- 1The First Clinical College, Gannan Medical University, Ganzhou, Jiangxi, China
- 2Department of Orthopedics, Ganzhou City Hospital, Ganzhou, Jiangxi, China
- 3Department of Orthopedics, The First Affiliated Hospital of Gannan Medical College, Ganzhou, Jiangxi, China
Venous thromboembolism is a very common and costly health problem. Deep-vein thrombosis (DVT) can cause permanent damage to the venous system and lead to swelling, ulceration, gangrene, and other symptoms in the affected limb. In addition, more than half of the embolus of pulmonary embolism comes from venous thrombosis, which is the most serious cause of death, second only to ischemic heart disease and stroke patients. It can be seen that deep-vein thrombosis has become a serious disease affecting human health. In recent years, with the deepening of research, inflammatory response is considered to be an important pathway to trigger venous thromboembolism, in which the transcription factor NF-κB is the central medium of inflammation, and the NF-κB signaling pathway can regulate the pro-inflammatory and coagulation response. Thus, to explore the mechanism and make use of it may provide new solutions for the prevention and treatment of thrombosis.
1 Introduction
Deep-vein thrombosis (DVT) is a serious disease threatening human life. The incidence of DVT is 10-40% after general surgery and 40-60% after major orthopedic surgery, and in the absence of preventive measures, DVT can lead to further diseases such as pulmonary hypertension, recurrent thrombosis, post-thrombotic syndrome, and even fatal pulmonary embolism (1, 2). Therefore, patients with acute DVT face a high risk of death. The treatment of DVT in modern medicine can be roughly divided into anticoagulation therapy and thrombectomy (3). Anticoagulation therapy and surgery have made great progress in the treatment of DVT and are widely used. If more methods can continue to be found to treat thrombosis, then the recovery probability of related patients will be increased. Therefore, research on the prevention and treatment of thrombosis has never stopped.
Blood flow retardation, blood hypercoagulability, and endothelial cell injury are the three major factors of venous thrombosis (4, 5). They play different roles in the mechanism of venous thrombosis but are related to each other. Vascular endothelial cells divide blood from subendothelial tissue, store and secrete factors that affect platelet function, prevent platelet adhesion, and allow blood to flow normally. However, when the endothelium is disturbed by physical or chemical factors, the endothelial cells will undergo programmed biochemical changes, transform into the front surface of the thrombus, express TF, and accelerate the activation of factor X and factor IX, thereby activating the coagulation system (6). Changes in hemodynamics promote changes in the state of the vascular endothelium, and the flow of blood through the vasculature generates wall shear stress, resulting in structural and functional changes in the vessel wall (7). Shear stress also strongly affects endothelial cell gene expression (8, 9). There are “shear-stress response elements” in the promoters of related genes (10, 11), various “mechanical transducers” and downstream signal pathways, which associate external mechanical stimuli with intracellular and nuclear events (12–14). The hypercoagulable state is one of the important factors of venous thrombosis (15). When too many clotting proteins are produced in the blood, abnormal clotting proteins are produced to resist decomposition, and too few proteins that prevent thrombosis are produced, which will cause the blood to become hypercoagulable (16). The combination of the hypercoagulable state and acquired risk factors (surgery, braking, or hormone therapy) increases the risk of thrombosis (17, 18). Proper thrombus prevention can prevent the risk of thrombosis from exceeding this critical threshold, but thrombosis occurs when internal and external forces exceed the critical threshold (19).
In recent years, studies have found that inflammation is closely related to the formation and development of deep venous thrombosis. Inflammation mediates vascular endothelial cell injury (20, 21), releases vascular cell adhesion molecules (VCAM) and intercellular adhesion molecules (ICAM), stagnates blood flow, and accelerates venous thrombosis (22–25). The formation of blood clots exacerbates the inflammatory response, and the two affect each other. Therefore, study of the occurrence of venous thrombosis and the discovery of related proteins regulating inflammatory factors are of great significance for delaying and alleviating the formation of venous thrombosis and judging the prognosis.
2 Overview of the NF-κB signaling pathway
The NF-κB family consists of a group of structurally related and evolutionarily conserved transcription factors that play a key role in inflammatory response, immune function, cell survival, and prevention of apoptosis (26). There are currently five members of the mammalian NF-κB family, known as RelA (also known as p65), RelB, c-Rel, NF-κB1 (p50 and its precursor p105), and NF-κB2 (p52 and its precursor p100) (27).
Despite the expanding complexity of NF-κB signaling, the two most recognized pathways in mammalian cells are the so-called classical and atypical pathways (28–30), both of which are important in inflammatory response and immune regulation, despite their differences in signaling composition and biological function. The classical NF-κB pathway is induced by pro-inflammatory cytokines and depends on the induced degradation of IκB, specifically Iκbα, to activate the NF-κB1 p50, RelA, or c-Rel complex (28). The non-classical NF-κB pathway is triggered by certain members of the TNF family of cytokines rather than by TNF-A itself and depends on the induction process of p100 rather than the degradation of IκBα, leading to the activation of the NF-κB2 P52 or RelB complex (31–33). Activated NF-κB is transferred from the cytoplasm to the nucleus, where it causes the expression of target genes associated with inflammation. The NF-κB family has been shown to activate more than 500 inflammation-related genes (34, 35) and can initiate the expression of cytokines necessary for inflammation. Some of these cytokines, such as IL-1 and TNF-α, activate NF-κB itself, leading to the formation of a positive feedback loop that has the potential to produce chronic and excessive inflammation when NF-κB becomes abnormally or persistently active.
3 Relationship between NF-κB, inflammation, and thrombosis
3.1 The inflammatory response promotes thrombosis
In general, DVT can be caused by a variety of risk factors, including genetics, dietary habits, obesity, aging, trauma, and cancer (2). In recent years, with the deepening of research, inflammation is considered to be an important way for various risk factors to trigger the formation of VTE (36–39). In our recent case, the novel coronavirus infection (COVID-19) is caused by the novel coronavirus SARS-CoV-2, which is characterized by an excessive inflammatory response. It has been reported that about half of the hospitalized patients with COVID-19 have serious symptoms, such as deep-vein thrombosis and coagulation dysfunction in the lower extremities, and some patients may die (40–44). It has also been reported that the injection of the COVID-19 vaccine can cause an immune inflammatory response, thus promoting thrombosis and thrombocytopenia (45–50). When studying the changes of inflammatory factors in the plasma of DVT patients, it was found that the expression level of IL-17A was up-regulated, and the level of platelet aggregation was increased, which promoted platelet activation and aggregation, thus playing a role in promoting the formation of DVT (51). In addition, pro-inflammatory factors represented by interleukin-1 (IL-1), IL-6, IL-1β, IL-18, cox-2, TNF-α (52–55), and other inflammatory factors can induce inflammatory response, accelerate tissue injury, and stimulate the release of inflammatory mediators, leading to vascular endothelial injury and apoptosis (20, 21). This indicates that inflammation has become a factor that cannot be ignored in the mechanism of thrombosis.
3.2 NF-κB induces an inflammatory response
Transcription factor NF-κB is the central mediator of inflammatory response, mainly in the form of p65 and p50 binding and in the form of dimer; when stimulated, NF-κB p65/p50 dissociates with IκBα and enters the nucleus to activate the corresponding gene transcription (56). The NF-κB signaling pathway is the central link of various inflammatory responses, which can up-regulate the expression of pro-inflammatory factors in the activated state, and inflammatory response can release inflammatory factor IL-1β through the NF-κB pathway, resulting in increased expression of monocyte chemotactic protein-1 (MCP-1) and activation of endothelial cells (57, 58). Releasing intercellular adhesion molecule-1 (ICAM-1), platelet endothelial cell adhesion molecule-1 (PECAM-1), and vascular cell adhesion molecule-1 (VCAM-1), etc. (59, 60), further activates NF-κB, amplifies inflammatory response, and releases more inflammatory factors. Increased platelet reactivity, activation of the plasma coagulation cascade, and impaired function of physiological anticoagulants result in the hypercoagulability of blood (61, 62).
3.3 NF-κB is involved in thrombosis
NF-κB signaling plays an important role in the vascular system and in the cell types involved in thromboinflammatory processes. By mediating the interaction between endothelial cells, platelets, and inflammatory response, NF-κB disrupts the coagulation–fibrinolysis balance and induces thrombosis (63).
3.3.1 Platelets
Platelets are not only involved in primary hemostasis but also in the formation of thrombus induced by inflammation. Platelet activators include not only thrombin and ADP but also molecules involved in inflammation (64). Platelets as non-nucleated cells also contain members of the NF-κB family and their corresponding signaling molecules, which are involved in platelet activation and secondary feedback loops (56). Activated platelets express or secrete pro-inflammatory and pro-coagulant substances on their surfaces, such as adhesion molecules, growth factors, cytokines, and the fibrinolytic inhibitor PAI-1, inducing surface aggregation of coagulation factors (65).
