- Department of Cardiopulmonary Bypass, Beijing Anzhen Hospital, Capital Medical University, Beijing, China
Acute respiratory distress syndrome (ARDS) is an acute inflammatory lung injury characterized by diffuse alveolar damage. The period prevalence of ARDS was 10.4% of ICU admissions in 50 countries. Although great progress has been made in supportive care, the hospital mortality rate of severe ARDS is still up to 46.1%. Moreover, up to now, there is no effective pharmacotherapy for ARDS and most clinical trials focusing on consistently effective drugs have met disappointing results. Mesenchymal stem cells (MSCs) and their derived extracellular vesicles (EVs) have spawned intense interest of a wide range of researchers and clinicians due to their robust anti-inflammatory, anti-apoptotic and tissue regeneration properties. A growing body of evidence from preclinical studies confirmed the promising therapeutic potential of MSCs and their EVs in the treatment of ARDS. Based on the inspiring experimental results, clinical trials have been designed to evaluate safety and efficacy of MSCs and their EVs in ARDS patients. Moreover, trials exploring their optimal time window and regimen of drug administration are ongoing. Therefore, this review aims to present an overview of the characteristics of mesenchymal stem cells and their derived EVs, therapeutic mechanisms for ARDS and research progress that has been made over the past 5 years.
1 Introduction
Acute respiratory distress syndrome (ARDS) is an acute respiratory failure caused by various pathogenic factors from pulmonary and non-pulmonary sources, among which pneumonia, aspiration of gastric contents and sepsis are the most common causes (1). The histopathological feature of ARDS is diffuse alveolar damage characterized by increased alveolocapillary permeability and hyaline-membrane formation due to an uncontrolled inflammatory response mediated by innate immune cells and their derived proinflammatory cytokines, also known as a “cytokine storm” in the lungs (1, 2). ARDS is one of the most severe complications of COVID-19, an emerging infectious disease triggered by the novel severe acute respiratory syndrome coronavirus 2 (SARS-CoV-2). It has been reported that approximately 42% patients with COVID-19 pneumonia develop ARDS (3). However, due to limited medical resources for ARDS diagnosis in some low-income countries and the fact that many patients with diffuse lung injury supported with high-flow nasal cannula do not meet the ARDS Berlin definition, the incidence of ARDS appears to be underestimated (1, 2). Although great progress has been made in supportive care and ventilator management, the mortality rate among ICU and hospital patients with ARDS is still high, reaching up to 35.3% and 40.0%, respectively (4). COVID-19 ARDS seems to have a higher mortality rate compared to ARDS from other causes (5). Other than that, survivors of ARDS often experience long-term complications, including functional impairments such as muscle wasting and weakness, as well as neurocognitive disorders, which significantly compromise their quality of life (QOL) (6–8). Thus, ARDS has been a major health problem and a difficult area of clinical treatment that need to be addressed all over the world. However, at present, there is no effective pharmacotherapy available for ARDS worldwide, and the treatment is primarily symptomatic and supportive with lung-protective ventilation, fluid-conservative management strategy and prone positioning despite the intense research (9). Although various pharmacotherapies, such as surfactant replacement, β-agonists, inhaled nitric oxide, statins and activated protein C, etc. have shown promise in animal models and preclinical researches, translating these findings into effective therapies for human ARDS has proven challenging and most clinical trials focusing on consistently effective drugs have met disappointing results (7). For patients with moderate to severe ARDS, when conventional treatment approaches failed to improve their clinical conditions, extracorporeal membrane oxygenation (ECMO) is considered as a rescue therapy and can serve as a bridge to recovery (10).
In recent years, mesenchymal stem cells (MSCs) as a cell-based therapy for various inflammatory diseases due to their potent anti-inflammatory, anti-apoptotic and tissue regeneration properties had spawned intense interest of a wide range of researchers and clinicians. A growing body of evidence highlights the potential benefits of stem cell-based therapy for ARDS (11). Animal models have demonstrated that MSCs possess lung protective capacities through suppressing inflammatory responses and attenuating pulmonary edema (12–14). Furthermore, preliminary clinical trials also have displayed encouraging therapeutic outcomes of MSCs for ARDS. However, stem cell-based therapy exists some worrying issues, for example, low stability, the risk of pulmonary embolism, and tumor formation (15, 16). Recent studies have revealed that the anti-inflammatory effect of MSCs is mainly achieved via paracrine pathway (17). The MSCs secretome such as extracellular vesicles (EVs) has been confirmed to be the main mediators of their therapeutic attributes (18). Compared with their original stem cells, EVs possess several attractive features like targeted delivery, lower immunogenicity and tumorigenicity, easy storage, high stability, and ability to cross blood barriers. Therefore, a large number of preclinical studies on the treatment of ARDS with MSCs-derived EVs have emerged recent years. Animal experimental results were exciting. And several relevant clinical studies are also ongoing.
This review aims to make an overview about the characteristics of MSCs and their derived EVs, therapeutic mechanisms for ARDS and research progress that has been made over the past 5 years. We will not focus on the pathogenesis of ARDS in this review, as it has been extensively covered in other published reviews (1, 2, 19). Finally, we will briefly summarize the possible future directions of MSCs and their derived EVs for ARDS therapy.
2 Overview of mesenchymal stem cells and their derived extracellular vesicles
Stem cell-based therapy has become a hotspot in the field of regenerative medicine. Among various types of stem cells, MSCs are evoking the most extraordinary interest due to their advantages of simplicity of isolation, low immunogenicity and tumorigenicity, high proliferation rate and free of ethical issues (11, 20–22). Additionally, EVs secreted by MSCs have the same effects as their parental cells. In this section, we will provide an overview about the characteristics of MSCs and their derived EVs, shedding light on their potential in inflammatory diseases (Figure 1).
2.1 Mesenchymal stem cells
The concept of MSCs originated from the non-hematopoietic stem cells present in bone marrow (BM) (23). Researches on MSCs can be traced to 1960s, when Tavassoli and Crosby clearly established the proof of bone and marrow generation in de novo potential of BM transplanted to extramedullary site (23, 24). Subsequently, a series of seminal studies conducted by Friedenstein and his colleagues demonstrated that only a small subset of BM cells was responsible for the osteogenic potential of BM allograft (25). Along with the development of hematopoietic stem cell research, the groundbreaking work from Tavasolli, Friedenstein, and Owen revealed possible existence of a second type of stem cell in bone marrow (23). The term “mesenchymal stem cells” was first coined by Caplan in 1991 (26). However, it was not until 1999 that the term gained widespread popularity (23, 27).
MSCs are considered as a heterogeneous subpopulation of adult multipotent cells with self-renewing and differentiation potential (28). MSCs show a fibroblast-like morphology under the microscope. According to the minimal criteria to define human MSCs proposed by the International Society for Cellular Therapy in 2006, MSCs must meet the following three criteria: 1) plastic adherence in standard culture conditions; 2) expression of specific surface antigens such as CD105, CD73, and CD90, but lacking expression of CD45, CD34, CD14 or CD11b, CD79α or CD19, and HLA-DR; 3) trilineage differentiation into osteocytes, chondrocytes and adipocytes in vitro (29). In addition to bone marrow, MSCs can also be isolated from the other multiple tissues, including adipose tissue (30), placenta (31), umbilical cord (32), Wharton’s jelly (33), dental pulp (34), periodontal ligament (35), synovial membrane (36), periosteum (37), skin (38), skeletal muscle (39), tonsil (40), amniotic fluid (41), uterine cervix (42), peripheral blood (43), among which bone marrow-derived mesenchymal stem cells (BM-MSCs) are studied most extensively (44).
MSCs have been shown to be a promising therapy for various diseases such as autoimmune disease (45), graft-versus-host disease (GvHD) (46), inflammatory disease (47), neurodegenerative disorder (48), cardiovascular disease (49), wound healing repair (50) and diabetes mellitus (51) due to their tissue repair and immunomodulatory properties (21, 52). One of the important properties that make MSCs play therapeutic role in diseases mentioned above is their capacity of migration to injured or inflammatory sites when injected systematically, which is called the homing effect (44, 53). Migration of MSCs occurs in response to a large amount of chemotactic factors, including both growth factors (GFs) and chemokines generated in the damaged tissues, such as platelet-derived growth factor-AB (PDGF-AB), insulin-like growth factor 1 (IGF-1), RANTES (regulated upon activation, normal T-cell expressed and secreted), macrophage-derived chemokine (MDC), and stromal-derived factor-1 (SDF-1) (54, 55). Meanwhile, a myriad of relevant receptors expressed on MSCs membranes have been detected, including tyrosine kinase receptors PDGF-receptor (R) α, PDGF-Rβ, and IGF-R and chemokine receptors CCR3, CCR4, CCR5, CXCR4 (55). Later, the surface proteins podocalyxin-like protein (PODXL) and α6-integrin (CD49f) highly expressed in the early cultures of MSCs have been found to enhance their migration to injured tissues after systemic administration (56). Adhesion molecules and integrins such as VCAM-1 and VLA-4 contribute to the migration of MSCs to the inflammatory sites as well (57). In targeted location, MSCs can mediate productive repair by secreting bioactive factors with the capacity of anti-apoptotic, immunomodulatory and angiogenic effects and interacting with injured host cells (12, 52, 58–60). Another reason for the widespread use of MSCs is its robust immunomodulatory property. MSCs exert immunomodulatory effects by suppressing innate and adaptive immune responses, which involve a variety of immune cells. MSCs have been demonstrated to not only interfere with all major stages of the dendritic cells (DCs) life cycle: differentiation, maturation, and activation, resulting in the formation of immature DCs with an inhibitory phenotype but also alter the cytokine secretion profile of DCs, as evidenced by decreased secretion of tumor necrosis factor α (TNF-α) and interleukin-2 (IL-2) and increased IL-10 secretion (17, 61, 62). In addition, MSCs inhibit the cytotoxic effects of natural killer (NK) cells and interferon γ (IFN-γ) secretion. They also modulate immune responses bysuppressing T lymphocyte activation and proliferation. MSCs can induce T-cell anergy and promote the formation of regulatory T cells (Tregs). They enable CD4+ T cells to generate a functional Treg population but suppress the activation, proliferation and differentiation of proinflammatory T cells during the CD4+ T cells induced to differentiate toward Th1 or Th17 cells (63). Furthermore, differentiation, antibody production, and chemotactic behavior of B cells are affected by MSCs as well (17). The exact mechanism underlying this immunosuppressive effect of MSCs is not well understood, but it is believed to involve soluble factors secreted by MSCs, such as prostaglandin E2 (PGE2) (61), tryptophan catabolizing enzyme indoleamine 2,3-dioxygenase (IDO) (64), nitric oxide (NO) (65) and transforming growth factor-β1 (TGF-β1) (66). Notably, there are complex interactions between antigen-presenting cells (APCs), adaptive immune cells, and MSCs. APCs, especially blood CD14+ monocytes, play a critical role in activating MSCs through the release of IL-1β, which then enables MSCs to inhibit T lymphocytes (66).
The mechanism of MSC-mediated immunosuppression varies among different species. For instance, a study demonstrated that MSCs from human and non-human primates mediated immunosuppression by IDO whereas MSCs from mouse by NO (67). Moreover, the immunosuppressive ability of MSCs may not be inherent, but rather is elicited in the presence of proinflammatory cytokines and is chemokine-dependent (46, 67). When exposed to certain combinations of cytokines, MSCs produce high levels of inducible nitric oxide synthases (iNOS) and chemokines which drive immune cells migration into proximity to MSCs, where immune cell function can be suppressed by the high levels of NO (46). Proinflammatory signals activated MSCs to secret PGE2 and TNFα stimulated gene/protein 6 (TSG-6), both of which are negative feedback loops of inflammation, leading to a shift in macrophages from the M1 phenotype (proinflammatory) to the M2 phenotype (anti-inflammatory) (68). MSCs can also be activated by microenvironment generated by injured tissues to express factors that appear to specifically meet the needs of the tissues (69). Special storage conditions, low cell engraftment and poor survival in the lung, and cell senescence during in vitro expansion are the main obstacles limiting MSCs clinical translation (15, 20, 70, 71). However, several strategies have been developed to optimize MSCs therapy in ARDS, such as genetic modification and pretreatment with a series of preconditioning strategies (20), which will be discussed in detail in the section of functional optimization of MSCs and their derived EVs.
