- 1The Second Clinical Medical College, Lanzhou University, Lanzhou, China
- 2Department of General Surgery, The Second Hospital of Lanzhou University, Lanzhou, China
- 3The First Clinical Medical College, Lanzhou University, Lanzhou, China
As a component of the innate immune system, there is emerging evidence to suggest that neutrophils may play a critical role in the initiation and progression of hepatocellular carcinoma (HCC). Neutrophil extracellular traps (NETs) are web-like chromatin structures that protrude from the membranes during neutrophil activation. Recent research has shown that NETs, which are at the forefront of the renewed interest in neutrophil studies, are increasingly intertwined with HCC. By exploring the mechanisms of NETs in HCC, we aim to improve our understanding of the role of NETs and gain deeper insights into neutrophil biology. Therefore, this article provides a summary of key findings and discusses the emerging field of NETs in HCC.
1 Introduction
Primary liver cancer is the third leading cause of cancer-related death worldwide (1). Hepatocellular carcinoma (HCC), the most common form of primary liver cancer, often develops in the setting of chronic liver disease and cirrhosis (2, 3). The progression of HCC involves the evasion of immune surveillance and the establishment of an immunosuppressive tumor microenvironment (TME), thereby inhibiting cytotoxic immune cells (4). HCC is characterized by a high infiltration of leukocytes from multiple immune cell lineages, which negatively affects effector lymphocyte activity and correlates with poor outcomes (5–7). Therefore, the current focus in HCC research lies not only on lymphocytes but also on exploring the interactions of multiple immune cells (4).
Neutrophils, one of the phagocytes, as the first line of defense against pathogen invasion by employing their potent antimicrobial arsenal and also contribute to the activation of adaptive immunity (8, 9). In addition, neutrophils play a critical role in tumor progression and tumorigenesis through the release of various mediators. Tumor-related inflammation, in contrast to conventional inflammation, promotes tumor initiation and growth by facilitating the evasion of immune surveillance (10). Neutrophils, as representatives of conventional inflammation, show distinct effects on the malignant phenotype of tumors, as supported by numerous lines of evidence (4, 11, 12).
Previous studies have focused primarily on the role of neutrophils as pathogen scavengers during conventional inflammation (13). However, it is now recognized that neutrophils have multiple and diverse functions (13). In humans, neutrophils represent 50-70% of all circulating leukocytes, whereas in mice they make up 10-25% (14, 15). Notably, neutrophils play a crucial role in chronic inflammation, specifically in chronic liver disease and malignancies, facilitating immune infiltration (16, 17). The challenge at hand is that tumor immunotherapy has shown limited efficacy in many patients with HCC, which represents a significant obstacle in the field (18–21). For certain subgroups of liver cancer patients, a combination of targeted therapy and immunotherapy targeting infiltrating immune cells (except for T lymphocytes), may prove more effective than immune checkpoint inhibitors such as atezolizumab plus bevacizumab. By modulating the functional characteristics of neutrophils, it may be possible to enhance the sensitivity of HCC patients to systemic therapy by altering the immune microenvironment (4).
In addition to producing oxidants, proteins, and granular enzymes, neutrophils also have the ability to generate NETs (9, 22, 23). NETs consist of aggregated DNA that serves as a backbone that interacts with various molecules either positively or negatively (24). By forming NETs, activated neutrophils can ensnare a wide range of microorganisms, including bacteria (24, 25), fungi (26, 27), and viruses (28–31), enabling an effective response and subsequent clearance through the action of effector molecules (23). Numerous studies have provided evidence for the involvement of NETs in tumor initiation, progression, and angiogenesis (32–34). Interestingly, NETs also play a similar role in HCC (35, 36).
In this article, we review evidence for the presence of NETs in HCC which may support the hypothesis that NETs play a pivotal role in the pathogenesis of liver cancer. We discuss the proposed mechanisms by which NETs may drive tumor progression. Furthermore, considering that research on NETs in HCC is still at an early stage, we examine studies conducted on NETs in other types of cancers and discuss emerging areas of interest.
2 NETs discovery, origin and diversity
2.1 The historical and current existence of NET
NET formation, the process responsible for the formation of NETs to eliminate invaders through the release of granular proteins and chromatin, can be classified into two subtypes: lytic and vital (24, 37). Lytic NET formation, also known as suicidal NET formation, involves a slow, active cell death that occurs over several hours, distinguishing it from other forms of cell death such as necrosis or apoptosis (37). In addition to suicidal NET formation, another rapid process known as “vital NET formation”, which rapidly expels DNA from the nucleus or mitochondria of living cells within a few minutes while maintaining cell functionality and viability.
Phorbol 12-myristate 13-acetate (PMA), a classic nonphysiological stimulus, induces a distinct form of neutrophil death that is fundamentally different from apoptosis and necrosis (38). PMA promotes cell death by increasing chromatin loosening and incorporating the nuclear envelope into organelles, a process that occurs within a few hours (39, 40). This process involves four key steps: (1) enhanced plasma membrane permeability, (2) accelerated disintegration of the nuclear envelope and cytoskeleton, (3) assembly of antimicrobial proteins onto chromatin scaffolds, and (4) decondensation of chromatin (41). In vitro experiments using isolated neutrophils treated with PMA have provided valuable mechanistic insights into lytic NET formation (Figure 1), demonstrating that neutrophil activation is accompanied by the assembly and activation of a multicomponent nicotinamide adenine dinucleotide phosphate (NADPH) oxidase and ROS (51–53). ROS can be generated by both NADPH oxidase and mitochondrial metabolism (53). In addition, G protein-coupled receptors (GPCRs) (54), CXC chemokine receptors (CXCRs) (55), toll-like receptors (TLRs) (56, 57), and cytokine receptors contribute to this process. Hydrogen peroxide (H2O2) is a robust oxidant generated by NADPH oxidase during the reduction of molecular oxygen through electron transfer, and it plays a crucial role in both NET and ROS generation (41). The correlation between neutrophil metabolism and ROS generation has been highlighted in mechanistic studies (58, 59). In NADPH oxidase-deficient neutrophils, PMA compensates for the lack of NADPH oxidase and, in combination with protein kinase C (PKC), triggers calcium release and subsequent activation of the Raf-MEK-ERK pathway (60)and ROS-dependent p38MAPK (61). However, in mice, NET formation may not be associated with ROS generated by NADPH oxidase (62). In addition to the classical pathway requiring ROS, two recent papers reported a novel pathway of NETosis mediated by a pore-forming protein, gasdermin D (GSDMD), that is independent of ROS (63, 64). Actin cytoskeletal dynamics plays a critical role in neutrophil activation, and during the first half hour after stimulation, limiting actin polymerization reduces NET formation (65).
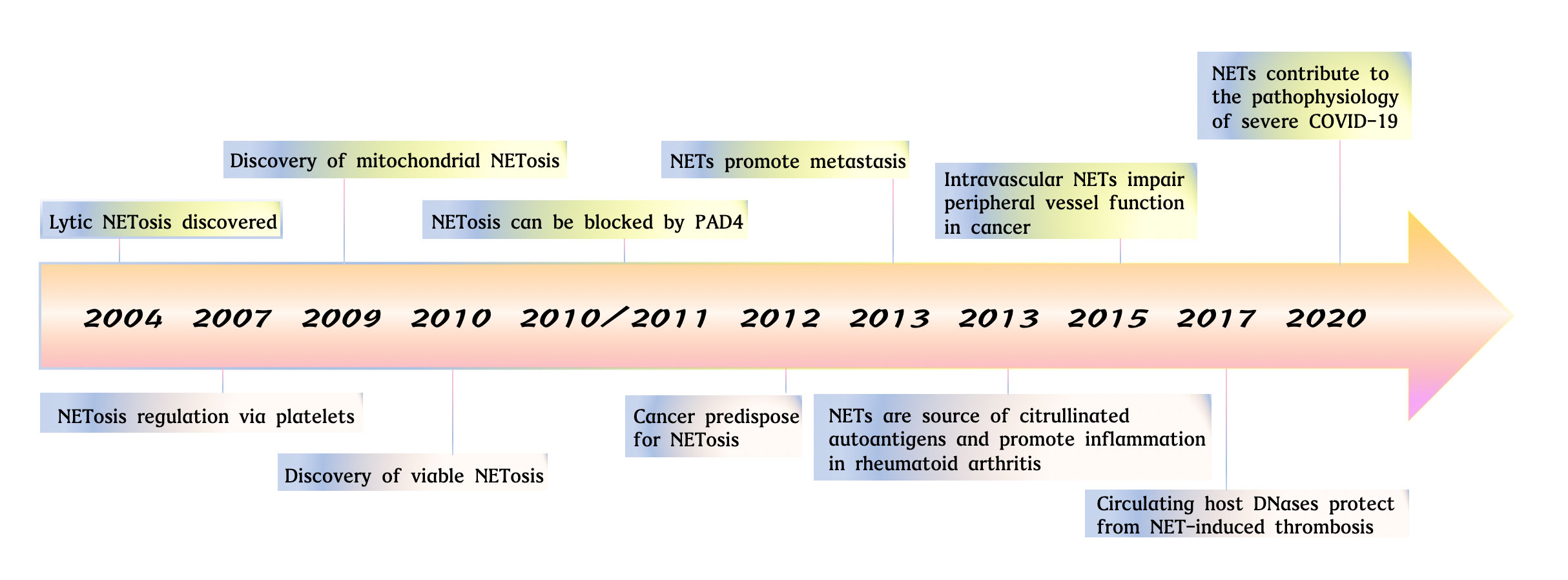
Figure 1 The studies of neutrophil extracellular traps from 2004 to 2021. Key observations in studies on NET formation are highlighted. Lytic NETosis discovered (24). NETosis regulation via platelets (42). Discovery of mitochondrial NETosis (23). Discovery pf viable NETosis (43). NETosis can be blocked by PAD4 (44, 45). Cancer predispose for NETosis (46). NETs promote metastasis (47). NETs are source of citrullinated autoantigens and promote inflammation in rheumatoid arthritis (48). Intravascular NETs impair peripheral vessel function in cancer (49). Circulating host DNases protect from NET-induced thrombosis (50). NETs contribute to the pathophysiology of severe COVID-19 (31).
Peptidyl-arginine deiminase 4 (PAD4), an intracellular calcium-dependent enzyme, activated by intracellular calcium levels. It converts arginine to citrulline. Upon translocation to the nucleus, PAD4 deaminates histones H2A, H3, and H4, resulting in decreased chromatin compactness (66, 67). Notably, mouse model studies indicate that NET formation explicitly relies on PAD4 and neutrophil elastase (NE) (62). However, recent research suggests that PAD4 is mainly required for NET formation in response to ionomycin and immune complexes, whereas it is dispensable for NET formation induced by cholesterol crystals, fungi, or PMA in human neutrophils (44, 68, 69). Inhibition of PAD2, another member of the PAD family, can reduce NET formation and inflammatory cytokine production (45). NE, which is released from intracellular granules into the cytoplasm during NET formation, may then degrade linker histones to promote chromatin decondensation. Interestingly, NE and myeloperoxidase (MPO), released from azurophilic granules and translocated to the nucleus, cooperate in facilitating chromatin decondensation independently of their enzymatic activities (70, 71).
In 2009, pivotal research identified a distinct form of NET formation known as vital NET formation, which is distinct from lytic NET formation. It is induced by granulocyte-macrophage colony-stimulating factor (GM-CSF), followed by stimulation with lipopolysaccharide (LPS) or C5a, and can occur either a ROS-dependent or independent manner (23, 72, 73). The entire process of vital NET formation proceeds by a unique and remarkably rapid mechanism, that takes only 5-60 minutes and being independent of oxidants (72). Notably, the existence of lytic NET formation upon LPS treatment suggests that different modes of NET formation under different circumstances synergistically contribute to immune defense (74). Importantly, there is heterogeneity in the propensity of neutrophils to undergo NET formation, with studies reporting a range of 10% to 60% of cells capable of NET formation at a given moment (37, 70, 75, 76).
At particular times in their lifespan, a subset of neutrophils expresses Olfactomedin 4 (OLFM4) and CD177, two molecular markers associated with NET biology, regardless of their maturation or activation stage (77, 78). Approximately 25% of circulating neutrophils in healthy individuals express OLFM4 [73]. This expression of OLFM4 in a subset of neutrophils is conserved in mice, and it is secreted and colocalized with NETs (79, 80). Clinical studies suggest that high numbers of OLFM4+ neutrophils in patients with septic shock are associated with risk of organ failure and death (81). This is consistent with the findings that OLFM4- neutrophils, in contrast to their OLFM4+ counterparts, provide protection against sepsis-induced mortality in OLFM4-/- mice following LPS stimulation (79). These studies suggest that OLFM4 may reflect NET-induced toxicity rather than serve as a marker of NET formation. Another neutrophil protein associated with NET formation is CD177, which is expressed by approximately half of the neutrophils in peripheral blood. Research has shown that CD177- neutrophils do not respond to LPS stimulation via NET formation (82, 83). Therefore, the regulation of OLFM4 and CD177 in NET formation may be a promising avenue for therapeutic intervention in NET-associated diseases, whether inflammatory or non-inflammatory.
Recently, a significant discovery had been found in the process of NETosis (84). They found that NETosis is triggered by mitogens and results in the upregulation of cell cycle markers, such as the phosphorylation of retinoblastoma proteins and laminin, disruption of the nuclear membrane, and replication of centrosomes. Further investigations revealed that CDK6, and possibly CDK4, are necessary for the signaling of NETosis. Interestingly, inhibition of CDK4/6 did not affect ROS production, leading to the hypothesis that CDK might function downstream of ROS activation. On the other hand, the translocation of NE to the nucleus relies on the activity of CDK4/6. However, the connection between CDK activity and ROS activation remains unclear, particularly since CDK6 also translocates to the nucleus during NETosis (84). CDK activation during NETosis occurs downstream of MAPK, although further experiments are required to confirm this. Future studies should focus on identifying the substrates of CDK4/6 involved in the NETosis pathway, as well as the mechanisms enabling neutrophils to respond to CDK4/6 activation, ultimately resulting in cell death instead of cell proliferation. Notably, investigating the role of CDKs in neutrophils using transfection or gene editing is challenging due to the short lifespan of neutrophils in culture (1-2 days). However, Amulic et al. ingeniously synthesized a peptide that mimics the inhibitory structural domain of p21 associated with CDKs (85). This peptide was coupled with a cell-penetrating sequence, providing an innovative approach for researchers facing difficulties in studying neutrophils due to their short half-life.
2.2 NETs content
The structure of NETs consists primarily of loosely packed chromatin composed of DNA and RNA, together with proteins composed predominantly of positively charged histones (86). The positive charge of these proteins facilitates the adsorption of small negatively charged foreign particles, while histones and nucleic acids themselves have bactericidal effects (87). In addition to histones, NET-associated proteins include cytoplasmic, granule, and cytosolic proteins, as well as neutrophil-derived metabolic enzymes (70, 88). Previous studies have shown that NETs not only contain their own effector molecules but also trap and release cytokines from other cells (89). Due to the diverse environmental conditions, both the structure and proteome of NETs exhibit considerable variation (52, 86). However, the core NET proteome, primarily derived from neutrophils, consists of histones, NE, proteinase 3, MPO, α-defensins, and cathepsin G (90, 91). Therefore, it is essential to identify disease-specific NETs (Figure 2).
