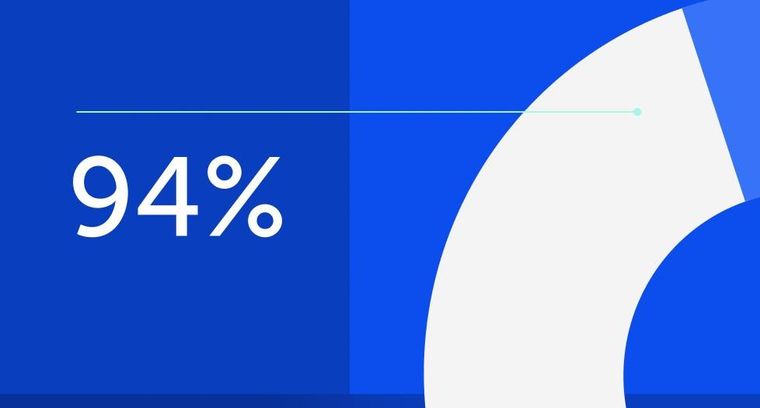
94% of researchers rate our articles as excellent or good
Learn more about the work of our research integrity team to safeguard the quality of each article we publish.
Find out more
ORIGINAL RESEARCH article
Front. Immunol., 14 September 2023
Sec. Inflammation
Volume 14 - 2023 | https://doi.org/10.3389/fimmu.2023.1250942
This article is part of the Research TopicBiology of C-reactive ProteinView all 17 articles
C-reactive protein (CRP) is an evolutionary highly conserved protein. Like humans, CRP acts as a major acute phase protein in pigs. While CRP regulatory mechanisms have been extensively studied in humans, little is known about the molecular mechanisms that control pig CRP gene expression. The main goal of the present work was to study the regulatory mechanisms and identify functional genetic variants regulating CRP gene expression and CRP blood levels in pigs. The characterization of the porcine CRP proximal promoter region revealed a high level of conservation with both cow and human promoters, sharing binding sites for transcription factors required for CRP expression. Through genome-wide association studies and fine mapping, the most associated variants with both mRNA and protein CRP levels were localized in a genomic region 39.3 kb upstream of CRP. Further study of the region revealed a highly conserved putative enhancer that contains binding sites for several transcriptional regulators such as STAT3, NF-kB or C/EBP-β. Luciferase reporter assays showed the necessity of this enhancer-promoter interaction for the acute phase induction of CRP expression in liver, where differences in the enhancer sequences significantly modified CRP activity. The associated polymorphisms disrupted the putative binding sites for HNF4α and FOXA2 transcription factors. The high correlation between HNF4α and CRP expression levels suggest the participation of HNF4α in the regulatory mechanism of porcine CRP expression through the modification of its binding site in liver. Our findings determine, for the first time, the relevance of a distal regulatory element essential for the acute phase induction of porcine CRP in liver and identify functional polymorphisms that can be included in pig breeding programs to improve immunocompetence.
The C-reactive Protein (CRP) is an evolutionary well-conserved protein that plays a significant role in the acute phase response to inflammation. This protein belongs to the pentraxins family and has two conformations: native CRP (nCRP) and monomeric CRP (mCRP). Although this protein is mainly produced by hepatocytes, it is also synthesized, in marginal concentrations, in neurons, monocytes, lymphocytes and adipocytes (1).
Depending on the conformation present in any given stage of the inflammatory process, CRP serves as a pro-inflammatory molecule through the activation of the initial stages of the complement system and the modulation of nitric oxide release and cytokine synthesis. Moreover, CRP functions as an anti-inflammatory compound by controlling the progression and intensity of the late stages of inflammation and modulating apoptosis and phagocytosis processes (2–5). CRP protein levels are currently being used as a stable marker in humans for the prediction, prevention and prognosis of cardiovascular disease as well as several other chronic diseases (6–8).
There is sufficient evidence that CRP blood levels are a complex phenotype with several environmental and genetic determinants. External factors such as the weight or the overall stress levels of individuals may potentially modulate the levels of this protein (9, 10). At genetic level, several studies in humans have determined the impact of polymorphisms in CRP and in other inflammatory-related genes on CRP blood levels (11–13). In pigs, a genome wide association study (GWAS) in a commercial Duroc population identified the genomic region in which CRP is annotated as associated with the variation in its translated protein levels (14). Furthermore, according to several studies, a variety of transcription factors affect the expression of this gene, highlighting the need to further study the interaction and effects of CRP regulatory pathways (15–19). While HNF-1, HNF-3, and OCT-1 transcriptions factors are involved in the regulation of basal CRP expression levels (20–22), the induction of the acute phase expression of CRP is mediated by the effect of cytokines, particularly IL-6, IL-1β and TNF-α, through the activation of STAT3, NF-kB and C/EBP-β transcription factors (16, 19, 23–25). In this regard, a recent study in human Hep3B cells identified an enhancer upstream of the CRP promoter enriched in binding sites for STAT3 and C/EBP-β with a major impact on the acute phase induction of CRP expression (26).
Considering the high resemblance between pig and human immune responses, understanding the molecular mechanisms that control porcine CRP gene expression may further support the use of the pig as a biomedical animal model for the study of human diseases. In other vein, genetic selection for immunity traits in livestock has been proposed as a promising approach for improving animal robustness and disease resistance, thus contributing to healthier livestock while reducing the emergence of antibiotic resistances (27–30).
The present work aimed to study the regulatory mechanisms affecting the expression of porcine CRP and identify causal genetic variants that influence CRP levels in blood to better understand the genomic physiology of immunocompetence in pigs.
All experimental protocols and procedures with pigs were approved by the Institut de Recerca i Tecnologia Agroalimentàries (IRTA) Ethical Committee in accordance with the Spanish Policy for Animal Protection RD53/2013, which meets the European Union Directive 2010/63/EU for the correct practices and protection of the animals used in experimentation.
The study was performed with a population of 432 healthy piglets (217 males and 215 females of around 60 days of age) belonging to a commercial Duroc pig line. The pigs came from six batches (72 ± 1 animals per batch) and were raised in the same farm with an ad libitum cereal-base commercial diet. At the moment of sampling, the animals did not present any sign of infection or pathology, and no antibiotics were supplied.