2.2 MSC-derived extracellular vesicles
It is now widely accepted that the therapeutic potential of MSCs is largely attributed to their secretome, especially their derived EVs. EVs are nanometer sized particles secreted by almost all cell types. Previously, EVs were generally classified into exosomes, microvesicles, and apoptotic bodies according to their size and intracellular origin (18). The Minimal Information for Studies of Extracellular Vesicles 2018 (MISEV2018) guidelines updated the nomenclature of EVs since there has not yet consensus on specific markers of EVs subtypes. Therefore, based on the physical characteristics such as size, EVs can be categorized into small EVs (sEVs) with a diameter <100nm or 200nm, and medium/large EVs (m/lEVs) with a diameter>200nm (72). MSC-derived EVs express some specific surface molecules reflecting the cells of origin, such as CD29, CD73, CD44, and CD105 (18). EVs have been considered to participate in the intercellular communication via delivering their contents into the recipient cells, including proteins, RNA, DNA, amino acids, lipids, metabolites, and even organelles, changing the function and/or phenotype of the recipient cells under the physiological and pathological processes (73, 74). For example, insulin-like growth factor-1 receptor (IGF-1R) mRNA contained within MSC-derived EVs could be transferred to tubular cells, where it was translated in the corresponding protein, resulting in increased cell proliferation (75). EVs originated from MSCs not only exhibit similar or even superior behavior and function as MSCs but also overcome the disadvantages of live cell injection (76). As a promising cell-free therapeutic approach, MSC-derived EVs have many compelling advantages like high stability and biocompatibility, easy storage, no risk of iatrogenic tumor formation, low immunogenicity and easy to cross the biological barrier compared to their parent cells (77, 78). Over 150 miRNAs and 850 proteins have been identified from cargos of MSCs-derived EVs, which contribute to their therapeutic efficacy in a wide range of pathological conditions, including cardiovascular disease, neurological disease, acute kidney injury, liver injury and inflammatory lung diseases (74, 78–83). miRNAs are small non-coding RNAs enriched in EVs that can be internalized into the target cells and subsequently interact with specific binding sites to alter behavior of cells in physiology or pathology conditions. In recent years, with the deepening of researches on contents of MSC-derived EVs, multiple cytokines, growth factors, and prostaglandins found in EVs have been revealed to mediate immuno-modulatory properties (84).
MSC-derived EVs inherit many of the properties of their parental MSCs in the treatment of inflammatory diseases, such as immunomodulation, homing effect and tissue repair functions. After intravenous injection, MSC-derived EVs can also migrate to the injury and inflammatory sites, which may be attributed to the increased vascular permeability at the site of inflammation (76, 83, 85). As with MSCs, MSC-derived EVs display a similar immunomodulatory ability through interacting with various effector cells of the immune system (84). Co-culture of MSC-derived EVs with peripheral blood mononuclear cells (PBMCs) in vitro reduces PBMC proliferation (83, 86). MSC-derived EVs also modulate CD4+ T cell subsets, as evidenced by suppressing T cell induction to Th1/Th17 subtypes, while enhancing Tregs level and function significantly (83, 87–89). The proliferation, activation, and apoptosis of B lymphocytes are also inhibited (90). MSC-derived EVs can induce macrophage polarization towards the anti-inflammatory M2 phenotype (91). Moreover, it was reported that the proliferation, activation, and cytotoxicity of NK cells were inhibited by human fetal liver (FL) MSC-derived EVs (92). The underlying mechanism by which MSC-derived EVs regulate the function of CD4+ T cells and NK cells may involve TGFβ signaling (92, 93). Nevertheless, MSC-derived EVs promote Tregs immunosuppression capacity not by contacting with CD4+ T cells directly, but by triggering the polarization of T cells through interacting with APCs such as monocytes and B cells in PBMCs, which may be the mediator between MSC-derived EVs and Tregs (88). Specific miRNAs contained in MSC-derived EVs, such as miR-191, have been identified to inhibit immune response through interacting with specific proteins, such as death-associated protein kinase 1 (DAPK1) after being transferred to macrophages (94). Phinney et al. demonstrated that MSCs modulated macrophage function by transfer of partially depolarized mitochondria and regulatory microRNAs employing two different types of EVs, which enhanced macrophage bioenergetics and suppressed Toll-like receptor (TLR) signaling, respectively (95). Proinflammatory stimuli can enhance the immunosuppressive functions of MSC-derived EVs, in which multiple anti-inflammatory miRNAs, such as miR-146, miR-34, miR-21 were strongly upregulated under the stimulation of inflammatory cytokines and transferred to macrophages, resulting in M2 polarization (83, 96, 97). In addition to miRNAs, deep RNA sequencing and proteomic analysis of MSC-derived EVs revealed that several mRNAs and proteins with anti-inflammatory properties were highly enriched in cytokine-stimulated EVs compared to non-stimulated EVs (83). Anti-inflammatory mRNAs and proteins of particular importance include IDO mRNA, macrophage inhibitory cytokine 1 (MIC-1), Galectin-1 (Gal-1), and latent-transforming growth factor β-binding protein (LTBP) (83). This may explain its potent anti-inflammatory effects and therapeutic potential in various inflammatory diseases. Furthermore, some chemokines and chemokine receptors such as CCL2, VEGFC, and CCL20 carried in MSC-derived EVs can recruit immune cells to the vicinity of EVs and subsequently induce immunosuppressive effects (98). At the same time, the complex composition of EVs allows them to target different pathogenic pathways and act synergistically to enhance their therapeutic function (83).
2.3 Differences between MSCs and their derived EVs
It has been reported that MSCs were initially trapped by the lung and gradually accumulated in the liver and other organs after intravenous infusion, which might be due to the cellular diameter and attachment potential, while intravenously administered EVs are predominantly distributed in the spleen followed by the liver, and then the lungs and kidneys (99, 100). Regarding to the homing capability, MSCs are considered to migrate towards damaged or inflammatory sites in response to chemokines produced at the site of injury and chemokine receptors expressed on their own surface (101), while MSC-derived EVs appears to exhibit an inherent tendency towards injured tissues (102). MSC-derived EVs have many advantages over MSCs in treating various inflammatory diseases. EVs are nanoscale particles with smaller diameter, which means virtually no risk of microvascular obstruction, especially pulmonary capillaries. This feature also allows them to reach target locations faster to execute their tissue repair functions (101, 103). Besides, MSCs require special storage conditions (liquid nitrogen), while EVs are more stable and can be stored at -80°C for a long time. Amplifying stem cells in vitro with inappropriate processes and culture conditions may increase their immunogenicity (104). In contrast, EVs are poorly immunogenic, which enables them to prevent therapeutic cargoes from rapid degradation in the body (99). And MSC-derived EVs have increased circulating half-life (105). Moreover, MSC-derived EVs have the ability to cross the blood-tissue barrier (101). Despite these advantages, there are still many technical challenges to overcome in the process of clinical translation of MSC-derived EVs, which will be elaborated in the sixth part.
3 Role of mesenchymal stem cells and their derived EVs in pathophysiology of ARDS
Increasing evidence have revealed good therapeutic prospects of MSCs and their derived EVs for ALI/ARDS. They play a protective role by intervening in multiple pathogenic pathways. This part aims to make an overview of the underlying mechanisms (Figure 2).
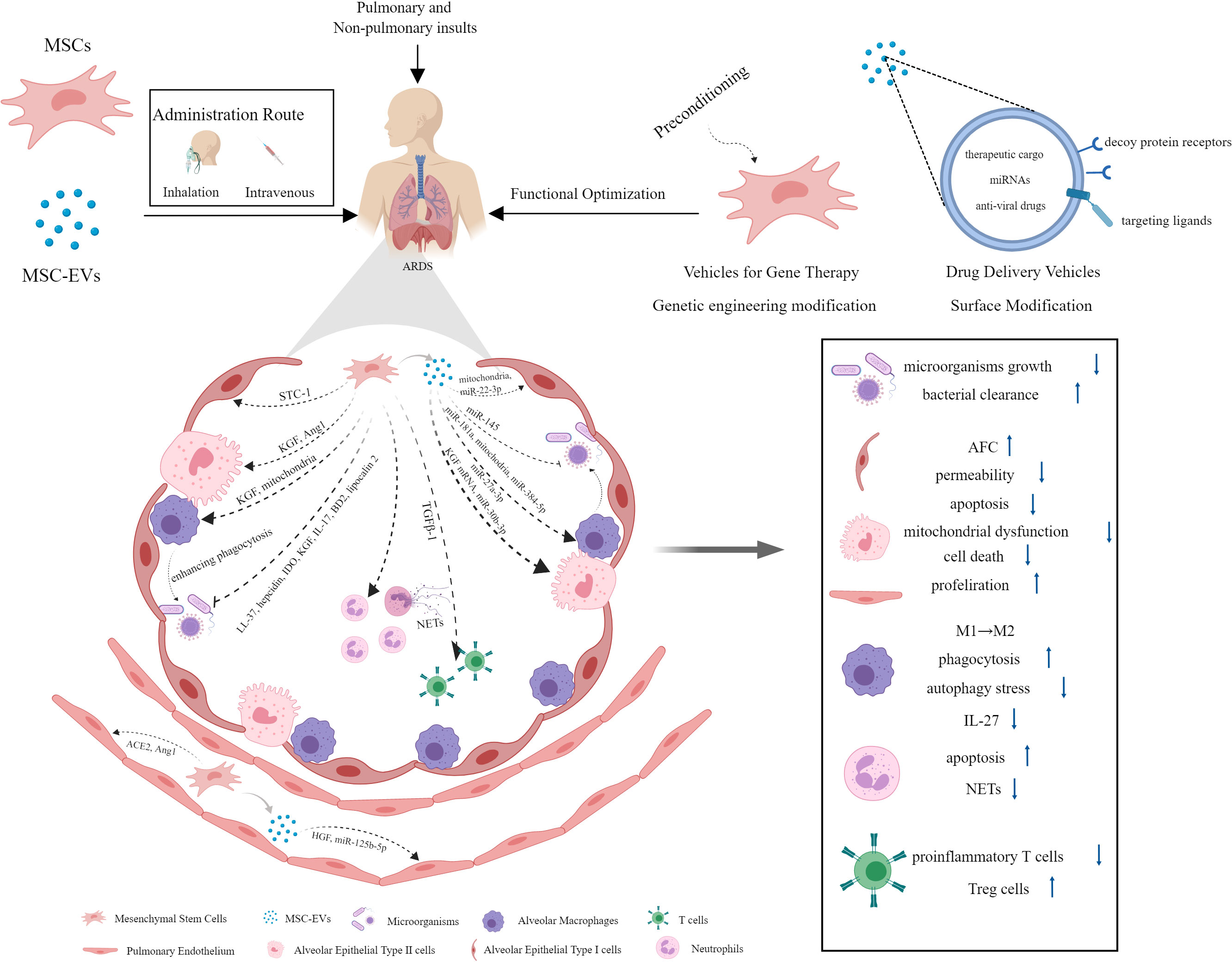
Figure 2 The mechanisms underlying the protective effect of MSCs and their derived EVs against ARDS.
3.1 Antimicrobial
Current data have suggested that MSCs and their EVs exert strong antimicrobial effects through indirect and direct mechanisms (106, 107). MSCs could inhibit a range of clinically relevant pathogenic microorganisms growth directly in vivo and in vitro, which was mediated in part by the secretion of antimicrobial factors, such as human cathelicidin antimicrobial peptide hCAP-18/LL-37 and hepcidin (108, 109), IDO (110), keratinocyte growth factor (KGF) (111), interleukin-17 (IL-17) (112), and β-defensin 2 (BD2) (113). In a mouse model of Gram-negative pneumonia, murine MSCs have been found to enhance bacterial clearance from the lung due to the upregulation of antibacterial protein lipocalin 2 in response to lipopolysaccharide (LPS) and inflammatory mediators (114). Besides that, the antimicrobial activity of MSCs could be achieved indirectly by enhancing alveolar macrophage (AM) phagocytosis against bacteria and the survival of blood monocytes partly through the secretion of KGF and transferring mitochondria to innate immune cells (111, 115). Macrophages of healthy individuals and those with sepsis that are exposed to MSCs have stronger ability of bacterial phagocytosis and killing (116). It has been revealed that MSC-EVs can enhance bacterial clearance in E. coli pneumonia mice and ex vivo perfused human lung models of E. coli pneumonia as well (117). The mechanisms underlying their antimicrobial activity could ascribe to the increased leukotriene (LT) B4 production in injured alveolus mediated by decreased multidrug resistance-associated protein 1 (MRP1) expression resulting from the transfer of miR-145 by MSC-EVs (118). The anti-microbial effect of MSC-EVs was also partly due to the enhancement of human monocytes and alveolar macrophages phagocytic capacity against bacteria (107, 117).
3.2 Restoration of alveolar-capillary barrier integrity
One of the pathological manifestations presented by ARDS patients is alveolar edema, which is ascribed to increased lung endothelial barrier permeability and reduced alveolar fluid clearance (AFC) (119). AFC is predominately dependent on the function of alveolar epithelial fluid transport. In experiments with perfused human lungs, treatment with human MSCs or MSC conditioned medium (MSC-CM) normalized AFC and restored lung endothelial permeability induced by E. coli endotoxin, thus improving pulmonary edema via secretion of paracrine soluble factor KGF in large part, which might traffick epithelial sodium channels subunit (αENaC) to the apical membrane of alveolar epithelial type II (AT II) cells (120). The researchers also found that human MSC-EVs were as effective as their parent stem cells in reducing lung protein permeability and inflammation in E. coli endotoxin-induced ALI and pneumonia in C57BL/6 mice, which might be due to the increased level of KGF mediated by expression of the mRNA from MSC MVs in the injured alveolus (107, 121). Similar findings were obtained in a study using an ex vivo perfused human lung injured with bacterial pneumonia, which showed increased AFC and reduced lung protein permeability following MSC-EVs administration (117). The presence of hepatocyte growth factor (HGF) in MSC-EVs has been verified to stabilize pulmonary endothelial barrier function, as evidenced by reduced endothelium permeability and apoptosis rate, as well as increased cell proliferation in LPS-induced endothelial cell model (122). Alveolar epithelium forms a tighter monolayer than the endothelium. Epithelial barrier dysfunction in ARDS facilitates accumulation of protein-rich edema fluid and inflammatory cells in alveolar cavity. Previous study noted that allogeneic human MSCs restored epithelial protein permeability across human AT II cells injured by cytomix (a combination of IL-1β, TNF-α, and IFN γ). This beneficial effect on human AT II cells was mediated by angiopoietin-1 (Ang1) release from MSCs to prevent actin stress fiber formation and claudin 18 disorganization following an inflammatory insult in part through suppression of NF-κB activity (123). MSC-EVs may also play a protective role in ARDS through mitochondria transfer. In the ARDS microenvironment, MSC-EVs could restore alveolar-capillary barrier integrity at least partially via mitochondrial transfer to rescue mitochondrial dysfunction (124).
