potent bactericidal effects against S. aureus cell membranes (70, 71, 137). Additionally, Du et al. found that supramolecular materials loaded with riboflavin were capable of killing gram-positive bacteria (e.g., S. aureus), gram-negative bacteria (e.g., E. coli), and multidrug-resistant S. aureus (71). These approaches aim to overcome the challenges associated with riboflavin solubility and improve its effectiveness in aPDT.
3 The photochemical mechanism and targets of aPDT
aPDT relies on the generation of ROS by PSs upon exposure to specific wavelengths of light. This process involves the transfer of electrons or energy from the excited PSs to molecular oxygen (138), leading to photochemical reactions of Type I or Type II (139). In type I reactions, the excited PS transfers high-energy electrons to nearby molecules, often molecular oxygen, resulting in the production of ROS, including hydrogen peroxide (H2O2), superoxide anion (O2•-), and hydroxyl radical (·OH), among others (134, 140). Type II reactions involve the transfer of energy from the PS to oxygen, generating highly reactive singlet oxygen (1O2) (52). These two reaction types induce oxidative stress and cellular damage, ultimately leading to cell death. The equilibrium between Type I and Type II reactions can be influenced by specific substrates, PSs, and oxygen levels (141). Recently, a novel mechanism termed the “Type III photochemical pathway” has been proposed, which is an oxygen-independent mechanism for antimicrobial photoinactivation. Currently, this mechanism has been primarily observed under anaerobic/hypoxic conditions, involving PSs such as psoralens and tetracyclines, as well as the addition of organic salts such as potassium iodide and sodium azide (142, 143).
aPDT is a multitarget process that inflicts damage on multiple levels. Natural product PSs can be categorized into three distinct types according to their proximity and interaction with bacterial cells: (i) PSs positioned in close proximity to the bacterial cell wall, (ii) PSs exhibiting affinity for bacterial cells, potentially causing oxidative damage to extracellular structures, and (iii) PSs capable of penetrating bacterial cells and reaching the cytoplasm, thereby exerting detrimental effects on intracellular components such as cytoplasmic proteins or DNA (144). Overall, aPDT operates through ROS generation and subsequent oxidative damage, with PSs targeting various cellular components depending on their location and interaction with bacterial cells. Understanding the mechanism and targets of aPDT is crucial for optimizing treatment strategies and developing effective antimicrobial interventions (Figure 2).
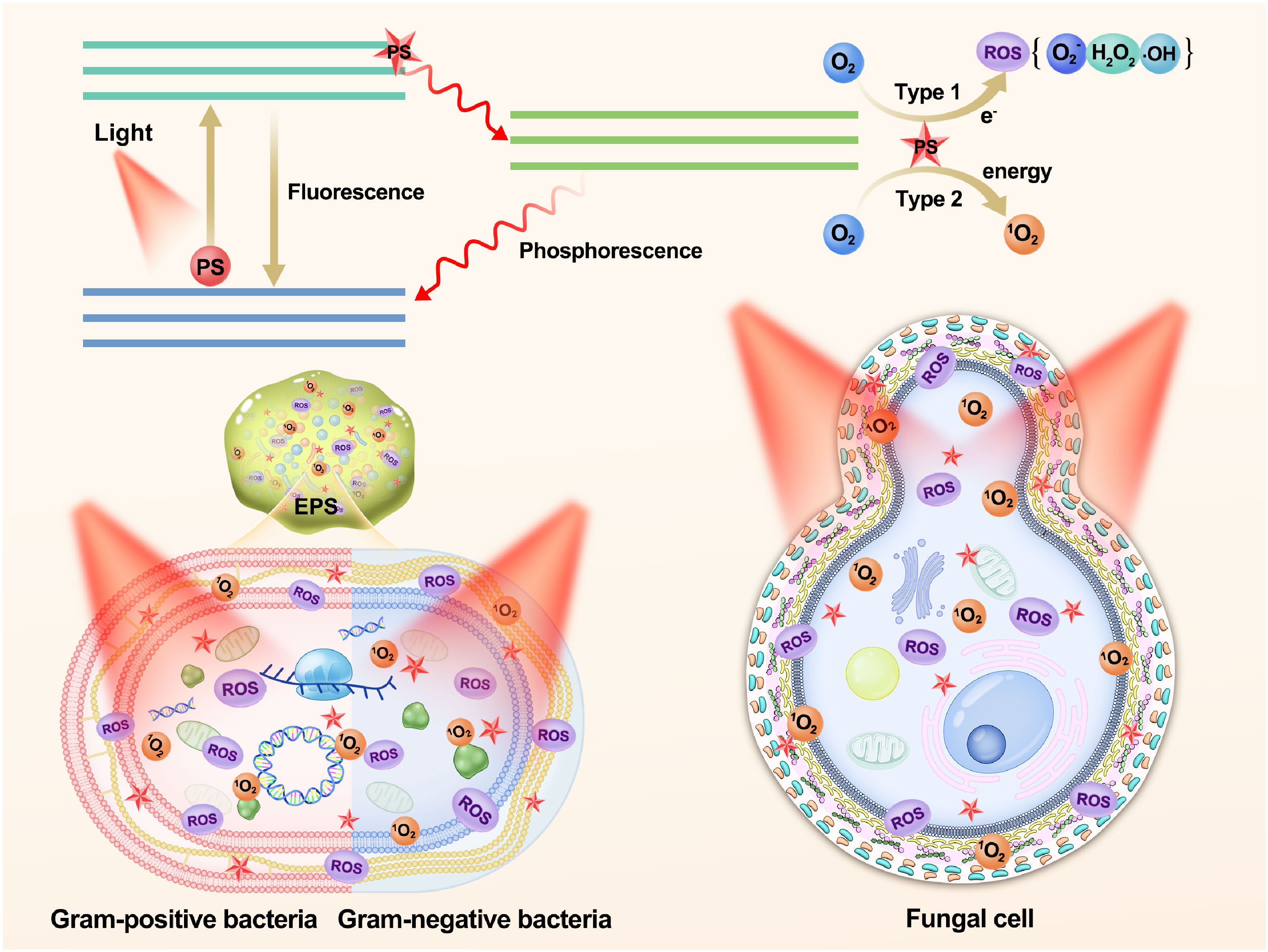
Figure 2 Schematic illustration of the photochemical mechanisms and the role of polyphenols as photosensitizers in aPDT targeting biofilms, Gram-positive bacteria, Gram-negative bacteria, and fungal cell. PS, photosensitizer; ROS, reactive oxygen species; EPS, extracellular polymeric substances.
3.1 biofilm
The formation of biofilms involves the adhesion and aggregation of bacteria on living or nonliving surfaces. Biofilms exhibit a complex and organized structure, providing protection and facilitating the survival and growth of the microorganisms within the community (145, 146). They represent a distinct lifestyle from planktonic states and serve as a survival strategy for microorganisms in challenging environments (15, 147). Extracellular polymeric substances (EPS), comprising proteins, extracellular DNA (eDNA), polysaccharides, humic substances, and water-insoluble compounds, such as cellulose, amyloid proteins, nonamyloid protein fibers, and lipids, surround and immobilize biofilm cells (148). Biofilms shield microorganisms from host defense systems, increasing their tolerance to various antibiotics and disinfectants. which can result in persistent and difficult-to-treat infections (149, 150). However, polyphenolic natural product-mediated aPDT has shown significant potential in targeting biofilms and inactivating clinically relevant microorganisms. Minhaco et al. reported that curcumin-loaded PLGA nanoparticles presented effective antimicrobial activity against endodontic biofilms. Notably, encapsulated curcumin demonstrated potent antibacterial effects on both mono- and multispecies biofilms (e.g., E. faecalis, S. mutans, and Streptococcus oralis) at a lower concentration (29). A study by Ribeiro et al. demonstrated that curcumin-mediated aPDT, when irradiated with LED light, effectively generated photoproducts, and ROS, such as singlet oxygen and free radicals, inducing phototoxicity. Thus, PDT with curcumin significantly reduced the viability of MRSA strains in biofilms (24).