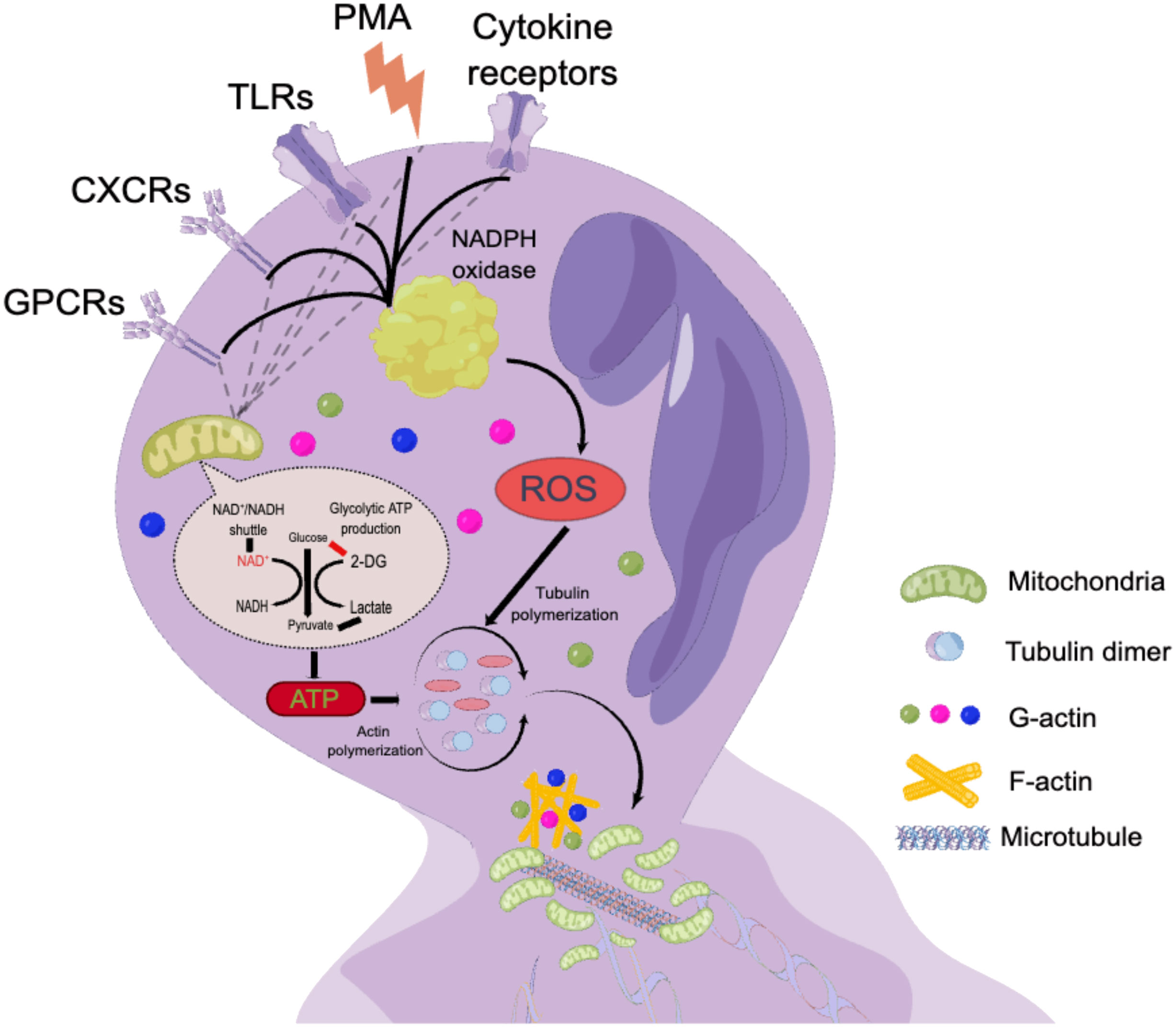
Figure 2 The mechanism of lytic NET formation. By processing isolated neutrophils with PMA in neutrophil, which in turn lead to the production of multicomponent NADPH oxidase and ROS (52–54). ROS can be produced by either NADPH oxidase or mitochondrial metabolism (54). GPCRs (55)、CXCRs (56)、TLRs (57) and cytokine receptors were also involved in this process.
2.3 Neutrophil and NETs
A growing body of research suggests that not all neutrophils possess the ability to secrete NETs, which have been observed in diverse tissues, organs, and species. It is becoming increasingly clear that the molecular properties of NETs secreted by neutrophils varies across different stages of neutrophil maturation and among different neutrophil subtypes. Traditionally, neutrophils have been viewed as a homogeneous population of cells, but recent studies have revealed their plasticity, allowing them to adapt their phenotype to the environment and fulfill different functional requirements (11, 13). This adaptability is exemplified by their differential capacity to release NETs. The significant involvement of NETs in disease pathogenesis is underscored by a considerable increase in research efforts over the past decade (Figure 3).
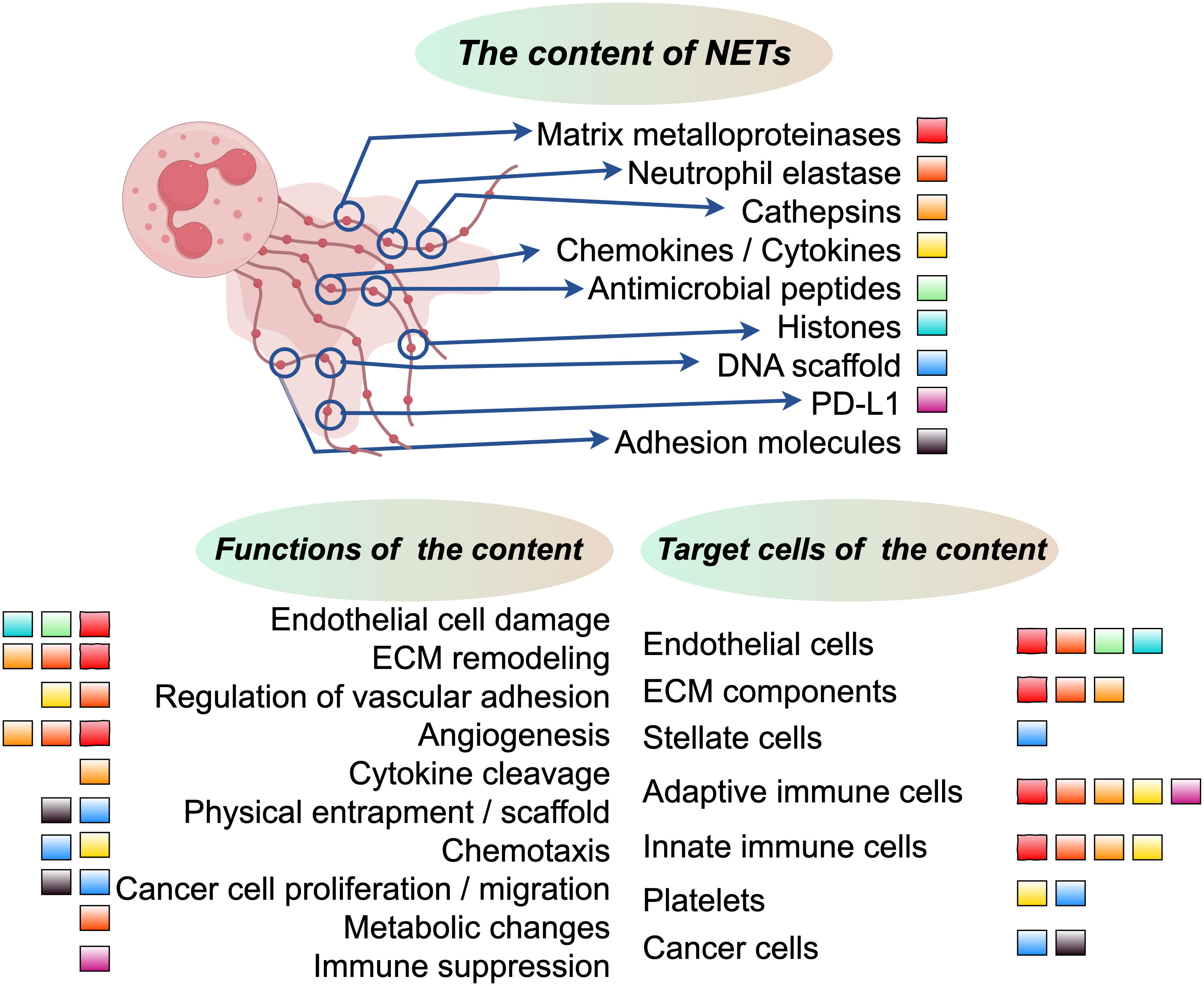
Figure 3 The upper section in the middle illustrates the core constituents of NETs, with each component represented by colored squares. The lower left section delineates the functional allocation of these core NET components, while the lower right section elucidates the distribution of cells or components that are targeted by these pivotal NET components.
Recent studies have shown that Only about 30% of mice neutrophils produce NETs whereas up to 60% of human neutrophils can produce NETs (70, 75). These observations suggest that not all types of neutrophils exhibit the same ability to release NETs under identical stimulation, though the underlying reasons for these differences remain unclear. Previous research has indicated that the increased capacity for NET formation corresponds to the transition from the immature stage to the mature stage in bone marrow-derived neutrophils of mice (92). However, circulating neutrophils, while still capable of releasing NETs, exhibit a reduced capacity for NET formation during their immature stage (93). The variation in the ability of neutrophils to generate NETs at different stages of disease in different tissue environments remains unclear and requires further investigation. It remains to be determined whether there are changes in the components of NETs under these circumstances. Investigation of such changes may provide insight into the initiation of NETs in different diseases and the subsequent progression of these diseases.
NET formation is subject to circadian regulation that is influenced by the contents of neutrophil, including proteolytic enzymes, antimicrobial peptides, and adhesion molecules (94). Intriguingly, degranulation has been observed under both acute activation and steady-state conditions. In support of this notion, CXCR2-mediated autocrine signals can induce degranulation, with mouse neutrophils exhibiting reduced content of primary granules during daylight hours after being mobilized into the circulation at night (55). Temporal degranulation which is tightly regulated by the circadian machinery, suggests a significant temporal dependence of NET formation, specifically within the 24-hour cycle. This finding is particularly relevant as recent studies have demonstrated remarkable discrepancies in NET formation in vivo for neutrophils isolated at different times, observed in both mice and humans, and replicated in cases of acute lung injury or liver ischemia-reperfusion injury (53). Disturbed circadian patterns have been linked to several diseases, including tumors (95–97). However, the mechanisms underlying the association between NETs and circadian pattern-related diseases are not yet fully understood, and further research is required in this area. It is also unclear whether NETs can serve as a clinically reliable marker to predict the impact of circadian pattern disruption on the development of specific diseases.
Recent studies have highlighted the critical role of the microbiome in regulating neutrophil aging (98). In inflammatory conditions, an overactive subset of aged neutrophils significantly enhances NET formation (98). Aging neutrophils, despite limited evidence for circadian regulation, exhibit a bias toward activation and NET formation in the context of stress-induced inflammation and vascular occlusion in mouse models of sickle cell disease (98–100). The quantity of NETs associated with Haemophilus sp. is elevated in the sputum of patients with chronic obstructive pulmonary disease (101). Accordingly, elimination of gastrointestinal bacteria in mice reduces NET formation; nevertheless, this strategy may have side effects (98). It is important to note that the absence of gut microbiota is correlated with increased NET formation in mouse models of acute mesenteric ischemia-reperfusion injury (102). Based on these observations, it can be concluded that the composition of the microbiome may have different effects on NET formation. Segmented filamentous bacteria promote NET formation in neutrophils, whereas in human neutrophils, Lactobacillus rhamnosus strain GG, a member of the probiotic system, inhibits NET formation by attenuating ROS production in vitro (98, 103). Interestingly, researchers have discovered that microorganism-derived metabolites can influence NET production in multiple and potentially deleterious ways. The regulation of NET production may depend on diurnal variations in the location, function of the microbiome, and the control of intestinal permeability (104).
The regulation of NET formation at different age and maturation stages is an important aspect to consider. A recent study has demonstrated that neutrophils egress from the bone marrow follows a circadian rhythm, with peak levels occurring in the morning (100). This observation implies that particular subsets of neutrophils, rather than only neutrophils of a certain age, are able to produce NETs. In addition, circadian fluctuations drive increased neutrophil output from the peripheral blood into tissues at night through the regulation of chemokine CXCL2 expression by the circadian clock gene Bmal1 and subsequent CXCR2-dependent signaling (100). Interestingly, NET formation in neutrophils also follows circadian rhythms, with recently released neutrophils being more susceptible to these rhythms. The Bmal1-CXCL2-CXCR2 axis plays a crucial role in NET formation, and any disruption to its components will impede this process (55). Another study found age-related variability in the ability of neutrophils to generate NETs, which may explain the increased susceptibility of the elderly to infection and age-related inflammation (105). This diminished capacity for NET formation was observed not only in mice (average age of 18 months) but also in humans (average age of ~70 years) (106, 107).
These factors may be attributed, among others, to the age-related increase in neutrophils within the bone marrow and the degranulation of circulating neutrophils. These processes have been linked to a decline in the capacity for NET formation (55). Aside from age, the distribution of neutrophils can also influence NET formation and exhibit significant associations with various diseases. In conditions such as sterile neutrophilia and septicemia, intravascular NETs can form clots in the circulation, obstructing blood vessels and causing organ damage in the absence of host DNases (50, 108). Similarly, the significant susceptibility to thrombosis and inflammatory injury in the liver and lungs may be attributed to the intensive ability of relatively immature neutrophils, which have not been completely cleared from the circulation, to produce NETs (49, 55, 109). In the context of persistent inflammation, neutrophil stimulation at various locations may lead to excessive NET formation, as observed in the lungs of SARS-Cov-2 patients (43), autoimmune diseases (52, 110), and mouse models of atherosclerosis (111, 112).
3 NETs in hepatocellular carcinoma
HCC typically begins insidiously and progresses rapidly. Unfortunately, by the time it is diagnosed, the majority of cases have already progressed to the intermediate and late stages, which are often associated with a grim prognosis. It has been observed that an elevated neutrophil-lymphocyte ratio (NLR) and a high infiltration of tumor-associated neutrophils are associated with worse outcomes in HCC (5, 7, 113). Nevertheless, the precise mechanisms through which neutrophils, particularly NETs, contribute significantly to the progression of HCC have yet to be thoroughly investigated. Apart from their well-known functions in innate immunity, NETs also play a crucial role in various stages of tumor initiation and progression. They promote tumor angiogenesis and growth, facilitate tumor expansion, and provide a protective shield for tumor cells against antitumor immunity (76, 114, 115). Notably, high expression of NETs has been observed in both the blood serum and tissue specimens of HCC patients, as well as in individuals with liver diseases such as nonalcoholic steatohepatitis and liver cirrhosis. This suggests a potential involvement of NETs in the development and progression of HCC (35, 46). Nevertheless, the specific molecular mechanism of NETs in HCC have yet to be fully elucidated. This review aims to summarize and provide further insights into these functions (Figure 4).
