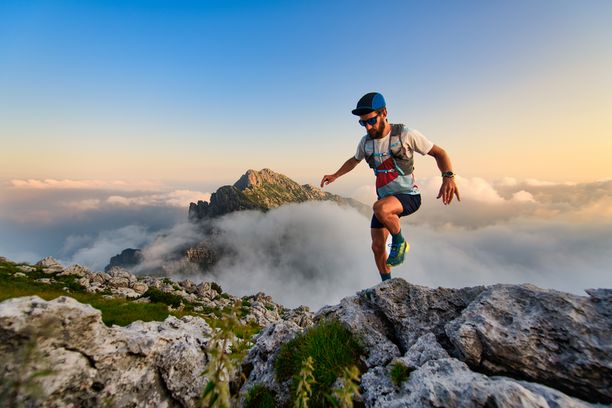
95% of researchers rate our articles as excellent or good
Learn more about the work of our research integrity team to safeguard the quality of each article we publish.
Find out more
ORIGINAL RESEARCH article
Front. Immunol., 11 January 2024
Sec. Microbial Immunology
Volume 14 - 2023 | https://doi.org/10.3389/fimmu.2023.1263352
Introduction: The attenuation of BCG has led to the loss of not only immunogenic proteins but also lipid antigens.
Methods: Thus, we compared the macrophage and T-cell responses to nonpolar lipid extracts harvested from BCG and Mycobacterium tuberculosis (Mtb) to better understand the role of BCG lipids in the already known diminished responses of the vaccine strain.
Results: Relative to Mtb, nonpolar lipid extract from BCG presented a reduced capacity to trigger the expression of the genes encoding TNF, IL-1b, IL-6 and IL-10 in RAW 264.7 macrophages. Immunophenotyping of PBMCs isolated from healthy individuals revealed that lipids from both BCG and Mtb were able to induce an increased frequency of CD4+ and CD8+ T cells, but only the lipid extract from Mtb enhanced the frequency of CD4-CD8-double-negative, γσ+, CD4+HLA-DR+, and γσ+HLA-DR+ T cells relative to the nonstimulated control. Interestingly, only the Mtb lipid extract was able to increase the frequency of CD4+ memory (CD45RO+) T cells, whereas the BCG lipid extract induced a diminished frequency of CD4+ central memory (CD45RO+CCR7-) T cells after 48 h of culture compared to Mtb.
Discussion: These findings show that the nonpolar lipids of the BCG bacilli presented diminished ability to trigger both proinflammatory and memory responses and suggest a potential use of Mtb lipids as adjuvants to increase the BCG vaccine efficacy.
Tuberculosis (TB), caused by Mycobacterium tuberculosis (Mtb), is one of the leading infectious diseases worldwide, with 10.6 million new cases and 1.6 million deaths in 2021 (1). BCG (Bacillus Calmette-Guérin) is currently the only licensed vaccine against pulmonary TB, despite its variable efficacy (0-80%) (2–4).
Composed of attenuated M. bovis bacilli, BCG has accumulated genomic polymorphisms that account for the absence of not only protein antigens but also key lipid antigens (5–9). A lipidomic analysis compared the lipid profiles of Mtb and BCG and revealed more than 1,000 differences between both strains (7). Recently, we performed an in silico evaluation and found 14 nonhomologous lipid-related genes absent in the six BCG strains most used worldwide relative to Mtb. Those genes were associated with the functional categories “cell wall and cell processes”, “virulence, detoxification and adaptation”, “lipid metabolism”, and “intermediary metabolism and respiration”, and together, these gene modifications may favor a dormant-like state of the BCG strains (10).
Mycobacterial lipids play a crucial role in the immunopathogenesis of TB (11). Petrilli et al. (2020) showed differential macrophage and T-cell responses to lipids extracted from two Mtb strains, an ATP-binding cassette transporter-knockout strain and its parental strain, highlighting the role of these molecules in controlling the inflammatory response (12). In BCG, the absence of lipid antigens has been associated with important changes in the host’s immune response, with consequent decreased control of mycobacterial burden and vaccine protection in vivo (13–15).
Protection against TB relies on the induction of a strong cellular immune response, although correlates of protection have not been identified. The results from a phase IIb clinical trial with the candidate MVA85A did not add protection against TB, despite significant induction of T-helper type 1 (Th1) cells (16, 17). Only recent clinical trial results have shown that it is possible to boost the protection already conferred by BCG throughout the revaccination of adolescents (18) and by the immunization of adults with the candidate M72/ASO1E (19). Other promising candidates have been proposed, including relevant findings from nonhuman primate models, that have been shown to induce not only CD4 and CD8 T cells but also polyfunctional Th17 cells and interleukin-10 production (20, 21). However, these results have not yet reached public health action.