Blood was collected via the external jugular vein into vacutainer tubes with or without anticoagulants (Sangüesa S.A., Spain), which required the restraint of the animals but not their sedation. Serum samples, in duplicate, diluted 1:3000 were used to measure CRP levels by ELISA kit (Abcam Plc., Spain) following manufacturer’s instructions. Absorbance was read at 450 nm using an ELISA plate reader (Bio-Rad) and analysed using the Microplate manager 5.2.1 sofware (Bio-Rad). Genomic DNA was extracted from blood samples using the NucleoSpin Blood (Macherey–Nagel, Germany). DNA concentration and purity were measured in a Nanodrop ND-1000 spectrophotometer.
The 432 animals of the Duroc population were genotyped with the GGP Porcine HD Array (Illumina, San Diego, CA) using the Infinium HD Assay Ultra protocol. The software PLINK/v1.90b3.42 (31) was used to remove those single-nucleotide polymorphisms (SNPs) with a minor allele frequency (MAF) lower than 5%, SNPs with more than 10% missing genotypes, and SNPs that did not map to the porcine reference genome (Sscrofa11.1). A subset of 42,641 SNPs remained for further analysis. Additionally, the rs327446000 SNP within the CRP gene was genotyped for the 432 animals by custom designed Taqman assays in a QuantStudioTM 12K Flex Real-Time PCR System (ThermoFisher Scientific).
Whole-genome sequences from 300 pigs (n=100 Landrace, n=100 Large White, and n=100 Duroc) (32) were used to identify and estimate the segregation of CRP polymorphisms. All DNA samples were sequenced (NovaSeq6000 platform) to a minimum read depth of 10X. DNA sequences were mapped against the reference genome (Sscrofa11.1 assembly) with BWA-MEM/0.7.17 (33) and 44,127,400 polymorphisms (SNPs and indels) were extracted using the GATK/4.1.8.0 Haplotype Caller (34). After filtering genetic variants with PLINK/v1.90b3.42 software a total of 25,315,878 polymorphisms remained for downstream analysis. Furthermore, the expression levels of CRP isoforms in the liver were quantified in RNA-seq data from the same 300 pigs (32). RNA-seq reads were mapped against the reference genome (Sscrofa11.1 assembly) with STAR/v2.5.3a (35) using ENCODE parameters. Annotated genes and isoforms in Ensembl Genes 101 database were quantified with RSEM/1.3.0 (36) using default parameters.
SNPs and indels within and in the vicinity of the CRP (between positions 90.7-90.8 Mb on SSC4) were extracted from whole genome sequencing (WGS) data. VEP software (37) was used to locate and predict the consequences of variants on the CRP protein sequence using the Ensembl Genes 106 annotation database and the Scrofa11.1 assembly. The promoter regions of the porcine CRP isoforms were aligned to the reference human and cow orthologue promoter sequences annotated in the Ensembl database with the Multalin software (38) in order to measure the level of conservation between them.
A computer-assisted identification of putative transcription factors binding sites in CRP regulatory regions (enhancer and proximal promoter) was performed with LASAGNA-Search 2.0 (39).
The association between polymorphisms identified in both the regulatory and coding regions of CRP and CRP blood levels (n=100 Duroc individuals) was analyzed by using the aov() function in R. Normality of CRP data was checked through Shapiro-Wilk test in R, and logarithm transformation was applied to reach normal distribution of residuals (P-value > 0.05). Systematic non-genetic putative effects (sex and batch) were tested by using the lm() function in R. When significant, sex and/or batch effects were considered for subsequent analyses. Multiple testing corrections were performed with the false discovery rate (FDR) method using the p.adjust function in R (40). Significance threshold for the association was set at FDR ≤ 0.05.
GWAS was performed using 42,641 SNPs, together with the rs327446000 CRP SNP, and the CRP levels in serum of 432 Duroc animals using the GCTA 1.94.1 software (41) with the following model:
Where yij corresponds to the phenotype (log transformed CRP levels in serum) of the ith individual in the jth batch; bj corresponds to the jth batch effect (6 levels); ui is the infinitesimal genetic effect of individual i, with u∼N(0, G2u), where G is the genomic relationship matrix (GRM) calculated using the filtered autosomal SNPs based on the methodology of (42) and σ2u is the additive genetic variance; sli is the genotype (coded as 0, 1, 2) for the lth SNP, being al its allele substitution effect on the trait under study; and eij is the residual term.
The false discovery rate (FDR) method of multiple testing described by Benjamini and Hochberg (40) was used to measure the statistical significance for association studies at genome-wide level with the p.adjust function of R. The significant association threshold was set at FDR ≤ 0.05. A Manhattan plot based on the resulting significance was generated using the R package qqman (43).
WGS data from 100 individuals of the same Duroc population was used to impute genotypes at the whole genome level of the 354 individuals (332 piglets and 22 boars) that had been previously genotyped with the GGP Porcine HD Array.
Imputation and haplotype reconstruction was performed with 19,610 SNPs (MAF ≥ 5%), covering the SSC4:88,251,177-92,759,955 bp genomic region, using DualPHASE/v.2.3 software (44). This haplotype-based approach exploits population (linkage disequilibrium; LD) and family information (Mendelian segregation and linkage analysis; LA) through a Hidden Markov model. Linkage was estimated based on the equivalence 1Mb~1cM.
GWAS for the CRP levels in serum and the 19,610 SNPs was performed using the fastGWA option of the GCTA 1.94.1 (41) software, following the previous model (1). QTL fine-mapping was performed with Qxpak/v5 (45) based on the reconstructed haplotypes to simultaneously exploiting LA and LD with the following mixed model:
Where corresponds to the vector containing the batch fixed effect, h is the vector of random QTL effects corresponding to the K cluster defined by the Hidden State (HS), u is the vector of random individual polygenic effects and e is the vector containing the residuals.
Multiple test correction was performed using the Bonferroni method (46). The significant association threshold was set at p adjust ≤ 0.05.
Expression GWAS (eGWAS) were performed with a total of 25,315,878 genetic variants and the RNA-seq expression data of each CRP isoform in the 300 pigs (n=100 Landrace, n=100 Large White and n=100 Duroc). The association was estimated by fitting the previously described model (1) using the GCTA software (41):
Where yijk corresponds to the gene expression in the ith individual of sex j and belonging to the kth breed (3 levels); ui, sli, al and eijk are as defined in the previous GWAS model. After multiple testing adjustment, association threshold was established at FDR ≤ 0.05. Manhattan plots were generated in R as previously mentioned.
