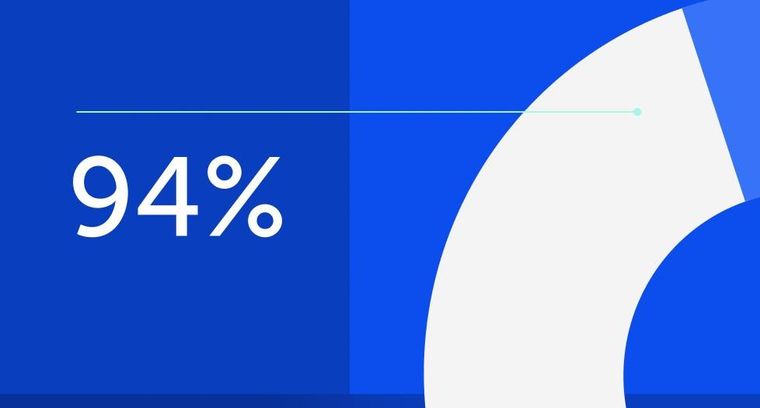
94% of researchers rate our articles as excellent or good
Learn more about the work of our research integrity team to safeguard the quality of each article we publish.
Find out more
REVIEW article
Front. Immunol., 06 September 2023
Sec. Cancer Immunity and Immunotherapy
Volume 14 - 2023 | https://doi.org/10.3389/fimmu.2023.1246682
This article is part of the Research TopicDelivery System of mRNA Cancer VaccineView all articles
Since the successful application of messenger RNA (mRNA) vaccines in preventing COVID-19, researchers have been striving to develop mRNA vaccines for clinical use, including those exploited for anti-tumor therapy. mRNA cancer vaccines have emerged as a promising novel approach to cancer immunotherapy, offering high specificity, better efficacy, and fewer side effects compared to traditional treatments. Multiple therapeutic mRNA cancer vaccines are being evaluated in preclinical and clinical trials, with promising early-phase results. However, the development of these vaccines faces various challenges, such as tumor heterogeneity, an immunosuppressive tumor microenvironment, and practical obstacles like vaccine administration methods and evaluation systems for clinical application. To address these challenges, we highlight recent advances from preclinical studies and clinical trials that provide insight into identifying obstacles associated with mRNA cancer vaccines and discuss potential strategies to overcome them. In the future, it is crucial to approach the development of mRNA cancer vaccines with caution and diligence while promoting innovation to overcome existing barriers. A delicate balance between opportunities and challenges will help guide the progress of this promising field towards its full potential.
Cancer is one of the tremendous challenges to human health globally and the leading cause of death. Based on the most recent statistical report, about 5370 new occurrences, and 1670 deaths each day are expected during 2023 in the United States (1). Due to high morbidity and mortality rates, tremendous efforts have been devoted to the search for anticancer modalities (2, 3). Although advances have been made in traditional therapeutic methods, including surgery, chemotherapy, and radiotherapy, the reliable cure is still limited. In recent years, immunotherapy has become an essential focus for cancer treatment, and multiple immune checkpoint inhibitors (ICIs) are approved as a therapy for cancer (4, 5). ICIs with apparent efficacy and low side effects raise new hope for cancer patients and lead to an increased awareness of the influential role of the immune system in the success of anticancer therapy (5, 6). However, limited benefit populations, drug resistance, and high costs remain significant concerns for ICIs (7). Hence, there is an imminent need to look for novel and effective ways to activate the immune system to fight tumors.
Recently, the successful applications of COVID-19 global pandemic offered a great opportunity for messenger RNA (mRNA)vaccines in antitumor therapy (8–10). Therapeutic mRNA cancer vaccines as a novel immunotherapeutic strategy, which aims to kill tumor cells via invoking antitumor adaptive immune responses, have attracted great attention (11, 12). Specifically, therapeutic mRNA cancer vaccines encode the key components for the process of the immune response, such as tumor-specific antigens (TSAs), tumor-associated antigens (TAAs), and immune modulatory factors, thus promoting immune activation to perform antitumor functions (13, 14). Therapeutic mRNA cancer vaccines have been reported to provide stronger cellular or humoral immunity than traditional inactivated pathogen or protein-based vaccines (15). Not only that, it has the advantages of low cost, rapid development, safety and flexibility, and potent immunogenicity (14). Currently, multiple therapeutic mRNA cancer vaccines are being evaluated in phase I/II trial trials with promising early-phase results (14, 16). In view of therapeutic mRNA cancer vaccines are undergoing early stages of clinical development, it is crucial to more fully understand current status and challenges of therapeutic mRNA cancer vaccines.
In this review, we evaluate the benefits of therapeutic mRNA cancer vaccines while providing a succinct overview of their classification and mechanism. Particularly, we highlight recent advancements from preclinical studies and clinical trials that identify the obstacles associated with the development of mRNA cancer vaccines and discuss potential strategies to overcome them.
mRNA vaccine has its origin in the 1990s when Wolff et al. found that mRNA could directly transfect muscle cells when injected in vivo, leading to the expression of the encoded related protein (16). However, its clinical application is restricted due to a lack of efficient synthesis, modifications, and delivery technologies (17). With recent breakthroughs and developments, the success of COVID-19 vaccines raised the hope for mRNA-based therapeutics for the treatment of various types of diseases, most notably in cancer.
The unique mechanism of action of the mRNA cancer vaccine has permitted the offer of advantages over conventional cancer therapy such as surgery, chemotherapy, and radiation therapy. Figure 1 demonstrates the advantages of mRNA vaccines for the treatment of cancer. A primary advantage of mRNA cancer vaccine is potent immunogenicity, which supports strong humoral immune response and the cell-mediated immune response, thus exhibiting a strong anti-tumour effect (18, 19). For metastatic tumours, which are not easily cured by surgery, the mRNA cancer vaccine was found to be effective as it can provoke a systemic immune response (20, 21). Apart from the above, mRNA cancer vaccines can build and maintain long-term immunological memory making preventing tumour recurrence possible (16). Several of mRNA cancer vaccines has been shown to have potent therapeutic efficacy in preclinical cancer models for primary tumor and metastases (6, 21).
Another significant advantage of mRNA cancer vaccines is the ability to support personalized therapies, which can increase therapeutic efficacy and minimize side effects (18, 22). mRNA vaccines with highly versatile allow mRNA sequences to be easily tailored to encode personalized antigens or cytokines of interest to against cancer (11, 22). mRNA cancer vaccine as nucleic acid vaccine, which can be translated immediately after it enters the cytoplasm and entry into the nucleus is not required (23). In contrast to DNA vaccines, mRNA cancer vaccines could avoid the requirement of nuclear localization and the risk of insertional mutagenesis associated with DNA (18). Furthermore, mRNA cancer vaccines do not carry the risks of accidental infection, making it an essential safety issue (24, 25). Of particular significance, mRNA production is faster, more flexible and less expensive compared to many current vaccination strategies (26). For example, mRNA vaccination enables the fast and secure production of vaccines during pandemics such as SARS-CoV2 (27). Moreover, mRNA vaccines can be manufactured without encountering the complex, time-consuming challenges associated with plasmid DNA, viral vectors, or recombinant proteins (16).
