- 1Immunology Department, Hospital Universitario de La Princesa and Instituto de Investigación Sanitaria Princesa, Madrid, Spain
- 2Department of Medicine, Universidad Autónoma de Madrid, Madrid, Spain
- 3Medical Oncology Department, Hospital Universitario de La Princesa and Instituto de Investigación Sanitaria Princesa, Madrid, Spain
- 4Cátedra Universidad Autónoma de Madrid (UAM)-Fundación Instituto Roche de Medicina Personalizada de Precisión, Madrid, Spain
- 5Centro de Investigación Biomédica en Red Cardiovascular, CIBERCV, Madrid, Spain
Tertiary lymphoid structures (TLSs) are clusters of lymphoid cells with an organization that resembles that of secondary lymphoid organs. Both structures share common developmental characteristics, although TLSs usually appear in chronically inflamed non-lymphoid tissues, such as tumors. TLSs contain diverse types of immune cells, with varying degrees of spatial organization that represent different stages of maturation. These structures support both humoral and cellular immune responses, thus the correlation between the existence of TLS and clinical outcomes in cancer patients has been extensively studied. The finding that TLSs are associated with better prognosis in some types of cancer has led to the design of therapeutic strategies based on promoting the formation of these structures. Agents such as chemokines, cytokines, antibodies and cancer vaccines have been used in combination with traditional antitumor treatments to enhance TLS generation, with good results. The induction of TLS formation therefore represents a novel and promising avenue for the treatment of a number of tumor types.
Introduction
Tumors form a complex structure composed by cancer cells and the tumor microenvironment. This microenvironment constitutes a heterogeneous and dynamic system that is the result of the interactions among different components such as immune and stromal cells, blood vessels and the extracellular matrix (1). Depending on the nature of the tumor, the immune component may be composed by cell populations with diverse functional activities, including: myeloid cells (neutrophils, macrophages, antigen-presenting cells (APC)), lymphocyte subsets such as Th-17 and Th-1 cells, follicular T helper lymphocytes (Tfh), regulatory T cells (Tregs), CD8+ cytotoxic cells, and B cells. The majority of myeloid cells (neutrophils, M2 macrophages, mast cells), Th-17 and Treg cells are known to promote tumor development, while cytotoxic, Tfh and Th-1 cells have anti-tumor activities (2). Accordingly, the composition of tumor immune microenvironment plays a key role in the response to therapy and patient outcome. Tertiary lymphoid structures (TLSs) are aggregates of organized immune cells (T and B lymphocytes, plasma cells, APC, macrophages) that appear in areas of chronic inflammation as tumors. Recent studies have reported the association of TLSs with both a better prognosis and the response to immune checkpoint-blocking therapies in a variety of cancers (3–7), suggesting that the presence of TLSs is involved in the progression and immune control of tumor growth. However, the functions of B lymphocytes in tumor development still remain unclear. Some studies suggest that these cells could have a protective function, while other authors support the opposite hypothesis (8, 9).
Here, we summarize the mechanisms of TLS generation and function, and provide insight into the role of antitumor therapies in the development and modulation of TLS characteristics and their consequences in cancer biology.
Characteristics and composition of tertiary lymphoid structures
TLSs, also known as tertiary lymphoid organs (TLOs) or ectopic lymphoid structures (ELSs) (10), are aggregates of immune cells located in non-lymphoid tissues. TLSs are not present in physiological conditions, and normally they arise as a consequence of chronical inflammation in processes such as: autoimmune diseases (11, 12), allograft rejection (13) and in tumors as ovarian cancer, non–small cell lung cancer (NSCLC), colorectal cancer (CRC), breast, stomach, and liver cancers, and melanoma. The number, composition and location of TLSs vary among different tumors (6, 7, 14–25).
Under certain conditions, TLSs are given specific names for example iBALT (inducible bronchus-associated lymphoid tissue), that are used to design TLS-like structures present in the lung that appear in allergies, chronic infections or autoimmune conditions (26, 27). Similar to other TLSs, opposite functions have been described for the iBALT: a protective role for iBALT was reported in some animal models of infection (28–30), whereas a deleterious activity was also reported for these structures in both human and animal models of chronical inflammation (26, 27) and graft rejection (26).
