- 1Department of Obstetrics & Gynecology, Laboratory Medicine and Pediatrics, West China Second University Hospital, Key Laboratory of Birth Defects and Related Diseases of Women and Children, Ministry of Education, Development and Related Diseases of Women and Children Key Laboratory of Sichuan Province, Center of Growth, Metabolism and Aging, State Key Laboratory of Biotherapy and Collaborative Innovation Center of Biotherapy, Frontiers Science Center for Disease-related Molecular Network, West China Hospital, Sichuan University, Chengdu, Sichuan, China
- 2Xinxiang Central Hospital, The Fourth Clinical College of Xinxiang Medical University, Xinxiang, Henan, China
- 3Institute of Respiratory Health, Frontiers Science Center for Disease-related Molecular Network, West China Hospital, Sichuan University, Chengdu, Sichuan, China
Immune checkpoint blockade (ICB) therapies, that is, using monoclonal antibodies to reinvigorate tumor-reactive, antigen-specific T cells from the inhibitory effects of CTLA-4, PD-1 and PD-L1 immune checkpoints, have revolutionized the therapeutic landscape of modern oncology. However, only a subset of patients can benefit from the ICB therapy. Biomarkers associated with ICB response, resistance and prognosis have been subjected to intensive research in the past decade. Early studies focused on the analysis of tumor specimens and their residing microenvironment. However, biopsies can be challenging to obtain in clinical practice, and do not reflect the dynamic changes of immunological parameters during the ICB therapy. Recent studies have investigated profiles of antigen-specific T cells derived from the peripheral compartment using multi-omics approaches. By tracking the clonotype and diversity of tumor-reactive T cell receptor repertoire, these studies collectively establish that de novo priming of antigen-specific T cells in peripheral blood occurs throughout the course of ICB, whereas preexisting T cells prior to ICB are exhausted to various degrees. Here, we review what is known about ICB-induced T cell phenotypic and functional changes in cancer patients both within the tumor microenvironment and in the peripheral compartment. A better understanding of parameters influencing the response to ICBs will provide rationales for developing novel diagnostics and combinatorial therapeutic strategies to maximize the clinical efficacies of ICB therapies.
1 Introduction
Cancer immunotherapies targeting immune checkpoint pathways have changed the therapeutical landscape of medical oncology. Immune checkpoint inhibitors, that is, antibodies blocking cytotoxic T-lymphocyte associated protein 4 (CTLA-4), programmed cell death 1 (PD-1) as well as its ligand PD-L1, have been approved by regulatory agencies around the world to treat multiple human cancer types (1). Beyond CTLA-4 and PD-1/L1, new therapeutical modalities targeting other immune checkpoints are also rapidly emerging. In the phase 3 study, Relatlimab (anti-LAG3, lymphocyte activation gene-3) in combination with Nivolumab (anti-PD-1) provided a greater progression-free survival (PFS) benefit than Nivolumab monotherapy in melanoma patients (2), leading to regulatory approval by USA FDA to treat unresectable or metastatic melanoma. T-cell immunoglobulin mucin-3 (TIM-3; encoded by HAVCR2) is a co-inhibitory receptor suppressing effector Th1 cell function (3). In several phase 1 and 2 studies, anti-TIM-3 antibodies, including TSR-022 (NCT02817633), Sabatolimab (NCT02608268), and LY3321367 (NCT03099109), were broadly safe and well tolerated (4–6). VISTA (V-domain Ig suppressor of T cell activation) is an immune checkpoint receptor expressed on tumor-infiltrating T lymphocytes (TILs) and myeloid cells, leading to the suppression of T cell activation, proliferation, and cytokine production (7). HMBD-002, an anti-VISTA monoclonal antibody, is currently under clinical evaluation in triple-negative breast cancer (TNBC) and non-small cell lung cancer (NSCLC) patients (NCT05082610). Icatolimab (anti-BTLA, B and T lymphocyte attenuator), is being tested in phase 1 trial to treat melanoma patients (NCT04137900). In parallel, reagents directly stimulating immune activators are being tested clinically. Vopratelimab, an agonist for ICOS (inducible co-stimulator), demonstrated a favorable safety profile in phase 2 study as monotherapy and in combination with Nivolumab in head and neck squamous cell carcinoma (HNSCC), NSCLC and TNBC patients (8). GWN323, an IgG1 monoclonal antibody (mAb) against GITR (glucocorticoid-induced TNFR-related protein) (NCT04021043), was well tolerated in patients with relapsed/refractory solid tumors (9, 10). CP-870893, an agonist for CD40 signaling pathway, exhibited encouraging anti-tumor activity patients with metastatic melanoma in phase 1 study (11). 4-1BB agonists (Urelumab and Utomilumab), and OX40 agonist (INCAGN01949) seem to display good safety and tolerability profiles (12–14). Some of these newly developed modulators of immune checkpoints and activators are likely to achieve clinical success in near future and empower the arsenal of cancer immunotherapies.
Biomarkers to predict the initial response to and outcome of immune checkpoint blockades (ICBs) are of high clinical relevance. PD-L1 expression was initially considered as a reasonable biomarker to predict the response to anti-PD-1/PD-L1 therapies. Clinical studies have shown that patients with higher intratumor PD-L1 levels are more likely to benefit from ICBs (15, 16). These studies lead to current clinical practice using the PD-L1 expression level as a diagnostic criterion to guide patient selection. However, this stratification method is suboptimal. Patients with PD-L1-negative tumors can sometimes respond to ICBs (16, 17), and conversely ovarian cancer patients with high PD-L1 expression fail to do so (18). In addition, PD-L1 expression levels show temporal and spatial variation during tumorigenesis and cancer treatment (19–22). These complications have prompted enormous interest in the research community to identify additional markers beyond the expression of PD-L1.
Early studies have focused on using tumor specimens to identify predictive markers, such as tumor mutation burden and infiltrating lymphocytes (23–27). However, there are several caveats and limitations when using biopsy to interpret the dynamic interactions between tumor and immune cells as well as to guide the ICB therapies. First, patients with metastatic diseases are usually not suitable for biopsy or surgical operation (28, 29). Secondly, it is likely that in many cases biopsies only reflect a portion of tumor mass due to sampling errors and tumor heterogeneity (30–32). Of note, current guidelines to select suitable patients for anti-PD-1 and anti-PD-L1 therapies are based on the percentage of PD-L1+ cells (33–36). Pathological assessments of tissues obtained from biopsy or surgery may be biased due to variations on the sampling technique and the heterogenous nature of advanced diseases (30–32, 37, 38). Biopsy techniques are known to affect the accuracy of assessment of PD-L1+ cells, thus affecting patient stratification (39–42). Constrained sampling space also limits the clinical extrapolation and generalizability of results derived from biopsies (43, 44). Thirdly, in most clinical scenarios, it is impossible to obtain consecutive biopsies during the course of ICB therapies. After initial treatment, longitudinal monitoring is often required for early detection of relapsed diseases in any antitumor regimens (45, 46). It will be difficult to assess PD-L1 expression because relapsed tumor specimens are usually inaccessible. Thus, the dynamic changes in immunological parameters during ICB therapy cannot be monitored to guide treatment options.
Recently, immunologic profiling and immunophenotyping of patient peripheral blood have attracted much attention as a means to comprehensively evaluate antitumor immunity. Cancer is a systemic disease in a sense that it constantly exchanges information with the immune system. Of note, several studies have demonstrated that the efficacy of ICB therapies mainly rely on the de novo priming of cytotoxic T cells from periphery, rather than the reinvigoration of pre-existing tumor infiltrating lymphocytes within tumor microenvironment (47, 48). Other studies have concluded that both peripheral and local expansion of antigen-specific T cells are critical (49–53). The differences between these studies may reflect different tumor types and disease stages. Nevertheless, they all highlight the importance of peripheral T cell response for ICB therapies to control tumor progression.
In this review, we summarize the current knowledge of ICB-induced T cell dynamics in the tumor microenvironment and the peripheral compartment. A better understanding of parameters influencing the response to ICBs will likely facilitate treatment decisions and improve cancer management.
2 Immune checkpoint blockades
Antitumor immunity refers to the innate and adaptive immune responses to halt tumor initiation and progression. Successful antitumor immunity largely relies on the activation of cytotoxic T cell responses (Figure 1). To avoid overactivation of T cells, the immune system utilizes negative regulators, or immune checkpoints, such as PD-1, PD-L1 and CTLA-4 to dampen the cytotoxic effects of T cells. ICB therapies are based on therapeutic antibodies capable of blocking the inhibitory activities of CTLA-4, PD-1 and PD-L1, with the intention to prolong the antitumor effect of T cells (54–56). CTLA-4 is thought to regulate immune responses mainly at the early stage of T-cell activation by interrupting costimulatory effect of CD28 (57–60). Ipilimumab and Tremelimumab, therapeutic antibodies blocking CTLA-4, have shown durable control of tumor growth in some patients. However, overall survival (OS) rate is not significantly improved, and many patients do not respond to Ipilimumab and Tremelimumab such as in ovarian cancer (61) and melanoma (62, 63). These observations indicate that early stage of T cell response may be intact in most cancer patients. On the other hand, the interaction between PD-1 (mainly expressed on T cells) and PD-L1 (mainly expressed on tumor cells) was originally thought to inhibit effector T-cell activity within the tumor microenvironment (64–67). Several therapeutic antibodies targeting either PD-1 (Pembrolizumab, Nivolumab, Cemiplimab and Dostarlimab) or PD-L1 (Atezolizumab, Durvalumab and Avelumab) have been approved to treat malignancies such as those of lung, liver and breast (68–71). Durable control over tumor progression and improvement of OS have been achieved in responsive patients. Unfortunately, many patients do not respond to anti-PD-1 or anti-PD-L1 ICB therapies.
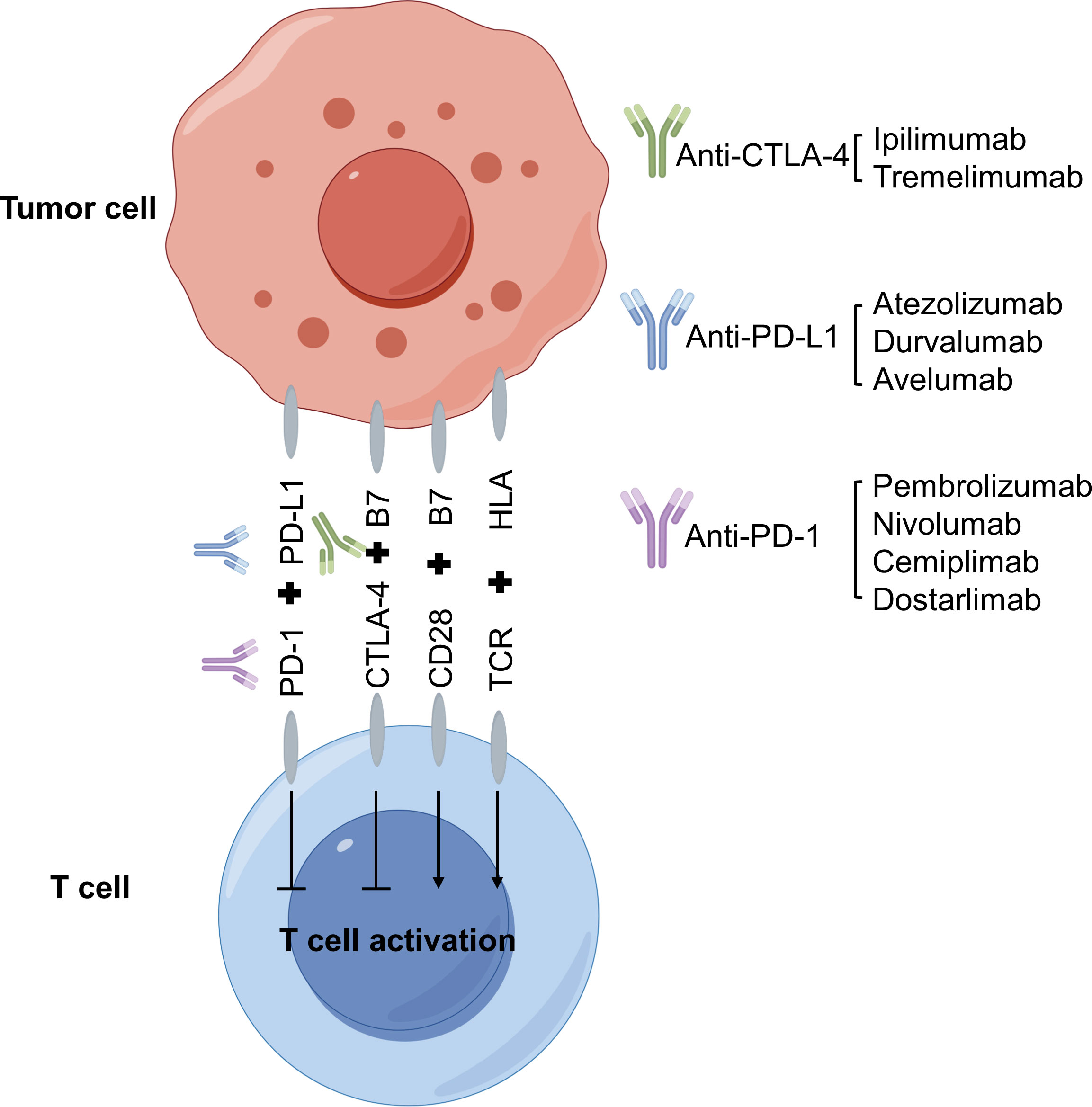
Figure 1 Mechanism of immune checkpoint blockade therapy. Immune checkpoints refer to the signaling pathways that dampen the activation of T cells by antigen presenting cells (APCs) or tumor cells. Immune checkpoint blockade (ICB) therapies use monoclonal antibodies to alleviate the inhibitory effects of immune checkpoints, including cytotoxic T-lymphocyte antigen 4 (CTLA-4) (Ipilimumab and Tremelimumab), PD-1 (Pembrolizumab, Nivolumab, Cemiplimab and Dostarlimab) and PD-L1 (Atezolizumab, Durvalumab and Avelumab). ICBs reinvigorate T cells via the activation of downstream signaling pathways such as MAPK and AKT-mTOR. Figure made by figdraw.