3.3 Immunomodulatory
At present, the most widely recognized pathogenesis of ARDS is excessive and uncontrolled inflammatory response in the lung. As a promising cell-based therapy candidates, MSCs and their derived EVs hold great potential for their ability to modulate/suppress innate and adaptive immune systems in the treatment to various inflammatory disease, including ARDS (125). MSCs harvested from different tissues and their EVs could increase survival rate and attenuate systemic and pulmonary inflammation in animals suffering from lung injury induced by various insults, whether administered systemically or intratracheally (13, 54, 121, 126–130). During the early phase of ARDS, neutrophils are recruited into the alveolar space in response to the chemokines released by macrophages and epithelial cells, activated excessively and undergo delayed apoptosis. Nevertheless, neutrophil apoptosis is essential for the resolution of ARDS. The study found that administration of MSC-CM attenuated LPS-induced ALI by inducing neutrophil apoptosis, which might be related to the inhibition of NF-κB signalling pathway (131). Neutrophil extracellular traps (NETs) are structures formed by dying neutrophils as a novel defence mechanism against bacterial infections. However, excessive NETs formation is associated with exacerbated immune responses and causes damage to lung tissue (132). Treatment with MSCs could reduce the oxidative stress and release of NETs, thereby alleviating lung injury and improving survival in LPS-induced ALI of mice (133). Cholinergic anti-inflammatory pathway (CAP) activation has been identified as a critical mechanism underlying the therapeutic effects of MSCs on ARDS, which is mediated by MSC-derived PGE2 (134). MSCs-derived EVs carrier multiple cargoes, in which miRNAs are extensively studied and considered to modulate gene expression and function of target cells under pathological conditions. In a rat model of traumatic ALI, miR-124-3p transferred by MSC-derived EVs could improve oxidative stress injury and inhibit inflammatory response through directly targeting and downregulating purinergic receptor P2X ligand-gated ion channel 7 (P2X7), which was overexpressed in traumatic ALI rats (128). Macrophages are key innate immune cells responsible for ARDS inflammatory response. Recent study revealed that the highly expressed miR-181a in MSC-EVs in the ARDS environment was crucial for the EVs immunomodulatory effect on human macrophages exposed to LPS as well as the mouse model of LPS-induced lung injury via PTEN-pSTAT5-SOCS1 axis (135). A study conducted by Wang et al. demonstrated that MSC-EVs could alleviate ALI via transfer of miR-27a-3p to alveolar macrophages and promoting M2 macrophage polarization (136). Apart from miRNA, mitochondrial components packed in MSC-derived EVs are increasingly attracting researchers interests and widely studied in recent years. Human MSCs could enhance phagocytic capacity and promote anti-inflammatory phenotype in human macrophages stimulated with LPS or ARDS bronchoalveolar lavage fluid (BALF) via EVs mitochondrial transfer (137). Furthermore, EVs from adipose-derived mesenchymal stem cells (AdMSCs) could restore metabolic homeostasis of macrophages and promote a functional shift of LPS-stimulated macrophages from M1 proinflammatory to M2 anti-inflammatory phenotype via transferring mitochondrial components (138). Cytokine IL-27 has been identified as a pro-inflammatory factor in the pathology of sepsis, which is primarily released by dendritic cells and macrophages (139). It was revealed that IL-27 in BALF and serum was elevated in ALI/ARDS patients and associated with disease severity. Blocking IL-27 with neutralizing antibodies could reduce pulmonary inflammation and improve survival rate in a mouse model of cecal ligation and puncture (CLP)-induced lung injury. From this, IL-27 is a critical cytokine in ALI/ARDS and inhibition of IL-27 may represent a promising therapy for ALI/ARDS patients (140). Recent study revealed that EVs from adipose-derived MSCs could alleviate sepsis-induced lung injury in mice by inhibiting the secretion of IL-27 in macrophages (141). Metabolic pathways of macrophages are closely related to their activation state and function. M1 macrophages rely on glycolysis for energy production whereas M2 macrophages rely on oxidative phosphorylation (OXPHOS). Metabolism shift between glycolysis and OXPHOS is involved in M1/M2 macrophage polarization (142). It was reported that EVs derived from BMSCs could attenuate LPS-induced ARDS by modulating alveolar macrophage polarization through inhibiting cellular glycolysis (143). However, it should be noted that not all MSCs exhibit immunosuppression properties. Bone marrow MSCs contain different functional subpopulations, in which IL-17+ MSCs possess antifungal effect but fail to execute MSC-based immunosuppression owing to the downregulation of TGF-β mediated by the NF-κB pathway (112).
3.4 Inhibition of cell death
It is well-known that extensive apoptosis of the endothelial and alveolar epithelial cells is involved in the pathogenesis of ARDS patients, leading to alveolo-capillary barrier dysfunction and disability of lung edema fluid clearance by alveolar epithelial cells (2, 144–147). MSCs could be activated to reduce apoptosis in UV-irradiated fibroblasts and lung cancer epithelial cells, which may be explained in part by the upregulation and secretion of stanniocalcin-1 (STC-1) (148). Exosomal miR-22-3p from human umbilical cord blood-derived MSCs could suppress inflammatory reaction and pulmonary cell apoptosis rate by reducing frizzled class receptor 6 (FDZ6) in LPS-induced ALI, which further benefited from the upregulated miR-22-3p (149). Ferroptosis is a novel and iron-dependent programmed cell death (PCD) modality (150). Excessive ferroptosis contributed to intestinal ischemia/reperfusion-induced ALI (151). A study revealed that specific delivery of miR-125b-5p by adipose derived stem cells (ADSCs) EVs could alleviate the inflammation induced pulmonary microvascular endothelial cells (PMVECs) ferroptosis in sepsis induced ALI via regulating Keap1/Nrf2/GPX4 expression, thereby improve the acute lung injury (152).
3.5 Modulating autophagy
Autophagy is a highly conserved lysosomal degradation pathway that primarily functions as an adaptive role to maintain homeostasis of organisms at both basal and stress-induced increase levels (153). Diverse stimuli including bacterial infection, LPS exposure, sepsis, and mechanical ventilation, etc. have been shown to promote autophagy in multiple lung cell types and animal models of lung injury (154). However, the exact role of autophagy in ALI still remains controversial. Autophagy may play a protective or detrimental role in ALI depending on the underlying causes of lung injury and the stages of disease progression (154). Changes in autophagic flux caused by autophagy dysfunction may underlie acute lung injury during sepsis (155). It was discovered that BMSC-derived EVs could relieve LPS-induced acute lung injury through inhibiting autophagy stress in alveolar macrophages via delivering miR-384-5p to combine with Beclin-1 directly (156).
4 Functional optimization of MSCs and their derived EVs
Priming MSCs with various agents or methods was found to further improve their biological function and therapeutic efficacy (157). A study noted that ischemic preconditioning of MSCs for 60 min could potentiate their treatment effect on endotoxin-induced ALI (158). Hypoxic preconditioning promoted migration and survival capacity of MSCs through upregulating LincRNA-p21 (159). Data from Tsoyi et al. suggested that preconditioning with carbon monoxide improved efficacy of MSCs during sepsis by production of specialized proresolving lipid mediators (160). Administrating staphylococcal enterotoxin B (SEB)-preconditioned murine MSCs intravenously could also improve survival rates and bacterial clearance in the peritoneal fluids, blood and organs in a mouse model of sepsis (161). Additionally, sublethal exposure to LPS enhanced MSCs survival, antibacterial activity, and immune regulatory properties in septic mice (162). Moreover, pretreatment with cytokines further enhanced the capacity of MSCs to facilitate injury resolution following ventilator-induced lung injury (VILI) and pulmonary epithelial wound healing via a KGF-dependent mechanism (163). In addition to therapeutic effects presented by MSCs per se, MSCs can also serve as a vehicle for gene therapy to attenuate ALI/ARDS (Figure 2). Overexpression of various bioactive factors in MSCs was confirmed as an effective way to optimize their treatment capacity. Overexpression of TGFβ-1 in murine MSCs could improve lung inflammation and attenuate lung injuries by modulating the imbalance of Th17/Treg in LPS-induced ARDS mice (164). The expression of angiotensin-converting enzyme 2 (ACE2) protein decreased significantly in LPS-induced ALI mice, while treatment with MSCs overexpressing ACE2 further improved lung endothelial function and mitigated lung inflammatory response compared with MSCs alone (165). The administration of MSCs overexpressing the Ang1 gene greatly reduced pulmonary vascular endothelial permeability and inflammatory reaction in the lung after LPS exposure, which might be mediated by the down-regulation of TNF-α gene expression (166). The study by Wang et al. have introduced a novel MSC-based delivery system. They removed the nuclei of MSCs using density gradient centrifugation and genetically engineered them with homing molecules (CCR2, CXCR4, and PSGL-1) to enhance their tissue-specific homing properties. The results showed that these modified MSCs improved the delivery of anti-inflammatory cytokine IL-10 to target tissue compared to normal MSCs and control EVs, thereby alleviating inflammation and pancreatitis (167).
As an important mediator involved in intracellular communication, EVs derived from miR-30b-3p-overexpressing MSCs could inhibit the apoptosis of type II alveolar epithelial cells (ACEs) and protect against ALI in mice after LPS delivery through transferring miR-30b-3p to the recipient ACEs to downregulate the expression of serum amyloid A3 (SAA3), which was a target gene of miR-30b-3p and significantly up-regulated in ALI (144). MSC-derived EVs exhibit therapeutic effects on ALI/ARDS not only by inherent properties but also as natural drug delivery nano-carriers due to their specific structure. The methods of loading the therapeutic cargo into the EVs include active and passive encapsulation (77). Moreover, several approaches are used for EVs surface modification to make them a valid target-specific drug delivery system, including genetic engineering, covalent and non-covalent modification, among which genetic engineering of EV producing cells using plasmid vectors is a widely used approach for producing surface modified EVs (168). Gupta and his colleagues simultaneously constructed two different decoy protein receptors for the pro-inflammatory TNF-α and IL-6 on the surface of EVs from HEK293T cells and MSCs using an optimized surface-display technology. The engineered EVs were observed to be able to ameliorate systemic inflammation in three different inflammation models through inhibiting IL-6 and TNF-α signalling pathways (169). The construction of this decoy EVs may provide a new direction for ALI/ARDS therapy. In the context of COVID-19, EV-based therapy optimization strategies include screening and incorporating a set of miRNAs that can specifically bind to the coronavirus genome into EVs to destroy the virus, and combining the therapeutic properties of MSC-EVs with loading anti-viral drugs (77, 170).