3.3.2 Endothelial cell
The injury of the vein wall or vein endothelial cells caused by various factors is one of the factors that cause DVT. Studies have confirmed that NF-κB signaling molecules exist in endothelial cells (63). When injured, endothelial cells are activated, which can inhibit the expression of thrombomodulin (TM) and activate the expression of endothelial tissue factor (TF), resulting in the activation of adhesion molecules such as P-selectin and clotting factor vWF (66), and the endothelial cells change from anticoagulant, anti-inflammatory, and vasodilator functions to proinflammatory and pre-thrombotic states.
3.3.3 NETs
Inflammatory cells represented by neutrophils and monocytes were able to rapidly aggregate and adhere to the venous endothelium (67). Among them, neutrophils can activate coagulation factors XII, initiate endogenous coagulation, and also form neutrophil extracellular traps (NETs) after apoptosis. NETs, as part of the body’s innate immunity, are an extracellular network of fibers made up of disaggregated chromatin (DNA fibers and histones) released by neutrophils and more than 30 granule proteins with antimicrobial properties (68, 69), providing a supporting basis for thrombosis through their fiber network structure (70). They interact with platelets to further stimulate platelet aggregation, activate thrombin, and accelerate the DVT process (71, 72) (Figure 1).
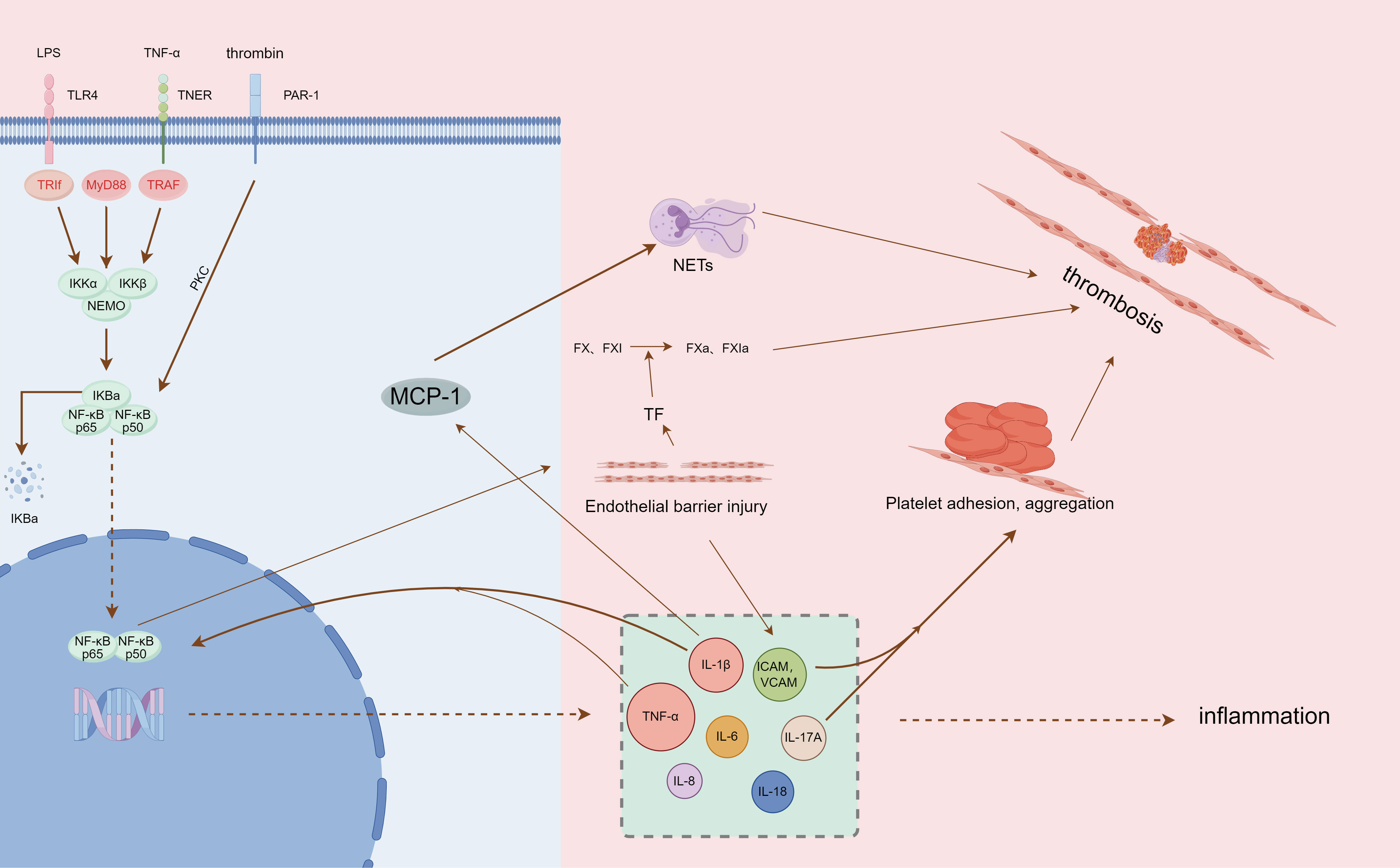
Figure 1 Summary of NF-κB pathway mediating inflammation and thrombosis (by Figdraw, authorization ID: PIWYI6b557).
4 Interference with NF-κB signaling pathway and deep-vein thrombosis
The NF-κB signaling pathway is closely related to thrombosis. Activation of the NF-κB signaling pathway can significantly increase the levels of thromboxane B2 (TXB2), interleukin6 (IL-6), tumor necrosis factor-α (TNF-α), and PAI and significantly decrease the levels of 6-keto-PGF1α and t-PA, exacerbating inflammation and thrombosis (73). Therefore, we may think that thrombosis can be prevented and that it can be reduced by inhibiting the NF-κB signaling pathway.
4.1 microRNA regulates NF-κB signaling pathway to interfere with deep-vein thrombosis
MiRNAs are key regulators of many biological processes (cell differentiation, proliferation, apoptosis, and metabolism), and abnormal expression of miRNAs is known to be associated with a variety of human diseases. Hugo’s (2016) study demonstrated the abnormal expression of miRNA in venous thrombosis and suggested that miRNA may be related to the molecular mechanism of DVT. There is evidence that miRNA plays an important role in hemostasis (74), and some foreign scholars (75) have confirmed that miR-181a-5p can inhibit the expression of F11 mRNA and coagulation factor XI. Some members of the miRNA family (miR-126 and miR-145) can promote the dissolution and recanalization of thrombus (76, 77), suggesting that miRNA may be involved in the formation of DVT.
4.1.1 miRNA-181b
DVT group and miR-181b overexpression and inhibition of rat models were constructed by producing a deep-vein thrombosis model and injecting normal saline, miR-181b mimics, and inhibitors into the tail vein. The changes of NF-κB (P65) in the venous endothelium of rats in each group were analyzed, and the expression level of NF-κB (P65) in the venous endothelium of rats in the Normal group was used as a reference. The results showed that the expression of NF-κB (P65) in the venous endothelium of rats in the 181b-i group was the highest, followed by the DVT group, and the 181b-m group was lower than the DVT group but higher than the Normal group; moreover, the difference was statistically significant (P<0.05). These results indicate that the expression of NF-κB (P65) is increased in the rat vena cava DVT model, and miR-181b can inhibit the expression of NF-κB (P65). By comparing the length and wet weight of the thrombus in each model group, no thrombus formation was observed in the Normal group. Overexpression of miR-181b can shorten the length of the thrombus and lighten the wet weight after thrombus formation in rats; inhibition of miR-181b expression can lengthen the length of thrombus and increase the wet weight after thrombus formation. These results suggest that miR-181b can reduce the formation of DVT by inhibiting the NF-κB signaling pathway to a certain extent (78).
4.1.2 miRNA-150
Up-regulating the expression of miR-150 inhibited thrombosis in DVT rats. The effect of miR-150 on inflammation was studied in vivo and in vitro. The rats were injected with LV-NC, and it was found that the administration of LV-miR-150 inhibited the platelet aggregation inhibition rate and TXB2 content in the rat model and significantly inhibited thrombosis. Transfected into in vivo skin cells, PAI-1, TNF-α, IL-6, and IL-8 levels were significantly reduced, indicating that miR-150 alleviated inflammation and inhibited apoptosis of vascular endothelial cells. The expression level of NF-κB p50 in vascular endothelial cells transfected with miR-150 mimics was significantly decreased, while the expression level of NF-κB p50 was significantly increased by miR-150 inhibitors. These results suggest that miR-150 may have a negative regulatory effect on NF-κB p50. Therefore, overexpression of miR-150 can be used as a potential therapeutic target for future DVT (79).
