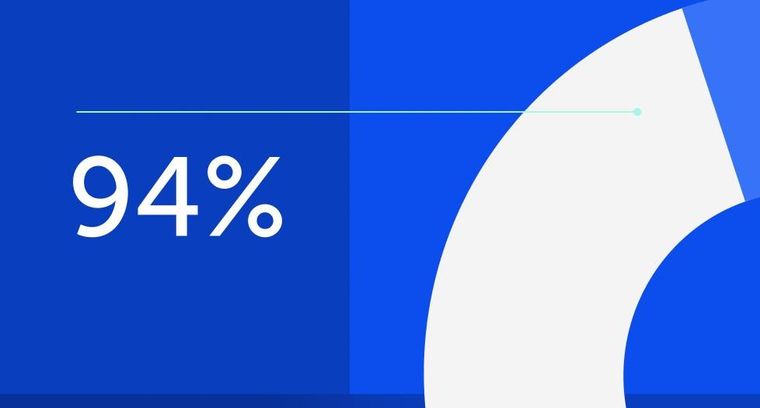
94% of researchers rate our articles as excellent or good
Learn more about the work of our research integrity team to safeguard the quality of each article we publish.
Find out more
REVIEW article
Front. Immunol., 14 August 2023
Sec. Cancer Immunity and Immunotherapy
Volume 14 - 2023 | https://doi.org/10.3389/fimmu.2023.1253064
This article is part of the Research TopicEpigenetic, Metabolic, and Transcriptional Regulation of Immune Cell Plasticity and Functions in Cancer and Non-Cancer DiseasesView all 11 articles
As critical executors regulating many cellular operations, proteins determine whether living activities can be performed in an orderly and efficient manner. Precursor proteins are inert and must be modified posttranslationally to enable a wide range of protein types and functions. Protein posttranslational modifications (PTMs) are well recognized as being directly associated with carcinogenesis and immune modulation and have emerged as important targets for cancer detection and treatment. Lactylation (Kla), a novel PTM associated with cellular metabolism found in a wide range of cells, interacts with both histone and nonhistone proteins. Unlike other epigenetic changes, Kla has been linked to poor tumor prognosis in all current studies. Histone Kla can affect gene expression in tumors and immunological cells, thereby promoting malignancy and immunosuppression. Nonhistone proteins can also regulate tumor progression and treatment resistance through Kla. In this review, we aimed to summarize the role of Kla in the onset and progression of cancers, metabolic reprogramming, immunosuppression, and intestinal flora regulation to identify new molecular targets for cancer therapy and provide a new direction for combined targeted therapy and immunotherapy.
Metabolic reprogramming is a vital characteristic of tumor cells and plays an important role in tumor therapy, with the Warburg effect being the most widely studied. Even when sufficient oxygen is available to support mitochondrial oxidative phosphorylation, tumor cells tend to convert glucose into lactate (1). Lactate was formerly considered to be a metabolic waste; however, recent research has shown that it can promote the formation and development of cancers through activating the Gi protein-coupled receptor 81 (GPR81) signaling pathway (2), influencing cell metabolism (3), and modulating the tumor microenvironment (TME) (4).
Protein posttranslational modifications (PTMs) refer to the process of changing the biochemical properties of proteins through adding chemical groups to one or more amino acid residues so that precursor proteins can have specific functions. Common modifications include acetylation, ubiquitination, methylation, phosphorylation, and glycosylation (5). PTMs are closely related to carcinogenesis, and their pathological significance involves all cancer characteristics, including maintenance of proliferation signals, resistance to cell death, induction of angiogenesis, and activation of invasion (6). PTMs also play vital roles in TME regulation. Swamy et al. (7) found that activated effector T cells contain more O-GlcNAcylation-modified proteins than naïve T cells, suggesting that O-GlcNAcylation plays an important role in T cell activation. Forkhead/winged helix transcriptional factor P3 (FoxP3) is a transcriptional regulator that plays an important role in regulatory T cell (Treg) growth. Acetylation can enhance its ability to bind to chromatin, thereby increasing the number and activity of Treg cells and inhibiting the antitumor effects of the immune system (8). Therefore, targeting PTMs to key proteins or pathways is an emerging strategy for improving early cancer detection and treatment.
Lactylation (Kla) is a novel PTM. Zhao et al. (9) conducted the first study on histone Kla in 2019, demonstrating that Kla refers to the addition of a lactyl (La) group to a lysine residue in the histone tail. Later studies established that Kla is prevalent in immune-related cells (10), non-small cell lung cancer (NSCLC) (11), and ocular melanoma (12), and that it is strongly associated with the development of malignancies (13). This systematic review aimed to examine the important role of Kla in tumor cell metabolism, microenvironment, and immunosuppression, explore the possibility of targeting Kla sites and catalytic enzymes for cancer therapy, and provide a new direction for combined targeted cancer therapy and immunotherapy.
Glucose primarily generates energy via two metabolic pathways: glycolysis and oxidative phosphorylation (Figure 1). Both pathways begin with the conversion of glucose to pyruvate, followed by the production of adenosine triphosphate (ATP) and nicotinamide adenine dinucleotide (NADH). When oxygen is available, the pyruvate produced during glycolysis is carried into the mitochondria and transformed into acetyl-CoA, which subsequently enters the tricarboxylic acid (TCA) cycle and generates considerable energy (14, 15). However, in the absence of oxygen, pyruvate is converted to lactate by lactate dehydrogenase (LDH) (16). Under aerobic conditions, the glycolytic process in normal mammalian cells is blocked, which is known as the Pasteur effect (17). However, the glycolytic metabolism of tumor cells is significantly active even in the presence of an ample oxygen supply, which is known as the Warburg effect (1).
Figure 1 The metabolic process of lactate. Lactate can be created intracellularly by glycolysis catalyzed by LDH, or it can be taken up from the outside via MCT1. Intracellular lactate inhibits glycolytic enzymes while promoting the tricarboxylic acid cycle, generating a negative feedback loop. Lactate can also be converted to lactoyl-CoA, which is involved in the lactylation of histone and non-histone proteins. HK-1, hemokinin-1; G6PD, glucose 6-phosphate dehydrogenase; PKM, glycolytic enzyme pyruvate kinase M; MCT1, monocarboxylate transporters 1; LDH, lactate dehydrogenase; PDH, Pyruvate dehydrogenase; SDH, succinate dehydrogenase; IDH, isocitrate dehydrogenase; TCA, Tricarboxylic acid; HDAC, histone deacetylase; Kla, lactylation.
The causes of the Warburg effect remain contentious, and several theories provide preliminary explanations. One possible explanation is that, under oxygen-sufficient conditions, the efficiency of ATP production is not a limiting factor for cell multiplication. When the capability of glucose metabolism to produce ATP is impaired in normally proliferating cells, they undergo cell cycle arrest and reactivate catabolism while activating signaling pathways, such as AMP-activated protein kinase (AMPK) and activating protein kinase B (AKT), to maintain energy homeostasis (18, 19). Furthermore, cancer cells constantly divide and require additional metabolic intermediates to form macromolecules for their daughter cells. However, mitochondrial oxidative phosphorylation converts all glucose into CO2 to maximize ATP generation, in conflict with the requirements of growing cells. During glycolysis, glucose can be transformed into additional macromolecular precursors, such as acetyl-CoA for fatty acid synthesis, glycolytic intermediates for nonessential amino acid synthesis, and ribose for nucleotide synthesis, to enhance cell proliferation (9). In addition, it has been shown that adverse metabolic conditions in TME can lead to the activation of transcription factors such as Krüppel-like factor 4 (KLF4) and nuclear factor-kappa B (NF-κB), leading to the selection of the Warburg phenotype through transcriptional reprogramming (20).
Previously, the lactate produced through glycolysis was considered to be merely metabolic waste. However, in recent years, an increasing number of studies have shown that lactate plays a role in tumor growth. Lactate can be employed as a GPR81 ligand to activate the GPR81 signaling pathway, which can then influence the expression of metabolism-related genes and promote tumor growth (2). In addition, Vegran et al. (21) found that lactate can enter endothelial cells through monocarboxylate transporter 1 (MCT1), causing degradation and phosphorylation of IκBα, and then stimulating the autocrine NF-κB/interleukin (IL)-8 pathway to cause cell migration and blood vessel formation. Lactate is also closely related to TME (22). Proton-coupled lactate transport in cancer or stromal cells creates an acidic environment with a pH ranging from 6–6.6, leading to tumor development and treatment resistance (23). Unlike exogenous pathogens, cancer cells are derived from normal cells and express almost all the proteins that normal cells express, so they are more difficult to distinguish by the immune system. In lymphocytes, B cells can act as antigen-presenting cells and secrete cytokines to exert anti-tumor effects (24); Cytotoxic T lymphocytes (CTLs) can specifically recognize tumor-associated antigens through major histocompatibility complex (MHC) I on their surface, bind to tumor cells and produce perforin and other cytotoxins to kill cancer cells (25); Natural killer (NK) cells have no MHC or human leukocyte antigen restriction and can release perforin, granzyme and cytokines to destroy tumor cells (26). Among myeloid cells, the frequency of dendritic cells in tumors was associated with a good prognosis, while tumor-associated macrophages (TAM), especially M2 macrophages, were associated with a poor prognosis (27). It has been reported that lactate generated from tumor cells can activate the production of vascular endothelial growth factor (VEGF) and arginase 1 (Arg1), promoting TAM polarization to M2 and assisting TAM in promoting tumor growth (28). Besides, the lactate concentration in the TME can limit the lactate efflux of CTLs, lowering the production of cytokines, perforin, and granzyme B, and inhibiting CTL cytotoxicity (29). NK cells are potent innate immune effectors that serve as the first line of defense against cancer. Lactate induces NK cell apoptosis (30). Natural killer T (NKT) cells are another type of immune cells with anti-tumor activity. Lactate can inhibit the production of interferon-γ (IFNγ) and IL-4 by NKT cells, inhibit their survival and proliferation, and promote tumor development (31).