The clinical efficacy of ICB therapies varies among different tumor types. The first category is exemplified by melanoma and NSCLC. Many patients with these malignancies exhibit a high and durable response to ICB therapies. In other cancers such as those of colon and endometrium, most patients do not respond. However, a small subset of patients demonstrates exceptional responses due to high microsatellite instability and mismatch repair deficiency in the tumor (72, 73). One unifying mechanistic explanation for clinical benefits in melanoma, NSCLC and mismatch repair-deficient cancers is that these tumors usually contain high mutational burden, thus increasing the chance to generate neoantigens to elicit antitumor immunity (74). The third group, exemplified by ovarian cancers, is completely refractory to PD-1- or PD-L1-targeting ICBs, either in the setting of monotherapy or in combination with other anti-cancer drugs (18, 75, 76). Ovarian cancers are the most lethal gynecological cancer. The lack of clinical efficacy is surprising because tumor-infiltrating lymphocytes (TILs) are present in a substantial portion of ovarian cancer patients (77). Currently, it is unknown why ovarian cancers do not respond to ICBs. One potential explanation is that other checkpoint pathways such as TIM-3 are at play (78). Alternatively, lack of response to ICBs is consistent with the fact that most T cell infiltrates in ovarian tumors are bystanders reactive to viruses rather than tumor antigens (79).
3 T-cell receptor repertoire analysis and its application to dissect the mechanisms of ICB therapies
At the cellular level, tumor-derived antigens are presented by antigen presenting cells, such as dendritic cells, to T-cell receptor (TCR) expressed on the plasma membrane of T cells. Most TCRs in humans are dimers of an alpha (α) and a beta (β) chain. The amino acid sequences of α and β chains are highly diverse due to a random joining of variable (V), junctional (J) and diversity (D) gene fragments (80, 81). The α chain is generated by the recombination of VJ segments, and the β chain by VDJ recombination. The vast sequence diversity of α and β underlies the ability of TCR to recognize numerous tumor antigens to initiate antitumor immunity. Thus, the TCR repertoire, that is, the collection of nucleotide composition of α and β chains, is used to infer immunological status of patients.
Recent advances in bulk and single-cell RNA sequencing (RNA-seq) have enabled measurement of patient TCR repertoire (82–84). To determine TCR repertoire by bulk RNA-seq, RNA extracted from thousands of T cells is used to amplify VDJ gene segments of α and β chains, followed by next-generation sequencing. Bioinformatic tools are then used to annotate the sequence and abundance of each α and β chain. Bulk RNA-seq is low cost, but does not generate information on the paired α and β chain in each T cell. Although bioinformatic algorithms are available to infer paired α and β chains, their accuracy is moderate. The information of paired α and β chain in each T cell is important as the affinity of TCR to antigens is dictated by sequences of both α and β chains. Thus, single-cell RNA-seq of TCR repertoire (sc-TCR-seq) fulfills this gap albeit at a higher cost. Information generated from sc-TCR-seq can be used for downstream functional assays to identify and validate antigen-specific TCRs (85). The combination of bulk- and sc-TCR-seq allows comprehensive analysis and sensitive tracking of T-cell dynamics at the clonal level to understand ICB-induced antigen-specific T-cell responses.
Early studies suggested that TILs may be an independent predictor of patient survival. Originally, the mechanism-of-action of ICB therapies was believed to activate TILs for durable tumor control. It was assumed that ICBs activate pre-existing, intratumoral T cells (86). However, by mapping TCR repertoire, recent studies have collectively demonstrated that in responsive patients the peripheral compartment is the major source of T cells activated by ICB therapy, and a majority of pre-existing intratumoral T cells are terminally exhausted and refractory to ICB therapies. Depending on the disease stage, a small subcluster of pre-exhausted CD8+ T cells within TME, termed precursors of exhausted T cells or Tpex, may also contribute to ICBs (87). These observations imply that tumor compartment actively communicates with the peripheral immune system (88). ICB-induced clonal expansion of antigen-specific T cells may be indicated by a decrease in TCR diversity. Conversely, ICB-induced migration of T cells to the tumor may increase the diversity of TCR repertoire. Thus, diversity score can be calculated to allow statistical analysis of TCR clonality during ICB treatment as well as differential responses among patients.
4 Overview of the CD8+ T cell infiltrates in human tumor microenvironment
Tumor microenvironment (TME) is composed of intertwining cancer cells, immune cells, stromal cells, and extracellular matrix (89, 90). Recent studies using single-cell transcriptomic profiling and TCR repertoire sequencing have revealed that T cell infiltrates in TME are populated with tumor-reactive CD8+ T cells, but also with bystander CD8+ T cells recognizing viral antigens (91, 92). Bystander T cells do not contribute to the antitumor immunity, nor do they respond to ICBs (93). Different tumor types have various amount of bystander T cells. Notably, more than 80% TILs in ovarian tumors are bystanders (94). This may explain why they are refractory to ICB therapies.
Tumor-reactive cytotoxic CD8+ T cells are central to successful antitumor immunity in numerous pre-clinical mouse models, and thus the main focus in a majority of studies using patient specimens. Historically, CD8+ T cells are classified as naive, effector and memory T cell subsets, based on their differentiation status (Figure 2). Naive T cells (Tn) are resting T cells yet to be exposed to antigenic stimulation. Once stimulated by antigens, Tn cells develop into effector T cells (Teff) acquiring cytotoxic functionalities. A subset of Teff cells further converts into resting memory T cells (Tm) to prepare the host for future challenge of cognate antigens. CD8+ Tn cells can be identified by the expression of CC chemokine receptor 7 (CCR7), lymphoid enhancer binding factor 1 (LEF1), interleukin-7 receptor (IL7R), transcription factor 7 (TCF7; also known as T cell factor 1,TCF1) and selectin L (SELL) (95, 96). Teff cells are commonly identified by the expression of perforin 1 (PRF1), granzyme A (GZMA), GZMB and natural killer cell granule 7 (NKG7). They also express other cytotoxicity markers including CX3C chemokine receptor 1 (CX3CR1), killer cell lectin-like receptor subfamily G member 1 (KLRG1) and Fcγ receptor IIIA (FCGR3A) (49, 97). Recent characterization of TILs by high dimensional techniques have revealed several subclusters with potentially different functionalities within each classical classification. Different research groups have named these subclusters with different names. Their functional implications for ICBs warrant further investigation (49, 87, 95, 96).
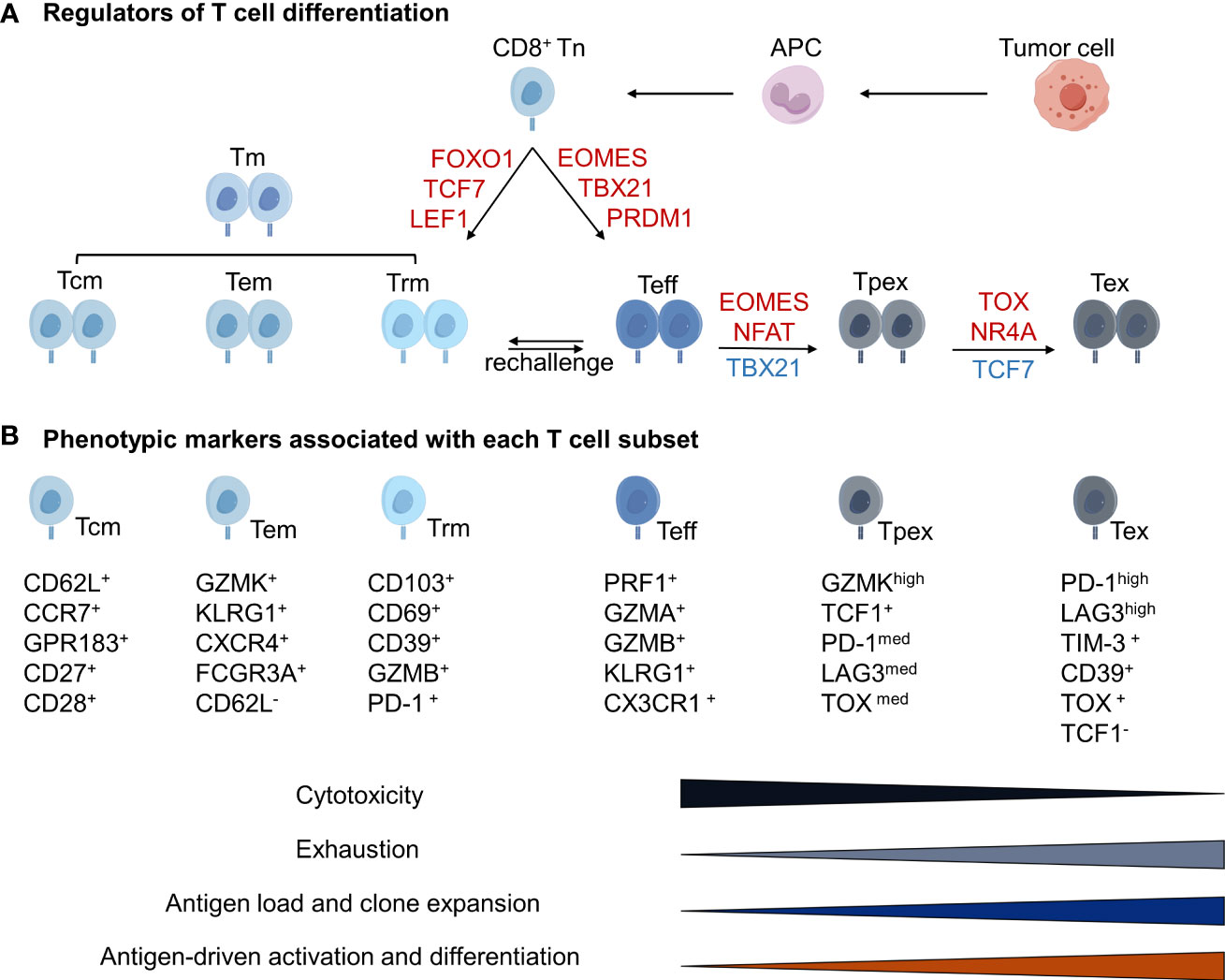
Figure 2 Diverse T cell states in human tumor and periphery compartments identified by single-cell analytic techniques. APCs promote resting native CD8+ T cells (Tn) to differentiate into cytotoxic, proliferative effector cells (Teff). Long-lived memory cells (Tm) are derived either from Teff or directly from Tn. Single-cell transcriptomic profiling studies further divide Tm into several subsets including central memory (Tcm), effector memory (Teff) and resident memory (Trm), based on differential gene expression profiles. Tm cells can differentiate into Teff cells when they are rechallenged by cognate antigens. In tumor microenvironment (TME), continuous exposure to tumor-derived antigens eventually induces Teff to enter a dysfunctional state, also known as exhausted state (Tex). Tex cells are characterized by increased expression of immune checkpoints and reduced cytotoxicity. Recent studies also identify a progenitor cellular state to Tex (Tpex), and have proposed that Tpex cells are the main cell population reinvigorated by ICB therapies. (A) Transcriptional regulators associated with each T cell state. Factors in red and blue denote increased and decreased expression, respectively. (B) Phenotypic markers associated with each T cell subset. FOXO1 (Gene ID: 2308); TCF7 (also known as TCF-1; Gene ID: 6932); LEF1 (Gene ID: 51176); EOMES (also known as TBR2; Gene ID: 8320); TBX21 (also known as T-bet; Gene ID: 30009); PRDM1 (also known as BLIMP1; Gene ID: 639); NFAT (Gene ID:32321); TOX (also known as TOX1; Gene ID: 9760); NR4A includes NR4A1 (Gene ID: 3164), NR4A2 (Gene ID: 4929) and NR4A3 (Gene ID: 8013). Figure made by figdraw.
The functional states of CD8+ Tm cell pool are the key to sustained tumor control. These long-lived cells can rapidly enter the mitotic division to generate a large quantity of Teff cells after restimulation by cognate antigens. CD8+ Tm cell pool consists of heterogeneous cell types differing in epigenetic, transcriptional and translational status (98). Two major subpopulations, CD8+ central memory T cells (Tcm) and CD8+ effector memory T cells (Tem), differ in several aspects. Tcm, abundant in the spleen, blood and lymph nodes, exhibits a high proliferative potential upon reactivation, usually identified by the expression of the lymphatic homing markers CD62L, CCR7, GPR183, CD27 and CD28 (51, 99). Tem, present in the spleen and blood, is highly cytotoxic upon reactivation, usually identified by the expression of GZMK, KLRG1, CXCR4 and FCGR3A but lack of CD62L (99, 100). In addition, recent studies have identified a third subcluster, tissue resident memory T cells (Trm), usually marked as CD103+CD69+ cell population. Trm is excluded from circulation and resides in tissues under steady-state conditions (53, 101, 102). Compared to Trm within adjacent normal tissues, Trm within tumors was found to increase the expression of dysfunctional markers such as TIM-3, PD-1, CTLA-4 and LAYN (51, 103), suggesting that they have experienced with tumor antigens.
Tumor-reactive, cytotoxic T cells eventually enter a terminally dysfunctional or exhausted cellular state (Tex) due to chronic and sustained stimulation by tumor antigens (49, 95, 96). Tex cells are characterized by increased expression of cell surface inhibitory receptors, PD-1, CTLA-4, LAG3 and TIM-3. Tex cells are also characterized by much reduced effector functions to produce inflammatory cytokines interleukin 2 (IL-2), tumor necrosis factor (TNF), and interferon gamma (IFNγ) (104–106). Additional regulators of Tex cells have been identified by recent studies, such as LAYN, CD39 and TOX (100, 107–112). Transcription factors TOX1 and TOX2 can cooperate with NR4A to induce the expression of multiple immune checkpoints including CTLA-4, PD-1 and TIGIT in CD8+ T cells (110), possibly by opening and increasing the chromatin accessibility of these genes for transcription (113). CD39 and CD73 are two ectonucleotidases that can convert extracellular ATP into adenosine, favoring immunosuppressive environment (114). Therapeutic monoclonal antibodies as well as small molecules targeting CD73 (115, 116), and therapeutic mAbs targeting CD39 are being evaluated by phase 1 studies (117). It will be of interest to see whether these reagents can activate Tex cells.
4.1 CD8+ T cell dysfunction is a progressive state within tumor microenvironment
Tex cells within tumors are composed of highly heterogenous cell populations, likely reflecting their natural history of responding to antigenic stimulation during tumorigenesis. Advanced, full-blown tumors contain highly exhausted, dysfunctional T cells due to persistent antigen stimulation, whereas tumors at early stages may contains T cells on the path to the terminally exhausted states (48, 118–120). In mice and humans, significant phenotypic diversity is observed in the intratumor T cell pool, reflected by various combinations and expression levels of inhibitory and co-stimulatory receptors, such as TIM-3, CTLA-4, CD39, 4-1BB and PD-1 (104, 121–123). A pre-dysfunctional cellular state (Tpex) has been identified in CD8+ T cells in various human cancers, characterized by higher expression of inhibitory receptor genes than naive and cytotoxic T cells, but lower than dysfunctional T cell population. These Tpex cells are defined by high expression of GZMK and moderate expression of genes such as PD-1 and LAG3 (96). In other studies, Tpex cells are also defined as GZMK+ and ZNF683+ cell population expressing low to moderate levels of inhibitory receptor genes (47, 49, 97, 99, 100). In contrast, terminally exhausted Tex cells express high levels of co-repressor molecules LAYN, PD-1, CTLA-4, TIM-3, LAG3 and TIGIT (47, 49, 95–97, 99, 100). These cells show much reduced ability to perform classical CD8+ T cell effector functions, diminished responsiveness to cytokine stimulation, and inability to form a memory T cell pool. These cellular characteristics are regulated by metabolic reprogramming and epigenetic modifications (124). Tex cells at early stage have stem cell-like and memory-like phenotypes, express low levels of effector transcripts, and have a strong proliferative capacity (125–128). In addition, they still express various effector molecules, such as IFN-γ and granzyme B. These observations suggest that Tex cells at early stage may still be functional, but also imply that they are true tumor-reactive T cells within tumor microenvironment (104, 105, 125, 129–131).