5 Therapeutic effects of mesenchymal stem cells and their derived EVs on ARDS patients
Due to the promising therapeutic effect of MSCs and their derived EVs on ALI/ARDS exhibited in animal models, increasing clinical trials have been designed to examine their safety and efficacy in ALI/ARDS patients (Tables 1, 2). A small sample size, randomized, placebo-controlled pilot study published in 2014 explored the safety and efficacy of allogeneic adipose-derived MSCs on ARDS. The results showed that systemic administration of allogeneic adipose-derived MSCs appeared to be safe and feasible in the treatment of ARDS. Levels of serum surfactant protein D (SP-D), an acute lung injury biomarker, were significantly lower at day 5 compared to day 0. However, the dosage of MSCs used in this clinical trial might be required to be optimized to improve efficacy (171). The STem cells for ARDS Treatment (START) trial, a phase 1 clinical trial conducted by Matthay et al., demonstrated that a single intravenous MSCs infusion was well tolerated in patients with moderate to severe ARDS, and no MSC-related adverse events were reported (172). In a randomized phase 2a safety trial as part of the START study, 40 patients with moderate to severe ARDS received either MSCs or placebo. The trial found no MSC-related predefined hemodynamic or respiratory adverse events, and there was no significantly difference in mortality between the MSC group and the placebo group (173). The larger scale clinical trials will be necessary to further assess the safety and efficacy of MSCs for ARDS treatment. However, the clinical application of MSCs is limited by their safety concerns, cell survivability, scalability and regulatory issues (174). MSC-derived EVs, as a novel, multitargeted, next generation biological agent and key paracrine factor, along with their superior safety profile, stability, and scalability, have emerged as a practical treatment option (174). Clinical trials aimed at exploring the safety and efficacy of MSC-EVs in the treatment of ARDS are currently underway (NCT04602104, NCT05354141). Patients infected with SARS-CoV-2 may develop ARDS in severe cases. Accumulating evidence demonstrated that patients with severe COVID-19 suffered from the hyperinflammatory syndrome or cytokine storm syndrome, characterized by hypercytokinaemia. Premised upon the antimicrobial and robust immunosuppressive property of MSCs and their derived EVs, they might inhibit SARS-CoV-2 theoretically and the resulting host inflammatory reaction (170). Recent studies have found that MSCs and their EVs are also effective in the treatment of COVID-19. An observational pilot study reported that the intravenous transplantation of MSCs improved the outcomes of patients with severe COVID-19 pneumonia (175). A small sample size randomized controlled trial aiming to determine safety and explore efficacy of MSCs infusion in subjects with COVID-19 ARDS have also demonstrated beneficial effect (176). A phase II randomized, multicenter clinical trial conducted by Zarrabi et al. enrolled 43 patients with COVID-19 induced ARDS, who were allocated into two intervention groups (MSC alone group, and MSC plus EV group) and a control group. Patients in MSC alone group received two consecutive doses of allogenic MSCs intravenously at a dose of 100×106 ± 10%, while those in MSC plus EV group received one dose of MSCs (100×106 ± 10% cells) intravenously followed by one dose of MSC-EVs (isolated from the 200×106 ± 10% cells) through respiratory inhalation. There was an interval of 48 h between the first dose and the second dose in both groups. The results indicated that the systemic administration of MSCs and nebulized inhalation of MSC-EVs were safe and could significantly reduce the serum levels of inflammatory markers in COVID-19 patients with ARDS (177). However, results from a recently published randomized controlled trial including 222 patients with moderate to severe SARS-CoV-2-related ARDS indicated that intravenous infusion of MSCs, while safe, did not reduce 30-day mortality or improve 60-day ventilator-free days compared with placebo (178). In a prospective non-randomized open-label cohort study published in 2020, EVs derived from allogeneic bone marrow mesenchymal stem cells (ExoFlo™) were a safe, efficient, and promising therapeutic candidate for severe COVID-19 cases (174). This result was further supported by a randomized placebo-controlled dosing clinical trial. The study enrolled and randomized 102 patients with COVID-19 associated moderate-to-severe ARDS receiving 10 mL ExoFlo™, 15 mL ExoFlo™, or placebo on day 1 and 4. Although there was no statistically significant difference in all-cause 60-day mortality in the Intention-to-Treat (ITT) population, two doses of 15 mL ExoFlo™ safely reduced 60-day mortality rate as compared to the placebo in participants aged 18-65 with respiratory failure or moderate to severe ARDS using post-hoc subgroup analyses (179). Several randomized controlled trials designed to evaluate the safety and effectiveness of EVs in moderate to severe ARDS related to COVID-19 are now in progress (NCT05387278, NCT04798716, NCT04747574).
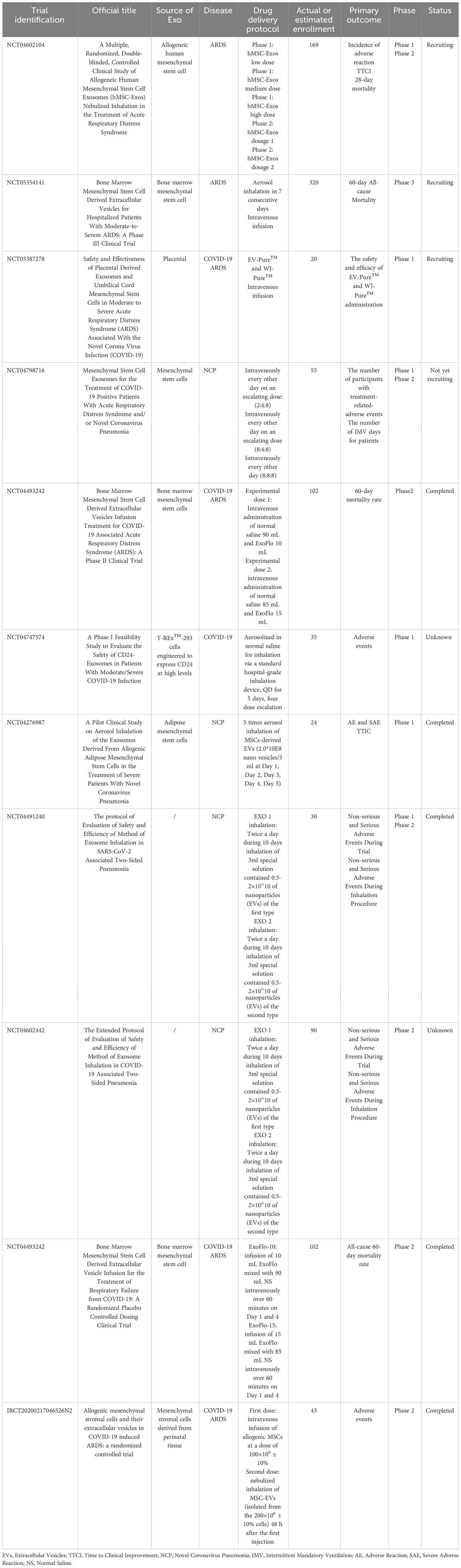
Table 2 Clinical Trials of the therapeutic effects of mesenchymal stem cell-derived EVs on ARDS patients.
Given that the results from published clinical trials have fallen short of expectations, it may be necessary to design larger scale, multicenter, and long-term follow-up clinical trials to assess the potential benefits of MSCs and their derived EVs for ARDS in the future. At present, two distinct subphenotypes of ARDS have been identified, termed hyperinflammatory and hypoinflammatory (180, 181). Different ARDS phenotypes and immune status of patients may affect the treatment response to MSCs and their derived EVs. Consequently, further studies are needed to determine whether there is a contrast in efficacy between patients with different ARDS phenotypes receiving MSCs and their derived EVs therapy. Reducing heterogeneity among enrolled patients with ARDS in clinical trials may also help improve the possible clinical benefits of MSCs and their derived EVs in the treatment of ARDS. Approaches to reduce heterogeneity in clinical trials include selecting patients for both phase 2 and 3 trials on the basis of physiological, radiographic, and biological criteria as well as exclusion of patients with significant comorbidities (182). With regard to COVID-19-induced ARDS, it has been demonstrated that aging may be associated with uncoordinated adaptive immune responses and poor disease outcomes (183). Therefore, higher doses or longer infusion of MSCs and their derived EVs may be required to achieve therapeutic benefit in the aged individuals. This aged-related dose-effect relationship should be further investigated in the future clinical trials. Additionally, the optimal administration route, dosage, frequency and time window for MSCs and MSC-EVs therapy are also issues that need to be addressed. Functionally optimized MSCs have not yet been applied in clinical trials, but in the future, it may be possible to attempt to apply functionally optimized MSCs and EVs to explore their therapeutic effects on ARDS.
6 Challenges and limitations
Despite numerous advantages, the clinical transformation and application of MSCs and their derived EVs remain to be facing many challenges and unsolved problems. One of the major hurdles is the technology of large-scale generation of MSCs. MSCs will gradually age and lose multipotency and proliferative capacities over continuous passages, which have a negative impact not only on the therapeutic effect themselves but also on the yield and quality of EVs. To overcome the limitation, one potential alternative is to derive MSCs from induced pluripotent stem cells (iPSCs) generated by somatic cell reprogramming. Compared with tissue-derived MSCs, iPSC-MSCs have shown superior proliferative capacity and immunomodulatory function even after 10 passages (184). Consequently, further investigations should focus on developing more efficient and cost-effective protocols for large-scale production of MSCs. Besides, heterogeneity of MSCs originated from different donors, cell isolation techniques, and cell culture environment also significantly influence the clinical application of MSCs, so it is imperative to strengthen the quality control of stem cell preparations (104). The yield, purity and quality control provision of EVs for clinical application remain a matter of much concern. At present, there are four main methods for isolating and purifying EVs: differential ultracentrifugation and density gradients centrifugation, size-exclusion-based method, precipitation, and immunoaffinity-based capture, among which differential ultracentrifugation is the most commonly used and reliable isolation method (185–187). This technique is simple and cost-effective, but also has many limitations, such as time-consuming procedure, non-vesicular macromolecule contamination and aggregation (186). Not only that, but huge differences in efficiency of EV recovery across research groups are reported (187). Nonspecific precipitation is an alternative method to sediment EVs using polyethylene glycol (PEG)-based solutions without the need for expensive ultracentrifuges. However, these sedimentation processes may not be appropriate for large scale production and may result in the co-isolation of nonexosomal vesicles (187). Using tangential-flow filtration to concentrate EVs based on their size is more promising than the sedimentation methods, yet the ultrafilters used are expensive and the co-isolation of material still exists (187). Immunoaffinity isolation, a method based on specific binding between antibodies and EV surface markers, seems to be the most promising method due to its superiority over ultracentrifugation in terms of product characterization and isolation (188). However, scaling up production when using this technology is still challenging. In summary, in the process of clinical translation, it is necessary to develop advanced and standardized platforms to improve EV purity and quantity. Combined sequential filtration with affinity-based chromatography may offer the best chance (187). Moreover, development of the optimal method for long-term preservation of EVs is also worthy of our attention (71). EVs are much more stable than MSCs at -80°C and can be stored for up to 1 year (77). Nevertheless, repeated freezing and thawing can lead to the EVs clustering and degradation of their cargo. Freeze drying formulations may be a good choice for preserving EVs at room temperature. A study noted that freeze-dried EVs performed better than the non-freeze-dried EVs both in terms of morphology and function (77).
7 Summary and future perspectives
The pathophysiology of ARDS involves multiple complex and overlapping mechanistic pathways, eventually triggering an exaggerated innate immune response in the lung, resulting in the diffuse alveolar damage characterized by neutrophilic alveolitis and hyaline membrane deposition (19, 189). As a multi-pathway targeted cell-based therapy, MSCs and their derived EVs show promise in the treatment of ALI/ARDS owing to their capacity of reducing lung vascular injury, restoring alveolar fluid clearance and switching the pro-inflammatory responses to a pro-resolving paradigm. However, despite promising preclinical and early clinical trial results, there is still a need for clinical studies with larger sample size and more rigorous design to clarify the role of MSC and their EVs in the treatment of ARDS. More importantly, current clinical trials have shown some variability in outcomes, which may be attributed to the heterogeneity of ARDS itself. Therefore, researchers should focus on and attempt to reduce the heterogeneity of ARDS in future clinical trials, which probably contributes to improve treatment outcomes.
ARDS is an inherently heterogeneous clinical syndrome, which can be categorized into subgroups based on clinical, physiological, radiographic imaging, and biological criteria (182). The clinical and biological heterogeneity of ARDS influences the responsiveness to treatment, which may explain why promising candidate therapies in preclinical experiments failed in human trials. Therefore, therapeutic discovery for ARDS requires new approaches that focus on the phenotypic and biological heterogeneity to target therapies toward specific subgroups of patients with ARDS (189). Precision medicine approach targeting patients on the basis of their molecular phenotypes of ARDS might help to develop more effective and individualized pharmacotherapies (190). Applying the platform trials, an innovative trial designs from other fields, to the study of ARDS can make more therapies tested in a time-efficient and cost-effective manner, and accelerate therapeutic discovery for ARDS (189). A deeper understanding of key nodes in mechanistic pathway involved in the pathogenesis of ARDS will facilitate the identification of therapeutic targets, ultimately reducing the mortality and morbidity in patients with ARDS.
Author contributions
XZ wrote the first draft of the manuscript. LF, LL, and ZD drew the figures and tables. YJ and XY supervised the work and provided the comments and additional scientific information. JZ and FH reviewed and revised the text. All authors contributed to the article and approved the submitted version.
Conflict of interest
The authors declare that the research was conducted in the absence of any commercial or financial relationships that could be construed as a potential conflict of interest.
Publisher’s note
All claims expressed in this article are solely those of the authors and do not necessarily represent those of their affiliated organizations, or those of the publisher, the editors and the reviewers. Any product that may be evaluated in this article, or claim that may be made by its manufacturer, is not guaranteed or endorsed by the publisher.
