- Department of Microbiology and Immunology, Institute of Marine and Environmental Technology, University of Maryland School of Medicine, Baltimore, MD, United States
Germinal centers (GCs) are distinct microanatomical structures that form in the secondary lymphoid organs of endothermic vertebrates (i.e., mammals and some birds). Within GCs, B cells undergo a Darwinian selection process to identify clones which can respond to pathogen insult as well as affinity mature the B cell repertoire. The GC response ultimately generates memory B cells and bone marrow plasma cells which facilitate humoral immunological memory, the basis for successful vaccination programs. GCs have not been observed in the secondary lymphoid organs of ectothermic jawed vertebrates (i.e., fishes, reptiles, and amphibians). However, abundant research over the past decades has indicated these organisms can produce antigen specific B cell responses and some degree of affinity maturation. This review examines data demonstrating that the fundamentals of B cell selection may be more conserved across vertebrate phylogeny than previously anticipated. Further, research in both conventional mammalian model systems and comparative models raises the question of what evolutionary benefit GCs provide endotherms if they are seemingly unnecessary for generating the basic functional components of jawed vertebrate humoral adaptive immune responses.
Introduction
B cells are the functional centerpiece of humoral adaptive immunity in jawed vertebrates. During the immune response, the host immune system selects B cell clones with B cell receptors (BCRs)/immunoglobulins (Igs) that recognize immunogens or antigens. These clones will proliferate and differentiate into antibody-secreting plasma cells, resulting in protective antibody titers that circulate in the blood and penetrate tissues to clear offending pathogens. In mammals and birds this response is facilitated by specialized microanatomical structures known as germinal centers (GCs). Over the course of a humoral response, GCs mediate maturation of peripheral antibody binding affinity (1, 2) and export effector cell types that generate long term immunological memory, namely long-lived bone marrow plasma cells (BMPCs) and memory B cells (MBCs) (3, 4).
GCs form in the secondary lymphoid organs (SLOs) after cognate B cells and T cells recognize antigen and interact at the follicular border (5, 6). Several functional characteristics define the GC reaction. B cells segregate into two poles or zones of the GC: the dark zone (DZ) containing cells known as centroblasts, and the light zone (LZ) containing cells known as centrocytes (7, 8). This compartmentalization is established by expression of specific chemokines and chemokine receptors. GC B cells expressing the receptor CXCR4 migrate to the DZ by sensing CXCL12 produced by stromal cells, while B cells expressing CXCR5 migrate towards CXCL13 produced in the LZ (7). Within the DZ B cells proliferate and express activation induced cytidine deaminase (AICDA) which mediates somatic hypermutation (SHM) of Ig variable (V) regions (2, 9). These clones can then exit the DZ and enter the LZ to undergo selection against antigen presented on the surface of follicular dendritic cells (FDCs) (7, 10). FDCs retain non-degraded antigen in immune complexes (ICs) (10) which preserve the non-linear epitopes BCRs can recognize. If SHM results in BCRs with increased binding affinity, the corresponding GC B cell clones can more efficiently extract antigen from the surface of FDCs (11, 12) and present antigenic peptides to T follicular helper (Tfh) cells present in the GC (13). Tfh cells supply signals that promote the survival of higher affinity clones (14, 15). Thus, through iterative rounds of proliferation/SHM and Darwinian selection, the GC reaction both identifies B cells which can bind antigens and supports affinity maturation of the polyclonal antibody response.
Bona fide GCs have only been observed in the SLOs of endothermic jawed vertebrates (mammals and birds); no ectothermic vertebrates studied have histologically observable GCs (16). The absence of GC structures in ectothermic vertebrates would suggest these taxa are incapable of generating effective B cell responses. Indeed, affinity maturation of antibodies in ectothermic vertebrates appears limited (approximately 10-fold maximum increase) (17–20) compared to endotherms (>100-fold increases) (1). Despite this, many studies demonstrate that ectothermic vertebrate antibody titers increase in an antigen-specific fashion in response to immunization or pathogen insult (18, 20–23), indicative of B cell clonal selection. AICDA-mediated SHM of Igs is also found in all jawed vertebrate lineages (20, 24–27), demonstrating a key role for mutation of BCRs in adaptive immunity. Finally, there is evidence for secondary recall responses in ectothermic jawed vertebrates (21, 28–30), indicative of B cell memory (although recall titers do not always exceed those of the primary response). Indeed, in some taxa immunological memory has been shown to persist for significant periods (>8 years) after primary exposure (31). Together these results demonstrate that ectothermic jawed vertebrate B cell responses are functionally protective in the absence of true GCs, even if affinity maturation is not as robust.
Interestingly, many functional characteristics deemed integral to mammalian B cell responses have since been shown to develop independently of the GC reaction. B cell clones which have undergone class switch recombination (CSR) of Ig isotypes, once believed to occur primarily within the GC, have been identified prior to GC-entry (32). Studies in mice indicate that affinity maturation of antibodies can proceed without GCs (33), and that extrafollicular B cell responses can facilitate both selection and affinity maturation (34). Finally, some evidence suggests that GCs may not be necessary for generating long-lived BMPCs (35, 36), and certainly memory B cells can be produced in a GC-independent fashion (37). Thus, if GCs are unnecessary for affinity maturation and immunological memory, and if ectothermic vertebrates can seemingly produce protective humoral responses without GCs, why did endothermic vertebrates evolve these intricately organized structures of B cell selection?
This review will examine what is known about B cell selection sites in jawed vertebrate lineages outside of mammals. Comparisons across taxa show that many fundamental components of the mammalian GC response are more conserved across jawed vertebrate phylogeny than previously appreciated.
Mammals: germinal centers are forever
Our understanding of the tissue architecture which supports B cell selection in mammals is derived primarily from studying the SLOs of mice (Mus musculus), specifically the C57BL/6 laboratory strain. The primordial SLO is the spleen, where antigen collects from the circulatory system and is brought into contact with the major cellular participants of the adaptive immune response: B cells, T cells, and antigen presenting cells (APCs). The spleen is present in all jawed vertebrates; in contrast, lymph nodes as SLOs for screening antigen in the afferent lymph are only observed in mammals and, reportedly, at least some avian species (38). Broadly, splenic immune architecture increases in complexity from basal jawed vertebrates, such as cartilaginous fishes, to those, like mammals, that emerged more recently (Figure 1) (reviewed in (39)). While the mouse spleen is not representative of every aspect of SLO architecture in mammals, or even exactly the same as the human spleen (reviewed in 40), it provides a good foundation for understanding mammalian B cell selection.
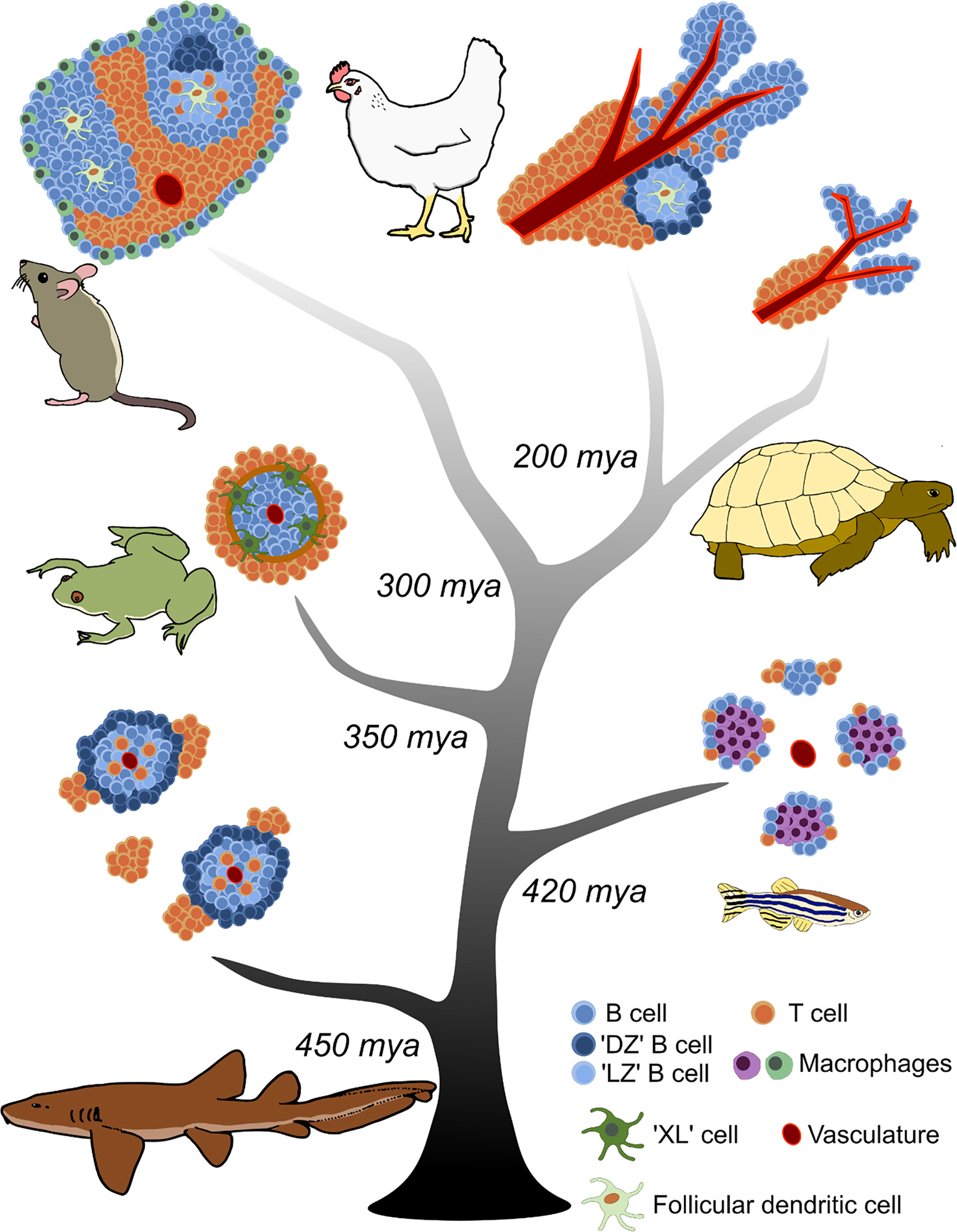
Figure 1 Evolution of splenic white pulp in jawed vertebrates. Increasing complexity in the organization of adaptive lymphocytes is observed from cartilaginous fishes to mammals and birds. The central tree represents the timeline of jawed vertebrate evolution; major branch points when taxonomic groups diverged from a common ancestor are indicated as millions of years ago (mya). Each jawed vertebrate lineage is represented adjacent to its branch by a depiction of the predominant comparative model species. Cell types and structures are annotated in the key.
