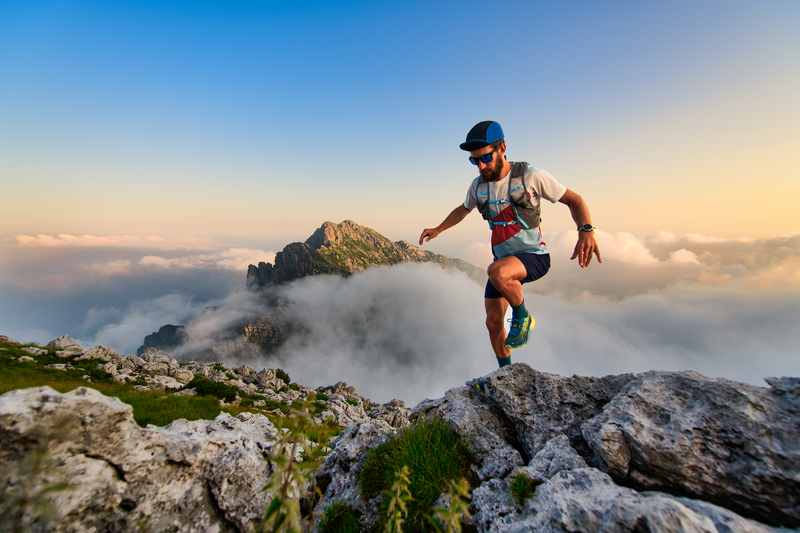
94% of researchers rate our articles as excellent or good
Learn more about the work of our research integrity team to safeguard the quality of each article we publish.
Find out more
ORIGINAL RESEARCH article
Front. Immunol. , 17 August 2023
Sec. Cancer Immunity and Immunotherapy
Volume 14 - 2023 | https://doi.org/10.3389/fimmu.2023.1240678
This article is part of the Research Topic Immunotherapeutic Strategies to Target Cancer Stem Cells: State of the Art in Basic Research to Clinical Application View all 5 articles
Background: Therapeutic cancer vaccination against mutant calreticulin (CALR) in patients with CALR-mutant (CALRmut) myeloproliferative neoplasms (MPN) induces strong T-cell responses against mutant CALR yet fails to demonstrate clinical activity. Infiltration of tumor specific T cells into the tumor microenvironment is needed to attain a clinical response to therapeutic cancer vaccination.
Aim: Determine if CALRmut specific T cells isolated from vaccinated patients enrich in the bone marrow upon completion of vaccination and explore possible explanations for the lack of enrichment.
Methods: CALRmut specific T cells from four of ten vaccinated patients were expanded, enriched, and analyzed by T-cell receptor sequencing (TCRSeq). The TCRs identified were used as fingerprints of CALRmut specific T cells. Bone marrow aspirations from the four patients were acquired at baseline and at the end of trial. T cells were enriched from the bone marrow aspirations and analyzed by TCRSeq to identify the presence and fraction of CALRmut specific T cells at the two different time points. In silico calculations were performed to calculate the ratio between transformed cells and effector cells in patients with CALRmut MPN.
Results: The fraction of CALRmut specific T cells in the bone marrow did not increase upon completion of the vaccination trial. In general, the T cell repertoire in the bone marrow remains relatively constant through the vaccination trial. The enriched and expanded CALRmut specific T cells recognize peripheral blood autologous CALRmut cells. In silico analyses demonstrate a high imbalance in the fraction of CALRmut cells and CALRmut specific effector T-cells in peripheral blood.
Conclusion: CALRmut specific T cells do not enrich in the bone marrow after therapeutic cancer peptide vaccination against mutant CALR. The specific T cells recognize autologous peripheral blood derived CALRmut cells. In silico analyses demonstrate a high imbalance between the number of transformed cells and CALRmut specific effector T-cells in the periphery. We suggest that the high burden of transformed cells in the periphery compared to the number of effector cells could impact the ability of specific T cells to enrich in the bone marrow.
The chronic myeloproliferative neoplasms (MPN) are neoplastic diseases of the hematopoietic stem cells in the bone marrow (1). The three types of MPN are essential thrombocythemia (ET), polycythemia vera and primary myelofibrosis (PMF). The mutational landscape in MPN is highly heterogenous as approximately 55% of patients harbor the Janus kinase 2 (JAK2)V617F mutation (2), and 20% of patients a mutation in exon 9 of the calreticulin (CALR) gene (3, 4). Even though more than 50 types of CALR mutations have been identified, they all generate a 36 amino acid sequence in the C-terminus of the CALR protein that is shared between all patients (5). We have demonstrated that the mutant C-terminus of mutant CALR is a cancer neo-antigen as the mutations are immunogenic (6), and both CD4+ and CD8+ T cells specific for peptides derived from the mutant CALR C-terminus recognize and kill autologous CALRmut cells (7, 8). Given the high immunogenicity of the mutations, and the identification of CALRmut specific T-cell memory responses in healthy donors (9), which suggest a defect in the tumor immune surveillance in CALRmut patients (10), we conducted a phase I clinical vaccination trial against mutant CALR (NCT 03566446) (11). In this trial, 10 patients with CALRmut MPN received 15 vaccines over the course of one year. The vaccines consisted of a peptide (CALRLong36) derived from the mutant CALR C-terminus with montanide as an adjuvant. The vaccines were safe and tolerable and induced T-cell responses to the vaccination epitope. Curiously, none of the patients displayed neither a hematological nor a molecular response to the vaccines (11). The failure of the vaccines may be attributed to several mechanisms that were recently reviewed (12). Yet, the lack of response could be ascribed to failure of CALRmut specific T cells to enrich in the bone marrow. In the present study we used T-cell receptor sequencing (TCRSeq) to investigate the homing of CALRmut specific T cells to the bone marrow in four vaccinated patients and show that the specific T cells do not home to the bone marrow. CALRmut specific T cells recognize autologous ex vivo isolated peripheral blood (PB) CALRmut monocytes which made us hypothesize that the tumor burden in PB outnumbers the specific T cells. This hypothesis was confirmed by in silico analyses as we showed a high imbalance between the number of transformed cells in CALRmut MPN compared to the amount of effector immune cells in patients, thus demonstrating that the burden of transformed cells in MPN is an important obstacle to overcome for cancer immune therapy to have effect in patients with MPN.