References
1. Thompson BT, Chambers RC, Liu KD. Acute respiratory distress syndrome. N Engl J Med (2017) 377(6):562–72. doi: 10.1056/NEJMra1608077
2. Meyer NJ, Gattinoni L, Calfee CS. Acute respiratory distress syndrome. Lancet (2021) 398(10300):622–37. doi: 10.1016/S0140-6736(21)00439-6
3. Wu C, Chen X, Cai Y, Xia J, Zhou X, Xu S, et al. Risk factors associated with acute respiratory distress syndrome and death in patients with coronavirus disease 2019 pneumonia in wuhan, China. JAMA Intern Med (2020) 180(7):934–43. doi: 10.1001/jamainternmed.2020.0994
4. Bellani G, Laffey JG, Pham T, Fan E, Brochard L, Esteban A, et al. Epidemiology, patterns of care, and mortality for patients with acute respiratory distress syndrome in intensive care units in 50 countries. JAMA (2016) 315(8):788–800. doi: 10.1001/jama.2016.0291
5. Gibson PG, Qin L, Puah SH. COVID-19 acute respiratory distress syndrome (ARDS): clinical features and differences from typical pre-COVID-19 ARDS. Med J Aust (2020) 213(2):54–6 e1. doi: 10.5694/mja2.50674
6. Herridge MS, Cheung AM, Tansey CM, Matte-Martyn A, Diaz-Granados N, Al-Saidi F, et al. One-year outcomes in survivors of the acute respiratory distress syndrome. N Engl J Med (2003) 348(8):683–93. doi: 10.1056/NEJMoa022450
7. Matthay MA, Zemans RL, Zimmerman GA, Arabi YM, Beitler JR, Mercat A, et al. Acute respiratory distress syndrome. Nat Rev Dis Primers (2019) 5(1):18. doi: 10.1038/s41572-019-0069-0
8. Hopkins RO, Weaver LK, Collingridge D, Parkinson RB, Chan KJ, Orme JF Jr. Two-year cognitive, emotional, and quality-of-life outcomes in acute respiratory distress syndrome. Am J Respir Crit Care Med (2005) 171(4):340–7. doi: 10.1164/rccm.200406-763OC
9. Lee JW, Fang X, Krasnodembskaya A, Howard JP, Matthay MA. Concise review: Mesenchymal stem cells for acute lung injury: role of paracrine soluble factors. Stem Cells (2011) 29(6):913–9. doi: 10.1002/stem.643
10. Rawal G, Yadav S, Kumar R. Acute respiratory distress syndrome: an update and review. J Transl Int Med (2018) 6(2):74–7. doi: 10.1515/jtim-2016-0012
11. Hayes M, Curley G, Ansari B, Laffey JG. Clinical review: Stem cell therapies for acute lung injury/acute respiratory distress syndrome - hope or hype? Crit Care (2012) 16(2):205. doi: 10.1186/cc10570
12. Curley GF, Hayes M, Ansari B, Shaw G, Ryan A, Barry F, et al. Mesenchymal stem cells enhance recovery and repair following ventilator-induced lung injury in the rat. Thorax (2012) 67(6):496–501. doi: 10.1136/thoraxjnl-2011-201059
13. Chimenti L, Luque T, Bonsignore MR, Ramirez J, Navajas D, Farre R. Pre-treatment with mesenchymal stem cells reduces ventilator-induced lung injury. Eur Respir J (2012) 40(4):939–48. doi: 10.1183/09031936.00153211
14. Xu F, Hu Y, Zhou J, Wang X. Mesenchymal stem cells in acute lung injury: are they ready for translational medicine? J Cell Mol Med (2013) 17(8):927–35. doi: 10.1111/jcmm.12063
15. Jung JW, Kwon M, Choi JC, Shin JW, Park IW, Choi BW, et al. Familial occurrence of pulmonary embolism after intravenous, adipose tissue-derived stem cell therapy. Yonsei Med J (2013) 54(5):1293–6. doi: 10.3349/ymj.2013.54.5.1293
16. Abraham A, Krasnodembskaya A. Mesenchymal stem cell-derived extracellular vesicles for the treatment of acute respiratory distress syndrome. Stem Cells Transl Med (2020) 9(1):28–38. doi: 10.1002/sctm.19-0205
17. Nauta AJ, Fibbe WE. Immunomodulatory properties of mesenchymal stromal cells. Blood (2007) 110(10):3499–506. doi: 10.1182/blood-2007-02-069716
18. Bruno S, Deregibus MC, Camussi G. The secretome of mesenchymal stromal cells: Role of extracellular vesicles in immunomodulation. Immunol Lett (2015) 168(2):154–8. doi: 10.1016/j.imlet.2015.06.007
19. Bos LDJ, Ware LB. Acute respiratory distress syndrome: causes, pathophysiology, and phenotypes. Lancet (2022) 400(10358):1145–56. doi: 10.1016/S0140-6736(22)01485-4
20. Qin H, Zhao A. Mesenchymal stem cell therapy for acute respiratory distress syndrome: from basic to clinics. Protein Cell (2020) 11(10):707–22. doi: 10.1007/s13238-020-00738-2
21. Nery AA, Nascimento IC, Glaser T, Bassaneze V, Krieger JE, Ulrich H. Human mesenchymal stem cells: from immunophenotyping by flow cytometry to clinical applications. Cytometry A (2013) 83(1):48–61. doi: 10.1002/cyto.a.22205
22. Golchin A, Seyedjafari E, Ardeshirylajimi A. Mesenchymal stem cell therapy for COVID-19: present or future. Stem Cell Rev Rep (2020) 16(3):427–33. doi: 10.1007/s12015-020-09973-w
23. Bianco P, Robey PG, Simmons PJ. Mesenchymal stem cells: revisiting history, concepts, and assays. Cell Stem Cell (2008) 2(4):313–9. doi: 10.1016/j.stem.2008.03.002
24. Tavassoli M, Crosby WH. Transplantation of marrow to extramedullary sites. Science (1968) 161(3836):54–6. doi: 10.1126/science.161.3836.54
25. Friedenstein AJ, Petrakova KV, Kurolesova AI, Frolova GP. Heterotopic of bone marrow. Analysis of precursor cells for osteogenic and hematopoietic tissues. Transplantation (1968) 6(2):230–47.
27. Pittenger MF, Mackay AM, Beck SC, Jaiswal RK, Douglas R, Mosca JD, et al. Multilineage potential of adult human mesenchymal stem cells. Science (1999) 284(5411):143–7. doi: 10.1126/science.284.5411.143
28. Zanoni M, Cortesi M, Zamagni A, Tesei A. The role of mesenchymal stem cells in radiation-induced lung fibrosis. Int J Mol Sci (2019) 20(16). doi: 10.3390/ijms20163876
29. Dominici M, Le Blanc K, Mueller I, Slaper-Cortenbach I, Marini F, Krause D, et al. Minimal criteria for defining multipotent mesenchymal stromal cells. The International Society for Cellular Therapy position statement. Cytotherapy (2006) 8(4):315–7. doi: 10.1080/14653240600855905
30. Zuk PA, Zhu M, Ashjian P, De Ugarte DA, Huang JI, Mizuno H, et al. Human adipose tissue is a source of multipotent stem cells. Mol Biol Cell (2002) 13(12):4279–95. doi: 10.1091/mbc.e02-02-0105
31. In ‘t Anker PS, Scherjon SA, Kleijburg-van der Keur C, de Groot-Swings GM, Claas FH, Fibbe WE, et al. Isolation of mesenchymal stem cells of fetal or maternal origin from human placenta. Stem Cells (2004) 22(7):1338–45. doi: 10.1634/stemcells.2004-0058
32. Erices A, Conget P, Minguell JJ. Mesenchymal progenitor cells in human umbilical cord blood. Br J Haematol (2000) 109(1):235–42. doi: 10.1046/j.1365-2141.2000.01986.x
33. Stone ML, Zhao Y, Robert Smith J, Weiss ML, Kron IL, Laubach VE, et al. Mesenchymal stromal cell-derived extracellular vesicles attenuate lung ischemia-reperfusion injury and enhance reconditioning of donor lungs after circulatory death. Respir Res (2017) 18(1):212. doi: 10.1186/s12931-017-0704-9
34. Gronthos S, Mankani M, Brahim J, Robey PG, Shi S. Postnatal human dental pulp stem cells (DPSCs) in vitro and in vivo. Proc Natl Acad Sci U.S.A. (2000) 97(25):13625–30. doi: 10.1073/pnas.240309797
35. Giacoppo S, Thangavelu SR, Diomede F, Bramanti P, Conti P, Trubiani O, et al. Anti-inflammatory effects of hypoxia-preconditioned human periodontal ligament cell secretome in an experimental model of multiple sclerosis: a key role of IL-37. FASEB J (2017) 31(12):5592–608. doi: 10.1096/fj.201700524R
36. De Bari C, Dell’Accio F, Tylzanowski P, Luyten FP. Multipotent mesenchymal stem cells from adult human synovial membrane. Arthritis Rheumatol (2001) 44(8):1928–42. doi: 10.1002/1529-0131(200108)44:8<1928::AID-ART331>3.0.CO;2-P
37. Debnath S, Yallowitz AR, McCormick J, Lalani S, Zhang T, Xu R, et al. Discovery of a periosteal stem cell mediating intramembranous bone formation. Nature (2018) 562(7725):133–9. doi: 10.1038/s41586-018-0554-8
38. Shih DT, Lee DC, Chen SC, Tsai RY, Huang CT, Tsai CC, et al. Isolation and characterization of neurogenic mesenchymal stem cells in human scalp tissue. Stem Cells (2005) 23(7):1012–20. doi: 10.1634/stemcells.2004-0125
39. Julien A, Kanagalingam A, Martínez-Sarrà E, Megret J, Luka M, Ménager M, et al. Direct contribution of skeletal muscle mesenchymal progenitors to bone repair. Nat Commun (2021) 12(1):2860. doi: 10.1038/s41467-021-22842-5
40. Lee KE, Choi DH, Joo C, Kang SW, Huh KM, Park YS. Octanoyl glycol chitosan enhances the proliferation and differentiation of tonsil-derived mesenchymal stem cells. Carbohydr Polym (2021) 264:117992. doi: 10.1016/j.carbpol.2021.117992
41. Nadri S, Soleimani M. Comparative analysis of mesenchymal stromal cells from murine bone marrow and amniotic fluid. Cytotherapy (2007) 9(8):729–37. doi: 10.1080/14653240701656061
42. Sendon-Lago J, Seoane S, Martinez-Ordoñez A, Eiro N, Saa J, Vizoso FJ, et al. Corneal regeneration by conditioned medium of human uterine cervical stem cells is mediated by TIMP-1 and TIMP-2. Exp Eye Res (2019) 180:110–21. doi: 10.1016/j.exer.2018.12.004
43. Debosschere Y, Depuydt E, Pauwelyn G, Beerts C, Van Hecke L, Verhaert L, et al. Safety and immunomodulatory properties of equine peripheral blood-derived mesenchymal stem cells in healthy cats. Vet Immunol Immunopathol (2020) 227:110083. doi: 10.1016/j.vetimm.2020.110083
44. Fernandez-Francos S, Eiro N, Gonzalez-Galiano N, Vizoso FJ. Mesenchymal stem cell-based therapy as an alternative to the treatment of acute respiratory distress syndrome: current evidence and future perspectives. Int J Mol Sci (2021) 22(15). doi: 10.3390/ijms22157850
45. Zappia E, Casazza S, Pedemonte E, Benvenuto F, Bonanni I, Gerdoni E, et al. Mesenchymal stem cells ameliorate experimental autoimmune encephalomyelitis inducing T-cell anergy. Blood (2005) 106(5):1755–61. doi: 10.1182/blood-2005-04-1496
46. Ren G, Zhang L, Zhao X, Xu G, Zhang Y, Roberts AI, et al. Mesenchymal stem cell-mediated immunosuppression occurs via concerted action of chemokines and nitric oxide. Cell Stem Cell (2008) 2(2):141–50. doi: 10.1016/j.stem.2007.11.014
47. Shi Y, Wang Y, Li Q, Liu K, Hou J, Shao C, et al. Immunoregulatory mechanisms of mesenchymal stem and stromal cells in inflammatory diseases. Nat Rev Nephrol (2018) 14(8):493–507. doi: 10.1038/s41581-018-0023-5
48. Kim SG, George NP, Hwang JS, Park S, Kim MO, Lee SH, et al. Human bone marrow-derived mesenchymal stem cell applications in neurodegenerative disease treatment and integrated omics analysis for successful stem cell therapy. Bioengineering (Basel) (2023) 10(5). doi: 10.3390/bioengineering10050621
49. Bagno L, Hatzistergos KE, Balkan W, Hare JM. Mesenchymal stem cell-based therapy for cardiovascular disease: progress and challenges. Mol Ther (2018) 26(7):1610–23. doi: 10.1016/j.ymthe.2018.05.009
50. Guillamat-Prats R. The role of MSC in wound healing, scarring and regeneration. Cells (2021) 10(7). doi: 10.3390/cells10071729
51. Li H, Zhu H, Ge T, Wang Z, Zhang C. Mesenchymal stem cell-based therapy for diabetes mellitus: enhancement strategies and future perspectives. Stem Cell Rev Rep (2021) 17(5):1552–69. doi: 10.1007/s12015-021-10139-5
52. Tolar J, Le Blanc K, Keating A, Blazar BR. Concise review: hitting the right spot with mesenchymal stromal cells. Stem Cells (2010) 28(8):1446–55. doi: 10.1002/stem.459
53. Stappenbeck TS, Miyoshi H. The role of stromal stem cells in tissue regeneration and wound repair. Science (2009) 324(5935):1666–9. doi: 10.1126/science.1172687
54. Rojas M, Xu J, Woods CR, Mora AL, Spears W, ROman J, et al. Bone marrow-derived mesenchymal stem cells in repair of the injured lung. Am J Respir Cell Mol Biol (2005) 33(2):145–52. doi: 10.1165/rcmb.2004-0330OC
55. Ponte AL, Marais E, Gallay N, Langonne A, Delorme B, Herault O, et al. The in vitro migration capacity of human bone marrow mesenchymal stem cells: comparison of chemokine and growth factor chemotactic activities. Stem Cells (2007) 25(7):1737–45. doi: 10.1634/stemcells.2007-0054
56. Lee RH, Seo MJ, Pulin AA, Gregory CA, Ylostalo J, Prockop DJ. The CD34-like protein PODXL and alpha6-integrin (CD49f) identify early progenitor MSCs with increased clonogenicity and migration to infarcted heart in mice. Blood (2009) 113(4):816–26. doi: 10.1182/blood-2007-12-128702