The spleen consists of red pulp and white pulp regions. Immune cells are primarily localized in the white pulp. The red pulp filters aged and dead red blood cells, and helps transport antigen to the white pulp (reviewed in (40)). The mouse splenic white pulp is demarcated from the red pulp by the marginal zone (Figure 1). The marginal zone contains multiple cell types that participate in antigen trafficking, including innate-like marginal zone B cells, marginal zone macrophages, and marginal metallophilic macrophages (41). The white pulp is permeable to small (<65 kDa) molecules, but larger antigens must be transported across the marginal zone to concentrate with cognate B and T cells (42). Embedded within the mouse white pulp are B cell follicles surrounded by a defined T cell zone, also known as the periarteriolar lymphoid sheath (PALS). Maintenance of the T cell zone is controlled by expression of the chemokine receptor CCR7 and corresponding ligands CCL19 and CCL21 produced by stromal cells (43, 44). B cells are localized to the B cell zone by expression of the chemokine receptor CXCR5 and its corresponding ligand CXCL13, produced by FDCs to maintain the structure of the follicle (45, 46). FDCs are specialized APCs derived from a nonhematopoietic precursor (47), are dependent on LTα1β2 signaling (48, 49), and express the cellular adhesion molecules VCAM-1 and ICAM-1, plus complement receptors CR1 and CR2 (50, reviewed in 51). Thus, the mammalian splenic architecture maintains clearly defined B and T cell zones separated from the surrounding red pulp in which B cell selection is orchestrated (Figure 1).
B cell selection occurs when B cells recognize their cognate antigens, migrate to the border of the B and T cell zones, receive T cell help, then proceed to form GCs in the B cell follicles (5, 6). It is within these specialized structures that four major processes occur: 1. Segregation of B cell clones into distinct functional zones based on chemokine receptor expression, 2. Presentation of nondegraded antigen in ICs by specialized APCs to B cells, 3. Proliferation of activated B cells and SHM of Ig V regions mediated by AICDA, and 4. Co-stimulation of B cells by Tfh cells. The combination of these processes results in Darwinian selection of antigen-specific B cells that subsequently affinity matures the peripheral antibody response. Mammalian B cells can express multiple Ig heavy (H) chain isotypes serving different effector functions: IgM, secreted as a high avidity pentamer; IgD, an enigmatic isotype expressed on the surface of naïve B cells; IgG, with various subclasses depending on the species; IgA, secreted as a dimer at mucosal barriers; and IgE, typically associated with defenses against helminth infections (reviewed in 52).
While placental mammals (Eutheria) arose ~160 million years ago (mya) (53), the ‘mammalian model’ of B cell selection is built upon ~500 million years of prior jawed vertebrate evolutionary history. Indeed, while many features of the mammalian GC reaction were long assumed to be exclusive characteristics, examination of other jawed vertebrate lineages reveals analogous features, suggesting their origin in a jawed vertebrate ancestor.
Aves: you only evolve germinal centers twice
Birds (Aves), the other vertebrate lineage to have evolved true endothermy, also exhibit specialized GC-like structures for B cell selection in their SLOs. Modern birds diverged from a common ancestor with other jawed vertebrates (specifically Reptilia) ~200 mya (54). While their SLO architecture shares similarities with mammalian white pulp, there are key anatomical differences in the organization of their lymphocyte zones.
The majority of our (limited) understanding of avian B cell selection is derived from studying the chicken (Gallus gallus domesticus) spleen, although there are reports that lymph nodes may be present in ducks (Anas platyrhynchos domesticus) (38). The chicken spleen contains defined lymphocyte regions, but they are not demarcated by a marginal zone as in the mouse spleen (Figure 1). A PALS surrounding the central arterioles contains T cells as well as reticular cells and macrophages (55). Adjacent to the PALS is a peri-ellipsoid sheath (PELS) at the termination of the splenic arterioles. This PELS region primarily contains B cells (55), and it is equivalent to the B cell follicles of the mammalian spleen. Between the PALS and PELS are additional white pulp regions containing observable lymphocytes, presumed to be a mixture of B and T cells (55).
Immunization with antigen produces GCs in the chicken spleen (55–57); these begin to form 3-4 days after immunization and are fully formed and discernable approximately 6-7 days post-immunization (55–57). While mammalian GCs form within the B cell follicle, chicken GCs form in the white pulp region between the PALS and PELS (55). In contrast to the mammalian GC which is divided into two poles, establishing the DZ and the LZ, Yasuda et al. showed the circumference of the chicken GC is the equivalent of the mammalian DZ (Figure 2). BrdU+ labeling in immunized chickens reveals that highly proliferative cells are localized around the outside of the GC in a ring-like formation. These cells are negative for surface Ig light (L) chain or IgM, similar to mammalian centroblasts in the DZ (55). The central region of the chicken GC is believed to be equivalent to the mammalian GC LZ, containing Ig+ positive cells as well as DCs able to trap ICs (55).
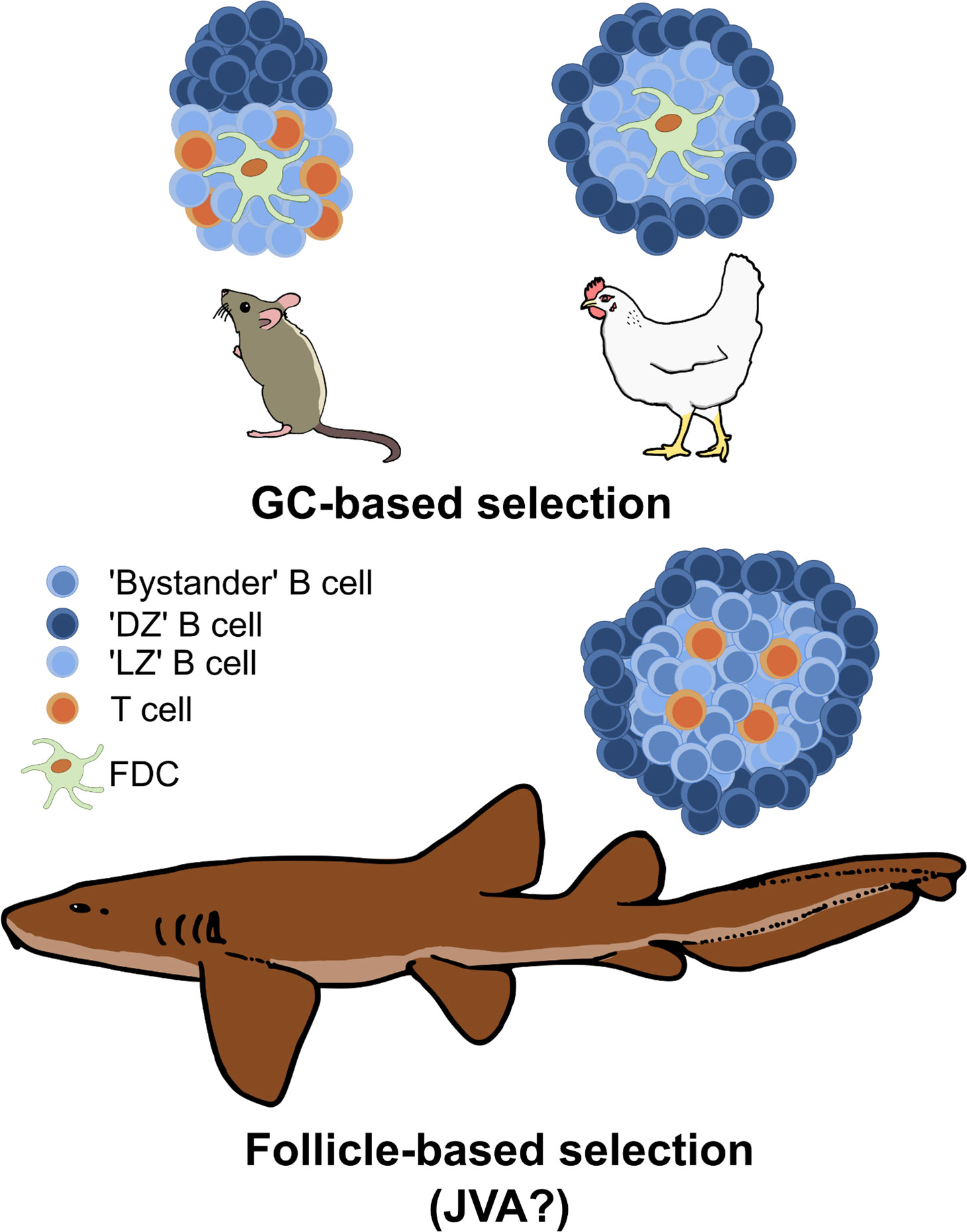
Figure 2 Comparison of GC structures in mammals and birds versus follicle-based selection in sharks. GCs segregate antigen-specific clones from the wider naïve B cell pool in the follicle, thus DZ and LZ B cells are predominantly derived from antigen-binding clonotypes. In contrast, B cell selection occurs across the entire follicle in sharks, with the central ‘LZ’ equivalent containing both ‘LZ’-like B cells (presumably antigen-specific) undergoing selection and ‘bystander’ B cells (non-antigen binding). This is hypothesized to produce a more ‘permissive’ but less efficient selection environment than found in GCs, and it may represent the primordial selection environment of the common jawed vertebrate ancestor (JVA). Cell types are annotated in the key.
CD3+ T cells are also observed within the chicken GC, primarily within the central LZ (55). Observations suggest that the proportion of CD3+ T cells does not change within the chicken GC over the course of an immune response, but there is evidence for CSR and expansion of IgY+ (the avian ortholog of mammalian IgG) B cells (56). Immunization with labeled human serum albumin demonstrates that protein antigen begins to localize to the chicken white pulp within 2-3 days, but only becomes present within GCs 4-7 days after immunization (57). White et al. hypothesized that antigen localization in GCs was dependent on the formation of antibody-antigen ICs, as the increase in GC-localized antigen correlated with an increase in circulating antigen-specific antibody titers (57). In this study, antigen was retained in GCs up to 42 days post-immunization. The antigen-bearing cells within chicken GCs are believed to be true FDCs because hematopoietic lineage negative (CD45-) cells expressing surface VCAM-1, ICAM-1, and Ig (presumably as ICs) have been characterized (58). However, it remains to be confirmed if chicken FDCs are truly of a non-hematopoietic origin equivalent to mammalian FDCs.