Two individuals of the Duroc population with different haplotypes (Haplotype P1: G – T – C – G – C – T – G – A – C and Haplotype P2: A – A – C – A – T – C – A – G – T) for CRP proximal promoter polymorphisms were selected for the cloning process. Genomic DNA was used to amplify two fragments of ~600bp corresponding to CRP promoter regions of the selected animals, using the forward primer 5’-GAGGATATCAAGATCGATCAAGCACATGTTTCACTGC-3’ and the reverse primer 5’-CCGGATTGCCAAGCTCCCCTTGGAGAAGATGCC-3’, containing the HindIII and BglII restriction sites. Amplification of the fragments was performed by PCR with Hot-Star Taq Master Mix Kit (Qiagen, Spain) under the following conditions: 15 min at 94°C, 35 cycles of 1 min at 94°C, 1 min at 60°C and 1 min at 72°C and a final extension of 10 min at 72°C.
PCR products were cloned into pNL1.2[NlucP] vector (Promega, Spain) with the In-Fusion Snap Assembly cloning kit (Takara, Japan). Plasmids were purified using PureYield™ Plasmid Miniprep System kit (Promega, Spain). Enhancer region fragments of ~280bp for the same individuals (Haplotype E1: G – C – T – Ø – A – A – G – C – G and Haplotype E2: A – A – C – TTCTGTTTGTGGGACCGGCCC – G – G – A – T – T) were amplified by PCR using the forward primer 5’-GCTCGCTAGCCTCGAGTGGAAGAGAGGGTGGGGTG-3’ and the reverse primer 5’-TTGATCGATCTTGATATCGCAGCTACCTCAGAACACAGTC-3’, containing the XhoI and EcoRV restriction sites, with Hot-Star Taq Master Mix Kit (Qiagen, Spain) and the previous conditions, and were inserted upstream of the promoter regions of the corresponding haplotypes. Nucleotide sequence of each DNA construct was confirmed by Sanger sequencing.
HepG2 cells (ATCC, USA) were seeded at a density of 3x104 cells per well in a 96 wells plate in DMEM supplemented with 10% inactivated fetal bovine serum, 1% penicillin-streptomycin, 1% L-glutamine and 1% pyruvate. After 24h cells were cotransfected with 500ng of pNL1.2[NlucP] vector, 12.5ng of pGL4.54[luc2/TK] and 487.5ng of transfection carrier DNA (Promega, Spain) using ViaFect™ Transfection Reagent (3:1) (Promega, Spain) in serum-free medium for 16h. Cells were then treated with IL-6 (10ng/ml) and IL-1β (1ng/ml). Luciferase activity measurements were performed 24h after stimulation with Dual-Luciferase Reporter Assay System (Promega, Spain). Expression of nanoluc luciferase driven by inserted promoters and enhancers was normalized to the cotransfected firefly control vectors. Every luciferase assay was made by triplicate in different days with three replicates for each vector in each experiment to increase the robustness of the results.
Since human CRP gene has been proved to be regulated at the transcriptional level by the binding of transcription factors in its promoter region (18, 47), we characterized the pig CRP promoter region in order to assess the level of conservation between human and cow species. A highly conserved region in Sus Scrofa chromosome 4 (SSC4) at position 90,782,578-90,782,833 bp was identified when compared to the human (GRCh38 1:159,714,589-159,716,089) and cow (ARS-UCD1.2 3:9,982,001-9,983,501) CRP promoter regions (Supplementary Figure 1). In addition, we performed an in-silico characterization of transcription factor binding sites (TFBSs) in the pig CRP promoter region, identifying four TFBSs conserved between pig and cow, four more between pig and human and three binding motifs maintained in all three species (Supplementary Figure 1).
A total of 133 polymorphisms, most of them associated with plasma CRP levels, were identified in the CRP gene region in the WGS data from 100 Duroc pigs (Supplementary Table 1). The most significantly associated variant was rs327446000 (4:90,800,879 bp), located in the 3’ untranslated region (UTR) of CRP. Furthermore, we identified five polymorphisms in the pig CRP promoter region affecting the binding sites for C/EBP, FOXA1 and p53 transcription factors (Figure 1).
Figure 1 Position of the transcription factor binding sites located in the CRP promoter region. Marked in red are the SNPs found in the studied population and the transcription factors binding sites affected by the SNPs. The arrow marks the start of CRP-202 exon 1.
The association between CRP polymorphisms and the variation of serum CRP levels was explored through GWAS with 42,641 SNPs plus the rs327446000 SNP from 432 Duroc pigs. A genomic region in SSC4 at 90.54-90.80 Mb was associated with serum CRP levels, with rs327446000 being the most significantly associated genetic variant (Table 1; Figure 2A).
Table 1 Significant polymorphisms associated to the CRP levels in serum: position, minimum allele frequency and allele substitution effect significance.
Figure 2 (A) Manhattan plot representing the association analysis between the CRP levels in serum and SNPs distributed along the pig chromosomes. (B) Scatter plot depicting P-value distribution of the CRP QTL at chromosome level. (C) Scatter plot depicting P-value distribution of the CRP QTL LDLA. Horizontal lines indicate the adjusted significance threshold (≤ 0.05). Vertical green lines encompass the CRP gene location.
Further exploration of the CRP QTL was performed by both GWAS and LDLA analyses using SSC4 genotypes from 19,610 SNPs comprising 4Mb (2Mb up and 2Mb down) of the previously declared associated genomic region. According to the GWAS results, a total of 1,482 SNPs located within a genomic region in SSC4 at 89.7-91.29 Mb were associated to the phenotype, being the top signal located at 90.80 Mb of SSC4 (rs327446000; P-value = 1.49 x 10-10) inside CRP (Figure 2B). In contrast, the LDLA study revealed a total of 483 significant signals which reduced the previous region to 90.53-90.80 Mb and positioned the maximum association at 90.53-90.62 Mb of SSC4 (P-value = 1.88 x 10-6) upstream of CRP (Figure 2C).
To identify potential functional variants affecting the expression of CRP, an eGWAS analysis was performed using 25,315,878 genetic variants and the expression of CRP isoforms in 300 pigs (n=100 Landrace, n=100 Large White, and n=100 Duroc).
