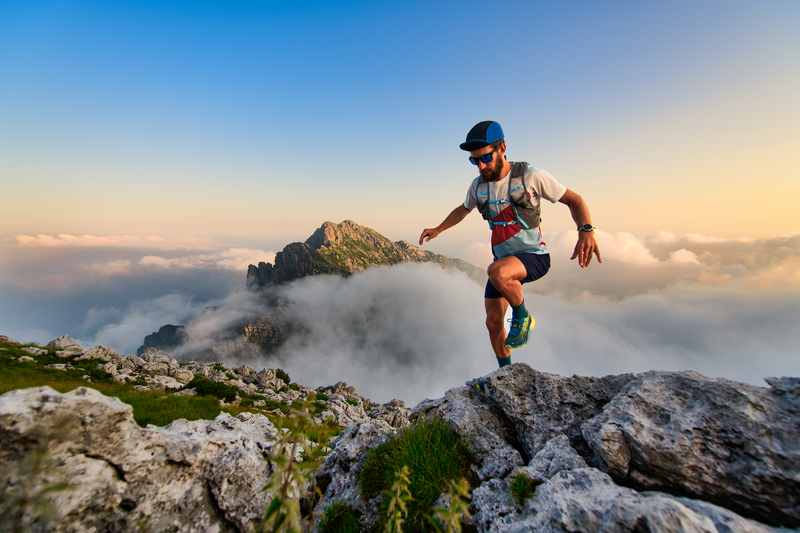
95% of researchers rate our articles as excellent or good
Learn more about the work of our research integrity team to safeguard the quality of each article we publish.
Find out more
REVIEW article
Front. Immunol. , 27 July 2023
Sec. Cancer Immunity and Immunotherapy
Volume 14 - 2023 | https://doi.org/10.3389/fimmu.2023.1237361
This article is part of the Research Topic Development of nanotherapeutics with multi-functionalities for targeting cancer cells View all 7 articles
Malignant tumors have a unique tumor microenvironment (TME), which includes mild acidity, hypoxia, overexpressed reactive oxygen species (ROS), and high glutathione (GSH) levels, among others. Recently, TME regulation approaches have attracted widespread attention in cancer immunotherapy. Nanoparticles as drug delivery systems have ability to modulate the hydrophilicity of drugs to affect drug uptake and efflux in tumor. Especially, the metal nanoparticles have been extensive applied for tumor immunotherapy due to their unique physical properties and elaborate design. However, the potential deficiencies of metal nanoparticles due to their low biodegradability, toxicity and treatment side effects restrict their clinical application. In this review, we briefly introduce the feature characteristics of the TME and the recent advances in tumor microenvironment responsive metal nanoparticles for tumor immunotherapy. In addition, nanoparticles could be combined with other treatments, such as chemotherapy, radiotherapy and photodynamic therapy also is presented. Finally, the challenges and outlook for improving the antitumor immunotherapy efficiency, side effect and potential risks of metal nanoparticles has been discussed.
Cancer is a general term for a broad group of various diseases that can afflict any area of the body. The alternative terms used are malignant tumors and superfluous organisms. It becomes one of the leading causes of death in the worldwide, and it is reported that nearly 10 million deaths were caused by cancer in 2020, accounting for about one-sixth of global deaths (1). It is therefore vital to promote and forge new approaches to fight against tumors. In an effort to more effectively treat cancer, many researchers are focusing on the cellular and molecular mechanisms of tumors, and the characterization of cancers to allow for the development of new highly effective antitumor drugs.
The tumor microenvironment (TME) generated by solid tumors is attributed to key parameters that promote tumor growth, proliferation, angiogenesis, invasiveness, and metastasis, which has been considered one of the major obstacles in the cancer treatment process (2). The TME is composed of multiple intertwined cellular and non-cellular components, the major components of which include immune cells, pericytes, tumor-associated endothelial cells (TECs), tumor-associated fibroblasts (CAF), extracellular matrix (ECM), and peripheral blood vessels (Figure 1). TME also has a series of unique pathophysiological features including hypoxia, acidic pH, high levels of reduced glutathione (GSH) and reactive oxygen species (ROS), vascular abnormalities, specific metabolism, and overexpression of certain specific enzymes (3). It is possible to design drug delivery systems with targeted and intelligent response release based on the distinctive biological properties of TME.
In recent years, with the profound research of tumor microenvironment, the tumor immunotherapy is becoming a proven and crucial therapy for cancer. Tumor immunotherapy avoids greater damage to normal cells by activating the patient’s own immune system to recognize and eliminate tumors, while improving systemic immunity to inhibit tumor metastasis. However, immunosuppression caused by tumor microenvironment is one of the most difficult obstacles in tumor immunotherapy (4, 5). During antitumor therapy, the tumor microenvironment can increase immunosuppression and tumor cell evasion through a series of mechanisms that induce immune tolerance and suppress antitumor immune responses. It is found that tumor-associated macrophages, fibroblasts, and regulatory T cells in the TME can mediate innate immunity and generate antitumor and pro-tumor responses according to the regulation of signaling pathways and chemokines (6). Furthermore, the high expression of immune checkpoints in TME contributes to tumor immune escape. TME can also regulate drug function and penetration as well as enhance drug resistance by protecting pro-proliferative factors in TME and tumors (7). The complex interactions between tumor cells and TME continue to influence tumor progression, therapeutic efficiency and drug resistance. Consequently, the targeted nano-drug delivery systems could be flexibly designed to reshape the tumor microenvironment to address the therapeutic barriers caused by the immunosuppressive tumor microenvironment, which is hopefully enhancing the effectiveness of tumor immunotherapy.
The TME of solid tumor has distinctive features including low pH, hypoxia and immunosuppressive environments that offer new insights and possibilities for specific cancer therapies. Metal nanoparticles have a variety of unique properties which being considered as the ideal candidate for TME modulation. Metal nanoparticles have been used in various studies of TME modulation, including acidic pH, hypoxia and immunosuppression of TME (8). It is reported that metal nanoparticles could be stimulated by different physical factors to produce highly toxic ROS in the TME. Oxidative stress occurs when ROS levels exceed the antioxidant capacity of cells, ultimately leading to tumor cell death (9). Furthermore, it has been shown that the efficacy of photodynamic therapy and radiotherapy is highly dependent on the level of oxygen in the tumor, moreover the hypoxic microenvironment in the tumor could reduce the effectiveness of the treatment (10, 11). Moreover, the hydrogen peroxide produced by the reaction of metal peroxides with water in an acidic environment not only induces oxidative stress, but also generates additional oxygen (12). The reason is that hydrogen peroxide acts as a reactive substrate for molecules such as catalase, alleviating hypoxia in the tumor microenvironment.
Metal nanoparticles have been developed for more than two decades, which have been a trendy topic in nanocarrier materials research due to their unique properties, suitable and tunable drug delivery functions. Owing to the complex tumor immune escape mechanism, the positive rate of immunotherapy in clinical treatment still needs to be improved. Moreover, immunotherapeutic drugs are often distributed to various tissues and organs throughout the body, which cannot achieve precise targeting to the lesion, and causing a series of immune-related adverse reactions. With the development of metal nanoparticles in synthesis and modification strategies, many metals and metallic elements can be formed into nanostructures that can be utilized in the treatment of cancer. It is reported that metal nanoparticles are capable of preventing sudden drug release and avoiding off-target effects, which gives it many advantages such as improved pharmacokinetic properties and flexible control of drug release (13, 14). Moreover, several studies have shown that metal nanoparticles, modulated by surface ligands or intrinsic properties, can respond to the unique chemical properties of TME by precisely delivering immunomodulatory factors to target tissues or specific immune cells, to achieve an effective and enhanced immune response for enhanced therapeutic efficacy (15–17). Recently, there are a large number of metal nanoparticles have been developed, such as gold, silver, copper, bismuth, manganese, iron, platinum and lead, which can be applied in various modalities of cancer therapy in different forms such as antibodies, solids and coatings (18–21). In this review, the current progress of TME-responsive metal nanoparticles for the field of tumor immunotherapy will be summarized, and the main challenges and directions for the development of this field will be outlined.
TME is a dynamic and sophisticated environment made up of diverse cellular and non-cellular components. Briefly, the TME represents all components of a solid tumor other than cancer cells. Cells in TME interact with each other to affect the progression of tumors (22). This specific broad range of cellular interactions will determine tumor responsiveness to drugs (23, 24).
There are multiple functionally distinct cells in TME. Endothelial cells in TME offer nutritional support for tumor growth and development and protect tumor cells from the immune system. Immune cells in TME include innate and adaptive immune cells. Immune cells are involved in various immune responses and activities that shift the behavior of the tumor and its response to therapy. Significantly, immune cells in TME can exert both opposite anti-tumor and pro-tumor effects through different activation states and localization. The fibroblasts in TME permit cancer cells to translocate from the primary tumor site into the bloodstream for systemic metastasis. The extracellular matrix further influences cancer cell migration by altering the physical properties, composition and topography of cancer cells.
In addition to multiple malignant cells, TME also contains secreted proteins, blood vessels and non-malignant cells that support and influence tumor growth. The cytokines and growth factors have been secreted by tumor cells or stromal cells in the TME, which might contribute to abnormal tumor growth, angiogenesis, metastasis and drug resistance (25–27). It has been found that vessels within tumors have ability to exhibit altered structural and functional properties and lead to hypoxia and limited nutrient supply. Furthermore, TME hypoxia can alter gene expression in tumor cells, thereby increasing tumor cell survival and resistance to apoptosis induction (28). However, it is well known that the actual composition of the tumor microenvironment and how it affects cancer progression may differ from patient and cancer type. The progression and development of cancer are affected by the TME components and are under the control of the host immune system. Therefore, targeting and manipulating cells and factors in the tumor microenvironment during cancer treatment might help control malignancy and achieve positive health outcomes (3).