Four patients (three patients with ET and one patient with post-ET myelofibrosis) that completed our CALRmut vaccination trial and displayed an immune response to the CALRLong36 epitope were chosen as donors for generation of specific T-cell cultures. Cultures were generated as previously described by stimulation with the CALRLong36 peptide (13). Specific T cells were enriched by overnight incubation after restimulation with CALRLong36 peptide and labelled with fluorescence labelled antibodies after 18 hours of stimulation. Specific T cells were sorted using a fluorescence activated cell sorting (FACS) Melody cell sorter (BD Biosciences, San José, CA, USA) with the sorting gate set on live, CD3+, CD4+ or CD8+, CD137+/CD107a+ double positive events (Supplementary Material 1, top). Enrichment purity was assessed, and pellets of specific cells were kept at -80 °C. Sorting of bone marrow derived T cells was performed on cryopreserved bone marrow derived mononuclear cells. T cells were enriched as live CD3+ positive events (Supplementary Material 2, bottom). Enrichment purity was assessed after FACS, and enriched T cells were pelleted and cryopreserved as described above. A visual abstract of the methods described above is provided in Figure 1. A list of fluorescence labelled antibodies is provided in Supplementary Material 2.
Figure 1 Visual abstract of the methods employed for the T cell receptor sequencing in this study. Four patients with a strong vaccine induced immune response to the CALRLong36 epitope were chosen as donors for expansion of CALRmut specific T cells. Firstly, patient peripheral blood mononuclear cells were stimulated in vitro with CALRLong36 peptide. Next CALRLong36 specific T cells were enriched using magnetically activated cells sorting of cytokine secreting cells, and the enriched cells were expanded using our rapid expansion protocol. Expanded cells were then restimulated with CALRLong36 peptide and enriched using fluorescense activated cells sorting (FACS) by gating on CD137+/CD107a+ double positive cells. Enriched cells were subjected to T-cell receptor sequencing (TCRSeq) which allowed us to identify sequence of the TCR in CALRmut specific T cells (Top, left to right). Bone marrow aspirations from the four patients were isolated at trial baseline and at end of trial. Bone marrow derived T cells from the aspirations were enriched using FACS by gating on live CD3+ events. The enriched cells were analyzed by TCRSeq and through our analyses of the TCR of CALRmut specific T cells above we ascertained the presence and fraction of CALRmut specific T cells (red TCR) in the bone marrow at baseline and at the end of the trial (Middle and below, left to right). Created with BioRender.com.
Autologous peripheral blood lymphocytes (PBL) and monocytes were enriched using a BD FACS Melody live cell sorter with monocytes sorted as live CD14+ events, and PBL sorted as live CD14- events (Supplementary Material 3). The sorted cells were setup in an IFN-γ Enzyme Linked ImmunoSPOT (ELISPOT) assay which was coated, developed and analyzed as previously reported (13). In short, CALRmut specific T cells were aliquoted in 105 aliquots in triplicates. T cells were stimulated with either CALRLong36 peptide (positive control), 104 autologous monocytes, 104 autologous PBL, or left unstimulated (negative control). To assess the cytokine activity from monocytes and PBL, 104 autologous monocytes and 104 autologous PBL were plated alone in triplicate wells serving as an additional negative control. Normalized counts were calculated by subtracting the spot count in the wells with unstimulated T cells and the wells with monocytes and PBL respectively from the spot count in wells with monocyte stimulated T cells and PBL stimulated T cells respectively.
Patient peripheral blood mononuclear cells (PBMC) were thawed and treated with Fc-block (Human TruStain FcX receptor blocking solution, Biolegend, San Diego, CA, USA) and stained with fluorescent antibodies (Supplementary Material 2). Cells were acquired on a Novocyte Quanteon Flow cytometer (Agilent, Santa Clara, CA, USA) for the assessment of HLA-I expression, and a FACS Canto II flow cytometer (BD Biosciences, San José, CA, USA) for assessment of HLA-II, both with appropriate compensation controls. Mean fluorescence intensity (MFI) for HLA-I and HLA-II was calculated by subtracting the MFI of the isotype control sample from the HLA-I/II stained sample.
Performed as previously described (14, 15).
Total RNA purification was performed on cell pellets of CALRLong36-specific T cells or bone marrow derived T cells using the RNEasy Plus Mini Kit (Qiagen, Germantown, MD, USA) according to the manufacturer’s supplied protocol. RNA concentration was measured on a NanoDrop2000 Spectrophotometer (Thermo Scientific, Waltham, MA, USA). T cell receptor sequencing was performed using the SMARTer Human TCR a/b Profiling kit (Takara 635016, San Jose, CA, USA) according to the manufacturer’s protocols. The TCR libraries were sequenced on an Illumina NovaSeq 6000 platform (Illumina, San Diego, CA, USA) using read lengths of 150 bp read 1, 8 bp i7 index, 150 bp read 2, 8 bp i5 index and 20% PhiX. TCR reads were analyzed using the Cogent NGS Immune Profiler Software v1.0 which enable data preprocessing, UMI based analysis, clonotype calling and statistical analysis. Output from the Cogent Profiler was further processed in R using the software package Immunarch (16). Clonotypes with less than two reads were excluded from further analysis. To filter out redundant TCR clonotypes identified in the specific T-cell cultures, clonotypes with a fraction< 1% were excluded from the analysis as they were deemed redundant non-specific T cells in the culture. Assessment of the number of clonotypes detected was performed using the repExplore-function and tracking of clonotypes using the trackClonotypes-function.
We based our calculations on the estimates reported by Sender and Milo (17) on total numbers and turnover of cells in the human organism. The total tumor burden can be computed from the number of neutrophils (N) plus monocytes (M) multiplied by the variant allele frequency (VAF) of mutant CALR multiplied by a factor of 2 since the mutation is heterozygous: 2·(N+M)·VAF.
The number of CALRmut specific T cells is a fraction (b) of the total T cell pool (T), CALRmut specific T cells=b·T. The number of kills per CALR mut specific T cell needed to eradicate the tumor load is then:
Each of these kills take a defined period of time, τ. The total time needed for the CALRmut specific T cells to eradicate the tumor load is then:
From Sender and Milo (17) we get the estimates: N=6·1011 cells, with a lifetime of 6,6 days and M=1·1010 cells with a lifetime of 3,5 days and T=7·1011. The neutrophils and monocytes have characteristic lifetimes, hence, the total kill time must be faster than the self-renewal time of tumor cells for successful eradication:
This relation was plotted for a range of parameter values using Matlab R2020b. The maximal VAF that can be eradicated is then: VAF ≤ 6,6 days·b·T/(2·τ· (N+M))
Statistics were performed using Graphpad Prism version 9 software. ELISPOT responses were evaluated using the distribution free resampling method by Moodie et al. (18) using the statistical software package R.