The role of protein antigens has already been comprehensively described, whereas the importance of lipid antigens in the host’s immune response has been less explored. Here, we aimed to compare the cellular immune response induced by BCG and Mtb lipid extracts to better understand the influence of lipid losses on strain attenuation. These findings could elucidate future studies on the use of this class of antigens in new vaccine candidates to promote a more effective response and protection in combination with proteins.
M. bovis BCG Moreau (BCG Moreau RDJ, FAP) and M. tuberculosis Erdman strains were used. Both strains were cultured in Middlebrook 7H9 broth (Difco, MD) supplemented with 10% ADC (Beckton-Dickinson, MD) and incubated at 37°C and 5% CO2 until stationary phase. Then, planktonic cultures of BCG Moreau and Mtb were harvested and used for extraction of nonpolar lipids (22, 23). Briefly, 5 mL of methanol with 0.3% NaCl (100:10) and 2.5 mL of petroleum ether were added to 30 mL of cultures and incubated for 30 min at room temperature. The upper petroleum ether layer containing the nonpolar lipids was collected after centrifugation and kept in glass flasks until complete solvent evaporation. Nonpolar lipid extracts of each strain were weighed and resuspended in hexane:isopropanol (1:1) at 0.02 mg/mL. The nonpolar fraction is expected to have phthiocerol dimycocerosates (PDIM), triacylglycerol (TAG), pentacyl trehalose (PAT), trehalose monomycolate (TMM), and dimycolate (TDM, the cord factor), among others (24). Finally, 24-well tissue culture plates were layered with 0.5 mL of lipid extracts or hexane:isopropanol. Solvent evaporation was allowed, and plates were kept at -20°C until use.
RAW 264.7 cells (ATCC TIB-71) were cultured in Dulbecco’s modified Eagle’s medium (DMEM; Gibco) supplemented with 10% FBS at 37°C and 5% CO2. After achieving 70% confluency, macrophages were seeded onto lipid-coated 24-well tissue culture plates at 3.7×10^5 cells/well and incubated at 37°C and 5% CO2 for 2 h, 12 h, 24 h or 72 h. For the control samples, wells were coated with hexane/isopropanol in the absence of lipid extracts. Staining with trypan blue (Gibco) was used to assess cell number and viability.
Total RNA was extracted from RAW cells using the TRIzol RNA extraction protocol (Invitrogen, Life Technologies) and treated with DNase (Qiagen). DNA-free RNA (500 ng) was mixed with 50 ng of random hexamers and 50 μM oligo (dT) (Invitrogen), and cDNA was synthesized by Superscript III reverse transcriptase (Invitrogen) following the manufacturer’s recommendations.
The expression of the TNFα, IL-1β, IL-6 and IL-10 genes was measured (Supplementary Table 1). Primers were designed to produce a 100–195 bp amplicon for each gene. qPCRs were performed using 25 ng of cDNA and Maxima SYBR Green/ROX qPCR Master Mix (2X) (Thermo Fisher) following the manufacturer’s recommendations. The expression levels of all target genes were normalized to β-actin and glyceraldehyde 3-phosphate dehydrogenase (GAPDH), and relative changes between lipid-stimulated and nonstimulated RAW cells were measured by 2-ΔΔCt (25).
Participants (n = 12) were enrolled in this study and recruited from Gonçalo Moniz Institute (FIOCRUZ). All participants had been vaccinated with BCG during infancy in accordance with national guidelines and tested negative for latent TB infection by interferon-γ release assay (QuantiFERON® TB Gold Plus) upon enrollment. The mean age of the volunteers was 28.08 years (SD = 6.37) with 66.7% identified as female. Information about previous contact with TB patients and HIV status was self-declared, adhering regulation from the Research Ethics Committee at Gonçalo Moniz Institute (FIOCRUZ) (approved protocol number: 57273322.4.0000.0040). Individuals with regular contact with TB patients, those who reported recent episodes of cough and/or fever, or those with positive or indeterminate interferon-γ release assay results were excluded from the study.
Peripheral blood mononuclear cells (PBMCs) were obtained by Ficoll-Paque (GE Healthcare) density gradient and cryopreserved in liquid nitrogen with inactive fetal bovine serum (FBS) and 10% DMSO before culture assays. Cryopreserved cells were then thawed, and PBMC concentrations were adjusted to 106 cells/mL in 1 mL of RPMI 1640 (with 2 mM L-glutamine and 30 mM HEPES) containing 1% gentamicin and 10% FBS (GIBCO). PBMCs were added to 24-well tissue culture plates previously prepared with nonpolar lipid extracts from BCG and Mtb. Phytohemagglutinin (PHA) (GIBCO) (10 μg/mL) was added as a positive control. Cells were cultured for 24 h, 48 h and 72 h at 37°C in a 5% CO2 humidified atmosphere, and the 48 h time-point was chosen for the analyses.