4.1.3 miRNA-141
This study found that by overexpression of miRNA-141, the expression of TLR4 and its signaling pathway-related proteins NF-κB, Rac1, and IL-1β in vascular tissues of thrombotic rats was significantly down-regulated, and by restoring the expression of TLR4 and NF-κB, the expression of Rac1 and IL-1β was restored at the same time, and multiple related indexes of miRNA-141 on thrombus were significantly reversed (80). Activated TLR4 induces late activation of NF-κB, which significantly increases TNF-α expression and causes widespread inflammation (53). Furthermore, TNF-α stimulates the TNF receptor (TNFR) and induces phosphorylation of IκB kinase (IKK), which in turn enhances NF-κB activity (81). NF-κB is the end point of the TLR4/NF-κB pathway and the regulatory hub of an inflammatory response, and its activation can enhance the inflammatory response and promote the formation of a thrombus. This may be a key mechanism by which miRNA-141 inhibits thrombus formation by regulating the TLR4/NF-κB signaling pathway (Table 1).
4.2 TCM preparations alleviate thrombosis by modulating NF-κB signaling
4.2.1 Qihong Tongluo prescription
Qihong Tongluo prescription is mainly composed of Astragalus and safflower. Astragalus has a variety of biological functions, including potent immunomodulatory, antioxidant, anti-inflammatory, and antitumor activities (82). Isoflavones, saponins, and polysaccharides are three types of beneficial compounds for their pharmacological activity and therapeutic efficacy (82–84). Astragalus polysaccharides decreased the expression of IL-1β, IL-6, TNF-α, and INF-γ by regulating the toll-like receptor 4 (TLR4)/NF-κB signaling pathway (85, 86). In addition, safflower can inhibit platelet activation, adhesion, and aggregation (87, 88). In summary, we believe that Qihong Tongluo prescription’s inhibition of thrombosis is the result of the joint action of its active components.
4.2.2 Gegen Qinlian pills
The high incidence of thrombotic events is one of the clinical features of coronavirus disease (COVID-19) due to the high inflammatory response caused by the virus. Gegen Qinlian pill (GQP) is a traditional Chinese medicine, which can inhibit toll-like receptor 4 (TLR4)/nuclear factor κB (NF-κB) signaling (89–91), has good anti-inflammatory activity, has a good effect on the treatment of COVID-19, and has shown anti-thrombotic potential. In our study, GQP treatment significantly reduced the expression of TNF-α, NLRP3, and NF-κB, reduced lung, liver, and tail thrombosis, and increased tail blood flow in mice (92). This at least partially supports the hypothesis that GQP can inhibit inflammation-induced thrombosis by inhibiting NF-κB/NLRP3 signaling.
4.2.3 Huanglianjiedu Decoction
HLJJD is a famous prescription in China, and its main compounds have been studied as baicalin and berberine (93). Baicalin, a flavonoid compound extracted from the root of Scutellaria baicalensis, has significant anti-inflammatory and antibacterial effects, scavenging oxygen free radicals and anti-allergic reactions (94, 95). Baicalin can inhibit the NF-κB signaling pathway, reduce the expression level of the p-NF-κB p65 protein, and reduce inflammatory response (96, 97). Berberine is an isoquinoline alkaloid isolated from Coptis chinensis, a Chinese medicinal plant, and has significant anti-inflammatory effects (98, 99). For the infection-induced tissue injury model, the gene and protein expression levels of TNF-α, TLRs, and NF-κB p65 were significantly reduced in the berberine treatment group (100–102). TLR4 is a pattern recognition receptor, and activated TLR4 induces late activation of NF-κB, which significantly increases TNF-α expression and causes widespread inflammation (53). TNF-α stimulates the phosphorylation of IκB kinase (IKK), which in turn enhances the activity of NF-κB (81). As the endpoint of the TLR4/NF-κB pathway and the regulatory hub of the inflammatory response, the activation of NF-κB can enhance the inflammatory response and promote the formation of thrombosis.
Through intravenous injection of the effective components of Huanglian Jidutang into the thrombus model, the results showed that the contents of IL-1β, IL-6, and TNF-α and the expression levels of TLR4, NF-κB, NLRP3, and Caspase-1 were decreased (103), and they also showed significantly reduced thrombus dry weight, Block platelet aggregation, and adhesion induced by collagen (104). In conclusion, the inhibitory effect of the active components of Huanglian Jiedu Decoction on the NF-κB pathway is the cause of alleviating inflammation and reducing thrombosis.
4.2.4 Liu Shen Wan
LSW is a classic proprietary Chinese medicine with anti-inflammatory and analgesic effects (105). Its main components are cow gallstone, musk secretion, toad secretion, pearl shell, realgar, and borneol (106). In PR8-infected cells, LSW significantly down-regulated the expression levels of IL-1β, TNF-α, IL-6, and IFN-γ. In mice infected with PR8, LSW reduced the secretion of TNF-α, IL-1β, IL-6, and IFN-γ in lung tissue, significantly improving survival. In addition, LSW significantly reduced the expression levels of TLR4, phosphor-NF-κB p65, and phosphor-iκBα (107). It is suggested that LSW exerts anti-inflammatory effects by regulating the TLR4/NF-κB signaling pathway.
4.2.5 Rhein
Rhein is widely found in a variety of Chinese herbs, including Rhubarb palmatum, aloe curacao, cassia stenophyllum, and polygonum multiflorum. It has antioxidant, antiviral, anti-inflammatory, anti-tumor, and immunomodulatory activities (108). In mice infected with PR8, rhein significantly improved survival and reduced the lung index and lung expression levels of IL-1β, IL-6, IL-8, and TNF-α. In addition, rhein significantly reduced the protein levels of TLR2, TLR3, and TLR4 and the phosphorylation of NF-κB p65 in PR8-infected cells. The addition of TLR4 and NF-κB activators can antagonize the inhibitory effect of rhein on viral replication (109), so the anti-inflammatory effect of rhein may be related to inhibiting the activation of the TLRs/NF-κB signaling pathway.
4.2.6 Flavonoids
H. cordatum Thunb. is an important plant medicine with antiviral, antibacterial, anti-inflammatory, and antioxidant activities (110–112). Flavonoids, one of the effective components of this phytomedicine, can reduce lung and intestinal damage in mice, inhibit the over-release of tumor necrosis factor-α, IL-1, IL-8, and MCP-1 in the lung tissue of infected mice, and inhibit the up-regulated expression of TLR and NF-κB p65 proteins (113). HCP may play an anti-inflammatory role by inhibiting the activation of the TLR/NF-κB signaling pathway (Table 2).
There are studies that Xiaoshuantongmai decoction can mediate microRNA-181b intervention in deep-vein thrombosis. According to the equivalent dose ratio of kg body weight of human and animal, the drug dose was converted, and based on the deep-vein thrombosis model, normal saline and Xiaoshuantongmai decoction were administered. The results showed that the expression level of miR-181b in the venous endothelium of the Xiaoshuantongmai Tang group was the highest, followed by the blank control group and the sham operation group, and the lowest was in the model control group, with a statistical difference. It was proved that Xiaoshuantongmai decoction can up-regulate the expression of miR-181b in the venous endothelium of DVT model rats (78).
4.3 Anticoagulant and antiplatelet agents inhibit thrombosis by regulating the NF-κB signaling pathway
4.3.1 Aspirin and salicylate
Acute pulmonary thromboembolism (APE) is a disorder of pulmonary circulation caused by a blockage of the pulmonary artery. Extensive inflammatory responses have been demonstrated in the lung tissue of APE rats, accompanied by significantly elevated levels of tumor necrosis factor-α, interleukin-1-β, and IL-8 (114, 115). High levels of NF-κB were also observed in rats after APE induction (116, 117). Aspirin and salicylate have been reported to inhibit NF-κB equally (118, 119). Healthy SD rats were randomly divided into a control group, sham operation group, APE model group, and aspirin low-dose, medium-dose, and high-dose groups. After APE induction for 6, 24, and 72 h, rats in the low-, medium-, and high-dose aspirin groups were given daily doses of aspirin at 150, 300, and 600 mg/kg, respectively, for 3 consecutive days. The other groups were given the same amount of normal saline. In the APE model group, thrombus formation, alveolar wall injury, pulmonary hemorrhage, and inflammatory cell infiltration occurred at all time points. After aspirin treatment, pathological changes such as pulmonary hemorrhage and inflammatory cell infiltration were reduced. Compared with the APE model group, the expression of the NF-κB protein measured by Western blotting was significantly decreased in other groups at each time point (P<0.05, P<0.001). The highest expression of the NF-κB protein was observed in the APE model group, and NF-κB protein expression decreased gradually in a dose-dependent manner in rats receiving aspirin (120). In summary, aspirin can significantly inhibit the NF-κB pathway in a dose-dependent manner to reduce inflammation and alleviate lung injury after APE.