Figure 2 outlines the advantages and disadvantages of other types of cancer vaccines in comparison to mRNA cancer vaccine. DNA vaccines have certain advantages over mRNA vaccines in terms of storage and stability (28). DNA vaccines can be stored for a long time under regular freezing conditions and are relatively stable, with less degradation (28). However, DNA vaccines also have some drawbacks. DNA vaccines carry a potential risk of integration into the host genome, which may result in insertional mutagenesis (29). Additionally, DNA vaccines have relatively poorer immunogenicity, partly due to their inefficient delivery strategy (29). Compared to mRNA cancer vaccines, bacterial and viral vector vaccines elicit stronger immune responses and exhibit high stability under different storage conditions (15, 30). However, they also face drawbacks such as safety concerns associated with live vectors and potential impact of pre-existing immunity on effectiveness (15, 30). Peptide-based cancer vaccines offer advantages in terms of facile manufacturing and lower cost compared to mRNA cancer vaccines (31). However, they are inferior to mRNA cancer vaccines in terms of immunogenicity, which may result in relatively weaker vaccine efficacy, necessitating the implementation of additional measures to enhance immune response (32). Adjuvants are typically required to enhance immune response for peptide-based vaccines (31, 32). Compared to mRNA cancer vaccine, dendritic cell (DC) vaccines have an advantage because DC cells comprise a versatile cell type capable of engaging multiple facets of the immune system, making them more applicable to a broad range of cancers (33). However, the production of DC cancer vaccines requires complex operational steps and higher costs than mRNA cancer vaccine (33). Despite the inherent advantages and disadvantages of different types of cancer vaccines, mRNA cancer vaccines exhibit a distinctive amalgamation of characteristics that hold great promise for revolutionizing cancer treatment. However, to fully harness their potential, extensive research and clinical trials are required to optimize the efficacy, safety, and implementation of mRNA cancer vaccines.
Figure 2 The advantages and disadvantages of other types of cancer vaccines in comparison to mRNA cancer vaccine.
mRNA cancer vaccines hold immense potential as a personalized cancer therapy that harnesses the patient’s own immune system to specifically target and eliminate tumor cells (12). The progress in next-generation sequencing technologies has made it possible to quickly and cost-effectively compare tumor and normal sequences, serving as the initial stage for identifying cancer targets (34). Figure 3 shows the comprehensive design and production process of mRNA cancer vaccines, which encompasses sample acquisition, gene sequencing and target identification, mRNA sequence design, vaccine production, as well as administration of the final vaccine product. These vaccines can be categorized into three distinct types - those encoding TSAs, TAAs, and immunostimulatory factors– with each type involving internalization of the vaccine into cells, transcription of mRNA sequences encoding selected targets, delivery to immune cells, stimulation of the immune system, and promotion of tumor cell killing (Figure 4) (13, 35, 36). Each type of vaccine has a unique mechanism of action, characteristics, and potential advantages for cancer treatment.
Figure 3 The design and production process of mRNA cancer vaccines. The design and production process of mRNA cancer vaccines include sample acquisition, gene sequencing and target identification, mRNA sequence design, vaccine production, as well as administration of the final vaccine product.
Figure 4 Mechanism of action of mRNA cancer vaccines. The mechanism of action of mRNA cancer vaccines involving internalization of the vaccine into cells, transcription of mRNA sequences encoding selected targets, delivery to immune cells, stimulation of the immune system, and promotion of tumor cell killing.
mRNA cancer vaccines encoding TSAs constitute a promising form of personalized cancer therapy, designed to selectively target and eliminate tumor cells while minimizing damage to healthy cells (37). The presence of TSAs is restricted to tumor cells, thereby supporting the generation of specific immune responses against individual tumor antigens in patients (38). This specificity grants mRNA cancer vaccines encoding TSAs several advantages over traditional anti-cancer therapy, including greater efficacy and reduced toxicity (39). Furthermore, these vaccines are highly immunogenic and effective in stimulating robust T cell responses, as the selected targets are specific to the tumor and less likely to have been eliminated during the development of immune self-tolerance (40). Notably, mRNA cancer vaccines encoding TSAs can be designed to target various types of mutations, including single nucleotide variants and indels resulting in frameshift mutations that may alter protein function. Once synthesized, these mRNA sequences are delivered to the patient’s immune cells either in vitro or in vivo using lipid nanoparticle delivery systems or electroporation techniques (41–43). This ensures that the patient’s immune cells are equipped with the necessary tools to identify and destroy cancer cells.
TAAs, which include cancer or germline genes and lineage-specific differentiation markers, have emerged as attractive targets for vaccine development (26). Among the promising strategies in cancer immunotherapy are mRNA cancer vaccines encoding TAAs (44). These vaccines are designed to stimulate an immune response against proteins expressed by both cancer cells and normal cells (13). The principle behind mRNA cancer vaccines encoding TAAs is to induce a specific immune response against antigens that are overexpressed on the surface of cancer cells relative to normal cells. The process involves identifying the TAAs expressed by the patient’s tumor cells and designing mRNA sequences that encode those antigens. Subsequently, the mRNA is delivered into immune cells, translated and processed into antigen-major histocombatibility complex (MHC) class I/II complexes and then presented on the surface of these cells. This presentation triggers an immune response specifically targeting cells expressing these antigens. Importantly, mRNA cancer vaccines encoding TAAs offer significant advantages, especially in treating certain types of cancer such as lung cancer or melanoma, which exhibit elevated levels of TAAs expression (13).
Immune-regulating factors are molecules capable of either stimulating or suppressing specific immune cell functions, inclusive of cytokines, co-stimulatory ligands and receptors (45). The mRNA-encoded immunostimulatory factors vaccine represents a novel class of vaccines that utilizes mRNA as a vehicle for encoding immune-regulating factors, ultimately augmenting the immune response against cancer cells. The fundamental principle that underlies the design of this vaccine is to increase the concentration of immune-regulating factors, thereby promoting immune system function and bolstering defense against cancer cells. The mRNA vaccine encoding immune stimulatory factors can simultaneously introduce encoded sequences of multiple immune stimulatory factors, activating and enhancing immune system responses through various pathways. In recent studies, the most commonly used cytokines include IL-2, IL-12 and OX40L (46). These cytokines have been shown to stimulate T-cell proliferation and enhance the anti-tumor immune response (47–49). Furthermore, the mRNA vaccine encoding immune stimulatory factors can serve as an adjuvant for the mRNA vaccine encoding TAAs. By using the mRNA encoding TAAs together with the mRNA encoding immune stimulatory factors, the immune activity and anti-tumor effects of the TAAs vaccine can be enhanced. The presence of immune stimulatory factors can augment the immune response elicited by the mRNA encoding TAAs, further facilitating effective recognition and targeting of tumor cells by the immune system. A recent prominent vaccine of interest is ECI-006, which is a combination mRNA cancer vaccine comprising TriMix (mRNA encoding DC activation molecules CD40L, CD70, and caTLR4) and mRNA encoding TAAs (tyrosinase, gp100, MAGE A3, MAGE C2, and PRAME). This innovative vaccine holds promise in boosting the immune response against cancer cells, making it a significant focus of current research.
Both preclinical and clinical evidence have shown that the use of mRNA for prophylaxis and therapy has potential in preventing infectious diseases and treating cancers (50). Additionally, mRNA vaccines have demonstrated a safe and well-tolerated profile in both animal models and human trials (51). As of April 30th, 2023, ClinicalTrials.gov reports that 35 clinical trials are actively assessing the safety and efficacy of mRNA cancer vaccines for select cancer types (Table 1). These trials comprise preliminary investigations into the pharmacological, dosing, and immunogenic features of mRNA vaccines, as well as larger-scale evaluations of their potential to mitigate tumor recurrence or enhance survival rates. However, it is noteworthy that these studies remain in their initial phases, and additional research and validation will be crucial to corroborate their effectiveness.
Table 1 Characteristics of ClinicalTrials.gov registered mRNA cancer vaccines, including those encoding TSAs, TAAs, and immunostimulatory factors.
Table 1 presents a summary of registered clinical trials based on clinicaltrials.gov for mRNA cancer vaccines encoding TSAs. One of these vaccines, mRNA-4157, is a personalized mRNA vaccine that has the capacity to encode up to 34 antigens and is currently being assessed for its effectiveness in treating melanoma (NCT03897881). Results from the phase IIb KEYNOTE-942 trial indicate that mRNA-4157/V940 combined with pembrolizumab could be a potential adjuvant therapy for melanoma, as patients receiving this combination had a significant reduction in disease recurrence risk compared to those who only received PD-1 inhibitor, showing promising results (52). In a phase 1 clinical trial, autogene cevumeran (BNT 122) containing up to 20 neoantigens was tested for its ability to stimulate immunity against neoantigens in patients with resected pancreas ductal adenocarcinoma (PDAC). The results revealed that patients who responded to the vaccine exhibited a longer recurrence-free survival than non-responders at an early median follow-up of 15 months, suggesting that vaccine-induced neoantigen-specific immunity may be associated with improved outcomes in PDAC (53). However, evidence for the effectiveness of mRNA-4650 in treating gastrointestinal cancer appears to be discouraging. A study by Cafri et al. evaluated the immunogenicity and clinical efficacy of mRNA-4650 and only observed an increase in the frequency of cancer-specific T cells, but no clinical benefit (22).