A strongly associated region in SSC4 at 86-93 Mb for the expression of CRP isoform 202 (ENSSSCT00000054270.2) was identified (Figure 3), whereas not significantly associated regions in SSC4 were identified for the other CRP isoforms. A total of 8,250 polymorphisms were found associated (FDR ≤ 0.05) along SSC4. The top variants (adjusted P-value = 3.40 x 10-23) were rs793561911 and rs713631040, located in the positions 4:90,743,523 bp and 4:90,743,532 bp respectively, around 39.3 Kb upstream the CRP gene (Table 2; Supplementary Table 2).
Figure 3 Manhattan plot representing the association analysis between the CRP mRNA expression of the isoform 202 and polymorphisms distributed along the pig chromosomes. Red line indicates the genome wide significance threshold (FDR ≤ 0.05).
Table 2 The 10 most significant polymorphisms associated to the CRP expression levels: position, alleles, minimum allele frequency and allele substitution effect significance.
The polymorphisms most significantly associated with CRP-202 isoform expression were located in an intergenic region conserved between several pig breeds and other species such as cow, sheep and horse (Supplementary Figure 2). The alignment of this region in the porcine genome (SSC4:90,743,525-90,743,526) against the human genome revealed the presence of a human conserved sequence corresponding to an enhancer element (ENSR00000931831). This distal enhancer region was also well conserved in the cow genome (ARS-UCD1.2 3:9,937,919:9,938,698). In the three species, this region was located at 39-44 Kb upstream of CRP. To better understand the regulatory role of this region on CRP-202 expression, an in-silico characterization of TFBSs was performed. Remarkably, a total of 26 TFBSs known to regulate CRP expression were found within this region. Eight of them were shared with the cow genome and another site was conserved in both cow and human genomes (Supplementary Figure 3).
In depth analysis of this region revealed that rs793561911 and rs713631040 variants were located within the binding site of the transcription factor HNF4α. Furthermore, the insertion allele of the rs793561911 polymorphism (-/TTCTGTTTGTGGGACCGGCCC) generated a binding site for the FOXA2 transcription factor. Seven other SNPs were found in this conserved region in the studied population (Figure 4). Moreover, a third polymorphism (rs338992142) in the position 4:90,743,570 bp was found to be in the potential binding site of both transcription factors HNF4α and FOXA2. This SNP was also found to be associated with CRP expression in the eGWAS (Table 2). These three polymorphisms and a fourth significant SNP (rs330141279) located in the position 4:90,743,549 bp were in total linkage disequilibrium resulting the following haplotype combinations: Haplotype E1: Ø – A – A – G and Haplotype E2: TTCTGTTTGTGGGACCGGCCC – G – G – A. Figure 4 shows the enhancer regions of animals with haplotypes E1 and E2.
Figure 4 Position of the transcription factor binding sites located in the enhancer region for the different transfected sequences. Marked in red are the polymorphisms found in the studied population and the TF binding sites affected by the changes in the sequence. Highlighted in yellow are the top polymorphisms found in the eGWAS (rs793561911 and rs713631040 respectively).
When we studied the correlation between CRP-202 mRNA expression and HNF4α and FOXA2 mRNA expression in 300 pigs, a higher correlation was observed between HNF4α and CRP gene expression when compared to FOXA2- CRP-202 correlation (Table 3). Remarkably, Duroc and Landrace animals presented higher correlations between HNF4α and CRP-202 mRNA levels than Large White animals (rp = 0.515 for Duroc, rp = 0.47 in Landrace and rp = 0.297 in Large White), in accordance with their higher frequency of the A allele in rs713631040, which creates a binding site for HNF4α (Figure 5, Table 3). A similar correlation pattern between HNF4α and CRP-202 was observed when all animals were classified according to the genotypes of rs713631040, with higher correlation levels observed for the AA genotype when compared to GA and GG.
Table 3 Correlation coefficients of CRP-202 mRNA expression with HNF4A and FOXA2 mRNA expression in liver by breed and rs713631040 (SSC4:90,743,532 bp) genotype.
Figure 5 Correlation plots between HNF4α and CRP-202 mRNA expression levels by breed and rs713631040 (SSC4:90,743,532 bp) alleles. (A) Scatter plot with regression lines, confidence intervals, concentration polygons, and correlation coefficients. (B) Scatter plot with marginal density plots.
To examine whether the pig proximal promoter is sufficient to mediate the induction of porcine CRP by IL-6 and IL-1β and whether the identified polymorphisms in its core promoter region may affect acute phase induction, luciferase reporter assays were carried out. Promoter activity was measured for two vectors containing different haplotypes (P1 and P2) of pig CRP promoter region in transfected HepG2 cells induced with IL6 and IL1-β. No substantial increase in luciferase activity (Figure 6) was observed in transfected cells with both CRP promoter constructs, suggesting that the promoter alone is insufficient for the acute phase induction of porcine CRP.
Figure 6 Relative luciferase activity of the transfected HepG2 cells treated with 10ng/ml IL-6 and 1ng/ml IL-1β for 24h after normalization with the cotransfected firefly luciferase activity. Vectors P1 and P2 are the constructs containing the different sequences of the promoter region alone, while vectors E1 and E2 have the respective enhancer haplotype inserted upstream of the promoter constructs P1 for the first haplotype and upstream of vector P2 for the second haplotype. Values with different superscript letters (a–c) indicate significant differences between groups (P-value ≤ 0.05), obtained by pairwise comparison by Fischer’s least significant difference (LSD) analysis adjusted with Bonferroni correction.
To further understand the functional contribution of the putative enhancer region associated to the expression of CRP, two sequences with different haplotypes (E1 and E2) of the enhancer were inserted upstream of CRP proximal promoter constructs. The inclusion of the enhancer sequences in the transfected vectors increased the induction of luciferase activity in HepG2 cells (Figure 6), revealing the involvement of this distal regulatory element in the acute phase induction of pig CRP by IL-6 and IL-1β. Furthermore, the vector containing the haplotype E1 (Ø – A – A – G) generated higher levels of luciferase activity than the rest (Figure 6), confirming the regulatory role of rs793561911 and rs713631040 on CRP gene expression.