4.3.2 Platelet P2Y12 receptor antagonist
Clinically, ticagrelor and clopidogrel (antiplatelet coagulants) are often combined with PCI for acute coronary syndromes (ACS) (121). They cure ACS12 adenosine diphosphate (ADP) receptors by targeting the platelet P2Y to inhibit platelet aggregation and reduce thrombosis (122). In this study, human umbilical vein endothelial cells (HUVECs) were cultured with ticagrelor or clopidogrel and given lipopolysaccharide (LPS) and CD14. Human umbilical vein endothelial cells (HUVECs) were cultured with ticagrelor or clopidogrel and given lipopolysaccharide (LPS) and CD14. Ticagrelor and clopidogrel reduce the expression of TNF-α, IL-1, IL-6, IL-8, and IL-2, inhibit p65 phosphorylation and IKB-α degradation, and significantly reduce the amount of nuclear translocation p65 (123, 124). These findings suggest that ticagrelor and clopidogrel inhibit the production of inflammatory cytokines by inhibiting the NF-KB pathway.
4.3.3 The PAR-1 antagonist
PAR-1 can be activated by thrombin to regulate platelet aggregation and endothelial permeability, so it is clinically used as a target for anti-platelet drugs to prevent thrombosis (125–127). Vorapaxar is a representative drug (128). IR induction was performed in rat lung models by perfusion in vitro. Male rats were treated with the specific PAR-1 antagonist vorapaxar or the control agent with 40 min of ischemia and 60 min of reperfusion. In vitro, mouse lung epithelial cells (MLE-12) were treated with vorapaxar and subjected to hypoxic reoxidation (HR). We found that vorapaxar reduced the production of thrombin, inflammatory factors, cytokine-induced neutrophil chemokine-1, interleukin-6, and tumor necrosis factor-α, pulmonary edema and neutrophil infiltration, and it alleviated lung cell apoptosis and down-regulated the nuclear factor-κB (NF-κB) pathway. It also blocked HR-induced NF-κB activation and the production of inflammatory chemokines in MLE12 cells. The results suggest that vorapaxar acts by blocking PAR-1 expression and modulating the NF-κB pathway (129) (Table 3).
5 Limitations in inhibiting NF-κB signaling pathways
At present, the targeted inhibition of the NF-κB signaling pathway for the treatment of thrombus has not reached the most perfect degree. On the one hand, these drugs/compounds can inhibit the inflammatory response and inhibit the expression of NF-κB, but there is no clear evidence of a targeted relationship, and studies have shown that combination drugs work better (130) and are a more clinically promising therapeutic strategy.
On the other hand, inhibition of the NF-κB pathway is a double-edged sword due to its broad effects. NF-κB mediates cell survival, cell differentiation, and cell proliferation (131), and inhibition of NF-κB has also been shown to play an important role in cancer treatment (132–135). However, long-term use of NF-κB inhibitors can cause side effects such as immune deficiency (136, 137) and intestinal homeostasis imbalance (138), and NF-κB inhibitors should be treated with for a short period. An ideal NF-κB inhibitor would only target the NF-κB pathway without affecting other signaling pathways. However, NF-κB inhibitors interfere with the NF-κB pathway by interfering with other pathways, such as PI3K/Akt and MAPK signaling (87, 115, 117).
6 Conclusion
In conclusion, the NF-κB signaling pathway plays an important role in inflammation and deep-vein thrombosis, and the regulation of the NF-κB pathway may bring new strategies for the treatment of thrombosis. Although the role of NF-κB signaling in venous thrombosis has been extensively studied in recent years, the application of NF-κB inhibitors in the treatment of thrombosis has a long way to go, regulating miRNAs or using drugs to interfere with the NF-κB signaling pathway. It may be a potential therapeutic option to improve thrombosis, but the dose and side effects of medication and whether regulating miRNAs will improve other downstream pathways of NF-κB signaling remain to be explored. Given the relationship between inflammation and blood clots, preventing inflammation is a better way to reduce blood clots. Therefore, in-depth study of the mechanism of the NF-κB signaling pathway inducing inflammation will help to elucidate the pathogenesis of venous thrombosis and will also have a far-reaching impact on the development of safer and more effective drugs and the prevention and treatment of thrombosis.
Author contributions
ZW: Writing – original draft, Writing – review & editing. CF: Investigation, Writing – review & editing. MY: Conceptualization, Investigation, Writing – review & editing. DW: Supervision, Writing – review & editing. MC: Supervision, Writing – review & editing. TG: Supervision, Writing – review & editing, Investigation. JM: Investigation, Supervision, Validation, Writing – review & editing.
Funding
The authors declare financial support was received for the research, authorship, and/or publication of this article. This research was supported by the National Natural Science Foundation of China (Approval/Award No. 82160375), the Natural Science Foundation of Jiangxi Province (Approval/Award No. 20202BABL206035), and the project funded by the Department of Education of Jiangxi Province (Approval/Award No. GJJ180811).
Conflict of interest
The authors declare that the research was conducted in the absence of any commercial or financial relationships that could be construed as a potential conflict of interest.
Publisher’s note
All claims expressed in this article are solely those of the authors and do not necessarily represent those of their affiliated organizations, or those of the publisher, the editors and the reviewers. Any product that may be evaluated in this article, or claim that may be made by its manufacturer, is not guaranteed or endorsed by the publisher.
References
1. Di Nisio M, van Es N, Büller HR. Deep vein thrombosis and pulmonary embolism. Lancet (2016) 388:3060–73. doi: 10.1016/S0140-6736(16)30514-1
2. Áinle FN, Kevane B. Which patients are at high risk of recurrent venous thromboembolism (deep vein thrombosis and pulmonary embolism)? Hematol Am Soc Hematol Educ Program (2020) 2020:201–12. doi: 10.1182/hematology.2020002268
3. Tritschler T, Kraaijpoel N, Le Gal G, Wells PS. Venous thromboembolism: advances in diagnosis and treatment. JAMA (2018) 320:1583–94. doi: 10.1001/jama.2018.14346
4. Blann AD, Lip GY. Virchow’s triad revisited: the importance of soluble coagulation factors, the endothelium, and platelets. Thromb Res (2001) 101:321–7. doi: 10.1016/s0049-3848(00)00419-9
5. Gimbrone MA, García-Cardeña G. Vascular endothelium, hemodynamics, and the pathobiology of atherosclerosis. Cardiovasc Pathol (2013) 22:9–15. doi: 10.1016/j.carpath.2012.06.006
6. Cines DB, Pollak ES, Buck CA, Loscalzo J, Zimmerman GA, McEver RP, et al. Endothelial cells in physiology and in the pathophysiology of vascular disorders. Blood (1998) 91:3527–61.