To our knowledge, there is no approved drugs to reverse the cellular state of Tex cells. In preclinical studies using animal models, recent findings have suggested that the manipulation of epigenetic and metabolic regulations might partially revive Tex cells (132–137). The efficacy of these interventions is yet to be evaluated by clinical trials. Because Tex cells within tumor bed express high levels of TIM-3 and LAG3, it is hypothesized that the combination of anti-PD-1/L1, anti-TIM-3 and anti-LAG3 treatment may reactivate Tex cells. This assumption is currently being tested in phase 1 studies (NCT03099109 and NCT02460224).
4.2 Characterization of CD8+ T cells in the tumor microenvironment during ICB therapies
ICBs hinge upon the reinvigoration of tumor-reactive cytotoxic immune cells, mainly CD8+ T cells (Table 1). In the context of PD-1 or PD-L1 blockade, the relevant CD8+ T populations within the tumor microenvironment (TME) are not exactly clear, but could be Tpex cells (87, 104, 125). There are highly heterogeneous Tex and Teff/Tm populations in tumors, and it is not fully understood which subpopulation is activated by the ICB therapies. Studies have suggested that PD-1 blockade promotes Tex cell (LAG3+TIM-3+GZMA+PD-1+) infiltration in TME (154). The exhausted CD8+ TILs could undergo clonal expansion after ICBs (47, 120, 139). In a study of NSCLC patients treated with Nivolumab or Pembrolizumab, high PD-1+ TILs were found to be related to significantly PFS and OS (140). In the neoadjuvant context of ICBs, tumor-specific CD8+ T cells isolated from non-major pathological response patients expressed high levels of genes associated with T cell dysfunction, such as TOX2, CTLA-4, TIM-3, and CD39. This observation confirms that terminally exhausted T cells cannot be reinvigorated by ICB therapies (138). Quantitative multiplex immunofluorescence analysis of melanoma tumors treated with ICBs revealed that the presence of some exhausted CD8+ T cells were in a progenitor state, termed Tpex, on the path to differentiate into Tex cells. Significantly, a higher percentage of Tpex cells was correlated with a longer duration of response to ICB therapy in melanoma patients (152). Similarly in NSCLC patients, Tpex cells, expressing low coinhibitory molecules (CD39- TIM-3- LAYN-) and high level of GZMK, increase in responsive tumors after treatment (50).

Table 1 Proposed biomarkers of clinical response to immune checkpoint blockade immunotherapy or prognosis based on immune cell populations.
Therefore, PD-1 inhibitors may reduce or revert the dysfunctional state of tumor-specific CD8+ T cells in TME. The extent that ICBs can reinvigorate Tex cells is influenced by several cell intrinsic features. The cellular state of Tex is determined by transcriptional and epigenetic factors (133, 179, 180). Several studies have demonstrated that PD-1 blocking antibodies can briefly revive Tex cells. However, this effect is not durable because these antibodies cannot reverse the epigenetic status of Tex cells (181–183). Nevertheless, a sustained response to the ICB therapies occur in some patients, indicating that Tex cells within TME may be in the progenitor rather than the fully differentiated state. However, other studies suggest that ICBs primarily promote the proliferation of a stem-like TILs subset, but not the reversal of T cell exhaustion programs. The majority of TME in stage IV metastatic melanoma patients who benefited from TIL-adoptive T cell therapy (ACT) had molecular characteristics of stem-like memory T cells, including the expression of TCF7, KLF2 and CD62L (184). Analysis in renal cancer TME revealed that a population of stem memory T cells with TCF7+TIM-3-CD28+ phenotype continued to proliferate and differentiate into tumor-killing effector T cells. Patients without such stem memory T cells in TME had an overall reduced T-cell infiltration and poor clinical prognosis (185). Similarly, in MSI-H gastric cancer, patients who responded to Pembrolizumab had a high proportion of stem-like exhausted cells in dysfunctional CD8+ TILs (177). Collectively, these studies suggest that different tumor stages, and thus the degrees of T cell exhaustion, is one of major determinants of ICB responsiveness.
The TCF7+PD-1+ stem memory T cell subpopulation was shown to be the primary source of T cells in mouse TME that could generate a sustained response to immunotherapeutic regimens such as ICBs and tumor vaccines. This subpopulation shares some transcriptomic similarity with Tm cells, but differs markedly at the epigenetic level (152). Other studies have shown that cells with effector-memory characteristics respond in ICB therapy (49, 153). In melanoma treated with Pembrolizumab, the CD8+ Tem subset (CD45RO+CCR7-CD27-CD57-) is the major expanded population in responsive patients (153). In another study, elevated frequencies of TCF7+ CD8+ T cells in melanoma specimens after ICBs were found to predict positive outcome (186). The molecular and phenotypic features, and the origin of this subpopulation of “tumor-specific Tm cells” in human TME are a subject of intensive research due to its relevance to ICB therapies (87, 103, 158, 186).
Many studies have indicated that Trm cells play a critical role in controlling tumor initiation and progression (51–53, 171, 187, 188). Trm cells mostly reside in tissues and do not recirculate in the bloodstream, even after reactivation by cognate antigens. RNA-seq analysis of excised NSCLC tumors shows that tissue-resident memory features are linked to the magnitude of cytotoxic T cell responses and better survival outcome (188). In NSCLC, Trm (TIM-3+PD-1+CD103+) cells expressing proliferation (Ki67+) and cytotoxicity (GZMB+IFN-γ+TNF+IL-2+) markers are enriched after anti-PD-1 therapy (51, 52). In TNBC, analysis by multicolor flow cytometry showed that Trm (CD39+CD69+CD103+) were enriched, correlated with OS, and could be reinvigorated by ICBs ex vivo (103, 171). In HNSCC, CD8+ T cells that underwent clonal expansion during ICB treatment expressed elevated tissue-resident memory (CD103+ZNF683+) and cytotoxicity programs (GZMB+PD-1+CTLA-4+) and show tumor-specific recognition (53).
5 Characterization of CD8+ T cells in peripheral blood during ICBs
In addition to TME, recent studies highlight the contribution of peripheral T cells during ICBs (Figure 3). In the peripheral compartment, lymph nodes are the primary location for antigen presentation cells to activate antigen-specific T cells (189, 190). Once activated, these T cells can migrate and infiltrate into the tumor to complement the effector population, emphasizing the importance of systemic immune responses (Table 1, Figure 3) (47, 48, 191).
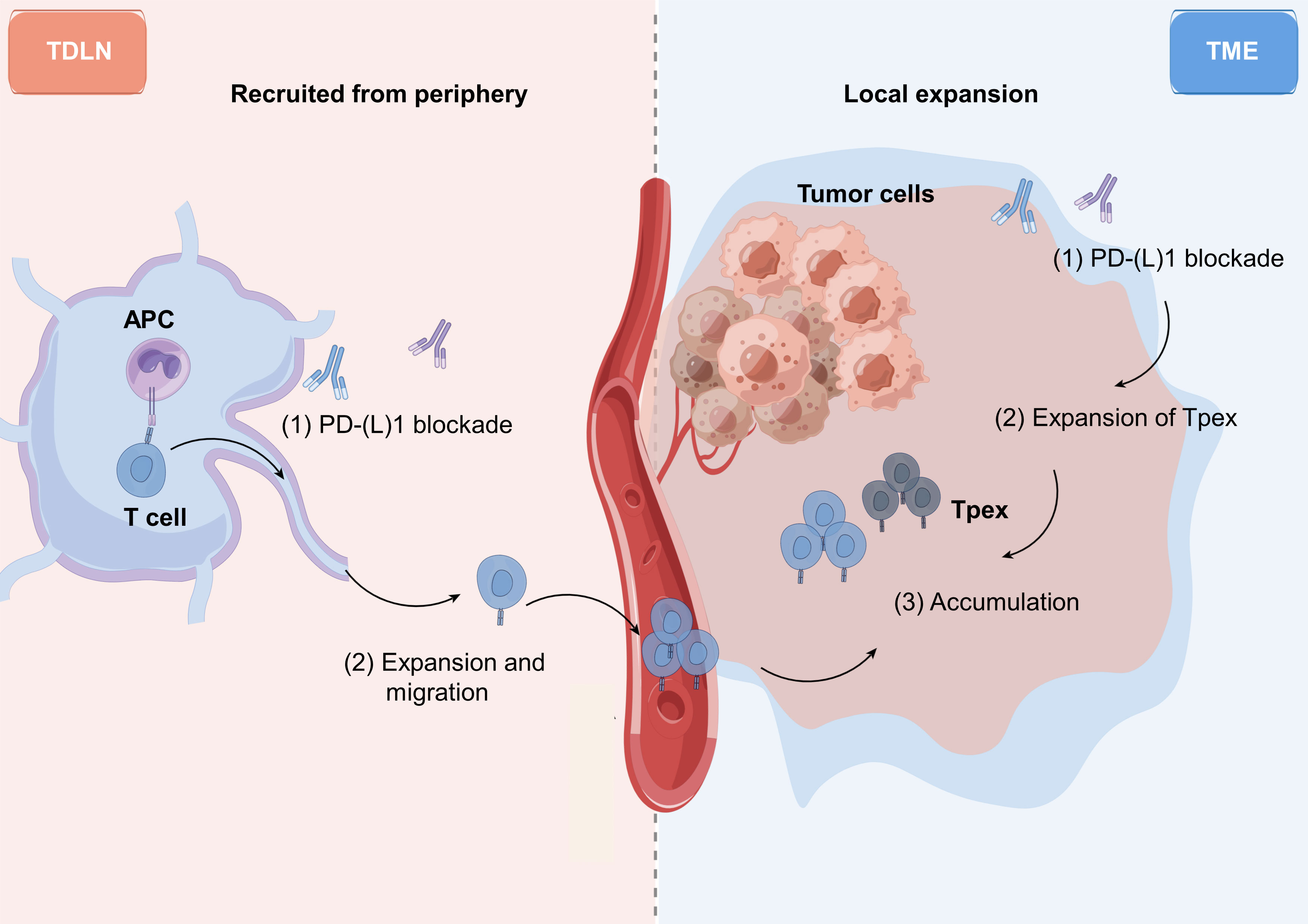
Figure 3 ICB therapies potentiate tumor-reactive CD8+ T cells in both periphery and TME. Single-cell transcriptomic and TCR repertoire analyses collectively suggest that the source of ICB-induced tumor-reactive T cells come from TME as well as peripheral lymph nodes. In ICB-responsive cancer patients, progenitor of exhausted T cells (Tpex) within TME, characterized by GZMKhigh, LAG3med and PD-1med, are likely the cell population revived by ICB therapies. In responsive patients, peripheral tumor-reactive CD8+ T cells, containing different TCR sequences from Tpex cells, are also evident. These cells can enter TME to kill tumor cells. The relative contributions of peripheral tumor-reactive CD8+ T cells and Tpex in TME likely vary in individual patient. TDLN, tumor-draining lymph node; TME, tumor microenvironment. Figure made by figdraw.
The core of successful ICBs relies on the activation and proliferation of antigen-specific T cells. Paired sc-RNA and sc-TCR-seq analysis of melanoma patient specimens demonstrated that clonal T cells in periphery are in a more activated cellular state (CCL5+GZMB+KLRG1+KLRK1+CX3CR1+) than their counterparts in TME (PD-1+LAG3+CTLA-4+TIM-3+TIGIT+) (155). In the peripheral blood of NSCLC patients treated with anti-PD-1, CD28-positive CD8+ T cells were activated and proliferative (142). Circulating PD-1+ CD8+ T cells have been reported to be tumor-specific and share TCR sequences with PD-1+CD8+ TILs (157). Studies suggest that ICBs stimulate the proliferation of peripheral PD-1+CD8+ T cells (161, 173), and the frequency of this subset predicts the response to ICBs (143, 144, 160, 177). In melanoma patients treated with anti-PD-1 antibodies, peripheral CD8+ T cells were proliferative and showed co-expression of PD-1 and CTLA-4 at day 7 after treatment, but only in responsive patients (159–161). This subset of peripheral CD8+ T cells, expressing conventional activation markers (HLA-DR+CD38+) and proliferation marker (Ki67+), was associated with better response to ICB therapies in a variety of cancer types (53, 143, 144, 162, 172, 192).
One would expect that ICB-stimulated periphery T cells should have different clonotypes from those in TME. Indeed, TCR sequencing analysis revealed the emergence of new clonotypes in responsive patients, suggesting de novo priming of tumor-specific T cells (162, 172). Interestingly, the status of exhaustion marker TIM-3 on CD8+ T cells is associated with contrasting outcome in different cancer types. In NSCLC and renal cell carcinoma (RCC) patients treated with anti-PD-1, the frequency of TIM-3+CD8+ T cells was higher in non-responders (145). In contrast, in esophageal squamous cell carcinoma, higher frequency of TIM-3+CD8+ T cells was associated with responsive patients (175). This discrepancy may reflect the fact that inhibitory checkpoints are expressed in both progenitors of exhausted cells and highly exhausted cells. Thus, using single marker, such as TIM-3 or PD-1, may not be reliable to determine the functional state.
Peripheral CD8+ T cells with effector characteristics are closely associated with the immune response to ICBs. In gastrointestinal (GI) cancers, CD8+ T cells from responsive patients acquired greater cytotoxic capacity after immunotherapy (176). This CD8+ T cell cytotoxicity is also associated with clinical response to ICBs in melanoma (164). The expansion of a subset of peripheral CD8+ Teff cells (CD45RA-CD45ROhighCD27-CCR7-) correlated with response in melanoma patients receiving anti-PD-1 and/or anti-CTLA-4 (163). In different types of cancers, greater expansion of effector-like T cells from periphery is highly correlated with the efficacy of ICBs (48). This response to ICBs is not limited to conventional Teff, but also occurs in Tpex that retain effector functions (50, 156). In NSCLC patients treated with Pembrolizumab, periphery-derived Tpex cells were increased in ICB responders (50). Similarly in melanoma, peripheral Tpex (TCF7negT-bethighToxmed) had an “effector-like” profile and increased after treatment with Pembrolizumab. These “effector-like” CD8+ Tpex can be activated in the periphery by PD-1/PD-L1 blockade but not in the tumor (156). However, increased frequencies of CD8+ Teff (GZMB+EOMES+) at 6 months were associated with relapse in melanoma patients with Ipilimumab (170), suggesting that these cells might be terminally exhausted.