Hypericin-mediated aPDT has shown effective activity against both methicillin-susceptible and methicillin-resistant S. aureus biofilms, as evidenced in the study conducted by García et al. (151); nevertheless, inactivation of S. aureus biofilms was not achieved with HYP alone, as shown in the study by Kashef et al. Interestingly, the combination of HYP with acetylcysteine exhibited remarkable efficacy in eradicating the preformed mature biofilms of S. aureus strains. The authors hypothesized that acetylcysteine’s ability to degrade the extracellular polysaccharide matrix of the biofilm enhances the susceptibility of biofilm-associated bacteria to the phototoxic properties of HYP (49). Xiang et al. observed that AE does not disrupt the anchoring of surface proteins to the cell wall. Instead, its inhibitory effect on biofilm development was attributed to the downregulation of specific surface protein expression or the direct obstruction of adhesion of these proteins to other matrix components (119).
3.2 Cell wall and cell membrane
Bacteria consist of three primary components: the cell wall, cell membrane, and cytoplasm (152). The cytoplasmic membrane shares a similar structure in both gram-negative bacteria and gram-positive bacteria, consisting of a phospholipid bilayer along with minor lipids and proteins (153). However, extensive research has demonstrated that gram-positive bacteria exhibit higher sensitivity to aPDT than gram-negative bacteria due to differences in their cell wall structures. Gram-positive cells have a single thick peptidoglycan layer surrounding their cytoplasmic membrane, resulting in higher porosity of their cell walls. Consequently, this increased porosity facilitates easier diffusion of the PSs into the intracellular space. In contrast, gram-negative bacteria possess a highly selective and complex outer membrane composed of lipopolysaccharides, lipoproteins and lipoteichoic acids, along with a thin peptidoglycan layer. These factors collectively make the penetration of PSs significantly more challenging (154, 155). In the study, Wang et al. demonstrated that quercetin had the ability to disrupt the cell wall and cell membrane structures in both gram-positive and gram-negative bacteria. This disruption increased the permeability of these structures, leading to the release of cellular cytoplasmic contents and impairment of adenosine triphosphate (ATP) activity (152). Furthermore, Lee et al. illustrated that the inactivation process of aPDT mediated by quercetin involved damage to E. coli O157:H7 and L. monocytogenes membranes through the generation of ROS. The predominant mechanism observed was type I, with O2•- and H2O2 identified as the main ROS involved (52). The fungal cell wall consists of a cell membrane containing various membrane proteins. At the outermost layer, mannoproteins form a protective fibrous layer that conceals the underlying β-glucan layer, while chitin is situated in close proximity to the cell membrane (156, 157). In their investigation, Jan et al. discovered that HB-mediated aPDT resulted in significant impairment to the cell wall, cell membrane, cytoplasm, and nucleus of C. albicans, suggesting that ROS might be accountable for the damage observed in the cytoplasm and cell wall components, signifying a distinct mechanisms from that of antifungal drugs (59) (Figure 2).
3.3 Nucleic acids, proteins and lipids
To date, there have been relatively few studies investigating the direct influence of polyphenolic natural product PSs on bacterial nucleic acids, proteins, and especially lipids in aPDT. Previous research suggested that the DNA of microorganisms was primarily affected when they were either inactivated or nonviable, rendering the probability of developing resistance mechanisms against aPDT extremely low (155, 158). In a study by Lee et al., quercetin was identified as an exogenous PS located outside bacterial cells that generates ROS. This process initiated the attack on bacterial cells from the outermost structures. Subsequently, quercetin diffused into the damaged bacteria, and the ROS generated upon its entry resulted in the degradation of bacterial DNA (52). Furthermore, quercetin exhibited the ability to reduce bacterial protein synthesis, thereby affecting protein expression within the cell. Ultimately, this disruption led to cell lysis and death (152). Despite the lipid-rich composition of the bacterial cytoplasm and outer membranes, our understanding of the lipid-related mechanisms underlying natural product-mediated aPDT remains limited. The complexity associated with identifying and characterizing lipid damage has contributed to this gap (155).
4 In vivo aPDT with polyphenols
Currently, research on polyphenol-based natural product-mediated aPDT is primarily focused on oral and skin diseases in both in vivo (Table 2). In a study conducted by Dascalu Rusu LM and colleagues, utilizing curcuma extract, arnica oil, and oregano essential oil, novel natural PSs mediated aPDT effectively improved induced periodontal disease in rats and reduced inflammation (12). Paolillo FR et al. discovered that a combination of curcumin (0.06 mL of 1.5% curcumin gel) and blue light (450 nm, 80 mW/cm2, at the dose of 60 J/cm2)-mediated aPDT, with artificial skin, accelerated bacterial inactivation (S. aureus 4.14 log10) and enhanced wound healing in Wistar rats without inducing adverse effects on the tissue (159). Muniz IPR et al. demonstrated that ex vivo activation of curcumin (100 μg) by blue LED light (450 nm) at a fluence of 13.5 J/cm2 effectively controlled S. aureus cutaneous infection in type I diabetic mice (36, 160). Alam et al. achieved significant eradication of Ampicillin-Resistant P. aeruginosa in the Caenorhabditis elegans (C. elegans) model by using HYP in conjunction with ampicillin and subsequent orange light treatment (48). Liu et al. assessed the antibacterial capabilities of Poly (lactic acid)-Hypocrellin A (PLA-HA) nanofiber membranes through in vivo photodynamic therapy in rats infected with C. albicans. The study revealed that PLA-HA-mediated aPDT significantly promoted wound healing, reduced the infected wound area, and increased the wound healing rate by approximately 10% compared to other groups (56). Guo et al. discovered that lipase-sensitive methoxy poly (ethylene glycol)-block-poly(ϵ-caprolactone) (mPEG-PCL)/HA micelles mediated aPDT (470 nm, 90 mW/cm2, 60 min) effectively eradicated MRSA in the abdominal cavity of mice, increasing the survival rate to 86% at a low concentration of 10 mg/kg (HA concentration) (58). Hashimoto et al. treated burn mice infected with P. aeruginosa with HB: La+3 and aPDT (LED, 24 J/cm2). They found that aPDT reduced bacterial burden at the burn wound, delayed bacteremia, and lowered bacterial levels in the blood by 2-3 logarithmic units. Survival rates of mice increased 24 hours after treatment (60). Dos Santos et al. observed that blue LED light (54 J/cm2) enhanced the antimicrobial effect of resveratrol (2 mg/mL, 100 µL) against MRSA. In a mouse abscess model, it induced the production of TNF-α and IL-17A cytokines, reduced bacterial burden, and consequently decreased inflammation 24 hours after infection (61). Ma et al. demonstrated that AE-mediated aPDT effectively treated tinea corporis caused by T. rubrum in a guinea pig model and tinea unguium in an ex vivo model (67). In vivo studies reported by Wang et al. showed that AE-mediated aPDT effectively treated skin infections caused by multidrug-resistant A. baumannii in mice following burn injuries (127). Alam et al. evaluated the efficacy of ethanol extract of Tripterygium wilfordii (TWE)-mediated aPDT against various pathogens (E. coli, S. aureus, MRSA, S. pyogenes, and C. albicans) in a nematode model. Their findings indicated that it effectively controlled the pathogens without inducing strong adverse effects. TWE-mediated aPDT reversed the growth inhibition caused by pathogen infection in the nematodes, reduced the viable pathogen count associated with C. elegans, and improved the survival rate of the nematodes infected with Pyogenic Streptococcus, in conjunction with aPDT (69). Du et al. uniformly applied riboflavin G4 hydrogel (2 mL) onto sterile dressings and treated wounds infected with MRSA in rats by irradiating them with blue light at a wavelength of 460 nm and a light power density of 80 mW/cm2 for 10 min. Their results revealed that the hydrogel exhibited robust antimicrobial activity in the rat infection wounds after irradiation (71).
5 Conclusions and perspectives
In recent years, aPDT has emerged as a pioneering modality specifically formulated for the inactivation of an extensive array of microorganisms, including bacteria, fungi, and viruses. Its application has grown progressively in diverse fields, notably in dermatology for conditions such as acne, in oral health for issues such as tooth decay and halitosis, and in managing fungal infections and viral diseases, notably COVID-19. Additionally, aPDT’s effectiveness in eliminating pathogens has paved its way into the food industry, bolstering food safety measures. PSs, a crucial component of aPDT, are responsible for generating ROS. Natural polyphenolic compounds derived from plants, fruits, vegetables, and other natural sources are increasingly used as PSs in aPDT due to their lower toxicity, structural diversity, and excellent biocompatibility. However, their clinical application is limited by factors such as water solubility. To overcome these limitations, innovative techniques such as nanotechnology have been employed. Nanoparticles, in particular, have proven to be efficacious drug delivery systems for hydrophobic PSs, facilitating their effective transport both in vitro and in vivo. They enable circumvention of physiological and biological barriers, thereby enhancing bacterial cell uptake. Despite these advancements, further research and technological innovation are imperative to fully exploit the potential of natural polyphenolic PSs and enhance their efficacy in treating a plethora of infectious diseases. Overcoming their limitations and achieving enhanced efficacy in the treatment of various infectious diseases will require continuous exploration and innovation.