Our goal was to clarify if T cells specific to mutant CALR enrich in the bone marrow in patients after completion of therapeutic cancer vaccination against mutant CALR. Hence, four patients that were included in our vaccination trial (11) and displayed strong in vitro immune responses to the CALRLong36 vaccination epitope were selected as donors to generate T-cell cultures specific to the CALRLong36 peptide. Patient 1 and 9 (both ET) gave rise to CD4+ T-cell cultures, and patient 2 (PMF) and 7 (ET) to CD8+ T-cell cultures. The in vitro ELISPOT immune responses in these patients can be found in Figure 2A in the report on the vaccine trial (11). Specific T cells from these cultures were enriched using live cell FACS by stimulating specific cells with the CALRLong36 peptide for 18 hours and enriching live T cells co-expressing the T-cell activation markers CD107a and CD137 with unstimulated cells used to set the sorting gate (Figure 2A, top and middle). Purity analysis showed satisfactory enrichment (Figure 2A, bottom). Next, bone marrow derived T cells were enriched using live cell FACS on cryopreserved bone marrow mononuclear cells acquired at baseline and after trial completion (Figure 2B, top) with purity assessed after enrichment (Figure 2B, bottom). The quantity and purity of the enriched populations is provided in Table 1. The medical records from all patients showed that the acquired bone marrow aspirations were indeed bone marrow aspirations and not peripheral blood, a feat that is not uncommon in patients with MPN. Additionally, the description by the hematopathologists showed that all bone marrow specimens harbored lymphocyte infiltrates.
Figure 2 Enrichment of CALRLong36 specific T cells and bone marrow derived T cells using fluorescence activated cell sorting (FACS). (A) Four vaccinated CALRmut MPN-patients with a strong immune response to CALRLong36 at the end of trial were chosen for generation of CALRLong36 specific T-cell cultures. Patient peripheral blood mononuclear cells were enriched for CALRmut specific T cells as described in the methods section. The specific T cells were restimulated with peptide and labelled with fluorescence tagged antibodies. Specific cells were enriched as live CD3+, CD4+, CD107a+/CD137+ double positive events (patient 1 and 9) or as live CD3+, CD8+, CD107a+/CD137+ double positive events (patient 2 and 7) (top). Unstimulated cells were used to set sorting gate (middle). All enriched cells were subjected to purity analysis after enrichment (bottom). (B) Cryopreserved bone marrow mononuclear cells from the four patients were thawed and stained with dead cell marker and CD3-APC-H7 for enrichment of T cells derived from bone marrows acquired at baseline and at end-of-trial (top). Purity of the enriched fraction was analyzed post-enrichment (bottom).
As the presence of tumor specific T cells in the tumor microenvironment is necessary to attain a clinical response to cancer immune therapy (19), we investigated if T cells specific for mutant CALR enriched in the bone marrow after completion of therapeutic cancer vaccines. Enriched CALRmut specific T cells underwent TCRSeq to identify the CALRmut specific TCR clonotypes. Next, FACS sorted bone marrow derived T cells were analyzed by TCRSeq. Only clonotypes with two or more reads were included in the in silico analysis. TCRSeq of bone marrow derived T cells showed a high variation in the frequency of TCRs identified (Figures 3, 4 and Table 2). For example, only 3601 different α-chains were identified in the sample acquired from patient 1 at baseline (Figure 3A and Table 2), whereas 52996 different β-chains were identified in the baseline sample from patient 7 (Figure 4A and Table 2). Naturally, the TCR repertoire from the specific cultures was much lower compared to the bone marrow derived T cells (Figures 3, 4). Low frequency TCRs in the specific T cells could be attributed to background activity from allogenic feeder cells used to expand the specific T cells, and in the downstream analysis it was assumed that only TCRs with a frequency of > 1% in the specific T-cell samples represented CALRmut specific T cells, thereby reducing the amount of TCRs identified in the specific T-cell fraction (Table 2).
Figure 3 Quantification and clonotype tracking of T cells in patient 1 and 2. (A) Quantification of the number of different clonotypes in patient 2 baseline sample, end-of-trial sample and specific T cell sample with α-chain shown to the left and β-chain shown to the right. (B) Clonotype tracking of the 100 most prevalent clonotypes in the end-of-trial sample from patient 2 showing tracking trajectories to baseline sample and specific T cells with α-chain shown to the left and β-chain shown to the right. (C) Same as A for patient 2. (D) Same as B for patient 2.
Figure 4 Quantification and clonotype tracking of T cells in patient 7 and 9. (A) Quantification of the number of different clonotypes in patient 7 baseline sample, end-of-trial sample and specific T cell sample with α-chain shown to the left and β-chain shown to the right. (B) Clonotype tracking of the 100 most prevalent clonotypes in the end-of-trial sample from patient 7 showing tracking trajectories to baseline sample and specific T cells with α-chain shown to the left and β-chain shown to the right. (C) Same as A for patient 9. (D) Same as B for patient 9.
Generally, the TCRs in the baseline and end-of-trial samples remained relatively constant as clonotype tracking of the 100 most frequent clonotypes in the end-of-trial samples were present at high frequencies in the baseline samples too (Figures 3B, D, 4B, D).
Next, we identified TCRs that were present in the specific T-cell cultures with a frequency of > 1% and tracked these clonotypes to the baseline and end-of-trial samples (Figure 5). Surprisingly, clonotypes identified in the CALRmut specific T-cell fraction were identified in very low fractions in both baseline and end-of-trial samples (Figure 5 and Table 2). For patient 1, 23 α- and 22 β-chains were identified in the specific T cells. None of these were identified in the baseline sample, but three α− and six β-chains were identified in the end-of-trial bone marrow T cells albeit at a very low frequency (Table 2 and Supplementary Material 4). For patient 2, five α- and five β−chains were identified in the specific T cells, however none of the α−chains were identified in neither the baseline nor the end-of-trial sample. No β-chains from the specific cells were identified in the baseline sample, and only one β-chain was found in the end-of-trial sample at a frequency of 0,001% of all clonotypes (Table 2 and Supplementary Material 4). The specific T- cell culture from patient 7 was almost monoclonal displaying only one β-chain and two α-chains. The β-chain was not identified in neither of the bone marrow samples, and only one of the α-chains was found in the end-of-trial sample at a low frequency of 0,018% (Table 2 and Supplementary Material 4). In the patient 9 derived specific T cells, we managed to detect 19 α-chains and 13 β-chains respectively. Of the former none were detected in neither baseline nor in the end-of-trial samples. Of the latter, one β-chain was identified in the baseline sample at a frequency of 0,016% but was not identified in the end-of-trial sample. Another β-chain from the specific T-cell culture was identified in the end-of-trial sample at a frequency of 0,046%. However, in the specific T cell culture, the clonotype was only the 8th most frequent clonotype and displayed a frequency of only 3,5% of the total T cell repertoire making it unlikely that this clonotype represented a TCR specific to mutant CALR (Table 1 and Supplementary Material 4). Thus overall, we have found that CALRmut specific T cells do not enrich in the bone marrow in patients that have received therapeutic cancer vaccines against mutant CALR.