57. Zargar MJ, Kaviani S, Vasei M, Soufi Zomorrod M, Heidari Keshel S, Soleimani M. Therapeutic role of mesenchymal stem cell-derived exosomes in respiratory disease. Stem Cell Res Ther (2022) 13(1):194. doi: 10.1186/s13287-022-02866-4
58. Attia N, Mashal M. Mesenchymal stem cells: the past present and future. Cell biology and translational medicine. Adv Exp Med Biol (2020) 11:107–29. doi: 10.1007/5584_2020_595
59. Phinney DG, Prockop DJ. Concise review: mesenchymal stem/multipotent stromal cells: the state of transdifferentiation and modes of tissue repair–current views. Stem Cells (2007) 25(11):2896–902. doi: 10.1634/stemcells.2007-0637
60. da Silva Meirelles L, Caplan AI, Nardi NB. In search of the in vivo identity of mesenchymal stem cells. Stem Cells (2008) 26(9):2287–99. doi: 10.1634/stemcells.2007-1122
61. Aggarwal S, Pittenger MF. Human mesenchymal stem cells modulate allogeneic immune cell responses. Blood (2005) 105(4):1815–22. doi: 10.1182/blood-2004-04-1559
62. Zhang W, Ge W, Li C, You S, Liao L, Han Q, et al. Effects of mesenchymal stem cells on differentiation, maturation, and function of human monocyte-derived dendritic cells. Stem Cells Dev (2004) 13(3):263–71. doi: 10.1089/154732804323099190
63. Luz-Crawford. P, Kurte. M, Bravo-Alegría. J, Contreras. R, Nova-Lamperti. E, Tejedor. G, et al. Mesenchymal stem cells generate a CD4+CD25+Foxp3+ regulatory T cell population during the differentiation process of Th1 and Th17 cells. Stem Cell Res Ther (2013) 4. doi: 10.1186/scrt216
64. Meisel R, Zibert A, Laryea M, Gobel U, Daubener W, Dilloo D. Human bone marrow stromal cells inhibit allogeneic T-cell responses by indoleamine 2,3-dioxygenase-mediated tryptophan degradation. Blood (2004) 103(12):4619–21. doi: 10.1182/blood-2003-11-3909
65. Sato K, Ozaki K, Oh I, Meguro A, Hatanaka K, Nagai T, et al. Nitric oxide plays a critical role in suppression of T-cell proliferation by mesenchymal stem cells. Blood (2007) 109(1):228–34. doi: 10.1182/blood-2006-02-002246
66. Groh ME, Maitra B, Szekely E, Koc ON. Human mesenchymal stem cells require monocyte-mediated activation to suppress alloreactive T cells. Exp Hematol (2005) 33(8):928–34. doi: 10.1016/j.exphem.2005.05.002
67. Ren G, Su J, Zhang L, Zhao X, Ling W, L’Huillie A, et al. Species variation in the mechanisms of mesenchymal stem cell-mediated immunosuppression. Stem Cells (2009) 27(8):1954–62. doi: 10.1002/stem.118
68. Prockop DJ. Concise review: two negative feedback loops place mesenchymal stem/stromal cells at the center of early regulators of inflammation. Stem Cells (2013) 31(10):2042–6. doi: 10.1002/stem.1400
69. Prockop DJ. Repair of tissues by adult stem/progenitor cells (MSCs): controversies, myths, and changing paradigms. Mol Ther (2009) 17(6):939–46. doi: 10.1038/mt.2009.62
70. Yousefi Dehbidi M, Goodarzi N, Azhdari MH, Doroudian M. Mesenchymal stem cells and their derived exosomes to combat Covid-19. Rev Med Virol (2022) 32(2):e2281. doi: 10.1002/rmv.2281
71. Tang Y, Zhou Y, Li HJ. Advances in mesenchymal stem cell exosomes: a review. Stem Cell Res Ther (2021) 12(1):71. doi: 10.1186/s13287-021-02138-7
72. Thery C, Witwer KW, Aikawa E, Alcaraz MJ, Anderson JD, Andriantsitohaina R, et al. Minimal information for studies of extracellular vesicles 2018 (MISEV2018): a position statement of the International Society for Extracellular Vesicles and update of the MISEV2014 guidelines. J Extracell Vesicles (2018) 7(1):1535750. doi: 10.1080/20013078.2018.1535750
73. Kalluri R, LeBleu VS. The biology, function, and biomedical applications of exosomes. Science (2020) 367(6478). doi: 10.1126/science.aau6977
74. Monsel A, Zhu YG, Gudapati V, Lim H, Lee JW. Mesenchymal stem cell derived secretome and extracellular vesicles for acute lung injury and other inflammatory lung diseases. Expert Opin Biol Ther (2016) 16(7):859–71. doi: 10.1517/14712598.2016.1170804
75. Tomasoni S, Longaretti L, Rota C, Morigi M, Conti S, Gotti E, et al. Transfer of growth factor receptor mRNA via exosomes unravels the regenerative effect of mesenchymal stem cells. Stem Cells Dev (2013) 22(5):772–80. doi: 10.1089/scd.2012.0266
76. Qiao Q, Liu X, Yang T, Cui K, Kong L, Yang C, et al. Nanomedicine for acute respiratory distress syndrome: The latest application, targeting strategy, and rational design. Acta Pharm Sin B (2021) 11(10):3060–91. doi: 10.1016/j.apsb.2021.04.023
77. Pinky, Gupta S, Krishnakumar V, Sharma Y, Dinda AK, Mohanty S. Mesenchymal stem cell derived exosomes: a nano platform for therapeutics and drug delivery in combating COVID-19. Stem Cell Rev Rep (2021) 17(1):33–43. doi: 10.1007/s12015-020-10002-z
78. Yin K, Wang S, Zhao RC. Exosomes from mesenchymal stem/stromal cells: a new therapeutic paradigm. biomark Res (2019) 7:8. doi: 10.1186/s40364-019-0159-x
79. Kim SY, Joglekar MV, Hardikar AA, Phan TH, Khanal D, Tharkar P, et al. Placenta stem/stromal cell-derived extracellular vesicles for potential use in lung repair. Proteomics (2019) 19(17):e1800166. doi: 10.1002/pmic.201800166
80. Chen TS, Lai RC, Lee MM, Choo AB, Lee CN, Lim SK. Mesenchymal stem cell secretes microparticles enriched in pre-microRNAs. Nucleic Acids Res (2010) 38(1):215–24. doi: 10.1093/nar/gkp857
81. Lai RC, Tan SS, Teh BJ, Sze SK, Arslan F, de Kleijn DP, et al. Proteolytic potential of the MSC exosome proteome: implications for an exosome-mediated delivery of therapeutic proteasome. Int J Proteomics (2012) 2012:971907. doi: 10.1155/2012/971907
82. Ranganath SH, Levy O, Inamdar MS, Karp JM. Harnessing the mesenchymal stem cell secretome for the treatment of cardiovascular disease. Cell Stem Cell (2012) 10(3):244–58. doi: 10.1016/j.stem.2012.02.005
83. Riazifar M, Mohammadi MR, Pone EJ, Yeri A, Lasser C, Segaliny AI, et al. Stem cell-derived exosomes as nanotherapeutics for autoimmune and neurodegenerative disorders. ACS Nano (2019) 13(6):6670–88. doi: 10.1021/acsnano.9b01004
84. Burrello J, Monticone S, Gai C, Gomez Y, Kholia S, Camussi G. Stem cell-derived extracellular vesicles and immune-modulation. Front Cell Dev Biol (2016) 4:83. doi: 10.3389/fcell.2016.00083
85. Qian X, An N, Ren Y, Yang C, Zhang X, Li L. Immunosuppressive effects of mesenchymal stem cells-derived exosomes. Stem Cell Rev Rep (2021) 17(2):411–27. doi: 10.1007/s12015-020-10040-7
86. Khare D, Or R, Resnick I, Barkatz C, Almogi-Hazan O, Avni B. Mesenchymal stromal cell-derived exosomes affect mRNA expression and function of B-lymphocytes. Front Immunol (2018) 9:3053. doi: 10.3389/fimmu.2018.03053
87. Chen W, Huang Y, Han J, Yu L, Li Y, Lu Z, et al. Immunomodulatory effects of mesenchymal stromal cells-derived exosome. Immunol Res (2016) 64(4):831–40. doi: 10.1007/s12026-016-8798-6
88. Du YM, Zhuansun YX, Chen R, Lin L, Lin Y, Li JG. Mesenchymal stem cell exosomes promote immunosuppression of regulatory T cells in asthma. Exp Cell Res (2018) 363(1):114–20. doi: 10.1016/j.yexcr.2017.12.021
89. Lai P, Chen X, Guo L, Wang Y, Liu X, Liu Y, et al. A potent immunomodulatory role of exosomes derived from mesenchymal stromal cells in preventing cGVHD. J Hematol Oncol (2018) 11(1):135. doi: 10.1186/s13045-018-0680-7
90. Zhao Y, Song W, Yuan Z, Li M, Wang G, Wang L, et al. Exosome derived from human umbilical cord mesenchymal cell exerts immunomodulatory effects on B cells from SLE patients. J Immunol Res (2023) 2023:3177584. doi: 10.1155/2023/3177584
91. Arabpour M, Saghazadeh A, Rezaei N. Anti-inflammatory and M2 macrophage polarization-promoting effect of mesenchymal stem cell-derived exosomes. Int Immunopharmacol (2021) 97:107823. doi: 10.1016/j.intimp.2021.107823
92. Fan Y, Herr F, Vernochet A, Mennesson B, Oberlin E, Durrbach A. Human fetal liver mesenchymal stem cell-derived exosomes impair natural killer cell function. Stem Cells Dev (2019) 28(1):44–55. doi: 10.1089/scd.2018.0015
93. Alvarez V, Sanchez-Margallo FM, Macias-Garcia B, Gomez-Serrano M, Jorge I, Vazquez J, et al. The immunomodulatory activity of extracellular vesicles derived from endometrial mesenchymal stem cells on CD4+ T cells is partially mediated by TGFbeta. J Tissue Eng Regener Med (2018) 12(10):2088–98. doi: 10.1002/term.2743
94. Liu H, Zhang L, Li M, Zhao F, Lu F, Zhang F, et al. Bone mesenchymal stem cell-derived extracellular vesicles inhibit DAPK1-mediated inflammation by delivering miR-191 to macrophages. Biochem Biophys Res Commun (2022) 598:32–9. doi: 10.1016/j.bbrc.2022.02.009
95. Phinney DG, Di Giuseppe M, Njah J, Sala E, Shiva S, St Croix CM, et al. Mesenchymal stem cells use extracellular vesicles to outsource mitophagy and shuttle microRNAs. Nat Commun (2015) 6:8472. doi: 10.1038/ncomms9472
96. Domenis R, Cifu A, Quaglia S, Pistis C, Moretti M, Vicario A, et al. Pro inflammatory stimuli enhance the immunosuppressive functions of adipose mesenchymal stem cells-derived exosomes. Sci Rep (2018) 8(1):13325. doi: 10.1038/s41598-018-31707-9
97. Song Y, Dou H, Li X, Zhao X, Li Y, Liu D, et al. Exosomal miR-146a contributes to the enhanced therapeutic efficacy of interleukin-1beta-primed mesenchymal stem cells against sepsis. Stem Cells (2017) 35(5):1208–21. doi: 10.1002/stem.2564
98. Mardpour S, Hamidieh AA, Taleahmad S, Sharifzad F, Taghikhani A, Baharvand H. Interaction between mesenchymal stromal cell-derived extracellular vesicles and immune cells by distinct protein content. J Cell Physiol (2019) 234(6):8249–58. doi: 10.1002/jcp.27669
99. Lai CP, Mardini O, Ericsson M, Prabhakar S, Maguire C, Chen JW, et al. Dynamic biodistribution of extracellular vesicles in vivo using a multimodal imaging reporter. ACS Nano (2014) 8(1):483–94. doi: 10.1021/nn404945r
100. Gao J, Dennis JE, Muzic RF, Lundberg M, Caplan AI. The dynamic in vivo distribution of bone marrow-derived mesenchymal stem cells after infusion. Cells Tissues Organs (2001) 169(1):12–20. doi: 10.1159/000047856
101. Haghighitalab A, Dominici M, Matin MM, Shekari F, Ebrahimi Warkiani M, Lim R, et al. Extracellular vesicles and their cells of origin: Open issues in autoimmune diseases. Front Immunol (2023) 14:1090416. doi: 10.3389/fimmu.2023.1090416
102. Choi H, Lee DS. Illuminating the physiology of extracellular vesicles. Stem Cell Res Ther (2016) 7(1):55. doi: 10.1186/s13287-016-0316-1
103. Xu B, Chen SS, Liu MZ, Gan CX, Li JQ, Guo GH. Stem cell derived exosomes-based therapy for acute lung injury and acute respiratory distress syndrome: A novel therapeutic strategy. Life Sci (2020) 254:117766. doi: 10.1016/j.lfs.2020.117766
104. Zhou T, Yuan Z, Weng J, Pei D, Du X, He C, et al. Challenges and advances in clinical applications of mesenchymal stromal cells. J Hematol Oncol (2021) 14(1):24. doi: 10.1186/s13045-021-01037-x
105. Ma X, Liu B, Fan L, Liu Y, Zhao Y, Ren T, et al. Native and engineered exosomes for inflammatory disease. Nano Res (2023) 16(5):6991–7006. doi: 10.1007/s12274-022-5275-5
106. Alcayaga-MIranda F, Cuenca J, Khoury M. Antimicrobial activity of mesenchymal stem cells: current status and new perspectives of antimicrobial peptide-based therapies. Front Immunol (2017) 8:339. doi: 10.3389/fimmu.2017.00339
107. Monsel A, Zhu YG, Gennai S, Hao Q, Hu S, Rouby JJ, et al. Therapeutic effects of human mesenchymal stem cell-derived microvesicles in severe pneumonia in mice. Am J Respir Crit Care Med (2015) 192(3):324–36. doi: 10.1164/rccm.201410-1765OC
108. Bailey CC, Zhong G, Huang IC, Farzan M. IFITM-family proteins: the cell’s first line of antiviral defense. Annu Rev Virol (2014) 1:261–83. doi: 10.1146/annurev-virology-031413-085537
109. Alcayaga-MIranda F, Cuenca J, Martin A, Contreras L, Figueroa FE, Khoury M. Combination therapy of menstrual derived mesenchymal stem cells and antibiotics ameliorates survival in sepsis. Stem Cell Res Ther (2015) 6:199. doi: 10.1186/s13287-015-0192-0