Overall, natural polyphenolic PSs-mediated aPDT, in combination with nanoparticle-based drug delivery systems, holds great potential in combating microbial infections and advancing the field of infectious disease treatment. With concerted efforts and ongoing research, it is expected that aPDT will continue to evolve and find wider applications in the future.
Author contributions
GH: Funding acquisition, Investigation, Writing – review & editing. XYW: Conceptualization, Visualization, Writing – original draft. RF: Writing – review & editing, Investigation. LW: Writing – original draft, Funding acquisition, Investigation. LZ: Writing – original draft, Investigation, Visualization. XJ: Funding acquisition, Writing – review & editing. XW: Conceptualization, Funding acquisition, Investigation, Writing – review & editing.
Funding
The author(s) declare financial support was received for the research, authorship, and/or publication of this article. This study was supported by grants from the National Natural Science Foundation of China (22177084, 82273559, 82103757 and 82073473), the China Postdoctoral Science Foundation (2022M722283), PostDoctor Research Project, West China Hospital, Sichuan University (2023HXBH076), Sichuan Natural Science Foundation Project (2023NSFSC1554), the Science and Technology Department of Sichuan Province (2022YFQ0054), Sichuan Provincial Administration of Traditional Chinese Medicine (2023MS324) and the 1.3.5 Project for Disciplines of Excellence, West China Hospital, Sichuan University (ZYJC21036).
Conflict of interest
The authors declare that the research was conducted in the absence of any commercial or financial relationships that could be construed as a potential conflict of interest.
Publisher’s note
All claims expressed in this article are solely those of the authors and do not necessarily represent those of their affiliated organizations, or those of the publisher, the editors and the reviewers. Any product that may be evaluated in this article, or claim that may be made by its manufacturer, is not guaranteed or endorsed by the publisher.
References
1. Berman D, Chandy SJ, Cansdell O, Moodley K, Veeraraghavan B, Essack SY. Global access to existing and future antimicrobials and diagnostics: antimicrobial subscription and pooled procurement. Lancet Glob Health (2022) 10:e293–7. doi: 10.1016/s2214-109x(21)00463-0
2. Urban-Chmiel R, Marek A, Stępień-Pyśniak D, Wieczorek K, Dec M, Nowaczek A, et al. Antibiotic resistance in bacteria—A review. Antibiotics (2022) 11:1079. doi: 10.3390/antibiotics11081079
3. Collaborators AR. Global burden of bacterial antimicrobial resistance in 2019: a systematic analysis. Lancet (London England) (2022) 399:629–55. doi: 10.1016/S0140-6736(21)02724-0
4. Ramana K, Kandil S, Bharatkumar PV, Sharada C, Rao R, Mani R, et al. Invasive fungal infections: a comprehensive review. Am J Infect Dis (2013) 1:64–9. doi: 10.12691/ajidm-1-4-2
5. Firacative C. Invasive fungal disease in humans: are we aware of the real impact? Memorias Do Instituto Oswaldo Cruz (2020) 115:e200430. doi: 10.1590/0074-02760200430
6. Li M, Zhao JP. Research progress on deep fungal drug resistance mechanisms and detection methods. Chin J Mycology (2023) 18(01):90–6. doi: 10.3969/j.issn.1673-3827.2023.01.018
7. Larsen J, Raisen CL, Ba X, Sadgrove NJ, Padilla-González GF, Simmonds MSJ, et al. Emergence of methicillin resistance predates the clinical use of antibiotics. Nature (2022) 602:135–41. doi: 10.1038/s41586-021-04265-w
8. Eleraky NE, Allam A, Hassan SB, Omar MM. Nanomedicine fight against antibacterial resistance: an overview of the recent pharmaceutical innovations. Pharmaceutics (2020) 12(2):142. doi: 10.3390/pharmaceutics12020142
9. Li J, Meng Z, Zhuang Z, Wang B, Dai J, Feng G, et al. Effective therapy of drug-resistant bacterial infection by killing planktonic bacteria and destructing biofilms with cationic photosensitizer based on Phosphindole oxide. Small (Weinheim an Der Bergstrasse Germany) (2022) 18:e2200743. doi: 10.1002/smll.202200743
10. Jia M, Mai B, Liu S, Li Z, Liu Q, Wang P. Antibacterial effect of S-Porphin sodium photodynamic therapy on Staphylococcus aureus and multiple drug resistance Staphylococcus aureus. Photodiagnosis Photodyn Ther (2019) 28:80–7. doi: 10.1016/j.pdpdt.2019.08.031
11. Gao Y, Mai B, Wang A, Li M, Wang X, Zhang K, et al. Antimicrobial properties of a new type of photosensitizer derived from phthalocyanine against planktonic and biofilm forms of Staphylococcus aureus. Photodiagnosis Photodyn Ther (2018) 21:316–26. doi: 10.1016/j.pdpdt.2018.01.003
12. Dascalu Rusu LM, Moldovan M, Sarosi C, Sava S, Dreanca A, Repciuc C, et al. Photodynamic therapy with natural photosensitizers in the management of periodontal disease induced in rats. Gels (Basel Switzerland) (2022) 8(2):134. doi: 10.3390/gels8020134
13. Dong L, Qin J, Tai L, Mou K, Liao X, Chen F, et al. Inactivation of Bacillus subtilis by curcumin-mediated photodynamic technology through inducing oxidative stress response. Microorganisms (2022) 10(4):802. doi: 10.3390/microorganisms10040802
14. Almeida A, Faustino MAF, Neves MGPMS. Antimicrobial photodynamic therapy in the control of COVID-19. Antibiotics (Basel Switzerland) (2020) 9(6):320. doi: 10.3390/antibiotics9060320
15. de Melo WC, Avci P, de Oliveira MN, Gupta A, Vecchio D, Sadasivam M, et al. Photodynamic inactivation of biofilm: taking a lightly colored approach to stubborn infection. Expert Rev Anti Infect Ther (2013) 11:669–93. doi: 10.1586/14787210.2013.811861
16. Afrasiabi S, Partoazar A, Chiniforush N, Goudarzi R. The potential application of natural photosensitizers used in antimicrobial photodynamic therapy against oral infections. Pharm (Basel Switzerland) (2022) 15(6):767. doi: 10.3390/ph15060767
17. Silva RFM, Pogačnik L. Polyphenols from food and natural products: neuroprotection and safety. Antioxidants (Basel Switzerland) (2020) 9(1):61. doi: 10.3390/antiox9010061
18. Rajagopal C, Lankadasari MB, Aranjani JM, Harikumar KB. Targeting oncogenic transcription factors by polyphenols: A novel approach for cancer therapy. Pharmacol Res (2018) 130:273–91. doi: 10.1016/j.phrs.2017.12.034
19. de Lima Cherubim DJ, Buzanello Martins CV, Oliveira Fariña L, da Silva de Lucca RA. Polyphenols as natural antioxidants in cosmetics applications. J Cosmetic Dermatol (2020) 19:33–7. doi: 10.1111/jocd.13093