Currently, several institutions such as BioNTech and Moderna have joined forces in the pursuit of developing personalized mRNA cancer vaccines, with numerous clinical trials currently underway. Despite having a theoretically proven efficacy, these vaccines face obstacles in areas such as design, production, and cost. Thus, additional optimization efforts are necessary to overcome these challenges. Furthermore, further preclinical and clinical trials are indispensable in validating the effectiveness of personalized mRNA cancer vaccines. These trials can identify opportunities for improvement and expedite the translation of this innovative approach from bench to bedside, ultimately benefiting cancer patients.
Fifteen clinical trials are currently in progress to assess mRNA cancer vaccines that encode TAAs, as demonstrated in Table 1. The BNT111 vaccine developed by BioNTech is an example of an mRNA cancer vaccine that encodes four melanoma-associated antigens (MAAs) including New York esophageal squamous cell carcinoma 1 (NY-ESO-1), melanoma-associated antigen A3 (MAGE-A3), tyrosinase, and transmembrane phosphatase with tensin homology (TPTE) (44). These RNAs are encapsulated in liposomes and administered intravenously to patients. In a phase I trial, this vaccine alone and in combination with ICIs induced durable objective responses and had a favorable safety profile among patients with advanced melanoma (44). Currently, a phase II trial is underway evaluating the vaccine candidate in combination with the anti-PD-1 antibody cemiplimab for patients with unresectable stage III or stage IV melanoma who are refractory to or have relapsed after anti-PD-1 therapy. These findings suggest that the BNT111 vaccine holds great promise as a treatment option for melanoma and may provide new hope for patients with advanced forms of the disease.
mRNA-5671 is a tetra-valent vaccine that has been formulated with lipid nanoparticle (LNP) technology and is based on messenger RNA (36). This innovative vaccine targets four of the most frequent KRAS mutations - G12D, G13D, G12C, and G12V (36). Pre-clinical investigations have indicated a substantial improvement in CD8 T cell responses to KRAS antigens post-immunization with mRNA encoding KRAS mutations (36). In a phase I trial, patients suffering from advanced or metastatic NSCLC, colorectal cancer, or pancreatic adenocarcinoma, and having KRAS mutations, are being enrolled to determine the efficacy of mRNA-5671 with or without pembrolizumab (NCT03948763).
A phase 1/2 clinical trial (NCT04382898) is currently underway to evaluate the effectiveness of the cancer vaccine BNT112. This vaccine encodes five different tumour-associated antigens and is being administered alone or in combination with cemiplimab to patients with metastatic castration-resistant prostate cancer. Another trial is a randomised phase 2 study (NCT04534205) evaluating the anti-human papillomavirus (HPV)-16-derived oncoprotein-encoding mRNA BNT113 vaccine in HPV16-positive, PD-L1-positive head and neck squamous cell carcinoma. BNT113 is also being tested in a two-arm phase 1/2 vaccine dose-escalation study (NCT03418480) for patients with previously treated or advanced HPV16-positive head and neck squamous cell carcinoma. Another phase 1 study (NCT04163094) is being conducted to evaluate the BNT115 that encodes ovarian-specific tumour-associated antigens. This vaccine is being administered both before and in combination with adjuvant and neoadjuvant chemotherapies to patients with ovarian cancer. Finally, a fifth clinical trial (NCT05142189) is evaluating the FixVac vaccine BNT116 in combination with cemiplimab or docetaxel in a phase 1 study for patients with advanced or metastatic non-small cell lung cancer.
The profound potential of mRNA cancer vaccines stems from their ability to encode a wide range of proteins, including immunostimulants that can modify the tumor immune microenvironment (TME) and enhance the efficacy of immune checkpoint inhibitors (51). This promising avenue of mRNA vaccine research has spurred clinical trials of cytokine-encoding mRNA products by BioNTech and Moderna, with highly encouraging results (13, 54). For instance, intratumorally administered mRNA-2416 produced by Moderna, which encodes OX40L, demonstrated safety and tolerability in a phase I trial and elicited broad proinflammatory activity with desirable changes in the TME (54). These findings provide critical support for its further investigation in combination therapy with anti-PD-L1 inhibitor durvalumab in solid tumors. Similarly, co-administration of mRNA-2752 encoding OX40L/IL23/IL36g with durvalumab in a dose escalation study (NCT03739931) exhibited antitumor effects, validating the potential of mRNA cancer vaccines as a therapeutic modality. Furthermore, BioNTech’s BNT131 (SAR441000), which encodes IL-12sc, IL-15sushi, IFN-α for intratumoral injection, is being tested as monotherapy and in combination with cemiplimab for patients with advanced solid tumors to alter the TME. Several other mRNA products have also shown promise, such as ECI-006, a combination of TriMix and melanoma-specific TAAs administered intranodularly and being tested in a phase 1 study of resected melanoma (NCT03394937); and MEDI1191, an immunomodulatory fusion protein containing IL-12α and IL-12β subunits developed for intratumoral injection (55). While only seven product candidates are currently undergoing clinical trials, the results thus far demonstrate the immense potential of mRNA cancer vaccines in improving cancer immunotherapy outcomes (Table 1). With these promising results, further research is needed to determine the optimal administration route and maximize vaccine efficacy.
Numerous clinical trials are currently under development or in progress to assess the safety and efficacy of mRNA cancer vaccines (51). Despite promising results in preclinical and early-phase clinical trials, the successful translation of mRNA cancer vaccines into clinical practice faces several obstacles. These challenges include tumor heterogeneity, an immunosuppressive tumor microenvironment, optimal vaccine administration routes, and the identification of biomarkers to monitor treatment response.
The complexity of treatment decisions is enhanced by tumour heterogeneity, which can be divided into spatial and temporal heterogeneity (56). Temporal heterogeneity refers to the dynamic evolution of the genomes through the tumour progression course, whereas spatial heterogeneity refers to the phenomenon that a tumour is composed of subclones of different genetic backgrounds (57–59). This heterogeneity leads to variable responses to therapies among individuals with cancers of the same tumour subtype and is believed to be one of the major causes of progressive disease and failure of therapy (60, 61). Typically, only a small fraction of a specimen is sampled for mRNA cancer vaccine design, which is unlikely to provide full information on tumour gene profile, contributing to increased uncertainties for the clinical efficacy of mRNA cancer vaccine (11). Thus, tumour heterogeneity largely limits the efficacy of mRNA cancer vaccine and how to overcome tumor heterogeneity is a difficult challenge for its clinical application.
To overcome spatial heterogeneity in tumours, one strategy is to use tumour tissue multipoint sampling to identify differences between tumour regions and inform the design of personalised mRNA cancer vaccines. Another approach involves using mRNA cancer vaccines that target multiple antigens expressed across various tumour regions, thereby compensating for spatial heterogeneity. Meanwhile, monitoring disease progression and adjusting treatment plans accordingly may help to address temporal heterogeneity. However, these strategies not only increase the complexity of vaccine design and administration but also raise costs and increase treatment time for patients. Another promising method is the use of artificial intelligence algorithms such as MHC-binding prediction, quantification of mutated transcript expression, and clonality of the mutation to predict neoantigens based on tumour genomic data, then used to prioritize these mutations as vaccine candidates based on their likelihood to elicit a T cell response, which could improve the efficiency and accuracy of vaccine design and overcome the heterogeneity of tumour (62–64). However, to date, there is still limited information on artificial intelligence for mRNA cancer vaccine design. This is certainly an important and interesting area worthy of future investigations.