Cells were first stained with CD3-FITC, CD4-PE, CD8-APC-Cy7, CD45RA PE-Cy7, CD45RO-APC, CCR7-BV510, HLA-DR-BV605, and TCRγδ-BV421 (BD Biosciences). For intracellular staining with IFNγ-PE-Cy7, TNF-AL700, IL-2-BV421, and IL-17-BV510, cells were fixed and permeabilized using the BD Biosciences Cytofix/Cytoperm Kit. Data were acquired on BD LSRFortessa® (50,000 events), and cell frequencies, as well as median fluorescence intensity (MFI), were measured using FlowJo 10 software (Tree Star Inc.). Supernatants of PBMC cultures were collected and stored at -20°C for cytokine assays. Concentrations of IFNγ and IL-10 were measured by ELISA (R&D Systems) according to the manufacturer’s instructions.
Statistical analyses were performed using GraphPad Prism 8 software (GraphPad Inc.). Normal distribution was assessed by the Shapiro−Wilk test. Statistical significance was assessed by Student’s t test, one-way ANOVA followed by Tukey’s posttest, or Kruskal−Wallis followed by Dunn’s posttest. The results were considered significant when p <0.05.
The transcriptional expression of genes encoding pro- and anti-inflammatory cytokines was measured in macrophages cultured with nonpolar lipid extracts harvested from both BCG and Mtb strains.
Lipids from the BCG strain induced lower transcript production than Mtb for all evaluated genes at most time points (Figure 1). Relative to the nonstimulated control, there was increased expression of IL-1β and IL-6 at 24 h of culture in macrophages cultured with both BCG and Mtb lipid extracts (p <0.0001) (Figures 1A, B). The expression of IL-1β and IL-6 increased by 48- and 9-fold in macrophages stimulated with BCG lipid extract and by 93- and 47-fold in Mtb lipid-induced cells, respectively (Supplementary Table 2). In addition, BCG lipids induced lower expression of TNF across the 2 h, 24 h and 72 h time points when compared with the stimulus triggered by Mtb lipids (Figure 1C). Whereas Mtb lipids sustained a 2-fold upregulation of this gene, the 2-ΔΔCt of TNF in BCG lipid-stimulated macrophages varied from 1.1 to 1.7 over the 2 h, 24 h and 72 h time points (Supplementary Table 2). After 12 h of incubation, the expression of IL-10 was 2- and 4-fold in cultures with lipids extracted from BCG and Mtb, respectively (p <0.001) (Figure 1D).
Figure 1 RT‒qPCR analyses of (A) IL-1β, (B) IL-6, (C) TNF, and (D) IL-10 after 2 h, 12 h, 24 h, and 72 h of cell exposure to BCG (orange) and Mtb (blue) lipid extracts. Data represent the mean fold-change difference between BCG and Mtb relative to untreated control. Gene expression was normalized to glyceraldehyde 3-phosphate dehydrogenase (GAPDH) and β -actin genes. p values were calculated by t test, with ***p <0.001; ****p <0.0001.
To evaluate whether lipids from BCG could also elicit a lymphocyte response, PBMCs obtained from healthy individuals were cultured with lipid extracts and stained for immunophenotyping (Supplemetary Figure 1). Relative to nonstimulated controls, lipids from both strains enhanced the frequencies of CD4+ and CD8+ T cells (p <0.05) (Figures 2A, B), whereas only Mtb significantly increased the frequencies of CD4-CD8- double-negative (DN) and γδ+ T cells (p <0.05 and p <0.01, respectively) (Figures 2C, D). Similarly, Mtb but not BCG lipids induced the proliferation of HLA-DR-positive CD4+ and γδ+ T cells (p <0.05) (Figures 2E, H).
Figure 2 Flow cytometry of conventional and nonconventional T cells after 48 h of in vitro culture of PBMCs from healthy individuals with lipid extracts of BCG and Mtb. (A) and (E) Frequencies of CD4+ and CD4+ HLA-DR+ T cells. (B) and (F) Frequencies of CD8+ and CD8+ HLA-DR+ T cells. (C) and (G) Frequencies of CD4-CD8- DN and CD4-CD8- DN HLA-DR+ T cells. (D) and (H) Frequencies of γδ+ and γδ+ HLA-DR+ T cells. Normal distribution was determined by the Shapiro‒Wilk test. p values for normal distributions were calculated by one-way ANOVA, and p values for nonnormal distributions were calculated by the Kruskal‒Wallis test. *p <0.05; **p <0.01; ***p <0.001. Nonstimulated control (negative control); Phytohemagglutinin, PHA (positive control).