Cell composition and distribution in TLSs may differ among cancer types (1, 31), ranging from disorganized cellular aggregates -often referred to as immature or early TLS-, to well-organized and structured organs similar to SLOs. Mature TLSs typically comprise an internal B-cell zone, surrounded by a peripheral T cell-rich area, mainly composed by CD8+ cytotoxic T lymphocytes, CD4+Th-1 lymphocytes, follicular T helper lymphocytes, and LAMP3+ dendritic cells (31, 32) (Figure 1). Whereas mature primary TLSs are devoid of germinal reaction areas, secondary TLSs show clear active germinal centers with distinct dark and light zones within the B-cell area. The dark zone contains Ki67+ proliferating B cells that express BCL6 and AID (activation-induced deaminase), the enzyme that controls somatic hypermutation and Ig class switch. In the light zone, B lymphocytes are in contact with CD21+ and CD23+ follicular dendritic and T follicular helper (Tfh) cells that participate in clonal selection of B lymphocytes (Figure 1). These structural and functional characteristics point to TLSs as active sites of production of high affinity antibodies (32, 33). Recent studies have reported other subtypes of immune cells located in the T area, as plasma cells and macrophages (9, 34) (Figure 1). Recently, some authors have reported new markers for a better understanding of TLS composition. Some examples of the differences in TLS composition and selected markers are shown in Table 1.
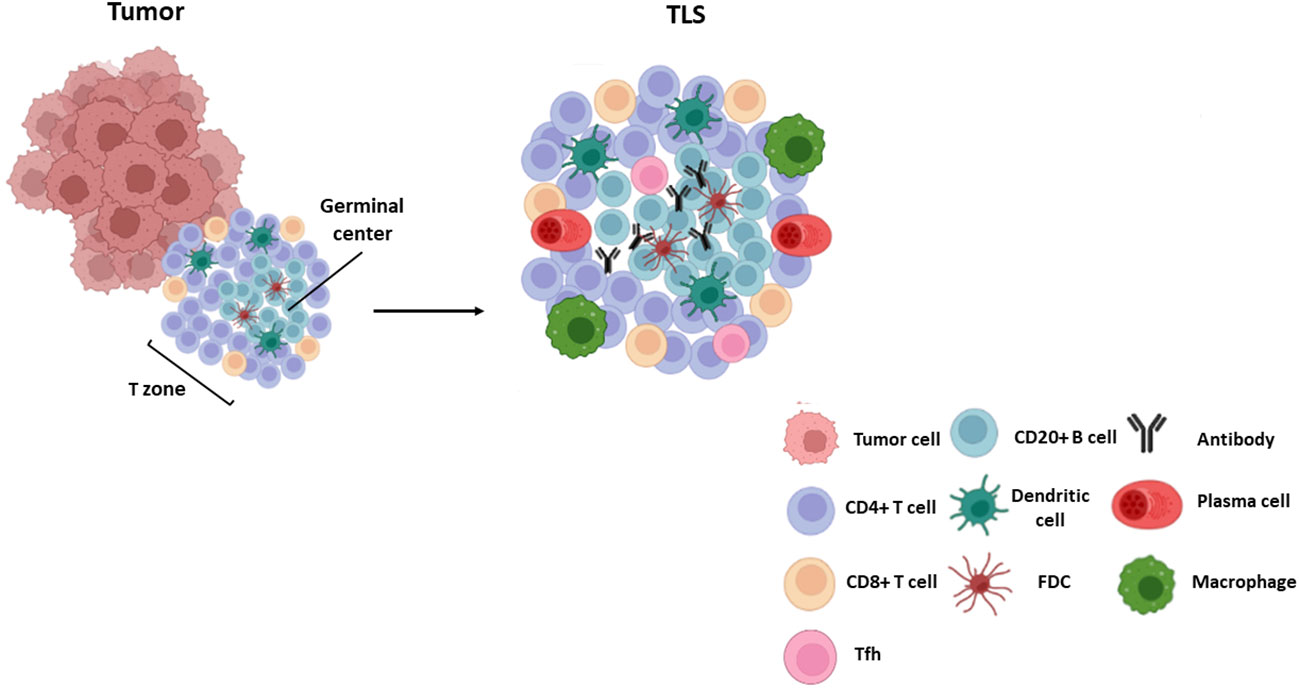
Figure 1 TLS composition. Mature TLSs are composed by a germinal center with B lymphocytes (CD20+ AID+ Ki67+ Bcl6+) and follicular dendritic cells (CD21+ CD23+) surrounded by a T zone composed by T follicular helper cells (Bcl6+ PD-1+ ICOS+ IL-21+), cytotoxic CD8+ T cells and CD4 + T lymphocytes. Other cells such as plasma cells (CD38+ CD138+), mature dendritic cells (DC-LAMP+) and macrophages (CD68+) are also found within these structures.