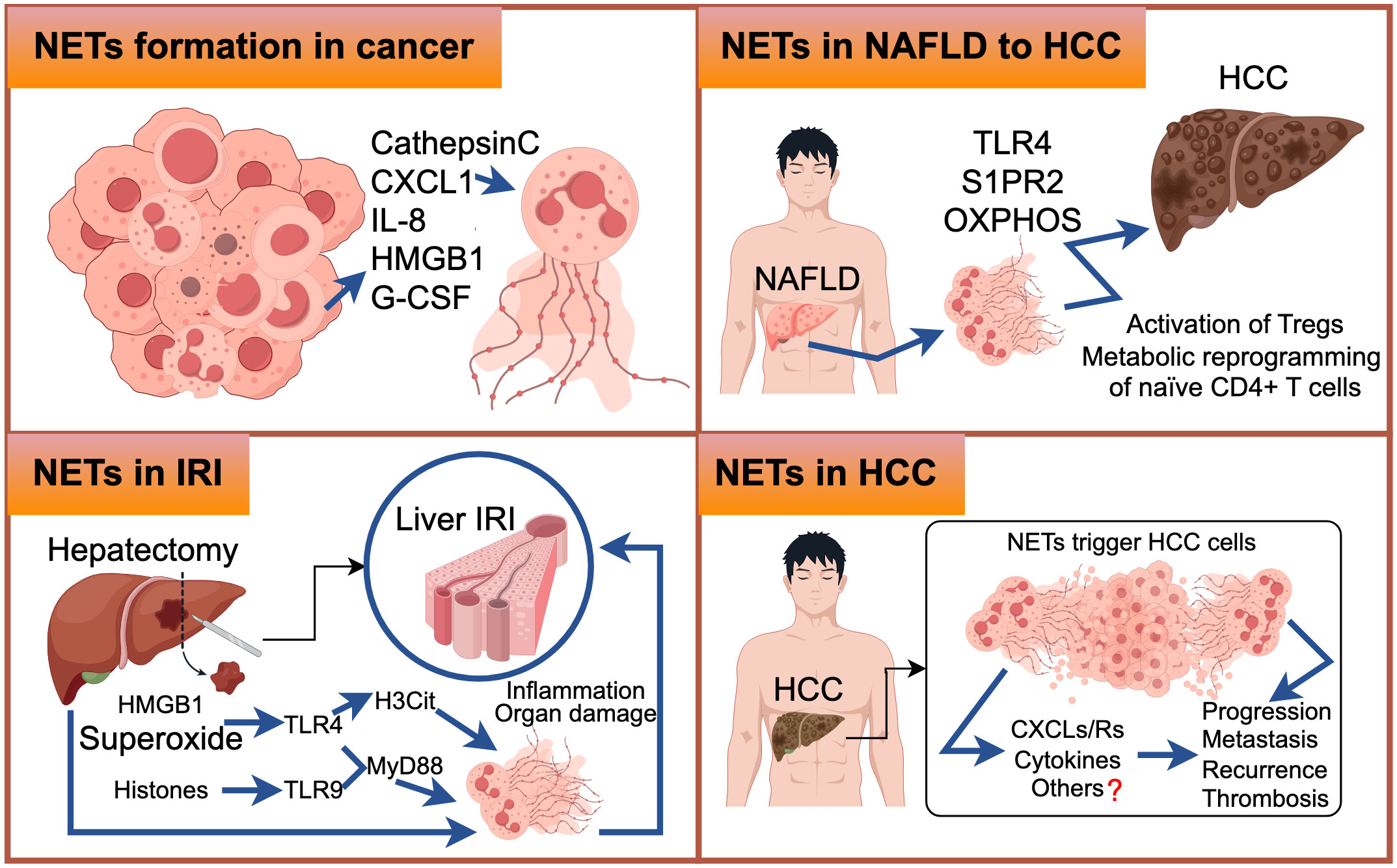
Figure 4 The upper-left figure illustrates selected molecular mechanisms underlying the formation of Neutrophil Extracellular Traps (NETs) in cancer. Similarly, the upper-right figure elucidates the molecular pathways through which NETs are generated during the transition from Non-Alcoholic Fatty Liver Disease (NAFLD) to Hepatocellular Carcinoma (HCC). In the lower-left figure, we delineate the molecular events that lead to NET formation in the context of Ischemia-Reperfusion Injury (IRI). The lower-right figure comprehensively outlines the intricate molecular processes associated with NET formation in HCC, with question marks indicating areas necessitating further exploration.
3.1 NETs in HCC initiation and growth
Non-alcoholic fatty liver disease (NAFLD) encompasses a range of chronic hepatic disorders characterized by the accumulation of fat in hepatocytes, known as steatosis. It is commonly associated with obesity, hyperlipidemia, and insulin resistance. Notably, more than 10% of NAFLD patients progress to nonalcoholic steatohepatitis (NASH) (116), which represents the most significant risk factor for HCC development (117). In the livers of STAM mice (induced by neonatal streptozotocin and a high-fat diet), early infiltration of neutrophils and the formation of NETs were observed, followed by the production of inflammatory cytokines, the influx of monocyte-derived macrophages, and the progression of HCC (36). NETs formation, triggered by S1P receptor 2 signaling, accelerates inflammation and maintains disease progression in the early stages of NASH (118), as well as playing a crucial role in the transition from NASH to HCC and HCC metastases in later stages (36). It is worth noting that while the accumulation of fat in liver cells is independent of NET formation, NETs have an initiating role in the pathogenesis of fully developed NASH by modulating the inflammatory environment and promoting the activation of novel monocyte-derived macrophages (36). Treatment with DNase therapy or the use of PAD4-/- mice did not affect the progression of hepatic steatosis but altered the subsequent hepatic inflammation pattern, ultimately resulting in reduced tumor growth (36). Inhibiting NETs in vivo, either through PAD4-deficient mice or treatment with DNase I, leads to reduced activity of regulatory T cells (Tregs) in NASH, and depletion of Tregs significantly inhibits the initiation and progression of HCC (119). RNA sequencing data revealed that NETs have an impact on gene expression profiles of naïve CD4+ T lymphocytes, particularly genes involved in oxidative phosphorylation in mitochondria (119). Enhancing mitochondrial respiration can promote the differentiation of regulatory T cells through the facilitation of NET formation (119) (Figure 5A). Future research should focus on investigating the specific components of NETs’ structure that interact with TLR4 on CD4+ T cells, as well as elucidating the properties of downstream signaling pathways. Moreover, further in vivo studies are necessary to understand the effect of NETs on Treg suppressive function. Targeting the interaction between neutrophils and Tregs or inhibiting Treg activity may enhance cancer immunosurveillance and prevent HCC formation. In HBV-infected HCC patients, NETs have been found to promote HCC proliferation both in vitro and in vivo, primarily through NETs-mediated cell entrapment, EMT-associated cellular migration, and MMPs-induced degradation of the extracellular matrix (ECM) (46).
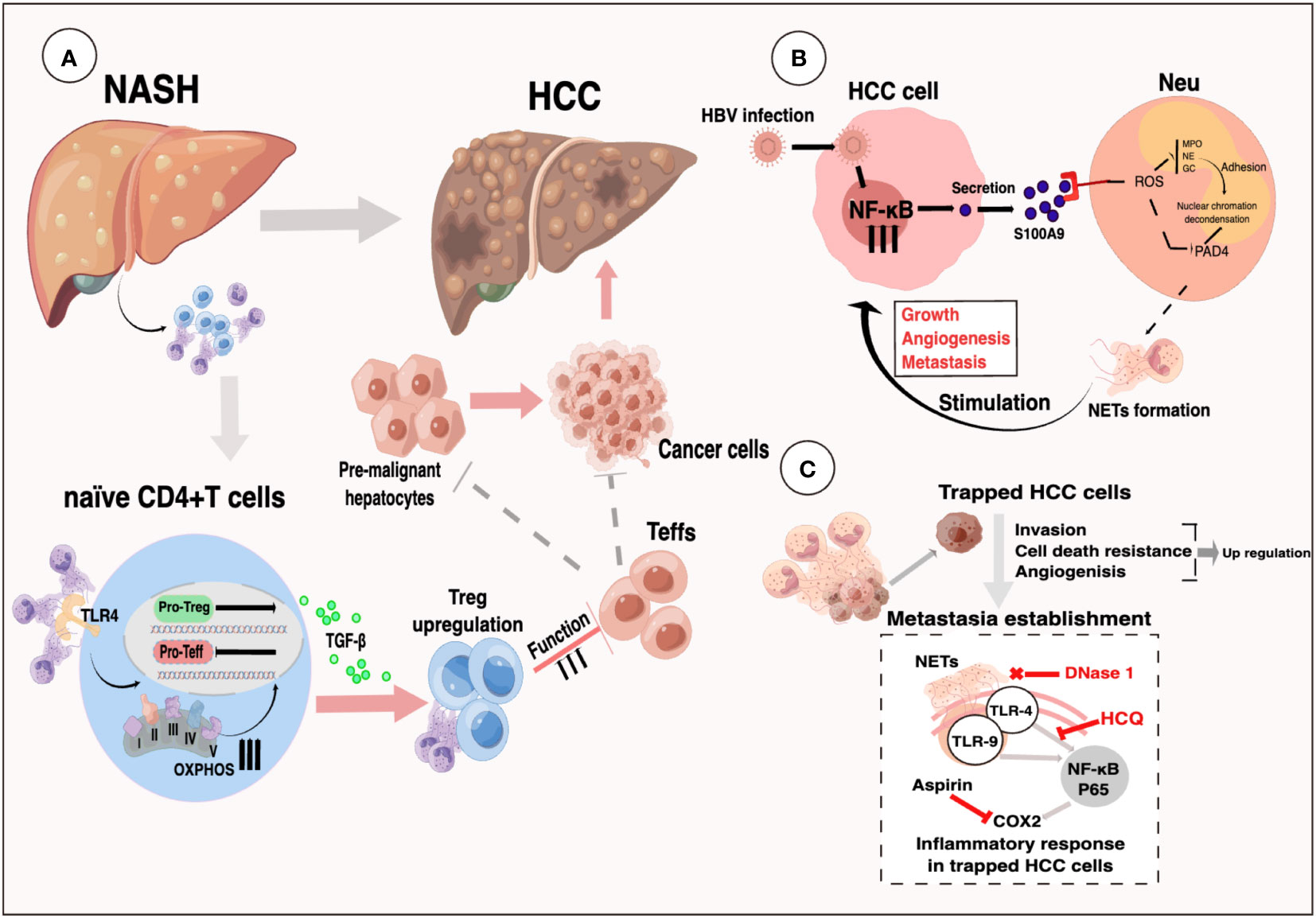
Figure 5 (A) Selectively increased intrahepatic Tregs can promote an immunosuppressive environment in NASH livers. NETs link innate and adaptive immunity by promoting Treg differentiation via metabolic reprogramming of naïve CD4+ T-cells (119). (B) Activation of RAGE/TLR4‐ROS signaling by HBV‐induced S100A9 resulted in abundant NETs formation, which subsequently facilitated the growth and metastasis of HCC cells (116). (C) System illustration of NETs to trap and fuel HCC metastasis potential through triggering an inflammatory response through TLR4/9-COX2 signaling (35).
The presence of NETs in the inflammatory microenvironment associated with liver cancer promotes tumor growth (120). In vitro experiments neutrophils from HCC patients showed a higher tendency to release NETs (35). A study revealed that plasma NET formation levels are increased in patients with liver cirrhosis and/or HCC, and these levels correlate with the severity of hepatic dysfunction (121). Elevated levels of MPO-DNA (NET serum markers) were associated with increased mortality after hepatectomy in HCC patients (122). There is growing evidence, albeit preliminary, suggesting that NET formation plays a crucial role in HCC development and proliferation. However, further research is needed to investigate the specific mechanisms of NET formation in HCC recurrence after surgical intervention, portal vein tumor thrombus, and systemic therapeutic resistance.
Liver transplantation offers benefits to patients with partial liver cancer. However, the occurrence of liver ischemia-reperfusion injury (IRI) is strongly associated with procedural failure in liver transplantation (123). Inflammation plays a significant role in the pathogenesis of liver IRI, with neutrophils being a key component of hepatic injury after reperfusion. Excessive activation and recruitment of neutrophils in the reperfusion tissue contribute to the development of ischemia-reperfusion injury. Neutrophils perform various functions throughout this intricate process, including activation, transport through the vasculature, and migration (124–126). A growing body of research has indicated that NETs could contribute to liver IRI. Released from damaged liver cells, HMGB1 proteins are associated with tissue damage and can trigger NET formation through the activation of TLR4 and TLR9 (127). In a study, extracellular superoxide treatment induced the release of NET DNA, citrullination of histone H3 (H3Cit) (128), and elevated levels of MPO-DNA complexes in neutrophils in a TLR-4 dependent manner. Pretreatment with allopurinol and N-acetylcysteine attenuated the formation of NETs and liver injury following ischemic injury in mice (56). Both neutrophils and NETs negatively correlate with the expression of histidine-rich glycoprotein (HRG) in mouse models of liver IRI. Overexpression of HRG in mice suppressed neutrophil infiltration and NET formation in the liver, thus reversing hepatic IRI (129). Another study demonstrated that hydroxychloroquine inhibits NET formation and alleviates liver IRI in mouse models of liver ischemia-reperfusion (48). Acrolein triggers neutrophil chemotaxis and exacerbates NET release both in vitro and in vivo. Inhibition of acrolein-induced NET release was observed in vitro, while suppression of inflammatory cytokine expression, P38MAPK-ERK activation, and apoptotic signals in the liver of mice subjected to IR resulted in a slower recovery rate of the liver after surgery (130). TMP, a compound derived from the plant Ligusticum wallichii Franchat, inhibited NET formation in rats after hepatic transplantation, improved hepatic function, and alleviated liver IRI (131). These studies have shown that antioxidant treatment can reduce NET formation and protect against hepatic IRI. However, when considering the therapeutic efficacy of inhibiting NETs, it is important to take into account the underlying preoperative disease and potential complications in immunocompromised individuals following transplantation.
3.2 NETs in hepatocellular carcinoma on the metastatic cascade
The previous study discovered that NETs sequester tumor cells in circulation and promote metastasis (132). Recently, researchers revealed that patients with liver cancer, particularly those with metastatic hepatocellular carcinoma, exhibit enhanced NET formation in neutrophils (133). NETs ensnare HCC cells, inducing resistance to cell death and enhancing invasiveness, thereby triggering metastatic potential. This process is mediated by the internalization of NETs into trapped HCC cells and activation of the TLR4/9-COX2 signaling pathway. In mouse models, a combination therapy involving DNase 1-mediated degradation of NETs and anti-inflammatory drugs such as aspirin/hydroxychloroquine effectively mitigates liver cancer metastasis (35). In HBV-infected HCC patients, the promotion of HCC metastasis by NETs was demonstrated both in vitro and in vivo, primarily through NETs-mediated cell entrapment, cell migration associated with epithelial-mesenchymal transition (EMT), and the degradation of ECM induced by MMP (46). Moreover, the induction of S100A9 by HBV accelerates the generation of NETs through the activation of TLR4 and the receptor for advanced glycation end products (RAGE), along with the signaling cascade involving ROS. Furthermore, the formation of NETs in the circulation was significantly associated with TNM stage and metastasis in HBV-induced liver cancer, and the presence of NETs was shown to have predictive value for extrahepatic metastasis (46) (Figure 5B). Deficient DNASE1L3 (an extracellular DNase) facilitates HCC invasion through NET formation by activating GMP-AMP synthase in circulation and the non-canonical pathway of NF-kappa B in diabetic HCC (47). Elevated levels of NET release have been observed in patients diagnosed with HCC, particularly those with portal vein tumor thrombosis (PVTT) (134). IL-8 produced by liver cancer cells triggers the formation of NETs via an NADPH oxidase-dependent pathway, and NET-associated cathepsin G (cG) facilitates hepatocellular carcinoma metastasis both in vitro and in vivo (134). The metabolic shift of tumor-associated neutrophils towards glycolysis and the pentose phosphate pathway, induced by tumors, promotes the formation of NETs in a ROS-dependent manner. Subsequently, NETs trigger tumor cell migration and suppress tight junction molecules on adjacent endothelial cells, thereby promoting tumor intravasation and metastasis (133). Activated neutrophils play a crucial role in the generation of ROS through their oxidative bursts (135). CXCL1-triggered hepatic impairment was found to be dependent on p47 phox, which mediates oxidative burst and is a constituent of the NADPH oxidase 2 complex (136, 137). In mice fed a high-fat diet, the absence of PAD4 or NE genes failed to abolish the capacity of elevated CXCL1 levels to induce NASH. This suggests that NETs or NE are not indispensable for the onset of CXCL1-induced NASH (138).Neutrophils in patients with liver cancer exhibit elevated levels of mitochondrial ROS (mitoROS) and generate NETs that are enriched with oxidation products of mitochondrial DNA (mtDNA) in a manner dependent on mitoROS (139). NET formation and oxidized mtDNA have clinical relevance. When bound to NET-associated proteins, oxidized mtDNA exhibits a heightened ability to elicit inflammatory mediators that promote metastasis in HepG2 cells (139). Targeting oxidized mtDNA with metformin can reduce the inflammatory state that facilitates metastasis, thereby undermining the metastatic capacity of hepatocellular carcinoma (139). Furthermore, high levels of NET infiltration have also been observed in patients with colon and breast cancers who have developed hepatic metastasis. Additionally, the level of NETs in serum can serve as a predictive marker for the incidence of liver metastases in early-stage breast cancer patients (17).