The tumor microenvironment is a main obstacle in immunotherapy, which might be associated with the metastasis, resurgence and drug resistance of malignant tumors. It is commonly known that the cancer progression process is not only influenced by the TME component, but also by the control of the host immune system (3). It is reported that TME remains an essential battlefield between the host immune system and tumors. It has been shown that tumors affect TME by actively recruiting and modulating various cellular phenotypes and functions, which aim to promote immunosuppression and tolerance to tumor-associated antigens (29, 30).
The first step in regulating TME is to regulate the surrounding cells, mainly including fibroblasts, immune cells and vascular cells (31). One of the mostly significant cell types in the tumor microenvironment is the immune-related cells, including pro-tumor cells and anti-tumor cells. Tumor antagonistic cells associated with antitumor immunity include memory T cells, neutrophils, M1-polarized macrophages, natural killer (NK) cells, and dendritic cells (DC). Tumor-promoting immune cells include regulatory T cells (Treg), M2 phenotype tumor-associated macrophages (TAM), bone marrow-derived suppressor cells (MDSCs), natural killer T Type 2 cells (NKT2), N2-polarized neutrophils, and ILC2s (Figure 2) (32–35). The relative numbers of these immune cells and their interrelationships are associated with immunosuppression (36). According to the underlying mechanisms, immunosuppressive pathways can be defined in cytokine-dependent, enzyme-dependent and immune checkpoint-dependent (37, 38). It is found that signaling pathways and chemokines in the tumor microenvironment have ability to regulate immune-related cells to exert anti-tumor and pro-tumor effects. Therefore, tumors can be made more sensitive for immunotherapy by modulating immune signaling pathways, particularly immunosuppressive pathways.
Figure 2 Tumor-associated immune cells in tumor microenvironment. T cells, DCs and M1 contribute to anti-tumor immunity by secreting different signaling molecules such as TNF-α, IL-6 and IL-8 respectively. M1 macrophages exert direct anti-tumor effects through the release of reactive oxygen/nitrogen species (ROS/RNS). Treg, M2, ILC2 and NK2 suppress antitumor immune responses by producing various immunosuppressive cytokines such as TGF-β, IL-10 and IL-13. N2 inhibits T cell proliferation by releasing arginase-1 (ARG1) and solvents the chemokine CCL17 to recruit Tregs.
Metal nanoparticles are nanoparticles derived from metal-containing compounds which represent a variety of nanosized materials that carry metal ions and are conjugated to other inorganic or organic molecules (39). These materials are suitable as diagnostic and drug delivery tools for cancer (40). In comparison to other nanoparticles, metal nanoparticles have their unique physical and chemical properties such as magnetic, optical, thermal, catalytic and electrical properties (41). Metal nanoparticles have been widely used in the development of oncology therapeutics due to the exploitation of their properties, which give them the advantage of overcoming biological barriers and alleviating unfavorable counter processing environments (36). Immunotherapy has now emerged as an attractive option for cancer treatment, which uses the body’s own immune system to fight tumors (42). Metal nanoparticles not only have a direct toxic effect on tumor cells by mediating the reorganization of the extracellular matrix, but also have ability to activate the immune response. Metal nanoparticles interact with Toll-like receptors in order to initiate pro-inflammatory polarization of macrophages. Subsequently, T cells can be activated by macrophages or metal nanoparticles to achieve anti-tumor cytotoxicity (39). Additionally, combining metal nanoparticles with immunotherapy endows unique advantages among numerous immunotherapy pathways, as metal nanoparticles can obtain safer and more effective cancer treatment by providing targeted distribution of immune drugs and greatly enhancing drug bioavailability (43, 44). Because of the physiological barriers, there is a great need for more advanced nanomedicine systems that can effectively and intelligently target TME to enhance treatment outcomes. Metal nanoparticles like magnetic nanoassemblies, gold-based nanoassemblies, calcium-based nanoassemblies, mesoporous nanoassemblies, metal-organic frameworks (MOFs) and manganese-based nanoassemblies have been exploited for improved cancer immunotherapy. At the very same time, the efficacy of a single therapeutic modality is often limited given the tumor suppressive effect. There is also a considerable amount of research into the combination of metal nanoparticles with various types of therapeutic modalities, such as chemodynamic therapy (CDT), photodynamic therapy (PDT), photothermal therapy (PTT), radiation therapy (RT) and immunotherapy. Many metals and metal elements have unique properties that allow precise pairing with other molecules, such as photosensitizers, chemotherapeutic agents and enzymes, ultimately enabling combination therapies including immunotherapy, photodynamic therapy and chemotherapy (45, 46). Metal-based nanoparticles have been demonstrated to reach superior anti-antitumor effects in combination with other therapies (47–49). For enhancing anti-tumor immunotherapy, various metal nanosystems have been reported as shown in Table 1.
Numerous studies have shown that the tumor microenvironment is weakly acidic with a pH of approximately 6.5 to 6.8 due to inadequate perfusion, accumulation of lactate, and dysregulated energy metabolism. It has been shown that the acidic tumor microenvironment is essential for the development of malignancy and the evolution of somatic cells. Furthermore, the acidic tumor microenvironment can influence the malignant behavior of tumors, including metastasis and invasion rates, and affect the mechanisms of immune surveillance (82). Therefore, the pH of the tumor microenvironment appears to be an essential therapeutic objective in the administration of cancer. Given the pH difference between normal tissue and tumor microenvironment, pH-responsive metal nanoparticles are now commonly designed for cancer therapy. Metal nanoparticles can be used as drug carriers for antitumor drug delivery, degrading based on the acidic tumor microenvironment environment, liberating metal ions and antitumor drugs to inhibit or kill tumor cells through combined photothermal therapy, chemotherapy and immunotherapy.
One of the fundamental properties of metallic nanoparticles, particularly nanoparticles such as gold, silver and copper, is the presence of surface plasmon resonance (SPR). SPR is a phenomenon in which photons cause the oscillation of free electron on the metal surface when light is incident on the metal-dielectric interface (83). The SPR will be more pronounced in nanoparticles because of their higher surface to volume ratio in comparison to their bulk counterparts. SPR properties have been broadly used for photodynamic therapy via nanoparticles. In particular, metal nanoparticles can be structured to react to near-infrared absorption to obtain photothermal agents to therapeutic tumors (84). Chen et al. incorporated gold nanobipyramids and copper sulfide in a core/shell structure to form pH-responsive nanoparticles AuNBP@CuS (48). In the acidic tumor microenvironment, Cu2+ released from CuS can be converted to Cu+ by glutathione after a Fenton-like reaction with hydrogen peroxide, producing a highly toxic hydroxyl radical in the tumor area. Meanwhile, AuNBP@CuS shows photothermal properties and photodynamic under NIR-II laser irradiation. Damage-associated molecular model molecules invoked by NIR-II photo/chemotherapy in dying cells can concurrently trigger adaptive immune responses. Damage-associated molecular pattern molecules include adenosine triphosphate, preapoptotic calmodulin, and high-mobility group box-1. This report reveals that AuNBP@CuS performs well in tumor suppressors with an effective immune cell death process.
A proactive approach to invigorate the tumor immune microenvironment is to induce immunogenic cell death (ICD) in tumor cells. ICD promotes dendritic cell maturation and infiltration of cytotoxic T lymphocytes by releasing danger-associated molecular patterns (DAMP) and tumor-associated antigens. This procedure may then reverse the tumor immunosuppressive microenvironment to maximize the sensitivity of immunotherapy (85, 86). It is reported that the tumor cell death could be induced by various therapeutic modalities such as radiotherapy and photothermal therapy in an immunogenic manner (87). Metallic nanomaterials offer the potential to deliver optimal doses of ICD inducers to target tissues, which can enhance the effects while reducing side effects. Dai et al. incorporated Gd3+ and Zn2+ in bimetallic MOF nanoparticles (Gd-MOF-5), which were used as immunomodulators and ICD inducers to modulate immunostimulatory signals (50). It was shown that MOF-5-mediated intracellular Zn2+ overload has a strong ability to activate endoplasmic reticulum stress to induce ICD, which greatly facilitates tumor immunotherapy. Gd-MOF-5 combined with anti-programmed death ligand 1 antibody (αPD-L1) triggered a robust immune response and significantly reduced tumor growth.
Tumor-associated macrophages are an integral part of the TME and perform a crucial function in cancer immunotherapy. Macrophages have different phenotypic and metabolic characteristics in response to various stimuli. TAMs mainly present an anti-inflammatory M2 phenotype stimulated by rich anti-inflammatory stimuli that facilitate tumor growth (88). It has been shown that there is multiple M2-associated signaling cues in TME that reduce the potency of M1-type macrophage polarization (89). Furthermore, it is found that certain metallic nanoparticles currently developed can modulate TAMs from pro-tumor M2 to anti-tumor M1-like phenotypes (90). The utilization of metal nanoparticles to remodel TAMs is a prospering modality in cancer immunotherapy. Zheng et al. developed an Fe-MOF nanoparticle loaded with insoluble RSL3 that can degrade and release Fe3+ in acidic TME (51). The Fentonian catalytic properties of Fe3+ promote lipid peroxidation and disrupt mitochondrial membrane structure, potentially leading to mitochondrial dysfunction. RSL3 is a hydrophobic molecular iron activator that enhances iron-dependent lipid peroxidation in cancer cells and sensitized macrophages. Fe-MOF acts synergistically with RSL3 by boosting iron death-related stress in macrophages that triggers potent pro-inflammatory signaling activation and metabolic conversion, which markedly boosted tumor killing.