The appearance of GCs in both mammals and birds is most likely a result of convergent evolution. No ectothermic vertebrates possess GCs, and birds share a more recent common ancestor with reptiles than with mammals, diverging approximately 300 mya (54). The absence of evidence for either GCs or endothermic metabolism before this divergence suggests these adaptations evolved at least twice in jawed vertebrates.
While different in cellular organization, avian GCs are functionally equivalent to mammalian GCs in their ability to select antigen-specific B cell clones. Increases in antigen-specific antibody titers are observed after immunization in birds (57, 59). Comparisons of antibody responses in rabbits versus chickens/ducks demonstrates that the avian B cell selection system can also increase the affinity of the polyclonal B cell repertoire (60, 61). In some animals, ongoing affinity maturation of the repertoire is observed after 50+ days, but this may be dependent on antigen availability (60). The avian Ig H isotypes are IgM and IgY, which comprise the majority of the primary and secondary responses, respectively, as well as IgA (reviewed in 62).
The endothermic jawed vertebrates thus define a paradigm of B cell adaptive immunity in which GCs are established early (2-4 days) after immunization, antigen-specific antibody titers increase relatively quickly, and affinity maturation results in significant (>100-fold) increases in antigen binding affinity.
Reptiles: PALS and PELS are not enough (for robust affinity maturation)
Traveling further down the vertebrate evolutionary tree brings us to the non-avian reptiles (turtles, crocodilians, squamates, and tuatara), which diverged from other jawed vertebrates ~300 mya (54). Reptiles are the lineage with which birds most recently shared a common ancestor, so it is not surprising that the reptilian splenic structure closely resembles that of birds. Reptilian white pulp contains defined PALS and PELS regions (Figure 1). As examined in the Caspian turtle (Mauremys caspica), the inner region of the PELS appears to consist of Ig+ cells surrounded by an outer region of Ig- cells (63), presumably B cells and T cells respectively. The PALS appears to consist primarily of T cells (63). Variation in this microarchitecture is observed across various reptile species, with squamates (lizards and snakes) described as having less complex white pulp compared to chelonians (turtles) (63–65). Antigen-trapping DC-like cells have been observed in the white pulp of the reticulated python (Malayopython reticulatus) (64). Reptilian splenic white pulp also displays a ‘seasonality’ correlated to seasonal changes in thymic structure. The thymus, the primary lymphoid organ for T cell development in jawed vertebrates, undergoes involution seasonally in some reptiles, resulting in variations in the splenic microarchitecture (66, 67). Thymectomy (68) and adult thymic involution (69) also result in reduced cellularity of the PALS region of the reptilian spleen, further evidence that this region is an organized T cell zone. While reptiles have organized B and T cell zones, they do not form observable GCs in their white pulp (63, 64).
Despite this, reptiles are capable of generating antigen-specific Ig responses upon pathogenic infection or immunization (70–73). Evidence also exists for isotype switching and SHM in reptilian Igs (24). However, these antibody responses are slower than those observed in mammals and birds, with antibodies detected around one week post-immunization but titers typically not peaking until 6-8 weeks (22, 74, 75). True anamnestic immune responses have not been widely documented in reptiles. Reptilian antibody titers typically neither increase in magnitude nor attain peak levels more rapidly after secondary exposure to antigen (70, 72, 74, 76). Maturation of the binding affinity of the reptilian antibody response has also not been reported (70, 74). However, circulating antigen-specific antibody titers can persist in reptiles for significant periods after immunization (70, 71, 77). Origgi et al. investigated secondary challenge of Greek tortoises (Testudo gracea) with tortoise herpesviruses, reporting serum neutralizing antibody titers that may be indicative of a shorter latency period after re-exposure to the pathogen (22, 72). However, due to the absence of data indicating whether these antibody titers had returned to baseline prior to secondary challenge, it is not possible to definitively determine if these results prove true humoral immunological memory in this species. The Ig H chain isotypes expressed by reptilian B cells are IgM, IgD, IgY, the functional equivalent of mammalian IgG, and IgA depending on the species (reviewed in 78). Additionally, some reptile species have a unique isotype, IgD2, which is a chimera of IgD- and IgA-related constant domains and may play a role in mucosal protection (79, 80).
There is a paucity of literature on reptilian immune responses, making it difficult to draw definitive conclusions about this lineage. However, from the available data it seems that reptilian antibody responses lack the robust affinity maturation observed in endotherms as well as the rapid increases in secondary antibody titers. The slower humoral response in reptiles has been attributed to the lower core body temperature of ectotherms, but increasing the ambient temperature to 37°C did not alter the kinetics of the antibody response in turtles (70). It thus appears that while reptiles possess distinct splenic microarchitecture, the absence of GCs deprives their immune system of a selective environment capable of significantly maturing their antibody repertoires over the time periods studied.
Amphibians: on double-duty antigen presenting service
Modern amphibians last shared a common ancestor with the other vertebrate groups ~350 mya (54) and represent the divergence point where tetrapods emerged and began to evolve terrestrial lifestyles. The best studied model of amphibian immunity is the African clawed frog (Xenopus laevis). In mammals, B cell follicles form around the central arterioles of the spleen during ontogeny and are later dispersed by the accumulation of T cells that form the PALS regions, establishing the mature splenic architecture. In Xenopus, B cell follicles also surround central arterioles, but there is no displacement to form observable T cell zones during development (Figure 1). Instead, the follicles are surrounded by a double layer of elongated cells termed the ‘Grenzschichtmembran of Sterba’ (GS) (81). T cells are dispersed outside this GS, but after immunization are recruited to the borders of the white pulp, presumably from the red pulp and peripheral circulation (82). The T cells form a corona surrounding the B cell follicle, and B cell-T cell contacts can be observed at the white pulp border (82). Thymectomy depletes lymphocytes from the red pulp and results in abolition of this T cell ring (83). AICDA is expressed in the follicles of the spleen after immunization (84), indicative of SHM. However, no GC-like structures or clusters of proliferating (BrdU+) cells have been observed in the spleen of Xenopus (85).
Amphibians are the first vertebrate class to have evolved canonical Ig CSR. The Xenopus laevis genome contains canonical switch (S)-regions to facilitate AICDA-mediated recombination (86). These S-regions can functionally replace mouse S-regions for CSR (87), and Xenopus AICDA can facilitate CSR in AICDA-deficient mammalian cells (88). It is possible that this adaptation co-evolved with the closer association of B cell-T cell interaction observed at the borders of the amphibian white pulp to facilitate efficient isotype switching. The amphibian Ig H chain isotypes as identified in Xenopus are IgM, IgD, IgX which plays an important role in mucosal immunity, IgY, and IgF (reviewed in 78).
APCs have been identified in amphibians that appear to fulfill the functional roles of both conventional dendritic cells (cDCs), presenting peptide antigens to T cells, and FDCs, presenting non-degraded antigen in ICs to B cells. These cells, first identified in Xenopus laevis and thus termed ‘XL’ cells, have the morphological characteristics of cDCs, including long cytoplasmic processes (89), and appear to be capable of trapping antigen at their plasma membrane (90). Following immunization with human IgG, antigen was found to localize at the perimeter of the Xenopus laevis white pulp, in a similar position to the XL cells (91). This led to the hypothesis that XL cells may be responsible for antigen trafficking and presentation in amphibian white pulp. This hypothesis was subsequently confirmed by Neely and colleagues who showed that in Xenopus immunized with fluorescent R-phycoerythrin (PE), antigen could be observed at the perimeter of the white pulp, where T cells subsequently colocalized (82). XL cells express both MHC class II and the T cell chemoattractant CCL19, indicative of a role in antigen presentation to T cells, but they are also capable of native antigen retention and presentation, exhibit positive surface staining for amphibian Igs, and express the B cell chemoattractant CXCL13 (82). Collectively, these results suggest XL cells may fulfill a ‘double-duty’ antigen presenting role for both T cells and B cells, preceding the evolution of canonical FDCs in endotherms.
The amphibian B cell response has similar kinetics to the reptilian response. IgM predominates the primary response for the first week or so, with IgY titers increasing approximately two weeks post-immunization, consistent with antigen-driven CSR (82). In Xenopus infected twice with the ranavirus Frog Virus 3 (FV3), FV3-specifc IgY titers were detectable around one week after the second infection, peaked at ~3 weeks, and remained detectable up to 4 weeks post-infection (92). These anti-FV3 antibodies could neutralize virus, and adult frogs challenged 15 months after infection displayed an anti-FV3 IgY memory response (93). Xenopus previously exposed to Batrachochytrium dendrobatidis, a chytrid fungus, exhibited pathogen-binding IgM, IgY, and IgX in mucus secretions (94), indicative of a role for Igs in amphibian mucosal immunity. However, the Xenopus IgY antigen-specific response to the hapten dinitrophenyl (DNP) displayed limited heterogeneity and affinity maturation compared to mammalian responses, hypothesized to be a result of ineffective B cell selection in the absence of GCs (20).
The amphibian adaptive immune system demonstrates a crossroads in evolution, where closer association of B cells and T cells in the splenic white pulp appears to be correlated with the emergence of bona fide CSR. ‘Double-duty’ APCs, capable of presenting antigen to both B cells and T cells, may define ectothermic B cell selection prior to the evolution of canonical FDCs and GCs.
Teleosts: live and let macrophage
The most diverse lineage of vertebrates on Earth is by far the bony fishes (Osteichthyes), dominated by the ray-finned fishes (Actinopterygii) containing the infraclass teleosts (Teleostei). Ray-finned fishes diverged ~420 mya, with teleosts separating ~300 mya (95). Much of our knowledge of bony fish immunity has come from studying this group. The teleost spleen diverges markedly from the microarchitecture observed in the previously described jawed vertebrate taxa. There are regions of red pulp and white pulp, but lymphocytes are distributed throughout both without identifiable B cell follicles or major regions of B cell/T cell organization (96, 97) (Figure 1). Instead, following immunization or infection, lymphocytes appear to aggregate around pigmented macrophages, producing foci in the spleen known as melanomacrophage centers (MMCs) (96, 98–100). MMC presence and size appears correlated with infection status in bony fishes, and thus MMCs have been utilized to asses health in commercially relevant fish species (101). Melanomacrophages are highly phagocytic and will uptake injected materials, such as microspheres or bacteria (102, 103). There is evidence that antigen can be trapped in ICs in teleost spleen, although it remains unclear if these ICs are primarily retained by the MMCs or splenic ellipsoids (96, 104, 105). However, AICDA expression has been detected in cells interspersed within MMCs, suggesting a role for MMCs in the adaptive response (106). Additionally, cells within these clusters express Ig H chains with others expressing T cell receptors and CD4 (106).
