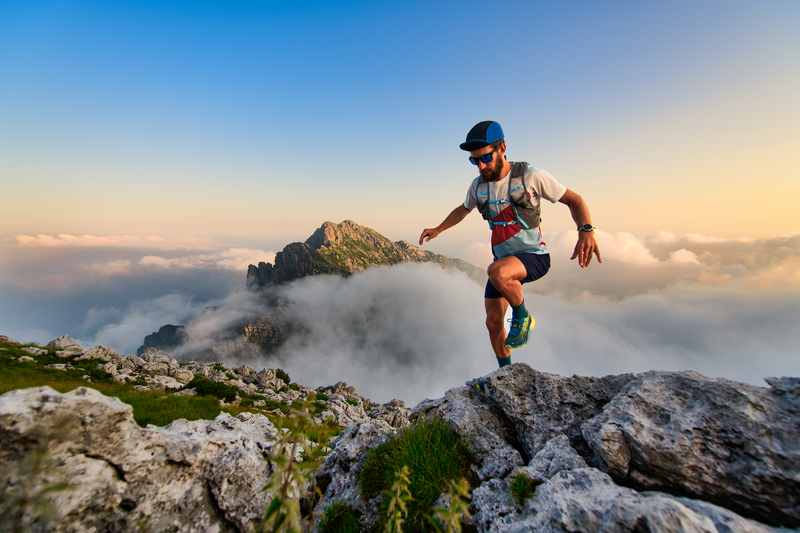
95% of researchers rate our articles as excellent or good
Learn more about the work of our research integrity team to safeguard the quality of each article we publish.
Find out more
REVIEW article
Front. Immunol. , 04 January 2024
Sec. Cancer Immunity and Immunotherapy
Volume 14 - 2023 | https://doi.org/10.3389/fimmu.2023.1238827
This article is part of the Research Topic Development of nanotherapeutics with multi-functionalities for targeting cancer cells View all 7 articles
Nanoparticles have unique physical and chemical properties and are currently widely used in disease diagnosis, drug delivery, and new drug development in biomedicine. In recent years, the role of nanomedical technology in cancer treatment has become increasingly obvious. Autophagy is a multi-step degradation process in cells and an important pathway for material and energy recovery. It is closely related to the occurrence and development of cancer. Because nanomaterials are highly targeted and biosafe, they can be used as carriers to deliver autophagy regulators; in addition to their favorable physicochemical properties, nanomaterials can be employed to carry autophagy inhibitors, reducing the breakdown of chemotherapy drugs by cancer cells and thereby enhancing the drug’s efficacy. Furthermore, certain nanomaterials can induce autophagy, triggering oxidative stress-mediated autophagy enhancement and cell apoptosis, thus constraining the progression of cancer cells.There are various types of nanoparticles, including liposomes, micelles, polymers, metal-based materials, and carbon-based materials. The majority of clinically applicable drugs are liposomes, though other materials are currently undergoing continuous optimization. This review begins with the roles of autophagy in tumor treatment, and then focuses on the application of nanomaterials with autophagy-regulating functions in tumor treatment.
Nanomaterials, including metal-based nanomaterials, carbon-based nanomaterials, lipid-based nanomaterials, and nano-polymer materials, have excellent physical or chemical characteristics such as controllable shape and size, large specific surface area, good biocompatibility, and easy surface modification (1). Particularly, some nanomedicines can improve drug pharmacokinetics and pharmacological properties, protect drugs from degradation in blood vessels, increase drug solubility, and deliver drugs in a tissue- or cell-specific manner, reducing drug accumulation in non-target organs and side effects (2). It appears to be successful to use nanoparticles as carriers for biomolecules, and some nanoparticles can influence numerous biochemical pathways and thus affect cellular metabolic activity. As a result, they are currently utilized extensively in biomedicine for a variety of purposes, including illness screening and diagnostics, drug delivery, the creation of novel drugs, etc.
Early cancer detection can result in prompt treatment and the best results, which contributes to much cheaper medical expenses and higher 5-year survival rates. Chemotherapy, when paired with surgery or radiotherapy, is the most crucial systemic therapy in the treatment of cancer because it increases the likelihood that local disease will be curable. Conventional chemotherapy can potentially harm normal cells and lacks a clear targeting mechanism. The patient’s quality of life will likely continue to decline and there is a chance that systemic toxicity will result (3). Nanotechnology’s development has given rise to hope for the early detection and treatment of cancer because it can improve the targeting capacity of anticancer drugs, boost local drug efficacy, thereby reducing systemic toxicity, improve diagnostic sensitivity, boost biological imaging, and improve radiotherapy (4).
The key material degradation system known as autophagy recycles big macromolecules or organelles to lysosomes where they are broken down into recycling-friendly tiny molecules like amino acids and monosaccharides. By removing defective or damaged organelles, autophagy serves a crucial role in preserving intracellular environmental homeostasis. Autophagy is closely linked to the occurrence and progression of diseases like cancer, diabetes, and neurological illnesses, according to recent studies (5). When compared to its involvement in other diseases, autophagy’s role in cancer is noticeably more complicated. It is thought to have a variety of functions depending on the stage of the disease; in the early stages of cancer, it mostly functions as an anti-cancer mechanism to slow the growth of the disease (6). It encourages the growth and spread of cancer in its advanced stages (via mechanisms such oxidative stress, nutritional restriction, or resistance) (7). As a result, controlling autophagy offers a potential means of preventing the growth of malignant tumors.
Several autophagy regulators face challenges, including poor solubility, non-specific distribution, and incompatibility with tumor acidic microenvironments (8). Using nanomaterials as drug carriers can enhance the therapeutic effect of the drug and reduce the occurrence of tumor resistance and chemotherapy side effects. In addition to acting as a nano-carrier, many nanoparticles themselves have autophagy-regulating effects, and specific material nanoparticles can also change multiple signaling molecule pathways in autophagy regulation. Therefore, research and development of nanomedicines for cancer targeted therapy with autophagy regulation may have great potential to provide ideas for the treatment of refractory and resistant cancers.
This article reviews the relationship between autophagy and tumors, the current application of drugs with autophagy effects in tumor treatment. Finally, we introduce the application of nanomaterials in tumor autophagy therapy and analyzes the development prospects of nanomedicines with important roles in regulating autophagy.
It is currently generally believed that autophagy consists of six steps: initiation of autophagy, nucleation of autophagosomes, extension of autophagosome membranes, closure of autophagosome membranes, combination of autophagosomes with lysosomes, degradation and reuse of contents. The process and important targets of autophagy are shown in Figure 1.
Figure 1 The basic process of autophagy. ULK1, unc-51-like kinase 1; mTORC1, Mammalian target of rapamycin complex 1; ATG, autophagy-related; AMPK, AMP-activated protein kinase; VPS34, vacuolar protein sorting 34; PI3P, Phosphatidylinositol 3-phosphate; AMBRA1, Activating molecule in Beclin-1-regulated autophagy.
The initiation of autophagy is closely related to the ULK1 complex, which includes the serine/threonine protein kinase ULK1, as well as ATG13, FIP200 (a protein that interacts with focal adhesion kinase FAK), and ATG101 (9), as shown in Figure 1. Under stable intracellular conditions, the mTORC1 complex binds to the ULK1 complex and phosphorylates ULK1 and ATG13, thereby inhibiting the initiation of autophagy (10). Under conditions of nutrient deprivation or starvation, AMPK responds to low ATP levels and can phosphorylate TSC2 (tuberous sclerosis protein), which enhances its inhibition of Rheb and suppresses mTORC1 activity (11). AMPK can also directly activate the ULK1 complex, thereby inducing autophagosome nucleation and elongation.
The ULK1 complex leads to the activation of the ATG6-VPS34 complex, which includes Beclin1 (ATG6), Bcl-2, VPS34, VPS15, ATG14L, and AMBRA-1 (12). This crucial complex is involved in the nucleation of the autophagosome, as illustrated in Figure 1. It generates phosphatidylinositol 3-phosphate (PI3P) on the cellular membrane in preparation for ensuing membrane curvature. Within the cytoplasm, cells form a small membranous structure called the isolation membrane, which extends continuously from both sides, flattens, and is referred to as the pre-autophagosome (PAS). The membrane originates from independent specialized membrane structures or organelles such as mitochondria, endoplasmic reticulum (ER), or Golgi complex, with the most common forming place at ER-mitochondria contact sites (13, 14).
The PAS interacts with the VPS34 complex under the action of two ubiquitin-like systems: the ATG12-ATG5-ATG16L conjugation system and the phosphatidylethanolamine/light chain 3 (PE/LC3) lipidation system, just as depicted in the second small box in Figure 1. This interaction extends the length of the membrane, which transforms from a crescent-shaped structure to a fully closed double-membrane structure called the autophagosome. The contents of the autophagosome consist of captured aged proteins, organelles, protein aggregates, and eventually become mature sealed autophagic vacuoles. Regulatory proteins such as Beclin1, ATG14L, AMBRA1, and ultraviolet radiation resistance-associated gene product (UVRAG) interact to modulate the extension of the double membrane (15). ATG12 is conjugated to ATG5 with the action of ATG10 and ATG7, and forms a complex with ATG16L. The precursor of LC3 is cleaved by ATG4 to generate a soluble form (LC3-I), which is then converted to a lipidated form (LC3-II) after the action of molecules such as ATG3 and ATG7 (16). LC3-II subsequently binds to the enriched lipid phosphatidylethanolamine (PE) in the newly formed autophagosomal membrane.
The autophagosome fuses with the lysosome, forming an autolysosome. This process is facilitated by proteins such as ATG15, ATG22, lysosome-associated membrane proteins (LAMPs), UVRAG, and the small GTPase Rab7 (12). UVRAG can form a complex with BECN1 and VPS34, regulating the formation of autophagolysosomes (17). The final step is the degradation and reuse of contents. Acid hydrolases inside the autolysosome degrade vesicle contents, which become degradative autolysosome contents. Degraded lysosomal enzymes release amino acids, monosaccharides, and other small molecules, which then exit through nutrient transport proteins for use in cell growth and development.
It is well known that many cancers have altered autophagy activity, and that autophagy can both promote and hinder the occurrence and progression of cancer. Some of the ways autophagy affects cancer are shown in Figure 2.