These studies show that metal nanoparticles can not only be employed as carriers of drugs for targeted release in the tumor microenvironment to induce immune responses to kill tumor cells, but also directly act as immunostimulatory signal inducers and immunomodulators to trigger immune responses to kill tumor cells, providing further applications of metal nanomaterials in tumor immunotherapy.
One of the richest reducing cellular metabolites is GSH, which plays an essential function in maintaining the redox balance in cells (91). In Addition, GSH is involved in regulating protein folding by mediating the generation and degradation of disulfide bonds in many proteins. Because of abnormal metabolism of tumor tissues, the concentrations of reducing substances in tumor are far higher than those in normal cells (92, 93). Consequently, GSH has been applied as a specific marker for the design and construction of metal nanocarrier materials targeting TME of malignant tumors. Du et al. utilized GSH-sensitive Cu-MOF packed with an inhibitor of the immunosuppressive enzyme IDO and a nitric oxide (NO) donor-nitrosothiol moiety to construct a nanomedicine drug (BMS-SNAP-MOF) (56). High levels of GSH in TME trigger a cascade reaction with Cu-MOF, releasing IDO inhibitors and producing plentiful NO in situ. IDO inhibitors and NO synergistically modulate the immunosuppressive tumor microenvironment, increasing CD8+ T cells and decreasing Treg cells, thereby reducing immune escape and enhancing immunotherapeutic efficacy. Biocompatible, safe and physiologically stable Cu-MOF displays significant future potentials as a drug carrier. Furthermore, metal-based nanoparticles can be used in combination with chemical drugs, physical therapy and photothermal therapy to co-induce ICD. Jiulong et al. co-assembled adriamycin (DOX), adenosine triphosphate and copper ions into ligand polymer nanoparticles, afterwards combined microRNA (miRNA) then further coated with polydopamine (PDA) finally form the miDAC@PDA (Figure 3) (57). The prepared miDAC@PDA nanoparticles can efficaciously accumulate into tumor tissues to liberate drugs in response to laser irradiation and high levels of GSH. Cu2+ mediated GSH depletion disrupts tumor redox homeostasis, thereby amplifying the immunogenic cell death cascade response. This composite metal nanoparticle also activates cytotoxic T-cell infiltration and reshapes the tumor immunosuppressive microenvironment, combining with chemotherapy and photothermal therapy for immunotherapy of tumors.
Figure 3 Schematic illustration of miDAC@PDA preparation and its potential mechanism for cancer immunotherapy (57). (A) Inspiration from the DNA double-helix structure and the potential nucleic acid delivery capability of ATP; (B) preparation pathway of miDAC@PDA; (C) potential therapeutic mechanism of miDAC@PDA for synergistic ICD induction.
In besides, GSH is an antioxidant of endogenous origin that protects cells from damage caused by various oxidants such as lipid peroxides and reactive oxygen species. High concentration of GSH in TME is strongly linked to tumor progression and increased resistance to antitumor drugs, which can diminish the efficacy of antitumor therapy by depleting ROS generated in multiple antitumor therapy types such as CDT and PDT. Therefore, down-regulation of over-expressed GSH in tumor might be an attractive tactic that could improve the effectiveness of tumor therapy. Wang et al. synthesized a core-shell form of gold-manganese selenide nanoparticles (Au@MnSe2 NPs) of which MnSe2 depletes GSH in TME by converting it to Mn2+ and the presence of Au further catalyzes the reaction (58). Au@MnSe2 NPs have robust photothermal conversion efficiencies and stabilization under NIR light irradiation, transforming the absorbed NIR light into heat for tumor eradication and accelerating the CDT and PCT effects. Au@MnSe2 NPs also activated cytotoxic and accessory T lymphocytes and augmented the anti-tumor efficacy of T cells.
Ferroptosis is a novel non-apoptotic programmed cell death that is strongly correlated with tumor progression (94). Ferroptosis is the result of cellular metabolism and dysregulation of redox homeostasis induced by lethal lipid peroxidation, which may be suppressed by directly blocking lipid peroxidation or by depleting iron through genetic means or pharmacological. Significantly, there is growing evidence for a potential physiological effect of Ferroptosis in tumor immunity and suppression (95). GSH consumption has been shown to be effective in enhancing ferroptosis. Dan Zhao et al. constructed fluorescent gold nanoclusters (TfRA-AuNCs), which induced efficient hydroxyl radical generation and GSH depletion, and inhibited tumor growth through enhanced ferroptosis effect (59). Meanwhile, Fe3O4 nanoparticles synthesized by Cong Wu et al. have the ability to induce ferroptosis in macrophages (60). They observed that Fe3O4 nanoparticles were capable of decreasing the glutathione/oxidized glutathione (GSH/GSSG) ratio and suppressing the oxidative stress state in tumor cells, reducing the macrophage viability, and inducing macrophage polarization toward the M1 phenotype. The present study demonstrates the enormous possibility of metal nanoparticles for Ferroptosis treatment in reversing drug resistance to cancer therapy.
It has recently been demonstrated that the increased metabolism due to abnormal cancer cell outgrowth leaves cancer cells in a continuous state of oxidative stress, contributing to elevated basal levels of ROS in TME. ROS plays a complicated and multi-faceted role in TME, facilitating tumor progression and metastasis by a multitude of mechanisms such as the promotion of cellular transformation and tumor angiogenesis, and can therefore be used to create ROS-responsive nanoparticles for cancer immunotherapy (96). Pre-drugs that are specifically activated by ROS in tumor cells can be medically enhanced for drug specificity to tumor cells. Tumor development can be suppressed by delivering and liberating therapeutic drugs such as αPD-L1 and chemotherapeutic agents into tumor cells by combining metal nanoparticles with ROS-responsive prodrugs. Ding et al. developed a ROS-responsive Fe3O4 nanoparticle that was cross-linked to αPD-L1 prodrug nanoparticles via a ROS-responsive linker (62). Fe3O4 nanoparticles can produce toxic hydroxyl radicals (·OH) in the tumor microenvironment for CDT by the Fenton reaction. The cumulative effect of CDT leads to substantial ROS formation to ablate tumor cells, while inducing ICD and the liberation of αPD-L1 out of pre-drug nanoparticles. The combination of ICD and αPD-L1-mediated immune checkpoint blockade therapeutics induces the initiation of effective T cells by boosting the maturation of DCs and decreases the number of regulatory T cells in the tumor, triggering a powerful anti-tumor immune effect that demonstrates a favorable inhibitory effect.
Oxidative stress occurs when ROS levels overwhelm the antioxidant capacity of cells, leading to cell death. Oxidative stress also affects immune cells in TME, where ROS is one of the essential signaling mediators involved in the activation of T cells and NK cells. Slight elevation of ROS induces T cell activation and differentiation, but ROS can also result in the death of activated T cells and maintenance of immune homeostasis. It is found that ROS has been applied by neutrophils and macrophages to destroy cancer cells. Increased ROS in TME is a feature of chronic inflammation, and the interplay between inflammation and oxidative stress mediators can impair the immune response to tumors (97). As a result, ROS in TME and other mechanisms combine to form immune tolerance to tumors. By delicately managing ROS levels in TME, the detrimental effects of ROS can be avoided to enhance antitumor efficacy. Metal nanoparticles are outstanding in terms of oxidative stress induction in cells, and hence many metal nanoparticles have been widely used in recent years to enhance tumor immunotherapy efficacy by modulating ROS levels in TME. Zhang et al. embedded copper ions (Cu2+) in a three-dimensional covalent organic framework to obtain multifunctional metal nanoparticles 3D Cu@COF-TATB (Figure 4) (63). Cu2+ can catalyzed the production of hydroxyl radicals (·OH) via the Fenton reaction, while different types of ROS are efficiently produced to enhance the anti-tumor therapeutic effect. The resulted ROS can be further induced by ICD of cancer cells to boost immunogenicity and further activate the body’s anti-tumor immune response.
Figure 4 Schematic illustration of 3D Cu@COF-TATB for cancer immunotherapy via PDT- and CDT- triggered ICD (63).
Radiotherapy has been demonstrated to be one of the most efficient local therapies for tumors. Radiation can ionize cellular DNA directly or generates a variety of ROS to liberate tumor-associated antigens and drives the secretory of damage-associated molecular patterns (DAMP) within the TME. Therefore, radiation can in practice theoretically induce ICD. but the immunogenic properties of tumor cells are apparently quite poor, and radiation alone can only induce a low level of ICD. Consequently, tumor targeted by metal nanoparticles piggybacking on drugs can be achieved to promote immune activation and enhance checkpoint blockade immunotherapy against sexual tumors. Huang et al. have constructed a nanoparticle (ZGd-NRs) based on gadolinium and zolayic acid (64). ZGd-NRs can be used to induce ICD by depositing X-rays, enhancing ROS production and accumulation, while consuming tumor-associated macrophages and inhibiting regulatory cytokines. This metal nanoparticle promotes antigen presentation, immune initiation and T-cell infiltration through a synergistic strategy of ICD induction and modulation of the immunosuppressive microenvironment, and greatly enhances the efficacy of tumor immunotherapy.
It was suggested that the different levels of ROS in TME have two different effects of supporting and inhibiting tumor growth. The accumulation of ROS in tumor cells has ability to be not only metabolic by-products but also positively contributes to the therapeutic effect. Hence the combined application of modulating ROS levels in TME by metal nanoparticles with immunotherapy is becoming a hopeful therapeutic strategy.