However, the specific mechanisms underlying lactate uptake and utilization by tumor cells have not yet been fully elucidated. The accumulation of lactate in the TME can be reduced through increasing its uptake and utilization by tumor cells, thus reducing its influence on tumor cells and immune cells, or through finding effective targets to cause tumors to undergo aerobic metabolism instead of the Warburg effect, thereby inhibiting tumor invasion. These issues require urgent attention in the field of lactate metabolism in tumors.
Kla is a PTM protein first reported in 2019 (32). Subsequent studies have further confirmed that Kla has an important function in relation to lactate and is involved in important activities such as tumor proliferation (33), nervous system regulation (34), and metabolic regulation (35).
Lysine is a basic amino acid and its sixth amino group is highly active. After modification, this amino acid changes from basic to acidic, leading to changes in protein polarity and function (36). Lysine acylation is a widespread and highly conserved PTM that affects various physiological and pathological processes through epigenetic regulation. Using mass spectrometry analysis, Zhao et al. (32) found a mass shift of 72.021 Da on lysine residues in three proteolytic peptides, which increased in a dose-dependent manner with an increase in lactate. Subsequently, they showed the existence of Kla through tracking isotopic sodium l-lactate (13C3). Inhibition of pyruvate dehydrogenase kinase (PDK) and LDH production by dichloroacetic acid (DCA) and oxalate was found to cause Kla inhibition (37, 38), whereas inhibition of mitochondrial oxidative phosphorylation by rotenone caused an increase in Kla. This implies that lactate levels are inextricably linked to local lactate concentrations. Furthermore, stressors such as hypoxia and bacterial infection can promote a change in cellular energy metabolism into a glycolysis-dependent pattern, resulting in increased lactate production and the activation of histone Kla (39).
Kla has also been shown to cause changes in the expression of genes related to inflammatory responses (40), macrophage polarization (32), and other processes. Several studies have shown that Kla plays an important role in disease occurrence and development (33, 34). However, research into “writers” and “erasers,” which are necessary for the occurrence and removal of Kla, remains in its infancy and has yet to be expanded.
Lactate, a chiral molecule, typically exists as three optical isomers: D-lactate, L-lactate, and racemic DL-lactate. L-Lactate is the most common type of lactate. It increases dramatically in pathological conditions such as tumors, sepsis, and autoimmune disorders (41). According to previous research, L-lactate can be activated through acyl-CoA synthetase and transported to lysine residues by histone acetyltransferases such as p300 (32). D-Lactate is primarily synthesized in cells through the metabolism of-carbonyl aldehydes with high glycation activity, such as methylglyoxal (MGO) (42). D-Lactate concentrations can increase dramatically when intestinal function is impaired (43). Galligan et al. (44) found that methylglyoxal, a byproduct of glycolysis, can combine with glutathione to form lactoylglutathione (LGSH), which is catalyzed by glyoxalase 1 (GLO1). Simultaneously, GLO2 hydrolyzes LGSH, cycling glutathione, and produces D-lactate. LGSH can be employed as a donor to supply lactate molecules to enhance the Kla of target proteins; this reaction does not require enzyme catalysis. In addition, Zhao et al. (45) found that common deacetylases HDAC1-3 and SIRT1-3 have the ability to eliminate Kla, among which HDAC3 is the most effective “eraser” for L- and D-lactylation (46) (Figure 2).
Figure 2 The regulatory process of lactylation. There are two known modification pathways for lactylation. L-lactate can form lactoyl-CoA, which mediates lactylation under the catalysis of P300. In addition, methylglyoxal can form LGSH under the catalysis of GLO1, and LGSH can also mediate the lactylation of proteins, which does not require enzyme catalysis. LGSH can be decomposed into glutathione and D-lactate under the action of GLO2. Both L-and D-lactate-mediated lactylation were abolished by HDAC3. LGSH, Lactoylglutathione; GLO, glyxoalase.
The study of Kla and its associated enzymes offers new perspectives for targeted cancer treatment and has promising practical applications. As an increasing number of histone Kla sites have been identified, the role of Kla in nonhistone proteins is becoming increasingly understood and recognized. Among glycolytic enzymes, Kla has been found to be widespread. For instance, the Kla of aldolase A (ALDOA) is present in numerous human tumor cell lines and can control glycolysis through controlling the activity of metabolic enzymes (47). Through thoroughly analyzing the tumor and nearby liver, Young et al. (48) identified 9,275 Kla sites, of which 9,256 were found on proteins other than histones, indicating that Kla is a broad alteration that extends beyond histone and transcriptional regulation. They also showed that the p53 pathway is controlled by adenylate kinase 2 (AK2) Kla in hepatocellular carcinoma (HCC), which contributes to a poor prognosis.
The metabolic reprogramming of tumor cells, which promotes rapid cell growth and proliferation through altering the metabolism, is considered to represent a new cancer characteristic (49). The most prevalent and basic type of research on tumor metabolism is aberrant glucose metabolism (50). Tumor cells have a distinctive glucose metabolism pattern compared with normal cells; even under oxygen-sufficient conditions, they prefer glycolysis to the tricarboxylic acid (TCA) cycle to obtain energy. Recent studies have also found that many other metabolic pathways, including fatty acid metabolism (51), cholesterol metabolism (52), and glutamine metabolism (53), undergo reprogramming changes in tumor cells.
Hypoxia-inducible factor alpha (HIF-1α) is a key gene that regulates the metabolic mode of tumor cells (54). Lactate concentration can inhibit the activity of proline hydroxylase (PHD) and weaken the ubiquitination and degradation of HIF-1α by PHD, which indicates that lactate is closely related to tumor metabolism (55). Previous studies have demonstrated that lactate can be used as a source of three-carbon compounds in mammals. Circulating lactate also allows uncoupling of glycolysis and TCA, allowing glucose use to be regulated according to more advanced bodily demands (56). Besides, lactate and pyruvate together can be used as a circulating REDOX buffer to balance the NADH/NAD ratio in cells and tissues (57). Chiarugi et al. (3) demonstrated that lactate can activate the key enzymes ATP citrate lyase and acetyl-CoA carboxylase in fatty acid synthesis, as well as the cholesterol pathway, by redirecting citrate in prostate cancer cells. Meanwhile, the current study showed that the accumulation of circulating lactate is negatively correlated with fat oxidation (58). In recent years, research concerning Kla has gain considerable momentum, and its role in tumor metabolism has gradually been revealed.
Wan et al. (47) found that Kla regulates numerous glycolysis-related enzymes. For example, Kla at the K147 site of fructose-bisphosphate ALDOA is found in both humans and animals. After Kla treatment, ALDOA activity was reduced, and its catalytic function was suppressed, establishing a negative feedback loop. Kla may also influence the function of other enzymes involved in glycolysis. Kla of the human recombinant enolase (ENO1) disrupts its interaction with substrates. Given the prevalence of metabolic dysfunction in the TME and high lactate levels resulting from the Warburg effect, it is plausible that Kla serves as a crucial link between lactate, tumor metabolism, and patient prognosis.
In a study concerning hepatocellular cancer, Kla was found to have primarily affected enzymes involved in metabolic pathways such as the TCA cycle, glucose, amino acid, fatty acid, and nucleotide metabolism. Kla at the K28 site then inhibited the function of AK2 and promoted the growth and spread of liver cancer cells (48). Studies concerning NSCLC have shown that increased histone Kla levels may cause downregulation of hemokinin-1 (HK-1) and pyruvate kinase M (PKM) in glycolysis, and upregulation of succinate dehydrogenase (SDHA) and isocitrate dehydrogenase 3 gamma (IDH3G) in the TCA cycle. As a result, glucose absorption and glycolysis are inhibited, mitochondrial homeostasis is maintained, and tumor development occurs (11). Furthermore, Kla has been shown to control the activity of enzymes involved in lipid metabolism in a nonalcoholic fatty liver disease model. The presence of Kla at the K673 site of fatty acid synthase inhibits its function and decreases lipid accumulation in the liver (59).