A higher percentage of specific subclusters of CD8+ Tm cells in peripheral blood at baseline is associated with better survival and enhanced clinical response (149, 151, 165, 169). NSCLC patients received Nivolumab with high Tcm (CCR7+CD45RA-)/Teff (CCR7-CD45RA+) ratios had longer PFS (151). High baseline frequencies of CD8+ Tem were correlated with longer OS and better clinical response in melanoma patients (165). The baseline levels of CD45RO+CD8+ Tm cells correlated with response rate and survival in melanoma patients treated with Ipilimumab (169). Amplification of CD8+ Tem in the peripheral blood during ICB therapies has also been reported to be associated with the response to ICBs. In stage IV NSCLC, ICB-induced expansion of peripheral CD8+ Tem cells (GZMK+PD-1+) with de novo primed TCRs are associated with favorable clinical outcome (146). In melanoma, responders have higher frequencies of Tem cell (CCR7-CD45RA-) after ICB treatment (168). In another melanoma study, increased CD8+ Tem expressing CX3CR1 was shown in patients responding to ICBs, and this subset has effector memory phenotypes and cytotoxic activity (167).
TCR diversity in peripheral blood is associated with clinical benefit in patients with ICB therapies (141, 150). Studies have shown that ICB therapies induce peripheral T cell repertoire rearrangements, and the recruitment of T cells from the periphery to the tumors in ICB responders in melanoma and NSCLC (163, 166). In a variety of tumor types, the effectiveness of ICBs correlates with the diversity in peripheral TCR repertoire either before or after the ICB therapy (147, 148, 174, 178). Indeed, various studies suggest that effective antitumor responses induced by ICB therapies may arise from de novo specificity generated in the periphery, and are therefore not affected by the immunosuppressive TME (47, 48, 166).
The role of CD4+ T cells in ICB-induced antitumor responses is far from clear. Only a few studies have mentioned the relationship between CD4+ T cells and response to ICBs. CD4+ Tem cell frequency in peripheral blood at baseline is associated with the responsiveness to ICB therapies (145, 193–196). Like CD8+ T cells, CD4+ T cells in responsive patients exhibited proliferative capacity, low expression of PD-1 and LAG3, and responded to PD-1 blockade in vitro and in vivo (195). In the context of melanoma, the frequency of peripheral CD4+ Tem (CD45RO+CD62L-) cells was higher in nonresponsive patients (159). The correlation of circulating Treg cells with ICBs is also unclear. Some studies suggested that responsive patients had a lower percentage of circulating Treg cells prior to anti-PD-1 than nonresponsive patients (194, 195), while others found no such correlation (159). Some studies even reported that a high percentage of circulating Treg cells before or after treatment was associated with better response (196–198). The discrepancy among these studies is unclear. To reconcile these findings, one could argue that higher percentage of circulating Treg cells may reflect a negative feedback mechanism to dampen tumor-reactive cytotoxic T cells during tumorigenesis.
Peripheral T cell analysis offers the advantage to monitor dynamic changes during the course of ICB therapies. However, there are also several limitations. The peripheral TCR repertoire is orders of magnitude higher than the TCR repertoire within the tumor environment, and dynamically influenced by environmental factors such as diet and infections. In contrast, the TCR repertoire within the tumor environment is relatively stable and simple, composed of limited TCRs reactive to tumor antigens. Thus, it is sometimes difficult to draw conclusions solely based on the analysis of the peripheral T cells. In fact, high levels of TIM-3+ CD8+ subset in the periphery are associated with both favorable and poor patient responses (145, 175).
Collectively, these studies highlight the positive association between the reinvigoration of tumor-reactive cells in the periphery and the responsiveness to anti-PD-1/PD-L1 therapies in cancer patients. Because the periphery is not constantly in contact with tumor cells, reinvigorated periphery T cells should be tumor-reactive and not in the exhausted state. In addition, ICBs are usually applied after the cytoreductive surgery, and the immunosuppressive tumor environment is alleviated. Thus, the combination of active T cell state and less suppressive microenvironment may allow reinvigorated periphery T cells to eliminate residual tumor cells after debulking surgery. However, these newly activated T cells will still succumb to mechanisms of primary and required resistance as their counterparts in the tumor bed, such as reduced antigen presentation due to loss of HLA molecules and attenuated interferon signaling pathways (199). Lastly, the exact nature of reinvigorated periphery T cells warrants further investigation. Tpex cells within the tumor bed are likely the primary target of ICBs. It is clear ICBs also activate tumor-reactive T cells in the periphery, and these cells can have the same TCRs as Tpex cells within tumor. However, shared coloniality does not necessarily mean common origin. Considering the enormous diversity of periphery TCR repertoire, it is possible that ICB-activated periphery T cells are de novo primed rather than from tumor Tpex cells. In fact, peripheral T cells from healthy donors can react to tumor associated antigens or neoantigens derived from cancer patients (200). In this case, enhanced priming of T cells, such as by CD40 signaling agonists and dendritic cell vaccines, will likely synergize with ICBs (201). Indeed, ICBs can enhance the ability of dendritic cells to active antigen-specific T cells to boost anti-tumor immunity (202–204).
6 Conclusions and perspectives
ICB therapy was thought to activate preexisting, tumor-infiltrating CD8+ T cells. Recent advances in single-cell analytic techniques have enabled the reassessment of this assumption. Single-cell transcriptomic and TCR analyses allow the deduction of various T cell states with distinct functionalities. They also facilitate the identification of tumor-reactive T cell clones during tumorigenesis and through the course of ICB therapy. Beyond the tumor-infiltrating CD8+ T cells, the contribution of periphery tumor-reactive T cells are now appreciated as an important determinant of the effectiveness of ICBs. Thus, monitoring periphery TCR repertoire and T cell states can be an effective approach to assess the response to ICB therapies. The understanding of parameters influencing ICB response would ultimately provide rationales to improve ICB strategies. Patients with higher percentage of CD8+ Tem and Tpex subsets in either their tumor biopsies or peripheral blood will likely benefit from anti-PD-1 or anti-PD-L1 treatment. Retrospective analyses of current studies may provide baselines of these subsets for future clinical trials to stratify patient population beyond PD-L1+ cells. On the other hand, a common thread of the Tex subset among different tumor type is that these cells express high levels of PD-1, LAG3, TIM-3 and CD39. Thus, the combination of anti-PD-1/L1 with anti-LAG3 and/or anti-TIM-3 monoclonal antibodies are expected to achieve higher clinical efficacy (205, 206). In addition, antibody-drug-conjugates based on anti-CD39 monoclonal antibody are being developed, providing additional means to drug Tex cells (207). Ultimately, a better understanding of molecular characteristics of tumor-reactive T cells, either in TME or derived from the periphery, may help develop novel strategies to improve the response rate and the duration of ICBs.
ICBs have achieved remarkable therapeutic success in a variety of human cancers. Nevertheless, a large portion of cancer patients have yet to benefit from ICB therapies. Recent investigations have shed light on the-mechanism-of-action of ICBs in patients. Building on these discoveries, several research avenues are worth pursuing. From a technical and practical perspective, systematic cross-platform validation and integration of large-scale datasets from patients is needed to harmonize key findings. For example, the field needs to reach a consensus on the phenotypic markers for various T cell subsets in order to better define and compare patient samples at different disease stages. It is still unclear whether the cellular state of Tpex cells, the key target of ICBs, will change in the course of tumor initiation and progression. Single-cell sequencing technology is instrumental to elucidate immune cell compositions and states in basic research, but difficult to apply in clinical settings. Future studies are needed to translate findings from single-cell sequencing studies into clinically actionable parameters. Perhaps most importantly, can we rely on information on the composition of T cell subsets to guide future clinical trial designs? New therapeutical modalities targeting additional immune checkpoints and activators beyond PD-1/L1 and CTLA-4 are rapidly emerging. Stratification of patients based on the composition and functional states of T cells would certainly be advantageous in clinical trials. To achieve this goal, major efforts are needed to develop clinically actionable parameters to define each T cell subset.
Author contributions
HL: Writing – original draft. WW: Writing – original draft. JM: Writing – original draft. RY: Writing – review & editing. XC: Writing – review & editing. QL: Writing – review & editing.
Funding
The authors declare financial support was received for the research, authorship, and/or publication of this article. The National Natural Science Foundation of China (Both to QL) (No.31971141, No.32271348), the National Natural Science Foundation of China (to XC) (No.81972911), and the Science and Technology Department of Sichuan Province (to QL)(2021YJ0012).
Conflict of interest
The authors declare that the research was conducted in the absence of any commercial or financial relationships that could be construed as a potential conflict of interest.
Publisher’s note
All claims expressed in this article are solely those of the authors and do not necessarily represent those of their affiliated organizations, or those of the publisher, the editors and the reviewers. Any product that may be evaluated in this article, or claim that may be made by its manufacturer, is not guaranteed or endorsed by the publisher.
Abbreviations
ICBs, immune checkpoint blockade; TCR:T-cell receptor;CTLA-4:cytotoxic T lymphocyte associate protein-4;PD-1:programmed death receptor1;PD-L1:programmed death ligend1;ICIs:immune checkpoint inhibitors;NSCLC, non-small cell lung carcinoma; TILs, tumor-infiltrating lymphocytes;LAG3, activation gene 3 protein; TIM-3:T-cell immunoglobulin mucin-3;OS:overall survival;PFS, progression-free survival; TME;tumor microenvironment;Tn:naive T cells;Teff:effector T cells;Tm:memory T cells;Tcm:central memory T cells;Tem:effector memory T cells;Trm:tissue resident memory T cells;Tex:terminally exhausted T cells;Tpex:precursor exhausted T cells;sc-RNA-seq:single-cell RNA sequencing; sc-TCR-seq, single-cell RNA-seq of TCR repertoire; ACT, TIL-adoptive T cell therapy; GI, gastrointestinal.
References
1. Vaddepally RK, Kharel P, Pandey R, Garje R, Chandra AB. Review of indications of FDA-approved immune checkpoint inhibitors per NCCN guidelines with the level of evidence. Cancers (Basel) (2020) 12(3):738. doi: 10.3390/cancers12030738
2. Tawbi HA, SChadendorf D, Lipson EJ, Ascierto PA, Matamala L, Castillo Gutiérrez E, et al. Relatlimab and nivolumab versus nivolumab in untreated advanced melanoma. New Engl J Med (2022) 386(1):24–34. doi: 10.1056/NEJMoa2109970
3. Das M, Zhu C, Kuchroo VK. Tim-3 and its role in regulating anti-tumor immunity. Immunol Rev (2017) 276(1):97–111. doi: 10.1111/imr.12520
4. Falchook GS, Ribas A, Davar D, Eroglu Z, Wang JS, Luke JJ, et al. Phase 1 trial of TIM-3 inhibitor cobolimab monotherapy and in combination with PD-1 inhibitors nivolumab or dostarlimab (AMBER). J Clin Oncol (2022) 40(16_suppl):2504–. doi: 10.1200/JCO.2022.40.16_suppl.2504
5. Curigliano G, Gelderblom H, Mach N, Doi T, Tai D, Forde PM, et al. Phase I/ib clinical trial of sabatolimab, an anti-TIM-3 antibody, alone and in combination with spartalizumab, an anti-PD-1 antibody, in advanced solid tumors. Clin Cancer Res (2021) 27(13):3620–9. doi: 10.1158/1078-0432.CCR-20-4746