Figure 2 The complex role of autophagy in tumorigenesis: (A) Autophagy can reduce reactive oxygen species (ROS) levels and accumulation of p62, thereby reducing DNA damage and carcinogenesis in normal cells. Excessive autophagy can induce apoptosis in cancer cells, thereby inhibiting the occurrence and growth of cancer. (B) The complex role of autophagy in tumorigenesis involves providing nutrients and energy to tumor cells, inducing drug resistance in tumor cells, promoting tumor cell metastasis, and facilitating tumor development (see main text for details).
Autophagy works by eliminating toxic compounds within cells, maintaining metabolic equilibrium, and reducing DNA damage. When autophagy levels decrease, cells are unable to break down defective long-lived proteins and damaged organelles, leading to the accumulation of damaged proteins and the assembly of oncogenic p62 protein. Reactive oxygen species (ROS), which impact DNA sequence, structure, and function directly or indirectly, are produced in greater quantities in aging or damaged mitochondria that cannot effectively eliminate them (18). This results in DNA damage and genetic changes, ultimately leading to cancer. Excessive autophagy can induce autophagic death of tumor cells, thereby inhibiting cancer. Under continued stress and progressive autophagy, cells will die due to excessive self-consumption. The death of such cells often exhibits autophagic characteristics, manifested by overexpression of Beclin1 in the cells and the production of a large number of autophagosomes in the cells (19).
Autophagy can help tumor cells survive and multiply once they have formed. Cancer cells use autophagic mechanisms to combat nutritional deprivation and hypoxia when rapidly expanding tumor cells in solid tumors lack nutrients and have poor blood supply. Autophagy fuels the growth of tumor cells by destroying intracellular organelles and proteins, which helps them survive under stressful circumstances (20). Additionally, tumor resistance to therapeutic medications may be a result of cell-protective autophagy, which favors the survival of tumor cells. According to research, autophagy can influence antigen presentation by lowering MHC-I surface levels, which lowers anti-tumor T cell responses and fosters tumor growth (21). The ability of cancer cells to migrate and invade can also be improved by autophagy. It can strengthen resistance to anoikis apoptosis, accelerate the epithelial-mesenchymal transition in tumor cells, increase the release of pro-migratory cytokines like IL-6 through pathways involving TGF-β, and promote cancer cell invasion and metastasis (22).
Given the complicated function that autophagy plays in cancer, autophagy modulators can be used to target autophagy and prevent the spread of cancer. For instance, the use of autophagy inducers in certain early-stage malignancies might cause apoptotic cell death, which can be used in cancer treatment. On the other side, autophagy aids in the survival and resilience of cancer cells to chemotherapy and targeted therapy. Therefore, the use of autophagy inhibitors is required to support the therapeutic benefits of chemotherapy and targeted therapy medicines. The stages of action and regulatory mechanisms of several autophagy modulators are shown in Figure 3.
Figure 3 Several pharmacological interventions available to modulate autophagy. 3-MA, 3-methyladenine; BECN1, Beclin 1; CQ, Chloroquine; HCQ, hydroxychloroquine.
When there is an initiation problem, stimulating upstream autophagosome formation in tumor cells holds the potential to normalize autophagic flux (23). Furthermore, besides inducing apoptotic cell death in cancer cells with apoptosis resistance, autophagy can also act as an alternative cell death mechanism.
Metformin, a first-line drug for type 2 diabetes, has demonstrated a notable capacity to inhibit tumor growth. Its mechanism of action involves activating AMPK and suppressing mTOR, resulting in the inhibition of tumor development (24). Researchers have uncovered that metformin may trigger autophagic cell death, enhancing the susceptibility of sorafenib-resistant liver cancer cells (25). However, a phase 2 double-blind, randomized, and placebo-controlled trial (NCT01210911) indicated that individuals with advanced pancreatic cancer did not significantly benefit from taking metformin at the typical diabetic levels. The more potent biguanides may work better when coupled in tumor patients who are more sensitive to energy stress.
Another AMPK activator is simvastatin, which belongs to the statin class of drugs. Simvastatin has poor solubility in water and is currently used as an effective drug for treating high cholesterol and high triglyceride levels. Multiple reports have confirmed the anti-tumor potential of statin drugs in various cancers (26–28). The exact molecular mechanism by which simvastatin exerts its anticancer activity is not fully understood but may be related to AMPK activity regulation. Simvastatin stimulates autophagy, according to Wei et al., by activating mTOR, increasing LC3B and BECN1, and decreasing autophagosome-lysosome fusion (29). Furthermore, simvastatin’s anticancer effects may also be associated with its inhibition of Shh signaling, increased levels of RhoA-GTP, increased levels of HIF-1α, and induction of p38 MAPK activation etc. (30–32).
Rapamycin is a first-generation mTOR inhibitor and a macrocyclic lactone compound. Its derivatives (Rapalogs), including temsirolimus, everolimus, and ridaforolimus, have similar mechanisms of action, i.e., binding to the FRB domain of mTOR, altering the conformation of mTOR, and inhibiting mTORC1 kinase activity. Rapalogs have been approved as anti-cancer drugs (33), and the close relationship between the antitumor activity of rapamycin and its regulation of autophagy is evident. In vitro research by Lupinacci et al. revealed that rapamycin-induced autophagy mediated by p75NTR is a significant mechanism for tumor regression in Kaposi’s sarcoma (34). Due to the fact that rapamycin primarily induces autophagy through mTOR inhibition, when used in combination with an autophagy inhibitor whose mechanism involves inhibiting autophagosome fusion and degradation, its autophagy-inducing effect may be enhanced. Recent studies have also demonstrated this, showing that the combination of chloroquine and rapamycin synergistically targets autophagy, leading to an increase in the number of autophagosomes and apoptosis in 93T449 WDLS cells, thereby exerting a therapeutic effect on well-differentiated liposarcoma (35). A phase II clinical trial evaluating the efficacy of temsirolimus in patients with recurrent glioblastoma (NCT00016328) demonstrated reduced tumor-associated T2 hyperintensity on neuroimaging using magnetic resonance imaging (36). Despite the demonstrated inhibitory effects of rapamycin on various cancer cells, it still exhibits limitations in the treatment of drug-resistant cancers.
Dactolisib (BEZ235) is a hydrophobic synthetic imidazole and quinoline derivative, belonging to the second-generation mTOR inhibitors. It is an ATP-competitive inhibitor that binds to the ATP-binding site in the kinase domain of mTOR, thus simultaneously inhibiting mTORC1 and mTORC2, and partially inhibiting PI3K (37). Oral administration of highly selective PI3K/mTOR dual inhibitors may play a crucial role in synergistic cancer therapy. Helmy et al. demonstrated the significant impact of BEZ-235, when co-administered with Osimerin (an NF-κB inhibitor), in inhibiting the PI3K/Akt/mTOR/NF-κB axis, inducing apoptosis in HCT-116 colorectal cancer cells (38). Despite the favorable synergistic chemotherapeutic effects demonstrated by this combination in cancer treatment, the potential toxicity also underscores the impetus for further preclinical and clinical research involving lower concentrations of BEZ-235. BEZ-235 also exhibits the capability to induce autophagy and enhance the sensitivity of hepatocellular carcinoma cells to sorafenib by inhibiting PI3K/Akt/mTOR (39). Similar to the aforementioned rapamycin, it can be combined with chloroquine to achieve a more potent therapeutic effect than monotherapy (40). Furthermore, BEZ-235 demonstrates favorable therapeutic effects on cancers with mutations in autophagy-related targets. A study revealed that BEZ-235 may exert anti-tumor effects in mutant p53 (mutp53) triple-negative breast cancer cells by inducing autophagy. Evidence suggests a positive feedback loop between mutp53 and autophagy in these cells, further contributing to the anti-tumor effects (41). However, a phase I clinical trial evaluating the efficacy of Dactolisib and everolimus combination therapy in patients with advanced or metastatic solid tumors (NCT01508104) showed limited tolerability to the combination therapy, preventing dose escalation to potentially effective levels (42). Therefore, further exploration is needed to achieve high effectiveness.
Obatoclax is a BH3 mimetic and Beclin1 contains a BH3 structural domain, thus Obatoclax is able to bind competitively to Bcl-2 and act as an inhibitor of Bcl-2 family proteins (43). Sulkshane et al.’s study found that Obatoclax induces autophagy through the mechanism mentioned above, leading to autophagy-dependent necrotic apoptosis in human oral cancer cells. The downregulation of ATG5 significantly inhibited autophagy and blocked Obatoclax-induced cell death (44). Another study by McCoy et al. demonstrated that Obatoclax independently induces ATG7-dependent autophagy in small cell lung cancer (45). On the other hand, Obatoclax can also affect lysosomal acidification, blocking the fusion of lysosomes with autophagosomes and exerting an inhibitory effect on autophagy (46). In a study focused on thyroid cancer cells, Obatoclax directly caused necrosis, and its cytotoxicity depended on its accumulation in lysosomes rather than its interaction with Bcl-2 family members (47). Additionally, there is research exploring Obatoclax as an autophagy inhibitor to assist in cancer treatment dominated by nutrient deprivation, providing potential help in cutting off existing energy sources during starvation therapy (48).
Autophagy plays a significant role in the pathogenesis of diseases. Inhibition of autophagy is anticipated to restore normal autophagic degradation and mediate the therapeutic effect or to increase the sensitivity of tumor cells to radiotherapy, when autophagy contributes mechanistically to the etiology of a disease or when autophagy can increase the resistance of tumor cells to radiotherapy at a specific stage.
3-methyladenine (3-MA) inhibits autophagy by blocking the formation of autophagosomes through the inhibition of type III PI3K, also known as VPS34. Research by Dong et al. demonstrated that autophagy may serve as a self-defense mechanism in colon cancer HCT116 cells during hypoxic treatment, and the combination of 3-MA with hypoxic treatment significantly suppressed hypoxia-induced autophagy, increased cell apoptosis, and exerted anticancer effects (49). Enhanced autophagy is associated with cisplatin resistance in cancer cells. Liu et al. utilized methods such as monodansylcadaverine staining and flow cytometry to demonstrate that the inhibition of autophagy by 3-MA augmented apoptosis in A549 cancer cells induced by cisplatin and paclitaxel (50). Another study found that, apart from inhibiting Beclin-1-mediated autophagy in pharyngeal squamous cell carcinoma, 3-MA also arrested the cell cycle, promoted apoptosis, and reversed cisplatin resistance (51). To date, 3-MA has not entered clinical trials.