Tumor metabolic reprogramming can successfully increase tumor cell proliferation, growth, migration, and invasion, among other important biological functions (49). Therefore, targeting tumor metabolism through exploiting metabolic variations between tumor and normal cells is a promising anticancer technique. As a new PTM, Kla plays an important role in the regulation of tumor metabolism, especially glucose metabolism. However, there have been few investigations of other basic metabolic pathways such as lipid and amino acid metabolism. Further exploration of the regulatory mechanism of Kla in tumor metabolism and the search for more Kla sites could provide more reliable targets for tumor therapy.
Changes in TME are also important factors affecting carcinogenesis. Lactate is one of the most prominent metabolites in TME. Lactate produced by tumor cells via the Warburg effect can enter the extracellular microenvironment and promote angiogenesis through upregulating the expression of angiogenesis-related proteins. At the same time, it can regulate the metabolism of immune cells and inhibit the proliferation of CD8+ T cells, NK cells, and dendritic cells, thereby mediating immune escape (60, 61). The degree of histone lysine Kla has been found to be higher in TAM than in other tissues, implying that Kla plays an important regulatory role in TME, which may provide a new direction for tumor immunotherapy, antiangiogenesis therapy, and targeted therapy.
Lactate accumulation can stimulate angiogenesis by activating VEGF, transforming growth factor (TGF), IL-1, HIF-1α, and other substances (62, 63). According to previous studies, Kla plays a crucial role in this process. The high expression of hyaluronic acid (HA)-binding protein KIAA1199 has been reported to be positively correlated with tumor stage, overexpression of HIF-1α and upregulation of angiogenesis markers (64, 65). Lactate enters prostate cancer cells via MCT1 and promotes the Kla of HIF-1α, keeping it stable under normoxic circumstances and boosting KIAA1199 transcription. This discovery could lead to a promising target for antiangiogenic tumor therapy (66).
Immune cells play a significant anticancer role in TME. They can detect extracellular lactate levels and send intracellular signals to alter their function in TME (29). As a result, the impact of Kla on the efficacy of immunotherapy has attracted interest (Figure 3). Tumor-infiltrating myeloid cells (TIMs) include TAMs, myeloid-derived suppressor cells (MDSCs), and tumor-associated neutrophils (TANs). They are closely related to tumor immune escape and their functions are regulated through a variety of epigenetic processes. The increased expression of methyltransferase-like 3 (METTL3) in TIMs has been linked to poor prognosis in patients with colon cancer (67). Lactate stimulates METTL3 expression in TIMs through the H3K18 Kla. METTL3 mediates the m6A modification of Janus kinase (JAK1) mRNA, enhancing its protein translation efficiency and mediating an increase in the phosphorylation level of its downstream protein signal transducer and activator of transcription 3 (STAT3). Phosphorylated STAT3 can act as a transcription factor to regulate the production and secretion of cytokines, such as IL-6 and IL-10, resulting in immunosuppression. In addition, the presence of two Kla sites in the zinc-finger domain of METTL3, which are required for it to capture target RNA, has been confirmed. These findings highlight the importance of Kla in promoting the immunosuppressive capacity of TIMs (68).
Figure 3 Lactylation regulates the function of immune cells to mediate immunosuppression. In TAM, histone lactylation will lead to the up-regulation of M2 phenotypically related genes, thereby mediating the M2 polarization. In TIMs, histone lactylation promote the expression of METTL3, and METTL3 mediates the m6A modification of JAK1 mRNA. At the same time, there are also lactylation sites in METTL3 that allow it to bind to the target RNA. Moesin can reduce the expression of TGF-β receptor and inhibit the production of Treg cells to restore anti-tumor immunity. Lactate can inhibit the function of moesin by promoting Lys72 site lactylation to mediate the generation of Treg cells and promote the immune escape of tumor cells. In conclusion, lactylation is closely related to the immunosuppression of tumors. LPS, Lipopolysaccharide; IFN γ, Interferon-gamma; IL-4, interleukin-4; IL-13, interleukin-13; TAM, tumor-associated macrophages; TIM, tumor -infiltrating myeloid cells; Arg1, arginase 1; METTL3, methyltransferase-like 3; JAK1, Janus Kinase 1; M6A, N6-methyladenosine; TGF-β, transforming growth factor beta.
Macrophages are one of the most important innate immune cells in the human body and are classified into M1 and M2 phenotypes. Lipopolysaccharide (LPS) and IFNγ activate M1 macrophages, while IL-4 or IL-13 stimulate M2 macrophages (69). TAMs are common in the TME and associated with the formation and progression of malignancies (70). Seventy percent of TAMs exhibit the M2 phenotype, which suppresses the immune system and promotes tumor development and metastasis (71). Studies have shown that M1 macrophages undergo metabolic reprogramming during aerobic glycolysis to produce lactate during polarization. In contrast, polarization of M2 macrophages enhances aerobic phosphorylation and fatty acid metabolism (72). Zhao et al. (32) found that activation of M1 macrophages leads to the activation of glycolysis, accompanied by the production of a large amount of lactate and upregulation of H3K18la. Histone Kla can cause an increase in M2 phenotype-related proteins, indicating that it promotes the transformation of TAMs from the proinflammatory, anticancer M1 phenotype to the antiinflammatory, procancer M2 phenotype. In a study concerning prostate cancer, Patnaik et al. (73) discovered that reducing phosphatidylinositol-3 kinase (PI3K) resulted in a decrease in the synthesis of lactate in tumor cells, thereby inhibiting the histone Kla of TAM and enhancing immune efficacy. In conclusion, histone Kla is closely related to M2 polarization of TAMs, and increased levels of Kla tend to cause immunosuppression and reduce the efficacy of immunotherapy.
TGF-β is a multifunctional cytokine belonging to the transforming growth factor superfamily. It plays a crucial role in stem cell differentiation and T cell regulation (74). When activated, T cells effectively attack and kill tumor cells. However, when the immune response is complete, TGF-β delivers a signal to naïve T cells to become Treg cells to regulate and destroy activated proinflammatory T cells, ensuring that they do not create too many immune factors and cause damage to their own cells and tissues (75). In tumor tissues, Treg cells suppress immune responses and protect cancer cells from killer T cells. Moesin reduces the expression of TGF-β receptors and inhibits the production of Treg cells to restore antitumor immunity (76). Studies have found that lactate can inhibit the function of moesin by promoting Kla at the Lys72 site to mediate the generation of Treg cells and promote the immune escape of tumor cells. Therefore, it is feasible to improve the efficacy of immunotherapy by inhibiting moesin Kla (10). In summary, the discovery of Kla provides a new direction for tumor immunotherapy.
Kla provides a new perspective on the regulatory function of lactate, which plays an important role in eukaryotic cell metabolism and gene transcription (32). Lactate is a key carbon source for bacteria and is linked to stress tolerance, cell wall remodeling, and virulence (77, 78). However, if Kla occurs in prokaryotes, the biological functions it may be involved in need further investigation.
YiaC and CobB are common lysine acetyltransferases and deacetylases found in prokaryotes, respectively (79). Dong et al. found that YiaC catalyzes Kla in Escherichia coli, whereas CobB scavenges PTM. CobB enhances glycolysis and bacterial growth through eliminating the Kla of pyruvate kinase I (PykF) (80), which provides a strong basis for controlling the growth and distribution of certain bacteria through regulating specific Kla sites.
Intestinal flora refers to a group of bacteria that live in the intestinal tract of humans and animals. They play important roles in regulating digestion, immunity, and nutrient absorption. In recent years, an increasing number of investigations have shown that certain bacteria can cause malignancies in gastrointestinal tissues (Figure 4). For example, Helicobacter pylori (HP) is closely related to atypical hyperplasia, intestinal metaplasia, and gastric cancer progression (81). Moreover, Fusobacterium nucleatum is closely related to the transformation of colorectal adenomas into adenocarcinomas (82). The carcinogenic mechanism of the intestinal flora is not yet fully understood. Several studies have shown that some members of Enterobacteriaceae produce colibactin, which results in DNA damage and promotes malignancies (83). In addition, they can affect cytokine synthesis and secretion, thereby activating various carcinogenic pathways (84). Furthermore, several Toll-like receptors (TLR), such as TLR4 and TLR5, have been linked to the interaction between malignancies and microorganisms. Kong et al. (85) found that F. nucleatum promotes colorectal cancer by activating TLR4/Keap1/NRF2 signaling. Moreover, studies have shown that lactate regulates the proliferation of intestinal flora and has a carcinogenic effect (86).