Neither BCG nor Mtb lipid extract stimulation resulted in significant changes in the frequencies of CD4+ and CD8+ naïve T cells (Figures 3A, B). Conversely, Mtb lipids induced both CD4+ and CD8+ memory T cells (p <0.0001), whereas lipid extract from BCG only increased the frequencies of the latter (p <0.01) when compared to the nonstimulated controls (Figures 3C, D). BCG lipids were not only unable to increase the frequency of CD4+ memory T cells but also induced significantly lower proliferation of this population than Mtb lipids (p <0.001) (Figure 3C).
Figure 3 Flow cytometry of memory T cells after 48 h of in vitro culture of PBMCs from healthy individuals with lipid extracts of BCG and Mtb. (A) and (B) Frequencies of CD4+ and CD8+ naïve T cells (CD45RA+). (C) and (D) Frequencies of CD4+ and CD8+ memory T cells (CD45RO+). (E) and (F) Frequencies of CD4+ and CD8+ central memory T cells (CD45RO+ CCR7+). (G) and (H) Frequencies of CD4+ and CD8+ effector memory T cells (CD45RO+ CCR7-). Normal distribution was determined by the Shapiro‒Wilk test. p values for normal distributions were calculated by one-way ANOVA, and p values for nonnormal distributions were calculated by the Kruskal‒Wallis test. **p <0.01; ***p <0.001; ****p <0.0001. Nonstimulated control (negative control); Phytohemagglutinin, PHA (positive control).
Nonpolar lipid extracts from both BCG and Mtb strains enhanced the frequency of CD4+ and CD8+ central memory T cells (p <0.0001) (Figures 3E, F) but induced lower proliferation of CD4+ and CD8+ effector memory T cells (p <0.0001) (Figures 3G, H) when compared to nonstimulated controls. Furthermore, compared to Mtb, BCG lipid extract induced significantly lower frequencies of central memory in CD4+ T-cell populations (p <0.01) (Figure 3E).
Intracellular cytokine levels in CD4+, CD8+, and CD4-CD8- DN T cells in lipid-induced PBMCs from healthy individuals were also assessed (Figure 4; Supplementary Table 3). Relative to nonstimulated cells, Mtb lipid extracts significantly increased the intracellular levels of TNF and IFNγ in all evaluated cell populations (Figures 4A-F). Lipids from Mtb also triggered the synthesis of IL-2 in CD4+ (Figure 4G) (p <0.05) and IL-17 in CD4-CD8- DN T cells (Figure 4L) (p <0.01). BCG lipid extract stimuli were able to only enhance the MFI of TNF and IFNγ+ in CD4-CD8- DN T cells (p <0.0001) (Figures 4C, F) and IL-17 in CD8+ T cells (Figure 4K) (p <0.05). Compared to Mtb, the BCG lipid extract induced significantly lower expression of TNF in CD4-CD8- DN cells (p <0.001) (Figure 4F).
Figure 4 Flow cytometry of CD4+, CD8+ and CD4-CD8- DN T cells producing TNF, IFNγ, IL-2, and IL-17 after 48 h of in vitro culture of PBMCs from healthy individuals with lipid extracts of BCG and Mtb. (A), (B), and (C) MFI of CD4+, CD8+ and CD4-CD8- DN T cells producing TNF. (D), (E), and (F) MFI of CD4+, CD8+ and CD4-CD8- DN T cells producing IFNγ. (G), (H), and (I) MFI of CD4+, CD8+ and CD4-CD8- DN T cells producing IL-2. (J), (K), and (L) MFI of CD4+, CD8+ and CD4-CD8- DN T cells producing IL-17. Normal distribution was determined by the Shapiro‒Wilk test. p values for normal distributions were calculated by one-way ANOVA, and p values for nonnormal distributions were calculated by the Kruskal‒Wallis test. *p <0.05; **p <0.01; ***p <0.001; ****p <0.0001. MFI: Median fluorescence intensity. Nonstimulated control (negative control); Phytohemagglutinin, PHA (positive control).
The concentrations of IFNγ and IL-10 in culture supernatants were measured at 48 h. Only Mtb lipid extract increased the concentration of both cytokines (p <0.01) (Figures 5A, B; Supplementary Table 5).