Research in zebrafish (Danio rerio), the classic teleost model organism, by Waly et al. indicates that MMCs may function as a selective environment for B cell responses. Waly et al. found that individual MMCs display low BCR clonal diversity with expansion of oligoclonal lineages, suggesting proliferation of selected B cell clonotypes (107). They also found higher replacement to silent mutation ratios (R/S) in the complementarity determining regions (CDRs) of zebrafish Ig VDJ repertoires compared to the framework regions (FWR), indicative of antigen-driven selection favoring mutations in the antigen-binding region of Ig V sequences (107). Finally, they suggest that MMCs in the teleost spleen may trap and retain antigen (107) similar to mammalian FDCs. However, the role of MMCs as the de facto APC for B cell antigen retention and presentation in fish remains debatable. It remains possible that MMC formation is a physiological adaptation only tangentially associated with teleost immune responses (108). Further, whether teleost melanomacrophages produce B cell-recruiting cytokines such as CXCL13, or if they can also present antigen to T cells like Xenopus XL cells, remains to be determined.
There is an extensive body of literature examining teleost antigen-specific antibody responses, particularly in the context of vaccination against pathogens which threaten commercial aquaculture species (reviewed in 109). The teleost Ig H chain isotypes are IgM, IgD, and IgT (reviewed in 110). IgM responses dominate the teleost serum, while IgT is functionally equivalent to mammalian IgA, playing an important role in mucosal defense (111). Antigen-specific antibody titers can be detected in teleosts anywhere from 2-4 weeks post-immunization, with titers peaking at approximately 10-12 weeks post-immunization (18, 112). Depending on the immunizing antigen used, secondary responses can be detected in teleosts indicative of immunological memory (28, 113, 114). Affinity maturation of peripheral antibody responses has been detected in bony fishes. Cain et al. reported a 2-3 fold increase in antibody-antigen binding in immunized trout (18). Kaattari et al. demonstrated that this affinity maturation results from the emerging dominance of high affinity subpopulations of antibody later in the response (19), although it is not certain if this is a result of SHM of initially selected clonotypes or the expansion of newly selected clonotypes later in the response. The high affinity subpopulations persist longer and achieve higher titers than low affinity subpopulations (115). This shift to high affinity subpopulations was also observed in channel catfish (Ictalurus punctaus), resulting in affinity maturation of the antigen-specific antibody response (112).
Considering the vast diversity of teleost species care must be taken not to generalize for the whole lineage using data obtained from only a few species. Indeed, the radiation of teleosts has resulted in extreme losses of adaptive immune function in several species. Genomic studies in Gadiformes (cod, haddock, pollock etc.) demonstrate a loss of both MHC class II and CD4 genes (116, 117), nullifying canonical T cell dependent antibody responses in this group. Additionally, cod AICDA is catalytically inactive, severely limiting secondary antibody diversification (118). The evolution of unique reproductive strategies also appears to have influenced the loss of immune genes in other bony fishes. Several genes of the MHC II pathway are lost in pipefishes (syngnathids) which exhibit male pregnancy (119), and some species of anglerfish (Lophiiformes), a lineage that has evolved extreme forms of sexual parasitism, have concomitantly lost many aspects of canonical adaptive immunity (120). These findings in teleosts underscore the malleability of adaptive immunity when confronted with singular evolutionary pressures.
While study of additional (varied) bony fish species will certainly improve our knowledge, current evidence supporting MMCs as the functional analogues of mammalian GCs in the bony fishes is far from conclusive. However, based on data from the other vertebrate lineages and recent studies performed in the more evolutionary ancient cartilaginous fishes (below), it is very unlikely that MMC-based selection represents the ‘primordial’ state found in the jawed vertebrate ancestor, but is more likely a bony fish-specific derivation.
Cartilaginous fishes: for B cell follicles only
Cartilaginous fishes (Chondrichthyes) are the oldest extant taxonomic group of vertebrates with Ig-based adaptive immunity. Chondrichthyes emerged in the early Silurian period ~450 mya (121, 122) and is divided into two subclasses: Holocephali (chimeras or ratfishes) and Elasmobranchii (sharks, skate, and rays). The elasmobranchs have been the focus of most studies on cartilaginous fish immunity; in particular, the little skate (Leucoraja erinacea) and nurse shark (Ginglymostoma cirratum) have been key model organisms for this lineage.
The tissue architecture of the nurse shark spleen reflects the basal position of this lineage in phylogeny. There are identifiable red pulp and white pulp regions, but no marginal zone or border surrounding the white pulp (123) (Figure 1). During development, B cells begin to populate the spleen around the central arterioles, forming nascent follicles (124). In adult animals, Ig+ B cells populate the follicles of the white pulp, and Ig secreting plasma cells are identifiable in the red pulp (125). Cells with dendritic-like processes have also been observed in nurse shark spleen which express MHC class II and may accumulate antigen on their plasma membranes (39, 124). No identifiable T cell zones are present in the shark spleen, and up until recently it was unclear how T cells were organized in cartilaginous fish SLOs. In situ hybridization experiments revealed T cells are found in small aggregates within the red pulp, external to the B cell follicles (126). Visually discernable GCs have not been found in the shark spleen (16, 123).
Our laboratory recently provided the first evidence for how B cell selection may be orchestrated within the cartilaginous fish SLO. Fluorescence in situ hybridization experiments revealed that the splenic follicles of immunized nurse sharks contain Ig+/AICDA+/Ki-67+ cells around the circumference of the white pulp (127) (Figure 2). CD3ϵ+ T cells were identified in aggregates associated with the borders of B cell follicles, as well as distributed within the follicles themselves (127). Single nuclei sequencing of nurse shark spleen samples revealed B cells with centrocyte- and centroblast-like gene expression signatures (127). Additionally, we identified a subset of T cells that expressed genes associated with mammalian Tfh cells (127). Finally, we demonstrated that nondegraded antigen in the form of fluorescent R-PE can be presented in the center of B cell follicles, and the Ig V regions of BCR sequences isolated from follicles show higher R/S ratios in the CDRs compared to FWRs (127). Collectively, these results suggest shark B cells undergo selection against antigen in the center of follicles, possibly receiving co-stimulation from Tfh-like cells. They then migrate to the outer edge of the follicle to proliferate/hypermutate, and eventually exit the follicle to differentiate into antibody-secreting plasma cells. Of note, the organization of the immunized shark B cell follicle with proliferating cells around the circumference resembles the structure of the avian GCs identified by BrdU+ staining (55) (Figure 2).
The presence of nondegraded antigen retained in shark B cell follicles suggests that the paradigm of ‘double-duty’ presenting cells may have evolved earlier than XL cells in amphibians. FDCs have not been identified in any ectothermic vertebrates, but more definitive work is necessary to determine if cartilaginous fishes have APC populations capable of presenting antigen to both T cells in an MHC class II dependent fashion, as well as B cells in the form of captured ICs. Certainly, the accumulating data demonstrates that the antigen presenting function of FDCs was present in jawed vertebrates long before the cell type emerged in mammals (82, 127).
Despite the lack of complexity in cartilaginous splenic architecture compared to mammals, the ‘B cell follicle only’ system still supports robust humoral immunity. Sharks have 3 Ig H chain isotypes: IgM, expressed in pentameric (pIgM) and monomeric (mIgM) forms; IgW, the ortholog of mammalian IgD; and Ig new antigen receptor (IgNAR), an H-chain only isotype that does not associate with L chain (reviewed in 128). Early antibody responses are dominated by low affinity, high avidity pIgM (21). After immunization, antigen-specific mIgM and IgNAR titers increase for up to 2-3 months and persist for long periods (21). Shark Ig genes mutate at very high rates (26, 129), and both mIgM and IgNAR undergo affinity maturation (17, 21). Humoral immunological memory has been described in nurse shark up to 8 years after primary exposure (31).
Even in cartilaginous fishes, a lineage that diverged very early in jawed vertebrate evolution, key hallmarks of GC-based B cell selection are present in the SLO.
No time for slow selection—why did endotherms evolve germinal centers?
From the studies highlighted above, two conclusions emerge. First, SLO architecture increases in complexity as one moves forward in jawed vertebrate evolution, culminating in the emergence of GCs in birds and mammals (Figure 1). The exception to this paradigm is the bony fishes, which lack discernable white pulp or organized lymphocyte zones. Second, the major functional characteristics of humoral immunity, specifically the capacity to produce antigen-specific antibody titers, to affinity mature antibody repertoires, and to generate secondary memory responses, are present in all jawed vertebrate lineages to some extent. If these key immunological adaptations are possible without GCs, it begs us to ask why did the specialized microstructure of the GC evolve? Based upon current data it seems that the emergence of GCs is not due to the presence of a more ‘advanced’ adaptive immune system in endothermic jawed vertebrates. Rather, the convergent evolution of endothermic metabolism in birds and mammals may have necessitated the development of a more stringent B cell selection environment in these lineages.
Cells able to present antigen to B cells are clearly present in ectothermic vertebrates even if bona fide FDCs are not. Interestingly, several lines of evidence demonstrate that some mammalian cDCs can present membrane-bound antigen to B cells (reviewed in 130). Specifically, the type 2 cDC subset (cDC2) which localizes to the B-T cell border may exhibit ‘double-duty’ abilities and present antigen to both B cells and T cells in mammals (131, 132). Indeed, such cDCs could be the primordial system of antigen display, with FDCs co-opting this role very late in jawed vertebrate evolution.
Our recent work shows that intact antigen is widely distributed across the center (and apparent LZ equivalent) of nurse shark B cell follicles (127). Given that B cell division rates are almost certainly slower at lower (i.e., exothermic) body temperatures and there is no visible segregation of antigen-specific clones from the wider population of B cells, we hypothesize that this reduces selection pressure, facilitating the retention of lower affinity clones while also supporting the production of an extensive and diverse memory B cell pool (133). Such a permissive selection environment would explain the delayed antibody responses and lower levels of affinity maturation reported in cartilaginous fishes and other ectothermic vertebrates.
In contrast, GCs in endotherms are founded by antigen-specific B cell clones that have received T cell help. These B cells can divide at a much faster rate at an endothermic core body temperature, and so the selective environment becomes increasingly segregated from the wider pool of non-specific clones. Further, only the FDCs at the center of the LZ act as a long-term reservoir of intact antigen in mice (134). Thus, it appears that the distinct microanatomical structure of the GC intensifies selection pressures by increasing competition for antigen and T cell help, thereby facilitating faster, more efficient selection of higher affinity antigen-specific B cells in endotherms. This would be evolutionary advantageous given that the maintenance of warmer core temperatures also supports the rapid proliferation of potentially infectious organisms (135–137), and may be required in endothermic vertebrates to avoid succumbing to pathogen infections before sufficient protective antibody titers can be mounted (138).