7. Resnick N, Yahav H, Shay-Salit A, Shushy M, Schubert S, Zilberman LCM, et al. Fluid shear stress and the vascular endothelium: for better and for worse. Prog Biophys Mol Biol (2003) 81:177–99. doi: 10.1016/s0079-6107(02)00052-4
8. Lehoux S, Tedgui A. Cellular mechanics and gene expression in blood vessels. J Biomech (2003) 36:631–43. doi: 10.1016/s0021-9290(02)00441-4
9. Chien S, Li S, Shyy YJ. Effects of mechanical forces on signal transduction and gene expression in endothelial cells. Hypertension (1998) 31:162–9. doi: 10.1161/01.hyp.31.1.162
10. Resnick N, Collins T, Atkinson W, Bonthron DT, Dewey CF, Gimbron MA. Platelet-derived growth factor B chain promoter contains a cis-acting fluid shear-stress-responsive element. Proc Natl Acad Sci U.S.A. (1993) 90:7908. doi: 10.1073/pnas.90.16.7908-d
11. Korenaga R, Ando J, Kosaki K, Isshiki M, Takada Y, Kamiya A. Negative transcriptional regulation of the VCAM-1 gene by fluid shear stress in murine endothelial cells. Am J Physiol (1997) 273:C1506–1515. doi: 10.1152/ajpcell.1997.273.5.c1506
12. Davies PF. Flow-mediated endothelial mechanotransduction. Physiol Rev (1995) 75:519–60. doi: 10.1152/physrev.1995.75.3.519
13. Hahn C, Schwartz MA. Mechanotransduction in vascular physiology and atherogenesis. Nat Rev Mol Cell Biol (2009) 10:53–62. doi: 10.1038/nrm2596
14. Nigro P, Abe J-I, Berk BC. Flow shear stress and atherosclerosis: a matter of site specificity. Antioxid Redox Signal (2011) 15:1405–14. doi: 10.1089/ars.2010.3679
15. Chan N, Eikelboom J. Hypercoagulability and thrombosis in COVID-19: a modifiable cause for mortality? Eur Heart J (2021) 42:3143–5. doi: 10.1093/eurheartj/ehab417
16. Piazza G. Cardiology patient page. Thrombophilia and hypercoagulability. Circulation (2014) 130:e9–10. doi: 10.1161/CIRCULATIONAHA.113.007665
17. Salomon O, Steinberg DM, Zivelin A, Gitel S, Dardik R, Rosenberg N, et al. Single and combined prothrombotic factors in patients with idiopathic venous thromboembolism: prevalence and risk assessment. Arterioscler Thromb Vasc Biol (1999) 19:511–8. doi: 10.1161/01.atv.19.3.511
18. Vandenbroucke JP, Koster T, Briët E, Reitsma PH, Bertina RM, Rosendaal FR. Increased risk of venous thrombosis in oral-contraceptive users who are carriers of factor V Leiden mutation. Lancet (1994) 344:1453–7. doi: 10.1016/s0140-6736(94)90286-0
19. Anderson JAM, Weitz JI. Hypercoagulable states. Crit Care Clin (2011) 27:933–52. doi: 10.1016/j.ccc.2011.09.007
20. Iba T, Helms J, Levi M, Levy JH. Thromboinflammation in acute injury: infections, heatstroke, and trauma. J Thromb Haemost (2023) 21:S1538-7836(23)00583-4. doi: 10.1016/j.jtha.2023.07.020
21. Fischetti F, Tedesco F. Cross-talk between the complement system and endothelial cells in physiologic conditions and in vascular diseases. Autoimmunity (2006) 39:417–28. doi: 10.1080/08916930600739712
22. Pilard M, Ollivier EL, Gourdou-Latyszenok V, Couturaud F, Lemarié CA. Endothelial cell phenotype, a major determinant of venous thrombo-inflammation. Front Cardiovasc Med (2022) 9:864735. doi: 10.3389/fcvm.2022.864735
23. Lopatko Fagerström I, Ståhl A-L, Mossberg M, Tati R, Kristoffersson A-C, Kahn R, et al. Blockade of the kallikrein-kinin system reduces endothelial complement activation in vascular inflammation. EBioMedicine (2019) 47:319–28. doi: 10.1016/j.ebiom.2019.08.020
24. Sano M, Takahashi R, Ijichi H, Ishigaki K, Yamada T, Miyabayashi K, et al. Blocking VCAM-1 inhibits pancreatic tumour progression and cancer-associated thrombosis/thromboembolism. Gut (2021) 70:1713–23. doi: 10.1136/gutjnl-2020-320608
25. Kong D-H, Kim YK, Kim MR, Jang JH, Lee S. Emerging roles of vascular cell adhesion molecule-1 (VCAM-1) in immunological disorders and cancer. Int J Mol Sci (2018) 19:1057. doi: 10.3390/ijms19041057
26. Gilmore TD. Introduction to NF-kappaB: players, pathways, perspectives. Oncogene (2006) 25:6680–4. doi: 10.1038/sj.onc.1209954
27. Hayden MS, Ghosh S. Shared principles in NF-kappaB signaling. Cell (2008) 132:344–62. doi: 10.1016/j.cell.2008.01.020
28. Vallabhapurapu S, Karin M. Regulation and function of NF-kappaB transcription factors in the immune system. Annu Rev Immunol (2009) 27:693–733. doi: 10.1146/annurev.immunol.021908.132641
29. Bonizzi G, Karin M. The two NF-kappaB activation pathways and their role in innate and adaptive immunity. Trends Immunol (2004) 25:280–8. doi: 10.1016/j.it.2004.03.008
30. Ghosh S, Hayden MS. New regulators of NF-kappaB in inflammation. Nat Rev Immunol (2008) 8:837–48. doi: 10.1038/nri2423
31. Sun S-C. The non-canonical NF-κB pathway in immunity and inflammation. Nat Rev Immunol (2017) 17:545–58. doi: 10.1038/nri.2017.52
32. Sun S-C. Non-canonical NF-κB signaling pathway. Cell Res (2011) 21:71–85. doi: 10.1038/cr.2010.177
33. Xiao G, Harhaj EW, Sun SC. NF-kappaB-inducing kinase regulates the processing of NF-kappaB2 p100. Mol Cell (2001) 7:401–9. doi: 10.1016/s1097-2765(01)00187-3
34. Gupta SC, Kim JH, Prasad S, Aggarwal BB. Regulation of survival, proliferation, invasion, angiogenesis, and metastasis of tumor cells through modulation of inflammatory pathways by nutraceuticals. Cancer Metastasis Rev (2010) 29:405–34. doi: 10.1007/s10555-010-9235-2
35. Gupta SC, Sundaram C, Reuter S, Aggarwal BB. Inhibiting NF-κB activation by small molecules as a therapeutic strategy. Biochim Biophys Acta (2010) 1799:775–87. doi: 10.1016/j.bbagrm.2010.05.004
36. Luo W, Li Y-X, Jiang L-J, Chen Q, Wang T, Ye D-W. Targeting JAK-STAT signaling to control cytokine release syndrome in COVID-19. Trends Pharmacol Sci (2020) 41:531–43. doi: 10.1016/j.tips.2020.06.007
37. Ackermann M, Verleden SE, Kuehnel M, Haverich A, Welte T, Laenger F, et al. Pulmonary vascular endothelialitis, thrombosis, and angiogenesis in covid-19. N Engl J Med (2020) 383:120–8. doi: 10.1056/NEJMoa2015432
38. Mo J, Huang H, Zhang C, Wang B, He F, Yin L, et al. To study influence of JAK-STAT signaling pathway in traumatic deep vein thrombosis. ZHONG GUO WU ZHEN XUE ZA ZHI (2007) 7:6212–4.
39. Zhuang S. The Study of Inflammation Factors in the Mechanism of Deep Venous Thrombosis (2005). Naval Medical University. Available at: https://kns.cnki.net/kcms2/article/abstract?v=3uoqIhG8C447WN1SO36whBaOoOkzJ23ELn_-3AAgJ5enmUaXDTPHrESUbr8kKkBIzUTQYESjzuMjzMXdnFBjhD9MuVtV0mqx&uniplatform=NZKPT (Accessed August 15, 2023). A dissertation submitted for the Degree of Doctor of Medicine.
40. Ren B, Yan F, Deng Z, Zhang S, Xiao L, Wu M, et al. Extremely high incidence of lower extremity deep venous thrombosis in 48 patients with severe COVID-19 in Wuhan. Circulation (2020) 142:181–3. doi: 10.1161/CIRCULATIONAHA.120.047407
41. Zhang L, Feng X, Zhang D, Jiang C, Mei H, Wang J, et al. Deep vein thrombosis in hospitalized patients with COVID-19 in Wuhan, China: prevalence, risk factors, and outcome. Circulation (2020) 142:114–28. doi: 10.1161/CIRCULATIONAHA.120.046702
42. Gorog DA, Storey RF, Gurbel PA, Tantry US, Berger JS, Chan MY, et al. Current and novel biomarkers of thrombotic risk in COVID-19: a Consensus Statement from the International COVID-19 Thrombosis Biomarkers Colloquium. Nat Rev Cardiol (2022) 19:475–95. doi: 10.1038/s41569-021-00665-7
43. Miesbach W, Makris M. COVID-19: coagulopathy, risk of thrombosis, and the rationale for anticoagulation. Clin Appl Thromb Hemost (2020) 26:1076029620938149. doi: 10.1177/1076029620938149
44. Flaumenhaft R, Enjyoji K, Schmaier AA. Vasculopathy in COVID-19. Blood (2022) 140:222–35. doi: 10.1182/blood.2021012250
45. Scully M, Singh D, Lown R, Poles A, Solomon T, Levi M, et al. Pathologic Antibodies to Platelet Factor 4 after ChAdOx1 nCoV-19 Vaccination. N Engl J Med (2021) 384:2202–11. doi: 10.1056/NEJMoa2105385
47. Gupta A, Sardar P, Cash ME, Milani RV, Lavie CJ. Covid-19 vaccine- induced thrombosis and thrombocytopenia-a commentary on an important and practical clinical dilemma. Prog Cardiovasc Dis (2021) 67:105–7. doi: 10.1016/j.pcad.2021.05.001
48. Hippisley-Cox J, Patone M, Mei XW, Saatci D, Dixon S, Khunti K, et al. Risk of thrombocytopenia and thromboembolism after covid-19 vaccination and SARS-CoV-2 positive testing: self-controlled case series study. BMJ (2021) 374:n1931. doi: 10.1136/bmj.n1931
49. Kim AY, Woo W, Yon DK, Lee SW, Yang JW, Kim JH, et al. Corrigendum to “Thrombosis patterns and clinical outcome of COVID-19 vaccine-induced immune thrombotic thrombocytopenia: A Systematic Review and Meta-Analysis” International Journal of Infectious Diseases, Volume 119, June 2022, Page 130-139. Int J Infect Dis (2022) 123:166. doi: 10.1016/j.ijid.2022.08.025
50. Hwang J, Lee SB, Lee SW, Lee MH, Koyanagi A, Jacob L, et al. Comparison of vaccine-induced thrombotic events between ChAdOx1 nCoV-19 and Ad26.COV.2.S vaccines. J Autoimmun (2021) 122:102681. doi: 10.1016/j.jaut.2021.102681
51. Ding P. The role and mechanism of IL-17A in the formation of deep vein thrombosis (2018). Huazhong University of Science and Technology. Available at: https://kns.cnki.net/kcms2/article/abstract?v=3uoqIhG8C447WN1SO36whLpCgh0R0Z-iDdIt-WSAdV5IJ_Uy2HKRAR6bP3qoZUcgSakIAbnlVhhMH0B_SQEPiC6IXYLGCRSz&uniplatform=NZKPT (Accessed August 15, 2023). A dissertation submitted for the Degree of Doctor of Medicine.