Tumour initiation, progression and maintenance depend highly on interactions between the tumour and the associated microenvironment (65). Tumour microenvironment refers to the surrounding microenvironment of tumour cells, including surrounding cells, signalling molecules, and extracellular matrix (66). The importance of the tumor microenvironment in cancer has been recognized since the late 1800s and then accumulating evidences suggested immunosuppressive TME not only promote immune evasion and tumor growth, but also lead to decrease the efficacy of immunotherapy (67–69). Immunosuppressive TME could decrease the efficacy of immunotherapy by inhibiting the function and activation of immune cells, such as T cells and natural killer cells, which are critical for attacking and eliminating cancer cells (14). Although mRNA cancer vaccine may have the capability to elicit a cellular immune response, the inhibitory tumor microenvironment can impede T cell infiltration into tumors and result in T cell exhaustion (50). As with many other immunotherapies, overcoming immunosuppressive TME is one of the most challenging and unsolved problems for mRNA cancer vaccine.
Combining mRNA cancer vaccines with other anti-cancer treatments, which is being constantly tried in cancer patients, can be an effective strategy for overcoming the immunosuppressive microenvironment. One commonly used combination therapy is to combine mRNA vaccines with immune checkpoint inhibitors, which can “release the brakes” on the immune system, allowing it to attack cancer cells more effectively (55, 70). Adoptive T-cell therapy, another type of immunotherapy, can also be used alongside mRNA vaccines. This innovative treatment involves extracting T-cells from a patient’s blood or tumor, modifying them in a laboratory to target specific cancer antigens, and then reintroducing them into the patient’s body. Combining these modified T-cells with mRNA vaccines can significantly enhance the immune response against cancer cells, thus improving the efficacy of this therapy (71). The combination of mRNA vaccines and radiotherapy has also shown promising therapeutic effects in preclinical and clinical models (72, 73). Overall, combining mRNA cancer vaccines with other anti-cancer treatments can be a powerful approach to overcoming the immunosuppressive microenvironment in tumors. Continued research and development of these combination therapies will be critical to improving outcomes for cancer patients.
Poor performance in any step of the mRNA delivery process would compromise the therapeutic efficacy, and administration routes should be a first-order consideration for the clinical usage of mRNA cancer vaccine (74, 75). The administration route of mRNA vaccines strongly influences the translation efficiency of the target protein and the distribution of mRNA cancer vaccine in vivo (16, 76). Currently, there is no consensus on the optimal route of administration for mRNA vaccines, although the SARS-CoV-2 mRNA vaccines that have received approval utilize intramuscular injection (50). Various routes, including intramuscular, subcutaneous, intranodal, intradermal, and intranasal vaccination, are actively being explored in the search for an optimal administration route for mRNA cancer vaccines (55). Each administration route for mRNA cancer vaccines has unique advantages and limitations. As depicted in Figure 5, the strengths and weaknesses of diverse mRNA cancer vaccines administration routes are presented.
Figure 5 The strengths and limitations inherent in a range of administration routes for mRNA cancer vaccines.
Intramuscular and intravenous injections are the two most frequently used administration routes in clinical trials for mRNA cancer vaccines (Table 1). Intramuscular administration is a widely used and feasible vaccination route that involves the direct injection of a vaccine into muscle tissue (14, 77). This method has demonstrated efficacy in inducing an immune response for mRNA cancer vaccines, being both easily executed and well-tolerated with the added benefit of offering flexible dosing options, while also causing minimal side effects at the injection site (78). Consequently, its use has become prevalent (14, 77, 78). Additionally, intravenous injection is the most frequently used direct administration route in current clinical trials for active therapeutic mRNA cancer vaccines (79). It has been shown to be safe, well-tolerated, and capable of inducing an immune response against cancer cells (80). Furthermore, it enables the vaccine to reach multiple lymphoid organs and allows for repeated dosing to maintain immunity over time (55, 80).
Intranasal administration of mRNA cancer vaccines can effectively deliver the vaccine to antigen-presenting cells in the peripheral lymph nodes (81, 82). However, intranodal injections provide a more direct route to reach lymphatic antigen-presenting cells (83). While both delivery methods have their advantages, they also face significant limitations. Intranasal administration may offer non-invasive delivery but is restricted by nasal cavity volume constraints (81, 82). Conversely, intranodal injections require specialized equipment and highly skilled personnel, and the injection volumes are small, which may not be sufficient for larger tumors or inducing a robust immune response (84). Accordingly, further research is necessary to optimize these methods for mRNA cancer vaccines.
Intratumoral injections of mRNA cancer vaccines, which can rapidly activate immune cells and minimize off-target, have been investigated in clinical and preclinical trials (85, 86). Although this approach aims to induce local inflammation with mRNA encoding immunostimulatory, its effectiveness is limited by the size and location of the tumor, which may restrict the amount of vaccine that can be delivered (87). Repeated injections may be required to maintain the immune response over time, and combining intratumoral injections with systemic delivery methods such as immune checkpoint inhibitors may offer a more comprehensive approach to treating cancer (55). Despite these limitations, intratumoral injections remain a promising area of research for directly interact with immune cells to activate an immune response mRNA cancer vaccine. Intradermal and subcutaneous injections are two common administration routes for mRNA cancer vaccines (74). Both methods allow regional antigen-presenting cells to easily process the mRNA, which is essential for eliciting an immune response against cancer cells (74). However, these administration routes often induce severe local injection-site reactions than intramuscular administration, which can negatively impact patient compliance and overall treatment efficacy (14, 74).
In view of the immense potential offered by various routes of administration for successful cancer therapy, it is of paramount importance to thoroughly assess the advantages and disadvantages of each vaccination route. Recently, investigators have utilized a non-invasive method involving a dual radionuclide near-infrared probe to track the spatiotemporal trafficking of the vaccine following intramuscular injection, offering essential guidance in precisely evaluating the dose, injection site, and biological distribution of the vaccine (88). In order to determine the most effective vaccination route and optimize vaccine efficacy, there remains a pressing need for novel approaches that can accurately monitor and analyze the spatiotemporal kinetics of vaccines (88). By carefully weighing the pros and cons of each administration route, we can identify the most promising strategies for delivering immunotherapies that can target cancer cells effectively while minimizing off-target effects. Furthermore, by leveraging advanced tools to track and analyze vaccine movement within the body, we can acquire critical insights into the factors that govern their biological activity and efficacy. Therefore, these innovative methods hold tremendous promise for advancing our understanding of how to optimize mRNA cancer vaccination routes and maximize the impact of cancer immunotherapy.
The introduction of mRNA cancer vaccines represents an exciting new frontier in cancer treatment, but also underscores the need for novel efficacy evaluation standards. Unlike traditional cancer treatments, such as chemotherapy, targeted therapy, and radiation therapy, which targeting the tumor cells directly and emphasizing tumor shrinkage as a successful response to treatment evaluated by radiological imaging, mRNA vaccines stimulate the immune system to produce an anti-tumor response indirectly that standard radiographic imaging techniques do not account for this unique mechanism of action and may not reflect the true clinical benefit of mRNA vaccines (89). mRNA cancer vaccines may lead to inflammatory reactions resulting in tumour swelling, which results in increased complexity of evaluating the efficacy of mRNA cancer vaccines (90). Moreover, cancer vaccines, particularly when used as monotherapy, may exhibit greater efficacy in cases of low disease burden and may not produce the striking radiographic responses typically observed with cytotoxic therapy (91). As a result, the development of cancer vaccines may encounter difficulties in demonstrating effectiveness when evaluated in late-stage disease using traditional assessment methods such as standard radiographic response evaluation criteria (92). Due to their highly individualized and customized nature, personalized mRNA cancer vaccines require different treatment plans and dosages tailored to each patient’s unique condition and cancer type (89). To meaningfully identify which mRNA cancer vaccines should advance beyond early phase trials and into larger phase III clinical trials, novel biomarkers need to establish that can accurately monitor the treatment response to these vaccines.