In addition to mature TLSs, immune cell aggregates without a defined structure may be observed scattered within the tumor. Although these aggregates have been considered as immature forms of TLSs, some authors have proposed that they should be considered as different entities designated lympho-myeloid aggregates (LMA) (44). LMAs have been attributed specific anti-tumoral functions related with their cellular composition. Thus, plasma cell-rich LMAs may represent places of B-cell extrafollicular response, although their functions have not yet been fully elucidated. Likewise, disseminated clusters of CD20+ B cells in the proximity of T lymphocytes and macrophages are found in ovarian cancer, melanoma and other tumor types, which are thought to display either pro- or anti-tumoral activities. Finally, LMAs composed of T lymphocytes, macrophages or NKs have been hypothesized to act as active sites for anti-tumor responses. The relevance of LMAs, their relationship with tumor evolution and patient outcome, and the modulation of their composition and functional characteristics by antineoplastic therapies are issues of great interest that require further research.
Formation of TLSs
The mechanisms of TLS development have not been fully elucidated. It is thought that initial events are similar to those of SLO formation, initiated by lymphoid tissue inducer cells (LTi), which are innate lymphoid cells characterized by the expression of the RORγt and ID2 transcription factors (45, 46). These cells secrete factors such as lymphotoxin α1β2 (LTα1β2) (38, 39) TNFα and IL-17 (47), which interact with their receptors on the surface of stromal cells known as LTo (lymphoid tissue organizers). Stromal cells in turn express adhesion molecules (ICAM1, VCAM1 and MADCAM1), and chemokines (CXCL12, CXCL13, CCL19 and CCL21), which regulate the recruitment of immune cells that participate in TLS formation from adjacent high endothelial venules (HEV) at later stages. These venules contain specialized endothelial cells that selectively express PNAd (peripheral lymph node addressin), a receptor for L-selectin, whose interaction is necessary for leukocyte trafficking to the TLSs (48). This assumption is supported by animal models where the deletion of IL-17Rα, CXCL13 or LTs impairs the development of TLSs. Likewise, the lack of RORγt, involved in LTi induction, also affects TLS generation (16, 49, 50).
Nevertheless, some authors have challenged the notion that LTis are actually necessary for the induction of TLS. Instead, other types of immune cells such as CD8+ T lymphocytes, Th17 cells, and M1-macrophagues among others, have been proposed as promoters of TLS development (51, 52). In addition, some articles highlight the relevance of fibroblasts, which are able to produce factors CXCL13, CCL19 and IL-17, as TLS initiators (53, 54). Similarly, other stromal and immune cells have been proposed as alternative LTo cells. Particularly, LT and TNFα signaling can promote the secretion by fibroblasts of certain chemokines such as CXCL13, CCL19 and CCL21, as well as B cell survival factors including BAFF, IL-7, and April (55). General mechanisms of TLS formation are depicted in Figure 2.