Hypoxia is an essential characteristic commonly found in many solid tumors and is mainly caused by an imbalance between the oxygen supply and oxygen consumption of the tumor tissue (98). Hypoxia could lead to immunosuppression, primarily by upregulating the expression of certain chemokines and the build-up of bone marrow-derived suppressor cells and regulatory T cells (99, 100). Hypoxia further promotes the transformation of macrophages and neutrophils into a tumor-promoting M2 phenotype and inhibits the killing effect of T cells and NK cells, giving tumor cells an immune escape potential. The hypoxic tumor microenvironment limits the antitumor immune response and drives the immune system toward accelerated tumor growth, resulting in further tumor deterioration. Yang et al. developed a hypoxia-sensitive metal nanoparticles, manganese ferrite nanoparticles (MFNs), while encapsulated with adriamycin (Dox), for surmounting tumor immune tolerance due to hypoxia as a way to promote chemoimmunotherapy of tumor (69). The DOX-loaded metal nanoparticles exhibited significant inhibition of primary tumor growth. Consequently, the modulation of hypoxic tumor microenvironment contributes to a durable immune memory effect to avoid tumor recurrence and metastasis.
Hypoxia has an incredibly significant impact on tumor vascularity, growth, and metastasis, and also directly as well as indirectly impairs therapeutic efficacy or leads to increased genetic instability, making it more likely that tumors will acquire drug resistance, and has been considered one of the main obstacles in the process of tumor treatment. Furthermore, hypoxia is among the causes of therapeutic resistance, particularly for PDT and radiotherapy, where oxygen molecules are essential for the eradication of tumor cells. Metal oxides can show functions similar to catalase (CAT) and superoxide dismutase and are commonly used to design nano-delivery systems for tumor hypoxic microenvironment environments. Zeng et al. developed a cerium oxide (CeO2) nanoparticle synergistic photosensitizer for improving the tumor hypoxic microenvironment to optimize the efficacy of PDT and PTT (Figure 5) (70). Cerium oxide nanoparticles were selectively enriched in TME and generated oxygen through the action of catalase, which significantly improved the hypoxic microenvironment in tumors. Zhao et al. developed a TiO2 and Pt-based metal nanoparticle TiO2@Pt/GOx (TPG) to mediate starvation therapy (ST) and sonodynamic therapy (SDT) to alleviate TME hypoxia and improve tumor immunosuppression, abnormal angiogenesis and lymphatic angiogenesis (71). TiO2@Pt has ability to promote the monomeric oxygen and hydroxyl radical generation, resulting in the accumulation of ROS. Therefore, the combination therapy of ST and SDT triggers strong immunogenetic cell death and produces inhibition of tumor growth and metastasis.
Figure 5 Illustration of in vivo regenerable cerium oxide nanozyme-loaded pH/H2O2-responsive nanovesicle for tumor-targeted PDT (70).
It has been suggested that enzymes in the tumor microenvironment exhibit distinct expression levels from normal tissues. TME displays excessive enzyme secretion, including esterase, hyaluronidases, proteases and γ-glutamyl transpeptidase (101). In particular, proteases play a vital role in catalyzing reaction that irreversibly degrade proteins and maintain the stability of the internal tumor environment (102). Abnormal hydrolysis of proteases in tumors will induce tissue remodeling, inflammation and activation of tumor growth factors, contributing to tumor growth and metastasis. The main proteases in TME include metalloproteinases, troponin and trypsin. Metalloproteinases (MMP) are of prominent importance among the proteases. MMP substantially affects tissue integrity, immune cell aggregation and tissue turnover by degrading extracellular matrix components and selectively releasing cell surface bound cytokines, growth factors or their receptors (103). The homeostasis of protein hydrolysis mediated by metalloproteinases actively promotes the generation of the tumor microenvironment. Accordingly, metal-nanoparticles were designed to target the proteases in TME for enhancing immunotherapy of cancer. Meng et al. developed a multifunctional delivery system based on iron oxide nanoparticles (72). It is discovered that iron oxide nanoparticles (SPIO NP@M) can be encapsulated in lung cancer cell membranes, which has been coupled with PD-L1 inhibitory peptide (TPP1) and MMP2 substrate peptide (PLGLLG). Through the specific action of both TPP1 and PLGLLG peptides, SPIO NP@M was delivered and released into the tumor microenvironment narratively. SPIO NP@M could effectively prolong the half-life of TPP1 peptide and has the ability to reactivate T cells and suppresses tumor development, exhibiting an array of merits including low toxicity, directed release and prolonged in vivo circulation.
The cyclic GMP-AMP synthase (cGAS)/interferon gene stimulator (STING) pathway, a key part of innate immunity, has lately been exploited as a progressive candidate for augmenting tumor therapy. Extensive studies have shown that Mn2+ can boost the activation of cGAS/STING to stimulate dendritic cell maturation and macrophage M1 polarization, ameliorate immunosuppression, and enhance the efficacy of tumor immunotherapy. Mn-based metal nanoparticles have been studied and synthesized in combination with DOX or PD-1 checkpoint blockade to synergistically enhance cancer immunotherapy. Zhao et al. encapsulated multi-enzyme-mimetic manganese oxide (MnOx) and αPD-1 with tumor cell membranes (CM) to form the nanoenzyme CM@Mn (Figure 6) (73). This nanoenzyme exhibited intrinsic peroxidase and oxidase-like activity in TME, generating toxic hydroxyl and superoxide radicals that can cause ICD and kill tumor cells. Furthermore, Mn2+ release in TME directly promotes dendritic cell maturation and macrophage M1 repolarization, leading to the reversal of immunosuppressive TME to an immune activating environment. Mn2+ in concert with PD-1 checkpoint blockers presents a potent tumor-specific T cell-mediated antitumor response, inhibits tumor proliferation and metastasis, and induces long-term immune memory effects. Lin et al. constructed hybrid nanoparticles (PL/APMP-DOX NPs) based on manganese phosphate nanoparticles loaded with DOX and phospholipids (74). PL/APMP-DOX NPs triggered the release of DOX in TME to induce DNA damage, and Mn2+ enhanced cGAS/STING activity and enhanced immune activation, presenting excellent antitumor efficacy.
Figure 6 Schematic illustration of the process of preparation of CM@Mn nanozyme and the therapeutic strategy of TME-activable manganese-boosted catalytic immunotherapy combined with PD-1 checkpoint blockade (73).
As an essential substance for providing energy to tumors, ATP plays a vital role in the pathogenesis of many tumors and in the interaction between host and tumor. It has meanwhile been indicated that the level of ATP is also correlated with tumor responsiveness to therapeutic agents (104). Due to the unusually high rapid accretion of cancer cells leading to accelerated metabolism and excessive glycolysis, the content of ATP in TME is eventually increased (105). Accordingly, ATP in TME has appeared as an up and coming target for tumor immunotherapy in the context of developing metal nanoparticles. Yu et al. developed a Zn2+-based composite nanoparticle (IR@ZIF-RGD), which was capable of releasing drugs to augment ATP hydrolysis and depletion based on high level of ATP-responsive degradation in TME (75). The ATP-responsive nanoparticles contributed to tumor metabolic disorders and reversed the immunosuppressive microenvironment by decreasing intracellular ATP level in tumor cells. IR@ZIF-RGD was confirmed to inhibit the growth and metastasis of primary tumors.
The accumulation of adenosine (ADO) and lactate may not only weaken the functionality of cytotoxic T cells, the maturation of dendritic cells may also be decreases, ultimately resulting in the appearance of an immunosuppressive microenvironment. Lactate in tumors is produced by glycolysis, while ADO is produced by ATP catabolism. The metabolic processes of glucose and ATP in tumors can be regulated by synthetic nanoparticles to alter the levels of lactate and ADO in TME, so as to boost the effectiveness of tumor immunotherapy. Dai et al. developed a nanoparticle (MnFe2O4-DCA), which can be efficiently accessed into mitochondria (76). MnFe2O4-DCA downregulates both glucose enzymatic and ATP catabolic processes by affecting immunosuppressive adenosine and lactate levels in TME. ATP responsive nanoparticles have therefore become a topical area in tumor immunotherapy, which may attract more concerns in the near future.
Given that cancer is a highly sophisticated disease, it is competent to evolve multiple immunosuppressive and drug resistance mechanisms during tumor progression. For this reason, combining various biological characteristics of TME such as pH, ROS, and GSH, the exploitation of multi-responsive metal nanoparticles presenting prominent anti-tumor advantages has become a trend of current research.
Low pH and high levels of GSH are two prominent characteristics of TME that may be used to collaboratively control drug release in TEM. Meng et al. constructed Versatility pH/GSH-responsive and Fenton-reactive nanohybrids of polycysteine/iron ions/tannic acid (PCFT) (77). PCFT releases high concentrations of iron ions into tumor cells in TME based on pH and GSH response, and iron ions catalytic endogenous H2O2 from tumor cells into highly toxic hydroxyl groups via the Fenton reaction to induce ferroptosis therapy (FT). FT kills cancer cells by membrane accumulation of lipid peroxides and downregulation of glutathione peroxidase-4. Several studies shown that tumor cells can be obliterated by PCFT and exhibit outstanding antitumor efficacy. Additionally, Tang et al. developed a pH-responsive and GSH-depleted metal nanoparticles DOX@MSN@CuO2 (Figure 7) (78). DOX@MSN@CuO2 was capable of decomposing into Cu2+ and exogenous H2O2, while DOX was released from the weakly acidic TME. subsequently, Cu2+ reacted with the high level of GSH in TME, which induced GSH depletion and Cu2+ was reduced to Cu+. Cu+ and exogenous H2O2 produce toxic hydroxyl groups via the Fenton reaction, leading to apoptosis of tumor cells and thus enhancing CDT.