Figure 5 Tracking of clonotypes in CALRmut specific T cells to baseline and end-of-trial samples. (A) Patient 1 clonotype tracking with α-chain to the left and β-chain to the right. (B) Patient 2 clonotype tracking with α-chain to the left and β-chain to the right. (C) Patient 7 clonotype tracking with α-chain to the left and β-chain to the right. (D) Patient 9 clonotype tracking with α-chain to the left and β-chain to the right.
We have previously demonstrated that CALRmut specific T cells recognize autologous PB myeloid cells and hematopoietic stem cells (HSC) (7, 8). However, the failure to identify enrichment of specific T cells in the bone marrow and target the transformed cells herein could be explained by the fact that the enriched T cells in this study are unable to recognize autologous CALRmut cells. Thus, we investigated the recognition of autologous myeloid cells and PBL. These were enriched by FACS (Figure 6A) and used as target cells in an IFN-γ ELISPOT assay. In accordance with earlier findings (7, 8), the specific T cells recognized myeloid derived target cells, whereas PBL were not recognized (Figure 6B). Analysis by ddPCR confirmed that the monocytes were indeed CALRmut with a CALRmut VAF ranging from 0,94% to 52%. Surprisingly, all PBL fractions also showed some degree of mutants with a CALRmut VAF ranging from 0,67% to 14%. The enriched cells showed a high purity of all fractions, but we cannot rule out the possibility that some PBL fractions were contaminated with monocytes. However, low/absent responses to PBL could also be explained by the lower expression of both HLA-I (Figure 6C) and HLA-II (Figure 6D) on PBL compared to monocytes.
Figure 6 Responses by CALRmut specific T cells to autologous monocytes and peripheral blood lymphocytes. (A) Purity analysis of enriched fractions of peripheral blood lymphocytes (PBL) and monocytes with the depiction of the CALR mutant variant allele frequency in each enrichment determined by digital droplet PCR. (B) Responses by 105 specific T cells stimulated with either 104 autologous PBL or 104 autologous monocytes in an overnight IFN-γ ELISPOT assay. Normalized spot count shown for each patient (top) with representative ELISPOT wells for PBL and monocyte stimulated T cells below. (C) Expression of HLA-I by patient 2 and 7 monocytes and PBL was assessed by fluorescent activated cell sorting (FACS). (D) Expression of HLA-II by patient 1 and 9 monocytes and PBL was assessed by FACS. Mean fluorescence intensities (MFIs) were calculated by subtracting the MFI of the isotype control from the real staining MFI. Error bars depict standard error of the mean. ** denotes p<0.05 according to the conservative distribution free resampling method (DFR2x) (18).
Previous data suggests that the CALRmut VAF in PB provides a good estimate of PB myeloid cell CALRmut VAF (20). Thus, a 40% CALRmut VAF in PB indicates a PB myeloid cell CALRmut VAF of approximately the same value. As essentially all patients with CALRmut are heterozygous (21), this translates into 80% of all myeloid cells carrying the mutation and consequently posing as targets of specific T cells. Both monocytes and neutrophils are produced in high quantities in man (17) implying a high tumor burden in CALRmut MPN. Thus, failure of T-cell enrichment in the bone marrow could be explained by an overload of transformed cells in the periphery.
Based on assumptions described in the materials section we performed in silico analyses to quantify the transformed neutrophils and monocytes in the human organism and compare this to the amount of CALRmut specific T cells. The calculations rely on the fraction of CALRmut specific T cells of the entire T-cell pool (b). An ex vivo ELISPOT on PBMC from patient 2 and 7 showed no response in the former, whereas a response was identified in the latter with approximately 45 of 6 x105 assayed cells recognizing the CALRLong36 peptide (Figure 7). This provided a fraction of CALRmut specific T cells in the entire T-cell pool (b) of approximately 10-4. As patient 2 did not show any ex vivo response 10-5 was included as a value, as were more optimistic T-cell fractions of 10-1, 10-2 and 10-3. The period of time (τ) a T cell requires to kill a cancer cells was derived from Halle et al. (22) and three different killing times (90 minutes, 4 hours and 12 hours) were assumed. The fraction between the CALRmut cells in the periphery and CALRmut specific T cells was plotted displaying the number of kills required by each specific T cell to break even with the number of transformed cells (Figure 8A). This showed that even with a high value of b (10-1), the amount of CALRmut cells outnumbers the T cells. The median CALRmut VAF in the vaccination trial was 41%, and calculations showed that even if a highly unrealistic 1/10 of all T cells were specific to mutant CALR, the CALRmut specific T cells would require to kill several CALRmut cells. Provided a more realistic fraction of specific T cells of 1/10 000 (b=10−4) and a CALRmut VAF of 40%, each specific T cell needs to kill approximately 7 000 target cells (Figure 8A, left). Another factor to account for is the time required for the T cell to kill the target cell. Thus, we calculated the total kill time required provided different values of τ (Figures 8B–D). Assuming a killing time of 4 hours per kill, 10−4 specific T cells and a CALRmut PB VAF of 40%, approximately 400 days is needed for the cellular immune system to clear the mutant cells (Figure 8C, left). Providing a killing time of only 90 minutes, cellular immunity can only match a CALRmut VAF< 1%. With τ=90 minutes and b=10−4, the maximal VAF that can be eradicated is 0,6% (Figure 7B, right). Next, the maximal time a T cell should use to kill a transformed cell to balance the amount of newly formed transformed cells was analyzed (Figure 8E). A realistic T-cell fraction of 10−4 shows that even with a killing time of only one hour, the maximally allowed VAF that the T cells can control is 1% (Figure 8E). Finally, the maximum CALRmut VAF which the immune system can control was calculated (Figure 8F). Even provided a short killing time and a high fraction of specific T cells, the CALRmut VAF is not controllable by T-cell immunity. In conclusion, by using in silico analyses we provide data which imply that the levels of transformed cells in CALRmut MPN massively exceeds the number of cells which the cellular immune system is able to curtail.