Figure 4 The mechanism of intestinal flora regulating the occurrence and development of tumors. Intestinal bacteria can directly cause intracellular DNA damage by producing genotoxins, or activate the NF-κB and STAT3 pathway by promoting cytokines secretion to promote tumorigenesis. Intestinal bacteria can also activate a range of oncogenic pathways. Increased release and translocation of β-catenin into the nucleus through degradation of the E-cadherin/β-catenin complex leads to aberrant activation of WNT signaling associated with various cancers. Besides, they can lead to the invasion and metastasis of cancer by activating TLR4/Keap1/NRF2 signaling. Furthermore, LPS produced by bacteria has been shown to promote the Kla of histones, which in turn promotes tumor progression. LPS, Lipopolysaccharide; TLR4, Toll-like receptor 4; Nrf2, Nuclear factor E2-related factor 2; NF-κB, Noncanonical nuclear factor-kappaB; STAT3, ignal transducer and activator of transcription 3.
Panagiotou et al. (87) found that the excessive growth of intestinal Candida in patients with lung cancer was related to an increase in lactic-producing bacteria and a decrease in short-chain fatty acid production. Candida species use lactate as a nutrient source to compete with other fungi in the intestine. In addition, gut microbes can participate in disease pathogenesis via their interaction with the host genome through epigenetic mechanisms, such as long non-coding RNA. Liu et al. (88) quantitatively analyzed transcriptomic changes in a human colon cell line after infection with the common intestinal pathogen Salmonella typhimurium SL1344. They found that LINC00152 expression was significantly increased and associated with intestinal microbiome-derived LPS. LPS induces histone Kla at the LINC00152 promoter and decreases its binding efficiency to the transcription factor yin-yang 1 (YY1), leading to increased LINC00152 expression, thereby promoting the migration and invasion of colon cancer cells.
Bacteria promote carcinogenesis by causing DNA damage and activating oncogenic pathways. Additionally, bacteria can affect tumors through controlling the body’s immune system and metabolism (89). It has been shown that the level of Kla affects the growth and dissemination of bacteria. However, whether specific Kla sites exist in different bacteria and whether tumor progression can be controlled by modulating the Kla level in the intestinal flora needs to be further explored.
Kla is involved in a variety of processes such as tumor metabolism, angiogenesis, and immunosuppression, and is closely related to the poor prognosis of tumors (32, 47). Therefore, targeting specific sites that inhibit the occurrence of Kla may be an effective cancer treatment strategy (Table 1).
In tumor cells, glucose undergoes glycolysis to produce pyruvate, which then reacts with LDH to form lactate. Lactate can inhibit glycolysis and promote the TCA cycle by regulating key enzymes and producing a negative feedback loop (95). Studies have shown that Kla levels are positively correlated with intracellular lactate content (32). Therefore, key enzymes involved in lactate metabolism may be potential targets for tumor treatment. LDH is a key enzyme in the conversion of pyruvate to lactate during glycolysis. It maintains glycolysis and ATP production by regenerating NAD to form NADH (96). Baumann et al. (97) showed that the carcinogenicity of LDH-deficient tumor cells was significantly reduced and, after LDH knockdown, the metabolic phenotype of the cells was disturbed and their proliferation ability was significantly decreased. Furthermore, the enzyme ULK1, as an upstream regulator, could mediate the phosphorylation of LDH and enhance its enzymatic activity to promote lactate production, thereby promoting tumor progression (98). In addition to being produced within cells, lactate can be secreted between cells through MCTs to participate in the regulation of cellular physiological and pathological processes (99). Several studies have shown that targeting MCT1 or MCT4 can effectively inhibit the growth of multiple types of tumors, such as breast (100), liver (101), and bladder cancers (102).
p300 and SIRT3 are present as “writer” and “eraser” for Kla. Studies have shown that p300 is involved in tumor proliferation, migration, and invasion (103). SIRT3 can eliminate lysine Kla, and cyclin E2 (CCNE2) acts as a substrate. SIRT3 induces apoptosis in HCC cells through regulating the Kla level of CCNE2 and prevents HCC growth in vivo (91). Therefore, further exploration of the mechanism of action of Kla and identification of Kla-related catalytic enzymes may provide more targets for tumor treatment.
In addition to the enzymes related to lactate metabolism and Kla, the identification of an increasing number of Kla and their regulatory sites opens up new directions for tumor therapy. The BRAFV600E oncogene has been shown to increase the level of the intracellular protein Kla through enhancing glycolytic flux. It can promote the proliferation of anaplastic thyroid cancer through inducing H4K12 Kla, mediating driver gene transcription, and cell cycle dysregulation (93). The ninth member of the proprotein convertase family, subtilisin-kexin type 9 (PCSK9), maintains lipoprotein homeostasis. Alterations in PCSK9 expression have been linked to carcinogenesis and progression (104). The levels of lactate, protein Kla, and macrophage migration inhibitory factor (MIF) in colon cancer cells were found to have significantly increased after PCSK9 overexpression, which inhibited the polarization of M1 macrophages and promoted the occurrence and development of colon cancer, providing a potential therapeutic method for the clinical control of colon cancer (33). Pan et al. (92) identified a triterpene antitumor drug that inhibited the carcinogenesis of liver cancer stem cells through interfering with H3K9la and H3K56la Kla. Inactive von Hippel-Lindau (VHL) protein is associated with metabolic reprogramming and is important in the development of clear cell renal cell carcinoma (ccRCC) (105). VHL inactivation promotes the progression of ccRCC through triggering histone Kla to activate the transcription of platelet-derived growth factor receptor β (PDGFRβ). Meanwhile, PDGFRβ signaling in turn stimulates histone Kla, thereby forming a positive feedback loop for carcinogenesis in ccRCC. The combined reduction in histone Kla and PDGFRβ signaling was shown to substantially increase the therapeutic efficacy. This suggests that addressing the positive feedback loop between histone Kla and PDGFRβ signaling could be a viable treatment for patients with ccRCC (94). Additionally, histone Kla could stimulate the production of YT521-B homology domain family member 2 (YTHDF2), which leads to the degradation of m6A-modified period1 and TP53 and accelerates the development of ocular melanoma (12).
It has also been reported that Kla has a role in the neuroendocrine transition of adenocarcinoma (90). Neuroendocrine differentiation in adenocarcinoma is an important cause of resistance to tumor treatment (106). Most neuroendocrine cancer cells are glycolytic in nature and contain fragmented mitochondria with low membrane potential (107). Numb plays a crucial role in mitochondrial quality control by attaching to Parkin and facilitating Parkin-mediated mitophagy (90). In prostate and lung adenocarcinomas, loss of the Numb/Parkin pathway results in metabolic reprogramming marked by a significant increase in lactate generation, which in turn causes an increase in histone Kla and the activation of the transcription of genes linked to neuroendocrine function. Collectively, Numb/Parkin is a promising therapeutic target for modulating cancer cell plasticity by regulating histone Kla.
At present, some drugs targeting lactate metabolism have entered clinical trials. Lonidamine (LND), a dechlorinated derivative of indolazole-3-carboxylic acid, can destroy tumor cells by decreasing lactate production and pyruvate uptake in mitochondria and interrupting the mitochondrial transmission chain. LND does not have good anticancer activity when used alone, but it has been widely researched in combination with standard chemotherapy for the treatment of solid tumors (108). Madrid et al. (109) revealed that the combination of LND and cisplatin was more effective than cisplatin alone in reducing tumor growth in MX-1 breast cancer and A2780 ovarian cancer. Dichloroacetate (DCA) is an oral small-molecule medication that reduces tumor growth by inhibiting pyruvate dehydrogenase kinase and encouraging glucose oxidation rather than glycolysis (110). DCA has shown promising efficacy in both recurrent glioblastoma and locally advanced head and neck squamous cell carcinoma (111, 112). AZD3965, a dual MCT1 and MCT2 inhibitor, has previously been shown to be effective in the treatment of breast cancer (113). A recently completed phase 1 clinical trial validated the safety and efficacy of AZD3965 in patients with advanced solid malignancies and lymphoma (114). As a result, we anticipate that investigating Kla will provide more possibilities for tumor treatment.
Kla is crucial in immunotherapy. The therapeutic efficacy of programmed cell death protein 1 (PD-1) blocking therapy is determined by the competition for the reactivation of PD-1-expressing CD8+ T cells and Treg cells in TME (115). Nishikawa et al. (4) showed that Treg cells express more PD-1 than effector T cells in highly glycolytic malignancies. Under low-glucose conditions, Treg cells rapidly absorb lactate through MCT1, stimulating the translocation of the nuclear factor of activated T cell 1 (NFAT1) into the nucleus and consequently increasing PD-1 expression, whereas effector T cells suppress PD-1 expression. Treatment failure has been reported to occur because PD-1 inhibition stimulated PD-1-expressing Treg cells. Furthermore, studies have shown that lactate can also promote the expression of PD-L1 on macrophages and neutrophils to mediate immune resistance (116, 117). Preclinical studies have indicated that combining the MCT1 inhibitor AZD3965 with anti-PD-1 therapy lowers lactate release into the TME and boosts anti-tumor immunity (118). Lu et al. (10) found that Kla levels in the Treg cells of patients with HCC who responded to anti-PD-1 therapy were lower. Moreover, the combination of anti-PD-1 drugs and lactate dehydrogenase inhibitors has a stronger antitumor effect than anti-PD-1 drugs alone. This indicates that combining Kla inhibition with immunotherapy may be effective in treating tumors.