20. Montenegro-Landívar MF, Tapia-Quirós P, Vecino X, Reig M, Valderrama C, Granados M, et al. Polyphenols and their potential role to fight viral diseases: An overview. Sci Total Environ (2021) 801:149719. doi: 10.1016/j.scitotenv.2021.149719
21. NJd M, Tovar JSD, LN D, LD D, VS B, Inada NM. Natural versus synthetic curcuminoids as photosensitizers: Photobleaching and antimicrobial photodynamic therapy evaluation. Photodiagnosis Photodyn Ther (2023) 42:103495. doi: 10.1016/j.pdpdt.2023.103495
22. Jayusman PA, Nasruddin NS, Mahamad Apandi NI, Ibrahim N, Budin SB. Therapeutic potential of polyphenol and nanoparticles mediated delivery in periodontal inflammation: A review of current trends and future perspectives. Front In Pharmacol (2022) 13:847702. doi: 10.3389/fphar.2022.847702
23. Yang L, Wang Z. Natural products, alone or in combination with FDA-approved drugs, to treat COVID-19 and lung cancer. Biomedicines (2021) 9(6):689. doi: 10.3390/biomedicines9060689
24. Ribeiro IP, Pinto JG, Souza BMN, Miñán AG, Ferreira-Strixino J. Antimicrobial photodynamic therapy with curcumin on methicillin-resistant Staphylococcus aureus biofilm. Photodiagnosis Photodyn Ther (2022) 37:102729. doi: 10.1016/j.pdpdt.2022.102729
25. Esatbeyoglu T, Huebbe P, Ernst IMA, Chin D, Wagner AE, Rimbach G. Curcumin–from molecule to biological function. Angewandte Chemie (International Ed In English) (2012) 51:5308–32. doi: 10.1002/anie.201107724
26. Su R, Yan H, Jiang X, Zhang Y, Li P, Su W. Orange-red to NIR emissive carbon dots for antimicrobial, bioimaging and bacteria diagnosis. J Materials Chem B (2022) 10:1250–64. doi: 10.1039/d1tb02457d
27. Paschoal MA, Tonon CC, Spolidório DMP, Bagnato VS, Giusti JSM, Santos-Pinto L. Photodynamic potential of curcumin and blue LED against Streptococcus mutans in a planktonic culture. Photodiagnosis Photodyn Ther (2013) 10:313–9. doi: 10.1016/j.pdpdt.2013.02.002
28. Hussain Y, Alam W, Ullah H, Dacrema M, Daglia M, Khan H, et al. Antimicrobial potential of curcumin: therapeutic potential and challenges to clinical applications. Antibiotics (Basel Switzerland) (2022) 11(3):322. doi: 10.3390/antibiotics11030322
29. Minhaco VMTR, Maquera Huacho PM, Mancim Imbriani MJ, Tonon CC, Chorilli M, ANdS R, et al. Improving antimicrobial activity against endodontic biofilm after exposure to blue light-activated novel curcumin nanoparticle. Photodiagnosis Photodyn Ther (2023) 42:103322. doi: 10.1016/j.pdpdt.2023.103322
30. Zhao P, Qiu J, Pan C, Tang Y, Chen M, Song H, et al. Potential roles and molecular mechanisms of bioactive ingredients in Curcumae Rhizoma against breast cancer. Phytomedicine Int J Phytotherapy Phytopharmacology (2023) 114:154810. doi: 10.1016/j.phymed.2023.154810
31. Mirzaei H, Bagheri H, Ghasemi F, Khoi JM, Pourhanifeh MH, Heyden YV, et al. Anti-cancer activity of curcumin on multiple myeloma. Anti-cancer Agents In Medicinal Chem (2021) 21:575–86. doi: 10.2174/1871520620666200918113625
32. Xu X, Zhang X, Zhang Y, Wang Z. Curcumin suppresses the Malignancy of non-small cell lung cancer by modulating the circ-PRKCA/miR-384/ITGB1 pathway. Biomedicine Pharmacotherapy = Biomedecine Pharmacotherapie (2021) 138:111439. doi: 10.1016/j.biopha.2021.111439
33. Fusar-Poli L, Vozza L, Gabbiadini A, Vanella A, Concas I, Tinacci S, et al. Curcumin for depression: a meta-analysis. Crit Rev In Food Sci Nutr (2020) 60:2643–53. doi: 10.1080/10408398.2019.1653260
34. Wang ZY, Jia YT, Li WY, Zhang M. Antimicrobial photodynamic inactivation with curcumin against Staphylococcus saprophyticus, in vitro and on fresh dough sheet. Lwt-Food Sci Technol (2021) 147:111567. doi: 10.1016/j.lwt.2021.111567
35. Abdulrahman H, Misba L, Ahmad S, Khan AU. Curcumin induced photodynamic therapy mediated suppression of quorum sensing pathway of Pseudomonas aeruginosa: An approach to inhibit biofilm. vitro. Photodiagnosis Photodyn Ther (2020) 30:101645. doi: 10.1016/j.pdpdt.2019.101645
36. Muniz IPR, Galantini MPL, Ribeiro IS, Gonçalves CV, Dos Santos DP, Moura TC, et al. Antimicrobial photodynamic therapy (aPDT) with curcumin controls intradermal infection by Staphylococcus aureus in mice with type 1 diabetes mellitus: a pilot study. J Photochem Photobiology. B Biol (2021) 224:112325. doi: 10.1016/j.jphotobiol.2021.112325
37. Cusicanqui Méndez DA, Gutierres E, José Dionisio E, Afonso Rabelo Buzalaf M, Cardoso Oliveira R, Andrade Moreira MaChado MA, et al. Curcumin-mediated antimicrobial photodynamic therapy reduces the viability and vitality of infected dentin caries microcosms. Photodiagnosis Photodyn Ther (2018) 24:102–8. doi: 10.1016/j.pdpdt.2018.09.007
38. de Oliveira EF, Tosati JV, Tikekar RV, Monteiro AR, Nitin N. Antimicrobial activity of curcumin in combination with light against Escherichia coli O157:H7 and Listeria innocua: Applications for fresh produce sanitation. Postharvest Biol Technol (2018) 137:86–94. doi: 10.1016/j.postharvbio.2017.11.014
39. Yang M-Y, Chang K-C, Chen L-Y, Hu A. Low-dose blue light irradiation enhances the antimicrobial activities of curcumin against Propionibacterium acnes. J Photochem Photobiology. B Biol (2018) 189:21–8. doi: 10.1016/j.jphotobiol.2018.09.021
40. Lee H-J, Kang S-M, Jeong S-H, Chung K-H, Kim B-I. Antibacterial photodynamic therapy with curcumin and Curcuma xanthorrhiza extract against Streptococcus mutans. Photodiagnosis Photodyn Ther (2017) 20:116–9. doi: 10.1016/j.pdpdt.2017.09.003
41. Comeau P, Panariello B, Duarte S, Manso A. Impact of curcumin loading on the physicochemical, mechanical and antimicrobial properties of a methacrylate-based experimental dental resin. Sci Rep (2022) 12:18691. doi: 10.1038/s41598-022-21363-5
42. Saitawee D, Teerakapong A, Morales NP, Jitprasertwong P, Hormdee D. Photodynamic therapy of Curcuma longa extract stimulated with blue light against Aggregatibacter actinomycetemcomitans. Photodiagnosis Photodyn Ther (2018) 22:101–5. doi: 10.1016/j.pdpdt.2018.03.001
43. Marques Meccatti V, de Souza Moura L, Guerra Pinto J, Ferreira-Strixino J, Abu Hasna A, Alves Figueiredo-Godoi LM, et al. Curcuma longa L. Extract and Photodynamic Therapy are Effective against Candida spp. and Do Not Show Toxicity In Vivo. Int J Dentistry (2022) 2022:5837864. doi: 10.1155/2022/5837864
44. Bastos-Filho TF, de Oliveira Caldeira EM, Frizera-Neto A. (2022). XXVII brazilian congress on biomedical engineering. Proceedings of CBEB 2020; October 26–30, 2020; Vitória, Brazil: Springer Nature.