Figure 7 Ex vivo IFN-γ ELISPOT responses in patient 2 and 7 against CALRLong36. (A) Spot formation in patient 2 and 7 PBMC upon stimulation with the CALRLong36 peptide with unstimulated cells as negative controls. (B) Images of ex vivo responses in patient 2 and 7 PBMC. Error bars depict standard error of the mean. ** denotes p<0.05 according to the conservative distribution free resampling method (DFR2x) (18).
Figure 8 In silico analysis of the burden of transformed cells compared to the amount of CALRmut specific T cells. (A) The amount of needed kills required by each CALRmut specific T cell to curtail the CALRmut monocytes and neutrophils as a function of the CALRmut variant allele frequency (VAF). The higher the CALRmut VAF, the more kills are required by each T cell. Left panel shows CALRmut VAF interval of 0-50% with the right panel displaying 0-2% interval for at better resolution of the kills required for a low CALRmut VAF. The value b denotes the frequency of CALRmut specific T cells of the entire T cell repertoire and shows that the lower the values of b, the higher number of kills are required per specific T cell. (B) The time required for CALRmut specific T cells to kill the CALRmut monocytes and neutrophils as a function of the CALRmut VAF provided a T cell needs 1,5 hours to kill one transformed cell. The value b denotes the frequency of CALRmut specific T cells of the entire T-cell repertoire. The horizontal line (red) denotes the lifetime of neutrophils i.e., the dominant self-renewal time of transformed cells. Hence, the total kill time must be below this line to allow for eradication of all transformed cells. (C) Same as B but with a killing time of 4 hours. (D) Same as B and C but with a killing time of 12 hours. (E) The maximum allowed CALRmut VAF allowed for the cellular immune system to break even with the amount of CALRmut monocytes and neutrophils as a function of the time required for each specific T cell to kill a transformed cell. The value b denotes the frequency of CALRmut specific T cells of the entire T-cell repertoire. Left panel shows a CALRmut VAF interval of 0-50% with the right panel displaying 0-2% interval for a better resolution of low CALRmut VAF. (F) The maximum allowed CALRmut VAF for the cellular immune system to break even with the amount of CALRmut monocytes and neutrophils as a function of the fraction of CALRmut specific T cells in the entire T-cell repertoire. The colored lines depict different values of the time (τ) required for each T cell to kill one transformed cell. Left panel shows a CALRmut VAF interval of 0-50% with the right panel displaying 0-2% interval for a better resolution of low CALRmut VAF.
Upon the discovery of the CALR exon 9 mutations in MPN it was speculated that the mutations could be targeted by the immune system and thus used for cancer immune therapy (4). Though it was shown that the CALR mutations induce a slightly lower expression of HLA-I in cells heterozygous for the mutation (23) which could result in immune escape, in vitro experiments demonstrated that autologous CALRmut peripheral blood myeloid cells and HSC are targets of CALRmut specific T cells (7, 8). This discovery spurred a therapeutic cancer vaccine trial against mutant CALR (11). The vaccines induced immune responses in PB T cells to mutant CALR but did not induce a clinical response. Even though immune responses can be difficult to compare between studies, it has been shown earlier that therapeutic peptide vaccines induce immune responses to the vaccination antigen which are comparable to the immune response identified in our vaccination trial, and that these immune responses were correlated to a clinical response (24, 25). Dendritic cells from patients with CALRmut PMF have been shown to express lower levels of costimulatory molecules which could have a negative impact on the immune response (26). The failure of the immune system to clear the mutant cells in MPN could be attributed to several additional immunosuppressive mechanisms that were just recently reviewed (12). In this study we aimed at identifying whether T cells specific to mutant CALR enrich in the bone marrow after therapeutic cancer vaccinations, as homing of T cells to the tumor microenvironment is important to attain a response to cancer immune therapy (19, 27). TCRSeq on specific T cells and bone marrow T cells showed that specific T cells do not enrich in the bone marrow in vaccinated patients. In addition, we found that the MPN bone marrow is not a T-cell excluded zone and demonstrated a relatively stable TCR repertoire in the bone marrow of patients over time. These data are in good line with a previous study in patients with PMF treated with anti-PD1 antibody pembrolizumab in which the TCR repertoire of PB T cells remained relatively stable (28).
In the current study we confirmed that the specific T cells generated from vaccinated patients recognize ex vivo isolated autologous CALRmut myeloid cells. ddPCR analysis of both monocytes and PBL showed that the monocytes were CALRmut, in addition to a fraction of PBL. This could be explained by either impurities in the enriched PBL, freely circulating DNA from mutant cells lysed during the enrichment procedure, or a fraction of PBL could in fact be CALRmut as earlier demonstrated (20). Analysis of HLA-I and HLA-II expression demonstrated a higher expression of both HLA-I and HLA-II by monocytes compared to PBL, which likely explains the higher response to these cells. The fact that CALRmut specific T cells recognize PB monocytes made us hypothesize that even in vaccinated patients, an imbalance between transformed cells and effector T cells in CALRmut MPN exists. This was confirmed by in silico analyses that were based with assumptions from the literature (17, 22). The fraction of CALRmut specific T cells in the T-cell pool was derived from an ex vivo ELISPOT on patient PBMC providing a rough estimate on the amount of T cells specific to mutant CALR of 1/10 000. One should bear in mind however, that the transformed cells may harbor other immunogenic antigens towards which a T-cell response might be evoked after the activation of CALRmut specific T cells. This phenomenon, termed epitope spreading (29), could result in a higher fraction of T cells specific to the transformed cells. Consequently, we included values of the fraction of CALRmut specific T cells in our calculations that might seem unrealistically high. Our results showed that even provided a high fraction of CALRmut specific T cells, each specific T cell would require to kill several hundreds of transformed cells to control the disease. As each T cell needs a certain time to kill a cancer cell, the time factor for elimination of the total pool of transformed cells was calculated. Results showed that in a CALRmut patient, T cells require hundreds or even thousands of days to control the transformed cells. Additionally, providing realistic parameters for tumor cell killing time and fraction of specific T cells, the maximum controllable CALRmut VAF was shown to be lower than 1%. Importantly, our calculations are based solely on PB myeloid cells and did not take bone marrow HSC into account. Considering the high amount of myeloid cell progenitors in the bone marrow and the fact that the CALR exon 9 mutations are identified in early hematopoietic progenitors (3) we envisage that the total burden of CALRmut cells is considerably higher than provided in our calculations. Thus, our calculations likely understate the total mass of transformed cells. Based on our findings we believe that the excess of transformed cells in the periphery results in a tumor cell overload for the specific T cells, which could be yet another factor explaining the lack of enrichment of specific T cells into the bone marrow.