Quantitative measurement of immune cell responses is essential for establishing surrogate biomarkers of efficacy, especially for tumor antigen-specific T cell responses, which are critical in selecting optimal doses for cancer vaccine trials and have the potential to lead to tumor rejection. Multiple studies are evaluating the effectiveness of mRNA therapies by measuring immune response indicators, such as cytokines, chemokines, or immune cells in the blood. A variety of immune monitoring techniques, including flow cytometry analysis of cell populations’ phenotypes, functionalities, and activation status, are employed (93). Additionally, enzyme-linked immunospot (ELISPOT) assays measure cytokine release after antigen-specific immune responses, while peripheral cytokine profiling using enzyme-linked immunosorbent assay evaluates innate immune responses (93–95). Another method, tetramer analysis with MHC multimers loaded with antigen peptides, is used to measure antigen-specific CD8 T cells, and T cell receptor analysis through sequencing and polymerase chain reaction helps to elucidate the immune repertoire, including genetic arrangement and specificity. These assays are applied to immune cells in peripheral blood mononuclear cells acquired from patients to detect and describe T cell responses. Although ELISPOT is one of the most commonly used techniques to identify CD8 T cell responses to a given antigen, its quantitative output cannot determine the absolute number of antigen-specific T cells (94). It is noteworthy that currently available immunological assays, such as ELISPOT, flow cytometry-based multimer staining, and intracellular cytokine staining, have been found to exhibit technical inconsistencies across different laboratories (96). For example, one study reported inter-laboratory variations of up to 50% in ELISPOT (96). Standardized and harmonized procedures, from specimen banking to assay validation and result reporting, are thus necessary for successful clinical development.
Over the past few decades, single-cell RNA sequencing (scRNA-seq) technology has garnered significant attention as a novel tool in assessing the immunotherapy response of cancer patients (97). The scRNA-seq technology refers to a collection of techniques that enable the untargeted quantification of transcripts present in individual cells, facilitating the identification of cell types and states associated with immunotherapy response (97, 98). The scRNA-seq technology allows for the comprehensive analysis of cellular transcriptomes, facilitating the identification of distinct immune cell subsets and their gene expression patterns correlated with immunotherapy response (99). Furthermore, this technique provides insights into the dynamic changes within the tumor microenvironment during immunotherapy, aiding in the understanding of mechanisms underlying treatment response (99). Recently, multiple studies have reported utilized scRNA-seq to explore immune cell subset infiltration and regulation changes pre- and post-mRNA vaccine administration to understand therapeutic response and analyze underlying mechanisms to determine mRNA vaccine efficacy. With further advancements in technology and deeper applications, scRNA-seq technology holds the promise of providing valuable insights into mRNA cancer vaccine efficacy detection.
Recently, the circulating tumour DNA (ctDNA) test has the advantages of sensitivity, flexibility, repeatability, and safe gain much attention to help the evaluation of treatment responses, and the application of ctDNA detection in monitoring mRNA cancer vaccine therapeutic response is a worthwhile attempt (100). Accumulating evidence suggests that changes in ctDNA levels during treatment compared to baseline can serve as a valuable biomarker for stratifying patients as molecular responders or nonresponders and distinguishing those with a favorable prognosis, especially those displaying stable disease according to RECIST v1.1 criteria (101). Palmer et al. investigated the utility of ctDNA as a potential biomarker for monitoring therapeutic responses to mRNA cancer vaccines (70). Their study demonstrated that a decrease in ctDNA levels among patients undergoing treatment was positively correlated with prolonged overall survival, thereby underscoring ctDNA’s potential as a promising biomarker for predicting treatment response (70). The future exploitation of efficacy evaluation system integration of immunologic and radiologic endpoints, the establishment of biomarkers, and standardization of evaluation protocols is needed to develop in the future, which will be necessary to realize the full potential of this promising technology in the fight against cancer.
Altogether, mRNA cancer vaccines present a promising new approach to anticancer therapies with both opportunities and challenges. The highly personalized and specific nature of this technology offers tremendous potential for precision medicine in the fight against cancer. However, further clinical trials are necessary to fully establish the safety and efficacy of mRNA cancer vaccines and additional preclinical studies are warranted to explore the combined use of mRNA cancer vaccine and other anticancer therapies. Additionally, addressing issues such as tumoral heterogeneity, routes of administration and development of methods to assess the efficacy processes will be critical for advancing this technology toward meaningful clinical outcomes. With continued research and investment, mRNA cancer vaccines hold great promise as a transformative therapy for cancer patients.
BW and JL collected relevant literature and wrote the manuscript. BW and JY conceptualized the main structure of this review, and revised and validated the final version. JP, SX and BW contributed to the literature analysis and manuscript editing. All authors contributed to the article and approved the submitted version.
This work was supported by the the foundation of National Natural Science Foundation of China (81627901), the foundation of National Natural Science Foundation of China (81972863, 82030082 and 8220103505), the National Key Research and Development Projects of China (2018YFC1312201), Radiation Oncology Innovate Unit, Chinese Academy of Medical Sciences (2019RU071), the foundation of Natural Science Foundation of Shandong (ZR2022QH017) and the Academic Promotion Program of Shandong First Medical University (2019ZL002 ).
The authors declare that the research was conducted in the absence of any commercial or financial relationships that could be construed as a potential conflict of interest.
All claims expressed in this article are solely those of the authors and do not necessarily represent those of their affiliated organizations, or those of the publisher, the editors and the reviewers. Any product that may be evaluated in this article, or claim that may be made by its manufacturer, is not guaranteed or endorsed by the publisher.
1. Siegel RL, Miller KD, Wagle NS, Jemal A. Cancer statistics, 2023. CA Cancer J Clin (2023) 73(1):17–48. doi: 10.3322/caac.21763
2. Geldof T, Rawal S, Dyck WV, Huys I. Comparative and combined effectiveness of innovative therapies in cancer: a literature review. J Comp Eff Res (2019) 8(4):205–16. doi: 10.2217/cer-2018-0131
3. Olgen S. Overview on anticancer drug design and development. Curr Med Chem (2018) 25(15):1704–19. doi: 10.2174/0929867325666171129215610
4. Szeto GL, Finley SD. Integrative approaches to cancer immunotherapy. Trends Cancer (2019) 5(7):400–10. doi: 10.1016/j.trecan.2019.05.010
5. Finck A, Gill SI, June CH. Cancer immunotherapy comes of age and looks for maturity. Nat Commun (2020) 11(1):3325. doi: 10.1038/s41467-020-17140-5
6. Mellman I, Coukos G, Dranoff G. Cancer immunotherapy comes of age. Nature (2011) 480(7378):480–9. doi: 10.1038/nature10673
7. Hegde PS, Chen DS. Top 10 challenges in cancer immunotherapy. Immunity (2020) 52(1):17–35. doi: 10.1016/j.immuni.2019.12.011
8. Boni MF, Lemey P, Jiang X, Lam TT, Perry BW, Castoe TA, et al. Evolutionary origins of the SARS-CoV-2 sarbecovirus lineage responsible for the COVID-19 pandemic. Nat Microbiol (2020) 5(11):1408–17. doi: 10.1038/s41564-020-0771-4
9. Chakraborty C, Sharma AR, Bhattacharya M, Lee SS. From COVID-19 to cancer mRNA vaccines: moving from bench to clinic in the vaccine landscape. Front Immunol (2021) 12:679344. doi: 10.3389/fimmu.2021.679344
10. Pardi N, Hogan MJ, Porter FW, Weissman D. mRNA vaccines - a new era in vaccinology. Nat Rev Drug Discov (2018) 17(4):261–79. doi: 10.1038/nrd.2017.243
11. Bevers S, Kooijmans SAA, Van de Velde E, Evers MJW, Seghers S, Gitz-Francois J, et al. mRNA-LNP vaccines tuned for systemic immunization induce strong antitumor immunity by engaging splenic immune cells. Mol Ther (2022) 30(9):3078–94. doi: 10.1016/j.ymthe.2022.07.007
12. Miao L, Zhang Y, Huang L. mRNA vaccine for cancer immunotherapy. Mol Cancer (2021) 20(1):41. doi: 10.1186/s12943-021-01335-5
13. Deng Z, Tian Y, Song J, An G, Yang P. mRNA vaccines: the dawn of a new era of cancer immunotherapy. Front Immunol (2022) 13:887125. doi: 10.3389/fimmu.2022.887125
14. Lorentzen CL, Haanen JB, Met O, Svane IM. Clinical advances and ongoing trials on mRNA vaccines for cancer treatment. Lancet Oncol (2022) 23(10):e450–e8. doi: 10.1016/S1470-2045(22)00372-2
15. Le T, Sun C, Chang J, Zhang G, Yin X. mRNA vaccine development for emerging animal and zoonotic diseases. Viruses (2022) 14(2):401. doi: 10.3390/v14020401
16. Heine A, Juranek S, Brossart P. Clinical and immunological effects of mRNA vaccines in Malignant diseases. Mol Cancer (2021) 20(1):52. doi: 10.1186/s12943-021-01339-1
17. Dolgin E. The tangled history of mRNA vaccines. Nature (2021) 597(7876):318–24. doi: 10.1038/d41586-021-02483-w
18. Tendeloo V, Ponsaerts P, Berneman ZN. mRNA-based gene transfer as a tool for gene and cell therapy. Curr Opin Mol Ther (2007) 9(5):423–31.