6. Borate U, Esteve J, Porkka K, Knapper S, Vey N, Scholl S, et al. Phase ib study of the anti-TIM-3 antibody MBG453 in combination with decitabine in patients with high-risk myelodysplastic syndrome (MDS) and acute myeloid leukemia (AML). Blood (2019) 134(Supplement_1):570–. doi: 10.1182/blood-2019-128178
7. Huang X, Zhang X, Li E, Zhang G, Wang X, Tang T, et al. VISTA: an immune regulatory protein checking tumor and immune cells in cancer immunotherapy. J Hematol Oncol (2020) 13(1):83. doi: 10.1186/s13045-020-00917-y
8. Yap TA, Gainor JF, Callahan MK, Falchook GS, Pachynski RK, LoRusso P, et al. First-in-human phase I/II ICONIC trial of the ICOS agonist vopratelimab alone and with nivolumab: ICOS-high CD4 T-cell populations and predictors of response. Clin Cancer Res (2022) 28(17):3695–708. doi: 10.1158/1078-0432.CCR-21-4256
9. Piha-Paul SA, Geva R, Tan TJ, Lim DW, Hierro C, Doi T, et al. First-in-human phase I/Ib open-label dose-escalation study of GWN323 (anti-GITR) as a single agent and in combination with spartalizumab (anti-PD-1) in patients with advanced solid tumors and lymphomas. J immunother Cancer (2021) 9(8):e002863. doi: 10.1136/jitc-2021-002863
10. Davar D, Zappasodi R, Wang H, Naik GS, Sato T, Bauer T, et al. Phase IB study of GITR agonist antibody TRX518 singly and in combination with gemcitabine, pembrolizumab, or nivolumab in patients with advanced solid tumors. Clin Cancer Res (2022) 28(18):3990–4002. doi: 10.1158/1078-0432.CCR-22-0339
11. Bajor DL, Mick R, Riese MJ, Huang AC, Sullivan B, Richman LP, et al. Long-term outcomes of a phase I study of agonist CD40 antibody and CTLA-4 blockade in patients with metastatic melanoma. Oncoimmunology (2018) 7(10):e1468956. doi: 10.1080/2162402X.2018.1468956
12. Segal NH, Logan TF, Hodi FS, McDermott D, Melero I, Hamid O, et al. Results from an integrated safety analysis of urelumab, an agonist anti-CD137 monoclonal antibody. Clin Cancer Res (2017) 23(8):1929–36. doi: 10.1158/1078-0432.CCR-16-1272
13. Segal NH, He AR, Doi T, Levy R, Bhatia S, Pishvaian MJ, et al. Phase I study of single-agent utomilumab (PF-05082566), a 4-1BB/CD137 agonist, in patients with advanced cancer. Clin Cancer Res (2018) 24(8):1816–23. doi: 10.1158/1078-0432.CCR-17-1922
14. Davis EJ, Martin-Liberal J, Kristeleit R, Cho DC, Blagden SP, Berthold D, et al. First-in-human phase I/II, open-label study of the anti-OX40 agonist INCAGN01949 in patients with advanced solid tumors. J immunother Cancer (2022) 10(10):e004235. doi: 10.1136/jitc-2021-004235
15. Awad MM, Gadgeel SM, Borghaei H, Patnaik A, Yang JC, Powell SF, et al. Long-term overall survival from KEYNOTE-021 cohort G: pemetrexed and carboplatin with or without pembrolizumab as first-line therapy for advanced nonsquamous NSCLC. J Thorac Oncol (2021) 16(1):162–8. doi: 10.1016/j.jtho.2020.09.015
16. Gadgeel S, Rodríguez-Abreu D, Speranza G, Esteban E, Felip E, Dómine M, et al. Updated analysis from KEYNOTE-189: pembrolizumab or placebo plus pemetrexed and platinum for previously untreated metastatic nonsquamous non-small-cell lung cancer. J Clin Oncol (2020) 38(14):1505–17. doi: 10.1200/JCO.19.03136
17. Powell SF, Rodríguez-Abreu D, Langer CJ, Tafreshi A, Paz-Ares L, Kopp HG, et al. Outcomes with pembrolizumab plus platinum-based chemotherapy for patients with NSCLC and stable brain metastases: pooled analysis of KEYNOTE-021, -189, and -407. J Thorac Oncol (2021) 16(11):1883–92. doi: 10.1016/j.jtho.2021.06.020
18. Moore KN, Bookman M, Sehouli J, Miller A, Anderson C, Scambia G, et al. Atezolizumab, bevacizumab, and chemotherapy for newly diagnosed stage III or IV ovarian cancer: placebo-controlled randomized phase III trial (IMagyn050/GOG 3015/ENGOT-OV39). J Clin Oncol (2021) 39(17):1842–55. doi: 10.1200/JCO.21.00306
19. Takahashi T, Tateishi A, Bychkov A, Fukuoka J. Remarkable alteration of PD-L1 expression after immune checkpoint therapy in patients with non-small-cell lung cancer: two autopsy case reports. Int J Mol Sci (2019) 20(10):2578. doi: 10.3390/ijms20102578
20. Choe EA, Cha YJ, Kim JH, Pyo KH, Hong MH, Park SY, et al. Dynamic changes in PD-L1 expression and CD8(+) T cell infiltration in non-small cell lung cancer following chemoradiation therapy. Lung Cancer (Amsterdam Netherlands) (2019) 136:30–6. doi: 10.1016/j.lungcan.2019.07.027
21. Lacour M, Hiltbrunner S, Lee SY, Soltermann A, Rushing EJ, Soldini D, et al. Adjuvant chemotherapy increases programmed death-ligand 1 (PD-L1) expression in non-small cell lung cancer recurrence. Clin Lung cancer (2019) 20(5):391–6. doi: 10.1016/j.cllc.2019.05.013
22. Fournel L, Wu Z, Stadler N, Damotte D, Lococo F, Boulle G, et al. Cisplatin increases PD-L1 expression and optimizes immune check-point blockade in non-small cell lung cancer. Cancer letters (2019) 464:5–14. doi: 10.1016/j.canlet.2019.08.005
23. Tumeh PC, Harview CL, Yearley JH, Shintaku IP, Taylor EJ, Robert L, et al. PD-1 blockade induces responses by inhibiting adaptive immune resistance. Nature (2014) 515(7528):568–71. doi: 10.1038/nature13954
24. Pan D, Kobayashi A, Jiang P, Ferrari de Andrade L, Tay RE, Luoma AM, et al. A major chromatin regulator determines resistance of tumor cells to T cell-mediated killing. Sci (New York NY) (2018) 359(6377):770–5. doi: 10.1126/science.aao1710
25. Miao D, Margolis CA, Vokes NI, Liu D, Taylor-Weiner A, Wankowicz SM, et al. Genomic correlates of response to immune checkpoint blockade in microsatellite-stable solid tumors. Nat Genet (2018) 50(9):1271–81. doi: 10.1038/s41588-018-0200-2
26. Davoli T, Uno H, Wooten EC, Elledge SJ. Tumor aneuploidy correlates with markers of immune evasion and with reduced response to immunotherapy. Sci (New York NY) (2017) 355(6322):eaaf8399. doi: 10.1126/science.aaf8399
27. Daud AI, Wolchok JD, Robert C, Hwu WJ, Weber JS, Ribas A, et al. Programmed death-ligand 1 expression and response to the anti-programmed death 1 antibody pembrolizumab in melanoma. J Clin Oncol (2016) 34(34):4102–9. doi: 10.1200/JCO.2016.67.2477
28. Fox AH, Nishino M, Osarogiagbon RU, Rivera MP, Rosenthal LS, Smith RA, et al. Acquiring tissue for advanced lung cancer diagnosis and comprehensive biomarker testing: A National Lung Cancer Roundtable best-practice guide. CA: Cancer J Clin (2023) 73(4):358–75. doi: 10.3322/caac.21774
29. Amir E, Miller N, Geddie W, Freedman O, Kassam F, Simmons C, et al. Prospective study evaluating the impact of tissue confirmation of metastatic disease in patients with breast cancer. J Clin Oncol (2012) 30(6):587–92. doi: 10.1200/JCO.2010.33.5232
31. Mocan T, Simão AL, Castro RE, Rodrigues CMP, Słomka A, Wang B, et al. Liquid biopsies in hepatocellular carcinoma: are we winning? J Clin Med (2020) 9(5):1541. doi: 10.3390/jcm9051541
32. Gerlinger M, Rowan AJ, Horswell S, Math M, Larkin J, Endesfelder D, et al. Intratumor heterogeneity and branched evolution revealed by multiregion sequencing. New Engl J Med (2012) 366(10):883–92. doi: 10.1056/NEJMoa1113205
33. Brahmer J, Reckamp KL, Baas P, Crinò L, Eberhardt WE, Poddubskaya E, et al. Nivolumab versus docetaxel in advanced squamous-cell non-small-cell lung cancer. New Engl J Med (2015) 373(2):123–35. doi: 10.1056/NEJMoa1504627
34. Herbst RS, Giaccone G, de Marinis F, Reinmuth N, Vergnenegre A, Barrios CH, et al. Atezolizumab for first-line treatment of PD-L1-selected patients with NSCLC. New Engl J Med (2020) 383(14):1328–39. doi: 10.1056/NEJMoa1917346
35. Aguilar EJ, Ricciuti B, Gainor JF, Kehl KL, Kravets S, Dahlberg S, et al. Outcomes to first-line pembrolizumab in patients with non-small-cell lung cancer and very high PD-L1 expression. Ann Oncol (2019) 30(10):1653–9. doi: 10.1093/annonc/mdz288
36. Jassem J, de Marinis F, Giaccone G, Vergnenegre A, Barrios CH, Morise M, et al. Updated overall survival analysis from IMpower110: atezolizumab versus platinum-based chemotherapy in treatment-naive programmed death-ligand 1-selected NSCLC. J Thorac Oncol (2021) 16(11):1872–82. doi: 10.1016/j.jtho.2021.06.019
37. Fintelmann FJ, Troschel FM, Kuklinski MW, McDermott S, Petranovic M, Digumarthy SR, et al. Safety and success of repeat lung needle biopsies in patients with epidermal growth factor receptor-mutant lung cancer. oncologist (2019) 24(12):1570–6. doi: 10.1634/theoncologist.2019-0158
38. Gregg JP, Li T, Yoneda KY. Molecular testing strategies in non-small cell lung cancer: optimizing the diagnostic journey. Trans Lung Cancer Res (2019) 8(3):286–301. doi: 10.21037/tlcr.2019.04.14
39. Ilie M, Long-Mira E, Bence C, Butori C, Lassalle S, Bouhlel L, et al. Comparative study of the PD-L1 status between surgically resected specimens and matched biopsies of NSCLC patients reveal major discordances: a potential issue for anti-PD-L1 therapeutic strategies. Ann Oncol (2016) 27(1):147–53. doi: 10.1093/annonc/mdv489
40. Munari E, Zamboni G, Lunardi G, Marchionni L, Marconi M, Sommaggio M, et al. PD-L1 expression heterogeneity in non-small cell lung cancer: defining criteria for harmonization between biopsy specimens and whole sections. J Thorac Oncol (2018) 13(8):1113–20. doi: 10.1016/j.jtho.2018.04.017
41. Xu H, Chen X, Lin D, Zhang J, Li C, Zhang D, et al. Conformance assessment of PD-L1 expression between primary tumour and nodal metastases in non-small-cell lung cancer. OncoTargets Ther (2019) 12:11541–7. doi: 10.2147/OTT.S223643
42. Haragan A, Field JK, Davies MPA, Escriu C, Gruver A, Gosney JR. Heterogeneity of PD-L1 expression in non-small cell lung cancer: Implications for specimen sampling in predicting treatment response. Lung Cancer (Amsterdam Netherlands) (2019) 134:79–84. doi: 10.1016/j.lungcan.2019.06.005
43. Licata L, Mariani M, Rossari F, Viale G, Notini G, Naldini MM, et al. Tissue- and liquid biopsy-based biomarkers for immunotherapy in breast cancer. Breast (Edinburgh Scotland) (2023) 69:330–41. doi: 10.1016/j.breast.2023.03.014
44. McKean WB, Moser JC, Rimm D, Hu-Lieskovan S. Biomarkers in precision cancer immunotherapy: promise and challenges. Am Soc Clin Oncol Educ book Am Soc Clin Oncol Annu Meeting (2020) 40:e275–e91. doi: 10.1200/EDBK_280571
45. Quandt D, Dieter Zucht H, Amann A, Wulf-Goldenberg A, Borrebaeck C, Cannarile M, et al. Implementing liquid biopsies into clinical decision making for cancer immunotherapy. Oncotarget (2017) 8(29):48507–20. doi: 10.18632/oncotarget.17397
46. Jamal-Hanjani M, Quezada SA, Larkin J, Swanton C. Translational implications of tumor heterogeneity. Clin Cancer Res (2015) 21(6):1258–66. doi: 10.1158/1078-0432.CCR-14-1429
47. Yost KE, Satpathy AT, Wells DK, Qi Y, Wang C, Kageyama R, et al. Clonal replacement of tumor-specific T cells following PD-1 blockade. Nat Med (2019) 25(8):1251–9. doi: 10.1038/s41591-019-0522-3
48. Wu TD, Madireddi S, de Almeida PE, Banchereau R, Chen YJ, Chitre AS, et al. Peripheral T cell expansion predicts tumour infiltration and clinical response. Nature (2020) 579(7798):274–8. doi: 10.1038/s41586-020-2056-8
49. Sade-Feldman M, Yizhak K, Bjorgaard SL, Ray JP, de Boer CG, Jenkins RW, et al. Defining T cell states associated with response to checkpoint immunotherapy in melanoma. Cell (2018) 175(4):998–1013 e20. doi: 10.1016/j.cell.2018.10.038
50. Liu B, Hu X, Feng K, Gao R, Xue Z, Zhang S, et al. Temporal single-cell tracing reveals clonal revival and expansion of precursor exhausted T cells during anti-PD-1 therapy in lung cancer. Nat cancer (2022) 3(1):108–21. doi: 10.1038/s43018-021-00292-8
51. Clarke J, Panwar B, Madrigal A, Singh D, Gujar R, Wood O, et al. Single-cell transcriptomic analysis of tissue-resident memory T cells in human lung cancer. J Exp Med (2019) 216(9):2128–49. doi: 10.1084/jem.20190249
52. Corgnac S, Malenica I, Mezquita L, Auclin E, Voilin E, Kacher J, et al. CD103(+)CD8(+) T(RM) cells accumulate in tumors of anti-PD-1-responder lung cancer patients and are tumor-reactive lymphocytes enriched with tc17. Cell Rep Med (2020) 1(7):100127. doi: 10.1016/j.xcrm.2020.100127
53. Luoma AM, Suo S, Wang Y, Gunasti L, Porter CBM, Nabilsi N, et al. Tissue-resident memory and circulating T cells are early responders to pre-surgical cancer immunotherapy. Cell (2022) 185(16):2918–35.e29. doi: 10.1016/j.cell.2022.06.018
54. Palucka AK, Coussens LM. The basis of oncoimmunology. Cell (2016) 164(6):1233–47. doi: 10.1016/j.cell.2016.01.049
55. Pol J, Bloy N, Buqué A, Eggermont A, Cremer I, Sautès-Fridman C, et al. Trial Watch: Peptide-based anticancer vaccines. Oncoimmunology (2015) 4(4):e974411. doi: 10.4161/2162402X.2014.974411
56. Postow MA, Callahan MK, Wolchok JD. Immune checkpoint blockade in cancer therapy. J Clin Oncol (2015) 33(17):1974–82. doi: 10.1200/JCO.2014.59.4358