Figure 5 Concentrations of (A) IFNγ and (B) IL-10 production after 48 h of in vitro culture of PBMCs from healthy individuals with lipid extracts of BCG and Mtb. Normal distribution was determined by the Shapiro‒Wilk test. p values for normal distributions were calculated by one-way ANOVA, and p values for nonnormal distributions were calculated by the Kruskal‒Wallis test. **p <0.01. Nonstimulated control (negative control); Phytohemagglutinin, PHA (positive control).
Consecutive in vitro passages of M. bovis gave rise to BCG, an attenuated strain with depletion of at least nine regions of difference (RD), including RD1, which encodes the proteins from the ESX-1 secretion system ESX-1, such as ESAT-6 and CFP-10 (9). Although these proteins are important virulence factors, their deletion does not completely explain the reduced ability of BCG to induce a protective immune response after vaccination (14, 15, 26). The lipid profiles of Mtb and BCG differ from each other in more than 1,000 species (7), whereas an in silico analysis showed that lipid-related gene deletions hinder the production of mce3, enoyl-CoA hydratase, and phospholipase C in BCG. Thus, we reasoned that losses of nonpolar lipid antigens in BCG would contribute to impairing host cell functions and might determine whether the vaccine can be improved by adding Mtb-derived lipid adjuvants.
Compared to Mtb, we observed that BCG lipids predominantly induced diminished macrophage expression of the pro-inflammatory markers IL-1β, IL-6, and TNF. This diminished ability of BCG nonpolar lipids to induce inflammatory responses was also evidenced by the synthesis of TNF and IFNγ in both CD4+ and CD8+ T cells. Conversely, the difference between each stimulus was less evident in TNF- and IFNγ-producing CD4-CD8- DN T cells. Considering that the frequencies of these populations are relatively low compared to conventional T cells, we can assume that the BCG strain also has an impaired ability to induce inflammation at the adaptive immune response level.
Lipid extracts from Mtb and BCG strains were able to, directly or indirectly, activate several T-cell subpopulations, according to the analyses performed here. Interestingly, there was no difference in the frequencies of CD4+ and CD8+ T cells in cultures with both lipid extracts. In addition, although there was a significant increase in the frequencies of CD4-CD8- DN and γδ+ T cells in cultures with Mtb lipid extract, relative to the untreated control, there were no distinguishable differences in the proportions of these cells between cultures with BCG and Mtb lipid extracts. These data suggest that despite the reduced capacity of BCG nonpolar lipids to induce inflammation, depletions in the genes related to lipid metabolism did not alter the ability of this strain to increase the frequency of some subsets of T cells.
Furthermore, different TB vaccine candidates and inoculation routes have been associated with the activation of distinct memory T-cell subsets, but there is no consensus about which cell subtype is responsible for providing protection. Here, Mtb lipid extracts induced a greater response from memory T cells than BCG, especially CD4+ T cells, which have been associated with vaccine candidates that use lipid formulations (27, 28). In addition, lipid extracts from both BCG and Mtb strains prompted higher and lower frequencies of effector and central memory T cells, respectively. In particular, effector memory cells are induced by BCG vaccination in humans and represent the predominant cell population in the lungs of vaccinated mice, which has been associated with stronger and more efficient protection against infection (29–32). Conversely, smaller populations of central memory T cells result in poorer memory responses (33–36). Notably, Mtb lipids were associated with a greater increase of central memory and decrease of effector memory T cells, whereas BCG lipids induced a similar yet diminished dynamic between these populations. This finding might indicate that Mtb lipids have a better chance to induce a long-lasting T-cell memory response than BCG lipids.
This study incorporated a wide range of lymphocyte and cytokine markers to compare the cellular immune response induced by total nonpolar lipid extracts from Mtb and BCG. To the best of our knowledge, no other study has performed T-cell immunophenotyping under such stimulation. To minimize potential biases arising from current or previous Mtb infections, only healthy and IGRA-negative participants were included in this study. However, this approach precluded us from investigating the responses of cells pre-activated by Mtb to both Mtb and BCG lipids.
Notably, measuring the levels of CD1a receptors would increase our comprehension of the abilities of Mtb and BCG nonpolar lipids in stimulating CD4+ and CD8+ T cells. Additionally, subsequent evaluations of different antigen delivery models that more accurately simulate bacteria-cell interactions and ex vivo experiments are important to assess whether the lipid-induced immune response contributes to bacterial clearance.
The question that arises from these analyses is whether the nonpolar lipids from Mtb could in fact induce long lasting memory during a BCG vaccination and, if that is the case, which lipid species are responsible for such activation. Although our study could not provide evidence of long-term protection induced by mycobacterial lipids, our results support the possibility of improvement of the BCG vaccine by including Mtb lipid molecules as adjuvants in the vaccination scheme against TB.