19. Wolff JA. Direct gene transfer into mouse muscle in vivo. Sci (1990) 247(4949):1465–8. doi: 10.1126/science.1690918
20. Sittplangkoon C, Alameh MG, Weissman D, Lin PJC, Tam YK, Prompetchara E, et al. mRNA vaccine with unmodified uridine induces robust type I interferon-dependent anti-tumor immunity in a melanoma model. Front Immunol (2022) 13:983000. doi: 10.3389/fimmu.2022.983000
21. Chen J, Ye Z, Huang C, Qiu M, Song D, Li Y, et al. Lipid nanoparticle-mediated lymph node-targeting delivery of mRNA cancer vaccine elicits robust CD8(+) T cell response. Proc Natl Acad Sci U.S.A. (2022) 119(34):e2207841119. doi: 10.1073/pnas.2207841119
22. Cafri G, Gartner JJ, Zaks T, Hopson K, Levin N, Paria BC, et al. mRNA vaccine-induced neoantigen-specific T cell immunity in patients with gastrointestinal cancer. J Clin Invest (2020) 130(11):5976–88. doi: 10.1172/JCI134915
23. Zhuang X, Qi Y, Wang M, Yu N, Nan F, Zhang H, et al. mRNA vaccines encoding the HA protein of influenza A H1N1 virus delivered by cationic lipid nanoparticles induce protective immune responses in mice. Vaccines (Basel) (2020) 8(1):123. doi: 10.3390/vaccines8010123
24. Qin S, Tang X, Chen Y, Chen K, Fan N, Xiao W, et al. mRNA-based therapeutics: powerful and versatile tools to combat diseases. Signal Transduct Target Ther (2022) 7(1):166. doi: 10.1038/s41392-022-01007-w
25. Vormehr M, Schrors B, Boegel S, Lower M, Tureci O, Sahin U. Mutanome engineered RNA immunotherapy: towards patient-centered tumor vaccination. J Immunol Res (2015) 2015:595363. doi: 10.1155/2015/595363
26. Coulie PG, Van den Eynde BJ, van der Bruggen P, Boon T. Tumour antigens recognized by T lymphocytes: at the core of cancer immunotherapy. Nat Rev Cancer (2014) 14(2):135–46. doi: 10.1038/nrc3670
27. Sahin U, Muik A, Derhovanessian E, Vogler I, Kranz LM, Vormehr M, et al. COVID-19 vaccine BNT162b1 elicits human antibody and T(H)1 T cell responses. Nature (2020) 586(7830):594–9. doi: 10.1038/s41586-020-2814-7
28. Lopes A, Vandermeulen G, Preat V. Cancer DNA vaccines: current preclinical and clinical developments and future perspectives. J Exp Clin Cancer Res (2019) 38(1):146. doi: 10.1186/s13046-019-1154-7
29. Wu C, Duan Y, Gong S, Osterhoff G, Kallendrusch S, Schopow N. Identification of tumor antigens and immune subtypes for the development of mRNA vaccines and individualized immunotherapy in soft tissue sarcoma. Cancers (Basel) (2022) 14(2):448. doi: 10.3390/cancers14020448
30. Shanmugaraj B, Priya LB, Mahalakshmi B, Subbiah S, Hu RM, Velmurugan BK, et al. Bacterial and viral vectors as vaccine delivery vehicles for breast cancer therapy. Life Sci (2020) 250:117550. doi: 10.1016/j.lfs.2020.117550
31. Liu W, Tang H, Li L, Wang X, Yu Z, Li J. Peptide-based therapeutic cancer vaccine: Current trends in clinical application. Cell Prolif (2021) 54(5):e13025. doi: 10.1111/cpr.13025
32. Song Q, Zhang CD, Wu XH. Therapeutic cancer vaccines: From initial findings to prospects. Immunol Lett (2018) 196:11–21. doi: 10.1016/j.imlet.2018.01.011
33. Perez CR, De Palma M. Engineering dendritic cell vaccines to improve cancer immunotherapy. Nat Commun (2019) 10(1):5408. doi: 10.1038/s41467-019-13368-y
34. Li L, Goedegebuure P, Mardis ER, Ellis MJ, Zhang X, Herndon JM, et al. Cancer genome sequencing and its implications for personalized cancer vaccines. Cancers (Basel) (2011) 3(4):4191–211. doi: 10.3390/cancers3044191
35. Sahin U, Tureci O. Personalized vaccines for cancer immunotherapy. Science (2018) 359(6382):1355–60. doi: 10.1126/science.aar7112
36. Wei J, Hui AM. The paradigm shift in treatment from Covid-19 to oncology with mRNA vaccines. Cancer Treat Rev (2022) 107:102405. doi: 10.1016/j.ctrv.2022.102405
37. Shemesh CS, Hsu JC, Hosseini I, Shen BQ, Rotte A, Twomey P, et al. Personalized cancer vaccines: clinical landscape, challenges, and opportunities. Mol Ther (2021) 29(2):555–70. doi: 10.1016/j.ymthe.2020.09.038
38. Zhang X, Cui H, Zhang W, Li Z, Gao J. Engineered tumor cell-derived vaccines against cancer: The art of combating poison with poison. Bioact Mater (2023) 22:491–517. doi: 10.1016/j.bioactmat.2022.10.016
39. Peng M, Mo Y, Wang Y, Wu P, Zhang Y, Xiong F, et al. Neoantigen vaccine: an emerging tumor immunotherapy. Mol Cancer (2019) 18(1):128. doi: 10.1186/s12943-019-1055-6
40. Zhang X, Sharma PK, Peter Goedegebuure S, Gillanders WE. Personalized cancer vaccines: Targeting the cancer mutanome. Vaccine (2017) 35(7):1094–100. doi: 10.1016/j.vaccine.2016.05.073
41. Semple SC, Leone R, Barbosa CJ, Tam YK, Lin PJC. Lipid nanoparticle delivery systems to enable mRNA-based therapeutics. Pharmaceutics (2022) 14(2):398. doi: 10.3390/pharmaceutics14020398
42. Swetha K, Kotla NG, Tunki L, Jayaraj A, Bhargava SK, Hu H, et al. Recent advances in the lipid nanoparticle-mediated delivery of mRNA vaccines. Vaccines (Basel) (2023) 11(3):658. doi: 10.3390/vaccines11030658