52. Zhang Y, Zhang Z, Wei R, Miao X, Sun S, Liang G, et al. IL (Interleukin)-6 contributes to deep vein thrombosis and is negatively regulated by miR-338-5p. Arterioscler Thromb Vasc Biol (2020) 40:323–34. doi: 10.1161/ATVBAHA.119.313137
53. Sierra-Mondragón E. Low expression of IL-6 and TNF-α correlates with the presence of the nuclear regulators of NF-κB, IκBNS and BCL-3, in the uterus of mice. Mol Immunol (2015) 68:333–40. doi: 10.1016/j.molimm.2015.09.020
54. Zheng X, Liu H, Ma M, Ji J, Zhu F, Sun L. Anti-thrombotic activity of phenolic acids obtained from Salvia miltiorrhiza f. alba in TNF-α-stimulated endothelial cells via the NF-κB/JNK/p38 MAPK signaling pathway. Arch Pharm Res (2021) 44:427–38. doi: 10.1007/s12272-021-01325-7
55. Guo T, Shi J, Chen X, Zeng J, Zhang F, Dai P, et al. The effect of COX-2 targeting interference on TDVT in rats. Orthopaedic Biomechanics Materials Clin Study (2022) 19:11–14+19.
56. Mussbacher M, Salzmann M, Brostjan C, Hoesel B, Schoegenhofer C, Datler H, et al. Cell type-specific roles of NF-κB linking inflammation and thrombosis. Front Immunol (2019) 10:85. doi: 10.3389/fimmu.2019.00085
57. Li YS, Shyy YJ, Wright JG, Valente AJ, Cornhill JF, Kolattukudy PE. The expression of monocyte chemotactic protein (MCP-1) in human vascular endothelium in vitro and in vivo. Mol Cell Biochem (1993) 126:61–8. doi: 10.1007/BF01772208
58. Deshmane SL, Kremlev S, Amini S, Sawaya BE. Monocyte chemoattractant protein-1 (MCP-1): an overview. J Interferon Cytokine Res (2009) 29:313–26. doi: 10.1089/jir.2008.0027
59. Albayati MA, Grover SP, Saha P, Lwaleed BA, Modarai B, Smith A. Postsurgical inflammation as a causative mechanism of venous thromboembolism. Semin Thromb Hemost (2015) 41:615–20. doi: 10.1055/s-0035-1556726
60. Delekta PC, Apel IJ, Gu S, Siu K, Hattori Y, McAllister-Lucas LM, et al. Thrombin-dependent NF-κB activation and monocyte/endothelial adhesion are mediated by the CARMA3·Bcl10·MALT1 signalosome. J Biol Chem (2010) 285:41432–42. doi: 10.1074/jbc.M110.158949
61. Margetic S. Inflammation and haemostasis. Biochem Med (Zagreb) (2012) 22:49–62. doi: 10.11613/BM.2012.006
62. Stakos DA, Kambas K, Konstantinidis T, Mitroulis I, Apostolidou E, Arelaki S, et al. Expression of functional tissue factor by neutrophil extracellular traps in culprit artery of acute myocardial infarction. Eur Heart J (2015) 36:1405–14. doi: 10.1093/eurheartj/ehv007
63. Rahman A, Fazal F. Blocking NF-κB: an inflammatory issue. Proc Am Thorac Soc (2011) 8:497–503. doi: 10.1513/pats.201101-009MW
64. Verhamme P, Hoylaerts MF. The pivotal role of the endothelium in haemostasis and thrombosis. Acta Clin Belg (2006) 61:213–9. doi: 10.1179/acb.2006.036
65. Chang M, Guo F, Zhou Z, Huang X, Yi L, Dou Y, et al. HBP induces the expression of monocyte chemoattractant protein-1 via the FAK/PI3K/AKT and p38 MAPK/NF-κB pathways in vascular endothelial cells. Cell Signal (2018) 43:85–94. doi: 10.1016/j.cellsig.2017.12.008
66. Xu XR, Zhang D, Oswald BE, Carrim N, Wang X, Hou Y, et al. Platelets are versatile cells: New discoveries in hemostasis, thrombosis, immune responses, tumor metastasis and beyond. Crit Rev Clin Lab Sci (2016) 53:409–30. doi: 10.1080/10408363.2016.1200008
67. Yau JW, Teoh H, Verma S. Endothelial cell control of thrombosis. BMC Cardiovasc Disord (2015) 15:130. doi: 10.1186/s12872-015-0124-z
68. Urban CF, Ermert D, Schmid M, Abu-Abed U, Goosmann C, Nacken W, et al. Neutrophil extracellular traps contain calprotectin, a cytosolic protein complex involved in host defense against Candida albicans. PloS Pathog (2009) 5:e1000639. doi: 10.1371/journal.ppat.1000639
69. Cao W, Shao Z, Duan M, Gao L, Zhang Y, Xu X. Research progress of neutrophil extracellular trap ( NETs) in related inflammatory diseases. ZHONG GUO MIAN YI XUE ZA ZHI (2019) 35:635–8.
70. Thailin C, Hisada Y, Lundstrom S, Mackman N, Wallen H. Neutrophil extracellular traps: villains and targets in arterial, venous, and cancer-associated thrombosis. Arteriosclerosis thrombosis Vasc Biol (2019) 39:1724–38. doi: 10.1161/ATVBAHA.119.312463
71. Klier M, Gowert NS, Jäckel S, Reinhardt C, Elvers M. Phospholipase D1 is a regulator of platelet-mediated inflammation. Cell Signal (2017) 38:171–81. doi: 10.1016/j.cellsig.2017.07.007
72. Mackman N. New insights into the mechanisms of venous thrombosis. J Clin Invest (2012) 122:2331–6. doi: 10.1172/JCI60229
73. Cheng Z, Jia W, Tian X, Jiang P, Zhang Y, Li J, et al. Cotinine inhibits TLR4/NF-κB signaling pathway and improves deep vein thrombosis in rats. Biosci Rep (2020) 40:BSR20201293. doi: 10.1042/BSR20201293
74. Starikova I, Jamaly S, Sorrentino A, Blondal T, Latysheva N, Sovershaev M, et al. Differential expression of plasma miRNAs in patients with unprovoked venous thromboembolism and healthy control individuals. Thromb Res (2015) 136:566–72. doi: 10.1016/j.thromres.2015.07.005
75. Salloum-Asfar S, Teruel-Montoya R, Arroyo AB, García-Barberá N, Chaudhry A, Schuetz E, et al. Regulation of coagulation factor XI expression by microRNAs in the human liver. PloS One (2014) 9:e111713. doi: 10.1371/journal.pone.0111713
76. Chen R, Chen S, Liao J, Chen X, Xu X. MiR-145 facilitates proliferation and migration of endothelial progenitor cells and recanalization of arterial thrombosis in cerebral infarction mice via JNK signal pathway. Int J Clin Exp Pathol (2015) 8:13770–6.
77. Cz C LL, Hf L, Dp B. MicroRNAs modulate hematopoietic lineage differentiation. Sci (New York NY) (2004) 303:83–6. doi: 10.1126/science.1091903
78. Pang X. Study on the Mechanism of Xiao Shuan Tong Mai Decoction Intervening Deep Vein Thrombosis by Mediating microRNA-181b Regulating NF-κB Signal Pathway. Shandong University of Traditional Chinese Medicine (2020). A dissertation submitted for the Degree of Doctor of Medicine. doi: 10.27282/d.cnki.gsdzu.2020.000031
79. Tian Y, Li X, Bai C, Yang Z, Zhang L, Luo J. Regulation of microRNA-150 on inflammatory response in rat models of deep vein thrombosis. Genomics Appl Biol (2020) 39:4753–60. doi: 10.13417/j.gab.039.004753
80. Feng Q, Xu C, Sun M. miRNA-141 inhibits thrombosis in vascular pathways through the TLR4 signaling pathway. XIBU YIXUE (2021) 33:186–90.