CRP is known to be highly conserved between different species (48). Multiples studies in humans have described the regulatory molecular mechanisms controlling CRP gene expression in liver, as well as identified mutations associated with CRP blood levels and cardiovascular disease risk (6, 49, 50). Since pig represents an ideal model for human diseases (51, 52), in the present work we have delved into the genetic architecture and regulatory mechanisms involved in CRP gene expression in pigs. Furthermore, the identification of functional genetic variants associated to CRP blood levels could be valuable to improve the accuracy of genomic selection for immunocompetence in pigs.
Previous studies in humans and pigs have identified polymorphisms in the 3’UTR of CRP affecting serum levels of CRP (53–55). A GWAS study in our Duroc population also pointed out the polymorphism rs327446000 in the 3’UTR of CRP as the genetic variant most significantly associated with CRP serum levels. However, the haplotype-based approach maximized a region at 90.53-90.62 Mb in SSC4 as the most associated region with CRP serum levels. In addition, eGWAS analysis using 300 animals of different breeds identified a region at 86-93 Mb in SSC4 as the most associated with CRP mRNA expression levels in liver. Although we cannot discard the role of the 3’UTR region in the variation of CRP serum levels, our eGWAS and fine-mapping results pinpointed a genomic region located upstream of CRP gene associated with both mRNA expression and protein CRP levels.
A more detailed analysis of this region revealed the presence of a putative enhancer element conserved between human and cow species, and containing transcription factor binding sites for STAT3, C/EBP, NF-kB, HNF4α, OCT-1 FOXA1 and FOXA2. These transcription factors have been widely described as being required for the constitutive expression and/or acute phase induction of CRP (21, 22, 56–59). Remarkably, a recent study performed in humans identified an enhancer (E1) located 37.7 Kb upstream of the CRP promoter. Transcription factors STAT3, C/EBP-β, and USF1/2 appear to mediate the regulatory effects of E1 acting in conjunction with CRP proximal promoter for the acute phase induction by IL-6 and IL-1β of human CRP (16, 25, 26, 60). Furthermore, the constitutive expression of human CRP at the basal state seems to be mediated by promoter binding of transcription factors such as HNF-1, HNF-3 and OCT-1 (21, 56). Comparative analysis between human, cattle and porcine CRP promoter sequences also revealed a high level of sequence conservation, with transcription factor binding sites for FOXA2, HNF-1 and STAT3 preserved in the three promoter regions. Furthermore, the porcine promoter sequence shared target sites with its bovine counterpart for C/EBP, c-Rel and p53 transcription factors, and, in different locations, with the human for C/EBP, p53, OCT-1 and FOXA1. It is worth noting that the identified OCT-1 binding site conserved in pigs and humans was previously found by Voleti et al. in 2012 (22) as a modulator of CRP expression in humans by positional competition with other binding sites in the region.
Similar to the results previously reported by Wang et al. (26), the interaction of the pig distal enhancer element with the CRP proximal promoter was required for the acute phase induction of porcine CRP by IL-6 and IL-1β, suggesting an evolutionary conservation of regulatory mechanisms involved in CRP expression between pigs and humans. These results are in agreement with the similar functions of this protein in humans and pigs compared to mouse CRP (48).
Several polymorphisms located in putative binding-regions of transcription factors and associated to CRP mRNA expression and protein levels were identified in the proximal promoter and distal enhancer of porcine CRP. In the proximal promoter region, five out of 17 described genetic variants were disrupting putative binding-sites for C/EBP, FOXA1 and p53. C/EBP has been described as an important transcription factor activated by IL-6 and necessary for the induction of CRP expression (60, 61). However, we did not observe differences in luciferase activity in transfected HepG2 cells with vectors containing different promoter haplotypes after cytokine stimulation, suggesting that the allelic variation in these putative C/EBP binding sites did not have a substantial effect in the expression of porcine CRP. By contrast, among the seven polymorphisms found in the porcine enhancer region, rs793561911, rs713631040 and rs338992142 were located within putative binding sites for HNF4α and FOXA2, and potentially disrupting their regulatory effect. In fact, our luciferase assay showed a significant increase in luciferase activity in HEPG2 cells transfected with the enhancer haplotype that conserved the HNF4α binding sites (E1), which is in accordance with the correlation observed between CRP and HNF4α mRNA expression levels in liver.
Hepatocyte nuclear factor 4 alpha (HNF4α) encodes for a protein that controls the expression of several hepatic genes, HNF1α among them, and plays a role in liver development (62, 63). Sucajtys−Szulc et al. (19) revealed a coordinated upregulation of both hepatic nuclear factors, as well as IL-6 and CRP in livers of rats affected with either chronic renal failure or lipopolysaccharide-induced inflammation.
In the light of the above, our results describe for the first time the role of a distal enhancer in the acute phase expression of porcine CRP. Our analysis on CRP serum levels was limited to a closed commercial Duroc line, which is reflected in a high linkage disequilibrium. A larger sample size including other pig breeds and commercial lines would reduce the presence of large associated blocks and allow the identification of the causal implicated variants. Further functional analyses are warranted to better understand the regulatory mechanisms involved in CRP expression as well as to locate the causal mutation(s).
Finally, taking into account the strong similarities between porcine and human CRP regulation, this work improves the understanding of the complex mechanisms governing CRP expression in both species and reiterates the advantages of using the pig as a biomedical model for inflammation and cardiovascular diseases in humans. In addition, the identified functional polymorphisms can be used in pig breeding programs to improve the immunocompetence profile of the herd.
The datasets presented in this study can be found in online repositories. The names of the repository/repositories and accession number(s) can be found below: https://data.faang.org/, PRJEB58030 and PRJEB58031.
The animal study was approved by Institut de Recerca i Tecnologia Agroalimentàries (IRTA) Ethical Committee. The study was conducted in accordance with the local legislation and institutional requirements.
MB designed the study. MB, M-JM and MCAMB supervised the generation of the material animal. MB, OG-R, YR-C and RQ performed the sampling. MB, OG-R, TJ-J and CH-B carried out the laboratory analyses. TJ-J, CH-B, DC-P, AE-C, MB and RQ analysed the data. CH-B and MB interpreted the results and wrote the manuscript. All authors contributed to the article and approved the submitted version.