Figure 7 Schematic of DOX@MSN@CuO2 synthesis and H2O2 self-supply and the mechanism of synergistic therapy against tumor cells (78).
ROS is intimately interrelated with the progression of tumors. Over-accumulated ROS might induce apoptosis of cancer cells through oxidative stress. As such increasing ROS level in TME is a prospective remedy to destroy tumor cells. Exploring ROS and pH dual-responsive metal nanoparticles is currently the hottest spot in the field of tumor immunotherapy, which can initiate specialized release of therapeutic drugs in tumors through the stimulation of microenvironment inside tumors, so as to elevate anti-tumor efficacy and decline drug adverse effects. Chen and his colleagues have developed pH/ROS-responsive nanoplatform (Fe2+@UCM-BBD), which contains DOX, Fe2+ and Ce6 (80). Fe2+@UCM-BBD releases adriamycin in the acidic tumor microenvironment, which can induce tumor apoptosis through DNA damage. In a further, DOX also enhances the production of H2O2, which was able to produce enough material for CDT. The nanoparticles then responsively release Fe2+ for CDT based on high level of ROS in the TME. Generally, Fe2+@UCM-BBD presented great potential for clinical oncology therapy by demonstrating targeted release of therapeutic drug capability and superior tumor suppression through combination therapy.
It has been demonstrated that aerobic glycolysis and excessive accumulation of lactate are both connected with the establishment of a tumor immunosuppressive microenvironment. M2 macrophages, which are known as anti-inflammatory immune cells, facilitate tumor growth and angiogenesis.
Weakly acidic TME can lead to the infiltration of M2 macrophages in tumors and foster tumor progression. Parallel to this, polarized M2 macrophages has ability to induce monocytes to express immunosuppressive transmembrane proteins, thereby blocking the adaptive immune response. Under such circumstances, synthetic multi-responsive metal nanoparticles may potentially revert the tumor immunosuppressive state and accomplish inhibition of tumor progression by alleviating the hypoxia and pH of the tumor microenvironment. Zhang et al. formed a nanocomplex (PALF) by cross-linking polyphenol derivatives with ferrous ions and then introducing lactate oxidase and the mitochondrial respiration inhibitor atovaquone (81). In this nanocomplex, lactate oxidase promoted lactate catabolism, polyphenol derivatives induced ROS accumulation, and atovaquone led to mitochondrial dysfunction, which together reduced oxygen consumption. PALF exhibited high efficiency immunostimulatory properties by modulating tumor microenvironment hypoxia and pH, promoting the release of inflammatory factors in tumor tissues, reduced polarization of M2 macrophages, and increased infiltration of activated T cells. PALF reversed the tumor immunosuppressive state and successfully inhibited tumor proliferation and metastasis, which has probably precluded the application of multi-responsive metal nanoparticles for tumor immunotherapy. Although multi-responsive metal nanoparticles could enhance the controlled release of drugs and improve therapeutic efficiency, the complexity of the design and fabrication processes may affect the potential for translation into clinical applications. It is therefore essential to improve and simplify the preparation of nanomedicines to boost the development of cancer immunotherapy.
Despite the considerable progression of tumor immunotherapy, the availability of immunosuppressive TME has hindered its clinical application. The application of targeted drugs has the potential to enhance the effectiveness of antitumor immunotherapy while decreasing side effects., However, the distinctive characteristic of TME provides an excellent possibility to exploit them as targets for cancer immunotherapy. Metal nanoparticles have been intensively investigated as one of the promising candidates for novel targeted antitumor therapies due to their superior flexible physical and chemical properties. Studies so far have shown that metal nanoparticles provide several advantages, including ease of synthesis, low fabrication cost and flexibility in controlling the form and size of nanoparticles.
Many metals and metallic elements could be formed into nanostructures, which can be engineered and manufactured by diverse synthetic and functionalization methods. It is reported that metal nanoparticles have ability to be surface functionalization with polymers, surfactants, drugs, antibodies, peptides, and oligonucleotides to enhance their ability to targeted delivery of drugs and therapeutic applications. The increasing number of studies related to metal nanoparticles in oncology therapy is due to their inherent characteristics such as unique high drug payload, ease of functionalization with biomolecules, low toxicity, optical properties, high surface area, and radiosensitizer properties. However, metal nanoparticles likewise have a special drawback regarding high aggregation, which due to the large weight, skin color changes, clearance problems and high price. Therefore, further studies should to be explored for evaluating the pharmacokinetic and toxicity properties of the metal nanoparticles before they could be used in clinical applications.
TME-responsive metal nanoparticles have shown great promise in addressing the challenges associated with cancer immunotherapy. TME-responsive metal nanoparticles focus on unique chemical features in TME such as pH, GSH, ROS, hypoxia, enzymes and ATP. TME-responsive metal nanoparticles are also capable of integrating immunotherapy with multiple therapeutic regimens, presenting the tremendous merit of enhancing the efficiency of tumor immunotherapy with reduced adverse effects through the controllable release of drugs and tumor site-specific accumulation. In spite of the widespread progress in research related to the use of metal nanoparticles for tumor immunotherapy, there are still some application constraints and challenges. The most pivotal challenges that require consideration in future research are targeting and toxicity
The biosafety of metal nanoparticles is a basic requirement for its medical application and clinical translation. Future studies should explore systematically the biosafety of metal nanoparticles in combination with immunotherapeutic agents so as to identify the immune system dysfunction and host tissue damage that may result from long-term application. In addition, the results of mouse models typically do not reflect the true effect on human tumour due to the high heterogeneity of human tumor, which increases the complexity of clinical translation of metal nanoparticles. The integration of metal nanoparticles with immunotherapy is in a thriving stage of evolution.
However, TME-responsive metal nanoparticles for immunotherapy have not been used in clinical trials yet. The fabrication of TME-responsive nanodrugs has high complexity, which may be the main constraint that makes it difficult to produce it in a large scale and stable manner. Consequently, it is also quite significant to reduce the fabrication complexity of current metal nanoparticles for their clinical translation. At the same time, it is essential to have a range of industry standards related to the manufacture and use of metal nanoparticles before using it in clinical trials. Specific precautions include controlling the reproducibility and stability of nanoparticle preparation techniques, determining the level of drug accumulation at the target site, and toxicological hazards. The regulatory agencies are required to play an influential role in setting standards for the clinical use of metal nanoparticles and in the assessment of efficiency.
While there are many problems that remain to be solved, metal nanoparticles have introduced innovative approaches to the treatment of tumor immunotherapy, and their application in biology warrants further investigation and development. TME-responsive metallic nanoparticles exhibit the advantage of controlled drug delivery and accumulation, which may reduce the toxicity of immunotherapy and immune-related adverse events. The combination of metal nanoparticles with immunotherapy allows not only to destroy tumors, but also to activate a systemic anti-tumor immune response by initiating the release of tumor antigens and intracellular danger signals, resulting in the suppression of tumor metastasis and recurrence. In spite of the significant advances currently being made in these technologies, the majority of nanoparticles described in this review remain in the preliminary design and optimization stages and in human patients have not yet been tried and tested. Among the greatest existing challenges in the field of TME-targeted metal nanoparticle antitumor immunotherapy is the translation to the clinic, which will eventually define the viability and effectiveness of the approach. Undoubtedly, immunotherapy in combination with TME-responsive metal nanoparticles has tremendous clinical potential and will become an invaluable clinical tool in the combat against cancer.
YZ conceived and designed the current study. RY and LC search and summarize the data. YW, LZ and YY analyzed the data. RY, LC and YY drafted the manuscript. All authors contributed to the article and approved the submitted version.
This work was supported by the Research Program of the Science and Technology Department of Sichuan Province [2022NSFSC0630, 2022YFS0059]; Research Program of the Science and Technology Department of Chengdu [2021-YF05-01034-SN]; Open Project of Central Nervous System Drug Key Laboratory of Sichuan Province (230015-01SZ); Research Program of Science and Technology Department of Sichuan Province (2021YJ0189); National Key Research and Development Program of China [2020YFC2005500].
The authors declare that the research was conducted in the absence of any commercial or financial relationships that could be construed as a potential conflict of interest.
All claims expressed in this article are solely those of the authors and do not necessarily represent those of their affiliated organizations, or those of the publisher, the editors and the reviewers. Any product that may be evaluated in this article, or claim that may be made by its manufacturer, is not guaranteed or endorsed by the publisher.