Kla has been found to be abundant on histone and nonhistone proteins in tumor cells, and it is linked to a poor prognosis. Therefore, exploration of Kla and its regulatory sites could help identify additional safe and effective therapeutic targets for cancer treatment and provide new directions for combination therapies.
Kla plays an important role in tumor metabolic reprogramming, angiogenesis, immune escape, and regulation of intestinal flora. It has also been linked to the occurrence and progression of malignancies. However, Kla-targeting techniques and clinical translation remain in their infancy. Numerous issues have yet to be addressed, such as, the need to investigate the synergistic or antagonistic effects of lactate and other epigenetic modifications to better understand the mechanism of tumor metabolic reprogramming, the need to investigate more nonhistone Kla sites to identify potential “writers” and “erasers,” and the need to investigate the effect of Kla on immune cells for improving the safety and effectiveness of immunotherapy. Targeted suppression of Kla coupled with standard chemotherapy, radiotherapy, and immunotherapy can be expected to provide additional cancer treatment options in the future.
Conceptualization: YX and XJ. Methodology: JS. Software: ZZ. Validation: YX, and XJ. Formal analysis: SC. Investigation: CB. Resources: XJ. Data curation: ZZ. Writing-original draft preparation: JS and HY. Writing-review and editing: JS and YX. Visualization: XJ. Supervision: XJ. Project administration: XJ. Funding acquisition: XJ. All authors contributed to the article and approved the submitted version.
This work was supported by the Jilin Provincial Science and Technology Foundation (grant number 20210402002GH and 20230508064RC), the Achievement Transformation Guiding Foundations of First Hospital of Jilin University (grant number CGZHYD202012-029).
We would like to thank Editage (www.editage.cn) for English language editing.
The authors declare that the research was conducted in the absence of any commercial or financial relationships that could be construed as a potential conflict of interest.
All claims expressed in this article are solely those of the authors and do not necessarily represent those of their affiliated organizations, or those of the publisher, the editors and the reviewers. Any product that may be evaluated in this article, or claim that may be made by its manufacturer, is not guaranteed or endorsed by the publisher.
1. Koppenol WH, Bounds PL, Dang CV. Otto warburg's contributions to current concepts of cancer metabolism. Nat Rev Cancer (2011) 11(5):325–37. doi: 10.1038/nrc3038
2. Hoque R, Farooq A, Ghani A, Gorelick F, Mehal WZ. Lactate reduces liver and pancreatic injury in toll-like receptor- and inflammasome-mediated inflammation via gpr81-mediated suppression of innate immunity. Gastroenterology (2014) 146(7):1763–74. doi: 10.1053/j.gastro.2014.03.014
3. Ippolito L, Comito G, Parri M, Iozzo M, Duatti A, Virgilio F, et al. Lactate rewires lipid metabolism and sustains a metabolic-epigenetic axis in prostate cancer. Cancer Res (2022) 82(7):1267–82. doi: 10.1158/0008-5472.Can-21-0914
4. Kumagai S, Koyama S, Itahashi K, Tanegashima T, Lin YT, Togashi Y, et al. Lactic acid promotes pd-1 expression in regulatory T cells in highly glycolytic tumor microenvironments. Cancer Cell (2022) 40(2):201–18.e9. doi: 10.1016/j.ccell.2022.01.001
5. Wang H, Yang L, Liu M, Luo J. Protein post-translational modifications in the regulation of cancer hallmarks. Cancer Gene Ther (2023) 30(4):529–47. doi: 10.1038/s41417-022-00464-3
6. Pan S, Chen R. Pathological implication of protein post-translational modifications in cancer. Mol aspects Med (2022) 86:101097. doi: 10.1016/j.mam.2022.101097
7. Zhu B, Kou C, Bai W, Yu W, Zhang L, Yu X, et al. Accelerated hyperfractionated radiotherapy versus conventional fractionation radiotherapy for head and neck cancer: A meta-analysis of randomized controlled trials. J Oncol (2019) 2019:7634746. doi: 10.1155/2019/7634746
8. van Loosdregt J, Vercoulen Y, Guichelaar T, Gent YY, Beekman JM, van Beekum O, et al. Regulation of treg functionality by acetylation-mediated foxp3 protein stabilization. Blood (2010) 115(5):965–74. doi: 10.1182/blood-2009-02-207118
9. Vander Heiden MG, Cantley LC, Thompson CB. Understanding the warburg effect: the metabolic requirements of cell proliferation. Sci (New York NY) (2009) 324(5930):1029–33. doi: 10.1126/science.1160809
10. Gu J, Zhou J, Chen Q, Xu X, Gao J, Li X, et al. Tumor metabolite lactate promotes tumorigenesis by modulating moesin lactylation and enhancing tgf-β Signaling in regulatory T cells. Cell Rep (2022) 40(3):111122. doi: 10.1016/j.celrep.2022.111122
11. Jiang J, Huang D, Jiang Y, Hou J, Tian M, Li J, et al. Lactate modulates cellular metabolism through histone lactylation-mediated gene expression in non-small cell lung cancer. Front Oncol (2021) 11:647559. doi: 10.3389/fonc.2021.647559
12. Yu J, Chai P, Xie M, Ge S, Ruan J, Fan X, et al. Histone lactylation drives oncogenesis by facilitating M(6)a reader protein ythdf2 expression in ocular melanoma. Genome Biol (2021) 22(1):85. doi: 10.1186/s13059-021-02308-z
13. Lv X, Lv Y, Dai X. Lactate, histone lactylation and cancer hallmarks. Expert Rev Mol Med (2023) 25:e7. doi: 10.1017/erm.2022.42
14. Borst P. The malate-aspartate shuttle (Borst cycle): how it started and developed into a major metabolic pathway. IUBMB Life (2020) 72(11):2241–59. doi: 10.1002/iub.2367
15. Fan H, Yang F, Xiao Z, Luo H, Chen H, Chen Z, et al. Lactylation: novel epigenetic regulatory and therapeutic opportunities. Am J Physiol Endocrinol Metab (2023) 324(4):E330–e8. doi: 10.1152/ajpendo.00159.2022
16. Jorfeldt L, Juhlin-Dannfelt A, Karlsson J. Lactate release in relation to tissue lactate in human skeletal muscle during exercise. J Appl physiology: respiratory Environ Exercise Physiol (1978) 44(3):350–2. doi: 10.1152/jappl.1978.44.3.350
17. Guppy M, Abas L, Arthur PG, Whisson ME. The pasteur effect in human platelets: implications for storage and metabolic control. Br J haematology (1995) 91(3):752–7. doi: 10.1111/j.1365-2141.1995.tb05381.x
18. Lum JJ, Bauer DE, Kong M, Harris MH, Li C, Lindsten T, et al. Growth factor regulation of autophagy and cell survival in the absence of apoptosis. Cell (2005) 120(2):237–48. doi: 10.1016/j.cell.2004.11.046
19. Zhou Y, Liu X, Huang C, Lin D. Lactate activates ampk remodeling of the cellular metabolic profile and promotes the proliferation and differentiation of C2c12 myoblasts. Int J Mol Sci (2022) 23(22):13996. doi: 10.3390/ijms232213996
20. Damaghi M, West J, Robertson-Tessi M, Xu L, Ferrall-Fairbanks MC, Stewart PA, et al. The harsh microenvironment in early breast cancer selects for a warburg phenotype. Proc Natl Acad Sci United States America (2021) 118(3):e2011342118. doi: 10.1073/pnas.2011342118
21. Végran F, Boidot R, Michiels C, Sonveaux P, Feron O. Lactate influx through the endothelial cell monocarboxylate transporter mct1 supports an nf-κb/il-8 pathway that drives tumor angiogenesis. Cancer Res (2011) 71(7):2550–60. doi: 10.1158/0008-5472.Can-10-2828
22. Yang M, Li J, Gu P, Fan X. The application of nanoparticles in cancer immunotherapy: targeting tumor microenvironment. Bioactive materials (2021) 6(7):1973–87. doi: 10.1016/j.bioactmat.2020.12.010