The authors declare financial support was received for the research, authorship, and/or publication of this article. The study was funded by grants AGL2016-75432-R and PID2020-112677RB-C21 awarded by MCIN/AEI/10.13039/501100011033 and GENE-SWitCH project (https://www.gene-switch.eu), which is funded by the European Union’s Horizon 2020 Research and Innovation Programme under the grant agreement n°817998. T. Jové-Juncà was funded with an IRTA fellowship (CPI1221) and C. Hernández-Banqué was supported by a FPI grant (PRE2021-097825) granted by the Spanish Ministry of Science and Innovation. YR-C was financially supported by a Ramon y Cajal contract (RYC2019-027244-I) from the Spanish Ministry of Science and Innovation. The authors are part to a Consolidated Research Group AGAUR, with the reference 2021-SGR-01552.
We gratefully acknowledge the technical staff from IRTA and Selección Batallé S.A. for their collaboration in the experimental protocols at farm and slaughterhouse.
Author MCAMB was employed by the company Hendrix Genetics.
The remaining authors declare that the research was conducted in the absence of any commercial or financial relationships that could be construed as a potential conflict of interest.
All claims expressed in this article are solely those of the authors and do not necessarily represent those of their affiliated organizations, or those of the publisher, the editors and the reviewers. Any product that may be evaluated in this article, or claim that may be made by its manufacturer, is not guaranteed or endorsed by the publisher.
The Supplementary Material for this article can be found online at: https://www.frontiersin.org/articles/10.3389/fimmu.2023.1250942/full#supplementary-material
1. Szalai AJ. The biological functions of C-reactive protein. Vascul Pharmacol (2002) 39(3):105–7. doi: 10.1016/S1537-1891(02)00294-X
2. Sproston NR, Ashworth JJ. Role of C-reactive protein at sites of inflammation and infection. Front Immunol (2018) 9(APR):1–11. doi: 10.3389/fimmu.2018.00754
3. Yao ZY, Zhang Y, Wu HB. Regulation of C-reactive protein conformation in inflammation. Inflammation Res (2019) 68(10):815–23. doi: 10.1007/s00011-019-01269-1
4. Rajab IM, Hart PC, Potempa LA. How C-reactive protein structural isoforms with distinctive bioactivities affect disease progression. Front Immunol (2020) 11. doi: 10.3389/fimmu.2020.02126
5. Ullah N, Wu Y. Regulation of conformational changes in C-reactive protein alters its bioactivity. Cell Biochem Biophysics (2022) 80:595–608. doi: 10.1007/s12013-022-01089-x
6. Boncler M, Wu Y, Watala C. The multiple faces of c-reactive protein-physiological and pathophysiological implications in cardiovascular disease. Molecules (2019) 24(11). doi: 10.3390/molecules24112062
7. Pepys MB, Hirschfield GM, Tennent GA, Gallimore JR, Kahan MC, Bellotti V, et al. Targeting C-reactive protein for the treatment of cardiovascular disease. Nature (2006) 440(7088):1217–21. doi: 10.1038/nature04672
8. Muthanna FM, Ibrahim HK, Al-Awkally NAM, Yousuf A. C-reactive protein in patients with COVID-19. Int J Health Sci (Qassim) (2022) 6:1610–20. doi: 10.53730/ijhs.v6nS5.8920
9. Möller K, Ostermann AI, Rund K, Thoms S, Blume C, Stahl F, et al. Influence of weight reduction on blood levels of C-reactive protein, tumor necrosis factor-α, interleukin-6, and oxylipins in obese subjects. Prostaglandins Leukot Essent Fatty Acids (2016) 106:39–49. doi: 10.1016/j.plefa.2015.12.001
10. Gutiérrez AM, Montes A, Gutiérrez-Panizo C, Fuentes P, de la Cruz-Sánchez E. Gender influence on the salivary protein profile of finishing pigs. J Proteomics (2018) 178(December 2017):107–13. doi: 10.1016/j.jprot.2017.11.023
11. Danik JS, Ridker PM. Genetic determinants of C-reactive protein. Curr Atheroscler Rep (2007) 9(3):195–203. doi: 10.1007/s11883-007-0019-2
12. Reiner AP, Barber MJ, Guan Y, Ridker PM, Lange LA, Chasman DII, et al. Polymorphisms of the HNF1A gene encoding hepatocyte nuclear factor-1α are associated with C-reactive protein. Am J Hum Genet (2008) 82(5):1193–201. doi: 10.1016/j.ajhg.2008.03.017
13. Ley SH, Hegele RA, Connelly PW, Harris SB, Mamakeesick M, Cao H, et al. Assessing the association of the HNF1A G319S variant with C-reactive protein in Aboriginal Canadians: A population-based epidemiological study. Cardiovasc Diabetol (2010) 9:1–6. doi: 10.1186/1475-2840-9-39
14. Ballester M, Ramayo-Caldas Y, González-Rodríguez O, Pascual M, Reixach J, Díaz M, et al. Genetic parameters and associated genomic regions for global immunocompetence and other health-related traits in pigs. Sci Rep (2020) 10(1). doi: 10.1038/s41598-020-75417-7
15. Agrawal A, Samols D, Kushner I. Transcription factor c-Rel enhances C-reactive protein expression by facilitating the binding of C/EBPbeta to the promoter. Mol Immunol (2003) 40(6):373–80. doi: 10.1016/S0161-5890(03)00148-2
16. Nishikawa T, Hagihara K, Serada S, Isobe T, Matsumura A, Song J, et al. Transcriptional complex formation of c-fos, STAT3, and hepatocyte NF-1α Is essential for cytokine-driven C-reactive protein gene expression. J Immunol (2008) 180(5):3492–501. doi: 10.4049/jimmunol.180.5.3492
17. Armendariz AD, Krauss RM. Hepatic nuclear factor 1-α: Inflammation, genetics, and atherosclerosis. Curr Opin Lipidol (2009) 20(2):106–11. doi: 10.1097/MOL.0b013e3283295ee9
18. Thirumalai A. Regulation of C-reactive protein gene expression and function. Thirumalai, Avinash East Tennessee State University (2014).