81. Zhang Q, Lenardo MJ, Baltimore D. 30 years of NF-κB: A blossoming of relevance to human pathobiology. Cell (2017) 168:37–57. doi: 10.1016/j.cell.2016.12.012
82. Guo Z, Lou Y, Kong M, Luo Q, Liu Z, Wu J. A systematic review of phytochemistry, pharmacology and pharmacokinetics on astragali radix: implications for astragali radix as a personalized medicine. Int J Mol Sci (2019) 20:1463. doi: 10.3390/ijms20061463
83. Li X, Qu L, Dong Y, Han L, Liu E, Fang S, et al. A review of recent research progress on the astragalus genus. Molecules (2014) 19:18850–80. doi: 10.3390/molecules191118850
84. Zhang K, Pugliese M, Pugliese A, Passantino A. Biological active ingredients of traditional Chinese herb Astragalus membranaceus on treatment of diabetes: a systematic review. Mini Rev Med Chem (2015) 15:315–29. doi: 10.2174/1389557515666150227113431
85. Liu T, Zhang M, Niu H, Liu J, Ruilian M, Wang Y, et al. Astragalus polysaccharide from Astragalus Melittin ameliorates inflammation via suppressing the activation of TLR-4/NF-κB p65 signal pathway and protects mice from CVB3-induced virus myocarditis. Int J Biol Macromol (2019) 126:179–86. doi: 10.1016/j.ijbiomac.2018.12.207
86. Chu X, Zhu Y, Su K, Zhang T, Ma Y. Protective effect of Qihong Tongluo prescription on vascular endothelial cells in rats with deep venous thrombosis based on NF-κB pathway. Chin J Exp Traditional Med Formulae (2023) 29:60–8. doi: 10.13422/j.cnki.syfjx.20230495
87. Yu G, Luo Z, Zhou Y, Zhang L, Wu Y, Ding L, et al. Uncovering the pharmacological mechanism of Carthamus tinctorius L. @ on cardiovascular disease by a systems pharmacology approach. BioMed Pharmacother (2019) 117:109094. doi: 10.1016/j.biopha.2019.109094
88. Cao J, Chen Z, Zhu Y, Li Y, Guo C, Gao K, et al. Huangqi-Honghua combination and its main components ameliorate cerebral infarction with Qi deficiency and blood stasis syndrome by antioxidant action in rats. J Ethnopharmacol (2014) 155:1053–60. doi: 10.1016/j.jep.2014.05.061
89. Li R, Chen Y, Shi M, Xu X, Zhao Y, Wu X, et al. Gegen Qinlian decoction alleviates experimental colitis via suppressing TLR4/NF-κB signaling and enhancing antioxidant effect. Phytomedicine (2016) 23:1012–20. doi: 10.1016/j.phymed.2016.06.010
90. Choo M-K, Park E-K, Yoon H-K, Kim D-H. Antithrombotic and antiallergic activities of daidzein, a metabolite of puerarin and daidzin produced by human intestinal microflora. Biol Pharm Bull (2002) 25:1328–32. doi: 10.1248/bpb.25.1328
91. Zhang Y, Ma X, Guo C, Wang M, Kou N, Qu H, et al. Pretreatment with a combination of ligustrazine and berberine improves cardiac function in rats with coronary microembolization. Acta Pharmacol Sin (2016) 37:463–72. doi: 10.1038/aps.2015.147
92. Wei X, Zhang B, Wei F, Ding M, Luo Z, Han X, et al. Gegen Qinlian pills alleviate carrageenan-induced thrombosis in mice model by regulating the HMGB1/NF-κB/NLRP3 signaling. Phytomedicine (2022) 100:154083. doi: 10.1016/j.phymed.2022.154083
93. He T, Wang M, Kong J, Wang Q, Tian Y, Li C, et al. Integrating network pharmacology and non-targeted metabolomics to explore the common mechanism of Coptis Categorized Formula improving T2DM zebrafish. J Ethnopharmacol (2022) 284:114784. doi: 10.1016/j.jep.2021.114784
94. Fu Y-J, Xu B, Huang S-W, Luo X, Deng X-L, Luo S, et al. Baicalin prevents LPS-induced activation of TLR4/NF-κB p65 pathway and inflammation in mice via inhibiting the expression of CD14. Acta Pharmacol Sin (2021) 42:88–96. doi: 10.1038/s41401-020-0411-9
95. Jiang M, Li Z, Zhu G. Immunological regulatory effect of flavonoid baicalin on innate immune toll-like receptors. Pharmacol Res (2020) 158:104890. doi: 10.1016/j.phrs.2020.104890
96. Shen J, Cheng J, Zhu S, Zhao J, Ye Q, Xu Y, et al. Regulating effect of baicalin on IKK/IKB/NF-kB signaling pathway and apoptosis-related proteins in rats with ulcerative colitis. Int Immunopharmacol (2019) 73:193–200. doi: 10.1016/j.intimp.2019.04.052
97. Zeng A, Liang X, Zhu S, Liu C, Luo X, Zhang Q, et al. Baicalin, a potent inhibitor of NF-κB signaling pathway, enhances chemosensitivity of breast cancer cells to docetaxel and inhibits tumor growth and metastasis both in vitro and in vivo. Front Pharmacol (2020) 11:879. doi: 10.3389/fphar.2020.00879
98. Liu S-J, Yin C-X, Ding M-C, Wang Y-Z, Wang H. Berberine inhibits tumor necrosis factor-α-induced expression of inflammatory molecules and activation of nuclear factor-κB via the activation of AMPK in vascular endothelial cells. Mol Med Rep (2015) 12:5580–6. doi: 10.3892/mmr.2015.4061
99. Kuo C-L, Chi C-W, Liu T-Y. The anti-inflammatory potential of berberine in vitro and in vivo. Cancer Lett (2004) 203:127–37. doi: 10.1016/j.canlet.2003.09.002
100. Li C, Ai G, Wang Y, Lu Q, Luo C, Tan L, et al. Oxyberberine, a novel gut microbiota-mediated metabolite of berberine, possesses superior anti-colitis effect: Impact on intestinal epithelial barrier, gut microbiota profile and TLR4-MyD88-NF-κB pathway. Pharmacol Res (2020) 152:104603. doi: 10.1016/j.phrs.2019.104603
101. Wu Y, Li J, Kim Y, Wu J, Wang Q, Hao Y. In vivo and in vitro antiviral effects of berberine on influenza virus. Chin J Integr Med (2011) 17:444–52. doi: 10.1007/s11655-011-0640-3
102. Yan Y-Q, Fu Y-J, Wu S, Qin H-Q, Zhen X, Song B-M, et al. Anti-influenza activity of berberine improves prognosis by reducing viral replication in mice. Phytother Res (2018) 32:2560–7. doi: 10.1002/ptr.6196
103. Liu J JIES, Chen B, Zeng H, MA Y. Mechanisms of Huanglian Jiedu decoction in treating acute gouty arthritis based on NLRP3 inflammasome and TLR4/NF-κB signal pathway. Chin J Exp Traditional Med Formulae (2023), 1–9. doi: 10.13422/j.cnki.syfjx.20230802
104. Liu H, Chen X, Liu Y, Fang C, Chen S. Antithrombotic effects of Huanglian Jiedu decoction in a rat model of ischaemia-reperfusion-induced cerebral stroke. Pharm Biol (2021) 59:823–7. doi: 10.1080/13880209.2021.1942505
105. Ma H-Y, Kou J-P, Wang J-R, Yu B-Y. Evaluation of the anti-inflammatory and analgesic activities of Liu-Shen-Wan and its individual fractions. J Ethnopharmacol (2007) 112:108–14. doi: 10.1016/j.jep.2007.02.008
106. Zhang L, Ye X, Liu Y, Zhang Z, Xia X, Dong S. Research progress on the effect of traditional Chinese medicine on the activation of PRRs-mediated NF-κB signaling pathway to inhibit influenza pneumonia. Front Pharmacol (2023) 14:1132388. doi: 10.3389/fphar.2023.1132388
107. Ma Q, Huang W, Zhao J, Yang Z. Liu Shen Wan inhibits influenza a virus and excessive virus-induced inflammatory response via suppression of TLR4/NF-κB signaling pathway in vitro and in vivo. J Ethnopharmacol (2020) 252:112584. doi: 10.1016/j.jep.2020.112584
108. Sun H, Luo G, Chen D, Xiang Z. A comprehensive and system review for the pharmacological mechanism of action of rhein, an active anthraquinone ingredient. Front Pharmacol (2016) 7:247. doi: 10.3389/fphar.2016.00247
109. Wang Q-W, Su Y, Sheng J-T, Gu L-M, Zhao Y, Chen X-X, et al. Anti-influenza A virus activity of rhein through regulating oxidative stress, TLR4, Akt, MAPK, and NF-κB signal pathways. PloS One (2018) 13:e0191793. doi: 10.1371/journal.pone.0191793
110. Li W, Fan T, Zhang Y, Fan T, Zhou P, Niu X, et al. Houttuynia cordata Thunb. volatile oil exhibited anti-inflammatory effects in vivo and inhibited nitric oxide and tumor necrosis factor-α production in LPS-stimulated mouse peritoneal macrophages in vitro. Phytother Res (2013) 27:1629–39. doi: 10.1002/ptr.4905
111. Cheng B-H, Chan JY-W, Chan BC-L, Lin H-Q, Han X-Q, Zhou X, et al. Structural characterization and immunomodulatory effect of a polysaccharide HCP-2 from Houttuynia cordata. Carbohydr Polym (2014) 103:244–9. doi: 10.1016/j.carbpol.2013.12.048
112. Ling L, Ren A, Lu Y, Zhang Y, Zhu H, Tu P, et al. The synergistic effect and mechanisms of flavonoids and polysaccharides from Houttuynia cordata on H1N1-induced pneumonia in mice. J Ethnopharmacol (2023) 302:115761. doi: 10.1016/j.jep.2022.115761
113. Ling L-J, Lu Y, Zhang Y-Y, Zhu H-Y, Tu P, Li H, et al. Flavonoids from Houttuynia cordata attenuate H1N1-induced acute lung injury in mice via inhibition of influenza virus and Toll-like receptor signalling. Phytomedicine (2020) 67:153150. doi: 10.1016/j.phymed.2019.153150
114. Sun C, Wang L, Jiang H, Yang R. [Effects of aspirin on CX3CL1 and CX3CR1 in acute pulmonary embolism rats]. Zhonghua Yi Xue Za Zhi (2013) 93:69–72.