Bafilomycin A1 (BafA1), Bafilomycin B1 (BafB1), Bafilomycin C1 (BafC1), and spautin-1 are inhibitors that hinder the fusion of autophagosomes with lysosomes. They are common V-ATPase inhibitors. V-ATPase is a multi-subunit transmembrane complex responsible for the acidification of lysosomes. Although not a direct target of autophagy, targeting V-ATPase can block autophagic flux. Researchers discovered that through fluorescence probes and protein blotting that BafA1, by inhibiting autophagy, increased the sensitivity of osteosarcoma cells to chemotherapy drugs (52).
CQ and HCQ are early and clinically used autophagy inhibitors. Originally developed as an antimalarial drug, it is currently applicable in clinical settings with relatively low cytotoxicity. In addition to inhibiting fusion, they can also prevent lysosomal acidification, thereby inhibiting the degradation of autophagic cargo. Furthermore, it has been shown that Lys 05 (a dimeric form of CQ) exhibits stronger autophagy inhibition than CQ or HCQ and demonstrates significant monotherapy anti-tumor activity without toxicity in mice treated with lower doses of Lys 05 (53). Numerous clinical trials with CQ and HCQ have been conducted for various types of cancer, including solid tumors, melanoma, sarcoma, glioblastoma, and breast cancer, among others (54).
Nanomaterials can also be specifically modified to possess high targeting specificity, delivery efficiency, and low systemic toxicity (55). Autophagy modulators face challenges such as limited solubility, nonspecific biological distribution, and restricted compatibility with the acidic tumor microenvironment (8). Utilizing nanomaterials for delivery presents a promising solution to overcome these issues. Cancer cells often exhibit higher sensitivity to certain nanoparticles than normal cells, and nanoparticles have great potential for passive tumor targeting. The high vascular permeability in tumors and the prolonged retention resulting from lymphatic obstruction also contribute to the specific accumulation of nanomedicines within tumor tissues. This phenomenon is known as the Enhanced Permeability and Retention effect (EPR). Additionally, nanoparticles (NPs) can enhance the solubility and stability of drugs in aqueous environments, extend their circulation time in the bloodstream, accumulate at the sites of tumor lesions, and increase the intracellular accumulation of anticancer compounds (56). This passive targeting effect on tumor tissues leads to increased therapeutic efficacy and reduced side effects. In addition, active targeting of cancer cells can be achieved by specific molecular modification or ligand functionalization of nanomaterials. Figure 4 illustrates the passive and active targeting of nanoparticles to tumor cells.
Moreover, beyond serving as drug carriers, nanoparticles (NPs) also act as foreign biomolecules within the body, falling within the size range of viruses and some small bacteria. Consequently, they possess inherent autophagy regulatory effects. Table 1 provides a compilation of the diverse applications of different nanomaterials in cancer therapy through their autophagy regulatory effects. Figure 5 illustrates the main principles of tumor cell inhibition through autophagy suppression or autophagy induction following the entry of nanocomposites into cells.
Figure 5 The main principles of the cancer inhibition of nanocomposites. (A) Nanocomposites induce apoptosis in cancer cells through mechanisms such as DNA damage, and overcome drug resistance by suppressing autophagy. (B) Some nanocomposites induce autophagy while concurrently inhibiting autolysosome formation, leading to autophagosome accumulation and ultimately resulting in cell death.
Liposomes, composed of one or multiple layers of phospholipid molecules, are one of the earliest drug nanocarriers used in cancer therapy (78). Liposomes can be modified on their surfaces to serve as environment-sensitive delivery systems or actively target specific cells through ligand conjugation (79). Due to the phospholipid bilayer’s similarity to biological membranes, drugs encapsulated in liposomes readily interact with cells. This imparts excellent biocompatibility and biodegradability to liposomes, effectively improving drug solubility and enhancing drug targeting.
In cases where cancer is closely associated with autophagy, nanomedicines loaded solely with autophagy modulators can achieve significant therapeutic efficacy. Currently, liposome-based nanocarriers approved by the United States Food and Drug Administration (FDA) are being used for cancer treatment (80). Research has demonstrated that liposomes loaded with rapamycin, prepared using a film hydration method and pre-loading technique, can achieve better mTOR inhibition to promote autophagy. This approach significantly suppresses bladder cancer tumor cell growth while reducing the drug’s side effects on other cells (81). In this study, folate-modified liposomes, due to folate receptor-mediated endocytosis, were shown to be taken up by cells more than twice as effectively, providing an important avenue for improving liposome targeting. As mentioned earlier, rapamycin generally exhibits limited efficacy in the treatment of drug-resistant cancers.
Inhibiting autophagy can effectively improve the treatment outcomes of drug-resistant cancers, as cancer cell resistance is frequently associated with autophagy. The mechanisms underlying drug resistance can be multifaceted, as cancer cells often enhance autophagy to reduce drug accumulation within cells, thereby mitigating the toxic effects (82). Moreover, in pancreatic ductal adenocarcinoma, autophagy promotes collagen production, leading to increased stromal density, which severely hampers the effectiveness of anticancer drugs (58). Chen et al. loaded hydroxychloroquine onto liposomes, which demonstrated excellent targeting and penetration effects in vitro and in vivo. This approach effectively inhibited autophagy in pancreatic cancer cells and adjacent cancer-associated fibroblasts, enhancing paclitaxel’s cytotoxicity against pancreatic cancer cells (58). Given the overexpression of integrin αvβ3 receptors on the surface of cancer cells and the acidic microenvironment in their vicinity, the design of integrin αvβ3-targeted and pH-sensitive liposomes in this study enhances the specificity of nanomedicines, offering intriguing prospects in the field of nanodrug design.
Inhibition of autophagy can potentially aid in the treatment of drug-resistant cancers, while excessive autophagy induction can also trigger autophagic cell death. Kang and colleagues developed a tumor-targeted co-delivery system by encapsulating dihydroartemisinin and DOX in mannosylated liposomes. This approach increased the accumulation of therapeutic agents in the nucleus, enhanced apoptosis in cancer cells, and exerted an inhibitory effect on drug-resistant colon cancer cells (83). Researchers developed the nano-drug 188Re-liposome, which can inhibit autophagy and mitophagy in ovarian cancer, thereby reversing drug resistance and resulting in significant tumor suppression (57). Even at the first-level dose in Phase I clinical trials, it showed promising results, suggesting a potential new strategy to overcome drug resistance in ovarian cancer. Another study loaded dihydroartemisinin and DOX onto liposomes and found that these nanoparticles, in addition to taking advantage of the EPR effect, downregulated the activity of Bcl-2 and accelerated its dissociation from BECN1, leading to excessive autophagy induced by BECN1 and type II programmed cell death in breast cancer cells. This approach enhanced the therapeutic efficacy against breast cancer (84). This research highlights that liposomes, while enhancing their targeting capabilities and extending drug circulation, also exhibit autophagy induction on their own.
Therefore, by properly modulating autophagy, it is possible to alter the response of drug-resistant cells to anticancer drugs, increase their sensitivity to drugs, and ultimately achieve better treatment outcomes. Furthermore, pH-sensitive liposomes modified with hydroxychloroquine can inhibit lysosomal acidification and prevent autophagosome degradation. Phase II clinical trials with these liposomes have also been completed (NCT00726596, NCT04841148).
Micelles are tiny spherical particles composed of one or multiple layers of surfactant molecules, typically consisting of a hydrophilic shell and a hydrophobic core. The hydrophobic core can load poorly water-soluble hydrophobic drugs, while the hydrophilic shell can prolong the drug’s circulation time in the body (85). Nanomicelles exhibit high optical transparency, excellent stability, small particle size, and a narrow size distribution, along with good biocompatibility and low toxicity (86). Micelles can also be surface-modified and offer ample structural adjustment space (73). The drug release characteristics of micelles depend on the nature and structure of the surfactants, typically involving drug release through surface diffusion or micelle disruption (87).
Nanomicelles can also be used to deliver rapamycin and directly inhibit cancer growth through autophagy regulation. Rapamycin is a commonly used autophagy inducer and has been developed as a potential anticancer drug for rapamycin-sensitive cancer models. However, its poor water solubility greatly hinders its application in cancer treatment. Chen et al. used dual-responsive mixed micelles to provide an appropriate delivery system for poorly water-soluble drugs like rapamycin in gastrointestinal oral administration for cancer treatment. They found that rapamycin dual-responsive micelles exhibited excellent antitumor activity and a high apoptosis rate in HCT116 cancer cells (88). Another study used self-assembled amphiphilic zein-ferritin micelles for the co-delivery of rapamycin and wogonin to target breast cancer tumors. These micelles demonstrated relatively rapid wogonin release and slow rapamycin release, facilitating the inhibition of efflux pumps by wogonin. This made cancer cells more sensitive to rapamycin, allowing for reduced drug dosages while minimizing side effects and maximizing antitumor efficacy against breast cancer (73). Another study co-delivered rapamycin with the anticancer drug 9-nitro-20(S)-camptothecin (9-NC). Rapamycin induced autophagy in tumor cells and enhanced their sensitivity, while surface-modified micelles directly targeted delivery to the cell nucleus, resulting in significant anticancer effects (89).
Similar to nanoliposomes, nanomicelles themselves can possess autophagy-inducing capabilities. While serving as drug carriers, they may induce intracellular autophagy, potentially affecting the action of loaded drugs (90). Therefore, autophagy inhibitors are often co-delivered with these cancer treatment drugs to assist in the therapeutic process (91). Zhang et al. prepared polymer micelles using a polymer membrane dialysis method and found that these micelles indeed induced intracellular autophagy, aggravating drug resistance. However, PEG-PLGA micelles loaded with docetaxel and chloroquine could effectively suppress autophagy in MCF-7 cells, enhancing their antitumor activity (90). Another study used cholesterol-penetratin self-assembled micelles as a drug delivery system, discovering that cationic cholesterol-penetratin micelles loaded with miR-124 could effectively induce autophagy, leading to the degradation of miR-124 in the corresponding autophagic lysosomes (92). However, when BH3 mimetic obatoclax (as mentioned earlier) was encapsulated, it inhibited the degradation of miR-124 and p62 in autophagic lysosomes, thereby maintaining the concentration of miR-124 in breast cancer cells. This enhanced the cytotoxicity of miR-124 against breast cancer cells. Cholesterol-penetratin is a classical cell-penetrating peptide that can aid in drug transmembrane delivery, providing important insights for subsequent synthesis and modification of nanocarrier micelles.