Taken into a broader context we cannot completely exclude the possibility that we failed to detect CALRmut specific T cells during the TCRSeq. Firstly, we only included four patients in our analyses. Secondly, our methods to detect specific T cells have some limitations as we enriched and expanded the CALRmut specific T cells by repeated antigen and cytokine stimulations. Thus, our enrichment step could have resulted in a loss of specific cells during the enrichment procedure or during the expansion step. Ex vivo isolation of specific T cells by tetramers would have ruled out this factor, however we did not have the necessary tetramers at our disposal. Additionally, one should bear in mind that enrichment by tetramers is not perfect for isolation of specific T cells and tetramers may fail to capture functional T cells (30). The final enrichment was performed on T cells that had been stimulated with CALRLong36 overnight. This could activate non-specific T cells in vitro through paracrine stimulation with pro-inflammatory cytokines and these non-specific T cells would be sorted as specific cells. Consequently, TCRs with a frequency< 1% in the bulk cultures were excluded. None of the CALRmut specific TCRs were identified among the most frequent TCRs in the end-of-trial bone marrow specimens, and neither did the specific cells increase from baseline to end of trial. Finally, it cannot be ruled out, that the failure of T-cell homing is explained by the lack of homing receptors on T cells or homing receptor ligands in the bone marrow endothelium – a question that is important to investigate, but outside the scope of the current project. Though there are many other possible explanations beyond the lack of clinical response to the vaccines, we believe that the in silico results provided above are noteworthy as they clearly demonstrate a state of tumor cell overload in MPN. Several publications support this notion. Firstly, a phase I trial of the PD1-inhibitor pembrolizumab in 10 patients with PMF, of whom two were CALRmut, failed to induce a clinical response (28). This could be ascribed to the lack of immunogenic neo-antigens in the CALRwt patients, however Schischlik and coworkers demonstrated that several somatic mutations in MPN generate neo-epitopes with a high HLA-I binding affinity (31). Thus, mutant CALR is potentially not the only target for cellular immunity, yet patients treated with check-point-inhibitors did not show any clinical response to therapy. This lack of response could be explained by the highly immunosuppressive TME in MPN (12, 32), but also by the fact, that the mutant VAF in the reported trial was relatively high reflecting a high peripheral tumor cell load. Of note, T cells from CALRmut patients included in the trial recognized mutant CALR underscoring that CALRmut specific T cells were present in these patients but failed to induce a clinical response (33). Secondly, a murine vaccination study in a del52 knock-in model of mutant CALR failed to induce a reduction in the CALRmut VAF. The lack of effect was ascribed to poor T-cell responses to the vaccination epitopes, however the high tumor load could also explain the lack of effect on the CALRmut VAF (34). Thirdly, another in vivo murine vaccination study in a CALRmut model demonstrated a clinical response to the vaccines. This study employed a different treatment schedule than the former, but the model also differed as the tumor cells were CALRmut transduced lymphoma cells (8). The important difference here being that the lymphoma model is a compartmentalized model of CALRmut MPN, whereas the del52 knock-in is a global model of MPN. In the former model, the CALRmut cells are present in a limited number, confined to the tumor and thereby present in a limited number whereas in the latter, transformed cells are present in the entire organism and at a much higher level.
The findings in the present study fits well with data on cancer immune therapy in other hematological cancers. In relapsed/refractory B cell acute lymphoblastic leukemia, an increased tumor burden is negatively associated with survival in patients treated with chimeric antigen receptor T cells (35). In acute myeloid leukemia (AML) and multiple myeloma an increased tumor burden is negatively correlated to overall survival in patients treated with allogeneic hematopoietic stem cell transplantation (allo-HSCT) (36, 37). To our knowledge the impact of the tumor burden on response to allo-HSCT in PMF has not been investigated. Hence, we are currently investigating this in a retrospective study in patients with PMF treated with allo-HSCT.
In conclusion we have shown that therapeutic cancer vaccination against mutant CALR does not result in enrichment of mutation specific T cells in the bone marrow. The recognition and killing of bone marrow resident CALRmut HSC is of utmost importance to attain a response to cancer immune therapy in MPN as killing of the transformed HSC in the bone marrow will prevent entry of more mature subsets into the peripheral blood and ultimately cure the patient. The lack of effect to therapeutic cancer vaccination against mutant CALR could be explained by the highly immunosuppressive TME in MPN. However, based on our in vitro and in silico experiments we speculate that the high tumor burden in PB of patients with MPN outcompetes cellular immunity. Thus, we envisage high tumor load and high tumor cell turnover as an additional immunosuppressive mechanism in MPN that needs to be accounted upon designing cancer immune therapy for patients with MPN.
The datasets presented in this article are not readily available because of the Danish Law on data protection and the GDPR rules. Requests to access the datasets should be directed to the corresponding author.
The studies involving human participants were reviewed and approved by Regional Ethics Committee for Zealand Region, Denmark Approval number SJ-680. The patients/participants provided their written informed consent to participate in this study.
MH conceived the project, performed experiments, analyzed data, and wrote the manuscript. MA performed experiments, analyzed data, and wrote the manuscript. ST performed experiments, analyzed data, and wrote the manuscript. SA performed experiments. TL performed experiments and wrote the manuscript. JH performed experiments. VS and LK performed experiments and analyzed data. JO performed experiments and analyzed the data. MG analyzed the data and provided vital reagents. HH conceived the project and analyzed data. MHA conceived the project, analyzed data, and wrote the manuscript. All authors contributed to the article and approved the submitted version.
The authors declare that this study received funding from the BRIDGE – Translational Excellence Programme (bridge.ku.dk) at the Faculty of Health and Medical Sciences, University of Copenhagen, funded by the Novo Nordisk Foundation. Grant agreement no. NNF20SA0064340. Sundhedsstyrelsen – sagsnummer 05-0400-18. Sundhedsstyrelsen – sagsnummer 05-0400-50, Det Frie Forskningsråd – sagsnummer 0134-00072B, Kræftens Bekæmpelse– sagsnummer R149-A10159. The funders were not involved in the study design, collection, analysis, interpretation of data, the writing of this article nor the decision to submit it for publication.
We thank technicians Merete Jonassen and Anette Højgaard Andersen for excellent technical assistance and MSc, PhD Christopher Aled Chamberlain for teaching MH to use the Immunarch software package.