43. Gerer KF, Hoyer S, Dorrie J, Schaft N. Electroporation of mRNA as universal technology platform to transfect a variety of primary cells with antigens and functional proteins. Methods Mol Biol (2017) 1499:165–78. doi: 10.1007/978-1-4939-6481-9_10
44. Sahin U, Oehm P, Derhovanessian E, Jabulowsky RA, Vormehr M, Gold M, et al. An RNA vaccine drives immunity in checkpoint-inhibitor-treated melanoma. Nature (2020) 585(7823):107–12. doi: 10.1038/s41586-020-2537-9
45. Beck JD, Reidenbach D, Salomon N, Sahin U, Tureci O, Vormehr M, et al. mRNA therapeutics in cancer immunotherapy. Mol Cancer (2021) 20(1):69. doi: 10.1186/s12943-021-01348-0
46. Yuan Y, Gao F, Chang Y, Zhao Q, He X. Advances of mRNA vaccine in tumor: a maze of opportunities and challenges. biomark Res (2023) 11(1):6. doi: 10.1186/s40364-023-00449-w
47. Hernandez R, Poder J, LaPorte KM, Malek TR. Engineering IL-2 for immunotherapy of autoimmunity and cancer. Nat Rev Immunol (2022) 22(10):614–28. doi: 10.1038/s41577-022-00680-w
48. Cirella A, Luri-Rey C, Di Trani CA, Teijeira A, Olivera I, Bolanos E, et al. Novel strategies exploiting interleukin-12 in cancer immunotherapy. Pharmacol Ther (2022) 239:108189. doi: 10.1016/j.pharmthera.2022.108189
49. Lu X. OX40 and OX40L interaction in cancer. Curr Med Chem (2021) 28(28):5659–73. doi: 10.2174/0929867328666201229123151
50. Barbier AJ, Jiang AY, Zhang P, Wooster R, Anderson DG. The clinical progress of mRNA vaccines and immunotherapies. Nat Biotechnol (2022) 40(6):840–54. doi: 10.1038/s41587-022-01294-2
51. Xu C, Cao CH, Tan T, Deng ST, Wu BH, Yang Q, et al. mRNA vaccine-A new cancer treatment strategy. Curr Cancer Drug Targets (2023) 23(9):669–81. doi: 10.2174/1568009623666230222124424
52. mRNA vaccine slows melanoma recurrence. Cancer Discov (2023) 13(6):1278. doi: 10.1158/2159-8290.CD-NB2023-0028
53. Balachandran VP, Rojas LA, Sethna Z, Soares K, Derhovanessian E, Mueller F, et al. Phase I trial of adjuvant autogene cevumeran, an individualized mRNA neoantigen vaccine, for pancreatic ductal adenocarcinoma. J Clin Oncol (2022) 40(16_suppl):2516. doi: 10.1200/JCO.2022.40.16_suppl.2516
54. Porciuncula A, Morgado M, Gupta R, Syrigos K, Meehan R, Zacharek SJ, et al. Spatial mapping and immunomodulatory role of the OX40/OX40L pathway in human non-small cell lung cancer. Clin Cancer Res (2021) 27(22):6174–83. doi: 10.1158/1078-0432.CCR-21-0987
55. Sayour EJ, De Leon G, Pham C, Grippin A, Kemeny H, Chua J, et al. Systemic activation of antigen-presenting cells via RNA-loaded nanoparticles. Oncoimmunology (2017) 6(1):e1256527. doi: 10.1080/2162402X.2016.1256527
56. Stratton MR. Exploring the genomes of cancer cells: progress and promise. Science (2011) 331(6024):1553–8. doi: 10.1126/science.1204040
57. Anderson K, Lutz C, van Delft FW, Bateman CM, Guo YP, Colman SM, et al. Genetic variegation of clonal architecture and propagating cells in leukaemia. Nature (2011) 469(7330):356–+. doi: 10.1038/nature09650
58. Lohr JG, Stojanov P, Carter SL, Cruz-Gordillo P, Lawrence MS, Auclair D, et al. Widespread genetic heterogeneity in multiple myeloma: implications for targeted therapy. Cancer Cell (2014) 25(1):91–101. doi: 10.1016/j.ccr.2013.12.015
59. Bolli N, Avet-Loiseau H, Wedge DC, Van Loo P, Alexandrov LB, Martincorena I, et al. Heterogeneity of genomic evolution and mutational profiles in multiple myeloma. Nat Commun (2014) 5:2997. doi: 10.1038/ncomms3997
60. Jones MR, Schrader KA, Shen Y, Pleasance E, Ch'ng C, Dar N, et al. Response to angiotensin blockade with irbesartan in a patient with metastatic colorectal cancer. Ann Oncol (2016) 27(5):801–6. doi: 10.1093/annonc/mdw060
61. Bedard PL, Hansen AR, Ratain MJ, Siu LL. Tumour heterogeneity in the clinic. Nature (2013) 501(7467):355–64. doi: 10.1038/nature12627
62. Russo G, Reche P, Pennisi M, Pappalardo F. The combination of artificial intelligence and systems biology for intelligent vaccine design. Expert Opin Drug Discov (2020) 15(11):1267–81. doi: 10.1080/17460441.2020.1791076
63. Thomas S, Abraham A, Baldwin J, Piplani S, Petrovsky N. Artificial intelligence in vaccine and drug design. Methods Mol Biol (2022) 2410:131–46. doi: 10.1007/978-1-0716-1884-4_6
64. McCaffrey P. Artificial intelligence for vaccine design. Methods Mol Biol (2022) 2412:3–13. doi: 10.1007/978-1-0716-1892-9_1
65. Rupaimoole R, Calin GA, Lopez-Berestein G, Sood AK. miRNA deregulation in cancer cells and the tumor microenvironment. Cancer Discov (2016) 6(3):235–46. doi: 10.1158/2159-8290.CD-15-0893
66. Hinshaw DC, Shevde LA. The tumor microenvironment innately modulates cancer progression. Cancer Res (2019) 79(18):4557–66. doi: 10.1158/0008-5472.CAN-18-3962
67. Paget S. The distribution of secondary growths in cancer of the breast. 1889. Cancer Metastasis Rev (1989) 8(2):98–101.