The original contributions presented in the study are included in the article/Supplementary Material. Further inquiries can be directed to the corresponding author.
The studies involving humans were approved by Research Ethics Committee at Gonçalo Moniz Institute. The studies were conducted in accordance with the local legislation and institutional requirements. The participants provided their written informed consent to participate in this study.
AS: Conceptualization, Formal analysis, Investigation, Writing – original draft. AL: Investigation, Writing – review & editing. CA: Investigation, Writing – review & editing. IM: Investigation, Writing – review & editing. LA: Investigation, Writing – review & editing. LP: Supervision, Writing – review & editing. AQ: Conceptualization, Formal analysis, Project administration, Supervision, Writing – original draft. SA: Conceptualization, Project administration, Supervision, Writing – review & editing.
The author(s) declare financial support was received for the research, authorship, and/or publication of this article. This study received financial support from the Bahia State Research Support Foundation, Bahia, Brazil (grant numbers 5303/2017, BOL0172/2019 and BOL1421/2021).
The authors declare that the research was conducted in the absence of any commercial or financial relationships that could be construed as a potential conflict of interest.
All claims expressed in this article are solely those of the authors and do not necessarily represent those of their affiliated organizations, or those of the publisher, the editors and the reviewers. Any product that may be evaluated in this article, or claim that may be made by its manufacturer, is not guaranteed or endorsed by the publisher.
The Supplementary Material for this article can be found online at: https://www.frontiersin.org/articles/10.3389/fimmu.2023.1263352/full#supplementary-material
2. Abubakar I, Pimpin L, Ariti C, Beynon R, Mangtani P, Sterne J, et al. Systematic review and meta-analysis of the current evidence on the duration of protection by bacillus Calmette–Guérin vaccination against tuberculosis. Health Technol Assess (2013) 17:. doi: 10.3310/hta17370
3. Mangtani P, Abubakar I, Ariti C, Beynon R, Pimpin L, Fine PEM, et al. Protection by BCG vaccine against tuberculosis: A systematic review of randomized controlled trials. Clin Infect Dis (2014) 58:470–80. doi: 10.1093/cid/cit790
4. Roy A, Eisenhut M, Harris RJ, Rodrigues LC, Sridhar S, Habermann S, et al. Effect of BCG vaccination against Mycobacterium tuberculosis infection in children: systematic review and meta-analysis. BMJ (2014) 349:1–11. doi: 10.1136/BMJ.G4643
5. Hayashi D, Takii T, Fujiwara N, Fujita Y, Yano I, Yamamoto S, et al. Comparable studies of immunostimulating activities in vitro among Mycobacterium bovis bacillus Calmette-Guérin (BCG) substrains. FEMS Immunol Med Microbiol (2009) 56:116–28. doi: 10.1111/j.1574-695X.2009.00559.x
6. Hayashi D, Takii T, Mukai T, Makino M, Yasuda E, Horita Y, et al. Biochemical characteristics among Mycobacterium bovis BCG substrains. FEMS Microbiol Lett (2010) 306:103–9. doi: 10.1111/j.1574-6968.2010.01947.x
7. Layre E, Lee HJ, Young DC, Jezek Martinot A, Buter J, Minnaard AJ, et al. Molecular profiling of Mycobacterium tuberculosis identifies tuberculosinyl nucleoside products of the virulence-associated enzyme Rv3378c. Proc Natl Acad Sci (2014) 111:2978–83. doi: 10.1073/pnas.1315883111
8. Bottai D, Brosch R. The BCG strain pool: diversity matters. Mol Ther (2016) 24:201–3. doi: 10.1038/mt.2016.18
9. Brosch R, Gordon SV, Garnier T, Eiglmeier K, Frigui W, Valenti P, et al. Genome plasticity of BCG and impact on vaccine efficacy. Proc Natl Acad Sci (2007) 104:5596–601. doi: 10.1073/pnas.0700869104
10. Sarno A, Bitencourt J, Queiroz A, Arruda S. In silico comparisons of lipid-related genes between Mycobacterium tuberculosis and BCG vaccine strains. Genet Mol Biol (2021) 44:1–7. doi: 10.1590/1678-4685-GMB-2021-0024