Another study adopted a similar strategy but made improvements to the micelle material. Rao et al. prepared nanomicelles for co-delivery of the chemotherapeutic drug DOX and the autophagy inhibitor wortmannin using a Cu(I)-catalyzed click chemistry-triggered azide/alkyne aggregation system. These nanomicelles exhibited excellent antitumor activity against melanoma and breast cancer (87). The size of these micelles increased in a time-dependent manner, offering better penetration and accumulation than fixed-sized nanoparticles (NPs), which enhanced their therapeutic efficacy against cancer cells. In another study, the nanomicelles did not have a fixed particle size. This research designed an in-situ self-assembling nanomicelle-dissolving microneedle (DMN) patch, co-encapsulating the immunogenic cell death inducer IR780 and CQ-loaded micelles (C/I-Mil). Photo-thermally mediated size-adjustable C/I-Mil effectively penetrated deep into tumor tissues, achieving highly targeted delivery to the deep tumor tissue through endocytosis and autophagy inhibition. This approach effectively reshaped the tumor’s immunosuppressive microenvironment, ultimately eliminating primary and distant tumors (93).
Apart from preventing the development of drug resistance, minimizing side effects is also a crucial consideration in the selection and preparation of nanomaterials. González-Pastor et al. encapsulated cisplatin and CQ using Pluronic F127 hybrid dendritic-linear-dendritic block copolymer micelles. Their research found that micelles loaded with cisplatin and chloroquine increased cytotoxicity in tumor cells while maintaining a lower level of cytotoxicity in non-tumor cells (94).
Currently, there are not many clinical trials involving micelles. The main focus has been on clinical trials involving cisplatin and docetaxel, where researchers have been studying the pharmacokinetics, safety, and tumor efficacy (NCT05478785, NCT05254665, NCT01023347). Once these clinical trials yield positive results, there may be future clinical trials involving micelles loaded with autophagy inhibitors to combat multidrug resistance.
Polymer nanoparticles (PNPs) are nanocarriers composed of high-molecular-weight compounds. They possess good stability, highly controlled drug release, desirable pharmacokinetics, and potential for biomedical imaging and photodynamic therapy (95). Some polymer nanoparticles can achieve controlled release delivery by changing their structure or responding to external stimuli, such as drug release triggered by ultrasound, which are known as stimulus-responsive nanoparticles (96). Currently, most nanopolymer research and use focus on biodegradable polymers such as polylactic acid (PLA), poly(lactic-co-glycolic acid) (PLGA), polyamino acids, and alginate, which offer good biocompatibility (97).
Nanopolymer carriers are often used to deliver autophagy inhibitors to counteract chemotherapy resistance induced by autophagy-promoting tumorigenesis. Zhang et al. found that PLGA nanoparticles could trigger autophagy in tumor cells, potentially affecting the efficacy of docetaxel treatment. However, incorporating autophagy inhibitors such as 3-MA and CQ within the nanoparticles significantly improved the therapeutic efficacy of the nanoparticle formulation (98). In another experiment, DOX and the autophagy inhibitor LY294002 were encapsulated in pH-sensitive hydrophilic polymer nanoparticles prepared from hyperbranched polyacylhydrazone (HPAH). Upon penetration into oral cancer cells, drug release was responsive to acidic pH values, and both worked together to inhibit cancer progression (99). In these nanoparticles, the hydrophobic DOX was linked to the hydrophilic HPAH, forming micelles and loading the autophagy inhibitor. This ensured the preferential release of LY294002, inhibiting autophagy in tumor cells, making them more sensitive to the subsequent release of DOX. This design of amphiphilic micelles exhibits good targeting and therapeutic effects, and the sequence of release has a significant impact on subsequent drug loading.
PNPs can also be induced through autophagy, showing enhanced cytotoxicity, especially in autophagy-sensitive tumor cells. Wang et al. prepared PNPs loaded with BECN1. The complex showed enhanced cytotoxicity to breast cancer cells by inducing autophagy, and the PNPs themselves also had a certain autophagy-inducing effect (100). Autophagy induction can also be used for effective targeted photothermal therapy. Another study used BECN1-derived peptides for tumor targeting and autophagy-promoting photothermal therapy, and found that it upregulated autophagy in cancer cells and further sensitized tumors to photothermal ablation (101). Especially at high concentrations, polymer nanoparticles are more likely to induce autophagic cell death (102).
In the context of polymer nanoparticles, there are currently relatively few clinical trials related to autophagy regulation in cancer treatment. However, there is an upcoming preclinical trial (NCT05456022) that will explore the close relationship between quercetin and its encapsulated nanoparticles in the treatment of oral squamous cell carcinoma, with a focus on cellular autophagy.
Metal-based nanoparticles are nanoscale particles composed of metal elements or metal compounds, which exhibit unique physical, chemical, and biological properties, showing significant cytotoxicity in a time-dependent and dose-dependent manner in cancer cells (103). Common examples include metal oxide nanoparticles, metal-organic frameworks (MOFs), and gold nanoparticles. Metal-based nanoparticles can regulate autophagy through mechanisms such as oxidative stress, Akt/AMPK/mTOR pathway dysregulation, mitochondrial damage, and ER stress (104). Compared with autophagy inducers, there are few inhibitory nanoparticles like gold nanospikes.
Gold nanoparticles offer a high degree of tunability in terms of size and shape, and their surfaces can be easily functionalized with chemotherapy drugs, antibodies, or nucleic acids, while maintaining good biocompatibility and biocompatibility, making them excellent inducers of autophagy for cancer treatment. Surface modifications can be performed using polyethylene glycol (PEG) or specific antibodies to enhance the targeting of gold nanoparticles to tumors, reduce toxicity to other tissues, and significantly increase the cellular uptake of nanodrugs (70). Furthermore, gold nanoparticles as carriers can mitigate the progression and vitality of breast cancer by providing photothermal therapy while also exhibiting bioimaging capabilities (105). Gold nanoparticles induce excessive ROS production, subsequently triggering apoptosis and autophagy, thereby exerting a killing effect on cancer cells (106). A study shows that the mechanism of coated gold nanobipyramids inhibiting autophagy in glioblastoma cells is mainly achieved by inhibiting lysosomal proteins and thereby blocking the autophagosome-lysosome fusion process (60). Another study showed that gold nanospike can inhibit the progression of oral epidermal cancer and cervical cancer through the increase of ROS, mitochondrial depolarization and cell cycle redistribution (62). Currently, there are preclinical and pilot human clinical studies involving gold nanoparticles in the treatment of breast cancer (107). The role of autophagy in these studies is still under investigation and requires further research to better understand its involvement and potential impact on the treatment outcomes.
Metal oxide nanoparticles are another commonly used nanomaterial, encompassing various types such as zinc oxide, iron oxide, copper oxide, and cerium oxide. Some nanoscale oxide materials exhibit unique effects in cancer treatment due to their specific physical properties. Superparamagnetic iron oxide nanoparticles (SPIONs) possess magnetic properties and can serve as drug carriers to address issues related to hydrophobic anticancer drugs. They exhibit excellent chemosens ity and, simultaneously, enable bioimaging. Moreover, they effectively induce autophagy, thus enhancing the sensitization of chemotherapy (76). On the other hand, porous cerium dioxide nanorods possess unique physical properties that catalytically scavenge ROS, inhibit autophagy, and activate the intrinsic antioxidant pathway of tumor cells, thereby suppressing squamous cell carcinoma of the skin (65).
Metal-organic frameworks (MOFs) are crystalline materials formed by orderly assembling organic linkers between metal nodes. These MOF materials exhibit distinctive characteristics, including a large surface area, high porosity, and tunable chemistry (108). Zeolitic imidazolate frameworks (ZIF-8), a prominent subclass of MOF nanoparticles, possess high loading capacity and pH-sensitive degradation capability. A study by chen et al. encapsulated 3-MA, a chemotherapy agent, within ZIF-8 nanoparticles and observed significantly inhibited autophagosome formation compared to free 3-MA, resulting in higher anticancer efficacy (109). Another study encapsulated CQ within ZIF-8 and further modified it with methoxy polyethylene glycol-folic acid (FA-PEG) for active targeting, which effectively disrupted autophagosome formation and autophagic flux, exerting therapeutic effects (110).
Overall, metal-based nanoparticles hold great promise for cancer treatment. Their unique physical and chemical properties, combined with tunable size, shape, and surface modifications, enable them to serve as drug carriers, inducers or inhibitors of autophagy, and therapeutic agents in various cancer types. Further research and development in this field will continue to expand their potential in precision medicine and targeted cancer therapy.
Carbon exists in various allotropes, and its nanomaterials exhibit diverse forms, ranging from one-dimensional to three-dimensional structures, such as carbon nanotubes and carbon dots (CDs), graphene, and diamond.
CDs, belonging to quantum dots, have sizes typically smaller than 10 nm and possess excellent biocompatibility, safety, and fluorescent activity. CDs can be utilized in cancer treatment through photothermal therapy and induction of mitochondrial stress (111, 112). Due to their small size, quantum dots often induce autophagy, which is one of the pathways for inhibiting cancer progression. Bajpai et al. found that nitrogen-phosphorus-doped carbon dots can increase the expression of ATG5 and LC3-II in B16F10 melanoma cells, reduce the expression of p62, thereby inducing autophagy and apoptosis in melanoma cells (113).