19. Sucajtys-Szulc E, Debska-Slizien A, Rutkowski B, Milczarek R, Pelikant-Malecka I, Sledzinski T, et al. Hepatocyte nuclear factors as possible C-reactive protein transcriptional inducer in the liver and white adipose tissue of rats with experimental chronic renal failure. Mol Cell Biochem (2018) 446(1–2):11–23. doi: 10.1007/s11010-018-3268-1
20. Li SP, Goldman ND. Regulation of human C-reactive protein gene expression by two synergistic IL-6 responsive elements. Biochemistry (1996) 35(28):9060–8. doi: 10.1021/bi953033d
21. Voleti B, Agrawal A. Regulation of basal and induced expression of C-reactive protein through an overlapping element for OCT-1 and NF-κB on the proximal promoter. J Immunol (2005) 175:3386–90. doi: 10.1021/bi953033d
22. Voleti B, Hammond DJ, Thirumalai A, Agrawal A. Oct-1 acts as a transcriptional repressor on the C-reactive protein promoter. Mol Immunol (2012) 52(3–4):242–8. doi: 10.1016/j.molimm.2012.06.005
23. Szalai AJ, van Ginkel FW, Wang Y, McGhee JR, Volanakis JE. Complement-dependent acute-phase expression of C-reactive protein and serum amyloid P-component. J Immunol (2000) 165(2):1030–5. doi: 10.4049/jimmunol.165.2.1030
24. Kramer F, Torzewski J, Kamenz J, Veit K, Hombach V, Dedio J, et al. Interleukin-1β stimulates acute phase response and C-reactive protein synthesis by inducing an NFκB- and C/EBPβ-dependent autocrine interleukin-6 loop. Mol Immunol (2008) 45(9):2678–89. doi: 10.1016/j.molimm.2007.12.017
25. Ngwa DN, Pathak A, Agrawal A. IL-6 regulates induction of C-reactive protein gene expression by activating STAT3 isoforms. Mol Immunol (2022) 146:50–6. doi: 10.1016/j.molimm.2022.04.003
26. Wang MY, Zhang CM, Zhou HH, Ge ZB, Su CC, Lou ZH, et al. Identification of a distal enhancer that determines the expression pattern of acute phase marker C-reactive protein. J Biol Chem (2022) 298(8). doi: 10.1016/j.jbc.2022.102160
27. Edfors-Lilja I, Wattrang E, Marklund L, Moller M, Andersson-Eklund L, Andersson L, et al. Mapping quantitative trait loci for immune capacity in the pig. J Immunol (1998) 161(2):829–35. doi: 10.4049/jimmunol.161.2.829
28. Millet S, Maertens L. The European ban on antibiotic growth promoters in animal feed: From challenges to opportunities. Veterinary J Vet J (2011) 187:143–4. doi: 10.1016/j.tvjl.2010.05.001
29. Merks JWM, Mathur PK, Knol EF. New phenotypes for new breeding goals in pigs. Animal (2012) 6(4):535–43. doi: 10.1017/S1751731111002266
30. Nowakiewicz A, Zięba P, Gnat S, Matuszewski Ł. Last call for replacement of antimicrobials in animal production: modern challenges, opportunities, and potential solutions. Antibiotics (Basel) (2020) 9(12):1–21. doi: 10.3390/antibiotics9120883
31. Purcell S, Neale B, Todd-Brown K, Thomas L, Ferreira MAR, Bender D, et al. PLINK: A tool set for whole-genome association and population-based linkage analyses. Am J Hum Genet (2007) 81(3):559–75. doi: 10.1086/519795
32. Crespo-Piazuelo D, Acloque H, González-Rodríguez O, Mongellaz M, Mercat MJ, Bink MCAM, et al. Identification of transcriptional regulatory variants in pig duodenum, liver, and muscle tissues. Gigascience (2023) 12:1–14. doi: 10.1093/gigascience/giad042
33. Li H. Aligning sequence reads, clone sequences and assembly contigs with BWA-MEM. arXiv (2013). doi: 10.48550/arXiv.1303.3997
34. McKenna A, Hanna M, Banks E, Sivachenko A, Cibulskis K, Kernytsky A, et al. The genome analysis toolkit: A MapReduce framework for analyzing next-generation DNA sequencing data. Genome Res (2010) 20(9):1297–303. doi: 10.1101/gr.107524.110
35. Dobin A, Davis CA, Schlesinger F, Drenkow J, Zaleski C, Jha S, et al. STAR: Ultrafast universal RNA-seq aligner. Bioinformatics (2013) 29(1):15–21. doi: 10.1093/bioinformatics/bts635
36. Li B, Dewey CN. RSEM: Accurate transcript quantification from RNA-Seq data with or without a reference genome. BMC Bioinf (2011) 12. doi: 10.1186/1471-2105-12-323
37. McLaren W, Gil L, Hunt SE, Riat HS, Ritchie GRS, Thormann A, et al. The ensembl variant effect predictor. Journal Genome Biology (2016) 17(1):. doi: 10.1186/s13059-016-0974-4
38. Corpet F. Multiple sequence alignment with hierarchical clustering. Nucleic Acids Res (1988) 16(22):10881–90. doi: 10.1093/nar/16.22.10881
39. Lee C, Huang CH. LASAGNA-search: An integrated web tool for transcription factor binding site search and visualization. Biotechniques (2013) 54(3):141–53. doi: 10.2144/000113999
40. Benjamini Y, Hochberg Y. Controlling the false discovery rate: A practical and powerful approach to multiple testing. J R Stat Society: Ser B (Methodological) (1995) 57(1):289–300. doi: 10.1111/j.2517-6161.1995.tb02031.x
41. Yang J, Lee SH, Goddard ME, Visscher PM. GCTA: A tool for genome-wide complex trait analysis. Am J Hum Genet (2011) 88(1):76–82. doi: 10.1016/j.ajhg.2010.11.011
42. Yang J, Benyamin B, McEvoy BP, Gordon S, Henders AK, Nyholt DR, et al. Common SNPs explain a large proportion of the heritability for human height. Nat Genet (2010) 42(7):565–9. doi: 10.1038/ng.608
43. Turner S D. qqman: an R package for visualizing GWAS results using Q-Q and manhattan plots. J Open Source Softw (2018) 3(25):731. doi: 10.21105/joss.00731
44. Druet T, Georges M. A hidden Markov model combining linkage and linkage disequilibrium information for haplotype reconstruction and quantitative trait locus fine mapping. Genetics (2010) 184(3):789–98. doi: 10.1534/genetics.109.108431
45. Pérez-Enciso M, Misztal I. Qxpak.5: Old mixed model solutions for new genomics problems. BMC Bioinf (2011) 12. doi: 10.1186/1471-2105-12-202
46. Bonferroni C. Teoria statistica delle classi e calcolo delle probabilita. Pubblicazioni del R Istituto Superiore di Sci Economiche e Commericiali di Firenze (1936) 8:3–62.