23. Becker HM, Deitmer JW. Transport metabolons and acid/base balance in tumor cells. Cancers (2020) 12(4):899. doi: 10.3390/cancers12040899
24. Sharonov GV, Serebrovskaya EO, Yuzhakova DV, Britanova OV, Chudakov DM. B cells, plasma cells and antibody repertoires in the tumour microenvironment. Nat Rev Immunol (2020) 20(5):294–307. doi: 10.1038/s41577-019-0257-x
25. Farhood B, Najafi M, Mortezaee K. Cd8(+) cytotoxic T lymphocytes in cancer immunotherapy: A review. J Cell Physiol (2019) 234(6):8509–21. doi: 10.1002/jcp.27782
26. Wu SY, Fu T, Jiang YZ, Shao ZM. Natural killer cells in cancer biology and therapy. Mol Cancer (2020) 19(1):120. doi: 10.1186/s12943-020-01238-x
27. Wang Y, Johnson KCC, Gatti-Mays ME, Li Z. Emerging strategies in targeting tumor-resident myeloid cells for cancer immunotherapy. J Hematol Oncol (2022) 15(1):118. doi: 10.1186/s13045-022-01335-y
28. Zhao P, Wang S, Jiang J, Gao Y, Wang Y, Zhao Y, et al. Targeting lactate metabolism and immune interaction in breast tumor via protease-triggered delivery. J Controlled release Off J Controlled Release Soc (2023) 358:706–17. doi: 10.1016/j.jconrel.2023.05.024
29. Fischer K, Hoffmann P, Voelkl S, Meidenbauer N, Ammer J, Edinger M, et al. Inhibitory effect of tumor cell-derived lactic acid on human T cells. Blood (2007) 109(9):3812–9. doi: 10.1182/blood-2006-07-035972
30. Brand A, Singer K, Koehl GE, Kolitzus M, Schoenhammer G, Thiel A, et al. Ldha-associated lactic acid production blunts tumor immunosurveillance by T and nk cells. Cell Metab (2016) 24(5):657–71. doi: 10.1016/j.cmet.2016.08.011
31. Xie D, Zhu S, Bai L. Lactic acid in tumor microenvironments causes dysfunction of nkt cells by interfering with mtor signaling. Sci China Life Sci (2016) 59(12):1290–6. doi: 10.1007/s11427-016-0348-7
32. Zhang D, Tang Z, Huang H, Zhou G, Cui C, Weng Y, et al. Metabolic regulation of gene expression by histone lactylation. Nature (2019) 574(7779):575–80. doi: 10.1038/s41586-019-1678-1
33. Wang L, Li S, Luo H, Lu Q, Yu S. Pcsk9 promotes the progression and metastasis of colon cancer cells through regulation of emt and pi3k/akt signaling in tumor cells and phenotypic polarization of macrophages. J Exp Clin Cancer Res CR (2022) 41(1):303. doi: 10.1186/s13046-022-02477-0
34. Pan RY, He L, Zhang J, Liu X, Liao Y, Gao J, et al. Positive feedback regulation of microglial glucose metabolism by histone H4 lysine 12 lactylation in alzheimer's disease. Cell Metab (2022) 34(4):634–48.e6. doi: 10.1016/j.cmet.2022.02.013
35. Izzo LT, Wellen KE. Histone lactylation links metabolism and gene regulation. Nature (2019) 574(7779):492–3. doi: 10.1038/d41586-019-03122-1
36. Wang JH, Mao L, Wang J, Zhang X, Wu M, Wen Q, et al. Beyond metabolic waste: lysine lactylation and its potential roles in cancer progression and cell fate determination. Cell Oncol (Dordrecht) (2023) 46(3):465–80. doi: 10.1007/s13402-023-00775-z
37. Lacey JH, Randle PJ. Inhibition of lactate glucogneogenesis in rat kidney by dichloroacetate. Biochem J (1978) 170(3):551–60. doi: 10.1042/bj1700551
38. Emerson PM, Wilkinson JH. Urea and oxalate inhibition of the serum lactate dehydrogenase. J Clin Pathol (1965) 18(6):803–7. doi: 10.1136/jcp.18.6.803
39. Ivashkiv LB. The hypoxia-lactate axis tempers inflammation. Nat Rev Immunol (2020) 20(2):85–6. doi: 10.1038/s41577-019-0259-8
40. Dichtl S, Lindenthal L, Zeitler L, Behnke K, Schlösser D, Strobl B, et al. Lactate and il6 define separable paths of inflammatory metabolic adaptation. Sci Adv (2021) 7(26):3505. doi: 10.1126/sciadv.abg3505
41. Horvat A, Zorec R, Vardjan N. Lactate as an astroglial signal augmenting aerobic glycolysis and lipid metabolism. Front Physiol (2021) 12:735532. doi: 10.3389/fphys.2021.735532
42. Brandt RB, Siegel SA. Methylglyoxal production in human blood. Ciba Foundation symposium (1978) 67):211–23. doi: 10.1002/9780470720493.ch14
43. Bongaerts G, Tolboom J, Naber T, Bakkeren J, Severijnen R, Willems H. D-lactic acidemia and aciduria in pediatric and adult patients with short bowel syndrome. Clin Chem (1995) 41(1):107–10. doi: 10.1093/clinchem/41.1.107
44. Gaffney DO, Jennings EQ, Anderson CC, Marentette JO, Shi T, Schou Oxvig AM, et al. Non-enzymatic lysine lactoylation of glycolytic enzymes. Cell Chem Biol (2020) 27(2):206–13.e6. doi: 10.1016/j.chembiol.2019.11.005
45. Moreno-Yruela C, Zhang D, Wei W, Bæk M, Liu W, Gao J, et al. Class I histone deacetylases (Hdac1-3) are histone lysine delactylases. Sci Adv (2022) 8(3):eabi6696. doi: 10.1126/sciadv.abi6696
46. Moreno-Yruela C, Bæk M, Monda F, Olsen CA. Chiral posttranslational modification to lysine e-amino groups. Accounts Chem Res (2022) 55(10):1456–66. doi: 10.1021/acs.accounts.2c00115
47. Wan N, Wang N, Yu S, Zhang H, Tang S, Wang D, et al. Cyclic immonium ion of lactyllysine reveals widespread lactylation in the human proteome. Nat Methods (2022) 19(7):854–64. doi: 10.1038/s41592-022-01523-1
48. Yang Z, Yan C, Ma J, Peng P, Ren X, Cai S, et al. Lactylome analysis suggests lactylation-dependent mechanisms of metabolic adaptation in hepatocellular carcinoma. Nat Metab (2023) 5(1):61–79. doi: 10.1038/s42255-022-00710-w
49. Martínez-Reyes I, Chandel NS. Cancer metabolism: looking forward. Nat Rev Cancer (2021) 21(10):669–80. doi: 10.1038/s41568-021-00378-6
50. Hay N. Reprogramming glucose metabolism in cancer: can it be exploited for cancer therapy? Nat Rev Cancer (2016) 16(10):635–49. doi: 10.1038/nrc.2016.77
51. Cheng C, Geng F, Cheng X, Guo D. Lipid metabolism reprogramming and its potential targets in cancer. Cancer Commun (London England) (2018) 38(1):27. doi: 10.1186/s40880-018-0301-4
52. Goossens P, Rodriguez-Vita J, Etzerodt A, Masse M, Rastoin O, GouIrand V, et al. Membrane cholesterol efflux drives tumor-associated macrophage reprogramming and tumor progression. Cell Metab (2019) 29(6):1376–89.e4. doi: 10.1016/j.cmet.2019.02.016
53. Son J, Lyssiotis CA, Ying H, Wang X, Hua S, Ligorio M, et al. Glutamine supports pancreatic cancer growth through a kras-regulated metabolic pathway. Nature (2013) 496(7443):101–5. doi: 10.1038/nature12040
54. Nelson JK, Thin MZ, Evan T, Howell S, Wu M, Almeida B, et al. Usp25 promotes pathological hif-1-driven metabolic reprogramming and is a potential therapeutic target in pancreatic cancer. Nat Commun (2022) 13(1):2070. doi: 10.1038/s41467-022-29684-9
55. Lee DC, Sohn HA, Park ZY, Oh S, Kang YK, Lee KM, et al. A lactate-induced response to hypoxia. Cell (2015) 161(3):595–609. doi: 10.1016/j.cell.2015.03.011
56. Gustafsson J, Eriksson J, Marcus C. Glucose metabolism in human adipose tissue studied by 13c-glucose and microdialysis. Scandinavian J Clin Lab Invest (2007) 67(2):155–64. doi: 10.1080/00365510600995259
57. Rabinowitz JD, Enerbäck S. Lactate: the ugly duckling of energy metabolism. Nat Metab (2020) 2(7):566–71. doi: 10.1038/s42255-020-0243-4
58. San-Millán I, Brooks GA. Assessment of metabolic flexibility by means of measuring blood lactate, fat, and carbohydrate oxidation responses to exercise in professional endurance athletes and less-fit individuals. Sports Med (Auckland NZ) (2018) 48(2):467–79. doi: 10.1007/s40279-017-0751-x
59. Gao R, Li Y, Xu Z, Zhang F, Xu J, Hu Y, et al. Mitochondrial pyruvate carrier 1 regulates fatty acid synthase lactylation and mediates treatment of nonalcoholic fatty liver disease. Hepatol (Baltimore Md) (2023) 77(1). doi: 10.1097/hep.0000000000000279