57. Schneider H, Downey J, Smith A, Zinselmeyer BH, Rush C, Brewer JM, et al. Reversal of the TCR stop signal by CTLA-4. Sci (New York NY) (2006) 313(5795):1972–5. doi: 10.1126/science.1131078
58. Riley JL, Mao M, Kobayashi S, Biery M, Burchard J, Cavet G, et al. Modulation of TCR-induced transcriptional profiles by ligation of CD28, ICOS, and CTLA-4 receptors. Proc Natl Acad Sci United States America (2002) 99(18):11790–5. doi: 10.1073/pnas.162359999
59. Qureshi OS, Zheng Y, Nakamura K, Attridge K, Manzotti C, Schmidt EM, et al. Trans-endocytosis of CD80 and CD86: a molecular basis for the cell-extrinsic function of CTLA-4. Sci (New York NY) (2011) 332(6029):600–3. doi: 10.1126/science.1202947
60. Krummel MF, Allison JP. CTLA-4 engagement inhibits IL-2 accumulation and cell cycle progression upon activation of resting T cells. J Exp Med (1996) 183(6):2533–40. doi: 10.1084/jem.183.6.2533
61. Hodi FS, Butler M, Oble DA, Seiden MV, Haluska FG, Kruse A, et al. Immunologic and clinical effects of antibody blockade of cytotoxic T lymphocyte-associated antigen 4 in previously vaccinated cancer patients. Proc Natl Acad Sci United States America (2008) 105(8):3005–10. doi: 10.1073/pnas.0712237105
62. Phan GQ, Yang JC, Sherry RM, Hwu P, Topalian SL, Schwartzentruber DJ, et al. Cancer regression and autoimmunity induced by cytotoxic T lymphocyte-associated antigen 4 blockade in patients with metastatic melanoma. Proc Natl Acad Sci United States America (2003) 100(14):8372–7. doi: 10.1073/pnas.1533209100
63. Ribas A, Camacho LH, Lopez-Berestein G, Pavlov D, Bulanhagui CA, Millham R, et al. Antitumor activity in melanoma and anti-self responses in a phase I trial with the anti-cytotoxic T lymphocyte-associated antigen 4 monoclonal antibody CP-675,206. J Clin Oncol (2005) 23(35):8968–77. doi: 10.1200/JCO.2005.01.109
64. Dong H, Strome SE, Salomao DR, Tamura H, Hirano F, Flies DB, et al. Tumor-associated B7-H1 promotes T-cell apoptosis: a potential mechanism of immune evasion. Nat Med (2002) 8(8):793–800. doi: 10.1038/nm730
65. Ishida Y, Agata Y, Shibahara K, Honjo T. Induced expression of PD-1, a novel member of the immunoglobulin gene superfamily, upon programmed cell death. EMBO J (1992) 11(11):3887–95. doi: 10.1002/j.1460-2075.1992.tb05481.x
66. Freeman GJ, Long AJ, Iwai Y, Bourque K, Chernova T, Nishimura H, et al. Engagement of the PD-1 immunoinhibitory receptor by a novel B7 family member leads to negative regulation of lymphocyte activation. J Exp Med (2000) 192(7):1027–34. doi: 10.1084/jem.192.7.1027
67. Keir ME, Liang SC, Guleria I, Latchman YE, Qipo A, Albacker LA, et al. Tissue expression of PD-L1 mediates peripheral T cell tolerance. J Exp Med (2006) 203(4):883–95. doi: 10.1084/jem.20051776
68. Topalian SL, Hodi FS, Brahmer JR, Gettinger SN, Smith DC, McDermott DF, et al. Safety, activity, and immune correlates of anti-PD-1 antibody in cancer. New Engl J Med (2012) 366(26):2443–54. doi: 10.1056/NEJMoa1200690
69. Hamid O, Robert C, Daud A, Hodi FS, Hwu WJ, Kefford R, et al. Safety and tumor responses with lambrolizumab (anti-PD-1) in melanoma. New Engl J Med (2013) 369(2):134–44. doi: 10.1056/NEJMoa1305133
70. Topalian SL, Sznol M, McDermott DF, Kluger HM, Carvajal RD, Sharfman WH, et al. Survival, durable tumor remission, and long-term safety in patients with advanced melanoma receiving nivolumab. J Clin Oncol (2014) 32(10):1020–30. doi: 10.1200/JCO.2013.53.0105
71. Brahmer JR, Tykodi SS, Chow LQM, Hwu W-J, Topalian SL, Hwu P, et al. Safety and activity of anti–PD-L1 antibody in patients with advanced cancer. N Engl J Med (2012) 366(26):2455–65. doi: 10.1056/NEJMoa1200694
72. Le DT, Uram JN, Wang H, Bartlett BR, Kemberling H, Eyring AD, et al. PD-1 blockade in tumors with mismatch-repair deficiency. New Engl J Med (2015) 372(26):2509–20. doi: 10.1056/NEJMoa1500596
73. Marabelle A, Le DT, Ascierto PA, Di Giacomo AM, De Jesus-Acosta A, Delord JP, et al. Efficacy of pembrolizumab in patients with noncolorectal high microsatellite instability/mismatch repair-deficient cancer: results from the phase II KEYNOTE-158 study. J Clin Oncol (2020) 38(1):1–10. doi: 10.1200/JCO.19.02105
74. Marabelle A, Fakih M, Lopez J, Shah M, Shapira-Frommer R, Nakagawa K, et al. Association of tumour mutational burden with outcomes in patients with advanced solid tumours treated with pembrolizumab: prospective biomarker analysis of the multicohort, open-label, phase 2 KEYNOTE-158 study. Lancet Oncol (2020) 21(10):1353–65. doi: 10.1016/S1470-2045(20)30445-9
75. Matulonis UA, Shapira-Frommer R, Santin AD, Lisyanskaya AS, Pignata S, Vergote I, et al. Antitumor activity and safety of pembrolizumab in patients with advanced recurrent ovarian cancer: results from the phase II KEYNOTE-100 study. Ann Oncol (2019) 30(7):1080–7. doi: 10.1093/annonc/mdz135
76. Monk BJ, Colombo N, Oza AM, Fujiwara K, Birrer MJ, Randall L, et al. Chemotherapy with or without avelumab followed by avelumab maintenance versus chemotherapy alone in patients with previously untreated epithelial ovarian cancer (JAVELIN Ovarian 100): an open-label, randomised, phase 3 trial. Lancet Oncol (2021) 22(9):1275–89. doi: 10.1016/S1470-2045(21)00342-9
77. Zhang L, Conejo-Garcia JR, Katsaros D, Gimotty PA, Massobrio M, Regnani G, et al. Intratumoral T cells, recurrence, and survival in epithelial ovarian cancer. New Engl J Med (2003) 348(3):203–13. doi: 10.1056/NEJMoa020177
78. Fucikova J, Rakova J, Hensler M, Kasikova L, Belicova L, Hladikova K, et al. TIM-3 dictates functional orientation of the immune infiltrate in ovarian cancer. Clin Cancer Res (2019) 25(15):4820–31. doi: 10.1158/1078-0432.CCR-18-4175
79. Yang B, Li X, Zhang W, Fan J, Zhou Y, Li W, et al. Spatial heterogeneity of infiltrating T cells in high-grade serous ovarian cancer revealed by multi-omics analysis. Cell Rep Med (2022) 3(12):100856. doi: 10.1016/j.xcrm.2022.100856
80. Okazaki K, Davis DD, Sakano H. T cell receptor beta gene sequences in the circular DNA of thymocyte nuclei: direct evidence for intramolecular DNA deletion in V-D-J joining. Cell (1987) 49(4):477–85. doi: 10.1016/0092-8674(87)90450-8
81. Chien YH, Gascoigne NR, Kavaler J, Lee NE, Davis MM. Somatic recombination in a murine T-cell receptor gene. Nature (1984) 309(5966):322–6. doi: 10.1038/309322a0
82. Freeman JD, Warren RL, Webb JR, Nelson BH, Holt RA. Profiling the T-cell receptor beta-chain repertoire by massively parallel sequencing. Genome Res (2009) 19(10):1817–24. doi: 10.1101/gr.092924.109
83. Redmond D, Poran A, Elemento O. Single-cell TCRseq: paired recovery of entire T-cell alpha and beta chain transcripts in T-cell receptors from single-cell RNAseq. Genome Med (2016) 8(1):80. doi: 10.1186/s13073-016-0335-7
84. De Simone M, Rossetti G, Pagani M. Single cell T cell receptor sequencing: techniques and future challenges. Front Immunol (2018) 9:1638. doi: 10.3389/fimmu.2018.01638
85. Robins HS, Campregher PV, Srivastava SK, Wacher A, Turtle CJ, Kahsai O, et al. Comprehensive assessment of T-cell receptor beta-chain diversity in alphabeta T cells. Blood (2009) 114(19):4099–107. doi: 10.1182/blood-2009-04-217604
86. Valpione S, Mundra PA, Galvani E, Campana LG, Lorigan P, De Rosa F, et al. The T cell receptor repertoire of tumor infiltrating T cells is predictive and prognostic for cancer survival. Nat Commun (2021) 12(1):4098. doi: 10.1038/s41467-021-24343-x
87. Zehn D, Thimme R, Lugli E, de Almeida GP, Oxenius A. 'Stem-like' precursors are the fount to sustain persistent CD8(+) T cell responses. Nat Immunol (2022) 23(6):836–47. doi: 10.1038/s41590-022-01219-w
88. Blank C, Brown I, Peterson AC, Spiotto M, Iwai Y, Honjo T, et al. PD-L1/B7H-1 inhibits the effector phase of tumor rejection by T cell receptor (TCR) transgenic CD8+ T cells. Cancer Res (2004) 64(3):1140–5. doi: 10.1158/0008-5472.CAN-03-3259
89. Arneth B. Tumor microenvironment. Medicina (Kaunas Lithuania) (2019) 56(1):15. doi: 10.3390/medicina56010015
90. Binnewies M, Roberts EW, Kersten K, Chan V, Fearon DF, Merad M, et al. Understanding the tumor immune microenvironment (TIME) for effective therapy. Nat Med (2018) 24(5):541–50. doi: 10.1038/s41591-018-0014-x
91. Simoni Y, Becht E, Fehlings M, Loh CY, Koo SL, Teng KWW, et al. Bystander CD8(+) T cells are abundant and phenotypically distinct in human tumour infiltrates. Nature (2018) 557(7706):575–9. doi: 10.1038/s41586-018-0130-2
92. Duhen T, Duhen R, Montler R, Moses J, Moudgil T, de Miranda NF, et al. Co-expression of CD39 and CD103 identifies tumor-reactive CD8 T cells in human solid tumors. Nat Commun (2018) 9(1):2724. doi: 10.1038/s41467-018-05072-0
93. Meier SL, Satpathy AT, Wells DK. Bystander T cells in cancer immunology and therapy. Nat cancer (2022) 3(2):143–55. doi: 10.1038/s43018-022-00335-8
94. Scheper W, Kelderman S, Fanchi LF, Linnemann C, Bendle G, de Rooij MAJ, et al. Low and variable tumor reactivity of the intratumoral TCR repertoire in human cancers. Nat Med (2019) 25(1):89–94. doi: 10.1038/s41591-018-0266-5
95. Tirosh I, Izar B, Prakadan SM, Wadsworth MH 2nd, Treacy D, Trombetta JJ, et al. Dissecting the multicellular ecosystem of metastatic melanoma by single-cell RNA-seq. Sci (New York NY) (2016) 352(6282):189–96. doi: 10.1126/science.aad0501
96. Li H, van der Leun AM, Yofe I, Lubling Y, Gelbard-Solodkin D, van Akkooi ACJ, et al. Dysfunctional CD8 T cells form a proliferative, dynamically regulated compartment within human melanoma. Cell (2019) 176(4):775–89.e18. doi: 10.1016/j.cell.2018.11.043
97. Guo X, Zhang Y, Zheng L, Zheng C, Song J, Zhang Q, et al. Global characterization of T cells in non-small-cell lung cancer by single-cell sequencing. Nat Med (2018) 24(7):978–85. doi: 10.1038/s41591-018-0045-3
98. Kok L, Masopust D, Schumacher TN. The precursors of CD8(+) tissue resident memory T cells: from lymphoid organs to infected tissues. Nat Rev Immunol (2022) 22(5):283–93. doi: 10.1038/s41577-021-00590-3
99. Zhang L, Yu X, Zheng L, Zhang Y, Li Y, Fang Q, et al. Lineage tracking reveals dynamic relationships of T cells in colorectal cancer. Nature (2018) 564(7735):268–72. doi: 10.1038/s41586-018-0694-x
100. Zheng C, Zheng L, Yoo JK, Guo H, Zhang Y, Guo X, et al. Landscape of infiltrating T cells in liver cancer revealed by single-cell sequencing. Cell (2017) 169(7):1342–56.e16. doi: 10.1016/j.cell.2017.05.035
101. Walsh DA, Borges da Silva H, Beura LK, Peng C, Hamilton SE, Masopust D, et al. The functional requirement for CD69 in establishment of resident memory CD8(+) T cells varies with tissue location. J Immunol (Baltimore Md 1950) (2019) 203(4):946–55. doi: 10.4049/jimmunol.1900052
102. Nose Y, Saito T, Yamamoto K, Yamashita K, Tanaka K, Yamamoto K, et al. The tissue-resident marker CD103 on peripheral blood T cells predicts responses to anti-PD-1 therapy in gastric cancer. Cancer immunol immunother CII (2023) 72(1):169–81. doi: 10.1007/s00262-022-03240-2
103. Savas P, Virassamy B, Ye C, Salim A, Mintoff CP, Caramia F, et al. Single-cell profiling of breast cancer T cells reveals a tissue-resident memory subset associated with improved prognosis. Nat Med (2018) 24(7):986–93. doi: 10.1038/s41591-018-0078-7
104. Thommen DS, Koelzer VH, Herzig P, Roller A, Trefny M, Dimeloe S, et al. A transcriptionally and functionally distinct PD-1(+) CD8(+) T cell pool with predictive potential in non-small-cell lung cancer treated with PD-1 blockade. Nat Med (2018) 24(7):994–1004. doi: 10.1038/s41591-018-0057-z
105. Baitsch L, Baumgaertner P, Devêvre E, Raghav SK, Legat A, Barba L, et al. Exhaustion of tumor-specific CD8+ T cells in metastases from melanoma patients. J Clin Invest (2011) 121(6):2350–60. doi: 10.1172/JCI46102
106. Blackburn SD, Shin H, Freeman GJ, Wherry EJ. Selective expansion of a subset of exhausted CD8 T cells by alphaPD-L1 blockade. Proc Natl Acad Sci United States America (2008) 105(39):15016–21. doi: 10.1073/pnas.0801497105
107. Gupta PK, Godec J, Wolski D, Adland E, Yates K, Pauken KE, et al. CD39 expression identifies terminally exhausted CD8+ T cells. PloS pathogens (2015) 11(10):e1005177. doi: 10.1371/journal.ppat.1005177
108. Alfei F, Kanev K, Hofmann M, Wu M, Ghoneim HE, Roelli P, et al. TOX reinforces the phenotype and longevity of exhausted T cells in chronic viral infection. Nature (2019) 571(7764):265–9. doi: 10.1038/s41586-019-1326-9
109. Scott AC, Dündar F, Zumbo P, Chandran SS, Klebanoff CA, Shakiba M, et al. TOX is a critical regulator of tumour-specific T cell differentiation. Nature (2019) 571(7764):270–4. doi: 10.1038/s41586-019-1324-y