Graphene, with its honeycomb structure, possesses high binding affinity and can be functionalized with various polymers to enhance active targeting of cancer cells. Graphene nanostructures can lead to autophagy and osteosarcoma inhibition by regulating various molecular pathways of autophagy such as upregulating ATG5, ATG7, and LC3-II (114). Like CD, graphene nanoparticles also have photothermal properties, which inhibit autophagy and conduct thermal stress, promoting apoptosis (115). When graphene nanoparticles are loaded with autophagy inhibitors, they can also exert an autophagy inhibitory effect by blocking autophagy flux and inducing the accumulation of autophagosomes in cells (116). Graphene oxide can also induce autophagic death of HCT116 cells by activating the ROS-dependent AMPK/mTOR/ULK1 pathway, thereby exerting obvious anti-colorectal cancer effects both in vivo and in vitro (72). And it can also synergize with cisplatin to significantly enhance the initiation and progression of autophagy in Skov-3 cells, enhance the toxicity of cisplatin to colon cancer cells, and mediate cell necrosis (117). Hybrid nanocomposites of graphene and silver nanoparticles can also have a synergistic effect with cisplatin on cancer inhibition, increasing the production of ROS in cervical tumors, thereby stimulating apoptosis and autophagy (117).
Diamond is another allotrope of carbon. High levels of autophagy under hypoxic conditions are an adaptive strategy for cancer cell survival, and nanodiamonds have been shown to synergistically inhibit autophagy under hypoxic conditions in tumors, thereby improving the arsenical-based therapy of solid tumors (61). This complex, Nano-DOX, stimulates the immunogenicity of glioblastoma cells by inducing autophagy and initiates anti-glioblastoma immune responses, which can effectively regulate its immunosuppressive microenvironment (77).
In this review, we summarized different types of nanocarriers that can be used to deliver autophagy regulators, including liposomes, micelles, polymers, carbon-based nanocarriers and metal-based nanocarriers, some of which have autophagy regulatory effects themselves. We also discussed various autophagy regulators loaded onto these nanocarriers and their targeting mechanisms.
Researchers have employed various strategies to enhance the efficacy of nanomaterials, including surface modification, pH sensitivity, adjustable size, and encapsulation of hydrophobic substances. These improvements have increased the materials’ targeting capabilities while reducing side effects and drug dosages. The underlying principles of using nanomaterials in cancer therapy through autophagy regulation encompass inducing autophagy in sensitive cells to inhibit tumor growth, suppressing autophagy to enhance the effectiveness of chemotherapy drugs, immunogenic cell death inducers, photothermal therapy, and inducing ROS to promote excessive autophagy and subsequent apoptosis in cancer cells.
The development of targeted anti-cancer nanomedicines with autophagy regulation has made significant progress, showing great potential in cancer treatment. Current research has gradually deepened and some drugs have entered phase I or II clinical trials. Despite decades of tremendous effort, the results achieved in technology/knowledge transfer have been limited so far. To date, the FDA has only approved five nanomedicines for the treatment of solid tumors.
Despite significant progress in this field, there are still some challenges to be addressed. One major challenge is to optimize the characteristics of nanocarriers such as size, surface charge and targeting ligands to improve their tumor targeting and cellular uptake efficiency. Another challenge is the lack of standardization and stability in the preparation and production processes of nanomaterials, and the heterogeneity of patient conditions and etiologies can also impact experimental results. Additionally, some nanomaterials may pose risks to human health and require comprehensive assessment and monitoring in clinical applications. Furthermore, the therapeutic effectiveness of certain nanomaterials may be incomplete, failing to meet clinical requirements.
Overall, different anti-cancer drugs have different physicochemical, pharmacokinetic and pharmacodynamic properties that determine their unique clinical efficacy and safety characteristics in human cancer patients. The development of targeted anti-cancer nanomedicines with autophagy regulation is a promising approach to cancer treatment. Additionally, different nano-delivery platforms should be developed for various drugs with a focus on tumor specificity due to the tissue specificity of many tumors. To enable nanomedicines with autophagy to enter clinical practice as soon as possible, future research directions may include reducing drug toxicity, stabilizing manufacturing methods, improving tumor inhibition efficiency, and lowering production costs. As nanocarrier properties continue to be optimized and more effective autophagy modulators are developed, these nanomedicines may provide more effective and personalized cancer treatment options.
JZ conceived and designed this manuscript. CR participated in the writing of some sections of the paper and provided suggestions for revisions after the completion of the paper. SD assisted in the modifications following the paper's review, made changes to figures, and conducted the final review. KH created the figures and wrote the majority of the paper. JL and MC summarized the literature. All authors contributed to this article and approved the final version.
The authors thank the Natural Science Foundation of Sichuan (No. 2023NSFSC1695), Health Commission of Sichuan Province (No. 21PJ149) and Xinglin Foundation of Chengdu University of TCM (No. YYZX2021043).
The authors declare that the research was conducted in the absence of any commercial or financial relationships that could be construed as a potential conflict of interest.
All claims expressed in this article are solely those of the authors and do not necessarily represent those of their affiliated organizations, or those of the publisher, the editors and the reviewers. Any product that may be evaluated in this article, or claim that may be made by its manufacturer, is not guaranteed or endorsed by the publisher.
1. Siafaka PI, Üstündağ Okur N, Karavas E, Bikiaris DN. Surface modified multifunctional and stimuli responsive nanoparticles for drug targeting: current status and uses. Int J Mol Sci (2016) 17:1440. doi: 10.3390/ijms17091440
2. Fang RH, Gao W, Zhang L. Targeting drugs to tumours using cell membrane-coated nanoparticles. Nat Rev Clin Oncol (2023) 20:33–48. doi: 10.1038/s41571-022-00699-x
3. Kemp JA, Kwon YJ. Cancer nanotechnology: current status and perspectives. Nano Converg (2021) 8:34. doi: 10.1186/s40580-021-00282-7
4. Cheng Z, Li M, Dey R, Chen Y. Nanomaterials for cancer therapy: current progress and perspectives. J Hematol Oncol (2021) 14:85. doi: 10.1186/s13045-021-01096-0
5. Klionsky DJ, Petroni G, Amaravadi RK, Baehrecke EH, Ballabio A, Boya P, et al. Autophagy in major human diseases. EMBO J (2021) 40. doi: 10.15252/embj.2021108863
6. Cordani M, Somoza Á. Targeting autophagy using metallic nanoparticles: a promising strategy for cancer treatment. Cell Mol Life Sci (2019) 76:1215–42doi: 10.1007/s00018-018-2973-y
7. Mukhopadhyay S, Mahapatra KK, Praharaj PP, Patil S, Bhutia SK. Recent progress of autophagy signaling in tumor microenvironment and its targeting for possible cancer therapeutics. Semin Cancer Biol (2022) 85:196–208. doi: 10.1016/j.semcancer.2021.09.003
8. Pellegrini P, Strambi A, Zipoli C, Hägg-Olofsson M, Buoncervello M, Linder S, et al. Acidic extracellular pH neutralizes the autophagy-inhibiting activity of chloroquine: implications for cancer therapies. Autophagy (2014) 10:562–71. doi: 10.4161/auto.27901
9. Qi S, Kim DJ, Stjepanovic G, Hurley JH. Structure of the human atg13-atg101 HORMA heterodimer: an interaction hub within the ULK1 complex. Structure (2015) 23:1848–57. doi: 10.1016/j.str.2015.07.011
10. Hosokawa N, Hara T, Kaizuka T, Kishi C, Takamura A, Miura Y, et al. Nutrient-dependent mTORC1 association with the ULK1-Atg13-FIP200 complex required for autophagy. Mol Biol Cell (2009) 20:1981–91. doi: 10.1091/mbc.e08-12-1248
11. Shaw RJ. LKB1 and AMP-activated protein kinase control of mTOR signalling and growth. Acta Physiol (Oxf) (2009) 196:65–80. doi: 10.1111/j.1748-1716.2009.01972.x
12. Li X, He S, Ma B. Autophagy and autophagy-related proteins in cancer. Mol Cancer (2020) 19:12. doi: 10.1186/s12943-020-1138-4
13. Amaravadi RK, Kimmelman AC, Debnath J. Targeting autophagy in cancer: recent advances and future directions. Cancer Discovery (2019) 9:1167–81. doi: 10.1158/2159-8290.CD-19-0292