60. Brown TP, Ganapathy V. Lactate/gpr81 signaling and proton motive force in cancer: role in angiogenesis, immune escape, nutrition, and warburg phenomenon. Pharmacol Ther (2020) 206:107451. doi: 10.1016/j.pharmthera.2019.107451
61. Tu CE, Hu Y, Zhou P, Guo X, Gu C, Zhang Y, et al. Lactate and tgf-β Antagonistically regulate inflammasome activation in the tumor microenvironment. J Cell Physiol (2021) 236(6):4528–37. doi: 10.1002/jcp.30169
62. Morland C, Andersson KA, Haugen ØP, Hadzic A, Kleppa L, Gille A, et al. Exercise induces cerebral vegf and angiogenesis via the lactate receptor hcar1. Nat Commun (2017) 8:15557. doi: 10.1038/ncomms15557
63. Young VJ, Brown JK, Maybin J, Saunders PT, Duncan WC, Horne AW. Transforming growth factor-β Induced warburg-like metabolic reprogramming may underpin the development of peritoneal endometriosis. J Clin Endocrinol Metab (2014) 99(9):3450–9. doi: 10.1210/jc.2014-1026
64. Wang H, Zhang B, Li R, Chen J, Xu G, Zhu Y, et al. Kiaa1199 drives immune suppression to promote colorectal cancer liver metastasis by modulating neutrophil infiltration. Hepatol (Baltimore Md) (2022) 76(4):967–81. doi: 10.1002/hep.32383
65. Zhai X, Wang W, Ma Y, Zeng Y, Dou D, Fan H, et al. Serum kiaa1199 is an advanced-stage prognostic biomarker and metastatic oncogene in cholangiocarcinoma. Aging (2020) 12(23):23761–77. doi: 10.18632/aging.103964
66. Luo Y, Yang Z, Yu Y, Zhang P. Hif1α Lactylation enhances kiaa1199 transcription to promote angiogenesis and vasculogenic mimicry in prostate cancer. Int J Biol macromolecules (2022) 222(Pt B):2225–43. doi: 10.1016/j.ijbiomac.2022.10.014
67. Lan H, Liu Y, Liu J, Wang X, Guan Z, Du J, et al. Tumor-associated macrophages promote oxaliplatin resistance via mettl3-mediated M(6)a of traf5 and necroptosis in colorectal cancer. Mol pharmaceutics (2021) 18(3):1026–37. doi: 10.1021/acs.molpharmaceut.0c00961
68. Xiong J, He J, Zhu J, Pan J, Liao W, Ye H, et al. Lactylation-driven mettl3-mediated rna M(6)a modification promotes immunosuppression of tumor-infiltrating myeloid cells. Mol Cell (2022) 82(9):1660–77.e10. doi: 10.1016/j.molcel.2022.02.033
69. Yunna C, Mengru H, Lei W, Weidong C. Macrophage M1/M2 polarization. Eur J Pharmacol (2020) 877:173090. doi: 10.1016/j.ejphar.2020.173090
70. Mantovani A, Sozzani S, Locati M, Allavena P, Sica A. Macrophage polarization: tumor-associated macrophages as a paradigm for polarized M2 mononuclear phagocytes. Trends Immunol (2002) 23(11):549–55. doi: 10.1016/s1471-4906(02)02302-5
71. Chen Y, Zhang S, Wang Q, Zhang X. Tumor-recruited M2 macrophages promote gastric and breast cancer metastasis via M2 macrophage-secreted chi3l1 protein. J Hematol Oncol (2017) 10(1):36. doi: 10.1186/s13045-017-0408-0
72. Wang S, Liu R, Yu Q, Dong L, Bi Y, Liu G. Metabolic reprogramming of macrophages during infections and cancer. Cancer Lett (2019) 452:14–22. doi: 10.1016/j.canlet.2019.03.015
73. Chaudagar K, Hieromnimon HM, Khurana R, Labadie B, Hirz T, Mei S, et al. Reversal of lactate and pd-1-mediated macrophage immunosuppression controls growth of pten/P53-deficient prostate cancer. Clin Cancer Res an Off J Am Assoc Cancer Res (2023) 29(10):1952–68. doi: 10.1158/1078-0432.Ccr-22-3350
74. Yang L, Pang Y, Moses HL. Tgf-beta and immune cells: an important regulatory axis in the tumor microenvironment and progression. Trends Immunol (2010) 31(6):220–7. doi: 10.1016/j.it.2010.04.002
75. Seoane J, Gomis RR. Tgf-β Family signaling in tumor suppression and cancer progression. Cold Spring Harbor Perspect Biol (2017) 9(12):a022277. doi: 10.1101/cshperspect.a022277
76. Ansa-Addo EA, Zhang Y, Yang Y, Hussey GS, Howley BV, Salem M, et al. Membrane-organizing protein moesin controls treg differentiation and antitumor immunity via tgf-β Signaling. J Clin Invest (2017) 127(4):1321–37. doi: 10.1172/jci89281
77. Jiang T, Gao C, Ma C, Xu P. Microbial lactate utilization: enzymes, pathogenesis, and regulation. Trends Microbiol (2014) 22(10):589–99. doi: 10.1016/j.tim.2014.05.008
78. Park SS, Gonzalez-Juarbe N, Martínez E, Hale JY, Lin YH, Huffines JT, et al. Streptococcus pneumoniae binds to host lactate dehydrogenase via pspa and pspc to enhance virulence. mBio (2021) 12(3):e00673–21. doi: 10.1128/mBio.00673-21
79. Christensen DG, Meyer JG, Baumgartner JT, D'Souza AK, Nelson WC, Payne SH, et al. Identification of novel protein lysine acetyltransferases in escherichia coli. mBio (2018) 9(5):e01905–18. doi: 10.1128/mBio.01905-18
80. Dong H, Zhang J, Zhang H, Han Y, Lu C, Chen C, et al. Yiac and cobb regulate lysine lactylation in escherichia coli. Nat Commun (2022) 13(1):6628. doi: 10.1038/s41467-022-34399-y
81. Amieva M, Peek RM Jr.Pathobiology of helicobacter pylori-induced gastric cancer. Gastroenterology (2016) 150(1):64–78doi: 10.1053/j.gastro.2015.09.004
82. Rubinstein MR, Wang X, Liu W, Hao Y, Cai G, Han YW. Fusobacterium nucleatum promotes colorectal carcinogenesis by modulating E-cadherin/β-catenin signaling via its fada adhesin. Cell Host Microbe (2013) 14(2):195–206. doi: 10.1016/j.chom.2013.07.012
83. Li ZR, Li J, Cai W, Lai JYH, McKinnie SMK, Zhang WP, et al. Macrocyclic colibactin induces DNA double-strand breaks via copper-mediated oxidative cleavage. Nat Chem (2019) 11(10):880–9. doi: 10.1038/s41557-019-0317-7
84. Xie Y, Xie F, Zhou X, Zhang L, Yang B, Huang J, et al. Microbiota in tumors: from understanding to application. Advanced Sci (Weinheim Baden-Wurttemberg Germany) (2022) 9(21):e2200470. doi: 10.1002/advs.202200470
85. Kong C, Yan X, Zhu Y, Zhu H, Luo Y, Liu P, et al. Fusobacterium nucleatum promotes the development of colorectal cancer by activating a cytochrome P450/epoxyoctadecenoic acid axis via tlr4/keap1/nrf2 signaling. Cancer Res (2021) 81(17):4485–98. doi: 10.1158/0008-5472.Can-21-0453
86. Zeng X, Xing X, Gupta M, Keber FC, Lopez JG, Lee YJ, et al. Gut bacterial nutrient preferences quantified in vivo. Cell (2022) 185(18):3441–56.e19. doi: 10.1016/j.cell.2022.07.020
87. Seelbinder B, Lohinai Z, Vazquez-Uribe R, Brunke S, Chen X, Mirhakkak M, et al. Candida expansion in the gut of lung cancer patients associates with an ecological signature that supports growth under dysbiotic conditions. Nat Commun (2023) 14(1):2673. doi: 10.1038/s41467-023-38058-8
88. Wang J, Liu Z, Xu Y, Wang Y, Wang F, Zhang Q, et al. Enterobacterial lps-inducible linc00152 is regulated by histone lactylation and promotes cancer cells invasion and migration. Front Cell infection Microbiol (2022) 12:913815. doi: 10.3389/fcimb.2022.913815
89. Park EM, Chelvanambi M, Bhutiani N, Kroemer G, Zitvogel L, Wargo JA. Targeting the gut and tumor microbiota in cancer. Nat Med (2022) 28(4):690–703. doi: 10.1038/s41591-022-01779-2
90. He Y, Ji Z, Gong Y, Fan L, Xu P, Chen X, et al. Numb/parkin-directed mitochondrial fitness governs cancer cell fate via metabolic regulation of histone lactylation. Cell Rep (2023) 42(2):112033. doi: 10.1016/j.celrep.2023.112033
91. Jin J, Bai L, Wang D, Ding W, Cao Z, Yan P, et al. Sirt3-dependent delactylation of cyclin E2 prevents hepatocellular carcinoma growth. EMBO Rep (2023) 24(5):e56052. doi: 10.15252/embr.202256052
92. Pan L, Feng F, Wu J, Fan S, Han J, Wang S, et al. Demethylzeylasteral targets lactate by inhibiting histone lactylation to suppress the tumorigenicity of liver cancer stem cells. Pharmacol Res (2022) 181:106270. doi: 10.1016/j.phrs.2022.106270