The authors declare that the research was conducted in the absence of any commercial or financial relationships that could be construed as a potential conflict of interest.
All claims expressed in this article are solely those of the authors and do not necessarily represent those of their affiliated organizations, or those of the publisher, the editors and the reviewers. Any product that may be evaluated in this article, or claim that may be made by its manufacturer, is not guaranteed or endorsed by the publisher.
The Supplementary Material for this article can be found online at: https://www.frontiersin.org/articles/10.3389/fimmu.2023.1240678/full#supplementary-material
1. Campbell PJ, Green AR. The myeloproliferative disorders. N Engl J Med (2006) 355:2452–66. doi: 10.1056/NEJMra063728
2. Kralovics R, Passamonti F, Buser AS, Teo S-S, Tiedt R, Passweg JR, et al. A gain-of-function mutation of JAK2 in myeloproliferative disorders. N Engl J Med (2005) 352:1779–90. doi: 10.1056/NEJMoa051113
3. Nangalia J, Massie CE, Baxter EJ, Nice FL, Gundem G, Wedge DC, et al. Somatic CALR mutations in myeloproliferative neoplasms with nonmutated JAK2. N Engl J Med (2013) 369:2391–405. doi: 10.1056/NEJMoa1312542
4. Klampfl T, Gisslinger H, Harutyunyan AS, Nivarthi H, Rumi E, Milosevic JD, et al. Somatic mutations of calreticulin in myeloproliferative neoplasms. N Engl J Med (2013) 369:2379–90. doi: 10.1056/NEJMoa1311347
5. Pietra D, Rumi E, Ferretti VV, Buduo CAD, Milanesi C, Cavalloni C, et al. Differential clinical effects of different mutation subtypes in CALR-mutant myeloproliferative neoplasms. Leukemia (2016) 30:431–8. doi: 10.1038/leu.2015.277
6. Holmström MO, Riley CH, Svane IM, Hasselbalch HC, Andersen MH. The CALR exon 9 mutations are shared neoantigens in patients with CALR mutant chronic myeloproliferative neoplasms. Leukemia (2016) 30:2413–6. doi: 10.1038/leu.2016.233
7. Holmström MO, Martinenaite E, Ahmad SM, Met Ö, Friese C, Kjær L, et al. The calreticulin (CALR) exon 9 mutations are promising targets for cancer immune therapy. Leukemia (2018) 32:429–37. doi: 10.1038/leu.2017.214
8. Gigoux M, Holmström MO, Zappasodi R, Park JJ, Pourpe S, Bozkus CC, et al. Calreticulin mutant myeloproliferative neoplasms induce MHC-I skewing, which can be overcome by an optimized peptide cancer vaccine. Sci Transl Med (2022) 14. doi: 10.1126/scitranslmed.aba4380
9. Holmström MO, Ahmad SM, Klausen U, Bendtsen SK, Martinenaite E, Riley CH, et al. High frequencies of circulating memory T cells specific for calreticulin exon 9 mutations in healthy individuals. Blood Cancer J (2019) 9. doi: 10.1038/s41408-018-0166-4
10. Holmström MO, Cordua S, Skov V, Kjær L, Pallisgaard N, Ellervik C, et al. Evidence of immune elimination, immuno-editing and immune escape in patients with hematological cancer. Cancer Immunol Immunother (2020) 69:315–24. doi: 10.1007/s00262-019-02473-y
11. Handlos Grauslund J, Holmström MO, Jørgensen NG, Klausen U, Weis-Banke SE, El Fassi D, et al. Therapeutic cancer vaccination with a peptide derived from the calreticulin exon 9 mutations induces strong cellular immune responses in patients with CALR-mutant chronic myeloproliferative neoplasms. Front Oncol (2021) 11:637420. doi: 10.3389/fonc.2021.637420
12. Holmström MO, Hasselbalch HC, Andersen MH. Cancer immune therapy for Philadelphia chromosome-negative chronic myeloproliferative neoplasms. Cancers (Basel) (2020) 12:1–21. doi: 10.3390/cancers12071763
13. Holmström MO, Andersen MH. Healthy donors harbor memory T cell responses to RAS neo-antigens. Cancers (Basel) (2020) 12:1–16. doi: 10.3390/cancers12103045
14. Kjær L, Cordua S, Holmström MO, Thomassen M, Kruse TA, Pallisgaard N, et al. Differential dynamics of CALR mutant allele burden in myeloproliferative neoplasms during interferon alfa treatment. PloS One (2016) 11:e0165336. doi: 10.1371/journal.pone.0165336
15. Cordua S, Kjaer L, Skov V, Pallisgaard N, Hasselbalch HC, Ellervik C. Prevalence and phenotypes of JAK2 V617F and Calreticulin mutations in a Danish general population. Blood (2019) 134:469–79. doi: 10.1182/blood.2019001113
16. ImmunoMind Team. immunarch: an R Package for Painless Bioinformatics Analysis of T-Cell and B-Cell Immune Repertoires. (2019). doi: 10.5281/zenodo.3367200
17. Sender R, Milo R. The distribution of cellular turnover in the human body. Nat Med (2021) 27:45–8. doi: 10.1038/s41591-020-01182-9
18. Moodie Z, Price L, Gouttefangeas C, Mander A, Janetzki S, Löwer M, et al. Response definition criteria for ELISPOT assays revisited. Cancer Immunol Immunother (2010) 59:1489–501. doi: 10.1007/s00262-010-0875-4
19. Sackstein R, Schatton T, Barthel SR. T-lymphocyte homing: An underappreciated yet critical hurdle for successful cancer immunotherapy. Lab Investig (2017) 97:669–97. doi: 10.1038/labinvest.2017.25
20. Kjaer L, Orebo Holmström M, Cordua S, Andersen MH, Svane IM, Thomassen M, et al. Sorted peripheral blood cells identify CALR mutations in B- and T-lymphocytes. Leuk Lymphoma (2017) 59:973–7. doi: 10.1080/10428194.2017.1359743
21. Jeromin S, Kohlmann A, Meggendorfer M, Schindela S, Perglerova K, Nadarajah N, et al. Next-generation deep-sequencing detects multiple clones of CALR mutations in patients with BCR-ABL1 negative MPN. Leukemia (2016) 30:973–6. doi: 10.1038/leu.2015.207
22. Halle S, Halle O, Förster R. Mechanisms and dynamics of T cell-mediated cytotoxicity in vivo. Trends Immunol (2017) 38:432–43. doi: 10.1016/j.it.2017.04.002
23. Arshad N, Cresswell P. Tumor-associated calreticulin variants functionally compromise the peptide loading complex and impair its recruitment of MHC-I. J Biol Chem (2018) 293:9555–69. doi: 10.1074/jbc.RA118.002836