However, ‘faster’ selection does not, on its own, appear to fully explain the evolutionary advantage of GCs (138). While endotherms attain peak antibody titers more quickly than ectotherms (138), pathogens generally exhibit far shorter replicative cycles than the host organisms they infect, whether endothermic or exothermic. Influenza-like viruses, for example, infect a wide range of endothermic and ectothermic vertebrate hosts and successfully replicate at a variety of temperatures (139–141). While viruses in ectotherms may replicate slower than in endotherms, surely those pathogens which escape immediate control by the innate immune system still pose a threat to host survival long before antibody responses begin to peak. Under this lens, antibody-based adaptive immunity would bestow little evolutionary advantage.
Rather, we propose that the initial selective advantage of antibody-based adaptive responses was the immunological memory conferred by antigen-experienced B cell clones. In this scenario an ectothermic jawed vertebrate ancestor with a ‘slow-but-permissive’ adaptive immune system could produce a diverse memory repertoire capable of combating future pathogen variants. Indeed, it has been suggested that the primordial role of SHM was to further diversify the repertoire of antigen-specific memory B cells (142). When endothermic metabolism emerged as an adaptation it accelerated B cell division in SLO selective sites, resulting in visually discernable GCs able to perform B cell selection quicker and more stringently. While not the driving force, a by-product of this evolutionary process would be more rapid primary responses and higher levels of affinity maturation, as observed in endotherms. Indeed, a possible drawback of faster B cell selection is immunodominance (reviewed in 143), leading to vaccine responses predominantly directed towards undesirable epitopes. Restrictive selection may also result in a low frequency of de novo clones responding to heterologous antigens (144, 145), thus limiting the final repertoire.
Understanding that the mammalian GC response is just an accelerated version of the selection models in ectotherms suggests that vaccine efficacy may be improved by modifying immunization strategies. Indeed, strategies such as slow release of antigen (146) or promotion of persistent GCs (147), mimicking the selection environment/kinetics in ectothermic vertebrates, seem to generate more diverse B cell responses better able to deal with pathogen variants.
Conclusion
It is increasingly apparent that sophisticated B cell selection mechanisms incorporating T cell help, SHM driven by AICDA, and specialized cells capable of presenting native antigen emerged early in jawed vertebrate evolution. As evolutionary pressures forced more efficient selection of antigen-specific B cell clones, the complexity of SLO tissue organization also increased to facilitate the coordination of B cell, T cell, and APC interactions, culminating in the appearance of GCs. While mammalian GC selection may be more stringent, this may come at the cost of antigenic imprinting and B cell immunodominance. New immunization strategies that mimic the selective environment/kinetics of ectothermic vertebrates may help in the fight against rapidly evolving pathogens such as influenza, HIV, or human coronaviruses.
Author contributions
HM and HD conceived the general topic of the review. HM wrote the manuscript and designed the figures. HD provided insightful feedback and criticism of the final manuscript.
Funding
This work was funded by National Institutes of Health Predoctoral Fellowship F31AI147532 awarded to HM.
Conflict of interest
The authors declare that the research was conducted in the absence of any commercial or financial relationships that could be construed as a potential conflict of interest.
Publisher’s note
All claims expressed in this article are solely those of the authors and do not necessarily represent those of their affiliated organizations, or those of the publisher, the editors and the reviewers. Any product that may be evaluated in this article, or claim that may be made by its manufacturer, is not guaranteed or endorsed by the publisher.
References
1. Eisen HN, Siskind GW. Variations in affinities of antibodies during the immune response. Biochemistry (1964) 3:996–1008. doi: 10.1021/bi00895a027
2. Berek C, Berger A, Apel M. Maturation of the immune response in germinal centers. Cell (1991) 67:1121–9. doi: 10.1016/0092-8674(91)90289-b
3. Dilosa RM, Maeda K, Masuda A, Szakal AK, Tew JG. Germinal center B cells and antibody production in the bone marrow. J Immunol (1991) 146:4071–7. doi: 10.4049/jimmunol.146.12.4071
4. Shinnakasu R, Inoue T, Kometani K, Moriyama S, Adachi Y, Nakayama M, et al. Regulated selection of germinal-center cells into the memory B cell compartment. Nat Immunol (2016) 17:861–9. doi: 10.1038/ni.3460
5. Okada T, Miller MJ, Parker I, Krummel MF, Neighbors M, Hartley SB, et al. Antigen-engaged B cells undergo chemotaxis toward the T zone and form motile conjugates with helper T cells. PloS Biol (2005) 3:e150. doi: 10.1371/journal.pbio.0030150
6. Garside P, Ingulli E, Merica RR, Johnson JG, Noelle RJ, Jenkins MK. Visualization of specific B and T lymphocyte interactions in the lymph node. Science (1998) 281:96–9. doi: 10.1126/science.281.5373.96
7. Allen CDC, Ansel KM, Low C, Lesley R, Tamamura H, Fujii N, et al. Germinal center dark and light zone organization is mediated by CXCR4 and CXCR5. Nat Immunol (2004) 5:943–52. doi: 10.1038/ni1100
8. Pereira JP, Kelly LM, Xu Y, Cyster JG. EBI2 mediates B cell segregation between the outer and centre follicle. Nature (2009) 460:1122–6. doi: 10.1038/nature08226
9. Muramatsu M, Kinoshita K, Fagarasan S, Yamada S, Shinkai Y, Honjo T. Class switch recombination and hypermutation require activation-induced cytidine deaminase (AID), a potential RNA editing enzyme. Cell (2000) 102:553–63. doi: 10.1016/s0092-8674(00)00078-7
10. Heesters BA, Chatterjee P, Kim Y-A, Gonzalez SF, Kuligowski MP, Kirchhausen T, et al. Endocytosis and recycling of immune complexes by follicular dendritic cells enhances B cell antigen binding and activation. Immunity (2013) 38:1164–75. doi: 10.1016/j.immuni.2013.02.023
11. Natkanski E, Lee W-Y, Mistry B, Casal A, Molloy JE, Tolar P. B cells use mechanical energy to discriminate antigen affinities. Science (2013) 340:1587–90. doi: 10.1126/science.1237572
12. Spillane KM, Tolar P. B cell antigen extraction is regulated by physical properties of antigen-presenting cells. J Cell Biol (2016) 216:217–30. doi: 10.1083/jcb.201607064
13. Kim CH, Rott LS, Clark-Lewis I, Campbell DJ, Wu L, Butcher EC. Subspecialization of CXCR5+ T cells: B helper activity is focused in a germinal center-localized subset of CXCR5+ T cells. J Exp Med (2001) 193:1373–81. doi: 10.1084/jem.193.12.1373
14. Dominguez-Sola D, Victora GD, Ying CY, Phan RT, Saito M, Nussenzweig MC, et al. The proto-oncogene MYC is required for selection in the germinal center and cyclic reentry. Nat Immunol (2012) 13:1083–91. doi: 10.1038/ni.2428
15. Shulman Z, Gitlin AD, Weinstein JS, Lainez B, Esplugues E, Flavell RA, et al. Dynamic signaling by T follicular helper cells during germinal center B cell selection. Science (2014) 345:1058–62. doi: 10.1126/science.1257861
16. Zapata A, Amemiya CT. “Phylogeny of Lower Vertebrates and Their Immunological Structures.,”. In: Du Pasquier L, Litman GW, editors. Origin and Evolution of the Vertebrate Immune System. Current Topics in Microbiology and Immunology. Berlin, Heidelberg: Springer (2000). p. 67–107. doi: 10.1007/978-3-642-59674-2_5
17. Dooley H, Stanfield RL, Brady RA, Flajnik MF. First molecular and biochemical analysis of in vivo affinity maturation in an ectothermic vertebrate. Proc Natl Acad Sci U.S.A. (2006) 103:1846–51. doi: 10.1073/pnas.0508341103
18. Cain KD, Jones DR, Raison RL. Antibody–antigen kinetics following immunization of rainbow trout (Oncorhynchus mykiss) with a T-cell dependent antigen. Dev Comp Immunol (2002) 26:181–90. doi: 10.1016/S0145-305X(01)00063-5
19. Kaattari SL, Zhang HL, Khor IW, Kaattari IM, Shapiro DA. Affinity maturation in trout: clonal dominance of high affinity antibodies late in the immune response. Dev Comp Immunol (2002) 26:191–200. doi: 10.1016/S0145-305X(01)00064-7
20. Wilson M, Hsu E, Marcuz A, Courtet M, Du Pasquier L, Steinberg C. What limits affinity maturation of antibodies in Xenopus–the rate of somatic mutation or the ability to select mutants? EMBO J (1992) 11:4337–47. doi: 10.1002/j.1460-2075.1992.tb05533.x
21. Dooley H, Flajnik MF. Shark immunity bites back: affinity maturation and memory response in the nurse shark, Ginglymostoma cirratum. Eur J Immunol (2005) 35:936–45. doi: 10.1002/eji.200425760
22. Origgi FC, Klein PA, Mathes K, Blahak S, Marschang RE, Tucker SJ, et al. Enzyme-Linked Immunosorbent Assay for Detecting Herpesvirus Exposure in Mediterranean Tortoises (Spur-Thighed Tortoise [Testudo graeca] and Hermann’s Tortoise [Testudo hermanni]). J Clin Microbiol (2001) 39:3156–63. doi: 10.1128/JCM.39.9.3156-3163.2001
23. Wetherall J, Turner K. Immune response of the lizard, tiliqua rugosa. Aust J Exp Biol Med Sci (1972) 50:79–95. doi: 10.1038/icb.1972.7