93. Wang X, Ying T, Yuan J, Wang Y, Su X, Chen S, et al. Brafv600e restructures cellular lactylation to promote anaplastic thyroid cancer proliferation. Endocrine-related Cancer (2023) 30(8):e220344. doi: 10.1530/erc-22-0344
94. Yang J, Luo L, Zhao C, Li X, Wang Z, Zeng Z, et al. A positive feedback loop between inactive vhl-triggered histone lactylation and pdgfrβ Signaling drives clear cell renal cell carcinoma progression. Int J Biol Sci (2022) 18(8):3470–83. doi: 10.7150/ijbs.73398
95. Hui S, Ghergurovich JM, Morscher RJ, Jang C, Teng X, Lu W, et al. Glucose feeds the tca cycle via circulating lactate. Nature (2017) 551(7678):115–8. doi: 10.1038/nature24057
96. Sharma D, Singh M, Rani R. Role of ldh in tumor glycolysis: regulation of ldha by small molecules for cancer therapeutics. Semin Cancer Biol (2022) 87:184–95. doi: 10.1016/j.semcancer.2022.11.007
97. Baumann F, Leukel P, Doerfelt A, Beier CP, Dettmer K, Oefner PJ, et al. Lactate promotes glioma migration by tgf-beta2-dependent regulation of matrix metalloproteinase-2. Neuro-oncology (2009) 11(4):368–80. doi: 10.1215/15228517-2008-106
98. Jia M, Yue X, Sun W, Zhou Q, Chang C, Gong W, et al. Ulk1-mediated metabolic reprogramming regulates vps34 lipid kinase activity by its lactylation. Sci Adv (2023) 9(22):eadg4993. doi: 10.1126/sciadv.adg4993
99. Halestrap AP. Monocarboxylic acid transport. Compr Physiol (2013) 3(4):1611–43. doi: 10.1002/cphy.c130008
100. Weng YS, Tseng HY, Chen YA, Shen PC, Al Haq AT, Chen LM, et al. Mct-1/mir-34a/il-6/il-6r signaling axis promotes emt progression, cancer stemness and M2 macrophage polarization in triple-negative breast cancer. Mol Cancer (2019) 18(1):42. doi: 10.1186/s12943-019-0988-0
101. Fan Q, Yang L, Zhang X, Ma Y, Li Y, Dong L, et al. Autophagy promotes metastasis and glycolysis by upregulating mct1 expression and wnt/β-catenin signaling pathway activation in hepatocellular carcinoma cells. J Exp Clin Cancer Res CR (2018) 37(1):9. doi: 10.1186/s13046-018-0673-y
102. Zhang G, Zhang Y, Dong D, Wang F, Ma X, Guan F, et al. Mct1 regulates aggressive and metabolic phenotypes in bladder cancer. J Cancer (2018) 9(14):2492–501. doi: 10.7150/jca.25257
103. Zeng Q, Wang K, Zhao Y, Ma Q, Chen Z, Huang W. Effects of the acetyltransferase P300 on tumour regulation from the novel perspective of posttranslational protein modification. Biomolecules (2023) 13(3):417. doi: 10.3390/biom13030417
104. Liu X, Bao X, Hu M, Chang H, Jiao M, Cheng J, et al. Inhibition of pcsk9 potentiates immune checkpoint therapy for cancer. Nature (2020) 588(7839):693–8. doi: 10.1038/s41586-020-2911-7
105. Gossage L, Eisen T, Maher ER. Vhl, the story of a tumour suppressor gene. Nat Rev Cancer (2015) 15(1):55–64. doi: 10.1038/nrc3844
106. Quintanal-Villalonga Á, Chan JM, Yu HA, Pe'er D, Sawyers CL, Sen T, et al. Lineage plasticity in cancer: A shared pathway of therapeutic resistance. Nat Rev Clin Oncol (2020) 17(6):360–71. doi: 10.1038/s41571-020-0340-z
107. Turkson S, Kloster A, Hamilton PJ, Neigh GN. Neuroendocrine drivers of risk and resilience: the influence of metabolism & Mitochondria. Front Neuroendocrinol (2019) 54:100770. doi: 10.1016/j.yfrne.2019.100770
108. Huang Y, Sun G, Sun X, Li F, Zhao L, Zhong R, et al. The potential of lonidamine in combination with chemotherapy and physical therapy in cancer treatment. Cancers (2020) 12(11):3332. doi: 10.3390/cancers12113332
109. De Cesare M, Pratesi G, Giusti A, Polizzi D, Zunino F. Stimulation of the apoptotic response as a basis for the therapeutic synergism of lonidamine and cisplatin in combination in human tumour xenografts. Br J Cancer (1998) 77(3):434–9. doi: 10.1038/bjc.1998.69
110. Michelakis ED, Webster L, Mackey JR. Dichloroacetate (Dca) as a potential metabolic-targeting therapy for cancer. Br J Cancer (2008) 99(7):989–94. doi: 10.1038/sj.bjc.6604554
111. Dunbar EM, Coats BS, Shroads AL, Langaee T, Lew A, Forder JR, et al. Phase 1 trial of dichloroacetate (Dca) in adults with recurrent Malignant brain tumors. Investigational New Drugs (2014) 32(3):452–64. doi: 10.1007/s10637-013-0047-4
112. Powell SF, Mazurczak M, Dib EG, Bleeker JS, Geeraerts LH, Tinguely M, et al. Phase ii study of dichloroacetate, an inhibitor of pyruvate dehydrogenase, in combination with chemoradiotherapy for unresected, locally advanced head and neck squamous cell carcinoma. Investigational New Drugs (2022) 40(3):622–33. doi: 10.1007/s10637-022-01235-5
113. Benyahia Z, Blackman M, Hamelin L, Zampieri LX, Capeloa T, Bedin ML, et al. In vitro and in vivo characterization of mct1 inhibitor azd3965 confirms preclinical safety compatible with breast cancer treatment. Cancers (2021) 13(3):569. doi: 10.3390/cancers13030569
114. Halford S, Veal GJ, Wedge SR, Payne GS, Bacon CM, Sloan P, et al. A phase I dose-escalation study of azd3965, an oral monocarboxylate transporter 1 inhibitor, in patients with advanced cancer. Clin Cancer Res an Off J Am Assoc Cancer Res (2023) 29(8):1429–39. doi: 10.1158/1078-0432.Ccr-22-2263
115. Curiel TJ, Coukos G, Zou L, Alvarez X, Cheng P, Mottram P, et al. Specific recruitment of regulatory T cells in ovarian carcinoma fosters immune privilege and predicts reduced survival. Nat Med (2004) 10(9):942–9. doi: 10.1038/nm1093
116. Deng H, Kan A, Lyu N, He M, Huang X, Qiao S, et al. Tumor-derived lactate inhibit the efficacy of lenvatinib through regulating pd-L1 expression on neutrophil in hepatocellular carcinoma. J immunotherapy Cancer (2021) 9(6):e002305. doi: 10.1136/jitc-2020-002305
117. Morrissey SM, Zhang F, Ding C, Montoya-Durango DE, Hu X, Yang C, et al. Tumor-derived exosomes drive immunosuppressive macrophages in a pre-metastatic niche through glycolytic dominant metabolic reprogramming. Cell Metab (2021) 33(10):2040–58.e10. doi: 10.1016/j.cmet.2021.09.002
Keywords: immunosuppression, lactylation (Kla), metabolic reprogramming, tumor microenvironment (TME), Warburg effect
Citation: Su J, Zheng Z, Bian C, Chang S, Bao J, Yu H, Xin Y and Jiang X (2023) Functions and mechanisms of lactylation in carcinogenesis and immunosuppression. Front. Immunol. 14:1253064. doi: 10.3389/fimmu.2023.1253064
Received: 04 July 2023; Accepted: 31 July 2023;
Published: 14 August 2023.
Edited by:
Dong Ren, UC Irvine Medical Center, United StatesReviewed by:
Ningbo Liu, Tianjin Medical University, ChinaCopyright © 2023 Su, Zheng, Bian, Chang, Bao, Yu, Xin and Jiang. This is an open-access article distributed under the terms of the Creative Commons Attribution License (CC BY). The use, distribution or reproduction in other forums is permitted, provided the original author(s) and the copyright owner(s) are credited and that the original publication in this journal is cited, in accordance with accepted academic practice. No use, distribution or reproduction is permitted which does not comply with these terms.
*Correspondence: Ying Xin, eGlueUBqbHUuZWR1LmNu; Xin Jiang, amlhbmd4QGpsdS5lZHUuY24=
Disclaimer: All claims expressed in this article are solely those of the authors and do not necessarily represent those of their affiliated organizations, or those of the publisher, the editors and the reviewers. Any product that may be evaluated in this article or claim that may be made by its manufacturer is not guaranteed or endorsed by the publisher.
Research integrity at Frontiers
Learn more about the work of our research integrity team to safeguard the quality of each article we publish.