45. Aponiene K, Paskeviciute E, Reklaitis I, Luksiene Z. Reduction of microbial contamination of fruits and vegetables by hypericin-based photosensitization: Comparison with other emerging antimicrobial treatments. J Food Eng (2015) 144:29–35. doi: 10.1016/j.jfoodeng.2014.07.012
46. Kashef N, Borghei YS, Djavid GE. Photodynamic effect of hypericin on the microorganisms and primary human fibroblasts. Photodiagnosis Photodyn Ther (2013) 10:150–5. doi: 10.1016/j.pdpdt.2012.11.007
47. Paz-Cristobal MP, Royo D, Rezusta A, Andrés-Ciriano E, Alejandre MC, Meis JF, et al. Photodynamic fungicidal efficacy of hypericin and dimethyl methylene blue against azole-resistant Candida albicans strains. Mycoses (2014) 57:35–42. doi: 10.1111/myc.12099
48. Alam ST, Le TAN, Park J-S, Kwon HC, Kang K. Antimicrobial biophotonic treatment of ampicillin-resistant pseudomonas aeruginosa with hypericin and ampicillin cotreatment followed by orange light. Pharmaceutics (2019) 11(12):641. doi: 10.3390/pharmaceutics11120641
49. Kashef N, Karami S, Djavid GE. Phototoxic effect of hypericin alone and in combination with acetylcysteine on Staphylococcus aureus biofilms. Photodiagnosis Photodyn Ther (2015) 12:186–92. doi: 10.1016/j.pdpdt.2015.04.001
50. Condat M, Babinot J, Tomane S, Malval JP, Kang IK, Spillebout F, et al. Development of photoactivable glycerol-based coatings containing quercetin for antibacterial applications. Rsc Adv (2016) 6:18235–45. doi: 10.1039/c5ra25267a
51. Pourhajibagher M, Alaeddini M, Etemad-Moghadam S, Rahimi Esboei B, Bahrami R, Miri Mousavi RS, et al. Quorum quenching of Streptococcus mutans via the nano-quercetin-based antimicrobial photodynamic therapy as a potential target for cariogenic biofilm. BMC Microbiol (2022) 22:125. doi: 10.1186/s12866-022-02544-8
52. Lee I-H, Kim S-H, Kang D-H. Quercetin mediated antimicrobial photodynamic treatment using blue light on Escherichia coli O157:H7 and Listeria monocytogenes. Curr Res In Food Sci (2023) 6:100428. doi: 10.1016/j.crfs.2022.100428
53. Pourhajibagher M, Bazarjani F, Bahador A. In silico and in vitro insights into the prediction and analysis of natural photosensitive compounds targeting Acinetobacter baumannii biofilm-associated protein. Photodiagnosis Photodyn Ther (2022) 40:103134. doi: 10.1016/j.pdpdt.2022.103134
54. Zhou JH, Xia SQ, Chen JR, Wang XS, Zhang BW. The photodynamic property improvement of hypocrellin A by chelation with lanthanum ions. Chem Commun (2003) (12):1372–3. doi: 10.1039/b302125d
55. Yang Y, Wang C, Zhuge Y, Zhang J, Xu K, Zhang Q, et al. Photodynamic antifungal activity of hypocrellin A against Candida albicans. Front Microbiol (2019) 10:1810. doi: 10.3389/fmicb.2019.01810
56. Liu XY, Guo CA, Zhuang KW, Chen W, Zhang MQ, Dai YL, et al. A recyclable and light-triggered nanofibrous membrane against the emerging fungal pathogen Candida auris. PloS Pathog (2022) 18(5):e1010534. doi: 10.1371/journal.ppat.1010534
57. Liu XY, Fang RJ, Feng R, Li QS, Su MQ, Hou CL, et al. Cage-modified hypocrellin against multidrug-resistant Candida spp. with unprecedented activity in light-triggered combinational photodynamic therapy. Drug Resistance Updates (2022) 65:100887. doi: 10.1016/j.drup.2022.100887
58. Guo LY, Yan SZ, Tao X, Yang Q, Li Q, Wang TS, et al. Evaluation of hypocrellin A-loaded lipase sensitive polymer micelles for intervening methicillin-resistant Staphylococcus Aureus antibiotic-resistant bacterial infection. Materials Sci Eng C-Materials Biol Appl (2020) 106:110230. doi: 10.1016/j.msec.2019.110230
59. Jan A, Liu CC, Deng H, Li J, Ma WP, Zeng XY, et al. In vitro photodynamic inactivation effects of hypocrellin B on azole-sensitive and resistant Candida albicans. Photodiagnosis Photodyn Ther (2019) 27:419–27. doi: 10.1016/j.pdpdt.2019.07.014
60. Hashimoto MC, Prates RA, Kato IT, Núñez SC, Courrol LC, Ribeiro MS. Antimicrobial photodynamic therapy on drug-resistant Pseudomonas aeruginosa-induced infection. Vivo study. Photochem Photobiol (2012) 88:590–5. doi: 10.1111/j.1751-1097.2012.01137.x
61. Dos Santos DP, Soares Lopes DP, de Moraes RC, Vieira Gonçalves C, Pereira Rosa L, da Silva Rosa FC, et al. Photoactivated resveratrol against Staphylococcus aureus infection in mice. Photodiagnosis Photodyn Ther (2019) 25:227–36. doi: 10.1016/j.pdpdt.2019.01.005
62. Cossu A, Ercan D, Wang QY, Peer WA, Nitin N, Tikekar RV. Antimicrobial effect of synergistic interaction between UV-A light and gallic acid against Escherichia coli O157:H7 in fresh produce wash water and biofilm. Innovative Food Sci Emerging Technol (2016) 37:44–52. doi: 10.1016/j.ifset.2016.07.020
63. de Oliveira EF, Cossu A, Tikekar RV, Nitin N. Enhanced antimicrobial activity based on a synergistic combination of sublethal levels of stresses induced by UV-A light and organic acids. Appl Environ Microbiol (2017) 83(11):e000383-17. doi: 10.1128/AEM.00383-17
64. Nakamura K, Yamada Y, Ikai H, Kanno T, Sasaki K, Niwano Y. Bactericidal action of photoirradiated gallic acid via reactive oxygen species formation. J Agric Food Chem (2012) 60:10048–54. doi: 10.1021/jf303177p
65. Li J, Qin MT, Liu CC, Ma WP, Zeng XY, Ji YH. Antimicrobial photodynamic therapy against multidrug-resistant Acinetobacter baumannii clinical isolates mediated by aloe-emodin: An in vitro study. Photodiagnosis Photodyn Ther (2020) 29:101632. doi: 10.1016/j.pdpdt.2019.101632
66. Ma WP, Liu CC, Li J, Hao M, Ji YH, Zeng XY. The effects of aloe emodin-mediated antimicrobial photodynamic therapy on drug-sensitive and resistant Candida albicans. Photochemical Photobiological Sci (2020) 19:485–94. doi: 10.1039/c9pp00352e
67. Ma WP, Zhang MM, Cui ZX, Wang XP, Niu XW, Zhu YY, et al. Aloe-emodin-mediated antimicrobial photodynamic therapy against dermatophytosis caused by Trichophyton rubrum. Microbial Biotechnol (2022) 15:499–512. doi: 10.1111/1751-7915.13875
68. Cui ZX, Zhang MM, Geng SM, Niu XW, Wang XP, Zhu YY, et al. Antifungal effect of antimicrobial photodynamic therapy mediated by haematoporphyrin monomethyl ether and aloe emodin on malassezia furfur. Front Microbiol (2021) 12:749106. doi: 10.3389/fmicb.2021.749106
69. Alam ST, Hwang H, Son JD, Nguyen UTT, Park J-S, Kwon HC, et al. Natural photosensitizers from Tripterygium wilfordii and their antimicrobial photodynamic therapeutic effects in a Caenorhabditis elegans model. J Photochem Photobiology. B Biol (2021) 218:112184. doi: 10.1016/j.jphotobiol.2021.112184
70. Borodina TN, Tolordava ER, Nikolaeva ME, Solov’ev AI, Romanova YM, Khaydukov EV, et al. Antimicrobial photodynamic activity of hydrophilic riboflavin derivatives. Mol Genetics Microbiol Virol (2021) 36:176–80. doi: 10.3103/S0891416821040042
71. Du P, Shen Y, Zhang B, Li S, Gao M, Wang T, et al. A H2 O2 -supplied supramolecular material for post-irradiated infected wound treatment. Advanced Sci (Weinheim Baden-Wurttemberg Germany) (2023) 10:e2206851. doi: 10.1002/advs.202206851