Previous research has revealed that soluble factors derived from tumors, such as hyaluronan fragments, metabolites, and cytokines, can have significant effects on the regulation of phenotypes and functions in tumor-infiltrating neutrophils. However, it is still uncertain which of these components, or if any other factors in the model, could be related to triggering the metastatic switch and leading to the release of NETs. In addition, DNase I has commonly been employed to eliminate NETs. However, a lingering question remains regarding whether DNase I eliminates the entire molecular structure of NETs or selectively targets specific components within the molecular structure. This uncertainty raises concerns about its potential impact on the specific mechanisms underlying NETs in HCC. Therefore, further investigations are warranted to address these inquiries thoroughly.
3.3 The role of NETS on hepatocellular cancer recurrence
Metastasis is the primary cause of carcinoma mortality, and neutrophils play a critical role in this process (140). Several studies have shown that NETs capture circulating tumor cells and release proteases, leading to tumor proliferation and metastasis (62, 141, 142). The capture of NETs has been associated with an increased incidence of microscopic liver metastases within 48 hours and a higher burden of gross metastatic disease at 2 weeks after tumor cell inoculation (132). One study suggests that elevated NET formation is linked to shorter recurrence-free survival and overall survival, making it a potential pre-surgery biomarker in serum for identifying patients at higher risk of recurrence (143). Another study demonstrated that HCC harbors NETs characterized by the enrichment of oxidized mitochondrial DNA, which promotes inflammation and metastasis (139). Enhanced circulating NET markers are also associated with an increased risk of recurrence in patients with advanced colorectal cancer and liver metastasis who have undergone major hepatic resection (34). Further study has found that NET formation may play a pivotal role in impeding emboli during metastasis, protecting them from NK cell attacks while traversing the bloodstream and infiltrating affected organs (144). Subsequently, another study combined this with engineering techniques: the application of an injectable hemostatic gel adhesive containing a neutralizer and NETs lyase has been shown to enhance adoptive natural killer cell immunotherapy and prevent liver cancer recurrence after resection (145). Additionally, NETs may act as a protective barrier, shielding cancer cells from the cytotoxicity of CD8+ T cells and NK cells. However, NETs are not the sole factor contributing to the impediment of antitumor immunity mediated by T cells and NK cells (144). The aforementioned studies indicate a positive correlation between elevated levels of NETs and an increased likelihood of HCC recurrence. However, the precise role of NETs in the specific mechanisms of HCC recurrence remains unclear. Additionally, it is yet to be determined whether the influence of NETs on HCC recurrence is influenced by circadian regulation, patient age, preoperative neoadjuvant therapy, postoperative chemotherapy, or other interactions that may impact HCC recurrence differently. Therefore, further investigations are needed to explore in-depth the specific mechanisms underlying the involvement of NETs in HCC recurrence.
3.4 The role of NETs in hepatocellular carcinoma on thrombosis
NETs are widely recognized for their crucial role in the pathogenesis of thrombosis and coagulation disorders, particularly in cancer (115, 146). Heparins, anticoagulant polyanions released by mast cells at pathogen entry sites, modify fibrin structure and stabilize fibrin clots through size-dependent modulation and kringle-dependent inhibition of plasmin-mediated fibrinolysis. All polyanions mechanically fortify the clots; however, smaller P45, P100, and low molecular weight heparin (LMWH) decrease the pliability of fibrin, while larger UFH and P700 increase the maximum tolerable deformation of blood clots (147). Administration of LMWHs inhibits the activation-induced autophagy of neutrophils and NET formation. Research has shown that LMWHs profoundly alter the capacity of neutrophils to produce NETs and mobilize primary granule contents in response to non-related inflammatory stimuli, such as HMGB1, PMA, and IL-8 in humans (148). Additionally, research has found that heparin and polyphosphate variants, procoagulant polyanions released by platelets and microorganisms, modulate the susceptibility of fibrin-histone clots to lysis and their mechanical stability. The size and charge of these variants play a crucial role in this process (42). Lu et al. developed a micellar nanoparticle loaded with doxorubicin, LMWH, and astaxanthin (LMWH-AST/DOX, LA/DOX NP) to prevent the occurrence of these loops and inhibit hepatic metastasis by inhibiting NET formation. These nanoparticles exhibited dual effects by not only inhibiting metastasis to the lungs but also mitigating the inflamed and immunosuppressed microenvironment within tumors (149).
Research has demonstrated that the interplay between neutrophils and platelets is especially significant in driving the formation of NETs and maintaining the process of diffuse coagulation (150–152). While this concept is substantiated in the context of sepsis, it necessitates further exploration in the context of HCC. This is especially pertinent for patients with HCC combined with portal vein thrombosis, as well as for those experiencing postoperative complications related to disseminated intravascular coagulation (DIC) in the context of HCC. Moreover, neutrophils primarily induce the formation of NETs through serine proteases, thereby activating both extrinsic and intrinsic coagulation pathways (109). A study investigating hepatic surgery observed that NETs and platelet-rich microthrombi contribute to microvascular alterations in injured organs. However, inhibiting NETs formation using DNase reduced immunothrombosis and organ damage (153). Another study demonstrated that the combined action of tissue plasminogen activator (tPA) and DNase I effectively triggers thrombolysis (154). Administering DNase I to rats with thrombosis proved successful in preventing myocardial infarction, recurrent cerebrovascular accidents, and deep vein thrombosis (155). Nevertheless, further research is needed to determine whether the degradation of NETs by DNase I could increase the risk of thrombosis and inflammation.
3.5 Approaches to targeting NETs in hepatocellular carcinoma
NETs have emerged as key contributors to the pathogenesis of various diseases, prompting extensive research into potential therapeutic targets. The presence of NETs has been linked to cancer patient survival (156, 157). However, it is crucial to carefully assess the risks associated with targeting NET formation. While NETs offer protection against severe infectious diseases, inhibiting NETs could potentially increase susceptibility to infections (44, 158). For instance, mice lacking the PAD4 gene (-/-) exhibited heightened vulnerability to bacterial diseases due to impaired NET formation, in contrast to their PAD4(+/+) counterparts (44). Conversely, a separate study found that PAD4 deficiency had no discernible impact on polymicrobial sepsis with concurrent bacteremia and the mitigation of endotoxemic shock (159). Notably, tumor cells, shielded from cytotoxicity by NET formation, play a pivotal role in the successful metastasis of cancer in mice. Moreover, the combination of PAD4 inhibitors and immune checkpoint inhibitors has demonstrated immunotherapeutic synergy by inhibiting the process of NET formation (144). Therefore, the risks associated with targeting NETs as therapeutic interventions may vary depending on the presence of specific disorders and the immunological state of the organism. Furthermore, the degradation of NET formation presents another significant hazard, as it leads to the release of DNA and histones derived from NETs, which have the potential to trigger inflammation. Currently, therapies aimed at modulating NET formation can be categorized into two distinct groups: inhibition of NET formation and destabilization/degradation of existing NETs.
Extensive research has already been conducted on the inhibition of NET formation. Inhibiting the expression of PAD4 can prevent NET formation (160). Another study demonstrated that suppressing the enzymatic function of PAD4 disrupts NET formation in both mice and humans, although it does not seem to affect bacteremia in the context of polymicrobial sepsis (161). Recent investigations have identified BMS-P5, GSK199, and GSK484 as inhibitors of NET development, capable of suppressing associated diseases in both in vivo and in vitro settings (161, 162). NE inhibitors, which hold great promise as therapeutic targets, play a pivotal role in suppressing NET formation (132). For instance, sivelestat, an NE inhibitor, was found to suppress NET formation in mice (163). Additionally, antibodies have also been recognized for their ability to impede NET formation. One such antibody, tACPA, restrains the development of NETs in neutrophil-mediated inflammatory conditions (164). However, the approach of targeting pre-existing NETs in HCC has not been thoroughly explored. NETs play a crucial role in the pathogenesis of HCC, and focusing on inhibitors or modulators of NETs in HCC may introduce a novel therapeutic approach for managing this malignancy.
An alternative approach to target NETs involves inducing their degradation. DNase I has been shown to possess the ability to partially lyse NETs (154). The suppression of NET production by DNase I effectively eliminates the promotion of HCC growth and metastasis induced by NET formation (46). In a murine model, the direct destruction of NET formation by DNase I, along with the administration of agents possessing anti-inflammatory properties such as hydroxychloroquine or aspirin, effectively attenuates liver cancer metastasis (35). Compelling evidence demonstrates that neutrophil-derived NET formation impedes the cytolytic activity of both cytotoxic T lymphocytes and NK cells during their interactions with cancer cells (144). Moreover, the presence of NETs and an acidic tumor microenvironment significantly counteract the potential benefits of cancer therapy involving NK cell infusion.
In experimental model, we consistently observed a concurrent occurrence of autophagy and the formation of NETs. The use of autophagy inhibitors, such as wortmannin and 3-MA, effectively hindered the formation of NETs (148, 165). Furthermore, the study found that pretreatment of neutrophils with low-molecular-weight heparin (LMWH) in vitro significantly impaired their ability to initiate autophagy. In a separate study involving healthy volunteers, a single administration of parnaparin as a preventive measure rendered neutrophils incapable of triggering autophagy and generating NETs (148) (refer to Table 1 for details).
4 Discussion, concluding marks and key questions
4.1 NETs in other cancers
The recent advancement in our comprehension of cancer-associated NET formation is the identification of the ability of carcinomas to enhance neutrophil production of thrombogenic NETs. This discovery was first reported in 2012 (115). Neutrophil extracellular traps have now been associated with various non-infectious diseases, such as cancer (166), diabetes (169), autoimmunity (167), cardiovascular disease (168), and systemic lupus erythematosus (170), all of which have an inflammatory component. In the following section, we present and review the current understanding of cancer-associated NET formation, including the diverse mechanisms underlying NET formation in different types of cancers.
Despite significant advancements in our understanding of the mechanism of NETs in tumors, they still remain enigmatic. In 2013, a groundbreaking discovery was made, demonstrating the correlation between NET formation and malignancy. This finding suggested that the presence of intratumoral NETs may be associated with an unfavorable prognosis in a subset of patients diagnosed with Ewing sarcoma (171). Subsequently, the potential of NETs has been extensively investigated across diverse malignancies, revealing their oncogenic effect in the majority of cancers, although not in all conditions. Notably, mice with tumors exhibited an increase in plasma levels of NET formation compared to control mice. Neutrophils in this context tended to spontaneously form NETs, leading to thrombus formation and a pro-coagulant state (115, 172). Moreover, it was found that tumor cells stimulated the formation of NETs, thereby promoting breast cancer lung metastasis (172).
Low-density neutrophils (LDNs), which are a subset of activated neutrophils with distinct phenotypic and functional characteristics, exhibit differences compared to normal-density neutrophils (NDNs) in human peripheral blood polymorphonuclear neutrophils (PMNs) (173). In a murine model of orthotopic pancreatic adenocarcinoma, suppression of PAD4 resulted in decreased levels of circulating NET formation, leading to reduced tumor growth and improved survival rates. Furthermore, NETs stimulated pancreatic stellate cells, which were found to promote tumor proliferation (174). Similar findings were observed in an experimental melanoma model, where NETs accumulated in the TME and promoted cancer growth (175). Interestingly, the researchers also observed the presence of NETs in ulcerated tissue of melanoma patients, which were negatively associated with the patients’ prognostic outlook. However, co-incubation of NET formation and melanoma cells disrupted melanoma cell migration and viability (176). This observation suggests that the potential factors in vivo may block the anti-tumor effects of NETs in melanoma, and further exploration in this regard could be a promising direction. While the presence of tumor-associated and circulating NETs has been linked to a worse prognosis in patients with diffuse large B-cell lymphoma, the exact correlation remains to be fully elucidated in humans. Both in vivo and in vitro studies have also demonstrated that NETs promote the proliferation and migration of tumor cells (177).
In recent studies by Yang et al., the role of NETs in promoting cancer metastasis, as well as the association between NETs and neutrophil DNA, has been strongly supported (17). They investigated specific biomarkers, such as MPO and H3Cit, to identify distinct neutrophils and their associated NETs. The results revealed that liver metastases exhibited the highest level of NET infiltration. Interestingly, higher levels of serum MPO-DNA were found to be independently associated with subsequent liver metastasis, while no such correlation was observed in other organs. These findings suggest that excessive hepatic NET formation may occur prior to the detection of metastases in breast cancer patients, thereby promoting subsequent hepatic metastasis (17). Furthermore, experiments conducted on PAD4-deficient C57BL/6 mice demonstrated a significant attenuation in the formation of NETs and hepatic metastases. Conversely, NET-DNA extracted from PMA-treated neutrophils exhibited an opposite effect. Additionally, tumor cells exhibited enhanced chemotaxis towards the DNA component of hepatic NETs through high-affinity binding with a transmembrane protein called CCDC25, which is expressed on carcinoma cells. The interaction between NET formation DNA and CCDC25 promotes cancer cell migration and initiates metastasis by triggering intracellular signaling pathways (17).
It is evident that the formation of NETs plays a crucial role in cancer metastasis, particularly in the context of liver metastases. Targeting the components of hepatic NETs, such as MPO and H3Cit, may hold significant therapeutic potential for the treatment of HCC. Moreover, exploring the interplay between immune cells and cancer cells, as well as the potential of injectable hydrogels in enhancing immunotherapy, and the impact of senescence-associated secretory phenotype (SASP) on the tumor microenvironment, could provide valuable insights for future research. Ultimately, by targeting heparin as a potential therapeutic intervention and modulating the tumor immune microenvironment through the interaction of cancer cells and immune cells, novel strategies for HCC treatment may be developed.
Metastasis, the spread of cancer cells to distant tissues, is the leading cause of mortality in cancer patients, surpassing the impact of the original primary tumor. The process of metastatic initiation can persist for an extended period, potentially due to the protective effects of immune cells or inadequate angiogenic capacity. This leads to a state of uncertainty between cell proliferation and apoptosis (10, 12, 178). The factors that disrupt this delicate balance remain elusive. D. Barkan and B.L. Pierce proposed an intriguing theory suggesting that low-grade inflammation may trigger the transition from dormancy to proliferation in metastatic cells (179, 180). Recently, a study found that the recruitment and activation of neutrophils occur prior to treatment with LPS in rats with dormant lung tumor cells (142, 181). Depleting neutrophils eliminated the awakening of dormant cancer cells induced by LPS. The presence of NETs in the lung was rapidly (within 4 hours) induced by LPS instillation and persisted for 24 hours. Similar observations were made using a PAD4 inhibitor. These findings were also confirmed in a prostate cancer model exposed to tobacco smoke, a proinflammatory stimulus. Therefore, various rat models exposed to multiple inflammatory stimuli induce the awakening of dormant cancer cells through NET formation. The authors also discovered that the reactivation of quiescent carcinoma cells relies on ECM remodeling and laminin cleavage, which require matrix metallopeptidases 9 (MMP9) and NE, an enzyme associated with NETs. The appearance of a novel epitope in laminin, resulting from proteolytic remodeling, promotes cancer cell proliferation through the α3β1 integrin pathway. The presence of NETs prompts quiescent cancer cells to initiate proliferation. However, when these disseminated cells embark on their proliferation journey, they become susceptible to recognition by T cells and NK cells (178, 182, 183). The specific impact of the adaptive immune system in mouse models remains unclear. Nevertheless, lipopolysaccharide exposure elevates glucocorticoid levels (184)while simultaneously suppressing adaptive immune cells. Consequently, the combination of lipopolysaccharide and smoking may facilitate the growth of dormant cells. This is achieved by triggering signaling pathways through NETs to initiate proliferation and concurrently disrupting immune control through glucocorticoids.
4.2 Conclusions
Over the past decade, the crucial role of neutrophils in both inflammatory and non-inflammatory diseases has been firmly established. One of the contributing factors is NET. In the context of cancer, particularly HCC, NET formation may play a significant role in disease progression. However, studying NETs presents challenges, as primary human neutrophils cannot be transfected, and inhibiting NET formation pathways in vivo is difficult. Furthermore, the heterogeneity between human and mouse neutrophils raises questions about the relevance of NET formation in tumors, as most studies rely on mouse models (13, 98, 185–187).