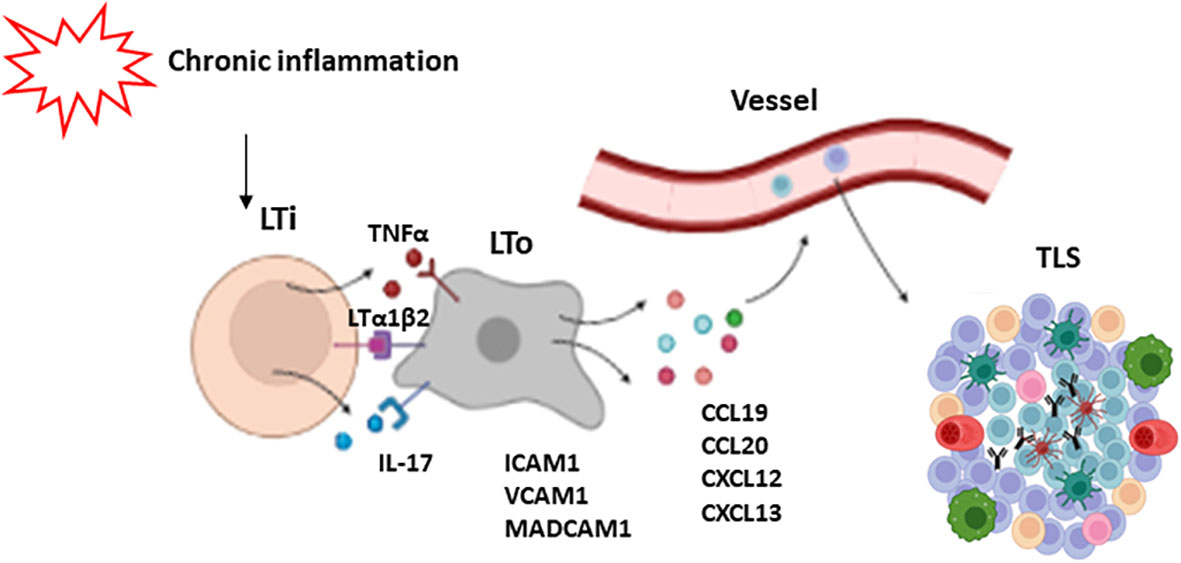
Figure 2 Mechanisms of TLS formation. Chronic inflammation leads to the activation of lymphoid tissue inducer cells (LTi). These cells secrete some factors such as LTα1β2, TNFα and IL-17 which activate LTo cells (lymphoid tissue organizers). As a response, LTo express some adhesion molecules like ICAM1, VCAM1 and MADCAM1, and chemokines like CXCL12, CXCL13, CCL19 and CCL21, which regulate the recruitment of immune cells that participate in TLS development.
TLSs in different types of cancers
Depending on the nature of the tumor, TLSs can be found in different proportions ranging from 10-20% in ovarian, hepatic and oral cancers (24, 40, 56) to 50% in breast cancer (35), NSCLC (17), melanoma (6) and gastrointestinal tumors (24), and more than 80% in colorectal cancer (21) and lung squamous cell carcinoma (57).
The presence of TLSs in tumors has been associated with variable outcomes. These structures may display either pro- or anti-tumoral activities, which are related to factors such as the number, composition and location of the TLSs. Higher densities of TLSs are related to a better prognosis in tumors including: ovarian (58), NSCLC (15), breast, renal cell, pancreatic (42), and hepatocellular (24) cancers, gastrointestinal tumors (59) and melanoma (6, 17). In this regard, in a murine model of metastatic melanoma, the use of a LTα fusion protein induces the formation of lymphoid structures in the tumor bed, where tumor antigen-specific lymphocytes are generated (60). Likewise, the proportion of mature dendritic cells in TLSs is highly associated with cytotoxic T cell responses and long survival in patients with non-small cell lung and rectal cancers (61, 62).
In contrast, in a murine model of hepatocarcinoma, incomplete TLS formation at the early stages of tumor development results in an impaired immune response and tumor growth. During tumor progression, TLSs may act as a niche for malignant progenitor cells, thus promoting tumor development (41). In addition, TLSs composed by high proportions of Tregs, which inhibit anti-tumoral responses, can promote neoplasia development. Indeed, tumor infiltration by Tregs has been linked to poorer outcomes in several types of cancer, including ovarian, breast and hepatocellular cancer (58, 63, 64). In support of these findings, another study demonstrated the presence of high proportions of Tregs in TLSs using a mouse lung adenocarcinoma model. Importantly, depletion of Tregs results in T cell proliferation and the triggering of anti-tumor responses (65).
Depending on the location of TLSs (intra- or peritumoral), they may also exhibit different immune responses. While intratumoral TLSs have been associated with antitumor responses, peritumoral TLSs have been mainly related to protumor responses and poorer outcomes in patients with breast cancer, intrahepatic cholangiocarcinoma and pancreatic cancer (58, 65). Furthermore, in colorectal cancers, the presence of peritumoral TLSs is associated with advanced stages of the disease, tumor progression and worse prognosis (66–68). On the contrary, in hepatocellular carcinoma, peritumoral TLSs have been related to better outcomes (69). The mechanisms involved in this differential functionality remain unknown, but some studies in animal models have suggested that peritumoral TLSs may act as a pathway for cancer dissemination, resulting in worse prognosis (41), although this needs to be studied in depth. These findings are summarized in Figure 3.