24. Turchin A, Hsu E. The generation of antibody diversity in the turtle. J Immunol (1996) 156:3797–805.
25. Quinlan EM, King JJ, Amemiya CT, Hsu E, Larijani M. Biochemical regulatory features of activation-induced cytidine deaminase remain conserved from lampreys to humans. Mol Cell Biol (2017) 37:e00077–17. doi: 10.1128/MCB.00077-17
26. Diaz M, Greenberg AS, Flajnik MF. Somatic hypermutation of the new antigen receptor gene (NAR) in the nurse shark does not generate the repertoire: possible role in antigen-driven reactions in the absence of germinal centers. Proc Natl Acad Sci U.S.A. (1998) 95:14343–8. doi: 10.1073/pnas.95.24.14343
27. Zhao Y, Pan-Hammarström Q, Zhao Z, Hammarström L. Identification of the activation-induced cytidine deaminase gene from zebrafish: an evolutionary analysis. Dev Comp Immunol (2005) 29:61–71. doi: 10.1016/j.dci.2004.05.005
28. Cossarini-Dunier M. Secondary response of rainbow trout (Salmo gairdneri Richardson) to DNP-haemocyanin and Yersinia ruckeri. Aquaculture (1986) 52:81–6. doi: 10.1016/0044-8486(86)90027-X
29. Tatner MF. The ontogeny of humoral immunity in rainbow trout,Salmo gairdneri. Veterinary Immunol Immunopathol (1986) 12:93–105. doi: 10.1016/0165-2427(86)90114-5
30. Work TM, Balazs GH, Rameyer RA, Chang SP, Berestecky J. Assessing humoral and cell-mediated immune response in Hawaiian green turtles, Chelonia mydas. Veterinary Immunol Immunopathol (2000) 74:179–94. doi: 10.1016/S0165-2427(00)00168-9
31. Eve O, Matz H, Dooley H. Proof of long-term immunological memory in cartilaginous fishes. Dev Comp Immunol (2020) 108:103674. doi: 10.1016/j.dci.2020.103674
32. Roco JA, Mesin L, Binder SC, Nefzger C, Gonzalez-Figueroa P, Canete PF, et al. Class-switch recombination occurs infrequently in germinal centers. Immunity (2019) 51:337–350.e7. doi: 10.1016/j.immuni.2019.07.001
33. Matsumoto M, Lo SF, Carruthers CJL, Min J, Mariathasan S, Huang G, et al. Affinity maturation without germinal centres in lymphotoxin-α-deficient mice. Nature (1996) 382:462–6. doi: 10.1038/382462a0
34. Di Niro R, Lee S-J, Vander Heiden JA, Elsner RA, Trivedi N, Bannock JM, et al. Salmonella infection drives promiscuous B cell activation followed by extrafollicular affinity maturation. Immunity (2015) 43:120–31. doi: 10.1016/j.immuni.2015.06.013
35. Bortnick A, Chernova I, Quinn WJ, Mugnier M, Cancro MP, Allman D. Long-lived bone marrow plasma cells are induced early in response to T cell-independent or T cell-dependent antigens. J Immunol (2012) 188:5389–96. doi: 10.4049/jimmunol.1102808
36. Koike T, Fujii K, Kometani K, Butler NS, Funakoshi K, Yari S, et al. Progressive differentiation toward the long-lived plasma cell compartment in the bone marrow. J Exp Med (2022) 220:e20221717. doi: 10.1084/jem.20221717
37. Pape KA, Taylor JJ, Maul RW, Gearhart PJ, Jenkins MK. Different B cell populations mediate early and late memory during an endogenous immune response. Science (2011) 331:1203–7. doi: 10.1126/science.1201730
38. Sugimura M, Hashimoto Y, Nakanishi YH. Thymus- and bursa-dependent areas in duck lymph nodes. Jpn J Vet Res (1977) 25(1-2):7-16. doi: 10.14943/jjvr.25.1-2.7
39. Neely HR, Flajnik MF. Emergence and evolution of secondary lymphoid organs. Annu Rev Cell Dev Biol (2016) 32:693–711. doi: 10.1146/annurev-cellbio-111315-125306
40. Lewis SM, Williams A, Eisenbarth SC. Structure and function of the immune system in the spleen. Sci Immunol (2019) 4:eaau6085. doi: 10.1126/sciimmunol.aau6085
41. Mebius RE, Kraal G. Structure and function of the spleen. Nat Rev Immunol (2005) 5:606–16. doi: 10.1038/nri1669
42. Nolte MA, Beliën JAM, SChadee-Eestermans I, Jansen W, Unger WWJ, van Rooijen N, et al. A conduit system distributes chemokines and small blood-borne molecules through the splenic white pulp. J Exp Med (2003) 198:505–12. doi: 10.1084/jem.20021801
43. Förster R, Schubel A, Breitfeld D, Kremmer E, Renner-Müller I, Wolf E, et al. CCR7 coordinates the primary immune response by establishing functional microenvironments in secondary lymphoid organs. Cell (1999) 99:23–33. doi: 10.1016/S0092-8674(00)80059-8
44. Gunn MD, Kyuwa S, Tam C, Kakiuchi T, Matsuzawa A, Williams LT, et al. Mice lacking expression of secondary lymphoid organ chemokine have defects in lymphocyte homing and dendritic cell localization. J Exp Med (1999) 189:451–60. doi: 10.1084/jem.189.3.451
45. Gunn MD, Ngo VN, Ansel KM, Ekland EH, Cyster JG, Williams LT. A B-cell-homing chemokine made in lymphoid follicles activates Burkitt’s lymphoma receptor-1. Nature (1998) 391:799–803. doi: 10.1038/35876
46. Ansel KM, Ngo VN, Hyman PL, Luther SA, Förster R, Sedgwick JD, et al. A chemokine-driven positive feedback loop organizes lymphoid follicles. Nature (2000) 406:309–14. doi: 10.1038/35018581
47. Krautler NJ, Kana V, Kranich J, Tian Y, Perera D, Lemm D, et al. Follicular dendritic cells emerge from ubiquitous perivascular precursors. Cell (2012) 150:194–206. doi: 10.1016/j.cell.2012.05.032
48. Endres R, Alimzhanov MB, Plitz T, Fütterer A, Kosco-Vilbois MH, Nedospasov SA, et al. Mature follicular dendritic cell networks depend on expression of lymphotoxin β Receptor by radioresistant stromal cells and of lymphotoxin β and tumor necrosis factor by B cells. J Exp Med (1999) 189:159–68. doi: 10.1084/jem.189.1.159
49. Ngo VN, Korner H, Gunn MD, Schmidt KN, Sean Riminton D, Cooper MD, et al. Lymphotoxin α/β and tumor necrosis factor are required for stromal cell expression of homing chemokines in B and T cell areas of the spleen. J Exp Med (1999) 189:403–12. doi: 10.1084/jem.189.2.403
50. Koopman G, Parmentier HK, Schuurman HJ, Newman W, Meijer CJ, Pals ST. Adhesion of human B cells to follicular dendritic cells involves both the lymphocyte function-associated antigen 1/intercellular adhesion molecule 1 and very late antigen 4/vascular cell adhesion molecule 1 pathways. J Exp Med (1991) 173:1297–304. doi: 10.1084/jem.173.6.1297
51. Allen CDC, Cyster JG. Follicular dendritic cell networks of primary follicles and germinal centers: Phenotype and function. Semin Immunol (2008) 20:14–25. doi: 10.1016/j.smim.2007.12.001
52. Bengtén E, Wilson M, Miller N, Clem LW, Pilström L, Warr GW. “Immunoglobulin Isotypes: Structure, Function, and Genetics.,”. In: Du Pasquier L, Litman GW, editors. Origin and Evolution of the Vertebrate Immune System. Current Topics in Microbiology and Immunology. Berlin, Heidelberg: Springer (2000). p. 189–219. doi: 10.1007/978-3-642-59674-2_9
53. Luo Z-X, Yuan C-X, Meng Q-J, Ji Q. A Jurassic eutherian mammal and divergence of marsupials and placentals. Nature (2011) 476:442–5. doi: 10.1038/nature10291
54. Kumar S, Hedges SB. A molecular timescale for vertebrate evolution. Nature (1998) 392:917–20. doi: 10.1038/31927
55. Yasuda M, Taura Y, Yokomizo Y, Ekino S. A comparative study of germinal center: fowls and mammals. Comp Immunol Microbiol Infect Dis (1998) 21:179–89. doi: 10.1016/s0147-9571(98)00007-1
56. Yasuda M, Kajiwara E, Ekino S, Taura Y, Hirota Y, Horiuchi H, et al. Changes in surface Ig class expression in the chicken splenic germinal center after antigenic stimulation. Dev Comp Immunol (2003) 27:159–66. doi: 10.1016/S0145-305X(02)00066-6
57. White RG, French VI, Stark JM. A study of the localisation of A protein antigen in the chicken spleen and its relation to the formation of germinal centres. J Med Microbiol (1970) 3:65–83. doi: 10.1099/00222615-3-1-65
58. Del Cacho E, Gallego M, Lillehoj HS, López-Bernard F, Sánchez-Acedo C. Avian follicular and interdigitating dendritic cells: Isolation and morphologic, phenotypic, and functional analyses. Veterinary Immunol Immunopathol (2009) 129:66–75. doi: 10.1016/j.vetimm.2008.12.015
59. Higgins DA, Henry RR, Kounev ZV. Duck immune responses to Riemerella anatipestifer vaccines. Dev Comp Immunol (2000) 24:153–67. doi: 10.1016/S0145-305X(99)00070-1
60. Higgins DA, Ko OK, Chan SW. Duck antibody responses to keyhole limpet haemocyanin, human immunoglobulin G and the trinitrophenyl hapten. Evidence affinity maturation. Avian Pathol (2001) 30:381–90. doi: 10.1080/03079450120066386
61. Svendsen Bollen L, Crowley A, Stodulski G, Hau J. Antibody production in rabbits and chickens immunized with human IgG A comparison of titre and avidity development in rabbit serum, chicken serum and egg yolk using three different adjuvants. J Immunol Methods (1996) 191:113–20. doi: 10.1016/0022-1759(96)00010-5
62. Härtle S, Magor KE, Göbel TW, Davison F, Kaspers B. “Chapter 6 - Structure and Evolution of Avian Immunoglobulins.,”. In: Schat KA, Kaspers B, Kaiser P, editors. Avian Immunology (Second Edition). Boston: Academic Press (2014). p. 103–20. doi: 10.1016/B978-0-12-396965-1.00006-6
63. Leceta J, Zapata AG. White pulp compartments in the spleen of the turtle Mauremys caspica. Cell Tissue Res (1991) 266:605–13. doi: 10.1007/BF00318603
64. Kroese FGM, Leceta J, Döpp EA, Herraez MP, Nieuwenhuis P, Zapata A. Dendritic immune complex trapping cells in the spleen of the snake, Pythonreticulatus. Dev Comp Immunol (1985) 9:641–52. doi: 10.1016/0145-305X(85)90029-1
65. Tanaka Y, Hirahara Y. Spleen of the snake (Elaphe climacophora) and intrasplenic vascular architecture. J Morphol (1995) 226:223–35. doi: 10.1002/jmor.1052260209
66. Hussein MF, Badir N, El Ridi R, Akef M. Lymphoid tissues of the snake, Spalerosophis diadema, in the different seasons. Dev Comp Immunol (1979) 3:77–88. doi: 10.1016/S0145-305X(79)80008-7
67. Hussein MF, Badir N, El Ridi R, Akef M. Differential effect of seasonal variation on lymphoid tissue of the lizard, Chalcides ocellatus. Dev Comp Immunol (1978) 2:297–309. doi: 10.1016/S0145-305X(78)80072-X
68. Pitchappan R, Muthukkaruppan V. Thymus-dependent lymphoid regions in the spleen of the lizard, Calotes versicolor. J Exp Zool (1977) 199:177–88. doi: 10.1002/jez.1401990203
69. Borysenko M, Cooper EL. Lymphoid tissue in the snapping turtle, Chelydra serpentina. J Morphol (1972) 138:487–97. doi: 10.1002/jmor.1051380408
70. Grey HM. Phylogeny of the immune response: studies on some physical chemical and serologic characteristics of antibody produced in the turtle1 2. J Immunol (1963) 91:819–25. doi: 10.4049/jimmunol.91.6.819
71. Herbst LH, Klein PA. Monoclonal antibodies for the measurement of class-specific antibody responses in the green turtle, Chelonia mydas. Veterinary Immunol Immunopathol (1995) 46:317–35. doi: 10.1016/0165-2427(94)05360-5
72. Origgi FC, Romero CH, Bloom DC, Klein PA, Gaskin JM, Tucker SJ, et al. Experimental transmission of a herpesvirus in greek tortoises (Testudo graeca). Vet Pathol (2004) 41:50–61. doi: 10.1354/vp.41-1-50
73. Ujvari B, Madsen T. Do natural antibodies compensate for humoral immunosenescence in tropical pythons? Funct Ecol (2011) 25:813–7.