Neutrophils can polarize into two subtypes: N1, which exhibits anti-tumorigenic properties (188, 189), and N2, which displays pro-tumorigenic characteristics (190–192). Both subtypes have the capacity to generate NETs. Additionally, other matrix cells in the TME, such as basophils (193–195), eosinophils (27, 195–198), mast cells (199, 200), and macrophages (201), can also release extracellular DNA traps. However, the specific components of NETs or extracellular DNA traps that possess anti-tumorigenic or pro-tumor effects remain unclear. Therefore, it is crucial to inhibit NET formation without compromising other effective constituents or generating detrimental degradation products of neutrophil extracellular traps. This requires a comprehensive understanding of the mechanisms involved in NET formation and degradation. Furthermore, targeting NETs and related enzymes may hold promise as a therapeutic strategy for combating cancer metastasis.
The concentration of NETs is elevated in both blood serum and tissue specimens from patients with HCC at different stages of liver cancer, including HCC patients with HBV, those who have undergone liver surgery (including liver IRI), NASH, and liver cirrhosis. While most research has focused on the role of NETs in regulating tumor progression and proliferation, further investigation is needed to understand the specific components of NETs that interact with signaling pathways. Additionally, more research is required to identify the mechanisms by which NETs are regulated in vivo. Although previous in vivo or in vitro studies have primarily induced NET formation using LPS or PMA, the detailed mechanisms underlying the interplay between NETs and other immune cell components, such as macrophages, CD4+ T cells, and Tregs, remain largely unexplored. Moreover, various risk factors associated with disease progression, including diabetes, age, and the presence of advanced cirrhosis or fibrosis, have been identified through clinical research. However, there are still gaps in our understanding of the pathobiology of HCC.
The formation of NETs has been identified as a valuable biomarker for diagnostic, predictive, and prognostic purposes in various human cancers, including HCC. Abundant evidence supports the notion that tumor-associated NET formation plays a pivotal role in facilitating the unchecked proliferation of malignant cells, creating a permissive environment for cancer development, expediting tumor progression during systemic infection, and promoting cancer-associated thrombosis in HCC. Furthermore, the presence of NETs in HCC patients has been shown to enhance metastatic potential and contribute to disease recurrence. The potential of NETs as therapeutic targets for HCC holds great promise, as targeting NETs could potentially prevent the progression of liver cancer, inhibit metastasis, mitigate cancer-associated thrombosis, and reduce post-operative recurrence. Clinical trials investigating anti-NET therapies, either alone or in combination with immune checkpoint inhibitors (ICI), are currently underway. It is hoped that combination therapy will yield a synergistic effect, effectively treating HCC patients by counteracting NET-mediated immunosuppression in the presence of ICI.
4.3 Key questions for further research
It is necessary to determine the immunomodulatory properties of NETs, such as their antibacterial activity, which play a crucial role in triggering or suppressing inflammation, in order to further elucidate their mechanisms. Conversely, changes in the composition and structure of NETs may result from variations in proteomic and transcriptomic profiles among neutrophil subpopulations, as well as activation by diverse stimuli. These aspects warrant further investigation. Moreover, the quantity, timing, and site of NET release exert a profound influence on the balance between detrimental and beneficial outcomes. Given the inherent heterogeneity of neutrophils within the hepatocellular carcinoma TME (11), exploring the involvement of distinct neutrophil subpopulations in the timing and quantity of NET release within the context of hepatocellular carcinoma (secondary dysregulation induced by disease initiation and other factors) may represent a promising research avenue. Furthermore, NETs have been identified in various human diseases, prompting the need to investigate their potential utility as markers of therapeutic efficacy. Additionally, further investigate the mechanisms observed in animal models, including those related to HCC, and determine their applicability and relevance in clinical settings is crucial. Nonetheless, it is worth noting that most current NET research remains mechanistic in nature, with clinical translation remaining a distant goal. Nevertheless, a more comprehensive understanding of the molecular mechanisms underlying NETs holds the potential to bridge this gap. Lastly, as strategies for neutrophil clearance are often unviable in the context of most human conditions, the field faces the challenge of identifying pathogenic cell subpopulations that can be effectively targeted during therapy (Figure 6).
Author contributions
WXZ: Data curation, Writing – original draft, Writing – review & editing, Conceptualization, Formal Analysis, Investigation, Software, Validation. CF: Writing – original draft. SD: Conceptualization, Investigation, Software, Writing – original draft. XL: Methodology, Supervision, Writing – original draft. HC: Data curation, Writing – original draft. WCZ: Writing – review & editing.
Funding
The author(s) declare financial support was received for the research, authorship, and/or publication of this article. This work was financially supported by the following funding: (1) National Natural Science Foundation of China [grant number 82260555]; (2) Medical Innovation and Development Project of Lanzhou University [grant number lzuyxcx-2022-177]; (3) Major Science and Technology Projects of Gansu Province [grant number 22ZD6FA021-4]; (4) Science and Technology Program of Gansu Province [grant number 23JRRA0996].
Acknowledgments
Thanks to HOME for Researchers, for providing drawing material.
Conflict of interest
The authors declare that the research was conducted in the absence of any commercial or financial relationships that could be construed as a potential conflict of interest.
Publisher’s note
All claims expressed in this article are solely those of the authors and do not necessarily represent those of their affiliated organizations, or those of the publisher, the editors and the reviewers. Any product that may be evaluated in this article, or claim that may be made by its manufacturer, is not guaranteed or endorsed by the publisher.
References
1. Sung H, Ferlay J, Siegel RL, Laversanne M, Soerjomataram I, Jemal A, et al. Global cancer statistics 2020: GLOBOCAN estimates of incidence and mortality worldwide for 36 cancers in 185 countries. CA Cancer J Clin (2021) 71:209–49. doi: 10.3322/caac.21660
2. Vogel A, Meyer T, Sapisochin G, Salem R, Saborowski A. Hepatocellular carcinoma. Lancet (2022) 400:1345–62. doi: 10.1016/s0140-6736(22)01200-4
3. Villanueva A. Hepatocellular carcinoma. N Engl J Med (2019) 380:1450–62. doi: 10.1056/NEJMra1713263
4. Geh D, Leslie J, Rumney R, Reeves HL, Bird TG, Mann DA. Neutrophils as potential therapeutic targets in hepatocellular carcinoma. Nat Rev Gastroenterol Hepatol (2022) 19:257–73. doi: 10.1038/s41575-021-00568-5
5. Zhou SL, Dai Z, Zhou ZJ, Wang XY, Yang GH, Wang Z, et al. Overexpression of CXCL5 mediates neutrophil infiltration and indicates poor prognosis for hepatocellular carcinoma. Hepatology (2012) 56:2242–54. doi: 10.1002/hep.25907
6. Zhou SL, Zhou ZJ, Hu ZQ, Huang XW, Wang Z, Chen EB, et al. Tumor-associated neutrophils recruit macrophages and T-regulatory cells to promote progression of hepatocellular carcinoma and resistance to sorafenib. Gastroenterology (2016) 150:1646–1658.e1617. doi: 10.1053/j.gastro.2016.02.040
7. Kuang DM, Zhao Q, Wu Y, Peng C, Wang J, Xu Z, et al. Peritumoral neutrophils link inflammatory response to disease progression by fostering angiogenesis in hepatocellular carcinoma. J Hepatol (2011) 54:948–55. doi: 10.1016/j.jhep.2010.08.041
8. Soehnlein O, Lindbom L. Phagocyte partnership during the onset and resolution of inflammation. Nat Rev Immunol (2010) 10:427–39. doi: 10.1038/nri2779
9. Nauseef WM, Borregaard N. Neutrophils at work. Nat Immunol (2014) 15:602–11. doi: 10.1038/ni.2921
10. Xiao Y, Cong M, Li J, He D, Wu Q, Tian P, et al. Cathepsin C promotes breast cancer lung metastasis by modulating neutrophil infiltration and neutrophil extracellular trap formation. Cancer Cell (2021) 39:423–437.e427. doi: 10.1016/j.ccell.2020.12.012
11. Xue R, Zhang Q, Cao Q, Kong R, Xiang X, Liu H, et al. Liver tumour immune microenvironment subtypes and neutrophil heterogeneity. Nature (2022) 612:141–7. doi: 10.1038/s41586-022-05400-x
12. Xia X, Zhang Z, Zhu C, Ni B, Wang S, Yang S, et al. Neutrophil extracellular traps promote metastasis in gastric cancer patients with postoperative abdominal infectious complications. Nat Commun (2022) 13:1017. doi: 10.1038/s41467-022-28492-5
13. Xie X, Shi Q, Wu P, Zhang X, Kambara H, Su J, et al. Single-cell transcriptome profiling reveals neutrophil heterogeneity in homeostasis and infection. Nat Immunol (2020) 21:1119–33. doi: 10.1038/s41590-020-0736-z
14. Doeing DC, Borowicz JL, Crockett ET. Gender dimorphism in differential peripheral blood leukocyte counts in mice using cardiac, tail, foot, and saphenous vein puncture methods. BMC Clin Pathol (2003) 3:3. doi: 10.1186/1472-6890-3-3
15. Mestas J, Hughes CC. Of mice and not men: differences between mouse and human immunology. J Immunol (2004) 172:2731–8. doi: 10.4049/jimmunol.172.5.2731
16. Calvente CJ, Tameda M, Johnson CD, Del Pilar H, Lin YC, Adronikou N, et al. Neutrophils contribute to spontaneous resolution of liver inflammation and fibrosis via microRNA-223. J Clin Invest (2019) 129:4091–109. doi: 10.1172/jci122258
17. Yang L, Liu Q, Zhang X, Liu X, Zhou B, Chen J, et al. DNA of neutrophil extracellular traps promotes cancer metastasis via CCDC25. Nature (2020) 583:133–8. doi: 10.1038/s41586-020-2394-6
18. Finn RS, Qin S, Ikeda M, Galle PR, Ducreux M, Kim TY, et al. Atezolizumab plus bevacizumab in unresectable hepatocellular carcinoma. N Engl J Med (2020) 382:1894–905. doi: 10.1056/NEJMoa1915745
19. El-Khoueiry AB, Sangro B, Yau T, Crocenzi TS, Kudo M, Hsu C, et al. Nivolumab in patients with advanced hepatocellular carcinoma (CheckMate 040): an open-label, non-comparative, phase 1/2 dose escalation and expansion trial. Lancet (2017) 389:2492–502. doi: 10.1016/s0140-6736(17)31046-2
20. Finn RS, Ryoo BY, Merle P, Kudo M, Bouattour M, Lim HY, et al. Pembrolizumab as second-line therapy in patients with advanced hepatocellular carcinoma in KEYNOTE-240: A randomized, double-blind, phase III trial. J Clin Oncol (2020) 38:193–202. doi: 10.1200/jco.19.01307
21. Finn RS, Qin S, Ikeda M, Galle PR, Ducreux M, Kim T-Y, et al. IMbrave150: Updated overall survival (OS) data from a global, randomized, open-label phase III study of atezolizumab (atezo) plus bevacizumab (bev) versus sorafenib (sor) in patients (pts) with unresectable hepatocellular carcinoma (HCC). J Clin Oncol (2021) 39. doi: 10.1200/JCO.2021.39.3_suppl.267
22. Caudrillier A, Kessenbrock K, Gilliss BM, Nguyen JX, Marques MB, Monestier M, et al. Platelets induce neutrophil extracellular traps in transfusion-related acute lung injury. J Clin Invest (2012) 122:2661–71. doi: 10.1172/jci61303
23. Yousefi S, Mihalache C, Kozlowski E, Schmid I, Simon HU. Viable neutrophils release mitochondrial DNA to form neutrophil extracellular traps. Cell Death Differ (2009) 16:1438–44. doi: 10.1038/cdd.2009.96
24. Brinkmann V, Reichard U, Goosmann C, Fauler B, Uhlemann Y, Weiss DS, et al. Neutrophil extracellular traps kill bacteria. Science (2004) 303:1532–5. doi: 10.1126/science.1092385
25. Yousefi S, Gold JA, Andina N, Lee JJ, Kelly AM, Kozlowski E, et al. Catapult-like release of mitochondrial DNA by eosinophils contributes to antibacterial defense. Nat Med (2008) 14:949–53. doi: 10.1038/nm.1855
26. Amini P, Stojkov D, Felser A, Jackson CB, Courage C, Schaller A, et al. Neutrophil extracellular trap formation requires OPA1-dependent glycolytic ATP production. Nat Commun (2018) 9:2958. doi: 10.1038/s41467-018-05387-y
27. Muniz VS, Silva JC, Braga YAV, Melo RCN, Ueki S, Takeda M, et al. Eosinophils release extracellular DNA traps in response to Aspergillus fumigatus. J Allergy Clin Immunol (2018) 141:571–585.e577. doi: 10.1016/j.jaci.2017.07.048
28. Skendros P, Mitsios A, Chrysanthopoulou A, Mastellos DC, Metallidis S, Rafailidis P, et al. Complement and tissue factor-enriched neutrophil extracellular traps are key drivers in COVID-19 immunothrombosis. J Clin Invest (2020) 130:6151–7. doi: 10.1172/jci141374
29. Sivanandham R, Brocca-Cofano E, Krampe N, Falwell E, Venkatraman SMK, Ribeiro RM, et al. Neutrophil extracellular trap production contributes to pathogenesis in SIV-infected nonhuman primates. J Clin Invest (2018) 128:5178–83. doi: 10.1172/jci99420
30. Yazdani HO, Roy E, Comerci AJ, van der Windt DJ, Zhang H, Huang H, et al. Neutrophil extracellular traps drive mitochondrial homeostasis in tumors to augment growth. Cancer Res (2019) 79:5626–39. doi: 10.1158/0008-5472.Can-19-0800
31. Middleton EA, He XY, Denorme F, Campbell RA, Ng D, Salvatore SP, et al. Neutrophil extracellular traps contribute to immunothrombosis in COVID-19 acute respiratory distress syndrome. Blood (2020) 136:1169–79. doi: 10.1182/blood.2020007008