11. Queiroz A, Riley LW. Bacterial immunostat: Mycobacterium tuberculosis lipids and their role in the host immune response. Rev Soc Bras Med Trop (2017) 50:9–18. doi: 10.1590/0037-8682-0230-2016
12. Petrilli JD, Müller I, Araújo LE, Cardoso TM, Carvalho LP, Barros BC, et al. Differential host pro-inflammatory response to mycobacterial cell wall lipids regulated by the mce1 operon. Front Immunol (2020) 11:1848. doi: 10.3389/fimmu.2020.01848
13. Chen JM, Islam ST, Ren H, Liu J. Differential productions of lipid virulence factors among BCG vaccine strains and implications on BCG safety. Vaccine (2007) 25:8114–22. doi: 10.1016/j.vaccine.2007.09.041
14. Tran V, Ahn SK, Ng M, Li M, Liu J. Loss of lipid virulence factors reduces the efficacy of the BCG vaccine. Sci Rep (2016) 6:29076. doi: 10.1038/srep29076
15. Zhang L, Ru H, Chen F, Jin C, Sun R, Fan X, et al. Variable virulence and efficacy of BCG vaccine strains in mice and correlation with genome polymorphisms. Mol Ther (2016) 24:398–405. doi: 10.1038/MT.2015.216
16. Tameris MD, Hatherill M, Landry BS, Scriba TJ, Snowden MA, Lockhart S, et al. Safety and efficacy of MVA85A, a new tuberculosis vaccine, in infants previously vaccinated with BCG: A randomised, placebo-controlled phase 2b trial. Lancet (2013) 381:1021–8. doi: 10.1016/S0140-6736(13)60177-4
17. Tameris M, McShane H, McClain JB, Landry B, Lockhart S, Luabeya AKK, et al. Lessons learnt from the first efficacy trial of a new infant tuberculosis vaccine since BCG. Tuberculosis (2013) 93:143–9. doi: 10.1016/j.tube.2013.01.003
18. Nemes E, Geldenhuys H, Rozot V, Rutkowski KT, Ratangee F, Bilek N, et al. Prevention of infection with mycobacterium tuberculosis by H4:IC31® Vaccination or BCG revaccination in adolescents. N Engl J Med (2018) 379:138. doi: 10.1056/NEJMOA1714021
19. van der Meeren O, Hatherill M, Nduba V, Wilkinson RJ, Muyoyeta M, Van Brakel E, et al. Phase 2b controlled trial of M72/AS01 E vaccine to prevent tuberculosis. New Engl J Med (2018) 379:1621–34. doi: 10.1056/NEJMOA1803484/SUPPL_FILE/NEJMOA1803484_DISCLOSURES.PDF
20. Darrah PA, Zeppa JJ, Hackney JA, Wadsworth MHI, Hughes TK, Pokkali S, et al. Prevention of tuberculosis in nonhuman primates following intravenous BCG immunization. Nat Press (2019) 577:95–102. doi: 10.1038/s41586-019-1817-8
21. Dijkman K, Sombroek CC, Vervenne RAW, Hofman SO, Boot C, Remarque EJ, et al. Prevention of tuberculosis infection and disease by local BCG in repeatedly exposed rhesus macaques. Nat Med (2019) 25(2):255–62. doi: 10.1038/s41591-018-0319-9
22. Reed MB, Domenech P, Manca C, Su H, Barczak AK, Kreiswirth BN, et al. A glycolipid of hypervirulent tuberculosis strains that inhibits the innate immune response. Nature (2004) 431(7004):84–7. doi: 10.1038/nature02837
23. Layre E, Sweet L, Hong S, Madigan CA, Desjardins D, Young DC, et al. A comparative lipidomics platform for Mycobacterium tuberculosis provides chemotaxonomic analysis for biomarker discovery. Chem Biol (2011) 18:1537–49. doi: 10.1016/j.chembiol.2011.10.013
24. Pirson C, Jones GJ, Steinbach S, Besra GS, Vordermeier HM. Differential effects of Mycobacterium bovis - Derived polar and apolar lipid fractions on bovine innate immune cells. Vet Res (2012) 43:54. doi: 10.1186/1297-9716-43-54
25. Livak KJ, Schmittgen TD. Analysis of relative gene expression data using real-time quantitative PCR and the 2–ΔΔCT method. Methods (2001) 25:402–8. doi: 10.1006/METH.2001.1262
26. Pym AS, Brodin P, Majlessi L, Brosch R, Demangel C, Williams A, et al. Recombinant BCG exporting ESAT-6 confers enhanced protection against tuberculosis. Nat Med (2003) 9:533–9. doi: 10.1038/nm859
27. Rai PK, Chodisetti SB, Zeng W, Nadeem S, Maurya SK, Pahari S, et al. A lipidated peptide of Mycobacterium tuberculosis resuscitates the protective efficacy of BCG vaccine by evoking memory T cell immunity. J Transl Med (2017) 15:201. doi: 10.1186/s12967-017-1301-x