1. Sung H, Ferlay J, Siegel RL, Laversanne M, Soerjomataram I, Jemal A, et al. Global cancer statistics 2020: GLOBOCAN estimates of incidence and mortality worldwide for 36 cancers in 185 countries. CA Cancer J Clin (2021) 71(3):209–49. doi: 10.3322/caac.21660
2. Xiao Y, Yu D. Tumor microenvironment as a therapeutic target in cancer. Pharmacol Ther (2021) 221:107753. doi: 10.1016/j.pharmthera.2020.107753
3. Arneth B. Tumor microenvironment. Medicina (Kaunas) (2019) 56(1):15. doi: 10.3390/medicina56010015
4. Hegde PS, Chen DS. Top 10 challenges in cancer immunotherapy. Immunity (2020) 52(1):17–35. doi: 10.1016/j.immuni.2019.12.011
5. Tang T, Huang X, Zhang G, Hong Z, Bai X, Liang T. Advantages of targeting the tumor immune microenvironment over blocking immune checkpoint in cancer immunotherapy. Signal Transduct Target Ther (2021) 6(1):72. doi: 10.1038/s41392-020-00449-4
6. Li L, Yu R, Cai T, Chen Z, Lan M, Zou T, et al. Effects of immune cells and cytokines on inflammation and immunosuppression in the tumor microenvironment. Int Immunopharmacol (2020) 88:106939. doi: 10.1016/j.intimp.2020.106939
7. Labani-Motlagh A, Ashja-Mahdavi M, Loskog A. The tumor microenvironment: A milieu hindering and obstructing antitumor immune responses. Front Immunol (2020) 11:940. doi: 10.3389/fimmu.2020.00940
8. Shariatzadeh S, Moghimi N, Khalafi F, Shafiee S, Mehrabi M, Ilkhani S, et al. Metallic nanoparticles for the modulation of tumor microenvironment; A new horizon. Front Bioeng Biotechnol (2022) 10:847433. doi: 10.3389/fbioe.2022.847433
9. Canaparo R, Foglietta F, Limongi T, Serpe L. Biomedical applications of reactive oxygen species generation by metal nanoparticles. Materials (Basel) (2020) 14(1):53. doi: 10.3390/ma14010053
10. Shih CY, Wang PT, Su WC, Teng H, Huang WL. Nanomedicine-based strategies assisting photodynamic therapy for hypoxic tumors: state-of-the-art approaches and emerging trends. Biomedicines (2021) 9(2):137. doi: 10.3390/biomedicines9020137
11. Zhu X, Xu N, Zhang L, Wang D, Zhang P. Novel design of multifunctional nanozymes based on tumor microenvironment for diagnosis and therapy. Eur J Med Chem (2022) 238:114456. doi: 10.1016/j.ejmech.2022.114456
12. Mbugua SN. Targeting tumor microenvironment by metal peroxide nanoparticles in cancer therapy. Bioinorg Chem Appl) (2022) 2022:5041399. doi: 10.1155/2022/5041399
13. Patra JK, Das G, Fraceto LF, Campos EVR, Rodriguez-Torres MDP, Acosta-Torres LS, et al. Nano based drug delivery systems: recent developments and future prospects. J Nanobiotechnol (2018) 16(1):71. doi: 10.1186/s12951-018-0392-8
14. Harish KK, Venkatesh N, Bhowmik H, Kuila A. Metallic nanoparticle: A review. Biomed J Sci Tech Res (2018) 4(2):3765–75. doi: 10.26717/BJSTR.2018.04.0001011
15. Naletova I, Tomasello B, Attanasio F, Pleshkan VV. Prospects for the use of metal-based nanoparticles as adjuvants for local cancer immunotherapy. Pharmaceutics (2023) 15(5):1346. doi: 10.3390/pharmaceutics15051346
16. Liu Y, Crawford BM, Vo-Dinh T. Gold nanoparticles-mediated photothermal therapy and immunotherapy. Immunotherapy (2018) 10(13):1175–88. doi: 10.2217/imt-2018-0029
17. Yang M, Li J, Gu P, Fan X. The application of nanoparticles in cancer immunotherapy: Targeting tumor microenvironment. Bioact Mater (2021) 6(7):1973–87. doi: 10.1016/j.bioactmat.2020.12.010
18. Alphandéry E. Natural metallic nanoparticles for application in nano-oncology. Int J Mol Sci (2020) 21(12):4412. doi: 10.3390/ijms21124412
19. Alvarez MM, Aizenberg J, Analoui M, Andrews AM, Bisker G, Boyden ES, et al. Emerging trends in micro- and nanoscale technologies in medicine: from basic discoveries to translation. ACS Nano (2017) 11(6):5195–214. doi: 10.1021/acsnano.7b01493
20. Griffith DM, Li H, Werrett MV, Andrews PC, Sun H. Medicinal chemistry and biomedical applications of bismuth-based compounds and nanoparticles. Chem Soc Rev (2021) 50(21):12037–69. doi: 10.1039/d0cs00031k
21. Khorasani A, Shahbazi-Gahrouei D, Safari A. Recent metal nanotheranostics for cancer diagnosis and therapy: A review. Diagnostics (Basel) (2023) 13(5):883. doi: 10.3390/diagnostics13050833
22. Barnestein R, Galland L, Kalfeist L, Ghiringhelli F, Ladoire S, Limagne E. Immunosuppressive tumor microenvironment modulation by chemotherapies and targeted therapies to enhance immunotherapy effectiveness. Oncoimmunology (2022) 11(1):2120676. doi: 10.1080/2162402x.2022.2120676
23. Bai Y, Wang Y, Zhang X, Fu J, Xing X, Wang C, et al. Potential applications of nanoparticles for tumor microenvironment remodeling to ameliorate cancer immunotherapy. Int J Pharm (2019) 570:118636. doi: 10.1016/j.ijpharm.2019.118636
24. Liu C, Wang M, Zhang H, Li C, Zhang T, Liu H, et al. Tumor microenvironment and immunotherapy of oral cancer. Eur J Med Res (2022) 27(1):198. doi: 10.1186/s40001-022-00835-4
25. Spill F, Reynolds DS, Kamm RD, Zaman MH. Impact of the physical microenvironment on tumor progression and metastasis. Curr Opin Biotechnol (2016) 40:41–8. doi: 10.1016/j.copbio.2016.02.007
26. LeBleu VS. Imaging the tumor microenvironment. Cancer J (2015) 21(3):174–8. doi: 10.1097/ppo.0000000000000118
27. Korneev KV, Atretkhany KN, Drutskaya MS, Grivennikov SI, Kuprash DV, Nedospasov SA. TLR-signaling and proinflammatory cytokines as drivers of tumorigenesis. Cytokine (2017) 89:127–35. doi: 10.1016/j.cyto.2016.01.021
28. Watnick RS. The role of the tumor microenvironment in regulating angiogenesis. Cold Spring Harb Perspect Med (2012) 2(12):a006676. doi: 10.1101/cshperspect.a006676
29. Mao Y, Keller ET, Garfield DH, Shen K, Wang J. Stromal cells in tumor microenvironment and breast cancer. Cancer Metastasis Rev (2013) 32(1-2):303–15. doi: 10.1007/s10555-012-9415-3
30. Tietze JK, Wilkins DE, Sckisel GD, Bouchlaka MN, Alderson KL, Weiss JM, et al. Delineation of antigen-specific and antigen-nonspecific CD8(+) memory T-cell responses after cytokine-based cancer immunotherapy. Blood (2012) 119(13):3073–83. doi: 10.1182/blood-2011-07-369736
31. Hinshaw DC, Shevde LA. The tumor microenvironment innately modulates cancer progression. Cancer Res (2019) 79(18):4557–66. doi: 10.1158/0008-5472.Can-18-3962
32. Guillerey C. NK cells in the tumor microenvironment. Adv Exp Med Biol (2020) 1273:69–90. doi: 10.1007/978-3-030-49270-0_4
33. Lei X, Lei Y, Li JK, Du WX, Li RG, Yang J, et al. Immune cells within the tumor microenvironment: Biological functions and roles in cancer immunotherapy. Cancer Lett (2020) 470:126–33. doi: 10.1016/j.canlet.2019.11.009
34. Marciscano AE, Anandasabapathy N. The role of dendritic cells in cancer and anti-tumor immunity. Semin Immunol (2021) 52:101481. doi: 10.1016/j.smim.2021.101481
35. Kwantwi LB. Exosome-mediated crosstalk between tumor cells and innate immune cells: implications for cancer progression and therapeutic strategies. J Cancer Res Clin Oncol (2023). doi: 10.1007/s00432-023-04833-9
36. Lv B, Wang Y, Ma D, Cheng W, Liu J, Yong T, et al. Immunotherapy: reshape the tumor immune microenvironment. Front Immunol (2022) 13:844142. doi: 10.3389/fimmu.2022.844142
37. Marzagalli M, Ebelt ND, Manuel ER. Unraveling the crosstalk between melanoma and immune cells in the tumor microenvironment. Semin Cancer Biol (2019) 59:236–50. doi: 10.1016/j.semcancer.2019.08.002
38. Traba J, Sack MN, Waldmann TA, Anton OM. Immunometabolism at the nexus of cancer therapeutic efficacy and resistance. Front Immunol (2021) 12:657293. doi: 10.3389/fimmu.2021.657293
39. Kuchur OA, Tsymbal SA, Shestovskaya MV, Serov NS, Dukhinova MS, Shtil AA. Metal-derived nanoparticles in tumor theranostics: Potential and limitations. J Inorg Biochem (2020) 209:111117. doi: 10.1016/j.jinorgbio.2020.111117
40. Del Solar V, Contel M. Metal-based antibody drug conjugates. Potential and challenges in their application as targeted therapies in cancer. J Inorg Biochem (2019) 199:110780. doi: 10.1016/j.jinorgbio.2019.110780