68. Munn DH, Bronte V. Immune suppressive mechanisms in the tumor microenvironment. Curr Opin Immunol (2016) 39:1–6. doi: 10.1016/j.coi.2015.10.009
69. Weiping. Z. Immunosuppressive networks in the tumour environment and their therapeutic relevance. Nat Rev Cancer (2005) 5(4):263–74. doi: 10.1038/nrc1586
70. Palmer CD, Rappaport AR, Davis MJ, Hart MG, Scallan CD, Hong SJ, et al. Individualized, heterologous chimpanzee adenovirus and self-amplifying mRNA neoantigen vaccine for advanced metastatic solid tumors: phase 1 trial interim results. Nat Med (2022) 28(8):1619–29. doi: 10.1038/s41591-022-01937-6
71. Birtel M, Voss RH, Reinhard K, Rengstl B, Ouchan Y, Michel K, et al. A TCR-like CAR promotes sensitive antigen recognition and controlled T-cell expansion upon mRNA vaccination. Cancer Res Commun (2022) 2(8):827–41. doi: 10.1158/2767-9764.CRC-21-0154
72. Basler L, Kowalczyk A, Heidenreich R, Fotin-Mleczek M, Tsitsekidis S, Zips D, et al. Abscopal effects of radiotherapy and combined mRNA-based immunotherapy in a syngeneic, OVA-expressing thymoma mouse model. Cancer Immunol Immunother (2018) 67(4):653–62. doi: 10.1007/s00262-018-2117-0
73. Iinuma H, Fukushima R, Inaba T, Tamura J, Inoue T, Ogawa E, et al. Phase I clinical study of multiple epitope peptide vaccine combined with chemoradiation therapy in esophageal cancer patients. J Transl Med (2014) 12:84. doi: 10.1186/1479-5876-12-84
74. He Q, Gao H, Tan D, Zhang H, Wang JZ. mRNA cancer vaccines: Advances, trends and challenges. Acta Pharm Sin B (2022) 12(7):2969–89. doi: 10.1016/j.apsb.2022.03.011
75. Pardi N, Hogan MJ, Weissman D. Recent advances in mRNA vaccine technology. Curr Opin Immunol (2020) 65:14–20. doi: 10.1016/j.coi.2020.01.008
76. Baharom F, Ramirez-Valdez RA, Tobin KKS, Yamane H, Dutertre CA, Khalilnezhad A, et al. Intravenous nanoparticle vaccination generates stem-like TCF1(+) neoantigen-specific CD8(+) T cells. Nat Immunol (2021) 22(1):41–52. doi: 10.1038/s41590-020-00810-3
77. Moyer TJ, Zmolek AC, Irvine DJ. Beyond antigens and adjuvants: formulating future vaccines. J Clin Invest (2016) 126(3):799–808. doi: 10.1172/JCI81083
78. Ols S, Yang L, Thompson EA, Pushparaj P, Tran K, Liang F, et al. Route of vaccine administration alters antigen trafficking but not innate or adaptive immunity. Cell Rep (2020) 30(12):3964–71 e7. doi: 10.1016/j.celrep.2020.02.111
79. Kranz LM, Diken M, Haas H, Kreiter S, Loquai C, Reuter KC, et al. Systemic RNA delivery to dendritic cells exploits antiviral defence for cancer immunotherapy. Nature (2016) 534(7607):396–401. doi: 10.1038/nature18300
80. Broos K, van der Jeught K, Puttemans J, Goyvaerts C, Heirman C, Dewitte H, et al. Particle-mediated intravenous delivery of antigen mRNA results in strong antigen-specific T-cell responses despite the induction of type I interferon. Mol Ther Nucleic Acids (2016) 5(6):e326. doi: 10.1038/mtna.2016.38
81. Phua KK, Staats HF, Leong KW, Nair SK. Intranasal mRNA nanoparticle vaccination induces prophylactic and therapeutic anti-tumor immunity. Sci Rep (2014) 4:5128. doi: 10.1038/srep05128
82. Mai Y, Guo J, Zhao Y, Ma S, Hou Y, Yang J. Intranasal delivery of cationic liposome-protamine complex mRNA vaccine elicits effective anti-tumor immunity. Cell Immunol (2020) 354:104143. doi: 10.1016/j.cellimm.2020.104143
83. Jiang H, Wang Q, Sun X. Lymph node targeting strategies to improve vaccination efficacy. J Control Release (2017) 267:47–56. doi: 10.1016/j.jconrel.2017.08.009
84. Bol KF, Figdor CG, Aarntzen EH, Welzen ME, van Rossum MM, Blokx WA, et al. Intranodal vaccination with mRNA-optimized dendritic cells in metastatic melanoma patients. Oncoimmunology (2015) 4(8):e1019197. doi: 10.1080/2162402X.2015.1019197
85. Deng Z, Yang H, Tian Y, Liu Z, Sun F, Yang P. An OX40L mRNA vaccine inhibits the growth of hepatocellular carcinoma. Front Oncol (2022) 12:975408. doi: 10.3389/fonc.2022.975408
86. Yang J, Zhu J, Sun J, Chen Y, Du Y, Tan Y, et al. Intratumoral delivered novel circular mRNA encoding cytokines for immune modulation and cancer therapy. Mol Ther Nucleic Acids (2022) 30:184–97. doi: 10.1016/j.omtn.2022.09.010
87. Arya S, Lin Q, Zhou N, Gao X, Huang JD. Strong immune responses induced by direct local injections of modified mRNA-lipid nanocomplexes. Mol Ther Nucleic Acids (2020) 19:1098–109. doi: 10.1016/j.omtn.2019.12.044
88. Lindsay KE, Bhosle SM, Zurla C, Beyersdorf J, Rogers KA, Vanover D, et al. Visualization of early events in mRNA vaccine delivery in non-human primates via PET-CT and near-infrared imaging. Nat BioMed Eng (2019) 3(5):371–80. doi: 10.1038/s41551-019-0378-3
89. Yu G, Wu Y, Cao X. Personalized neoantigen vaccines for tumor immunotherapy: a long hard journey to promising land. Chin J Cancer Biother (2022) 001):029.
90. Kudrin A. Cancer vaccines: What do we need to measure in clinical trials? Hum Vacc Immunother (2014) 10(11):3236–40. doi: 10.4161/hv.27586
91. Gulley JL, Arlen PM, Madan RA, Tsang KY, Pazdur MP, Skarupa L, et al. Immunologic and prognostic factors associated with overall survival employing a poxviral-based PSA vaccine in metastatic castrate-resistant prostate cancer. Cancer Immunol Immunother (2010) 59(5):663–74. doi: 10.1007/s00262-009-0782-8
92. Clifton GT, Kohrt HE, Peoples GE. Critical issues in cancer vaccine trial design. Vaccine (2015) 33(51):7386–92. doi: 10.1016/j.vaccine.2015.09.019
93. Caushi JX, Smith KN. Quantifying the anti-tumor immune response in patients receiving immunotherapy. Discov Med (2017) 24(130):59–68.
94. Slota M, Lim JB, Dang Y, Disis ML. ELISpot for measuring human immune responses to vaccines. Expert Rev Vaccines (2011) 10(3):299–306. doi: 10.1586/erv.10.169
95. Lehmann PV, Zhang W. Unique strengths of ELISPOT for T cell diagnostics. Methods Mol Biol (2012) 792:3–23. doi: 10.1007/978-1-61779-325-7_1
96. Li L, Goedegebuure SP, Gillanders WE. Preclinical and clinical development of neoantigen vaccines. Ann Oncol (2017) 28(suppl_12):xii11–xii7. doi: 10.1093/annonc/mdx681
97. Gohil SH, Iorgulescu JB, Braun DA, Keskin DB, Livak KJ. Applying high-dimensional single-cell technologies to the analysis of cancer immunotherapy. Nat Rev Clin Oncol (2021) 18(4):244–56. doi: 10.1038/s41571-020-00449-x
98. Zhang Y, Wang D, Peng M, Tang L, Ouyang J, Xiong F, et al. Single-cell RNA sequencing in cancer research. J Exp Clin Cancer Res (2021) 40(1):81. doi: 10.1186/s13046-021-01874-1
99. Liu Z, Li H, Dang Q, Weng S, Duo M, Lv J, et al. Integrative insights and clinical applications of single-cell sequencing in cancer immunotherapy. Cell Mol Life Sci (2022) 79(11):577. doi: 10.1007/s00018-022-04608-4
100. Tivey A, Church M, Rothwell D, Dive C, Cook N. Circulating tumour DNA - looking beyond the blood. Nat Rev Clin Oncol (2022) 19(9):600–12. doi: 10.1038/s41571-022-00660-y
Keywords: mRNA cancer vaccines, cancer, cancer vaccine, personalized vaccine, cancer therapy, immunotherapy
Citation: Wang B, Pei J, Xu S, Liu J and Yu J (2023) Recent advances in mRNA cancer vaccines: meeting challenges and embracing opportunities. Front. Immunol. 14:1246682. doi: 10.3389/fimmu.2023.1246682
Received: 26 June 2023; Accepted: 17 August 2023;
Published: 06 September 2023.
Edited by:
Zhiwei Fang, Johns Hopkins University, United StatesReviewed by:
Hongzhe Yu, Johns Hopkins University, United StatesCopyright © 2023 Wang, Pei, Xu, Liu and Yu. This is an open-access article distributed under the terms of the Creative Commons Attribution License (CC BY). The use, distribution or reproduction in other forums is permitted, provided the original author(s) and the copyright owner(s) are credited and that the original publication in this journal is cited, in accordance with accepted academic practice. No use, distribution or reproduction is permitted which does not comply with these terms.
*Correspondence: Jie Liu, bGluY2h1YW5nbGl1amllQDE2My5jb20=; Jinming Yu, c2R5dWppbm1pbmdAMTYzLmNvbQ==
Disclaimer: All claims expressed in this article are solely those of the authors and do not necessarily represent those of their affiliated organizations, or those of the publisher, the editors and the reviewers. Any product that may be evaluated in this article or claim that may be made by its manufacturer is not guaranteed or endorsed by the publisher.
Research integrity at Frontiers
Learn more about the work of our research integrity team to safeguard the quality of each article we publish.