28. Ancelet LR, Aldwell FE, Rich FJ, Kirman JR. Oral vaccination with lipid-formulated BCG induces a long-lived, multifunctional CD4+ T cell memory immune response. PloS One (2012) 7:e45888. doi: 10.1371/JOURNAL.PONE.0045888
29. Warr AJ, Anterasian C, Shah JA, De Rosa SC, Nguyen FK, Maleche-Obimbo E, et al. A CD4+ TNF+ monofunctional memory T-cell response to BCG vaccination is associated with Mycobacterium tuberculosis infection in infants exposed to HIV. EBioMedicine (2022) 80:1–13. doi: 10.1016/j.ebiom.2022.104023
30. Kumar NP, Padmapriyadarsini C, Rajamanickam A, Bhavani PK, Nancy A, Jayadeepa B, et al. BCG vaccination induces enhanced frequencies of memory T cells and altered plasma levels of common γc cytokines in elderly individuals. PloS One (2021) 16:1–13. doi: 10.1371/JOURNAL.PONE.0258743
31. Li L, Qiao D, Zhang X, Liu Z, Wu C. The immune responses of central and effector memory BCG-specific CD4+ T cells in BCG-vaccinated PPD+ donors were modulated by Treg cells. Immunobiology (2011) 216:477–84. doi: 10.1016/J.IMBIO.2010.09.003
32. Henao-Tamayo MI, Ordway DJ, Irwin SM, Shang S, Shanley C, Orme IM. Phenotypic definition of effector and memory T-lymphocyte subsets in mice chronically infected with mycobacterium tuberculosis. Clin Vaccine Immunol (2010) 17:618. doi: 10.1128/CVI.00368-09
33. Basile JI, Liu R, Mou W, Gao Y, Carow B, Rottenberg ME. Mycobacteria-specific T cells are generated in the lung during mucosal BCG immunization or infection with mycobacterium tuberculosis. Front Immunol (2020) 11:566319/BIBTEX. doi: 10.3389/FIMMU.2020.566319/BIBTEX
34. Kaushal D, Foreman TW, Gautam US, Alvarez X, Adekambi T, Rangel-Moreno J, et al. Mucosal vaccination with attenuated Mycobacterium tuberculosis induces strong central memory responses and protects against tuberculosis. Nat Commun (2015) 6:1–14. doi: 10.1038/NCOMMS9533
35. Vogelzang A, Perdomo C, Zedler U, Kuhlmann S, Hurwitz R, Gengenbacher M, et al. Central memory CD4+ T cells are responsible for the recombinant bacillus calmette-guérin ΔureC::hly vaccine’s superior protection against tuberculosis. J Infect Dis (2014) 210:1928. doi: 10.1093/INFDIS/JIU347
Keywords: Bacillus Calmette-Guerin, Mycobacterium tuberculosis, nonpolar lipid extracts, macrophage gene expression, memory T-cell responses
Citation: Sarno A, Leite A, Augusto C, Muller I, de Ângelis L, Pimentel L, Queiroz A and Arruda S (2024) Impaired macrophage and memory T-cell responses to Bacillus Calmette-Guerin nonpolar lipid extract. Front. Immunol. 14:1263352. doi: 10.3389/fimmu.2023.1263352
Received: 19 July 2023; Accepted: 26 December 2023;
Published: 11 January 2024.
Edited by:
Subash Babu, International Centers for Excellence in Research (ICER), IndiaReviewed by:
Ved Prakash Dwivedi, International Centre for Genetic Engineering and Biotechnology, IndiaCopyright © 2024 Sarno, Leite, Augusto, Muller, de Ângelis, Pimentel, Queiroz and Arruda. This is an open-access article distributed under the terms of the Creative Commons Attribution License (CC BY). The use, distribution or reproduction in other forums is permitted, provided the original author(s) and the copyright owner(s) are credited and that the original publication in this journal is cited, in accordance with accepted academic practice. No use, distribution or reproduction is permitted which does not comply with these terms.
*Correspondence: Sergio Arruda, c2VyZ2lvLmFycnVkYUBmaW9jcnV6LmJy
†These authors have contributed equally to this work
Disclaimer: All claims expressed in this article are solely those of the authors and do not necessarily represent those of their affiliated organizations, or those of the publisher, the editors and the reviewers. Any product that may be evaluated in this article or claim that may be made by its manufacturer is not guaranteed or endorsed by the publisher.
Research integrity at Frontiers
Learn more about the work of our research integrity team to safeguard the quality of each article we publish.