74. Marchalonis JJ, Ealey EH, Diener E. Immune response of the Tuatara, Sphenodon punctatum. Aust J Exp Biol Med Sci (1969) 47:367–80. doi: 10.1038/icb.1969.40
75. Ingram GA, Molyneux DH. The humoral immune response of the spiny-tailed agamid lizard (Agama caudospinosum) to injection with Leishmania agamae promastigotes. Veterinary Immunol Immunopathol (1983) 4:479–91. doi: 10.1016/0165-2427(83)90008-9
76. Kanakambika P, Muthukkaruppan VR. The immune response to sheep erythrocytes in the lizard calotes versicolor1. J Immunol (1972) 109:415–9. doi: 10.4049/jimmunol.109.3.415
77. Sandmeier FC, Tracy CR, Dupré S, Hunter K. A trade-off between natural and acquired antibody production in a reptile: implications for long-term resistance to disease. Biol Open (2012) 1:1078–82. doi: 10.1242/bio.20122527
78. Pettinello R, Dooley H. The immunoglobulins of cold-blooded vertebrates. Biomolecules (2014) 4:1045–69. doi: 10.3390/biom4041045
79. Magadán-Mompó S, Sánchez-Espinel C, Gambón-Deza F. IgH loci of American alligator and saltwater crocodile shed light on IgA evolution. Immunogenetics (2013) 65:531–41. doi: 10.1007/s00251-013-0692-y
80. Gambón-Deza F, Espinel CS. IgD in the reptile leopard gecko. Mol Immunol (2008) 45:3470–6. doi: 10.1016/j.molimm.2008.02.027
81. Manning MJ, Horton JD. Histogenesis of lymphoid organs in larvae of the South African clawed toad, Xenopus laevis (Daudin). Development (1969) 22:265–77. doi: 10.1242/dev.22.2.265
82. Neely HR, Guo J, Flowers EM, Criscitiello MF, Flajnik MF. “Double-duty” conventional dendritic cells in the amphibian Xenopus as the prototype for antigen presentation to B cells. Eur J Immunol (2018) 48:430–40. doi: 10.1002/eji.201747260
83. Manning MJ. The effect of early thymectomy on histogenesis of the lymphoid organs in Xenopus laevis. J Embryol Exp Morphol (1971) 26:219–29.
84. Marr S, Morales H, Bottaro A, Cooper M, Flajnik M, Robert J. Localization and Differential Expression of Activation-Induced Cytidine Deaminase in the Amphibian Xenopus upon Antigen Stimulation and during Early Development1. J Immunol (2007) 179:6783–9. doi: 10.4049/jimmunol.179.10.6783
85. Du Pasquier L, Robert J, Courtet M, Mußmann R. B-cell development in the amphibian Xenopus. Immunol Rev (2000) 175:201–13. doi: 10.1111/j.1600-065X.2000.imr017501.x
86. Mussmann R, Courtet M, Schwager J, Du Pasquier L. Microsites for immunoglobulin switch recombination breakpoints from Xenopus to mammals. Eur J Immunol (1997) 27:2610–9. doi: 10.1002/eji.1830271021
87. Zarrin AA, Alt FW, Chaudhuri J, Stokes N, Kaushal D, Du Pasquier L, et al. An evolutionarily conserved target motif for immunoglobulin class-switch recombination. Nat Immunol (2004) 5:1275–81. doi: 10.1038/ni1137
88. Ichikawa HT, Sowden MP, Torelli AT, Bachl J, Huang P, Dance GSC, et al. Structural phylogenetic analysis of activation-induced deaminase function12. J Immunol (2006) 177:355–61. doi: 10.4049/jimmunol.177.1.355
89. Baldwin WM, Cohen N. A giant cell with dendritic cell properties in spleens of the anuran amphibian Xenopus laevis. Dev Comp Immunol (1981) 5:461–73. doi: 10.1016/s0145-305x(81)80058-4
90. Baldwin WM. Antigen trapping cells in xenopus laevis: Tissue distribution. Dev Comp Immunol (1983) 7:709–10. doi: 10.1016/0145-305X(83)90101-5
91. Horton JD, Manning MJ. Effect of early thymectomy on the cellular changes occurring in the spleen of the clawed toad following administration of soluble antigen. Immunology (1974) 26:797–807.
92. Maniero GD, Morales H, Gantress J, Robert J. Generation of a long-lasting, protective, and neutralizing antibody response to the ranavirus FV3 by the frog Xenopus. Dev Comp Immunol (2006) 30:649–57. doi: 10.1016/j.dci.2005.09.007
93. Gantress J, Maniero GD, Cohen N, Robert J. Development and characterization of a model system to study amphibian immune responses to iridoviruses. Virology (2003) 311:254–62. doi: 10.1016/S0042-6822(03)00151-X
94. Ramsey JP, Reinert LK, Harper LK, Woodhams DC, Rollins-Smith LA. Immune Defenses against Batrachochytrium dendrobatidis, a Fungus Linked to Global Amphibian Declines, in the South African Clawed Frog, Xenopus laevis. Infection Immun (2010) 78:3981–92. doi: 10.1128/IAI.00402-10
95. Near TJ, Eytan RI, Dornburg A, Kuhn KL, Moore JA, Davis MP, et al. Resolution of ray-finned fish phylogeny and timing of diversification. Proc Natl Acad Sci U.S.A. (2012) 109:13698–703. doi: 10.1073/pnas.1206625109
96. Lamers CHJ, De Haas MJH. Antigen localization in the lymphoid organs of carp (Cyprinus carpio). Cell Tissue Res (1985) 242:491–8. doi: 10.1007/BF00225413
97. Fänge R, Nilsson S. The fish spleen: structure and function. Experientia (1985) 41:152–8. doi: 10.1007/BF02002607
98. Fournier-Betz V, Quentel C, Lamour F, Leven A. Immunocytochemical detection of Ig-positive cells in blood, lymphoid organs and the gut associated lymphoid tissue of the turbot (Scophthalmus maximus). Fish Shellfish Immunol (2000) 10:187–202. doi: 10.1006/fsim.1999.0235
99. Herraez MP, Zapata AG. Structure and function of the melano-macrophage centres of the goldfishCarassius auratus. Veterinary Immunol Immunopathol (1986) 12:117–26. doi: 10.1016/0165-2427(86)90116-9
100. Vogelbein WK, Fournie JW, Overstreet RM. Sequential development and morphology of experimentally induced hepatic melano-macrophage centres in Rivulus marmoratus. J Fish Biol (1987) 31:145–53. doi: 10.1111/j.1095-8649.1987.tb05306.x
101. Widdicombe M, Power C, Van Gelderen R, Nowak BF, Bott NJ. Relationship between Southern Bluefin Tuna, Thunnus maccoyii, melanomacrophage centres and Cardicola spp. (Trematoda: Aporocotylidae) infection. Fish Shellfish Immunol (2020) 106:859–65. doi: 10.1016/j.fsi.2020.09.004
102. Ziegenfuss MC, Wolke RE. The use of fluorescent microspheres in the study of piscine macrophage aggregate kinetics. Dev Comp Immunol (1991) 15:165–71. doi: 10.1016/0145-305X(91)90007-L
103. Meseguer J, López-Ruiz A, Esteban MA. Melano-macrophages of the seawater teleosts, sea bass (Dicentrarchus labrax) and gilthead seabream (Sparus aurata): morphology, formation and possible function. Cell Tissue Res (1994) 277:1–10. doi: 10.1007/BF00303074
104. Secombes CJ, Manning MJ, Ellis AE. Localization of immune complexes and heat-aggregated immunoglobulin in the carp Cyprinus carpio L. Immunology (1982) 47:101–5.
105. Secombes CJ, Manning MJ. Comparative studies on the immune system of fishes and amphibians: antigen localization in the carp Cyprinus carpio L. J Fish Dis (1980) 3:399–412. doi: 10.1111/j.1365-2761.1980.tb00424.x
106. Saunders HL, Oko AL, Scott AN, Fan CW, Magor BG. The cellular context of AID expressing cells in fish lymphoid tissues. Dev Comp Immunol (2010) 34:669–76. doi: 10.1016/j.dci.2010.01.013
107. Waly D, Muthupandian A, Fan C-W, Anzinger H, Magor BG. Immunoglobulin VDJ repertoires reveal hallmarks of germinal centers in unique cell clusters isolated from zebrafish (Danio rerio) lymphoid tissues(2022) (Accessed April 25, 2023).