110. Seo H, Chen J, González-Avalos E, Samaniego-Castruita D, Das A, Wang YH, et al. TOX and TOX2 transcription factors cooperate with NR4A transcription factors to impose CD8(+) T cell exhaustion. Proc Natl Acad Sci United States America (2019) 116(25):12410–5. doi: 10.1073/pnas.1905675116
111. Yao C, Sun HW, Lacey NE, Ji Y, Moseman EA, Shih HY, et al. Single-cell RNA-seq reveals TOX as a key regulator of CD8(+) T cell persistence in chronic infection. Nat Immunol (2019) 20(7):890–901. doi: 10.1038/s41590-019-0403-4
112. Aliahmad P, Seksenyan A, Kaye J. The many roles of TOX in the immune system. Curr Opin Immunol (2012) 24(2):173–7. doi: 10.1016/j.coi.2011.12.001
113. Khan O, Giles JR, McDonald S, Manne S, Ngiow SF, Patel KP, et al. TOX transcriptionally and epigenetically programs CD8(+) T cell exhaustion. Nature (2019) 571(7764):211–8. doi: 10.1038/s41586-019-1325-x
114. Xia C, Yin S, To KKW, Fu L. CD39/CD73/A2AR pathway and cancer immunotherapy. Mol cancer (2023) 22(1):44. doi: 10.1186/s12943-023-01733-x
115. Hay CM, Sult E, Huang Q, Mulgrew K, Fuhrmann SR, McGlinchey KA, et al. Targeting CD73 in the tumor microenvironment with MEDI9447. Oncoimmunology (2016) 5(8):e1208875. doi: 10.1080/2162402X.2016.1208875
116. Lawson KV, Kalisiak J, Lindsey EA, Newcomb ET, Leleti MR, Debien L, et al. Discovery of AB680: A potent and selective inhibitor of CD73. J medicinal Chem (2020) 63(20):11448–68. doi: 10.1021/acs.jmedchem.0c00525
117. Li XY, Moesta AK, Xiao C, Nakamura K, Casey M, Zhang H, et al. Targeting CD39 in cancer reveals an extracellular ATP- and inflammasome-driven tumor immunity. Cancer discovery (2019) 9(12):1754–73. doi: 10.1158/2159-8290.CD-19-0541
118. Wherry EJ, Kurachi M. Molecular and cellular insights into T cell exhaustion. Nat Rev Immunol (2015) 15(8):486–99. doi: 10.1038/nri3862
119. Fooksman DR, Vardhana S, Vasiliver-Shamis G, Liese J, Blair DA, Waite J, et al. Functional anatomy of T cell activation and synapse formation. Annu Rev Immunol (2010) 28:79–105. doi: 10.1146/annurev-immunol-030409-101308
120. Oliveira G, Stromhaug K, Klaeger S, Kula T, Frederick DT, Le PM, et al. Phenotype, specificity and avidity of antitumour CD8(+) T cells in melanoma. Nature (2021) 596(7870):119–25. doi: 10.1038/s41586-021-03704-y
121. Fehlings M, Simoni Y, Penny HL, Becht E, Loh CY, Gubin MM, et al. Checkpoint blockade immunotherapy reshapes the high-dimensional phenotypic heterogeneity of murine intratumoural neoantigen-specific CD8(+) T cells. Nat Commun (2017) 8(1):562. doi: 10.1038/s41467-017-00627-z
122. Chevrier S, Levine JH, Zanotelli VRT, Silina K, Schulz D, Bacac M, et al. An immune atlas of clear cell renal cell carcinoma. Cell (2017) 169(4):736–49.e18. doi: 10.1016/j.cell.2017.04.016
123. Wagner J, Rapsomaniki MA, Chevrier S, Anzeneder T, Langwieder C, Dykgers A, et al. A single-cell atlas of the tumor and immune ecosystem of human breast cancer. Cell (2019) 177(5):1330–45.e18. doi: 10.1016/j.cell.2019.03.005
124. McLane LM, Abdel-Hakeem MS, Wherry EJ. CD8 T cell exhaustion during chronic viral infection and cancer. Annu Rev Immunol (2019) 37:457–95. doi: 10.1146/annurev-immunol-041015-055318
125. Paley MA, Kroy DC, Odorizzi PM, Johnnidis JB, Dolfi DV, Barnett BE, et al. Progenitor and terminal subsets of CD8+ T cells cooperate to contain chronic viral infection. Sci (New York NY) (2012) 338(6111):1220–5. doi: 10.1126/science.1229620
126. Im SJ, Hashimoto M, Gerner MY, Lee J, Kissick HT, Burger MC, et al. Defining CD8+ T cells that provide the proliferative burst after PD-1 therapy. Nature (2016) 537(7620):417–21. doi: 10.1038/nature19330
127. Utzschneider DT, Charmoy M, Chennupati V, Pousse L, Ferreira DP, Calderon-Copete S, et al. T cell factor 1-expressing memory-like CD8(+) T cells sustain the immune response to chronic viral infections. Immunity (2016) 45(2):415–27. doi: 10.1016/j.immuni.2016.07.021
128. He R, Hou S, Liu C, Zhang A, Bai Q, Han M, et al. Follicular CXCR5- expressing CD8(+) T cells curtail chronic viral infection. Nature (2016) 537(7620):412–28. doi: 10.1038/nature19317
129. Sakuishi K, Apetoh L, Sullivan JM, Blazar BR, Kuchroo VK, Anderson AC. Targeting Tim-3 and PD-1 pathways to reverse T cell exhaustion and restore anti-tumor immunity. J Exp Med (2010) 207(10):2187–94. doi: 10.1084/jem.20100643
130. Schietinger A, Philip M, Krisnawan VE, Chiu EY, Delrow JJ, Basom RS, et al. Tumor-specific T cell dysfunction is a dynamic antigen-driven differentiation program initiated early during tumorigenesis. Immunity (2016) 45(2):389–401. doi: 10.1016/j.immuni.2016.07.011
131. Utzschneider DT, Legat A, Fuertes Marraco SA, Carrié L, Luescher I, Speiser DE, et al. T cells maintain an exhausted phenotype after antigen withdrawal and population reexpansion. Nat Immunol (2013) 14(6):603–10. doi: 10.1038/ni.2606
132. Han HS, Jeong S, Kim H, Kim HD, Kim AR, Kwon M, et al. TOX-expressing terminally exhausted tumor-infiltrating CD8(+) T cells are reinvigorated by co-blockade of PD-1 and TIGIT in bladder cancer. Cancer letters (2021) 499:137–47. doi: 10.1016/j.canlet.2020.11.035
133. Ghoneim HE, Fan Y, Moustaki A, Abdelsamed HA, Dash P, Dogra P, et al. De novo epigenetic programs inhibit PD-1 blockade-mediated T cell rejuvenation. Cell (2017) 170(1):142–57.e19. doi: 10.1016/j.cell.2017.06.007
134. Wang X, He Q, Shen H, Xia A, Tian W, Yu W, et al. TOX promotes the exhaustion of antitumor CD8(+) T cells by preventing PD1 degradation in hepatocellular carcinoma. J hepatol (2019) 71(4):731–41. doi: 10.1016/j.jhep.2019.05.015
135. Qorraj M, Bruns H, Böttcher M, Weigand L, Saul D, Mackensen A, et al. The PD-1/PD-L1 axis contributes to immune metabolic dysfunctions of monocytes in chronic lymphocytic leukemia. Leukemia (2017) 31(2):470–8. doi: 10.1038/leu.2016.214
136. Ho PC, Bihuniak JD, Macintyre AN, Staron M, Liu X, Amezquita R, et al. Phosphoenolpyruvate is a metabolic checkpoint of anti-tumor T cell responses. Cell (2015) 162(6):1217–28. doi: 10.1016/j.cell.2015.08.012
137. Scharping NE, Menk AV, Whetstone RD, Zeng X, Delgoffe GM. Efficacy of PD-1 blockade is potentiated by metformin-induced reduction of tumor hypoxia. Cancer Immunol Res (2017) 5(1):9–16. doi: 10.1158/2326-6066.CIR-16-0103
138. Caushi JX, Zhang J, Ji Z, Vaghasia A, Zhang B, Hsiue EH, et al. Transcriptional programs of neoantigen-specific TIL in anti-PD-1-treated lung cancers. Nature (2021) 596(7870):126–32. doi: 10.1016/j.celrep.2022.110331
139. Hanada KI, Zhao C, Gil-Hoyos R, Gartner JJ, Chow-Parmer C, Lowery FJ, et al. A phenotypic signature that identifies neoantigen-reactive T cells in fresh human lung cancers. Cancer Cell (2022) 40(5):479–93.e6. doi: 10.1016/j.ccell.2022.03.012
140. Hummelink K, van der Noort V, Muller M, Schouten RD, Lalezari F, Peters D, et al. PD-1T TILs as a predictive biomarker for clinical benefit to PD-1 blockade in patients with advanced NSCLC. Clin Cancer Res (2022) 28(22):4893–906. doi: 10.1158/1078-0432.CCR-22-0992
141. Zhang J, Ji Z, Caushi JX, El Asmar M, Anagnostou V, Cottrell TR, et al. Compartmental analysis of T-cell clonal dynamics as a function of pathologic response to neoadjuvant PD-1 blockade in resectable non-small cell lung cancer. Clin Cancer Res (2020) 26(6):1327–37. doi: 10.1038/s41586-021-03752-4
142. Kamphorst AO, Wieland A, Nasti T, Yang S, Zhang R, Barber DL, et al. Rescue of exhausted CD8 T cells by PD-1-targeted therapies is CD28-dependent. Sci (New York NY) (2017) 355(6332):1423–7. doi: 10.1038/s41590-019-0312-6
143. Kamphorst AO, Pillai RN, Yang S, Nasti TH, Akondy RS, Wieland A, et al. Proliferation of PD-1+ CD8 T cells in peripheral blood after PD-1-targeted therapy in lung cancer patients. Proc Natl Acad Sci United States America (2017) 114(19):4993–8. doi: 10.1038/nature22367
144. Kim KH, Cho J, Ku BM, Koh J, Sun JM, Lee SH, et al. The first-week proliferative response of peripheral blood PD-1(+)CD8(+) T cells predicts the response to anti-PD-1 therapy in solid tumors. Clin Cancer Res (2019) 25(7):2144–54. doi: 10.1073/pnas.1524490113
145. Juliá EP, Mandó P, Rizzo MM, Cueto GR, Tsou F, Luca R, et al. Peripheral changes in immune cell populations and soluble mediators after anti-PD-1 therapy in non-small cell lung cancer and renal cell carcinoma patients. Cancer immunol immunother CII (2019) 68(10):1585–96. doi: 10.1038/nri.2017.146
146. Kim H, Park S, Han KY, Lee N, Kim H, Jung HA, et al. Clonal expansion of resident memory T cells in peripheral blood of patients with non-small cell lung cancer during immune checkpoint inhibitor treatment. J immunother Cancer (2023) 11(2):e005509. doi: 10.1126/science.aaf2807
147. Li Y, Wang J, Wu L, Li X, Zhang X, Zhang G, et al. Diversity of dominant peripheral T cell receptor clone and soluble immune checkpoint proteins associated with clinical outcomes following immune checkpoint inhibitor treatment in advanced cancers. Front Immunol (2021) 12:649343. doi: 10.1126/science.aae0491
148. Han J, Duan J, Bai H, Wang Y, Wan R, Wang X, et al. TCR repertoire diversity of peripheral PD-1(+)CD8(+) T cells predicts clinical outcomes after immunotherapy in patients with non-small cell lung cancer. Cancer Immunol Res (2020) 8(1):146–54. doi: 10.1126/science.abb9847
149. Kim CG, Kim KH, Pyo KH, Xin CF, Hong MH, Ahn BC, et al. Hyperprogressive disease during PD-1/PD-L1 blockade in patients with non-small-cell lung cancer. Ann Oncol (2019) 30(7):1104–13. doi: 10.1038/s41586-019-1836-5
150. Yang X, Yin R, Xu L. Neoadjuvant PD-1 blockade in resectable lung cancer. New Engl J Med (2018) 379(9):e14. doi: 10.1158/2159-8290.CD-21-0219
151. Manjarrez-Orduño N, Menard LC, Kansal S, Fischer P, Kakrecha B, Jiang C, et al. Circulating T cell subpopulations correlate with immune responses at the tumor site and clinical response to PD1 inhibition in non-small cell lung cancer. Front Immunol (2018) 9:1613. doi: 10.1158/2326-6066.CIR-15-0210
152. Miller BC, Sen DR, Al Abosy R, Bi K, Virkud YV, LaFleur MW, et al. Subsets of exhausted CD8(+) T cells differentially mediate tumor control and respond to checkpoint blockade. Nat Immunol (2019) 20(3):326–36. doi: 10.1016/j.cell.2018.12.034
153. Ribas A, Shin DS, Zaretsky J, Frederiksen J, Cornish A, Avramis E, et al. PD-1 blockade expands intratumoral memory T cells. Cancer Immunol Res (2016) 4(3):194–203. doi: 10.1016/j.immuni.2018.12.021
154. Nagasaki J, Inozume T, Sax N, Ariyasu R, Ishikawa M, Yamashita K, et al. PD-1 blockade therapy promotes infiltration of tumor-attacking exhausted T cell clonotypes. Cell Rep (2022) 38(5):110331. doi: 10.1016/j.it.2019.06.002
155. Pauken KE, Shahid O, Lagattuta KA, Mahuron KM, Luber JM, Lowe MM, et al. Single-cell analyses identify circulating anti-tumor CD8 T cells and markers for their enrichment. J Exp Med (2021) 218(4):e20200920. doi: 10.1038/ni.3775
156. Beltra JC, Manne S, Abdel-Hakeem MS, Kurachi M, Giles JR, Chen Z, et al. Developmental relationships of four exhausted CD8(+) T cell subsets reveals underlying transcriptional and epigenetic landscape control mechanisms. Immunity (2020) 52(5):825–41.e8. doi: 10.1126/sciimmunol.abn8390
157. Gros A, Parkhurst MR, Tran E, Pasetto A, Robbins PF, Ilyas S, et al. Prospective identification of neoantigen-specific lymphocytes in the peripheral blood of melanoma patients. Nat Med (2016) 22(4):433–8. doi: 10.1016/j.immuni.2022.12.002
158. Siddiqui I, Schaeuble K, Chennupati V, Fuertes Marraco SA, Calderon-Copete S, Pais Ferreira D, et al. Intratumoral tcf1(+)PD-1(+)CD8(+) T cells with stem-like properties promote tumor control in response to vaccination and checkpoint blockade immunotherapy. Immunity (2019) 50(1):195–211.e10. doi: 10.1016/j.ccell.2020.09.001
159. Krieg C, Nowicka M, Guglietta S, Schindler S, Hartmann FJ, Weber LM, et al. High-dimensional single-cell analysis predicts response to anti-PD-1 immunotherapy. Nat Med (2018) 24(2):144–53. doi: 10.1016/j.cell.2018.07.009
160. Huang AC, Orlowski RJ, Xu X, Mick R, George SM, Yan PK, et al. A single dose of neoadjuvant PD-1 blockade predicts clinical outcomes in resectable melanoma. Nat Med (2019) 25(3):454–61. doi: 10.1084/jem.20200920
161. Huang AC, Postow MA, Orlowski RJ, Mick R, Bengsch B, Manne S, et al. T-cell invigoration to tumour burden ratio associated with anti-PD-1 response. Nature (2017) 545(7652):60–5. doi: 10.1126/science.aaf0683
162. Simon S, Voillet V, Vignard V, Wu Z, Dabrowski C, Jouand N, et al. PD-1 and TIGIT coexpression identifies a circulating CD8 T cell subset predictive of response to anti-PD-1 therapy. J immunother Cancer (2020) 8(2):e001631. doi: 10.1038/nm.4051