115. Wang L, Wu J, Zhang W, Zhi Y, Wu Y, Jiang R, et al. Effects of aspirin on the ERK and PI3K/Akt signaling pathways in rats with acute pulmonary embolism. Mol Med Rep (2013) 8:1465–71. doi: 10.3892/mmr.2013.1676
116. Lu W-J, Lee J-J, Chou D-S, Jayakumar T, Fong T-H, Hsiao G, et al. A novel role of andrographolide, an NF-kappa B inhibitor, on inhibition of platelet activation: the pivotal mechanisms of endothelial nitric oxide synthase/cyclic GMP. J Mol Med (Berl) (2011) 89:1261–73. doi: 10.1007/s00109-011-0800-0
117. Lu WJ, Lin KH, Hsu MJ, Chou DS, Hsiao G, Sheu JR. Suppression of NF-κB signaling by andrographolide with a novel mechanism in human platelets: regulatory roles of the p38 MAPK-hydroxyl radical-ERK2 cascade. Biochem Pharmacol (2012) 84:914–24. doi: 10.1016/j.bcp.2012.06.030
118. Frantz B, O’Neill EA. The effect of sodium salicylate and aspirin on NF-kappa B. Science (1995) 270:2017–9. doi: 10.1126/science.270.5244.2017
119. Kopp E, Ghosh S. Inhibition of NF-kappa B by sodium salicylate and aspirin. Science (1994) 265:956–9. doi: 10.1126/science.8052854
120. Wang L-C, Jiang R-L, Zhang W, Wei L-L, Yang R-H. Effects of aspirin on the expression of nuclear factor-κB in a rat model of acute pulmonary embolism. World J Emerg Med (2014) 5:229–33. doi: 10.5847/wjem.j.issn.1920-8642.2014.03.013
121. Pels K, Schwimmbeck PL, Rosenthal P, Loddenkemper C, Dang-Heine C, Rauch U, et al. Long-term clopidogrel administration following severe coronary injury reduces proliferation and inflammation via inhibition of nuclear factor-kappaB and activator protein 1 activation in pigs. Eur J Clin Invest (2009) 39:174–82. doi: 10.1111/j.1365-2362.2009.02089.x
122. Schneider DJ. Mechanisms potentially contributing to the reduction in mortality associated with ticagrelor therapy. J Am Coll Cardiol (2011) 57:685–7. doi: 10.1016/j.jacc.2010.11.016
123. Jia Z, Huang Y, Ji X, Sun J, Fu G. Ticagrelor and clopidogrel suppress NF-κB signaling pathway to alleviate LPS-induced dysfunction in vein endothelial cells. BMC Cardiovasc Disord (2019) 19:318. doi: 10.1186/s12872-019-01287-1
124. Liu X, Wang Y, Zhang M, Liu Y, Hu L, Gu Y. Ticagrelor reduces ischemia-reperfusion injury through the NF-κB-dependent pathway in rats. J Cardiovasc Pharmacol (2019) 74:13–9. doi: 10.1097/FJC.0000000000000675
125. Ramachandran R, Noorbakhsh F, Defea K, Hollenberg MD. Targeting proteinase-activated receptors: therapeutic potential and challenges. Nat Rev Drug Discovery (2012) 11:69–86. doi: 10.1038/nrd3615
126. Chambers RC, Scotton CJ. Coagulation cascade proteinases in lung injury and fibrosis. Proc Am Thorac Soc (2012) 9:96–101. doi: 10.1513/pats.201201-006AW
127. De Ceunynck K, Peters CG, Jain A, Higgins SJ, Aisiku O, Fitch-Tewfik JL, et al. PAR1 agonists stimulate APC-like endothelial cytoprotection and confer resistance to thromboinflammatory injury. Proc Natl Acad Sci U.S.A. (2018) 115:E982–91. doi: 10.1073/pnas.1718600115
128. Gupta R, Lin M, Mehta A, Aedma SK, Shah R, Ranchal P, et al. Protease-activated receptor antagonist for reducing cardiovascular events - A review on vorapaxar. Curr Probl Cardiol (2023) 48:101035. doi: 10.1016/j.cpcardiol.2021.101035
129. Chu S-J, Tang S-E, Pao H-P, Wu S-Y, Liao W-I. Protease-activated receptor-1 antagonist protects against lung ischemia/reperfusion injury. Front Pharmacol (2021) 12:752507. doi: 10.3389/fphar.2021.752507
130. Li F, Xu D, Hou K, Gou X, Lv N, Fang W, et al. Pretreatment of Indobufen and Aspirin and their Combinations with Clopidogrel or Ticagrelor Alleviates Inflammasome Mediated Pyroptosis via Inhibiting NF-κB/NLRP3 Pathway in Ischemic Stroke. J Neuroimmune Pharmacol (2021) 16:835–53. doi: 10.1007/s11481-020-09978-9
131. Cheng W, Cui C, Liu G, Ye C, Shao F, Bagchi AK, et al. NF-κB, A potential therapeutic target in cardiovascular diseases. Cardiovasc Drugs Ther (2023) 37:571–84. doi: 10.1007/s10557-022-07362-8
132. Yu H, Lin L, Zhang Z, Zhang H, Hu H. Targeting NF-κB pathway for the therapy of diseases: mechanism and clinical study. Signal Transduct Target Ther (2020) 5:209. doi: 10.1038/s41392-020-00312-6
133. Taniguchi K, Karin M. NF-κB, inflammation, immunity and cancer: coming of age. Nat Rev Immunol (2018) 18:309–24. doi: 10.1038/nri.2017.142
134. Rasmi RR, Sakthivel KM, Guruvayoorappan C. NF-κB inhibitors in treatment and prevention of lung cancer. BioMed Pharmacother (2020) 130:110569. doi: 10.1016/j.biopha.2020.110569
135. Patel M, Horgan PG, McMillan DC, Edwards J. NF-κB pathways in the development and progression of colorectal cancer. Transl Res (2018) 197:43–56. doi: 10.1016/j.trsl.2018.02.002
136. Pflug KM, Sitcheran R. Targeting NF-κB-inducing kinase (NIK) in immunity, inflammation, and cancer. Int J Mol Sci (2020) 21:8470. doi: 10.3390/ijms21228470
137. Barnabei L, Laplantine E, Mbongo W, Rieux-Laucat F, Weil R. NF-κB: at the borders of autoimmunity and inflammation. Front Immunol (2021) 12:716469. doi: 10.3389/fimmu.2021.716469
Keywords: thrombosis, NF-κB signal pathway, inflammation, miRNA, TCM, natural compounds, drugs
Citation: Wang Z, Fang C, Yao M, Wu D, Chen M, Guo T and Mo J (2023) Research progress of NF-κB signaling pathway and thrombosis. Front. Immunol. 14:1257988. doi: 10.3389/fimmu.2023.1257988
Received: 13 July 2023; Accepted: 06 September 2023;
Published: 29 September 2023.
Edited by:
Li Wu, Nanjing University of Chinese Medicine, ChinaReviewed by:
Anita Sahu, National Jewish Health, United StatesBhuvnesh Kumar, Sharda University, India
Copyright © 2023 Wang, Fang, Yao, Wu, Chen, Guo and Mo. This is an open-access article distributed under the terms of the Creative Commons Attribution License (CC BY). The use, distribution or reproduction in other forums is permitted, provided the original author(s) and the copyright owner(s) are credited and that the original publication in this journal is cited, in accordance with accepted academic practice. No use, distribution or reproduction is permitted which does not comply with these terms.
*Correspondence: Jianwen Mo, bWp3MTk5N0AxMjYuY29t