32. Pieterse E, Rother N, Garsen M, Hofstra JM, Satchell SC, Hoffmann M, et al. Neutrophil extracellular traps drive endothelial-to-mesenchymal transition. Arterioscler Thromb Vasc Biol (2017) 37:1371–9. doi: 10.1161/atvbaha.117.309002
33. Hisada Y, Grover SP, Maqsood A, Houston R, Ay C, Noubouossie DF, et al. Neutrophils and neutrophil extracellular traps enhance venous thrombosis in mice bearing human pancreatic tumors. Haematologica (2020) 105:218–25. doi: 10.3324/haematol.2019.217083
34. Tohme S, Yazdani HO, Al-Khafaji AB, Chidi AP, Loughran P, Mowen K, et al. Neutrophil extracellular traps promote the development and progression of liver metastases after surgical stress. Cancer Res (2016) 76:1367–80. doi: 10.1158/0008-5472.Can-15-1591
35. Yang LY, Luo Q, Lu L, Zhu WW, Sun HT, Wei R, et al. Increased neutrophil extracellular traps promote metastasis potential of hepatocellular carcinoma via provoking tumorous inflammatory response. J Hematol Oncol (2020) 13:3. doi: 10.1186/s13045-019-0836-0
36. van der Windt DJ, Sud V, Zhang H, Varley PR, Goswami J, Yazdani HO, et al. Neutrophil extracellular traps promote inflammation and development of hepatocellular carcinoma in nonalcoholic steatohepatitis. Hepatology (2018) 68:1347–60. doi: 10.1002/hep.29914
37. Fuchs TA, Abed U, Goosmann C, Hurwitz R, Schulze I, Wahn V, et al. Novel cell death program leads to neutrophil extracellular traps. J Cell Biol (2007) 176:231–41. doi: 10.1083/jcb.200606027
38. Takei H, Araki A, Watanabe H, Ichinose A, Sendo F. Rapid killing of human neutrophils by the potent activator phorbol 12-myristate 13-acetate (PMA) accompanied by changes different from typical apoptosis or necrosis. J Leukoc Biol (1996) 59:229–40. doi: 10.1002/jlb.59.2.229
39. Azevedo EP, Rochael NC, Guimarães-Costa AB, de Souza-Vieira TS, Ganilho J, Saraiva EM, et al. A metabolic shift toward pentose phosphate pathway is necessary for amyloid fibril- and phorbol 12-myristate 13-acetate-induced neutrophil extracellular trap (NET) formation. J Biol Chem (2015) 290:22174–83. doi: 10.1074/jbc.M115.640094
40. Sprenkeler EGG, Tool ATJ, Henriet SSV, van Bruggen R, Kuijpers TW. Formation of neutrophil extracellular traps requires actin cytoskeleton rearrangements. Blood (2022) 139:3166–80. doi: 10.1182/blood.2021013565
41. Chen F, Chu C, Wang X, Yang C, Deng Y, Duan Z, et al. Hesperetin attenuates sepsis-induced intestinal barrier injury by regulating neutrophil extracellular trap formation via the ROS/autophagy signaling pathway. Food Funct (2023) 14:4213–27. doi: 10.1039/d2fo02707k
42. Komorowicz E, Balázs N, Tanka-Salamon A, Varga Z, Szabó L, Bóta A, et al. Size- and charge-dependent modulation of the lytic susceptibility and mechanical stability of fibrin-histone clots by heparin and polyphosphate variants. J Thromb Haemost (2021) 19:1307–18. doi: 10.1111/jth.15258
43. Barnes BJ, Adrover JM, Baxter-Stoltzfus A, Borczuk A, Cools-Lartigue J, Crawford JM, et al. Targeting potential drivers of COVID-19: Neutrophil extracellular traps. J Exp Med (2020) 217. doi: 10.1084/jem.20200652
44. Li P, Li M, Lindberg MR, Kennett MJ, Xiong N, Wang Y. PAD4 is essential for antibacterial innate immunity mediated by neutrophil extracellular traps. J Exp Med (2010) 207:1853–62. doi: 10.1084/jem.20100239
45. Wu Z, Deng Q, Pan B, Alam HB, Tian Y, Bhatti UF, et al. Inhibition of PAD2 improves survival in a mouse model of lethal LPS-induced endotoxic shock. Inflammation (2020) 43:1436–45. doi: 10.1007/s10753-020-01221-0
46. Zhan X, Wu R, Kong XH, You Y, He K, Sun XY, et al. Elevated neutrophil extracellular traps by HBV-mediated S100A9-TLR4/RAGE-ROS cascade facilitate the growth and metastasis of hepatocellular carcinoma. Cancer Commun (Lond) (2023) 43:225–45. doi: 10.1002/cac2.12388
47. Li N, Zheng X, Chen M, Huang L, Chen L, Huo R, et al. Deficient DNASE1L3 facilitates neutrophil extracellular traps-induced invasion via cyclic GMP-AMP synthase and the non-canonical NF-κB pathway in diabetic hepatocellular carcinoma. Clin Transl Immunol (2022) 11:e1386. doi: 10.1002/cti2.1386
48. Zhang S, Zhang Q, Wang F, Guo X, Liu T, Zhao Y, et al. Hydroxychloroquine inhibiting neutrophil extracellular trap formation alleviates hepatic ischemia/reperfusion injury by blocking TLR9 in mice. Clin Immunol (2020) 216:108461. doi: 10.1016/j.clim.2020.108461
49. Casanova-Acebes M, Nicolás-Ávila JA, Li JL, Garcja-Silva S, Balachander A, Rubio-Ponce 142. A, et al. Neutrophils instruct homeostatic and pathological states in naive tissues. J Exp Med (2018) 215:2778–95. doi: 10.1084/jem.20181468
50. Cedervall J, Zhang Y, Huang H, Zhang L, Femel J, Dimberg A, et al. Neutrophil extracellular traps accumulate in peripheral blood vessels and compromise organ function in tumor-bearing animals. Cancer Res (2015) 75:2653–62. doi: 10.1158/0008-5472.Can-14-3299
51. Karlsson A, Dahlgren C. Assembly and activation of the neutrophil NADPH oxidase in granule membranes. Antioxid Redox Signal (2002) 4:49–60. doi: 10.1089/152308602753625852
52. Lood C, Blanco LP, Purmalek MM, Carmona-Rivera C, De Ravin SS, Smith CK, et al. Neutrophil extracellular traps enriched in oxidized mitochondrial DNA are interferogenic and contribute to lupus-like disease. Nat Med (2016) 22:146–53. doi: 10.1038/nm.4027
53. Bianchi M, Hakkim A, Brinkmann V, Siler U, Seger RA, Zychlinsky A, et al. Restoration of NET formation by gene therapy in CGD controls aspergillosis. Blood (2009) 114:2619–22. doi: 10.1182/blood-2009-05-221606
54. Billings EA, Lee CS, Owen KA, D'Souza RS, Ravichandran KS, Casanova JE. The adhesion GPCR BAI1 mediates macrophage ROS production and microbicidal activity against Gram-negative bacteria. Sci Signal (2016) 9:ra14. doi: 10.1126/scisignal.aac6250
55. Adrover JM, Aroca-Crevillén A, Crainiciuc G, Ostos F, Rojas-Vega Y, Rubio-Ponce A, et al. Programmed ‘disarming’ of the neutrophil proteome reduces the magnitude of inflammation. Nat Immunol (2020) 21:135–44. doi: 10.1038/s41590-019-0571-2
56. Al-Khafaji AB, Tohme S, Yazdani HO, Miller D, Huang H, Tsung A, et al. Superoxide induces Neutrophil Extracellular Trap Formation in a TLR-4 and NOX-dependent mechanism. Mol Med (2016) 22:621–31. doi: 10.2119/molmed.2016.00054
57. Tong M, Smith AH, Abrahams VM. Activated neutrophils propagate fetal membrane inflammation and weakening through ERK and neutrophil extracellular trap-induced TLR-9 signaling. J Immunol (2021) 206:1039–45. doi: 10.4049/jimmunol.2001268
58. Sadiku P, Willson JA, Ryan EM, Sammut D, Coelho P, Watts ER, et al. Neutrophils fuel effective immune responses through gluconeogenesis and glycogenesis. Cell Metab (2021) 33:411–423.e414. doi: 10.1016/j.cmet.2020.11.016
59. Hsu BE, Tabariès S, Johnson RM, Andrzejewski S, Senecal J, Lehuédé C, et al. Immature low-density neutrophils exhibit metabolic flexibility that facilitates breast cancer liver metastasis. Cell Rep (2019) 27:3902–3915.e3906. doi: 10.1016/j.celrep.2019.05.091
60. Hakkim A, Fuchs TA, Martinez NE, Hess S, Prinz H, Zychlinsky A, et al. Activation of the Raf-MEK-ERK pathway is required for neutrophil extracellular trap formation. Nat Chem Biol (2011) 7:75–7. doi: 10.1038/nchembio.496
61. Keshari RS, Verma A, Barthwal MK, Dikshit M. Reactive oxygen species-induced activation of ERK and p38 MAPK mediates PMA-induced NETs release from human neutrophils. J Cell Biochem (2013) 114:532–40. doi: 10.1002/jcb.24391
62. Kolaczkowska E, Jenne CN, Surewaard BG, Thanabalasuriar A, Lee WY, Sanz MJ, et al. Molecular mechanisms of NET formation and degradation revealed by intravital imaging in the liver vasculature. Nat Commun (2015) 6:6673. doi: 10.1038/ncomms7673
63. Chen KW, Monteleone M, Boucher D, Sollberger G, Ramnath D, Condon ND, et al. Noncanonical inflammasome signaling elicits gasdermin D-dependent neutrophil extracellular traps. Sci Immunol (2018) 3. doi: 10.1126/sciimmunol.aar6676
64. Sollberger G, Choidas A, Burn GL, Habenberger P, Di Lucrezia R, Kordes S, et al. Gasdermin D plays a vital role in the generation of neutrophil extracellular traps. Sci Immunol (2018) 3. doi: 10.1126/sciimmunol.aar6689
65. Neubert E, Meyer D, Rocca F, Günay G, Kwaczala-Tessmann A, Grandke J, et al. Chromatin swelling drives neutrophil extracellular trap release. Nat Commun (2018) 9:3767. doi: 10.1038/s41467-018-06263-5
66. Neeli I, Dwivedi N, Khan S, Radic M. Regulation of extracellular chromatin release from neutrophils. J Innate Immun (2009) 1:194–201. doi: 10.1159/000206974
67. Wang Y, Li M, Stadler S, Correll S, Li P, Wang D, et al. Histone hypercitrullination mediates chromatin decondensation and neutrophil extracellular trap formation. J Cell Biol (2009) 184:205–13. doi: 10.1083/jcb.200806072
68. Kenny EF, Herzig A, Krüger R, Muth A, Mondal S, Thompson PR, et al. Diverse stimuli engage different neutrophil extracellular trap pathways. Elife (2017) 6. doi: 10.7554/eLife.24437
69. Warnatsch A, Ioannou M, Wang Q, Papayannopoulos V. Inflammation. Neutrophil extracellular traps license macrophages for cytokine production in atherosclerosis. Science (2015) 349:316–20. doi: 10.1126/science.aaa8064
70. Papayannopoulos V, Metzler KD, Hakkim A, Zychlinsky A. Neutrophil elastase and myeloperoxidase regulate the formation of neutrophil extracellular traps. J Cell Biol (2010) 191:677–91. doi: 10.1083/jcb.201006052
71. Metzler KD, Goosmann C, Lubojemska A, Zychlinsky A. & Papayannopoulos, V. A myeloperoxidase-containing complex regulates neutrophil elastase release and actin dynamics during NETosis. Cell Rep (2014) 8:883–96. doi: 10.1016/j.celrep.2014.06.044
72. Pilsczek FH, Salina D, Poon KK, Fahey C, Yipp BG, Sibley CD, et al. A novel mechanism of rapid nuclear neutrophil extracellular trap formation in response to Staphylococcus aureus. J Immunol (2010) 185:7413–25. doi: 10.4049/jimmunol.1000675
73. Jorch SK, Kubes P. An emerging role for neutrophil extracellular traps in noninfectious disease. Nat Med (2017) 23:279–87. doi: 10.1038/nm.4294
74. Bukong TN, Cho Y, Iracheta-Vellve A, Saha B, Lowe P, Adejumo A, et al. Abnormal neutrophil traps and impaired efferocytosis contribute to liver injury and sepsis severity after binge alcohol use. J Hepatol (2018) 69:1145–54. doi: 10.1016/j.jhep.2018.07.005
75. Ermert D, Urban CF, Laube B, Goosmann C, Zychlinsky A, Brinkmann V, et al. Mouse neutrophil extracellular traps in microbial infections. J Innate Immun (2009) 1:181–93. doi: 10.1159/000205281
76. Cho Y, Bukong TN, Tornai D, Babuta M, Vlachos IS, Kanata E, et al. Neutrophil extracellular traps contribute to liver damage and increase defective low-density neutrophils in alcohol-associated hepatitis. J Hepatol (2023) 78:28–44. doi: 10.1016/j.jhep.2022.08.029
77. Zhang R, Su L, Fu M, Wang Z, Tan L, Chen H, et al. CD177(+) cells produce neutrophil extracellular traps that promote biliary atresia. J Hepatol (2022) 77:1299–310. doi: 10.1016/j.jhep.2022.06.015
78. Clemmensen SN. Olfactomedin 4 defines a subset of human neutrophils. J Leukoc Biol (2012) 91:495–500. doi: 10.1189/jlb.0811417
79. Alder MN, Mallela J, Opoka AM, Lahni P, Hildeman DA, Wong HR, et al. Olfactomedin 4 marks a subset of neutrophils in mice. Innate Immun (2019) 25:22–33. doi: 10.1177/1753425918817611
80. Welin A, Amirbeagi F, Christenson K, Björkman L, Björnsdottir H, Forsman H, et al. The human neutrophil subsets defined by the presence or absence of OLFM4 both transmigrate into tissue in vivo and give rise to distinct NETs in vitro. PloS One (2013) 8:e69575. doi: 10.1371/journal.pone.0069575
81. Alder MN, Opoka AM, Lahni P, Hildeman DA, Wong HR. Olfactomedin-4 is a candidate marker for a pathogenic neutrophil subset in septic shock. Crit Care Med (2017) 45:e426–32. doi: 10.1097/ccm.0000000000002102
82. Zhou GX, Liu ZJ. Potential roles of neutrophils in regulating intestinal mucosal inflammation of inflammatory bowel disease. J Dig Dis (2017) 18:495–503. doi: 10.1111/1751-2980.12540
83. Zhou G, Yu L, Fang L, Yang W, Yu T, Miao Y, et al. CD177(+) neutrophils as functionally activated neutrophils negatively regulate IBD. Gut (2018) 67:1052–63. doi: 10.1136/gutjnl-2016-313535
84. Amulic B, Knackstedt SL, Abu Abed U, Deigendesch N, Harbort CJ, Caffrey BE, et al. Cell-cycle proteins control production of neutrophil extracellular traps. Dev Cell (2017) 43:449–462.e445. doi: 10.1016/j.devcel.2017.10.013
85. Albrengues J, Wysocki RW, Maiorino L, Egeblad M. Re-cyclin’ Cell-cycle components to make NETs. Dev Cell (2017) 43:379–80. doi: 10.1016/j.devcel.2017.11.002
86. Döring Y, Soehnlein O, Weber C. Neutrophil extracellular traps in atherosclerosis and atherothrombosis. Circ Res (2017) 120:736–43. doi: 10.1161/circresaha.116.309692
87. Halverson TW, Wilton M, Poon KK, Petri B, Lewenza S. DNA is an antimicrobial component of neutrophil extracellular traps. PloS Pathog (2015) 11:e1004593. doi: 10.1371/journal.ppat.1004593
88. Metzemaekers M, Gouwy M, Proost P. Neutrophil chemoattractant receptors in health and disease: double-edged swords. Cell Mol Immunol (2020) 17:433–50. doi: 10.1038/s41423-020-0412-0
89. Schauer C, Janko C, Munoz LE, Zhao Y, Kienhöfer D, Frey B, et al. Aggregated neutrophil extracellular traps limit inflammation by degrading cytokines and chemokines. Nat Med (2014) 20:511–7. doi: 10.1038/nm.3547
90. Csomós K, Kristóf E, Jakob B, Csomós I, Kovács G, Rotem O, et al. Protein cross-linking by chlorinated polyamines and transglutamylation stabilizes neutrophil extracellular traps. Cell Death Dis (2016) 7:e2332. doi: 10.1038/cddis.2016.200
91. Knight JS, Carmona-Rivera C, Kaplan MJ. Proteins derived from neutrophil extracellular traps may serve as self-antigens and mediate organ damage in autoimmune diseases. Front Immunol (2012) 3:380. doi: 10.3389/fimmu.2012.00380