14. Hamasaki M, Furuta N, Matsuda A, Nezu A, Yamamoto A, Fujita N, et al. Autophagosomes form at ER-mitochondria contact sites. Nature (2013) 495:389–93. doi: 10.1038/nature11910
15. Yazdankhah M, Farioli-Vecchioli S, Tonchev AB, Stoykova A, Cecconi F. The autophagy regulators Ambra1 and Beclin 1 are required for adult neurogenesis in the brain subventricular zone. Cell Death Dis (2014) 5:e1403. doi: 10.1038/cddis.2014.358
16. Walczak M, Martens S. Dissecting the role of the Atg12-Atg5-Atg16 complex during autophagosome formation. Autophagy (2013) 9:424–5. doi: 10.4161/auto.22931
17. Liang C, Feng P, Ku B, Dotan I, Canaani D, Oh B-H, et al. Autophagic and tumour suppressor activity of a novel Beclin1-binding protein UVRAG. Nat Cell Biol (2006) 8:688–99. doi: 10.1038/ncb1426
18. Zhou J, Li X-Y, Liu Y-J, Feng J, Wu Y, Shen H-M, et al. Full-coverage regulations of autophagy by ROS: from induction to maturation. Autophagy (2022) 18:1240–55. doi: 10.1080/15548627.2021.1984656
19. Wang S, Deng Z, Ma Y, Jin J, Qi F, Li S, et al. The role of autophagy and mitophagy in bone metabolic disorders. Int J Biol Sci (2020) 16:2675–91. doi: 10.7150/ijbs.46627
20. Zhao H, Shi L, Kong R, Li Z, Liu F, Zhao H, et al. Autophagy induction in tumor surrounding cells promotes tumor growth in adult Drosophila intestines. Dev Biol (2021) 476:294–307. doi: 10.1016/j.ydbio.2021.04.008
21. Yamamoto K, Venida A, Yano J, Biancur DE, Kakiuchi M, Gupta S, et al. Autophagy promotes immune evasion of pancreatic cancer by degrading MHC-I. Nature (2020) 581:100–5. doi: 10.1038/s41586-020-2229-5
22. Tong H, Yin H, Hossain MA, Wang Y, Wu F, Dong X, et al. Starvation-induced autophagy promotes the invasion and migration of human bladder cancer cells via TGF-β1/Smad3-mediated epithelial-mesenchymal transition activation. J Cell Biochem (2019) 120:5118–27. doi: 10.1002/jcb.27788
23. Galluzzi L, Bravo-San Pedro JM, Levine B, Green DR, Kroemer G. Pharmacological modulation of autophagy: therapeutic potential and persisting obstacles. Nat Rev Drug Discovery (2017) 16:487–511. doi: 10.1038/nrd.2017.22
24. Wang Y, Xu W, Yan Z, Zhao W, Mi J, Li J, et al. Metformin induces autophagy and G0/G1 phase cell cycle arrest in myeloma by targeting the AMPK/mTORC1 and mTORC2 pathways. J Exp Clin Cancer Res (2018) 37:63. doi: 10.1186/s13046-018-0731-5
25. Lai H-Y, Tsai H-H, Yen C-J, Hung L-Y, Yang C-C, Ho C-H, et al. Metformin resensitizes sorafenib-resistant HCC cells through AMPK-dependent autophagy activation. Front Cell Dev Biol (2020) 8:596655. doi: 10.3389/fcell.2020.596655
26. Oechsle CM, Showalter LE, Novak CM, Czerniecki BJ, Koski GK. Statin drugs plus th1 cytokines potentiate apoptosis and ras delocalization in human breast cancer lines and combine with dendritic cell-based immunotherapy to suppress tumor growth in a mouse model of HER-2pos disease. Vaccines (Basel) (2020) 8:72. doi: 10.3390/vaccines8010072
27. Mangelinck A, Habel N, Mohr A, Gaspar N, Stefanovska B, Fromigué O. Synergistic anti-tumor effect of simvastatin combined to chemotherapy in osteosarcoma. Cancers (Basel) (2021) 13:5869. doi: 10.3390/cancers13225869
28. Zhou C, Zhong X, Gao P, Wu Z, Shi J, Guo Z, et al. Statin use and its potential therapeutic role in esophageal cancer: a systematic review and meta-analysis. Cancer Manag Res (2019) 11:5655–63. doi: 10.2147/CMAR.S193945
29. Wei Y-M, Li X, Xu M, Abais JM, Chen Y, Riebling CR, et al. Enhancement of autophagy by simvastatin through inhibition of Rac1-mTOR signaling pathway in coronary arterial myocytes. Cell Physiol Biochem (2013) 31:925–37. doi: 10.1159/000350111
30. Kamel WA, Sugihara E, Nobusue H, Yamaguchi-Iwai S, Onishi N, Maki K, et al. Simvastatin-induced apoptosis in osteosarcoma cells: A key role of rhoA-AMPK/p38 MAPK signaling in antitumor activity. Mol Cancer Ther (2017) 16:182–92. doi: 10.1158/1535-7163.MCT-16-0499
31. Yin Y, Liu L, Zhao Z, Yin L, Bauer N, Nwaeburu CC, et al. Simvastatin inhibits sonic hedgehog signaling and stemness features of pancreatic cancer. Cancer Lett (2018) 426:14–24. doi: 10.1016/j.canlet.2018.04.001
32. Tu X, Zhang J, Yuan W, Wu X, Xu Z, Qing C. Simvastatin Enhanced Anti-tumor Effects of Bevacizumab against Lung Adenocarcinoma A549 Cells via Abating HIF-1α-Wnt/β-Catenin Signaling Pathway. Anticancer Agents Med Chem (2023) 23:2083–94. doi: 10.2174/1871520623666230816090914
33. Shams R, Matsukawa A, Ochi Y, Ito Y, Miyatake H. In silico and in cell hybrid selection of nonrapalog ligands to allosterically inhibit the kinase activity of mTORC1. J Med Chem (2022) 65:1329–41. doi: 10.1021/acs.jmedchem.1c00536
34. Lupinacci S, Perri A, Toteda G, Vizza D, Lofaro D, Pontrelli P, et al. Rapamycin promotes autophagy cell death of Kaposi’s sarcoma cells through P75NTR activation. Exp Dermatol (2022) 31:143–53. doi: 10.1111/exd.14438
35. Masaki N, Aoki Y, Obara K, Kubota Y, Bouvet M, Miyazaki J, et al. Targeting autophagy with the synergistic combination of chloroquine and rapamycin as a novel effective treatment for well-differentiated liposarcoma. Cancer Genomics Proteomics (2023) 20:317–22. doi: 10.21873/cgp.20384
36. Galanis E, Buckner JC, Maurer MJ, Kreisberg JI, Ballman K, Boni J, et al. Phase II trial of temsirolimus (CCI-779) in recurrent glioblastoma multiforme: a North Central Cancer Treatment Group Study. J Clin Oncol (2005) 23:5294–304. doi: 10.1200/JCO.2005.23.622
37. Chen Y, Zhou X. Research progress of mTOR inhibitors. Eur J Med Chem (2020) 208:112820. doi: 10.1016/j.ejmech.2020.112820
38. Helmy MW, Ghoneim AI, Katary MA, Elmahdy RK. The synergistic anti-proliferative effect of the combination of diosmin and BEZ-235 (dactolisib) on the HCT-116 colorectal cancer cell line occurs through inhibition of the PI3K/Akt/mTOR/NF-κB axis. Mol Biol Rep (2020) 47:2217–30. doi: 10.1007/s11033-020-05327-4
39. Cao W, Liu X, Zhang Y, Li A, Xie Y, Zhou S, et al. BEZ235 increases the sensitivity of hepatocellular carcinoma to sorafenib by inhibiting PI3K/AKT/mTOR and inducing autophagy. BioMed Res Int (2021) 2021:5556306. doi: 10.1155/2021/5556306
40. Abdelwahab M, Saeed H, Elnikhely N, Nematalla H. Synergistic effect of Dactolisib/Lys05 combination on autophagy in A549 cells. Acta Biochim Pol (2023) 70:615–22. doi: 10.18388/abp.2020_6694
41. Cai J, Xia J, Zou J, Wang Q, Ma Q, Sun R, et al. The PI3K/mTOR dual inhibitor NVP-BEZ235 stimulates mutant p53 degradation to exert anti-tumor effects on triple-negative breast cancer cells. FEBS Open Bio (2020) 10:535–45. doi: 10.1002/2211-5463.12806
42. Wise-Draper TM, Moorthy G, Salkeni MA, Karim NA, Thomas HE, Mercer CA, et al. A phase ib study of the dual PI3K/mTOR inhibitor dactolisib (BEZ235) combined with everolimus in patients with advanced solid Malignancies. Target Oncol (2017) 12:323–32. doi: 10.1007/s11523-017-0482-9
43. Yu L, Liu S. Autophagy contributes to modulating the cytotoxicities of Bcl-2 homology domain-3 mimetics. Semin Cancer Biol (2013) 23:553–60. doi: 10.1016/j.semcancer.2013.08.008
44. Sulkshane P, Teni T. BH3 mimetic Obatoclax (GX15-070) mediates mitochondrial stress predominantly via MCL-1 inhibition and induces autophagy-dependent necroptosis in human oral cancer cells. Oncotarget (2017) 8:60060–79. doi: 10.18632/oncotarget.11085
45. McCoy F, Hurwitz J, McTavish N, Paul I, Barnes C, O’Hagan B, et al. Obatoclax induces Atg7-dependent autophagy independent of beclin-1 and BAX/BAK. Cell Death Dis (2010) 1:e108. doi: 10.1038/cddis.2010.86
46. Yu L, Wu WKK, Gu C, Zhong D, Zhao X, Kong Y, et al. Obatoclax impairs lysosomal function to block autophagy in cisplatin-sensitive and -resistant esophageal cancer cells. Oncotarget (2016) 7:14693–707. doi: 10.18632/oncotarget.7492
47. Champa D, Orlacchio A, Patel B, Ranieri M, Shemetov AA, Verkhusha VV, et al. Obatoclax kills anaplastic thyroid cancer cells by inducing lysosome neutralization and necrosis. Oncotarget (2016) 7:34453–71. doi: 10.18632/oncotarget.9121
48. Wang X, Li Y, Jia F, Cui X, Pan Z, Wu Y. Boosting nutrient starvation-dominated cancer therapy through curcumin-augmented mitochondrial Ca2+ overload and obatoclax-mediated autophagy inhibition as supported by a novel nano-modulator GO-Alg@CaP/CO. J Nanobiotechnology (2022) 20:225. doi: 10.1186/s12951-022-01439-0
49. Dong Y, Wu Y, Zhao G-L, Ye Z-Y, Xing C-G, Yang X-D. Inhibition of autophagy by 3-MA promotes hypoxia-induced apoptosis in human colorectal cancer cells. Eur Rev Med Pharmacol Sci (2019) 23:1047–54. doi: 10.26355/eurrev_201902_16992
50. Liu F, Liu D, Yang Y, Zhao S. Effect of autophagy inhibition on chemotherapy-induced apoptosis in A549 lung cancer cells. Oncol Lett (2013) 5:1261–5. doi: 10.3892/ol.2013.1154
51. Zhang J, Mao W, Liu Y, Ding J, Wang J, Yu Z, et al. 3-MA enhanced chemosensitivity in cisplatin resistant hypopharyngeal squamous carcinoma cells via inhibiting beclin -1 mediated autophagy. Curr Pharm Des (2021) 27:996–1005. doi: 10.2174/1381612826666201221150431