108. Steinel NC, Bolnick DI. Melanomacrophage centers as a histological indicator of immune function in fish and other poikilotherms. Front Immunol (2017) 8:827. doi: 10.3389/fimmu.2017.00827
109. Munang’andu HM, Evensen Ø.. Correlates of protective immunity for fish vaccines. Fish Shellfish Immunol (2019) 85:132–40. doi: 10.1016/j.fsi.2018.03.060
110. Fillatreau S, Six A, Magadan S, Castro R, Sunyer JO, Boudinot P. The astonishing diversity of ig classes and B cell repertoires in teleost fish. Front Immunol (2013) 4:28. doi: 10.3389/fimmu.2013.00028
111. Zhang Y-A, Salinas I, Li J, Parra D, Bjork S, Xu Z, et al. IgT, a primitive immunoglobulin class specialized in mucosal immunity. Nat Immunol (2010) 11:827–35. doi: 10.1038/ni.1913
112. Wu L, Fu S, Yin X, Leng W, Guo Z, Wang A, et al. Affinity maturation occurs in channel catfish (Ictalurus punctaus) following immunization with a T-cell dependent antigen. Fish Shellfish Immunol (2019) 84:781–6. doi: 10.1016/j.fsi.2018.10.057
113. Craig Findly R, Zhao X, Noe J, Camus AC, Dickerson HW. B cell memory following infection and challenge of channel catfish with Ichthyophthirius multifiliis. Dev Comp Immunol (2013) 39:302–11. doi: 10.1016/j.dci.2012.08.007
114. Kurath G, Garver KA, Corbeil S, Elliott DG, Anderson ED, LaPatra SE. Protective immunity and lack of histopathological damage two years after DNA vaccination against infectious hematopoietic necrosis virus in trout. Vaccine (2006) 24:345–54. doi: 10.1016/j.vaccine.2005.07.068
115. Ye J, Kaattari IM, Kaattari SL. The differential dynamics of antibody subpopulation expression during affinity maturation in a teleost. Fish Shellfish Immunol (2011) 30:372–7. doi: 10.1016/j.fsi.2010.11.013
116. Star B, Nederbragt AJ, Jentoft S, Grimholt U, Malmstrøm M, Gregers TF, et al. The genome sequence of Atlantic cod reveals a unique immune system. Nature (2011) 477:207–10. doi: 10.1038/nature10342
117. Malmstrøm M, Matschiner M, Tørresen OK, Star B, Snipen LG, Hansen TF, et al. Evolution of the immune system influences speciation rates in teleost fishes. Nat Genet (2016) 48:1204–10. doi: 10.1038/ng.3645
118. Ghorbani A, Khataeipour SJ, Solbakken MH, Huebert DNG, Khoddami M, Eslamloo K, et al. Ancestral reconstruction reveals catalytic inactivation of activation-induced cytidine deaminase concomitant with cold water adaption in the Gadiformes bony fish. BMC Biol (2022) 20:293. doi: 10.1186/s12915-022-01489-8
119. Roth O, Solbakken MH, Tørresen OK, Bayer T, Matschiner M, Baalsrud HT, et al. Evolution of male pregnancy associated with remodeling of canonical vertebrate immunity in seahorses and pipefishes. Proc Natl Acad Sci (2020) 117:9431–9. doi: 10.1073/pnas.1916251117
120. Swann JB, Holland SJ, Petersen M, Pietsch TW, Boehm T. The immunogenetics of sexual parasitism. Science (2020) 369:1608–15. doi: 10.1126/science.aaz9445
121. Andreev PS, Sansom IJ, Li Q, Zhao W, Wang J, Wang C-C, et al. Spiny chondrichthyan from the lower Silurian of South China. Nature (2022) 609:969–74. doi: 10.1038/s41586-022-05233-8
122. Irisarri I, Baurain D, Brinkmann H, Delsuc F, Sire J-Y, Kupfer A, et al. Phylotranscriptomic consolidation of the jawed vertebrate timetree. Nat Ecol Evol (2017) 1:1370–8. doi: 10.1038/s41559-017-0240-5
123. Zapata A g., Torroba M, Sacedón R, Varas A, Vicente A. Structure of the lymphoid organs of elasmobranchs. J Exp Zool (1996) 275:125–43. doi: 10.1002/(SICI)1097-010X(19960601/15)275:2/3<125::AID-JEZ6>3.0.CO;2-F
124. Rumfelt LL, McKinney EC, Taylor E, Flajnik MF. The development of primary and secondary lymphoid tissues in the nurse shark Ginglymostoma cirratum: B-cell zones precede dendritic cell immigration and T-cell zone formation during ontogeny of the spleen. Scand J Immunol (2002) 56:130–48. doi: 10.1046/j.1365-3083.2002.01116.x
125. Castro CD, Ohta Y, Dooley H, Flajnik MF. Noncoordinate expression of J-chain and Blimp-1 define nurse shark plasma cell populations during ontogeny. Eur J Immunol (2013) 43:3061–75. doi: 10.1002/eji.201343416
126. Kinlein A, Janes ME, Kincer J, Almeida T, Matz H, Sui J, et al. Analysis of shark NCR3 family genes reveals primordial features of vertebrate NKp30. Immunogenetics (2021) 73:333–48. doi: 10.1007/s00251-021-01209-6
127. Matz H, Taylor RS, Redmond AK, Hill TM, Ruiz Daniels R, Beltran M, et al. Organized B cell sites in cartilaginous fishes reveal the evolutionary foundation of germinal centers. Cell Rep (2023) 42(7):112664. doi: 10.1016/j.celrep.2023.112664
128. Matz H, Munir D, Logue J, Dooley H. The immunoglobulins of cartilaginous fishes. Dev Comp Immunol (2021) 115:103873. doi: 10.1016/j.dci.2020.103873
129. Diaz M, Velez J, Singh M, Cerny J, Flajnik MF. Mutational pattern of the nurse shark antigen receptor gene (NAR) is similar to that of mamMalian Ig genes and to spontaneous mutations in evolution: the translesion synthesis model of somatic hypermutation. Int Immunol (1999) 11:825–33. doi: 10.1093/intimm/11.5.825
130. Heath WR, Kato Y, Steiner TM, Caminschi I. Antigen presentation by dendritic cells for B cell activation. Curr Opin Immunol (2019) 58:44–52. doi: 10.1016/j.coi.2019.04.003
131. Chappell CP, Draves KE, Giltiay NV, Clark EA. Extrafollicular B cell activation by marginal zone dendritic cells drives T cell–dependent antibody responses. J Exp Med (2012) 209:1825–40. doi: 10.1084/jem.20120774
132. Lu E, Dang EV, McDonald JG, Cyster JG. Distinct oxysterol requirements for positioning naïve and activated dendritic cells in the spleen. Sci Immunol (2017) 2:eaal5237. doi: 10.1126/sciimmunol.aal5237
133. Garg AK, Mitra T, Schips M, Bandyopadhyay A, Meyer-Hermann M. Amount of antigen, T follicular helper cells and affinity of founder cells shape the diversity of germinal center B cells: A computational study. Front Immunol (2023) 14:1080853. doi: 10.1101/2022.10.26.513835
134. Martínez-Riaño A, Wang S, Boeing S, Minoughan S, Casal A, Spillane KM, et al. Long-term retention of antigens in germinal centers is controlled by the spatial organization of the follicular dendritic cell network. Nat Immunol (2023) 24(8):1281–94. doi: 10.1038/s41590-023-01559-1
135. Bisht K, te Velthuis AJW. Decoding the role of temperature in RNA virus infections. mBio (2022) 13:e02021–22. doi: 10.1128/mbio.02021-22
136. Franke F, Armitage SAO, Kutzer MAM, Kurtz J, Scharsack JP. Environmental temperature variation influences fitness trade-offs and tolerance in a fish-tapeworm association. Parasites Vectors (2017) 10:252. doi: 10.1186/s13071-017-2192-7
137. Thomas MB, Blanford S. Thermal biology in insect-parasite interactions. Trends Ecol Evol (2003) 18:344–50. doi: 10.1016/S0169-5347(03)00069-7
138. Hsu E. Mutation, selection, and memory in B lymphocytes of exothermic vertebrates. Immunol Rev (1998) 162:25–36. doi: 10.1111/j.1600-065X.1998.tb01426.x
139. Chen H, Smith GJD, Zhang SY, Qin K, Wang J, Li KS, et al. Avian flu: H5N1 virus outbreak in migratory waterfowl. Nature (2005) 436:191–2. doi: 10.1038/nature03974
140. Tong S, Li Y, Rivailler P, Conrardy C, Castillo DAA, Chen L-M, et al. A distinct lineage of influenza A virus from bats. Proc Natl Acad Sci (2012) 109:4269–74. doi: 10.1073/pnas.1116200109
141. Parry R, Wille M, Turnbull OMH, Geoghegan JL, Holmes EC. Divergent influenza-like viruses of amphibians and fish support an ancient evolutionary association. Viruses (2020) 12:1042. doi: 10.3390/v12091042
142. Longo NS, Lipsky PE. Why do B cells mutate their immunoglobulin receptors? Trends Immunol (2006) 27:374–80. doi: 10.1016/j.it.2006.06.007
143. Abbott RK, Crotty S. Factors in B cell competition and immunodominance. Immunol Rev (2020) 296:120–31. doi: 10.1111/imr.12861
144. Alsoussi WB, Malladi SK, Zhou JQ, Liu Z, Ying B, Kim W, et al. SARS-CoV-2 Omicron boosting induces de novo B cell response in humans. Nature (2023) 617(7961):592–8. doi: 10.1038/s41586-023-06025-4
145. Abreu RB, Kirchenbaum GA, Clutter EF, Sautto GA, Ross TM. Preexisting subtype immunodominance shapes memory B cell recall response to influenza vaccination. JCI Insight (2020) 5(1):e132155. doi: 10.1172/jci.insight.132155
146. Cirelli KM, Carnathan DG, Nogal B, Martin JT, Rodriguez OL, Upadhyay AA, et al. Slow delivery immunization enhances HIV neutralizing antibody and germinal center responses via modulation of immunodominance. Cell (2019) 177:1153–1171.e28. doi: 10.1016/j.cell.2019.04.012
Keywords: germinal center, antibody, affinity maturation, secondary lymphoid organs, B cells, T follicular helper cells, follicular dendritic cells, evolution
Citation: Matz H and Dooley H (2023) 450 million years in the making: mapping the evolutionary foundations of germinal centers. Front. Immunol. 14:1245704. doi: 10.3389/fimmu.2023.1245704
Received: 23 June 2023; Accepted: 25 July 2023;
Published: 11 August 2023.
Edited by:
Chris Hauton, University of Southampton, United KingdomReviewed by:
Susana Magadan, University of Vigo, SpainSofia Kossida, Université de Montpellier, France
Copyright © 2023 Matz and Dooley. This is an open-access article distributed under the terms of the Creative Commons Attribution License (CC BY). The use, distribution or reproduction in other forums is permitted, provided the original author(s) and the copyright owner(s) are credited and that the original publication in this journal is cited, in accordance with accepted academic practice. No use, distribution or reproduction is permitted which does not comply with these terms.
*Correspondence: Hanover Matz, bWF0ekB3dXN0bC5lZHU=
†Present address: Hanover Matz, Department of Pathology and Immunology, Washington University School of Medicine, St Louis, MO, United States