72. Meng X, Guan J, Lai S, Fang L, Su J. pH-responsive curcumin-based nanoscale ZIF-8 combining chemophotodynamic therapy for excellent antibacterial activity. RSC Adv (2022) 12:10005–13. doi: 10.1039/d1ra09450e
73. Mushtaq S, Yasin T, Saleem M, Dai T, Yameen MA. Potentiation of antimicrobial photodynamic therapy by curcumin-loaded graphene quantum dots. Photochem Photobiol (2022) 98:202–10. doi: 10.1111/php.13503
74. Crugeira PJL, Almeida HHS, Teixeira LG, Barreiro MF. Photodynamic inactivation of Staphylococcus aureus by ecological antibacterial solutions associating LED (ʎ 450 ± 10 nm) with curcumin and olive leaf extracts. J Photochem Photobiology. B Biol (2023) 238:112626. doi: 10.1016/j.jphotobiol.2022.112626
75. Trigo-Gutierrez JK, Vega-Chacón Y, Soares AB, Mima E. Antimicrobial activity of curcumin in nanoformulations: A comprehensive review. Int J Mol Sci (2021) 22(13):7130. doi: 10.3390/ijms22137130
76. Plenagl N, Seitz BS, Reddy Pinnapireddy S, Jedelska J, Brussler J, Bakowsky U. Hypericin loaded liposomes for anti-microbial photodynamic therapy of gram-positive bacteria. Physica Status Solidi a-Applications Materials Sci (2018) 215(15):1700837. doi: 10.1002/pssa.201700837
77. Yow CMN, Tang HM, Chu ESM, Huang Z. Hypericin-mediated photodynamic antimicrobial effect on clinically isolated pathogens. Photochem Photobiol (2012) 88:626–32. doi: 10.1111/j.1751-1097.2012.01085.x
78. Galinari CB, TdP B, RS Gonçalves, GB C, EV B, Malacarne LC, et al. Photoactivity of hypericin: from natural product to antifungal application. Crit Rev In Microbiol (2023) 49:38–56. doi: 10.1080/1040841X.2022.2036100
79. Rezusta A, López-Chicón P, Paz-Cristobal MP, Alemany-Ribes M, Royo-Díez D, Agut M, et al. In vitro fungicidal photodynamic effect of hypericin on Candida species. Photochem Photobiol (2012) 88:613–9. doi: 10.1111/j.1751-1097.2011.01053.x
80. Kiesslich T, Krammer B, Plaetzer K. Cellular mechanisms and prospective applications of hypericin in photodynamic therapy. Curr Medicinal Chem (2006) 13:2189–204. doi: 10.2174/092986706777935267
81. Nafee N, Youssef A, El-Gowelli H, Asem H, Kandil S. Antibiotic-free nanotherapeutics: hypericin nanoparticles thereof for improved in vitro and in vivo antimicrobial photodynamic therapy and wound healing. Int J Pharmaceutics (2013) 454:249–58. doi: 10.1016/j.ijpharm.2013.06.067
82. Plenagl N, Seitz BS, Duse L, Pinnapireddy SR, Jedelska J, Brüßler J, et al. Hypericin inclusion complexes encapsulated in liposomes for antimicrobial photodynamic therapy. Int J Pharmaceutics (2019) 570:118666. doi: 10.1016/j.ijpharm.2019.118666
83. Malacrida AM, Dias VHC, Silva AF, Dos Santos AR, Cesar GB, Bona E, et al. Hypericin-mediated photoinactivation of polymeric nanoparticles against Staphylococcus aureus. Photodiagnosis Photodyn Ther (2020) 30:101737. doi: 10.1016/j.pdpdt.2020.101737
84. Li Y, Yao JY, Han CY, Yang JX, Chaudhry MT, Wang SN, et al. Quercetin, inflammation and immunity. Nutrients (2016) 8(3). doi: 10.3390/nu8030167
85. Catauro M, Papale F, Bollino F, Piccolella S, Marciano S, Nocera P, et al. Silica/quercetin sol-gel hybrids as antioxidant dental implant materials. Sci Technol Advanced Materials (2015) 16(3):035001. doi: 10.1088/1468-6996/16/3/035001
86. Zhenjun D, Lown JW. Hypocrellins and their use in photosensitization. Photochem Photobiol (1990) 52:609–16.
87. Wang T, Xu L, Shen H, Cao X, Wei Q, Ghiladi RA, et al. Photoinactivation of bacteria by hypocrellin-grafted bacterial cellulose. Cellulose (2020) 27(2):991–1007. doi: 10.1007/s10570-019-02852-9
88. Diwu Z. Novel therapeutic and diagnostic applications of hypocrellins and hypericins. Photochem Photobiol (1995) 61(6):529–39. doi: 10.1111/j.1751-1097.1995.tb09903.x
89. Hu YQ, Zhang CM, Li SL, Jiao Y, Qi TG, Wei G, et al. Effects of photodynamic therapy using yellow LED-light with concomitant hypocrellin B on apoptotic signaling in keloid fibroblasts. Int J Biol Sci (2017) 13:319–26. doi: 10.7150/ijbs.17920
90. Wang P, Xu CS, Xu J, Wang XN, Leungy AW. HYPOCRELLIN B ENHANCES ULTRASOUND-INDUCED CELL DEATH OF NASOPHARYNGEAL CARCINOMA CELLS. Ultrasound Med Biol (2010) 36:336–42. doi: 10.1016/j.ultrasmedbio.2009.09.007
91. Xu SJ, Zhang XX, Chen S, Zhang MH, Shen T. EPR studies of the photodynamic properties of a novel potential photodynamic therapeutic agent: photogeneration of semiquinone radical anion and active oxygen species (O-2(.-), OH., H2O2 and O-1(2)). Photochemical Photobiological Sci (2003) 2:871–6. doi: 10.1039/b303293k
92. Chio-Srichan S, Oudrhiri N, Bennaceur-Griscelli A, Turhan AG, Dumas P, Refregiers M. Toxicity and phototoxicity of Hypocrellin A on Malignant human cell lines, evidence of a synergistic action of photodynamic therapy with Imatinib mesylate. J Photochem Photobiology. B Biol (2010) 99:100–4. doi: 10.1016/j.jphotobiol.2010.03.001
93. Qi SS, Lin X, Zhang MM, Yan SZ, Yu SQ, Chen SL. Preparation and evaluation of hypocrellin A loaded poly(lactic-co-glycolic acid) nanoparticles for photodynamic therapy. Rsc Adv (2014) 4:40085–94. doi: 10.1039/c4ra05796a
94. Law S, Lo CM, Han J, Leung AW, Xu CS. Antimicrobial photodynamic therapy with hypocrellin B against SARS-CoV-2 infection? Photodiagnosis Photodyn Ther (2021) 34:102297. doi: 10.1016/j.pdpdt.2021.102297
95. Kamarehei F, Mehdiabadi M, Naderi F. Antibacterial effects of natural compounds on biofilm formation of Streptococcus mutans. Clin Exp Dental Res (2022) 8:1426–33. doi: 10.1002/cre2.673
96. Li J, Wu T, Peng W, Zhu Y. Effects of resveratrol on cariogenic virulence properties of Streptococcus mutans. BMC Microbiol (2020) 20:99. doi: 10.1186/s12866-020-01761-3
97. Pourhajibagher M, Bahador A. Molecular docking study of potential antimicrobial photodynamic therapy as a potent inhibitor of SARS-CoV-2 main protease: an in silico insight. Infect Disord Drug Targets (2023) 23:46–55. doi: 10.2174/1871526522666220901164329
98. Tsai H-Y, Ho C-T, Chen Y-K. Biological actions and molecular effects of resveratrol, pterostilbene, and 3'-hydroxypterostilbene. J Food Drug Anal (2017) 25:134–47. doi: 10.1016/j.jfda.2016.07.004
99. Kugaji MS, Kumbar VM, Peram MR, Patil S, Bhat KG, Diwan PV. Effect of Resveratrol on biofilm formation and virulence factor gene expression of Porphyromonas gingivalis in periodontal disease. APMIS Acta Pathologica Microbiologica Et Immunologica Scandinavica (2019) 127:187–95. doi: 10.1111/apm.12930
100. Paudel RC, Kiviluoto S, Parys JB, Bultynck G. Resveratrol is not compatible with a Fura-2-based assay for measuring intracellular Ca2 signaling. Biochem Biophys Res Commun (2014) 450:1626–30. doi: 10.1016/j.bbrc.2014.07.049
101. Klančnik A, Šikić Pogačar M, Trošt K, Tušek Žnidarič M, Mozetič Vodopivec B, Smole Možina S. Anti-Campylobacter activity of resveratrol and an extract from waste Pinot noir grape skins and seeds, and resistance of Camp. jejuni planktonic and biofilm cells, mediated via the CmeABC efflux pump. J Appl Microbiol (2017) 122:65–77. doi: 10.1111/jam.13315