52. Xie Z, Xie Y, Xu Y, Zhou H, Xu W, Dong Q. Bafilomycin A1 inhibits autophagy and induces apoptosis in MG63 osteosarcoma cells. Mol Med Rep (2014) 10:1103–7. doi: 10.3892/mmr.2014.2281
53. McAfee Q, Zhang Z, Samanta A, Levi SM, Ma X-H, Piao S, et al. Autophagy inhibitor Lys05 has single-agent antitumor activity and reproduces the phenotype of a genetic autophagy deficiency. Proc Natl Acad Sci U.S.A. (2012) 109:8253–8. doi: 10.1073/pnas.1118193109
54. Ferreira PMP, de SRWR, Ferreira JR de O, Militão GCG, Bezerra DP. Chloroquine and hydroxychloroquine in antitumor therapies based on autophagy-related mechanisms. Pharmacol Res (2021) 168:105582. doi: 10.1016/j.phrs.2021.105582
55. Bamburowicz-Klimkowska M, Poplawska M, Grudzinski IP. Nanocomposites as biomolecules delivery agents in nanomedicine. J Nanobiotechnology (2019) 17:48. doi: 10.1186/s12951-019-0479-x
56. Rehan F, Ahemad N, Islam RA, Gupta M, Gan SH, Chowdhury EH. Optimization and formulation of nanostructured and self-assembled caseinate micelles for enhanced cytotoxic effects of paclitaxel on breast cancer cells. Pharmaceutics (2020) 12:984. doi: 10.3390/pharmaceutics12100984
57. Chang C-M, Lan K-L, Huang W-S, Lee Y-J, Lee T-W, Chang C-H, et al. 188Re-liposome can induce mitochondrial autophagy and reverse drug resistance for ovarian cancer: from bench evidence to preliminary clinical proof-of-concept. Int J Mol Sci (2017) 18:903. doi: 10.3390/ijms18050903
58. Chen X, Yu Q, Liu Y, Sheng Q, Shi K, Wang Y, et al. Synergistic cytotoxicity and co-autophagy inhibition in pancreatic tumor cells and cancer-associated fibroblasts by dual functional peptide-modified liposomes. Acta Biomater (2019) 99:339–49. doi: 10.1016/j.actbio.2019.09.003
59. Hu C, Gu F, Gong C, Xia Q, Gao Y, Gao S. Co-delivery of the autophagy inhibitor si-Beclin1 and the doxorubicin nano-delivery system for advanced prostate cancer treatment. J Biomater Appl (2022) 36:1317–31. doi: 10.1177/08853282211060252
60. Wan H-Y, Chen J-L, Zhu X, Liu L, Wang J, Zhu X-M. Titania-coated gold nano-bipyramids for blocking autophagy flux and sensitizing cancer cells to proteasome inhibitor-induced death. Adv Sci (Weinh) (2018) 5:1700585. doi: 10.1002/advs.201700585
61. Cui Z, Zhang Y, Xia K, Yan Q, Kong H, Zhang J, et al. Nanodiamond autophagy inhibitor allosterically improves the arsenical-based therapy of solid tumors. Nat Commun (2018) 9:4347. doi: 10.1038/s41467-018-06749-2
62. Ma N, Liu P, He N, Gu N, Wu F-G, Chen Z. Action of gold nanospikes-based nanoradiosensitizers: cellular internalization, radiotherapy, and autophagy. ACS Appl Mater Interfaces (2017) 9:31526–42. doi: 10.1021/acsami.7b09599
63. Liu X, Gao P, Shi M, Chen Y, Pan W, Li N, et al. An autophagy-inhibitory MOF nanoreactor for tumor-targeted synergistic therapy. Biomater Sci (2022) 10:3088–91. doi: 10.1039/d2bm00579d
64. Qian R, Cao G, Su W, Zhang J, Jiang Y, Song H, et al. Enhanced sensitivity of tumor cells to autophagy inhibitors using fasting-mimicking diet and targeted lysosomal delivery nanoplatform. Nano Lett (2022) 22:9154–62. doi: 10.1021/acs.nanolett.2c03890
65. Wang Y, Huang Y, Fu Y, Guo Z, Chen D, Cao F, et al. Reductive damage induced autophagy inhibition for tumor therapy. Nano Res (2022) 16:5226–36. doi: 10.1007/s12274-022-5139-z
66. Liang Q, Yu F, Cai H, Wu X, Ma M, Li Z, et al. Photo-activated autophagy-associated tumour cell death by lysosome impairment based on manganese-doped graphene quantum dots. J Mater Chem B (2023) 11:2466–77. doi: 10.1039/d2tb02761e
67. Nirosha Yalamandala B, Chen P-H, Moorthy T, Huynh TMH, Chiang W-H, Hu S-H. Programmed catalytic therapy-mediated ROS generation and T-cell infiltration in lung metastasis by a dual metal-organic framework (MOF) nanoagent. Pharmaceutics (2022) 14:527. doi: 10.3390/pharmaceutics14030527
68. Li X, Wang Z-G, Zhu H, Wen H-P, Ning D, Liu H-Y, et al. Inducing autophagy and blocking autophagic flux via a virus-mimicking nanodrug for cancer therapy. Nano Lett (2022) 22:9163–73. doi: 10.1021/acs.nanolett.2c04091
69. Yi J, Luo X, Xing J, Gedanken A, Lin X, Zhang C, et al. Micelle encapsulation zinc-doped copper oxide nanocomposites reverse Olaparib resistance in ovarian cancer by disrupting homologous recombination repair. Bioeng Transl Med (2023) 8:e10507. doi: 10.1002/btm2.10507
70. Zhang M, Kim HS, Jin T, Moon WK. Near-infrared photothermal therapy using EGFR-targeted gold nanoparticles increases autophagic cell death in breast cancer. J Photochem Photobiol B (2017) 170:58–64. doi: 10.1016/j.jphotobiol.2017.03.025
71. Yang R, Wu R, Mei J, Hu F-R, Lei C-J. Zinc oxide nanoparticles promotes liver cancer cell apoptosis through inducing autophagy and promoting p53. Eur Rev Med Pharmacol Sci (2021) 25:1557–63. doi: 10.26355/eurrev_202102_24864
72. Shen J, Dong J, Shao F, Zhao J, Gong L, Wang H, et al. Graphene oxide induces autophagy and apoptosis via the ROS-dependent AMPK/mTOR/ULK-1 pathway in colorectal cancer cells. Nanomedicine (Lond) (2022) 17:591–605. doi: 10.2217/nnm-2022-0030
73. Sabra SA, Elzoghby AO, Sheweita SA, Haroun M, Helmy MW, Eldemellawy MA, et al. Self-assembled amphiphilic zein-lactoferrin micelles for tumor targeted co-delivery of rapamycin and wogonin to breast cancer. Eur J Pharm Biopharm (2018) 128:156–69. doi: 10.1016/j.ejpb.2018.04.023
74. Houdaihed L, Evans JC, Allen C. Codelivery of paclitaxel and everolimus at the optimal synergistic ratio: A promising solution for the treatment of breast cancer. Mol Pharm (2018) 15:3672–81. doi: 10.1021/acs.molpharmaceut.8b00217
75. Fan YL, Hou HW, Tay HM, Guo WM, Berggren P-O, Loo SCJ. Preservation of anticancer and immunosuppressive properties of rapamycin achieved through controlled releasing particles. AAPS PharmSciTech (2017) 18:2648–57. doi: 10.1208/s12249-017-0745-x
76. Wang G, Qian K, Mei X. A theranostic nanoplatform: magneto-gold@fluorescence polymer nanoparticles for tumor targeting T1&T2-MRI/CT/NIR fluorescence imaging and induction of genuine autophagy mediated chemotherapy. Nanoscale (2018) 10:10467–78. doi: 10.1039/c8nr02429d
77. Li T-F, Xu Y-H, Li K, Wang C, Liu X, Yue Y, et al. Doxorubicin-polyglycerol-nanodiamond composites stimulate glioblastoma cell immunogenicity through activation of autophagy. Acta Biomater (2019) 86:381–94. doi: 10.1016/j.actbio.2019.01.020
78. Tenchov R, Bird R, Curtze AE, Zhou Q. Lipid nanoparticles─From liposomes to mRNA vaccine delivery, a landscape of research diversity and advancement. ACS Nano (2021) 15:16982–7015. doi: 10.1021/acsnano.1c04996
79. Li M, Du C, Guo N, Teng Y, Meng X, Sun H, et al. Composition design and medical application of liposomes. Eur J Med Chem (2019) 164:640–53. doi: 10.1016/j.ejmech.2019.01.007
80. Qiu M, Sun H, Meng F, Cheng R, Zhang J, Deng C, et al. Lipopepsomes: A novel and robust family of nano-vesicles capable of highly efficient encapsulation and tumor-targeted delivery of doxorubicin hydrochloride. vivo. J Control Release (2018) 272:107–13. doi: 10.1016/j.jconrel.2018.01.011
81. Yoon HY, Chang IH, Goo YT, Kim CH, Kang TH, Kim S-Y, et al. Intravesical delivery of rapamycin via folate-modified liposomes dispersed in thermo-reversible hydrogel. Int J Nanomedicine (2019) 14:6249–68. doi: 10.2147/IJN.S216432
82. Condello M, Mancini G, Meschini S. The exploitation of liposomes in the inhibition of autophagy to defeat drug resistance. Front Pharmacol (2020) 11:787. doi: 10.3389/fphar.2020.00787
83. Kang X-J, Wang H-Y, Peng H-G, Chen B-F, Zhang W-Y, Wu A-H, et al. Codelivery of dihydroartemisinin and doxorubicin in mannosylated liposomes for drug-resistant colon cancer therapy. Acta Pharmacol Sin (2017) 38:885–96. doi: 10.1038/aps.2017.10
84. Hu Y-J, Zhang J-Y, Luo Q, Xu J-R, Yan Y, Mu L-M, et al. Nanostructured dihydroartemisinin plus epirubicin liposomes enhance treatment efficacy of breast cancer by inducing autophagy and apoptosis. Nanomaterials (Basel) (2018) 8:804. doi: 10.3390/nano8100804
85. Qiu L, Xu J, Ahmed KS, Zhu M, Zhang Y, Long M, et al. Stimuli-responsive, dual-function prodrug encapsulated in hyaluronic acid micelles to overcome doxorubicin resistance. Acta Biomater (2022) 140:686–99. doi: 10.1016/j.actbio.2021.11.050
86. Tavakol S, Ashrafizadeh M, Deng S, Azarian M, Abdoli A, Motavaf M, et al. Autophagy modulators: mechanistic aspects and drug delivery systems. Biomolecules (2019) 9:18. doi: 10.3390/biom9100530
87. Rao J, Mei L, Liu J, Tang X, Yin S, Xia C, et al. Size-adjustable micelles co-loaded with a chemotherapeutic agent and an autophagy inhibitor for enhancing cancer treatment via increased tumor retention. Acta Biomater (2019) 89:300–12. doi: 10.1016/j.actbio.2019.03.022