110. Meisel R, Brockers S, Heseler K, Degistirici O, Bulle H, Woite C, et al. Human but not murine multipotent mesenchymal stromal cells exhibit broad-spectrum antimicrobial effector function mediated by indoleamine 2,3-dioxygenase. Leukemia (2011) 25(4):648–54. doi: 10.1038/leu.2010.310
111. Lee JW, Krasnodembskaya A, McKenna DH, Song Y, Abbott J, Matthay MA. Therapeutic effects of human mesenchymal stem cells in ex vivo human lungs injured with live bacteria. Am J Respir Crit Care Med (2013) 187(7):751–60. doi: 10.1164/rccm.201206-0990OC
112. Yang R, Liu Y, Kelk P, Qu C, Akiyama K, Chen C, et al. A subset of IL-17(+) mesenchymal stem cells possesses anti-Candida albicans effect. Cell Res (2013) 23(1):107–21. doi: 10.1038/cr.2012.179
113. Sung DK, Chang YS, Sung SI, Yoo HS, Ahn SY, Park WS. Antibacterial effect of mesenchymal stem cells against Escherichia coli is mediated by secretion of beta- defensin- 2 via toll- like receptor 4 signalling. Cell Microbiol (2016) 18(3):424–36. doi: 10.1111/cmi.12522
114. Gupta N, Krasnodembskaya A, Kapetanaki M, Mouded M, Tan X, Serikov V, et al. Mesenchymal stem cells enhance survival and bacterial clearance in murine Escherichia coli pneumonia. Thorax (2012) 67(6):533–9. doi: 10.1136/thoraxjnl-2011-201176
115. Jackson MV, Morrison TJ, Doherty DF, McAuley DF, Matthay MA, Kissenpfennig A, et al. Mitochondrial transfer via tunneling nanotubes is an important mechanism by which mesenchymal stem cells enhance macrophage phagocytosis in the in vitro and in vivo models of ARDS. Stem Cells (2016) 34(8):2210–23. doi: 10.1002/stem.2372
116. Rabani R, Volchuk A, Jerkic M, Ormesher L, Garces-Ramirez L, Canton J, et al. Mesenchymal stem cells enhance NOX2-dependent reactive oxygen species production and bacterial killing in macrophages during sepsis. Eur Respir J (2018) 51(4). doi: 10.1183/13993003.02021-2017
117. Park J, Kim S, Lim H, Liu A, Hu S, Lee J, et al. Therapeutic effects of human mesenchymal stem cell microvesicles in an ex vivo perfused human lung injured with severe E. Coli Pneumonia Thorax (2019) 74(1):43–50. doi: 10.1136/thoraxjnl-2018-211576
118. Hao Q, Gudapati V, Monsel A, Park JH, Hu S, Kato H, et al. Mesenchymal stem cell-derived extracellular vesicles decrease lung injury in mice. J Immunol (2019) 203(7):1961–72. doi: 10.4049/jimmunol.1801534
119. Ware. LB, Matthay. MA. Alveolar fluid clearance is impaired in the majority of patients with acute lung injury and the acute respiratory distress syndrome. Am J Respir Crit Care Med (2001) 163:1376–83. doi: 10.1164/ajrccm.163.6.2004035
120. Lee JW, Fang X, Gupta N, Serikov V, Matthay MA. Allogeneic human mesenchymal stem cells for treatment of E. coli endotoxin-induced acute lung injury in the ex vivo perfused human lung. Proc Natl Acad Sci U S A (2009) 106(38):16357–62. doi: 10.1073/pnas.0907996106
121. Zhu YG, Feng XM, Abbott J, Fang XH, Hao Q, Monsel A, et al. Human mesenchymal stem cell microvesicles for treatment of Escherichia coli endotoxin-induced acute lung injury in mice. Stem Cells (2014) 32(1):116–25. doi: 10.1002/stem.1504
122. Wang H, Zheng R, Chen Q, Shao J, Yu J, Hu S. Mesenchymal stem cells microvesicles stabilize endothelial barrier function partly mediated by hepatocyte growth factor (HGF). Stem Cell Res Ther (2017) 8(1):211. doi: 10.1186/s13287-016-0450-9
123. Fang X, Neyrinck AP, Matthay MA, Lee JW. Allogeneic human mesenchymal stem cells restore epithelial protein permeability in cultured human alveolar type II cells by secretion of angiopoietin-1. J Biol Chem (2010) 285(34):26211–22. doi: 10.1074/jbc.M110.119917
124. Dutra Silva J, Su Y, Calfee CS, Delucchi KL, Weiss D, McAuley DF, et al. Mesenchymal stromal cell extracellular vesicles rescue mitochondrial dysfunction and improve barrier integrity in clinically relevant models of ARDS. Eur Respir J (2021) 58(1). doi: 10.1183/13993003.02978-2020
125. Buzas EI. The roles of extracellular vesicles in the immune system. Nat Rev Immunol (2023) 23(4):236–50. doi: 10.1038/s41577-022-00763-8
126. Li J, Li D, Liu X, Tang S, Wei F. Human umbilical cord mesenchymal stem cells reduce systemic inflammation and attenuate LPS-induced acute lung injury in rats. J Inflammation (Lond) (2012) 9(1):33. doi: 10.1186/1476-9255-9-33
127. Xu J, Woods CR, Mora AL, Joodi R, Brigham KL, Iyer S, et al. Prevention of endotoxin-induced systemic response by bone marrow-derived mesenchymal stem cells in mice. Am J Physiol Lung Cell Mol Physiol (2007) 293(1):L131–41. doi: 10.1152/ajplung.00431.2006
128. Li QC, Liang Y, Su ZB. Prophylactic treatment with MSC-derived exosomes attenuates traumatic acute lung injury in rats. Am J Physiol Lung Cell Mol Physiol (2019) 316(6):L1107–L17. doi: 10.1152/ajplung.00391.2018
129. Zhao R, Wang L, Wang T, Xian P, Wang H, Long Q. Inhalation of MSC-EVs is a noninvasive strategy for ameliorating acute lung injury. J Control Release (2022) 345:214–30. doi: 10.1016/j.jconrel.2022.03.025
130. Gupta N, Su X, Popov B, Lee JW, Serikov V, Matthay MA. Intrapulmonary delivery of bone marrow-derived mesenchymal stem cells improves survival and attenuates endotoxin-induced acute lung injury in mice. J Immunol (2007) 179(3):1855–63. doi: 10.4049/jimmunol.179.3.1855
131. Su VY, Lin CS, Hung SC, Yang KY. Mesenchymal stem cell-conditioned medium induces neutrophil apoptosis associated with inhibition of the NF-kappaB pathway in endotoxin-induced acute lung injury. Int J Mol Sci (2019) 20(9). doi: 10.3390/ijms20092208
132. Liu S, Su X, Pan P, Zhang L, Hu Y, Tan H, et al. Neutrophil extracellular traps are indirectly triggered by lipopolysaccharide and contribute to acute lung injury. Sci Rep (2016) 6:37252. doi: 10.1038/srep37252
133. Pedrazza L, Cunha AA, Luft C, Nunes NK, Schimitz F, Gassen RB, et al. Mesenchymal stem cells improves survival in LPS-induced acute lung injury acting through inhibition of NETs formation. J Cell Physiol (2017) 232(12):3552–64. doi: 10.1002/jcp.25816
134. Zhang X, Wei X, Deng Y, Yuan X, Shi J, Huang W, et al. Mesenchymal stromal cells alleviate acute respiratory distress syndrome through the cholinergic anti-inflammatory pathway. Signal Transduct Target Ther (2022) 7(1):307. doi: 10.1038/s41392-022-01124-6
135. Su Y, Silva JD, Doherty D, Simpson DA, Weiss DJ, Rolandsson-Enes S, et al. Mesenchymal stromal cells-derived extracellular vesicles reprogramme macrophages in ARDS models through the miR-181a-5p-PTEN-pSTAT5-SOCS1 axis. Thorax (2022) 1–14. doi: 10.1136/thoraxjnl-2021-218194
136. Wang J, Huang R, Xu Q, Zheng G, Qiu G, Ge M, et al. Mesenchymal stem cell-derived extracellular vesicles alleviate acute lung injury via transfer of miR-27a-3p. Crit Care Med (2020) 48(7):e599–610. doi: 10.1097/CCM.0000000000004315
137. Morrison TJ, Jackson MV, Cunningham EK, Kissenpfennig A, McAuley DF, O’Kane CM, et al. Mesenchymal stromal cells modulate macrophages in clinically relevant lung injury models by extracellular vesicle mitochondrial transfer. Am J Respir Crit Care Med (2017) 196(10):1275–86. doi: 10.1164/rccm.201701-0170OC
138. Xia L, Zhang C, Lv N, Liang Z, Ma T, Cheng H, et al. AdMSC-derived exosomes alleviate acute lung injury via transferring mitochondrial component to improve homeostasis of alveolar macrophages. Theranostics (2022) 12(6):2928–47. doi: 10.7150/thno.69533
139. Fan J, Zhang YC, Zheng DF, Zhang M, Liu H, He M, et al. IL-27 is elevated in sepsis with acute hepatic injury and promotes hepatic damage and inflammation in the CLP model. Cytokine (2020) 127:154936. doi: 10.1016/j.cyto.2019.154936
140. Xu F, Liu Q, Lin S, Shen N, Yin Y, Cao J. IL-27 is elevated in acute lung injury and mediates inflammation. J Clin Immunol (2013) 33(7):1257–68. doi: 10.1007/s10875-013-9923-0
141. Wang X, Liu D, Zhang X, Yang L, Xia Z, Zhang Q. Exosomes from adipose-derived mesenchymal stem cells alleviate sepsis-induced lung injury in mice by inhibiting the secretion of IL-27 in macrophages. Cell Death Discovery (2022) 8(1):18. doi: 10.1038/s41420-021-00785-6
142. Dong T, Chen X, Xu H, Song Y, Wang H, Gao Y, et al. Mitochondrial metabolism mediated macrophage polarization in chronic lung diseases. Pharmacol Ther (2022) 239:108208. doi: 10.1016/j.pharmthera.2022.108208
143. Deng H, Wu L, Liu M, Zhu L, Chen Y, Zhou H, et al. Bone marrow mesenchymal stem cell-derived exosomes attenuate LPS-induced ARDS by modulating macrophage polarization through inhibiting glycolysis in macrophages. Shock (2020) 54(6):828–43. doi: 10.1097/SHK.0000000000001549
144. Yi X, Wei X, Lv H, An Y, Li L, Lu P, et al. Exosomes derived from microRNA-30b-3p-overexpressing mesenchymal stem cells protect against lipopolysaccharide-induced acute lung injury by inhibiting SAA3. Exp Cell Res (2019) 383(2):111454. doi: 10.1016/j.yexcr.2019.05.035
145. Fujita. M, Kuwano. K, Kunitake. R, Hagimoto. N, Miyazaki. H, Kaneko. Y, et al. Endothelial cell apoptosis in lipopolysaccharide-lnduced lung injury in mice. Int Arch Allergy Immunol (1998) 117:202–8. doi: 10.1159/000024011
146. Abadie Y, Bregeon F, Papazian L, Lange F, Chailley-Heu B, Thomas P, et al. Decreased VEGF concentration in lung tissue and vascular injury during ARDS. Eur Respir J (2005) 25(1):139–46. doi: 10.1183/09031936.04.00065504
147. Gill SE, Rohan M, Mehta S. Role of pulmonary microvascular endothelial cell apoptosis in murine sepsis-induced lung injury in vivo. Respir Res (2015) 16(1):109. doi: 10.1186/s12931-015-0266-7
148. Block GJ, Ohkouchi S, Fung F, Frenkel J, Gregory C, Pochampally R, et al. Multipotent stromal cells are activated to reduce apoptosis in part by upregulation and secretion of stanniocalcin-1. Stem Cells (2009) 27(3):670–81. doi: 10.1002/stem.20080742
149. Zheng Y, Liu J, Chen P, Lin L, Luo Y, Ma X, et al. Exosomal miR-22-3p from human umbilical cord blood-derived mesenchymal stem cells protects against lipopolysaccharid-induced acute lung injury. Life Sci (2021) 269:119004. doi: 10.1016/j.lfs.2020.119004
150. Jiang X, Stockwell BR, Conrad M. Ferroptosis: mechanisms, biology and role in disease. Nat Rev Mol Cell Biol (2021) 22(4):266–82. doi: 10.1038/s41580-020-00324-8
151. Li Y, Cao Y, Xiao J, Shang J, Tan Q, Ping F, et al. Inhibitor of apoptosis-stimulating protein of p53 inhibits ferroptosis and alleviates intestinal ischemia/reperfusion-induced acute lung injury. Cell Death Differ (2020) 27(9):2635–50. doi: 10.1038/s41418-020-0528-x
152. Shen K, Wang X, Wang Y, Jia Y, Zhang Y, Wang K, et al. miR-125b-5p in adipose derived stem cells exosome alleviates pulmonary microvascular endothelial cells ferroptosis via Keap1/Nrf2/GPX4 in sepsis lung injury. Redox Biol (2023) 62:102655. doi: 10.1016/j.redox.2023.102655
153. Levine B, Kroemer G. Autophagy in the pathogenesis of disease. Cell (2008) 132(1):27–42. doi: 10.1016/j.cell.2007.12.018
154. Ornatowski W, Lu Q, Yegambaram M, Garcia AE, Zemskov EA, Maltepe E, et al. Complex interplay between autophagy and oxidative stress in the development of pulmonary disease. Redox Biol (2020) 36:101679. doi: 10.1016/j.redox.2020.101679
155. Liu Q, Wu J, Zhang X, Li X, Wu X, Zhao Y, et al. Circulating mitochondrial DNA-triggered autophagy dysfunction via STING underlies sepsis-related acute lung injury. Cell Death Dis (2021) 12(7):673. doi: 10.1038/s41419-021-03961-9
156. Liu X, Gao C, Wang Y, Niu L, Jiang S, Pan S. BMSC-derived exosomes ameliorate LPS-induced acute lung injury by miR-384-5p-controlled alveolar macrophage autophagy. Oxid Med Cell Longev (2021) 2021:9973457. doi: 10.1155/2021/9973457
157. Gorman E, Millar J, McAuley D, O’Kane C. Mesenchymal stromal cells for acute respiratory distress syndrome (ARDS), sepsis, and COVID-19 infection: optimizing the therapeutic potential. Expert Rev Respir Med (2021) 15(3):301–24. doi: 10.1080/17476348.2021.1848555
158. Li LX, Jin S, Zhang YM. Ischemic preconditioning potentiates the protective effect of mesenchymal stem cells on endotoxin-induced acute lung injury in mice through secretion of exosome. Int J Clin Exp Med (2015) 8(3):3825–32.