102. Duracka M, Lukac N, Kacaniova M, Kantor A, Hleba L, Ondruska L, et al. Antibiotics versus natural biomolecules: the case of in vitro induced bacteriospermia by enterococcus faecalis in rabbit semen. Molecules (Basel Switzerland) (2019) 24(23):4329. doi: 10.3390/molecules24234329
103. Mao JY, Chen L, Cai ZW, Qian ST, Liu ZM, Zhao BF, et al. Advanced biomaterials for regulating polarization of macrophages in wound healing. Advanced Funct Materials (2022) 32(12):202111003. doi: 10.1002/adfm.202111003
104. Choubey S, Varughese LR, Kumar V, Beniwal V. Medicinal importance of gallic acid and its ester derivatives: a patent review. Pharm patent analyst (2015) 4:305–15. doi: 10.4155/ppa.15.14
105. Petrisor G, Ficai D, Motelica L, Trusca RD, Bîrcă AC, Vasile BS, et al. Mesoporous silica materials loaded with gallic acid with antimicrobial potential. Nanomaterials (Basel Switzerland) (2022) 12(10):1648. doi: 10.3390/nano12101648
106. Fernandes FHA, Salgado HRN. Gallic acid: review of the methods of determination and quantification. Crit Rev In Analytical Chem (2016) 46:257–65. doi: 10.1080/10408347.2015.1095064
107. Brewer M. Natural antioxidants: sources, compounds, mechanisms of action, and potential applications. Compr Rev Food Sci Food Saf (2011) 10:221–47. doi: 10.1111/j.1541-4337.2011.00156.x
108. Locatelli C, Filippin-Monteiro FB, Creczynski-Pasa TB. Alkyl esters of gallic acid as anticancer agents: a review. Eur J Medicinal Chem (2013) 60:233–9. doi: 10.1016/j.ejmech.2012.10.056
109. Saeed N, Khan MR, Shabbir M. Antioxidant activity, total phenolic and total flavonoid contents of whole plant extracts Torilis leptophylla L. BMC Complementary Altern Med (2012) 12:221. doi: 10.1186/1472-6882-12-221
110. Karimova NV, Luo M, Sit I, Grassian VH, Gerber RB. Absorption Spectra and the Electronic Structure of Gallic Acid in Water at Different pH: Experimental Data and Theoretical Cluster Models. J Phys Chem A (2022) 126:190–7. doi: 10.1021/acs.jpca.1c07333
111. Hostnik G, Tošović J, Štumpf S, Petek A, Bren U. The influence of pH on UV/Vis spectra of gallic and ellagic acid: A combined experimental and computational study. Spectrochimica Acta Part A Mol Biomolecular Spectrosc (2022) 267:120472. doi: 10.1016/j.saa.2021.120472
112. Shao D, Li J, Li J, Tang R, Liu L, Shi J, et al. Inhibition of Gallic Acid on the Growth and Biofilm Formation of Escherichia coli and Streptococcus mutans. J Food Sci (2015) 80:M1299–305. doi: 10.1111/1750-3841.12902
113. Kahkeshani N, Farzaei F, Fotouhi M, Alavi SS, Bahramsoltani R, Naseri R, et al. Pharmacological effects of gallic acid in health and diseases: A mechanistic review. Iranian J Basic Med Sci (2019) 22:225–37. doi: 10.22038/ijbms.2019.32806.7897
114. Kang M-S, Oh J-S, Kang I-C, Hong S-J, Choi C-H. Inhibitory effect of methyl gallate and gallic acid on oral bacteria. J Microbiol (Seoul Korea) (2008) 46:744–50. doi: 10.1007/s12275-008-0235-7
115. Dong XX, Zeng YW, Liu Y, You LT, Yin XB, Fu J, et al. Aloe-emodin: A review of its pharmacology, toxicity, and pharmacokinetics. Phytotherapy Res (2020) 34:270–81. doi: 10.1002/ptr.6532
116. Wu J, Liu D, Sun J. Photodynamic inactivation of staphylococcus Aureus with aloe-emodin and its potential application on fresh-cut apples. doi: 10.2139/ssrn.4332273
117. Zang LX, Zhao HM, Ji XY, Cao WW, Zhang ZG, Meng PS. Photophysical properties, singlet oxygen generation efficiency and cytotoxic effects of aloe emodin as a blue light photosensitizer for photodynamic therapy in dermatological treatment. Photochemical Photobiological Sci (2017) 16:1088–94. doi: 10.1039/c6pp00453a
118. Chen SH, Lin KY, Chang CC, Fang CL, Lin CP. Aloe-emodin-induced apoptosis in human gastric carcinoma cells. Food Chem Toxicol (2007) 45:2296–303. doi: 10.1016/j.fct.2007.06.005
119. Xiang H, Cao FJ, Ming D, Zheng YY, Dong XY, Zhong XB, et al. Aloe-emodin inhibits Staphylococcus aureus biofilms and extracellular protein production at the initial adhesion stage of biofilm development. Appl Microbiol Biotechnol (2017) 101:6671–81. doi: 10.1007/s00253-017-8403-5
120. Park MY, Kwon HJ, Sung MK. Evaluation of aloin and aloe-emodin as anti-inflammatory agents in aloe by using murine macrophages. Bioscience Biotechnol Biochem (2009) 73:828–32. doi: 10.1271/bbb.80714
121. Pecere T, Gazzola MV, Mucignat C, Parolin C, Dalla Vecchia F, Cavaggioni A, et al. Aloe-emodin is a new type of anticancer agent with selective activity against neuroectodermal tumors. Cancer Res (2000) 60(11):2800–4.
122. Lin KY, Uen YH. Aloe-emodin, an anthraquinone, in vitro inhibits proliferation and induces apoptosis in human colon carcinoma cells. Oncol Lett (2010) 1:541–7. doi: 10.3892/ol_00000096
123. Giuliani C, Atieri B, Bombelli C, Galantini L, Mancini G, Stringaro A. Remote loading of aloe emodin in gemini-based cationic liposomes. Langmuir (2015) 31:76–82. doi: 10.1021/la5038074
124. Sevcovicova A, Bodnarova K, Loderer D, Imreova P, Galova E, Miadokova E. Dual activities of emodin - DNA protectivity vs mutagenicity. Neuroendocrinol Lett (2014) 35:149–54.
125. Tu PH, Huang Q, Ou YS, Du X, Li KT, Tao Y, et al. Aloe-emodin-mediated photodynamic therapy induces autophagy and apoptosis in human osteosarcoma cell line MG-63 through the ROS/JNK signaling pathway. Oncol Rep (2016) 35:3209–15. doi: 10.3892/or.2016.4703
126. Li KT, Duan QQ, Chen Q, He JW, Tian S, Lin HD, et al. The effect of aloe emodin-encapsulated nanoliposome-mediated r-caspase-3 gene transfection and photodynamic therapy on human gastric cancer cells. Cancer Med (2016) 5:361–9. doi: 10.1002/cam4.584
127. Wang Y, Li J, Geng S, Wang X, Cui Z, Ma W, et al. Aloe-emodin-mediated antimicrobial photodynamic therapy against multidrug-resistant Acinetobacter baumannii: An in vivo study. Photodiagnosis Photodyn Ther (2021) 34:102311. doi: 10.1016/j.pdpdt.2021.102311
128. Li J, Wang X, Jiang H, Lu X, Zhu Y, Chen B. New strategy of photodynamic treatment of TiO2 nanofibers combined with celastrol for HepG2 proliferation in vitro. Nanoscale (2011) 3:3115–22. doi: 10.1039/c1nr10185d
129. Xu Y, Li W, Wen R, Sun J, Liu X, Zhao S, et al. Voltage-gated sodium channels, potential targets of Tripterygium wilfordii Hook. f. to exert activity and produce toxicity. J Ethnopharmacology (2023) 311:116448. doi: 10.1016/j.jep.2023.116448
130. Cascão R, Fonseca JE, Moita LF. Celastrol: A spectrum of treatment opportunities in chronic diseases. Front In Med (2017) 4:69. doi: 10.3389/fmed.2017.00069
131. Chen S-R, Dai Y, Zhao J, Lin L, Wang Y, Wang Y. A mechanistic overview of Triptolide and Celastrol, natural products from Tripterygium Wilfordii Hook F. Front In Pharmacol (2018) 9:104. doi: 10.3389/fphar.2018.00104
132. Law S. Could celastrol be a photosensitizer for photodynamic therapy to combat SARS-CoV-2? Pharm Biomed Res (2022) 8:163–6. doi: 10.18502/pbr.v8i3.11030
133. Caruso F, Singh M, Belli S, Berinato M, Rossi M. Interrelated mechanism by which the methide quinone celastrol, obtained from the roots of Tripterygium wilfordii, inhibits main protease 3CLpro of COVID-19 and acts as superoxide radical scavenger. Int J Mol Sci (2020) 21(23):9266. doi: 10.3390/ijms21239266