88. Chen Y-C, Lo C-L, Lin Y-F, Hsiue G-H. Rapamycin encapsulated in dual-responsive micelles for cancer therapy. Biomaterials (2013) 34:1115–27. doi: 10.1016/j.biomaterials.2012.10.034
89. Yan J, Shan C, Zhang Z, Li F, Sun Y, Wang Q, et al. Autophagy-induced intracellular signaling fractional nano-drug system for synergistic anti-tumor therapy. J Colloid Interface Sci (2023) 645:986–96. doi: 10.1016/j.jcis.2023.05.031
90. Zhang X, Zeng X, Liang X, Yang Y, Li X, Chen H, et al. The chemotherapeutic potential of PEG-b-PLGA copolymer micelles that combine chloroquine as autophagy inhibitor and docetaxel as an anti-cancer drug. Biomaterials (2014) 35:9144–54. doi: 10.1016/j.biomaterials.2014.07.028
91. Liu Y, Baba Y, Ishimoto T, Tsutsuki H, Zhang T, Nomoto D, et al. Fusobacterium nucleatum confers chemoresistance by modulating autophagy in oesophageal squamous cell carcinoma. Br J Cancer (2021) 124:963–74. doi: 10.1038/s41416-020-01198-5
92. Zhang N, Huang Y, Wu F, Zhao Y, Li X, Shen P, et al. Codelivery of a miR-124 mimic and obatoclax by cholesterol-penetratin micelles simultaneously induces apoptosis and inhibits autophagic flux in breast cancer in vitro and in vivo. Mol Pharm (2016) 13:2466–83. doi: 10.1021/acs.molpharmaceut.6b00211
93. Chen M, Yang D, Sun Y, Liu T, Wang W, Fu J, et al. In situ self-assembly nanomicelle microneedles for enhanced photoimmunotherapy via autophagy regulation strategy. ACS Nano (2021) 15:3387–401. doi: 10.1021/acsnano.0c10396
94. González-Pastor R, Lancelot A, Morcuende-Ventura V, San Anselmo M, Sierra T, Serrano JL, et al. Combination chemotherapy with cisplatin and chloroquine: effect of encapsulation in micelles formed by self-assembling hybrid dendritic-linear-dendritic block copolymers. Int J Mol Sci (2021) 22:5223. doi: 10.3390/ijms22105223
95. Khdary NH, Almuarqab BT, El Enany G. Nanoparticle-embedded polymers and their applications: A review. Membranes (Basel) (2023) 13:537. doi: 10.3390/membranes13050537
96. Hao Y, Chen Y, He X, Yu Y, Han R, Li Y, et al. Polymeric nanoparticles with ROS-responsive prodrug and platinum nanozyme for enhanced chemophotodynamic therapy of colon cancer. Adv Sci (Weinh) (2020) 7:2001853. doi: 10.1002/advs.202001853
97. Chen J, Wu Q, Luo L, Wang Y, Zhong Y, Dai H-B, et al. Dual tumor-targeted poly(lactic-co-glycolic acid)-polyethylene glycol-folic acid nanoparticles: a novel biodegradable nanocarrier for secure and efficient antitumor drug delivery. Int J Nanomedicine (2017) 12:5745–60. doi: 10.2147/IJN.S136488
98. Zhang X, Dong Y, Zeng X, Liang X, Li X, Tao W, et al. The effect of autophagy inhibitors on drug delivery using biodegradable polymer nanoparticles in cancer treatment. Biomaterials (2014) 35:1932–43. doi: 10.1016/j.biomaterials.2013.10.034
99. Saiyin W, Wang D, Li L, Zhu L, Liu B, Sheng L, et al. Sequential release of autophagy inhibitor and chemotherapeutic drug with polymeric delivery system for oral squamous cell carcinoma therapy. Mol Pharm (2014) 11:1662–75. doi: 10.1021/mp5000423
100. Wang Y, Lin Y-X, Qiao Z-Y, An H-W, Qiao S-L, Wang L, et al. Self-assembled autophagy-inducing polymeric nanoparticles for breast cancer interference. in-vivo. Adv Mater (2015) 27:2627–34. doi: 10.1002/adma.201405926
101. Zhou Z, Yan Y, Wang L, Zhang Q, Cheng Y. Melanin-like nanoparticles decorated with an autophagy-inducing peptide for efficient targeted photothermal therapy. Biomaterials (2019) 203:63–72. doi: 10.1016/j.biomaterials.2019.02.023
102. Lin Y-X, Wang Y, Qiao S-L, An H-W, Zhang R-X, Qiao Z-Y, et al. pH-sensitive polymeric nanoparticles modulate autophagic effect via lysosome impairment. Small (2016) 12:2921–31. doi: 10.1002/smll.201503709
103. Salas-Orozco MF, Lorenzo-Leal AC, de Alba Montero I, Marín NP, Santana MAC, Bach H. Mechanism of escape from the antibacterial activity of metal-based nanoparticles in clinically relevant bacteria: A systematic review. Nanomedicine (2023) 55:102715. doi: 10.1016/j.nano.2023.102715
104. Xia L, Wang Y, Chen Y, Yan J, Hao F, Su X, et al. Cuprous oxide nanoparticles inhibit the growth of cervical carcinoma by inducing autophagy. Oncotarget (2017) 8:61083–92. doi: 10.18632/oncotarget.17854
105. Wang R, Yang H, Fu R, Su Y, Lin X, Jin X, et al. Biomimetic upconversion nanoparticles and gold nanoparticles for novel simultaneous dual-modal imaging-guided photothermal therapy of cancer. Cancers (Basel) (2020) 12:3136. doi: 10.3390/cancers12113136
106. Song J-Y, Fan B, Che L, Pan Y-R, Zhang S-M, Wang Y, et al. Suppressing endoplasmic reticulum stress-related autophagy attenuates retinal light injury. Aging (Albany NY) (2020) 12:16579–96. doi: 10.18632/aging.103846
107. Khoobchandani M, Katti KK, Karikachery AR, Thipe VC, Srisrimal D, Dhurvas Mohandoss DK, et al. New approaches in breast cancer therapy through green nanotechnology and nano-ayurvedic medicine - pre-clinical and pilot human clinical investigations. Int J Nanomedicine (2020) 15:181–97. doi: 10.2147/IJN.S219042
108. Mokri N, Sepehri Z, Faninam F, Khaleghi S, Kazemi NM, Hashemi M. Chitosan-coated Zn-metal-organic framework nanocomposites for effective targeted delivery of LNA-antisense miR-224 to colon tumor: in vitro studies. Gene Ther (2022) 29:680–90. doi: 10.1038/s41434-021-00265-7
109. Chen X, Tong R, Shi Z, Yang B, Liu H, Ding S, et al. MOF nanoparticles with encapsulated autophagy inhibitor in controlled drug delivery system for antitumor. ACS Appl Mater Interfaces (2018) 10:2328–37. doi: 10.1021/acsami.7b16522
110. Shi Z, Chen X, Zhang L, Ding S, Wang X, Lei Q, et al. FA-PEG decorated MOF nanoparticles as a targeted drug delivery system for controlled release of an autophagy inhibitor. Biomater Sci (2018) 6:2582–90. doi: 10.1039/c8bm00625c
111. Geng B, Shen W, Li P, Fang F, Qin H, Li XK, et al. Carbon dot-passivated black phosphorus nanosheet hybrids for synergistic cancer therapy in the NIR-II window. ACS Appl Mater Interfaces (2019) 11:44949–60. doi: 10.1021/acsami.9b15569
112. Gong N, Ma X, Ye X, Zhou Q, Chen X, Tan X, et al. Carbon-dot-supported atomically dispersed gold as a mitochondrial oxidative stress amplifier for cancer treatment. Nat Nanotechnol (2019) 14:379–87. doi: 10.1038/s41565-019-0373-6
113. Bajpai VK, Khan I, Shukla S, Kang S-M, Aziz F, Tripathi KM, et al. Multifunctional N-P-doped carbon dots for regulation of apoptosis and autophagy in B16F10 melanoma cancer cells and in vitro imaging applications. Theranostics (2020) 10:7841–56. doi: 10.7150/thno.42291
114. Tang Z, Zhao L, Yang Z, Liu Z, Gu J, Bai B, et al. Mechanisms of oxidative stress, apoptosis, and autophagy involved in graphene oxide nanomaterial anti-osteosarcoma effect. Int J Nanomedicine (2018) 13:2907–19. doi: 10.2147/IJN.S159388
115. Jia X, Xu W, Ye Z, Wang Y, Dong Q, Wang E, et al. Functionalized graphene@Gold nanostar/lipid for pancreatic cancer gene and photothermal synergistic therapy under photoacoustic/photothermal imaging dual-modal guidance. Small (2020) 16:e2003707. doi: 10.1002/smll.202003707
116. Arya BD, Mittal S, Joshi P, Pandey AK, Ramirez-Vick JE, Singh SP. Graphene oxide-chloroquine nanoconjugate induce necroptotic death in A549 cancer cells through autophagy modulation. Nanomedicine (Lond) (2018) 13:2261–82. doi: 10.2217/nnm-2018-0086
Keywords: nanomaterial, multi-functional, cancer, autophagy, autophagy inhibitor
Citation: He K, Chen M, Liu J, Du S, Ren C and Zhang J (2024) Nanomedicine for cancer targeted therapy with autophagy regulation. Front. Immunol. 14:1238827. doi: 10.3389/fimmu.2023.1238827
Received: 12 June 2023; Accepted: 13 December 2023;
Published: 04 January 2024.
Edited by:
Rohimah Mohamud, University of Science Malaysia (USM), MalaysiaReviewed by:
Bin Yu, Zhengzhou University, ChinaCopyright © 2024 He, Chen, Liu, Du, Ren and Zhang. This is an open-access article distributed under the terms of the Creative Commons Attribution License (CC BY). The use, distribution or reproduction in other forums is permitted, provided the original author(s) and the copyright owner(s) are credited and that the original publication in this journal is cited, in accordance with accepted academic practice. No use, distribution or reproduction is permitted which does not comply with these terms.
*Correspondence: Jifa Zhang, empmMjk4MjU3QDE2My5jb20=; Shufang Du, ZHVzaHVmYW5nQHNjdS5lZHUuY24=; Changyu Ren, NjU0MDc2ODM1QHFxLmNvbQ==
Disclaimer: All claims expressed in this article are solely those of the authors and do not necessarily represent those of their affiliated organizations, or those of the publisher, the editors and the reviewers. Any product that may be evaluated in this article or claim that may be made by its manufacturer is not guaranteed or endorsed by the publisher.
Research integrity at Frontiers
Learn more about the work of our research integrity team to safeguard the quality of each article we publish.