163. Valpione S, Galvani E, Tweedy J, Mundra PA, Banyard A, Middlehurst P, et al. Immune-awakening revealed by peripheral T cell dynamics after one cycle of immunotherapy. Nat cancer (2020) 1(2):210–21. doi: 10.1016/j.cell.2023.02.021
164. Watson RA, Tong O, Cooper R, Taylor CA, Sharma PK, de Los Aires AV, et al. Immune checkpoint blockade sensitivity and progression-free survival associates with baseline CD8(+) T cell clone size and cytotoxicity. Sci Immunol (2021) 6(64):eabj8825. doi: 10.1038/nature22079
165. Wistuba-Hamprecht K, Martens A, Heubach F, Romano E, Geukes Foppen M, Yuan J, et al. Peripheral CD8 effector-memory type 1 T-cells correlate with outcome in ipilimumab-treated stage IV melanoma patients. Eur J Cancer (Oxford Engl 1990) (2017) 73:61–70. doi: 10.1038/s41591-019-0357-y
166. Fairfax BP, Taylor CA, Watson RA, Nassiri I, Danielli S, Fang H, et al. Peripheral CD8(+) T cell characteristics associated with durable responses to immune checkpoint blockade in patients with metastatic melanoma. Nat Med (2020) 26(2):193–9. doi: 10.1073/pnas.1705327114
167. Yan Y, Cao S, Liu X, Harrington SM, Bindeman WE, Adjei AA, et al. CX3CR1 identifies PD-1 therapy-responsive CD8+ T cells that withstand chemotherapy during cancer chemoimmunotherapy. JCI Insight (2018) 3(8):e97828. doi: 10.1158/1078-0432.CCR-18-1449
168. de Coaña YP, Wolodarski M, Poschke I, Yoshimoto Y, Yang Y, Nyström M, et al. Ipilimumab treatment decreases monocytic MDSCs and increases CD8 effector memory T cells in long-term survivors with advanced melanoma. Oncotarget (2017) 8(13):21539–53. doi: 10.1038/nm.4466
169. Tietze JK, Angelova D, Heppt MV, Reinholz M, Murphy WJ, Spannagl M, et al. The proportion of circulating CD45RO(+)CD8(+) memory T cells is correlated with clinical response in melanoma patients treated with ipilimumab. Eur J Cancer (Oxford Engl 1990) (2017) 75:268–79. doi: 10.1136/jitc-2020-001631
170. Wang W, Yu D, Sarnaik AA, Yu B, Hall M, Morelli D, et al. Biomarkers on melanoma patient T cells associated with ipilimumab treatment. J Trans Med (2012) 10:146. doi: 10.1016/j.lungcan.2020.07.028
171. Lee YJ, Kim JY, Jeon SH, Nam H, Jung JH, Jeon M, et al. CD39(+) tissue-resident memory CD8(+) T cells with a clonal overlap across compartments mediate antitumor immunity in breast cancer. Sci Immunol (2022) 7(74):eabn8390. doi: 10.1136/jitc-2022-004803
172. Carlisle JW, Jansen CS, Cardenas MA, Sobierajska E, Reyes AM, Greenwald R, et al. Clinical outcome following checkpoint therapy in renal cell carcinoma is associated with a burst of activated CD8 T cells in blood. J immunother Cancer (2022) 10(7):e004803. doi: 10.1007/s00262-019-02391-z
173. Rahim MK, Okholm TLH, Jones KB, McCarthy EE, Liu CC, Yee JL, et al. Dynamic CD8(+) T cell responses to cancer immunotherapy in human regional lymph nodes are disrupted in metastatic lymph nodes. Cell (2023) 186(6):1127–43.e18. doi: 10.1007/s00262-018-2225-x
174. Wang X, Muzaffar J, Kirtane K, Song F, Johnson M, Schell MJ, et al. T cell repertoire in peripheral blood as a potential biomarker for predicting response to concurrent cetuximab and nivolumab in head and neck squamous cell carcinoma. J immunother Cancer (2022) 10(6):e004512. doi: 10.1073/pnas.1918937117
175. Kato R, Yamasaki M, Urakawa S, Nishida K, Makino T, Morimoto-Okazawa A, et al. Increased Tim-3(+) T cells in PBMCs during nivolumab therapy correlate with responses and prognosis of advanced esophageal squamous cell carcinoma patients. Cancer immunol immunother CII (2018) 67(11):1673–83. doi: 10.1126/sciimmunol.abj8825
176. Griffiths JI, Wallet P, Pflieger LT, Stenehjem D, Liu X, Cosgrove PA, et al. Circulating immune cell phenotype dynamics reflect the strength of tumor-immune cell interactions in patients during immunotherapy. Proc Natl Acad Sci United States America (2020) 117(27):16072–82. doi: 10.1038/s43018-019-0022-x
177. Kwon M, An M, Klempner SJ, Lee H, Kim KM, Sa JK, et al. Determinants of response and intrinsic resistance to PD-1 blockade in microsatellite instability-high gastric cancer. Cancer discovery (2021) 11(9):2168–85. doi: 10.1016/j.immuni.2020.04.014
178. Cader FZ, Hu X, Goh WL, Wienand K, Ouyang J, Mandato E, et al. A peripheral immune signature of responsiveness to PD-1 blockade in patients with classical Hodgkin lymphoma. Nat Med (2020) 26(9):1468–79. doi: 10.1186/1479-5876-10-146
179. Philip M, Fairchild L, Sun L, Horste EL, Camara S, Shakiba M, et al. Chromatin states define tumour-specific T cell dysfunction and reprogramming. Nature (2017) 545(7655):452–6. doi: 10.3389/fimmu.2018.01613
180. Ladle BH, Li KP, Phillips MJ, Pucsek AB, Haile A, Powell JD, et al. De novo DNA methylation by DNA methyltransferase 3a controls early effector CD8+ T-cell fate decisions following activation. Proc Natl Acad Sci United States America (2016) 113(38):10631–6. doi: 10.1016/j.ejca.2016.12.011
181. Henning AN, Roychoudhuri R, Restifo NP. Epigenetic control of CD8(+) T cell differentiation. Nat Rev Immunol (2018) 18(5):340–56. doi: 10.1016/j.ejca.2016.12.031
182. Pauken KE, Sammons MA, Odorizzi PM, Manne S, Godec J, Khan O, et al. Epigenetic stability of exhausted T cells limits durability of reinvigoration by PD-1 blockade. Sci (New York NY) (2016) 354(6316):1160–5. doi: 10.1093/annonc/mdz123
183. Sen DR, Kaminski J, Barnitz RA, Kurachi M, Gerdemann U, Yates KB, et al. The epigenetic landscape of T cell exhaustion. Sci (New York NY) (2016) 354(6316):1165–9. doi: 10.1136/jitc-2022-005509
184. Krishna S, Lowery FJ, Copeland AR, Bahadiroglu E, Mukherjee R, Jia L, et al. Stem-like CD8 T cells mediate response of adoptive cell immunotherapy against human cancer. Sci (New York NY) (2020) 370(6522):1328–34. doi: 10.1126/science.abb9847
185. Jansen CS, Prokhnevska N, Master VA, Sanda MG, Carlisle JW, Bilen MA, et al. An intra-tumoral niche maintains and differentiates stem-like CD8 T cells. Nature (2019) 576(7787):465–70. doi: 10.1172/jci.insight.97828
186. Sade-Feldman M, Yizhak K, Bjorgaard SL, Ray JP, de Boer CG, Jenkins RW, et al. Defining T cell states associated with response to checkpoint immunotherapy in melanoma. Cell (2019) 176(1-2):404. doi: 10.1158/1078-0432.CCR-19-2931
187. Park SL, Gebhardt T, Mackay LK. Tissue-resident memory T cells in cancer immunosurveillance. Trends Immunol (2019) 40(8):735–47. doi: 10.1016/j.it.2019.06.002
188. Ganesan AP, Clarke J, Wood O, Garrido-Martin EM, Chee SJ, Mellows T, et al. Tissue-resident memory features are linked to the magnitude of cytotoxic T cell responses in human lung cancer. Nat Immunol (2017) 18(8):940–50. doi: 10.1038/s41591-019-0734-6
189. Prokhnevska N, Cardenas MA, Valanparambil RM, Sobierajska E, Barwick BG, Jansen C, et al. CD8(+) T cell activation in cancer comprises an initial activation phase in lymph nodes followed by effector differentiation within the tumor. Immunity (2023) 56(1):107–24.e5. doi: 10.1136/jitc-2022-004512
190. Dammeijer F, van Gulijk M, Mulder EE, Lukkes M, Klaase L, van den Bosch T, et al. The PD-1/PD-L1-checkpoint restrains T cell immunity in tumor-draining lymph nodes. Cancer Cell (2020) 38(5):685–700.e8. doi: 10.1038/s41591-020-1006-1
191. Dijkstra KK, Cattaneo CM, Weeber F, Chalabi M, van de Haar J, Fanchi LF, et al. Generation of tumor-reactive T cells by co-culture of peripheral blood lymphocytes and tumor organoids. Cell (2018) 174(6):1586–98.e12. doi: 10.3389/fimmu.2021.649343
192. Mazzaschi G, Minari R, Zecca A, Cavazzoni A, Ferri V, Mori C, et al. Soluble PD-L1 and circulating CD8+PD-1+ and NK cells enclose a prognostic and predictive immune effector score in immunotherapy treated NSCLC patients. Lung Cancer (Amsterdam Netherlands) (2020) 148:1–11. doi: 10.1158/2326-6066.CIR-19-0398
193. Spitzer MH, Carmi Y, Reticker-Flynn NE, Kwek SS, Madhireddy D, Martins MM, et al. Systemic immunity is required for effective cancer immunotherapy. Cell (2017) 168(3):487–502.e15. doi: 10.1016/j.cell.2016.12.022
194. Kagamu H, Kitano S, Yamaguchi O, Yoshimura K, Horimoto K, Kitazawa M, et al. CD4(+) T-cell immunity in the peripheral blood correlates with response to anti-PD-1 therapy. Cancer Immunol Res (2020) 8(3):334–44. doi: 10.1158/2326-6066.CIR-19-0574
195. Zuazo M, Arasanz H, Fernández-Hinojal G, García-Granda MJ, Gato M, Bocanegra A, et al. Functional systemic CD4 immunity is required for clinical responses to PD-L1/PD-1 blockade therapy. EMBO Mol Med (2019) 11(7):e10293. doi: 10.1093/annonc/mdz253.058
196. Koh J, Hur JY, Lee KY, Kim MS, Heo JY, Ku BM, et al. Regulatory (FoxP3(+)) T cells and TGF-β predict the response to anti-PD-1 immunotherapy in patients with non-small cell lung cancer. Sci Rep (2020) 10(1):18994. doi: 10.1038/s41598-020-76130-1
197. Kim HR, Park SM, Seo SU, Jung I, Yoon HI, Gabrilovich DI, et al. The ratio of peripheral regulatory T cells to lox-1(+) polymorphonuclear myeloid-derived suppressor cells predicts the early response to anti-PD-1 therapy in patients with non-small cell lung cancer. Am J Respir Crit Care Med (2019) 199(2):243–6. doi: 10.1164/rccm.201808-1502LE
198. Woods DM, Ramakrishnan R, Laino AS, Berglund A, Walton K, Betts BC, et al. Decreased suppression and increased phosphorylated STAT3 in regulatory T cells are associated with benefit from adjuvant PD-1 blockade in resected metastatic melanoma. Clin Cancer Res (2018) 24(24):6236–47. doi: 10.1158/1078-0432.CCR-18-1100
199. Paschen A, Melero I, Ribas A. Central role of the antigen-presentation and interferon-γ Pathways in resistance to immune checkpoint blockade. Annu Rev Cancer Biol (2022) 6(1):85–102. doi: 10.1146/annurev-cancerbio-070220-111016
200. Ali M, Foldvari Z, Giannakopoulou E, Böschen ML, Strønen E, Yang W, et al. Induction of neoantigen-reactive T cells from healthy donors. Nat Protoc (2019) 14(6):1926–43. doi: 10.1038/s41596-019-0170-6
201. Harari A, Graciotti M, Bassani-Sternberg M, Kandalaft LE. Antitumour dendritic cell vaccination in a priming and boosting approach. Nat Rev Drug discovery (2020) 19(9):635–52. doi: 10.1038/s41573-020-0074-8
202. Oh SA, Wu DC, Cheung J, Navarro A, Xiong H, Cubas R, et al. PD-L1 expression by dendritic cells is a key regulator of T-cell immunity in cancer. Nat cancer (2020) 1(7):681–91. doi: 10.1038/s43018-020-0075-x
203. Mayoux M, Roller A, Pulko V, Sammicheli S, Chen S, Sum E, et al. Dendritic cells dictate responses to PD-L1 blockade cancer immunotherapy. Sci Trans Med (2020) 12(534):eaav7431. doi: 10.1126/scitranslmed.aav7431
204. Peng Q, Qiu X, Zhang Z, Zhang S, Zhang Y, Liang Y, et al. PD-L1 on dendritic cells attenuates T cell activation and regulates response to immune checkpoint blockade. Nat Commun (2020) 11(1):4835. doi: 10.1038/s41467-020-18570-x
205. Huuhtanen J, Kasanen H, Peltola K, Lönnberg T, Glumoff V, Brück O, et al. Single-cell characterization of anti-LAG-3 and anti-PD-1 combination treatment in patients with melanoma. J Clin Invest (2023) 133(6):e164809. doi: 10.1172/JCI164809
206. Fourcade J, Sun Z, Benallaoua M, Guillaume P, Luescher IF, Sander C, et al. Upregulation of Tim-3 and PD-1 expression is associated with tumor antigen-specific CD8+ T cell dysfunction in melanoma patients. J Exp Med (2010) 207(10):2175–86. doi: 10.1084/jem.20100637
Keywords: immunotherapy, peripheral blood, T cell, TCR repertoire, immune checkpoint blockade, PD-1, PD-L1, single-cell analysis
Citation: Luo H, Wang W, Mai J, Yin R, Cai X and Li Q (2023) The nexus of dynamic T cell states and immune checkpoint blockade therapy in the periphery and tumor microenvironment. Front. Immunol. 14:1267918. doi: 10.3389/fimmu.2023.1267918
Received: 27 July 2023; Accepted: 18 September 2023;
Published: 10 October 2023.
Edited by:
Nancy H. Ruddle, Yale University, United StatesReviewed by:
Penghui Zhou, Dana–Farber Cancer Institute, United StatesLukman Olalekan Afolabi, Chinese Academy of Sciences (CAS), China
Yutian Peng, Genentech Inc., United States
Copyright © 2023 Luo, Wang, Mai, Yin, Cai and Li. This is an open-access article distributed under the terms of the Creative Commons Attribution License (CC BY). The use, distribution or reproduction in other forums is permitted, provided the original author(s) and the copyright owner(s) are credited and that the original publication in this journal is cited, in accordance with accepted academic practice. No use, distribution or reproduction is permitted which does not comply with these terms.
*Correspondence: Rutie Yin, yinrutie@scu.edu.cn; Xuyu Cai, epigenome24@163.com; Qintong Li, liqintong@scu.edu.cn