47. Salazar J, Martínez MS, Chávez-Castillo M, Núñez V, Añez R, Torres Y, et al. C-reactive protein: an in-depth look into structure, function, and regulation. Int Sch Res Notices (2014) 2014:653045. doi: 10.1155/2014/653045
48. Pathak A, Agrawal A. Evolution of C-reactive protein. Front Immunol (2019) 10. doi: 10.3389/fimmu.2019.00943
49. Hage FG, Szalai AJ. C-reactive protein gene polymorphisms, C-reactive protein blood levels, and cardiovascular disease risk. . J Am Coll Cardiol (2007) 50(12):1115–22. doi: 10.1016/j.jacc.2007.06.012
50. Carlson CS, Aldred SF, Lee PK, Tracy RP, Schwartz SM, Rieder M, et al. Polymorphisms within the C-reactive protein (CRP) promoter region are associated with plasma CRP levels. Am J Hum Genet (2008) 82(1):251. doi: 10.1016/j.ajhg.2007.12.007
51. Bassols A, Costa C, Eckersall PD, Osada J, Sabrià J, Tibau J. The pig as an animal model for human pathologies: A proteomics perspective. Proteomics - Clin Applications. Wiley-VCH Verlag; (2014) 8:715–31. doi: 10.1002/prca.201300099
52. Pabst R. The pig as a model for immunology research. Cell Tissue Res (2020) 380:287–304. doi: 10.1007/s00441-020-03206-9
53. Brull DJ, Serrano N, Zito F, Jones L, Montgomery HE, Rumley A, et al. Human CRP gene polymorphism influences CRP levels: implications for the prediction and pathogenesis of coronary heart disease. Arterioscler Thromb Vasc Biol (2003) 23(11):2063–9. doi: 10.1161/01.ATV.0000084640.21712.9C
54. Łaszyn M, Sielawa H, Zyczko K. The relationship between crp gene polymorphism and the serum concentrations of C-reactive protein, total cholesterol and hdl cholesterol in suckling piglets. Ann Anim Sci (2013) 13(3):503–12. doi: 10.2478/aoas-2013-0033
55. Fransén K, Pettersson C, Hurtig-Wennlöf A. CRP levels are significantly associated with CRP genotype and estrogen use in The Lifestyle, Biomarker and Atherosclerosis (LBA) study. BMC Cardiovasc Disord (2022) 22(1). doi: 10.1186/s12872-022-02610-z
56. Toniatti C, Demartis A, Monaci P, Nicosia A, Ciliberto G. Synergistic trans-activation of the human C-reactive protein promoter by transcription factor HNF-1 binding at two distinct sites. EMBO J (1990) 9:4467–75. doi: 10.1002/j.1460-2075.1990.tb07897.x
57. Aghadi M, Elgendy R, Abdelalim EM. Loss of FOXA2 induces ER stress and hepatic steatosis and alters developmental gene expression in human iPSC-derived hepatocytes. Cell Death Dis (2022) 13(8). doi: 10.1038/s41419-022-05158-0
58. Heslop JA, Duncan SA. FoxA factors: the chromatin key and doorstop essential for liver development and function. Genes Dev (2020) 34:1003–4. doi: 10.1101/gad.340570.120
59. Bochkis IM, Shin S, Kaestner KH. Bile acid-induced inflammatory signaling in mice lacking Foxa2 in the liver leads to activation of mTOR and age-onset obesity. Mol Metab (2013) 2(4):447–56. doi: 10.1016/j.molmet.2013.08.005
60. Young DP, Kushner I, Samols D. Binding of C/EBPβ to the C-reactive protein (CRP) promoter in hep3B cells is associated with transcription of CRP mRNA. J Immunol (2008) 181(4):2420–7. doi: 10.4049/jimmunol.181.4.2420
61. Zhang SC, Wang MY, Feng JR, Chang Y, Ji SR, Wu Y. Reversible promoter methylation determines fluctuating expression of acute phase proteins. Elife (2020) 9:2420–7. doi: 10.7554/eLife.51317
62. Kyithar MP, Bonner C, Bacon S, Kilbride SM, Schmid J, Graf R, et al. Effects of hepatocyte nuclear factor-1A and -4A on pancreatic stone protein/regenerating protein and C-reactive protein gene expression: Implications for maturity-onset diabetes of the young. J Transl Med (2013) 11(1). doi: 10.1186/1479-5876-11-156
Keywords: C-reactive protein, fine-mapping, luciferase assays, enhancer, pig, RNA-seq
Citation: Hernández-Banqué C, Jové-Juncà T, Crespo-Piazuelo D, González-Rodríguez O, Ramayo-Caldas Y, Esteve-Codina A, Mercat M-J, Bink MCAM, Quintanilla R and Ballester M (2023) Mutations on a conserved distal enhancer in the porcine C-reactive protein gene impair its expression in liver. Front. Immunol. 14:1250942. doi: 10.3389/fimmu.2023.1250942
Received: 30 June 2023; Accepted: 28 August 2023;
Published: 14 September 2023.
Edited by:
Yi Wu, Xi’an Jiaotong University, ChinaReviewed by:
Hong Lei, The Affiliated Children’s Hospital of Xi’an Jiaotong University, ChinaCopyright © 2023 Hernández-Banqué, Jové-Juncà, Crespo-Piazuelo, González-Rodríguez, Ramayo-Caldas, Esteve-Codina, Mercat, Bink, Quintanilla and Ballester. This is an open-access article distributed under the terms of the Creative Commons Attribution License (CC BY). The use, distribution or reproduction in other forums is permitted, provided the original author(s) and the copyright owner(s) are credited and that the original publication in this journal is cited, in accordance with accepted academic practice. No use, distribution or reproduction is permitted which does not comply with these terms.
*Correspondence: Maria Ballester, bWFyaWEuYmFsbGVzdGVyQGlydGEuY2F0
Disclaimer: All claims expressed in this article are solely those of the authors and do not necessarily represent those of their affiliated organizations, or those of the publisher, the editors and the reviewers. Any product that may be evaluated in this article or claim that may be made by its manufacturer is not guaranteed or endorsed by the publisher.
Research integrity at Frontiers
Learn more about the work of our research integrity team to safeguard the quality of each article we publish.