41. Sintov AC, Velasco-Aguirre C, Gallardo-Toledo E, Araya E, Kogan MJ. Metal nanoparticles as targeted carriers circumventing the blood-brain barrier. Int Rev Neurobiol (2016) 130:199–227. doi: 10.1016/bs.irn.2016.06.007
42. Tan S, Li D, Zhu X. Cancer immunotherapy: Pros, cons and beyond. BioMed Pharmacother (2020) 124:109821. doi: 10.1016/j.biopha.2020.109821
43. Surendran SP, Moon MJ, Park R, Jeong YY. Bioactive nanoparticles for cancer immunotherapy. Int J Mol Sci (2018) 19(12):3877. doi: 10.3390/ijms19123877
44. Xu JJ, Zhang WC, Guo YW, Chen XY, Zhang YN. Metal nanoparticles as a promising technology in targeted cancer treatment. Drug Delivery (2022) 29(1):664–78. doi: 10.1080/10717544.2022.2039804
45. Patel M, Prabhu A. Smart nanocomposite assemblies for multimodal cancer theranostics. Int J Pharm (2022) 618:121697. doi: 10.1016/j.ijpharm.2022.121697
46. Karges J. Clinical development of metal complexes as photosensitizers for photodynamic therapy of cancer. Angew Chem Int Ed Engl (2022) 61(5):e202112236. doi: 10.1002/anie.202112236
47. Li X, Xi D, Yang M, Sun W, Peng X, Fan J. An organic nanotherapeutic agent self-assembled from cyanine and Cu (II) for combined photothermal and chemodynamic therapy. Adv Healthc Mater (2021) 10(20):e2101008. doi: 10.1002/adhm.202101008
48. Chen Y, Liu P, Zhou C, Zhang T, Zhou T, Men D, et al. Gold nanobipyramid@copper sulfide nanotheranostics for image-guided NIR-II photo/chemodynamic cancer therapy with enhanced immune response. Acta Biomater (2023) 158:649–59. doi: 10.1016/j.actbio.2022.12.072
49. Chen Y, Cai Y, Yu X, Xiao H, He H, Xiao Z, et al. A photo and tumor microenvironment activated nano-enzyme with enhanced ROS generation and hypoxia relief for efficient cancer therapy. J Mater Chem B (2021) 9(39):8253–62. doi: 10.1039/d1tb01437d
50. Dai Z, Wang Q, Tang J, Wu M, Li H, Yang Y, et al. Immune-regulating bimetallic metal-organic framework nanoparticles designed for cancer immunotherapy. Biomaterials (2022) 280:121261. doi: 10.1016/j.biomaterials.2021.121261
51. Gu Z, Liu T, Liu C, Yang Y, Tang J, Song H, et al. Ferroptosis-strengthened metabolic and inflammatory regulation of tumor-associated macrophages provokes potent tumoricidal activities. Nano Lett (2021) 21(15):6471–9. doi: 10.1021/acs.nanolett.1c01401
52. Sun Z, Wang Z, Wang T, Wang J, Zhang H, Li Z, et al. Biodegradable MnO-based nanoparticles with engineering surface for tumor therapy: simultaneous fenton-like ion delivery and immune activation. ACS Nano (2022) 16(8):11862–75. doi: 10.1021/acsnano.2c00969
53. Koo S, Park OK, Kim J, Han SI, Yoo TY, Lee N, et al. Enhanced chemodynamic therapy by Cu-Fe peroxide nanoparticles: tumor microenvironment-mediated synergistic fenton reaction. ACS Nano (2022) 16(2):2535–45. doi: 10.1021/acsnano.1c09171
54. Xie L, Li J, Wang G, Sang W, Xu M, Li W, et al. Phototheranostic metal-phenolic networks with antiexosomal PD-L1 enhanced ferroptosis for synergistic immunotherapy. J Am Chem Soc (2022) 144(2):787–97. doi: 10.1021/jacs.1c09753
55. Xu Y, Guo Y, Zhang C, Zhan M, Jia L, Song S, et al. Fibronectin-coated metal-phenolic networks for cooperative tumor chemo-/chemodynamic/immune therapy via enhanced ferroptosis-mediated immunogenic cell death. ACS Nano (2022) 16(1):984–96. doi: 10.1021/acsnano.1c08585
56. Du L, He H, Xiao Z, Xiao H, An Y, Zhong H, et al. GSH-responsive metal-organic framework for intratumoral release of NO and IDO inhibitor to enhance antitumor immunotherapy. Small (2022) 18(15):e2107732. doi: 10.1002/smll.202107732
57. Zhang J, Sun X, Zhao X, Liu L, Cheng X, Yang C, et al. Watson-crick base pairing-inspired laser/GSH activatable miRNA-coordination polymer nanoplexes for combined cancerCchemo-Immuno-Photothermal therapy. ACS Appl Mater Interfaces (2022) 14(18):20762–77. doi: 10.1021/acsami.2c03523
58. Wang Y, Sun X, Han Y, Wang K, Cheng L, Sun Y, et al. Au@MnSe(2) core-shell nanoagent enabling immediate generation of hydroxyl radicals and simultaneous glutathione deletion free of pre-reaction for chemodynamic-photothermo-photocatalytic therapy with significant immune response. Adv Healthc Mater (2022) 11(14):e2200041. doi: 10.1002/adhm.202200041
59. Zhao D, Huang X, Tian Y, Zou J, Wang F, Chen X. Fluorescence imaging-incorporated transcriptome study of glutathione depletion-enhanced ferroptosis therapy via targeting gold nanoclusters. ACS Appl Mater Interfaces (2023) 15(5):6385–96. doi: 10.1021/acsami.2c18289
60. Wu C, Shen Z, Lu Y, Sun F, Shi H. p53 promotes ferroptosis in macrophages treated with Fe(3)O(4) nanoparticles. ACS Appl Mater Interfaces (2022) 14(38):42791–803. doi: 10.1021/acsami.2c00707
61. Zhu L, Li J, Guo Z, Kwok HF, Zhao Q. Synergistic combination of targeted nano-nuclear-reactors and anti-PD-L1 nanobodies evokes persistent T cell immune activation for cancer immunotherapy. J Nanobiotechnol (2022) 20(1):521. doi: 10.1186/s12951-022-01736-8
62. Ding M, Fan Y, Lv Y, Liu J, Yu N, Kong D, et al. A prodrug hydrogel with tumor microenvironment and near-infrared light dual-responsive action for synergistic cancer immunotherapy. Acta Biomater (2022) 149:334–46. doi: 10.1016/j.actbio.2022.06.041
63. Zhang X, Wang S, Tang K, Pan W, Xu H, Li Y, et al. Cu(2+) embedded three-dimensional covalent organic framework for multiple ROS-based cancer immunotherapy. ACS Appl Mater Interfaces (2022) 14(27):30618–25. doi: 10.1021/acsami.2c07739
64. Huang Z, Yao D, Ye Q, Jiang H, Gu R, Ji C, et al. Zoledronic acid-gadolinium coordination polymer nanorods for improved tumor radioimmunotherapy by synergetically inducing immunogenic cell death and reprogramming the immunosuppressive microenvironment. ACS Nano (2021) 15(5):8450–65. doi: 10.1021/acsnano.0c10764
65. Choi B, Choi H, Yu B, Kim DH. Synergistic local combination of radiation and anti-programmed death ligand 1 immunotherapy using radiation-responsive splintery metallic nanocarriers. ACS Nano (2020) 14(10):13115–26. doi: 10.1021/acsnano.0c04701
66. You J, Yuan F, Cheng S, Kong Q, Jiang Y, Luo X, et al. AIEgen-based Sp2 carbon-conjugated covalent organic frameworks with high stability and emission for activatable imaging and ferroptosis in target tumor cells. Chem Materials (2022) 34(15):7078–89. doi: 10.1021/acs.chemmater.2c01726
67. Wang T, Zhang H, Qiu W, Han Y, Liu H, Li Z. Biomimetic nanoparticles directly remodel immunosuppressive microenvironment for boosting glioblastoma immunotherapy. Bioact Mater (2022) 16:418–32. doi: 10.1016/j.bioactmat.2021.12.029
68. Zhao Y, Bian Y, Xiao X, Liu B, Ding B, Cheng Z, et al. Tumor microenvironment-responsive Cu/CaCO(3) -based nanoregulator for mitochondrial homeostasis disruption-enhanced chemodynamic/sonodynamic therapy. Small (2022) 18(38):e2204047. doi: 10.1002/smll.202204047
69. Yang K, Yu G, Tian R, Zhou Z, Chen X. Oxygen-evolving manganese ferrite nanovesicles forhypoxia-responsive drug delivery and enhanced cancer chemoimmunotherapy. Advanced Funct Materials (2021) 31(11):2008078. doi: 10.1002/adfm.202008078
70. Zeng L, Cheng H, Dai Y, Su Z, Wang C, Lei L, et al. In vivo regenerable cerium oxide nanozyme-loaded pH/H(2)O(2)-responsive nanovesicle for tumor-targeted photothermal and photodynamic therapies. ACS Appl Mater Interfaces (2021) 13(1):233–44. doi: 10.1021/acsami.0c19074
71. Zhao Y, Liu J, He M, Dong Q, Zhang L, Xu Z, et al. Platinum-titania schottky junction as nanosonosensitizer, glucose scavenger, and tumor microenvironment-modulator for promoted cancer treatment. ACS Nano (2022) 16(8):12118–33. doi: 10.1021/acsnano.2c02540
72. Meng X, Wang J, Zhou J, Tian Q, Qie B, Zhou G, et al. Tumor cell membrane-based peptide delivery system targeting the tumor microenvironment for cancer immunotherapy and diagnosis. Acta Biomater (2021) 127:266–75. doi: 10.1016/j.actbio.2021.03.056
73. Zhao Z, Dong S, Liu Y, Wang J, Ba L, Zhang C, et al. Tumor microenvironment-activable manganese-boosted catalytic immunotherapy combined with PD-1 checkpoint blockade. ACS Nano (2022) 16(12):20400–18. doi: 10.1021/acsnano.2c06646