24. Iversen TZ, Engell-Noerregaard L, Ellebaek E, Andersen R, Larsen SK, Bjoern J, et al. Long-lasting disease stabilization in the absence of toxicity in metastatic lung cancer patients vaccinated with an epitope derived from indoleamine 2,3 dioxygenase. Clin Cancer Res (2014) 20:221–32. doi: 10.1158/1078-0432.CCR-13-1560
25. Rahma OE, Hamilton JM, Wojtowicz M, Dakheel O, Bernstein S, Liewehr DJ, et al. The immunological and clinical effects of mutated ras peptide vaccine in combination with IL-2, GM-CSF, or both in patients with solid tumors. J Transl Med (2014) 12:1–12. doi: 10.1186/1479-5876-12-55
26. Romano M, Sollazzo D, Trabanelli S, Barone M, Polverelli N, Perricone M, et al. Mutations in JAK2 and Calreticulin genes are associated with specific alterations of the immune system in myelofibrosis. Oncoimmunology (2017) 6. doi: 10.1080/2162402X.2017.1345402
27. Franciszkiewicz K, Boissonnas A, Boutet M, Combadière C, Mami-Chouaib F. Role of chemokines and chemokine receptors in shaping the effector phase of the antitumor immune response. Cancer Res (2012) 72:6325–32. doi: 10.1158/0008-5472.CAN-12-2027
28. Hobbs G, Bozkus CC, Moshier E, Dougherty M, Bar-Natan M, Sandy L, et al. PD-1 inhibition in advanced myeloproliferative neoplasms. Blood Adv (2021) 5:5086–97. doi: 10.1182/BLOODADVANCES.2021005491
29. Gulley JL, Madan RA, Pachynski R, Mulders P, Sheikh NA, Trager J, et al. Role of antigen spread and distinctive characteristics of immunotherapy in cancer treatment. J Natl Cancer Inst (2017) 109:1–9. doi: 10.1093/jnci/djw261
30. Dolton G, Tungatt K, Lloyd A, Bianchi V, Theaker SM, Trimby A, et al. More tricks with tetramers: A practical guide to staining T cells with peptide-MHC multimers. Immunology (2015) 146. doi: 10.1111/imm.12499
31. Schischlik F, Jäger R, Rosebrock F, Hug E, Schuster M, Holly R, et al. Mutational landscape of the transcriptome offers putative targets for immunotherapy of myeloproliferative neoplasms. Blood (2019) 134:199–210. doi: 10.1182/blood.2019000519
32. Barosi G. An immune dysregulation in MPN. Curr Hematol Malig Rep (2014) 9:331–9. doi: 10.1007/s11899-014-0227-0
33. Bozkus CC, Roudko V, Finnigan JP, Mascarenhas J, Hoffman R, Iancu-Rubin C, et al. Immune checkpoint blockade enhances shared neoantigen-induced T-cell immunity directed against mutated calreticulin in myeloproliferative neoplasms. Cancer Discov (2019) 9:1192–207. doi: 10.1158/2159-8290.CD-18-1356
34. Balligand T, Achouri Y, Pecquet C, Gaudray G, Colau D, Hug E, et al. Knock-in of murine Calr del52 induces essential thrombocythemia with slow-rising dominance in mice and reveals key role of Calr exon 9 in cardiac development. Leukemia (2020) 34:510–21. doi: 10.1038/s41375-019-0538-1
35. Li M, Xue SL, Tang X, Xu J, Chen S, Han Y, et al. The differential effects of tumor burdens on predicting the net benefits of ssCART-19 cell treatment on r/r B-ALL patients. Sci Rep (2022) 12. doi: 10.1038/s41598-021-04296-3
36. Ogawa H, Ikegame K, Daimon T, Uchida N, Fukuda T, Kakihana K, et al. Impact of pretransplant leukemic blast% in bone marrow and peripheral blood on transplantation outcomes of patients with acute myeloid leukemia undergoing allogeneic stem cell transplantation in non-CR. Bone Marrow Transplant (2018) 53:478–82. doi: 10.1038/s41409-017-0028-x
37. Mosebach J, Shah S, Delorme S, Hielscher T, Goldschmidt H, Schlemmer HP, et al. Prognostic significance of tumor burden assessed by whole-body magnetic resonance imaging in multiple myeloma patients treated with allogeneic stem cell transplantation. Haematologica (2018) 103:336–43. doi: 10.3324/haematol.2017.176073
Keywords: myeloproliferative neoplasms, calreticulin, cancer vaccines, immune escape, adaptive immunity
Citation: Holmström MO, Andersen M, Traynor S, Ahmad SM, Lisle TL, Handlos Grauslund J, Skov V, Kjær L, Ottesen JT, Gjerstorff MF, Hasselbalch HC and Andersen MH (2023) Therapeutic cancer vaccination against mutant calreticulin in myeloproliferative neoplasms induces expansion of specific T cells in the periphery but specific T cells fail to enrich in the bone marrow. Front. Immunol. 14:1240678. doi: 10.3389/fimmu.2023.1240678
Received: 15 June 2023; Accepted: 12 July 2023;
Published: 17 August 2023.
Edited by:
Amirhesam Babajani, Iran University of Medical Sciences, IranReviewed by:
Jifeng Yu, First Affiliated Hospital of Zhengzhou University, ChinaCopyright © 2023 Holmström, Andersen, Traynor, Ahmad, Lisle, Handlos Grauslund, Skov, Kjær, Ottesen, Gjerstorff, Hasselbalch and Andersen. This is an open-access article distributed under the terms of the Creative Commons Attribution License (CC BY). The use, distribution or reproduction in other forums is permitted, provided the original author(s) and the copyright owner(s) are credited and that the original publication in this journal is cited, in accordance with accepted academic practice. No use, distribution or reproduction is permitted which does not comply with these terms.
*Correspondence: Morten Orebo Holmström, bWhvbDAxMjlAcmVnaW9uaC5kaw==
†ORCID: Morten Orebo Holmström, orcid.org/0000-0002-3764-8578
Disclaimer: All claims expressed in this article are solely those of the authors and do not necessarily represent those of their affiliated organizations, or those of the publisher, the editors and the reviewers. Any product that may be evaluated in this article or claim that may be made by its manufacturer is not guaranteed or endorsed by the publisher.
Research integrity at Frontiers
Learn more about the work of our research integrity team to safeguard the quality of each article we publish.