134. Farah N, Chin VK, Chong PP, Lim WF, Lim CW, Basir R, et al. Riboflavin as a promising antimicrobial agent? A multi-perspective review. Curr Res In Microbial Sci (2022) 3:100111. doi: 10.1016/j.crmicr.2022.100111
135. Saedisomeolia A, Ashoori M. Riboflavin in human health: A review of current evidences. Adv In Food Nutr Res (2018) 83:57–81. doi: 10.1016/bs.afnr.2017.11.002
136. Fawzy AS, Nitisusanta LI, Iqbal K, Daood U, Neo J. Riboflavin as a dentin crosslinking agent: ultraviolet A versus blue light. Dental Materials Off Publ Acad Dental Materials (2012) 28:1284–91. doi: 10.1016/j.dental.2012.09.009
137. Zhang C, Zhang Y, Fang Q, Li R, Yuan Y, Zhuang H. Nanoemulsions loaded with compound photosensitisers: synergistic photodynamic inactivation effects of curcumin and riboflavin tetra butyrate. Int J Food Sci Technol (2023) 58:1728–40. doi: 10.1111/ijfs.16258
138. do Prado-Silva L, Brancini GTP, Braga GUL, Liao XY, Ding T, Sant'Ana AS. Antimicrobial photodynamic treatment (aPDT) as an innovative technology to control spoilage and pathogenic microorganisms in agri-food products: An updated review. Food Control (2022) 132:108527. doi: 10.1016/j.foodcont.2021.108527
139. Gnanasekar S, Kasi G, He X, Zhang K, Xu L, Kang ET. Recent advances in engineered polymeric materials for efficient photodynamic inactivation of bacterial pathogens. Bioact Mater (2023) 21:157–74. doi: 10.1016/j.bioactmat.2022.08.011
140. Polat E, Kang K. Natural photosensitizers in antimicrobial photodynamic therapy. Biomedicines (2021) 9(6):584. doi: 10.3390/biomedicines9060584
141. Mackay AM. The evolution of clinical guidelines for antimicrobial photodynamic therapy of skin. Photochem Photobiol Sci (2022) 21:385–95. doi: 10.1007/s43630-021-00169-w
142. Hamblin MR, Abrahamse H. Oxygen-independent antimicrobial photoinactivation: type III photochemical mechanism? Antibiotics (Basel Switzerland) (2020) 9(2):53. doi: 10.3390/antibiotics9020053
143. Gholami L, Shahabi S, Jazaeri M, Hadilou M, Fekrazad R. Clinical applications of antimicrobial photodynamic therapy in dentistry. Front In Microbiol (2022) 13:1020995. doi: 10.3389/fmicb.2022.1020995
144. Chrubasik-Hausmann S, Hellwig E, Müller M, Al-Ahmad A. Antimicrobial photodynamic treatment with mother juices and their single compounds as photosensitizers. Nutrients (2021) 13(3):710. doi: 10.3390/nu13030710
145. Brindhadevi K, LewisOscar F, Mylonakis E, Shanmugam S, Verma TN, Pugazhendhi A. Biofilm and Quorum sensing mediated pathogenicity in Pseudomonas aeruginosa. Process Biochem (2020) 96:49–57. doi: 10.1016/j.procbio.2020.06.001
146. Rodrigues ME, Gomes F, Rodrigues CF. Candida spp./bacteria mixed biofilms. J Fungi (Basel) (2019) 6(1):5. doi: 10.3390/jof6010005
147. Flemming H-C, Wingender J. The biofilm matrix. Nat Rev Microbiol (2010) 8:623–33. doi: 10.1038/nrmicro2415
148. Flemming HC, van Hullebusch ED, Neu TR, Nielsen PH, Seviour T, Stoodley P, et al. The biofilm matrix: multitasking in a shared space. Nat Rev Microbiol (2023) 21:70–86. doi: 10.1038/s41579-022-00791-0
149. Hu X, Huang YY, Wang Y, Wang X, Hamblin MR. Antimicrobial photodynamic therapy to control clinically relevant biofilm infections. Front Microbiol (2018) 9:1299. doi: 10.3389/fmicb.2018.01299
150. Martins Antunes de Melo WC, Celiešiūtė-Germanienė R, Šimonis P, Stirkė A. Antimicrobial photodynamic therapy (aPDT) for biofilm treatments. Possible synergy between aPDT and pulsed electric fields. Virulence (2021) 12:2247–72. doi: 10.1080/21505594.2021.1960105
151. García I, Ballesta S, Gilaberte Y, Rezusta A, Pascual Á. Antimicrobial photodynamic activity of hypericin against methicillin-susceptible and resistant Staphylococcus aureus biofilms. Future Microbiol (2015) 10:347–56. doi: 10.2217/fmb.14.114
152. Wang S, Yao J, Zhou B, Yang J, Chaudry MT, Wang M, et al. Bacteriostatic effect of quercetin as an antibiotic alternative in vivo and its antibacterial mechanism in vitro. J Food Prot (2018) 81:68–78. doi: 10.4315/0362-028x.Jfp-17-214
153. Silhavy TJ, Kahne D, Walker S. The bacterial cell envelope. Cold Spring Harb Perspect Biol (2010) 2:a000414. doi: 10.1101/cshperspect.a000414
154. de Annunzio SR, de Freitas LM, Blanco AL, da Costa MM, Carmona-Vargas CC, de Oliveira KT, et al. Susceptibility of Enterococcus faecalis and Propionibacterium acnes to antimicrobial photodynamic therapy. J Photochem Photobiol B (2018) 178:545–50. doi: 10.1016/j.jphotobiol.2017.11.035
155. Almeida A, Faustino MA, Tomé JP. Photodynamic inactivation of bacteria: finding the effective targets. Future Med Chem (2015) 7:1221–4. doi: 10.4155/fmc.15.59
156. Garcia-Rubio R, de Oliveira HC, Rivera J, Trevijano-Contador N. The fungal cell wall: Candida, Cryptococcus, and Aspergillus species. Front Microbiol (2019) 10:2993. doi: 10.3389/fmicb.2019.02993
157. Vega K, Kalkum M. Chitin, chitinase responses, and invasive fungal infections. Int J Microbiol (2012) 2012:920459. doi: 10.1155/2012/920459
158. Alves E, Faustino MAF, Tomé JPC, Neves MGPMS, Tomé AC, Cavaleiro JAS, et al. Nucleic acid changes during photodynamic inactivation of bacteria by cationic porphyrins. Bioorganic Medicinal Chem (2013) 21:4311–8. doi: 10.1016/j.bmc.2013.04.065
159. Paolillo FR, Rodrigues PGS, Bagnato VS, Alves F, Pires L, Corazza AV. The effect of combined curcumin-mediated photodynamic therapy and artificial skin on Staphylococcus aureus-infected wounds in rats. Lasers In Med Sci (2021) 36:1219–26. doi: 10.1007/s10103-020-03160-6
Keywords: polyphenols, natural products, photodynamic therapy, photosensitizers, antibacterial
Citation: Wang X, Wang L, Fekrazad R, Zhang L, Jiang X, He G and Wen X (2023) Polyphenolic natural products as photosensitizers for antimicrobial photodynamic therapy: recent advances and future prospects. Front. Immunol. 14:1275859. doi: 10.3389/fimmu.2023.1275859
Received: 10 August 2023; Accepted: 17 October 2023;
Published: 31 October 2023.
Edited by:
Lei Zhang, University of Waterloo, CanadaReviewed by:
Ewerton Garcia de Oliveira Mima, São Paulo State University, BrazilTianhong Dai, Massachusetts General Hospital and Harvard Medical School, United States
Copyright © 2023 Wang, Wang, Fekrazad, Zhang, Jiang, He and Wen. This is an open-access article distributed under the terms of the Creative Commons Attribution License (CC BY). The use, distribution or reproduction in other forums is permitted, provided the original author(s) and the copyright owner(s) are credited and that the original publication in this journal is cited, in accordance with accepted academic practice. No use, distribution or reproduction is permitted which does not comply with these terms.
*Correspondence: Gu He, aGVndUBzY3UuZWR1LmNu; Xiang Wen, eGlhbmd3ZW5fd2N1bXNAMTYzLmNvbQ==; Lu Zhang, emhhbmdsdWh4QHNjdS5lZHUuY24=
†These authors have contributed equally to this work