74. Hou L, Tian C, Yan Y, Zhang L, Zhang H, Zhang Z. Manganese-based nanoactivator optimizes cancer immunotherapy via enhancing innate immunity. ACS Nano (2020) 14(4):3927–40. doi: 10.1021/acsnano.9b06111
75. Yu M, Zeng W, Ouyang Y, Liang S, Yi Y, Hao H, et al. ATP-exhausted nanocomplexes for intratumoral metabolic intervention and photoimmunotherapy. Biomaterials (2022) 284:121503. doi: 10.1016/j.biomaterials.2022.121503
76. Dai Z, Wang Q, Tang J, Qu R, Wu M, Li H, et al. A Sub-6 nm MnFe(2)O(4)-dichloroacetic acid nanocomposite modulates tumor metabolism and catabolism for reversing tumor immunosuppressive microenvironment and boosting immunotherapy. Biomaterials (2022) 284:121533. doi: 10.1016/j.biomaterials.2022.121533
77. He M, Du C, Xia J, Zhang ZG, Dong CM. Multivalent polypeptide and tannic acid cooperatively iron-coordinated nanohybrids for synergistic cancer photothermal ferroptosis therapy. Biomacromolecules (2022) 23(6):2655–66. doi: 10.1021/acs.biomac.2c00409
78. Tang Z, Jiang S, Tang W, He Q, Wei H, Jin C, et al. H(2)O(2) self-supplying and GSH-depleting nanocatalyst for copper metabolism-based synergistic chemodynamic therapy and chemotherapy. Mol Pharm (2023) 20(3):1717–28. doi: 10.1021/acs.molpharmaceut.2c00937
79. Guo H, Liu F, Liu E, Wei S, Sun W, Liu B, et al. Dual-responsive nano-prodrug micelles for MRI-guided tumor PDT and immune synergistic therapy. J Mater Chem B (2022) 10(22):4261–73. doi: 10.1039/d1tb02790e
80. Chen M, Yang J, Zhou L, Hu X, Wang C, Chai K, et al. Dual-responsive and ROS-augmented nanoplatform for chemo/photodynamic/chemodynamic combination therapy of triple negative breast cancer. ACS Appl Mater Interfaces (2022) 14(1):57–68. doi: 10.1021/acsami.1c14135
81. Zhang Z, Li B, Xie L, Sang W, Tian H, Li J, et al. Metal-phenolic network-enabled lactic acid consumption reverses immunosuppressive tumor microenvironment for sonodynamic therapy. ACS Nano (2021) 15(10):16934–45. doi: 10.1021/acsnano.1c08026
82. Wang JX, Choi SYC, Niu X, Kang N, Xue H, Killam J, et al. Lactic acid and an acidic tumor microenvironment suppress anticancer immunity. Int J Mol Sci (2020) 21(21):8363. doi: 10.3390/ijms21218363
83. Li J, Wang H, Li Z, Su Z, Zhu Y. Preparation and application of metal nanoparticals elaborated fiber sensors. Sensors (Basel) (2020) 20(18):5155. doi: 10.3390/s20185155
84. Singh P, Pandit S, Mokkapati V, Garg A, Ravikumar V, Mijakovic I. Gold nanoparticles in diagnostics and therapeutics for human cancer. Int J Mol Sci (2018) 19(7):1979. doi: 10.3390/ijms19071979
85. Ahmed A, Tait SWG. Targeting immunogenic cell death in cancer. Mol Oncol (2020) 14(12):2994–3006. doi: 10.1002/1878-0261.12851
86. Li Z, Lai X, Fu S, Ren L, Cai H, Zhang H, et al. Immunogenic cell death activates the tumor immune microenvironment to boost the immunotherapy efficiency. Adv Sci (Weinh) (2022) 9(22):e2201734. doi: 10.1002/advs.202201734
87. Duan X, Chan C, Lin W. Nanoparticle-mediated immunogenic cell death enables and potentiates cancer immunotherapy. Angew Chem Int Ed Engl (2019) 58(3):670–80. doi: 10.1002/anie.201804882
88. DeNardo DG, Ruffell B. Macrophages as regulators of tumour immunity and immunotherapy. Nat Rev Immunol (2019) 19(6):369–82. doi: 10.1038/s41577-019-0127-6
89. Biswas SK. Metabolic reprogramming of immune cells in cancer progression. Immunity (2015) 43(3):435–49. doi: 10.1016/j.immuni.2015.09.001
90. Yang F, Xiao Y, Ding JH, Jin X, Ma D, Li DQ, et al. Ferroptosis heterogeneity in triple-negative breast cancer reveals an innovative immunotherapy combination strategy. Cell Metab (2023) 35(1):84–100.e108. doi: 10.1016/j.cmet.2022.09.021
91. Kennedy L, Sandhu JK, Harper ME, Cuperlovic-Culf M. Role of glutathione in cancer: from mechanisms to therapies. Biomolecules (2020) 10(10):1429. doi: 10.3390/biom10101429
92. Bien M, Longen S, Wagener N, Chwalla I, Herrmann JM, Riemer J. Mitochondrial disulfide bond formation is driven by intersubunit electron transfer in Erv1 and proofread by glutathione. Mol Cell (2010) 37(4):516–28. doi: 10.1016/j.molcel.2010.01.017
93. Liu Y, Tian Y, Tian Y, Wang Y, Yang W. Carbon-dot-based nanosensors for the detection of intracellular redox state. Adv Mater (2015) 27(44):7156–60. doi: 10.1002/adma.201503662
94. Zhu L, Meng D, Wang X, Chen X. Ferroptosis-driven nanotherapeutics to reverse drug resistance in tumor microenvironment. ACS Appl Bio Mater (2022) 5(6):2481–506. doi: 10.1021/acsabm.2c00199
95. Jiang X, Stockwell BR, Conrad M. Ferroptosis: mechanisms, biology and role in disease. Nat Rev Mol Cell Biol (2021) 22(4):266–82. doi: 10.1038/s41580-020-00324-8
96. Cheung EC, Vousden KH. The role of ROS in tumour development and progression. Nat Rev Cancer (2022) 22(5):280–97. doi: 10.1038/s41568-021-00435-0
97. Aboelella NS, Brandle C, Kim T, Ding ZC, Zhou G. Oxidative stress in the tumor microenvironment and its relevance to cancer immunotherapy. Cancers (Basel) (2021) 13(5):986. doi: 10.3390/cancers13050986
98. Jing X, Yang F, Shao C, Wei K, Xie M, Shen H, et al. Role of hypoxia in cancer therapy by regulating the tumor microenvironment. Mol Cancer (2019) 18(1):157. doi: 10.1186/s12943-019-1089-9
99. Chiu DK, Tse AP, Xu IM, Di Cui J, Lai RK, Li LL, et al. Hypoxia inducible factor HIF-1 promotes myeloid-derived suppressor cells accumulation through ENTPD2/CD39L1 in hepatocellular carcinoma. Nat Commun (2017) 8(1):517. doi: 10.1038/s41467-017-00530-7
100. Li Y, Patel SP, Roszik J, Qin Y. Hypoxia-driven immunosuppressive metabolites in the tumor microenvironment: new approaches for combinational immunotherapy. Front Immunol (2018) 9:1591. doi: 10.3389/fimmu.2018.01591
101. Zhang ZT, Huang-Fu MY, Xu WH, Han M. Stimulus-responsive nanoscale delivery systems triggered by the enzymes in the tumor microenvironment. Eur J Pharm Biopharm (2019) 137:122–30. doi: 10.1016/j.ejpb.2019.02.009
102. Shimoda M, Ohtsuka T, Okada Y, Kanai Y. Stromal metalloproteinases: Crucial contributors to the tumor microenvironment. Pathol Int (2021) 71(1):1–14. doi: 10.1111/pin.13033
103. Niland S, Riscanevo AX, Eble JA. Matrix metalloproteinases shape the tumor microenvironment in cancer progression. Int J Mol Sci (2021) 23(1):146. doi: 10.3390/ijms23010146
104. Zanoni M, Pegoraro A, Adinolfi E, De Marchi E. Emerging roles of purinergic signaling in anti-cancer therapy resistance. Front Cell Dev Biol (2022) 10:1006384. doi: 10.3389/fcell.2022.1006384
Keywords: metal nanoparticles, tumor microenvironment, cancer immunotherapy, unique responsive, tumor targeting
Citation: Yang R, Chen L, Wang Y, Zhang L, Zheng X, Yang Y and Zhu Y (2023) Tumor microenvironment responsive metal nanoparticles in cancer immunotherapy. Front. Immunol. 14:1237361. doi: 10.3389/fimmu.2023.1237361
Received: 09 June 2023; Accepted: 10 July 2023;
Published: 27 July 2023.
Edited by:
Hao Yang, Sichuan University, ChinaCopyright © 2023 Yang, Chen, Wang, Zhang, Zheng, Yang and Zhu. This is an open-access article distributed under the terms of the Creative Commons Attribution License (CC BY). The use, distribution or reproduction in other forums is permitted, provided the original author(s) and the copyright owner(s) are credited and that the original publication in this journal is cited, in accordance with accepted academic practice. No use, distribution or reproduction is permitted which does not comply with these terms.
*Correspondence: Yong Yang, eXl4cG93ZXJAMTI2LmNvbQ==; Yuxuan Zhu, emh1eXV4dWFuNjY4OEAxNjMuY29t
†These authors have contributed equally to this work
Disclaimer: All claims expressed in this article are solely those of the authors and do not necessarily represent those of their affiliated organizations, or those of the publisher, the editors and the reviewers. Any product that may be evaluated in this article or claim that may be made by its manufacturer is not guaranteed or endorsed by the publisher.
Research integrity at Frontiers
Learn more about the work of our research integrity team to safeguard the quality of each article we publish.