92. Martinelli S, Urosevic M, Daryadel A, Oberholzer PA, Baumann C, Fey MF, et al. Induction of genes mediating interferon-dependent extracellular trap formation during neutrophil differentiation. J Biol Chem (2004) 279:44123–32. doi: 10.1074/jbc.M405883200
93. Blazkova J, Gupta S, Liu Y, Gaudilliere B, Ganio EA, Bolen CR, et al. Multicenter systems analysis of human blood reveals immature neutrophils in males and during pregnancy. J Immunol (2017) 198:2479–88. doi: 10.4049/jimmunol.1601855
94. Wang Z, Yang F, He Z, Liang C. Light-induced circadian rhythm disorder leads to microvascular dysfunction via up-regulating NETs. Microvasc Res (2023) 150:104592. doi: 10.1016/j.mvr.2023.104592
95. He L, Fan Y, Zhang Y, Tu T, Zhang Q, Yuan F, et al. Single-cell transcriptomic analysis reveals circadian rhythm disruption associated with poor prognosis and drug-resistance in lung adenocarcinoma. J Pineal Res (2022) 73:e12803. doi: 10.1111/jpi.12803
96. Aiello I, Fedele MLM, Román F, Marpegan L, Caldart C, Chiesa JJ, et al. Circadian disruption promotes tumor-immune microenvironment remodeling favoring tumor cell proliferation. Sci Adv (2020) 6. doi: 10.1126/sciadv.aaz4530
97. Yu S, Tang Q, Chen G, Lu X, Yin Y, Xie M, et al. Circadian rhythm modulates endochondral bone formation via MTR1/AMPKβ1/BMAL1 signaling axis. Cell Death Differ (2022) 29:874–87. doi: 10.1038/s41418-021-00919-4
98. Zhang D, Chen G, Manwani D, Mortha A, Xu C, Faith JJ, et al. Neutrophil ageing is regulated by the microbiome. Nature (2015) 525:528–32. doi: 10.1038/nature15367
99. Xu C, Lee SK, Zhang D, Frenette PS. The gut microbiome regulates psychological-stress-induced inflammation. Immunity (2020) 53:417–428.e414. doi: 10.1016/j.immuni.2020.06.025
100. Adrover JM, Del Fresno C, Crainiciuc G, Cuartero MI, Casanova-Acebes M, Weiss LA, et al. A neutrophil timer coordinates immune defense and vascular protection. Immunity (2019) 50:390–402.e310. doi: 10.1016/j.immuni.2019.01.002
101. Dicker AJ, Crichton ML, Pumphrey EG, Cassidy AJ, Suarez-Cuartin G, Sibila O, et al. Neutrophil extracellular traps are associated with disease severity and microbiota diversity in patients with chronic obstructive pulmonary disease. J Allergy Clin Immunol (2018) 141:117–27. doi: 10.1016/j.jaci.2017.04.022
102. Ascher S, Wilms E, Pontarollo G, Formes H, Bayer F, Müller M, et al. Gut microbiota restricts NETosis in acute mesenteric ischemia-reperfusion injury. Arterioscler Thromb Vasc Biol (2020) 40:2279–92. doi: 10.1161/atvbaha.120.314491
103. Vong L, Lorentz RJ, Assa A, Glogauer M, Sherman PM. Probiotic Lactobacillus rhamnosus inhibits the formation of neutrophil extracellular traps. J Immunol (2014) 192:1870–7. doi: 10.4049/jimmunol.1302286
104. Tuganbaev T, Lorentz RJ, Assa A, Glogauer M, Sherman PM. Diet diurnally regulates small intestinal microbiome-epithelial-immune homeostasis and enteritis. Cell (2020) 182:1441–1459.e1421. doi: 10.1016/j.cell.2020.08.027
105. Kauffman CA. Fungal infections in older adults. Clin Infect Dis (2001) 33:550–5. doi: 10.1086/322685
106. Xu F, Zhang C, Zou Z, Fan EKY, Chen L, Li Y, et al. Aging-related Atg5 defect impairs neutrophil extracellular traps formation. Immunology (2017) 151:417–32. doi: 10.1111/imm.12740
107. Hazeldine J, Harris P, Chapple IL, Grant M, Greenwood H, Livesey A, et al. Impaired neutrophil extracellular trap formation: a novel defect in the innate immune system of aged individuals. Aging Cell (2014) 13:690–8. doi: 10.1111/acel.12222
108. Jiménez-Alcázar M, Rangaswamy C, Panda R, Bitterling J, Simsek YJ, Long AT, et al. Host DNases prevent vascular occlusion by neutrophil extracellular traps. Science (2017) 358:1202–6. doi: 10.1126/science.aam8897
109. Fuchs TA, Brill A, Duerschmied D, Schatzberg D, Monestier M, Myers DD Jr, et al. Extracellular DNA traps promote thrombosis. Proc Natl Acad Sci U.S.A. (2010) 107:15880–5. doi: 10.1073/pnas.1005743107
110. Garcia-Romo GS, Caielli S, Vega B, Connolly J, Allantaz F, Xu Z, et al. Netting neutrophils are major inducers of type I IFN production in pediatric systemic lupus erythematosus. Sci Transl Med (2011) 3:73ra20. doi: 10.1126/scitranslmed.3001201
111. Schumski A, Ortega-Gómez A, Wichapong K, Winter C, Lemnitzer P, Viola JR, et al. Endotoxinemia accelerates atherosclerosis through electrostatic charge-mediated monocyte adhesion. Circulation (2021) 143:254–66. doi: 10.1161/circulationaha.120.046677
112. Silvestre-Roig C, Braster Q, Wichapong K, Lee EY, Teulon JM, Berrebeh N, et al. Externalized histone H4 orchestrates chronic inflammation by inducing lytic cell death. Nature (2019) 569:236–40. doi: 10.1038/s41586-019-1167-6
113. Li XF, Chen DP, Ouyang FZ, Chen MM, Wu Y, Kuang DM, et al. Increased autophagy sustains the survival and pro-tumourigenic effects of neutrophils in human hepatocellular carcinoma. J Hepatol (2015) 62:131–9. doi: 10.1016/j.jhep.2014.08.023
114. Kaltenmeier C, Yazdani HO, Morder K, Geller DA, Simmons RL, Tohme S, et al. Neutrophil extracellular traps promote T cell exhaustion in the tumor microenvironment. Front Immunol (2021) 12:785222. doi: 10.3389/fimmu.2021.785222
115. Demers M, Krause DS, Schatzberg D, Martinod K, Voorhees JR, Fuchs TA, et al. Cancers predispose neutrophils to release extracellular DNA traps that contribute to cancer-associated thrombosis. Proc Natl Acad Sci U.S.A. (2012) 109:13076–81. doi: 10.1073/pnas.1200419109
116. Katsarou A, Moustakas II, Pyrina I, Lembessis P, Koutsilieris M, Chatzigeorgiou A, et al. Metabolic inflammation as an instigator of fibrosis during non-alcoholic fatty liver disease. World J Gastroenterol (2020) 26:1993–2011. doi: 10.3748/wjg.v26.i17.1993
117. Zhang P, Chen Z, Kuang H, Liu T, Zhu J, Zhou L, et al. Neuregulin 4 suppresses NASH-HCC development by restraining tumor-prone liver microenvironment. Cell Metab (2022) 34:1359–1376.e1357. doi: 10.1016/j.cmet.2022.07.010
118. Zhao X, Yang L, Chang N, Hou L, Zhou X, Yang L, et al. Neutrophils undergo switch of apoptosis to NETosis during murine fatty liver injury via S1P receptor 2 signaling. Cell Death Dis (2020) 11:379. doi: 10.1038/s41419-020-2582-1
119. Wang H, Zhang H, Wang Y, Brown ZJ, Xia Y, Huang Z, et al. Regulatory T-cell and neutrophil extracellular trap interaction contributes to carcinogenesis in non-alcoholic steatohepatitis. J Hepatol (2021) 75:1271–83. doi: 10.1016/j.jhep.2021.07.032
120. Xin H, Lai Q, Zhou Y, He J, Song Y, Liao M, et al. Noninvasive evaluation of neutrophil extracellular traps signature predicts clinical outcomes and immunotherapy response in hepatocellular carcinoma. Front Immunol (2023) 14:1134521. doi: 10.3389/fimmu.2023.1134521
121. Zenlander R, Havervall S, Magnusson M, Engstrand J, Ågren A, Thålin C, et al. Neutrophil extracellular traps in patients with liver cirrhosis and hepatocellular carcinoma. Sci Rep (2021) 11:18025. doi: 10.1038/s41598-021-97233-3
122. Velliou RI, Mitroulis I, Chatzigeorgiou A. Neutrophil extracellular traps contribute to the development of hepatocellular carcinoma in NASH by promoting Treg differentiation. Hepatobil Surg Nutr (2022) 11:415–8. doi: 10.21037/hbsn-21-557
123. Liu Y, Lu T, Zhang C, Xu J, Xue Z, Busuttil RW, et al. Activation of YAP attenuates hepatic damage and fibrosis in liver ischemia-reperfusion injury. J Hepatol (2019) 71:719–30. doi: 10.1016/j.jhep.2019.05.029
124. Oliveira THC, Marques PE, Proost P, Teixeira MMM. Neutrophils: a cornerstone of liver ischemia and reperfusion injury. Lab Invest (2018) 98:51–62. doi: 10.1038/labinvest.2017.90
125. Evrard M, Kwok IWH, Chong SZ, Teng KWW, Becht E, Chen J, et al. Developmental analysis of bone marrow neutrophils reveals populations specialized in expansion, trafficking, and effector functions. Immunity (2018) 48:364–379.e368. doi: 10.1016/j.immuni.2018.02.002
126. Imaeda AB, Watanabe A, Sohail MA, Mahmood S, Mohamadnejad M, Sutterwala FS, et al. Acetaminophen-induced hepatotoxicity in mice is dependent on Tlr9 and the Nalp3 inflammasome. J Clin Invest (2009) 119:305–14. doi: 10.1172/jci35958
127. Huang H, Tohme S, Al-Khafaji AB, Tai S, Loughran P, Chen L, et al. Damage-associated molecular pattern-activated neutrophil extracellular trap exacerbates sterile inflammatory liver injury. Hepatology (2015) 62:600–14. doi: 10.1002/hep.27841
128. Khandpur R, Carmona-Rivera C, Vivekanandan-Giri A, Gizinski A, Yalavarthi S, Knight JS, et al. NETs are a source of citrullinated autoantigens and stimulate inflammatory responses in rheumatoid arthritis. Sci Transl Med (2013) 5:178ra140. doi: 10.1126/scitranslmed.3005580
129. Guo J, Akahoshi T, Mizuta Y, Murata M, Narahara S, Kawano T, et al. Histidine-rich glycoprotein alleviates liver ischemia/reperfusion injury in mice with nonalcoholic steatohepatitis. Liver Transpl (2021) 27:840–53. doi: 10.1002/lt.25960
130. Arumugam S, Girish Subbiah K, Kemparaju K, Thirunavukkarasu C. Neutrophil extracellular traps in acrolein promoted hepatic ischemia reperfusion injury: Therapeutic potential of NOX2 and p38MAPK inhibitors. J Cell Physiol (2018) 233:3244–61. doi: 10.1002/jcp.26167
131. Liu Y, Qin X, Lei Z, Chai H, Huang Z, Wu Z. Tetramethylpyrazine inhibits neutrophil extracellular traps formation and alleviates hepatic ischemia/reperfusion injury in rat liver transplantation. Exp Cell Res (2021) 406:112719. doi: 10.1016/j.yexcr.2021.112719
132. Cools-Lartigue J, Spicer J, McDonald B, Gowing S, Chow S, Giannias B. Neutrophil extracellular traps sequester circulating tumor cells and promote metastasis. J Clin Invest (2013) 123:3446–58. doi: 10.1172/jci67484
133. Jiang ZZ, Peng ZP, Liu XC, Guo HF, Zhou MM, Jiang D, et al. Neutrophil extracellular traps induce tumor metastasis through dual effects on cancer and endothelial cells. Oncoimmunology (2022) 11:2052418. doi: 10.1080/2162402x.2022.2052418
134. Guan X, Lu Y, Zhu H, Yu S, Zhao W, Chi X, et al. The crosstalk between cancer cells and neutrophils enhances hepatocellular carcinoma metastasis via neutrophil extracellular traps-associated cathepsin G component: A potential therapeutic target. J Hepatocell Carcinoma (2021) 8:451–65. doi: 10.2147/jhc.S303588
135. Stojkov D, Amini P, Oberson K, Sokollik C, Duppenthaler A, Simon HU, et al. ROS and glutathionylation balance cytoskeletal dynamics in neutrophil extracellular trap formation. J Cell Biol (2017) 216:4073–90. doi: 10.1083/jcb.201611168
136. Castier Y, Brandes RP, Leseche G, Tedgui A, Lehoux S. p47phox-dependent NADPH oxidase regulates flow-induced vascular remodeling. Circ Res (2005) 97:533–40. doi: 10.1161/01.Res.0000181759.63239.21
137. Zhou Z, Xu MJ, Cai Y, Wang W, Jiang JX, Varga ZV, et al. Neutrophil-hepatic stellate cell interactions promote fibrosis in experimental steatohepatitis. Cell Mol Gastroenterol Hepatol (2018) 5:399–413. doi: 10.1016/j.jcmgh.2018.01.003
138. Hwang S, He Y, Xiang X, Seo W, Kim SJ, Ma J, et al. Interleukin-22 ameliorates neutrophil-driven nonalcoholic steatohepatitis through multiple targets. Hepatology (2020) 72:412–29. doi: 10.1002/hep.31031
139. Yang LY, Shen XT, Sun HT, Zhu WW, Zhang JB, Lu L, et al. Neutrophil extracellular traps in hepatocellular carcinoma are enriched in oxidized mitochondrial DNA which is highly pro-inflammatory and pro-metastatic. J Cancer (2022) 13:1261–71. doi: 10.7150/jca.64170
140. Szczerba BM, Castro-Giner F, Vetter M, Krol I, Gkountela S, Landin J, et al. Neutrophils escort circulating tumour cells to enable cell cycle progression. Nature (2019) 566:553–7. doi: 10.1038/s41586-019-0915-y
141. Wang H, Zhang Y, Wang Q, Wei X, Wang H, Gu K. The regulatory mechanism of neutrophil extracellular traps in cancer biological behavior. Cell Biosci (2021) 11:193. doi: 10.1186/s13578-021-00708-z
142. Albrengues J, Shields MA, Ng D, Park CG, Ambrico A, Poindexter ME, et al. Neutrophil extracellular traps produced during inflammation awaken dormant cancer cells in mice. Science (2018) 361. doi: 10.1126/science.aao4227
143. Kaltenmeier CT, Yazdani H, van der Windt D, Molinari M, Geller D, Tsung A, et al. Neutrophil extracellular traps as a novel biomarker to predict recurrence-free and overall survival in patients with primary hepatic Malignancies. HPB (Oxford) (2021) 23:309–20. doi: 10.1016/j.hpb.2020.06.012
144. Teijeira Á, Garasa S, Gato M, Alfaro C, Migueliz I, Cirella A, et al. CXCR1 and CXCR2 chemokine receptor agonists produced by tumors induce neutrophil extracellular traps that interfere with immune cytotoxicity. Immunity (2020) 52:856–871.e858. doi: 10.1016/j.immuni.2020.03.001
145. Cheng Y, Gong Y, Chen X, Zhang Q, Zhang X, He Y, et al. Injectable adhesive hemostatic gel with tumor acidity neutralizer and neutrophil extracellular traps lyase for enhancing adoptive NK cell therapy prevents post-resection recurrence of hepatocellular carcinoma. Biomaterials (2022) 284:121506. doi: 10.1016/j.biomaterials.2022.121506
146. Schoeps B, Eckfeld C, Prokopchuk O, Böttcher J, Häußler D, Steiger K, et al. TIMP1 triggers neutrophil extracellular trap formation in pancreatic cancer. Cancer Res (2021) 81:3568–79. doi: 10.1158/0008-5472.Can-20-4125
147. Komorowicz E, Balázs N, Tanka-Salamon A, Varga Z, Szabó L, Bóta A, et al. Biorelevant polyanions stabilize fibrin against mechanical and proteolytic decomposition: Effects of polymer size and electric charge. J Mech Behav BioMed Mater (2020) 102:103459. doi: 10.1016/j.jmbbm.2019.103459
148. Manfredi AA, Rovere-Querini P, D’Angelo A, Maugeri N. Low molecular weight heparins prevent the induction of autophagy of activated neutrophils and the formation of neutrophil extracellular traps. Pharmacol Res (2017) 123:146–56. doi: 10.1016/j.phrs.2016.08.008
149. Lu Z, Long Y, Li J, Li J, Ren K, Zhao W, et al. Simultaneous inhibition of breast cancer and its liver and lung metastasis by blocking inflammatory feed-forward loops. J Control Release (2021) 338:662–79. doi: 10.1016/j.jconrel.2021.08.047