159. Meng SS, Xu XP, Chang W, Lu ZH, Huang LL, Xu JY, et al. LincRNA-p21 promotes mesenchymal stem cell migration capacity and survival through hypoxic preconditioning. Stem Cell Res Ther (2018) 9(1):280. doi: 10.1186/s13287-018-1031-x
160. Tsoyi K, Hall SR, Dalli J, Colas RA, Ghanta S, Ith B, et al. Carbon monoxide improves efficacy of mesenchymal stromal cells during sepsis by production of specialized proresolving lipid mediators. Crit Care Med (2016) 44(12):e1236–e45. doi: 10.1097/CCM.0000000000001999
161. Saeedi P, Halabian R, Fooladi AAI. Mesenchymal stem cells preconditioned by staphylococcal enterotoxin B enhance survival and bacterial clearance in murine sepsis model. Cytotherapy (2019) 21(1):41–53. doi: 10.1016/j.jcyt.2018.11.002
162. Saeedi P, Halabian R, Fooladi AAI. Antimicrobial effects of mesenchymal stem cells primed by modified LPS on bacterial clearance in sepsis. J Cell Physiol (2019) 234(4):4970–86. doi: 10.1002/jcp.27298
163. Horie S, Gaynard S, Murphy M, Barry F, Scully M, O’Toole D, et al. Cytokine pre-activation of cryopreserved xenogeneic-free human mesenchymal stromal cells enhances resolution and repair following ventilator-induced lung injury potentially via a KGF-dependent mechanism. Intensive Care Med Exp (2020) 8(1):8. doi: 10.1186/s40635-020-0295-5
164. Chen J, Zhang X, Xie J, Xue M, Liu L, Yang Y, et al. Overexpression of TGFbeta1 in murine mesenchymal stem cells improves lung inflammation by impacting the Th17/Treg balance in LPS-induced ARDS mice. Stem Cell Res Ther (2020) 11(1):311. doi: 10.1186/s13287-020-1556-7
165. He H, Liu L, Chen Q, Liu A, Cai S, Yang Y, et al. Mesenchymal stem cells overexpressing angiotensin-converting enzyme 2 rescue lipopolysaccharide-induced lung injury. Cell Transplant (2015) 24(9):1699–715. doi: 10.3727/096368914X685087
166. Xu J, Qu J, Cao L, Sai Y, Chen C, He L, et al. Mesenchymal stem cell-based angiopoietin-1 gene therapy for acute lung injury induced by lipopolysaccharide in mice. J Pathol (2008) 214(4):472–81. doi: 10.1002/path.2302
167. Wang H, Alarcon CN, Liu B, Watson F, Searles S, Lee CK, et al. Genetically engineered and enucleated human mesenchymal stromal cells for the targeted delivery of therapeutics to diseased tissue. Nat BioMed Eng (2022) 6(7):882–97. doi: 10.1038/s41551-021-00815-9
168. Salunkhe S, Dheeraj, Basak M, Chitkara D, Mittal A. Surface functionalization of exosomes for target-specific delivery and in vivo imaging & tracking: Strategies and significance. J Control Release (2020) 326:599–614. doi: 10.1016/j.jconrel.2020.07.042
169. Gupta D, Wiklander OPB, Gorgens A, Conceicao M, Corso G, Liang X, et al. Amelioration of systemic inflammation via the display of two different decoy protein receptors on extracellular vesicles. Nat BioMed Eng (2021) 5(9):1084–98. doi: 10.1038/s41551-021-00792-z
170. Rezakhani L, Kelishadrokhi AF, Soleimanizadeh A, Rahmati S. Mesenchymal stem cell (MSC)-derived exosomes as a cell-free therapy for patients Infected with COVID-19: Real opportunities and range of promises. Chem Phys Lipids (2021) 234:105009. doi: 10.1016/j.chemphyslip.2020.105009
171. Zheng G, Huang L, Tong H, Shu Q, Hu Y, Ge M, et al. Treatment of acute respiratory distress syndrome with allogeneic adipose-derived mesenchymal stem cells: a randomized, placebo-controlled pilot study. Respir Res (2014) 15(1):39. doi: 10.1186/1465-9921-15-39
172. Wilson JG, Liu KD, Zhuo H, Caballero L, McMillan M, Fang X, et al. Mesenchymal stem (stromal) cells for treatment of ARDS: a phase 1 clinical trial. Lancet Respir Med (2015) 3(1):24–32. doi: 10.1016/S2213-2600(14)70291-7
173. Matthay MA, Calfee CS, Zhuo H, Thompson BT, Wilson JG, Levitt JE, et al. Treatment with allogeneic mesenchymal stromal cells for moderate to severe acute respiratory distress syndrome (START study): a randomised phase 2a safety trial. Lancet Respir Med (2019) 7(2):154–62. doi: 10.1016/S2213-2600(18)30418-1
174. Sengupta V, Sengupta S, Lazo A, Woods P, Nolan A, Bremer N. Exosomes derived from bone marrow mesenchymal stem cells as treatment for severe COVID-19. Stem Cells Dev (2020) 29(12):747–54. doi: 10.1089/scd.2020.0080
175. Leng Z, Zhu R, Hou W, Feng Y, Yang Y, Han Q, et al. Transplantation of ACE2(-) mesenchymal stem cells improves the outcome of patients with COVID-19 pneumonia. Aging Dis (2020) 11(2):216–28. doi: 10.14336/AD.2020.0228
176. Lanzoni G, Linetsky E, Correa D, Messinger Cayetano S, Alvarez RA, Kouroupis D, et al. Umbilical cord mesenchymal stem cells for COVID-19 acute respiratory distress syndrome: A double-blind, phase 1/2a, randomized controlled trial. Stem Cells Transl Med (2021) 10(5):660–73. doi: 10.1002/sctm.20-0472
177. Zarrabi M, Shahrbaf MA, Nouri M, Shekari F, Hosseini SE, Hashemian SR, et al. Allogenic mesenchymal stromal cells and their extracellular vesicles in COVID-19 induced ARDS: a randomized controlled trial. Stem Cell Res Ther (2023) 14(1):169. doi: 10.1186/s13287-023-03402-8
178. Bowdish ME, Barkauskas CE, Overbey JR, Gottlieb RL, Osman K, Duggal A, et al. A randomized trial of mesenchymal stromal cells for moderate to severe acute respiratory distress syndrome from COVID-19. Am J Respir Crit Care Med (2023) 207(3):261–70. doi: 10.1164/rccm.202201-0157OC
179. Lightner AL, Sengupta V, Qian S, Ransom JT, Suzuki S, Park DJ, et al. Bone marrow mesenchymal stem cell derived extracellular vesicle infusion for the treatment of respiratory failure from COVID-19: A randomized placebo controlled dosing clinical trial. Chest (2023). doi: 10.1016/j.chest.2023.06.024
180. Sinha P, Delucchi KL, McAuley DF, O’Kane CM, Matthay MA, Calfee CS. Development and validation of parsimonious algorithms to classify acute respiratory distress syndrome phenotypes: a secondary analysis of randomised controlled trials. Lancet Respir Med (2020) 8(3):247–57. doi: 10.1016/S2213-2600(19)30369-8
181. Sinha P, Calfee CS, Cherian S, Brealey D, Cutler S, King C, et al. Prevalence of phenotypes of acute respiratory distress syndrome in critically ill patients with COVID-19: a prospective observational study. Lancet Respir Med (2020) 8(12):1209–18. doi: 10.1016/S2213-2600(20)30366-0
182. Matthay MA, McAuley DF, Ware LB. Clinical trials in acute respiratory distress syndrome: challenges and opportunities. Lancet Respir Med (2017) 5(6):524–34. doi: 10.1016/S2213-2600(17)30188-1
183. Rydyznski Moderbacher C, Ramirez SI, Dan JM, Grifoni A, Hastie KM, Weiskopf D, et al. Antigen-specific adaptive immunity to SARS-CoV-2 in acute COVID-19 and associations with age and disease severity. Cell (2020) 183(4):996–1012 e19. doi: 10.1016/j.cell.2020.09.038
184. Abdal Dayem A, Lee SB, Kim K, Lim KM, Jeon TI, Seok J, et al. Production of mesenchymal stem cells through stem cell reprogramming. Int J Mol Sci (2019) 20(8). doi: 10.3390/ijms20081922
185. Moghadasi S, Elveny M, Rahman HS, Suksatan W, Jalil AT, Abdelbasset WK, et al. A paradigm shift in cell-free approach: the emerging role of MSCs-derived exosomes in regenerative medicine. J Transl Med (2021) 19(1):302. doi: 10.1186/s12967-021-02980-6
186. Gardiner C, Di Vizio D, Sahoo S, Thery C, Witwer KW, Wauben M, et al. Techniques used for the isolation and characterization of extracellular vesicles: results of a worldwide survey. J Extracell Vesicles (2016) 5:32945. doi: 10.3402/jev.v5.32945
187. Colao IL, Corteling R, Bracewell D, Wall I. Manufacturing exosomes: A promising therapeutic platform. Trends Mol Med (2018) 24(3):242–56. doi: 10.1016/j.molmed.2018.01.006
188. Tauro BJ, Greening DW, Mathias RA, Ji H, Mathivanan S, Scott AM, et al. Comparison of ultracentrifugation, density gradient separation, and immunoaffinity capture methods for isolating human colon cancer cell line LIM1863-derived exosomes. Methods (2012) 56(2):293–304. doi: 10.1016/j.ymeth.2012.01.002
189. Beitler JR, Thompson BT, Baron RM, Bastarache JA, Denlinger LC, Esserman L, et al. Advancing precision medicine for acute respiratory distress syndrome. Lancet Respir Med (2022) 10(1):107–20. doi: 10.1016/S2213-2600(21)00157-0
Keywords: ARDS, ALI, MSCs, extracellular vesicles, cell-based therapy
Citation: Zhuang X, Jiang Y, Yang X, Fu L, Luo L, Dong Z, Zhao J and Hei F (2023) Advances of mesenchymal stem cells and their derived extracellular vesicles as a promising therapy for acute respiratory distress syndrome: from bench to clinic. Front. Immunol. 14:1244930. doi: 10.3389/fimmu.2023.1244930
Received: 26 June 2023; Accepted: 14 August 2023;
Published: 29 August 2023.
Edited by:
Faezeh Shekari, Royan Institute for Stem Cell Biology and Technology (RI-SCBT), IranReviewed by:
Xiaoxiao Pang, Stomatological Hospital of Chongqing Medical University, ChinaGustavo Jesus Vazquez-Zapien, Escuela Medico Militar, Mexico
Copyright © 2023 Zhuang, Jiang, Yang, Fu, Luo, Dong, Zhao and Hei. This is an open-access article distributed under the terms of the Creative Commons Attribution License (CC BY). The use, distribution or reproduction in other forums is permitted, provided the original author(s) and the copyright owner(s) are credited and that the original publication in this journal is cited, in accordance with accepted academic practice. No use, distribution or reproduction is permitted which does not comply with these terms.
*Correspondence: Feilong Hei, aGVpZmVpbG9uZ0AxMjYuY29t