150. Clark SR, Ma AC, Tavener SA, McDonald B, Goodarzi Z, Kelly MM, et al. Platelet TLR4 activates neutrophil extracellular traps to ensnare bacteria in septic blood. Nat Med (2007) 13:463–9. doi: 10.1038/nm1565
151. McDonald B, Urrutia R, Yipp BG, Jenne CN, Kubes P. Intravascular neutrophil extracellular traps capture bacteria from the bloodstream during sepsis. Cell Host Microbe (2012) 12:324–33. doi: 10.1016/j.chom.2012.06.011
152. McDonald B, Urrutia R, Yipp BG, Jenne CN, Kubes P. Platelets and neutrophil extracellular traps collaborate to promote intravascular coagulation during sepsis in mice. Blood (2017) 129:1357–67. doi: 10.1182/blood-2016-09-741298
153. Zhang H, Goswami J, Varley P, van der Windt DJ, Ren J, Loughran P, et al. Hepatic surgical stress promotes systemic immunothrombosis that results in distant organ injury. Front Immunol (2020) 11:987. doi: 10.3389/fimmu.2020.00987
154. Mangold A, Alias S, Scherz T, Hofbauer M, Jakowitsch J, Panzenböck A, et al. Coronary neutrophil extracellular trap burden and deoxyribonuclease activity in ST-elevation acute coronary syndrome are predictors of ST-segment resolution and infarct size. Circ Res (2015) 116:1182–92. doi: 10.1161/circresaha.116.304944
155. Ducroux C, Di Meglio L, Loyau S, Delbosc S, Boisseau W, Deschildre C, et al. Thrombus neutrophil extracellular traps content impair tPA-induced thrombolysis in acute ischemic stroke. Stroke (2018) 49:754–7. doi: 10.1161/strokeaha.117.019896
156. Rosell A, Gautam G, Wannberg F, Ng H, Gry H, Vingbäck E, et al. Neutrophil extracellular trap formation is an independent risk factor for occult cancer in patients presenting with venous thromboembolism. J Thromb Haemost (2023) 21(11):3166–3174. doi: 10.1016/j.jtha.2023.07.007
157. Rosell A, Aguilera K, Hisada Y, Schmedes C, Mackman N, Wallen H, et al. Prognostic value of circulating markers of neutrophil activation, neutrophil extracellular traps, coagulation and fibrinolysis in patients with terminal cancer. Sci Rep (2021) 11:5074. doi: 10.1038/s41598-021-84476-3
158. Guimarães-Costa AB, Aguilera K, Hisada Y, Schmedes C, Mackman N, Wallén H, et al. Leishmania amazonensis promastigotes induce and are killed by neutrophil extracellular traps. Proc Natl Acad Sci U.S.A. (2009) 106:6748–53. doi: 10.1073/pnas.0900226106
159. Martinod K, Fuchs TA, Zitomersky NL, Wong SL, Demers M, Gallant M, et al. PAD4-deficiency does not affect bacteremia in polymicrobial sepsis and ameliorates endotoxemic shock. Blood (2015) 125:1948–56. doi: 10.1182/blood-2014-07-587709
160. Luo Y, Arita K, Bhatia M, Knuckley B, Lee YH, Stallcup MR, et al. Inhibitors and inactivators of protein arginine deiminase 4: functional and structural characterization. Biochemistry (2006) 45:11727–36. doi: 10.1021/bi061180d
161. Lewis HD, Liddle J, Coote JE, Atkinson SJ, Barker MD, Bax BD, et al. Inhibition of PAD4 activity is sufficient to disrupt mouse and human NET formation. Nat Chem Biol (2015) 11:189–91. doi: 10.1038/nchembio.1735
162. Li M, Lin C, Deng H, Strnad J, Bernabei L, Vogl DT, et al. A novel peptidylarginine deiminase 4 (PAD4) inhibitor BMS-P5 blocks formation of neutrophil extracellular traps and delays progression of multiple myeloma. Mol Cancer Ther (2020) 19:1530–8. doi: 10.1158/1535-7163.Mct-19-1020
163. Rayes RF, Mouhanna JG, Nicolau I, Bourdeau F, Giannias B, Rousseau S, et al. Primary tumors induce neutrophil extracellular traps with targetable metastasis promoting effects. JCI Insight (2019) 5. doi: 10.1172/jci.insight.128008
164. Chirivi RGS, van Rosmalen JWG, van der Linden M, Euler M, Schmets G, Bogatkevich G, et al. Therapeutic ACPA inhibits NET formation: a potential therapy for neutrophil-mediated inflammatory diseases. Cell Mol Immunol (2021) 18:1528–44. doi: 10.1038/s41423-020-0381-3
165. Li S, Ma Y, Ye S, Hu D, Xiao F. ERK/p38/ROS burst responses to environmentally relevant concentrations of diphenyl phosphate-evoked neutrophil extracellular traps formation: Assessing the role of autophagy. J Hazard Mater (2022) 421:126758. doi: 10.1016/j.jhazmat.2021.126758
166. Mousset A, Lecorgne E, Bourget I, Lopez P, Jenovai K, Cherfils-Vicini J, et al. Neutrophil extracellular traps formed during chemotherapy confer treatment resistance via TGF-β activation. Cancer Cell (2023) 41:757–775.e710. doi: 10.1016/j.ccell.2023.03.008
167. O’Neil LJ, Oliveira CB, Wang X, Navarrete M, Barrera-Vargas A, Merayo-Chalico J, et al. Neutrophil extracellular trap-associated carbamylation and histones trigger osteoclast formation in rheumatoid arthritis. Ann Rheum Dis (2023) 82:630–8. doi: 10.1136/ard-2022-223568
168. Zhang Z, Ding S, Wang Z, Zhu X, Zhou Z, Zhang W, et al. Prmt1 upregulated by Hdc deficiency aggravates acute myocardial infarction via NETosis. Acta Pharm Sin B (2022) 12:1840–55. doi: 10.1016/j.apsb.2021.10.016
169. Josefs T, Barrett TJ, Brown EJ, Quezada A, Wu X, Voisin M, et al. Neutrophil extracellular traps promote macrophage inflammation and impair atherosclerosis resolution in diabetic mice. JCI Insight (2020) 5. doi: 10.1172/jci.insight.134796
170. Frangou E, Chrysanthopoulou A, Mitsios A, Kambas K, Arelaki S, Angelidou I, et al. REDD1/autophagy pathway promotes thromboinflammation and fibrosis in human systemic lupus erythematosus (SLE) through NETs decorated with tissue factor (TF) and interleukin-17A (IL-17A). Ann Rheum Dis (2019) 78:238–48. doi: 10.1136/annrheumdis-2018-213181
171. Berger-Achituv S, Brinkmann V, Abed UA, Kühn LI, Ben-Ezra J, Elhasid R, et al. A proposed role for neutrophil extracellular traps in cancer immunoediting. Front Immunol (2013) 4:48. doi: 10.3389/fimmu.2013.00048
172. Park J, Wysocki RW, Amoozgar Z, Maiorino L, Fein MR, Jorns J, et al. Cancer cells induce metastasis-supporting neutrophil extracellular DNA traps. Sci Transl Med (2016) 8:361ra138. doi: 10.1126/scitranslmed.aag1711
173. Guglietta S, Chiavelli A, Zagato E, Krieg C, Gandini S, Ravenda PS, et al. Coagulation induced by C3aR-dependent NETosis drives protumorigenic neutrophils during small intestinal tumorigenesis. Nat Commun (2016) 7:11037. doi: 10.1038/ncomms11037
174. Miller-Ocuin JL, Liang X, Boone BA, Doerfler WR, Singhi AD, Tang D, et al. DNA released from neutrophil extracellular traps (NETs) activates pancreatic stellate cells and enhances pancreatic tumor growth. Oncoimmunology (2019) 8:e1605822. doi: 10.1080/2162402x.2019.1605822
175. Demers M, Wong SL, Martinod K, Gallant M, Cabral JE, Wang Y, et al. Priming of neutrophils toward NETosis promotes tumor growth. Oncoimmunology (2016) 5:e1134073. doi: 10.1080/2162402x.2015.1134073
176. Schedel F, Mayer-Hain S, Pappelbaum KI, Metze D, Stock M, Goerge T, et al. Evidence and impact of neutrophil extracellular traps in Malignant melanoma. Pigment Cell Melanoma Res (2020) 33:63–73. doi: 10.1111/pcmr.12818
177. Nie M, Yang L, Bi X, Wang Y, Sun P, Yang H, et al. Neutrophil extracellular traps induced by IL8 promote diffuse large B-cell lymphoma progression via the TLR9 signaling. Clin Cancer Res (2019) 25:1867–79. doi: 10.1158/1078-0432.Ccr-18-1226
178. Malladi S, Macalinao DG, Jin X, He L, Basnet H, Zou Y, et al. Metastatic latency and immune evasion through autocrine inhibition of WNT. Cell (2016) 165:45–60. doi: 10.1016/j.cell.2016.02.025
179. Barkan D, El Touny LH, Michalowski AM, Smith JA, Chu I, Davis AS, et al. Metastatic growth from dormant cells induced by a col-I-enriched fibrotic environment. Cancer Res (2010) 70:5706–16. doi: 10.1158/0008-5472.Can-09-2356
180. Pierce BL, Ballard-Barbash R, Bernstein L, Baumgartner RN, Neuhouser ML, Wener MH, et al. Elevated biomarkers of inflammation are associated with reduced survival among breast cancer patients. J Clin Oncol (2009) 27:3437–44. doi: 10.1200/jco.2008.18.9068
181. De Cock JM, Shibue T, Dongre A, Keckesova Z, Reinhardt F, Weinberg RA. Inflammation triggers zeb1-dependent escape from tumor latency. Cancer Res (2016) 76:6778–84. doi: 10.1158/0008-5472.Can-16-0608
182. Romero I, Garrido F, Garcia-Lora AM. Metastases in immune-mediated dormancy: a new opportunity for targeting cancer. Cancer Res (2014) 74:6750–7. doi: 10.1158/0008-5472.Can-14-2406
183. Pommier A, Anaparthy N, Memos N, Kelley ZL, Gouronnec A, Yan R, et al. Unresolved endoplasmic reticulum stress engenders immune-resistant, latent pancreatic cancer metastases. Science (2018) 360. doi: 10.1126/science.aao4908
184. Nakano K, Suzuki S, Oh C. Significance of increased secretion of glucocorticoids in mice and rats injected with bacterial endotoxin. Brain Behav Immun (1987) 1:159–72. doi: 10.1016/0889-1591(87)90018-3
185. Sagiv JY, Michaeli J, Assi S, Mishalian I, Kisos H, Levy L, et al. Phenotypic diversity and plasticity in circulating neutrophil subpopulations in cancer. Cell Rep (2015) 10:562–73. doi: 10.1016/j.celrep.2014.12.039
186. Hua X, Hu G, Hu Q, Chang Y, Hu Y, Gao L, et al. Single-cell RNA sequencing to dissect the immunological network of autoimmune myocarditis. Circulation (2020) 142:384–400. doi: 10.1161/circulationaha.119.043545
187. Huerga Encabo H, Aramburu IV, Garcia-Albornoz M, Piganeau M, Wood H, Song A, et al. Loss of TET2 in human hematopoietic stem cells alters the development and function of neutrophils. Cell Stem Cell (2023) 30:781–799.e789. doi: 10.1016/j.stem.2023.05.004
188. Gungabeesoon J, Gort-Freitas NA, Kiss M, Bolli E, Messemaker M, Siwicki M, et al. A neutrophil response linked to tumor control in immunotherapy. Cell (2023) 186:1448–1464.e1420. doi: 10.1016/j.cell.2023.02.032
189. Kalafati L, Kourtzelis I, Schulte-Schrepping J, Li X, Hatzioannou A, Grinenko T, et al. Innate immune training of granulopoiesis promotes anti-tumor activity. Cell (2020) 183:771–785.e712. doi: 10.1016/j.cell.2020.09.058
190. Chung JY, Tang PC, Chan MK, Xue VW, Huang XR, Ng CS, et al. Smad3 is essential for polarization of tumor-associated neutrophils in non-small cell lung carcinoma. Nat Commun (2023) 14:1794. doi: 10.1038/s41467-023-37515-8
191. Tyagi A, Sharma S, Wu K, Wu SY, Xing F, Liu Y, et al. Nicotine promotes breast cancer metastasis by stimulating N2 neutrophils and generating pre-metastatic niche in lung. Nat Commun (2021) 12:474. doi: 10.1038/s41467-020-20733-9
192. Zhang X, Shi H, Yuan X, Jiang P, Qian H, Xu W. Tumor-derived exosomes induce N2 polarization of neutrophils to promote gastric cancer cell migration. Mol Cancer (2018) 17:146. doi: 10.1186/s12943-018-0898-6
193. Ehrens A, Rüdiger N, Heepmann L, Linnemann L, Hartmann W, Hübner MP, et al. Eosinophils and Neutrophils Eliminate Migrating Strongyloides ratti Larvae at the Site of Infection in the Context of Extracellular DNA Trap Formation. Front Immunol (2021) 12:715766. doi: 10.3389/fimmu.2021.715766
194. Yousefi S, Morshed M, Amini P, Stojkov D, Simon D, von Gunten S, et al. Basophils exhibit antibacterial activity through extracellular trap formation. Allergy (2015) 70:1184–8. doi: 10.1111/all.12662
195. Schorn C, Janko C, Latzko M, Chaurio R, Schett G, Herrmann M. Monosodium urate crystals induce extracellular DNA traps in neutrophils, eosinophils, and basophils but not in mononuclear cells. Front Immunol (2012) 3:277. doi: 10.3389/fimmu.2012.00277
196. Dworski R, Simon HU, Hoskins A, Yousefi S. Eosinophil and neutrophil extracellular DNA traps in human allergic asthmatic airways. J Allergy Clin Immunol (2011) 127:1260–6. doi: 10.1016/j.jaci.2010.12.1103
197. Ehrens A, Lenz B, Neumann AL, Giarrizzo S, Reichwald JJ, Frohberger SJ, et al. Microfilariae trigger eosinophil extracellular DNA traps in a dectin-1-dependent manner. Cell Rep (2021) 34:108621. doi: 10.1016/j.celrep.2020.108621
198. Ueki S, Konno Y, Takeda M, Moritoki Y, Hirokawa M, Matsuwaki Y, et al. Eosinophil extracellular trap cell death-derived DNA traps: Their presence in secretions and functional attributes. J Allergy Clin Immunol (2016) 137:258–67. doi: 10.1016/j.jaci.2015.04.041
199. Lopes JP, Stylianou M, Nilsson G, Urban CF. Opportunistic pathogen Candida albicans elicits a temporal response in primary human mast cells. Sci Rep (2015) 5:12287. doi: 10.1038/srep12287
200. von Köckritz-Blickwede M, Goldmann O, Thulin P, Heinemann K, Norrby-Teglund A, Rohde M, et al. Phagocytosis-independent antimicrobial activity of mast cells by means of extracellular trap formation. Blood (2008) 111:3070–80. doi: 10.1182/blood-2007-07-104018
Keywords: hepatocellular carcinoma (HCC), neutrophil extracellular trap (NET) formation, tumorigenesis and progression, liver transplantation, liver ischemia-reperfusion injury (liver I/R injury)
Citation: Zhu W, Fan C, Dong S, Li X, Chen H and Zhou W (2023) Neutrophil extracellular traps regulating tumorimmunity in hepatocellular carcinoma. Front. Immunol. 14:1253964. doi: 10.3389/fimmu.2023.1253964
Received: 06 July 2023; Accepted: 23 November 2023;
Published: 18 December 2023.
Edited by:
Virginie Lafont, Institut National de la Santé et de la Recherche Médicale (INSERM), FranceReviewed by:
Julien Faget, INSERM U1194 Institut de Recherche en, Cancérologie de Montpellier (IRCM), FranceXue-Yan He, Washington University in St. Louis, United States
Steven Edwards, University of Liverpool, United Kingdom
Copyright © 2023 Zhu, Fan, Dong, Li, Chen and Zhou. This is an open-access article distributed under the terms of the Creative Commons Attribution License (CC BY). The use, distribution or reproduction in other forums is permitted, provided the original author(s) and the copyright owner(s) are credited and that the original publication in this journal is cited, in accordance with accepted academic practice. No use, distribution or reproduction is permitted which does not comply with these terms.
*Correspondence: Wence Zhou, emhvdXdjMTI5QDE2My5jb20=
†These authors have contributed equally to this work