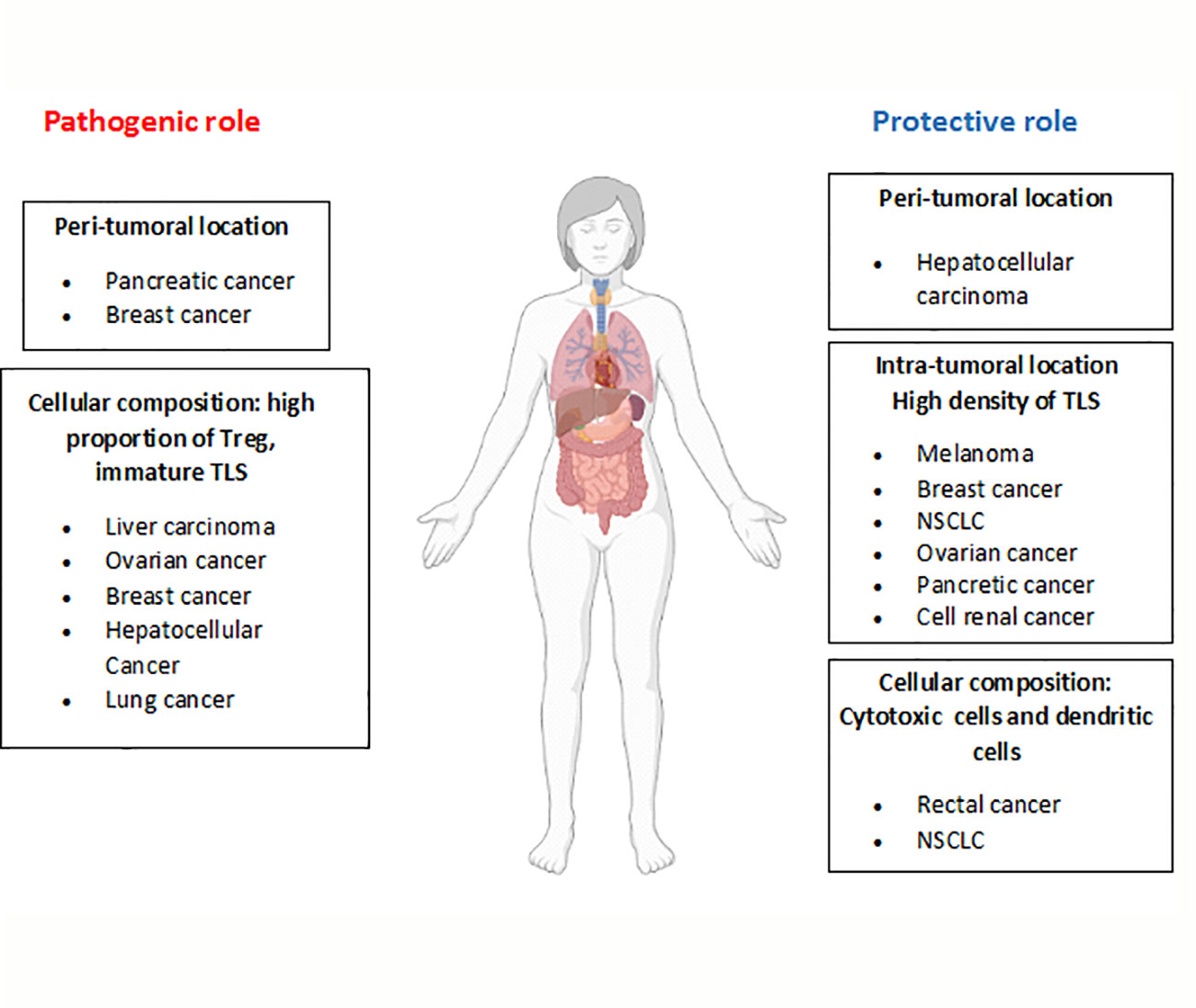
Figure 3 Dual role of TLSs in tumor development. Depending on the characteristics of TLSs (location, cellular composition, etc), opposite roles have been described for these structures in the development of different tumor types. The figure summarizes TLS features related to their pathogenic or protective role (bold letters) and the tumor types involved.
B cells in tumor TLSs
Different subsets of B cells can be found in the tumor microenvironment, mainly associated with TLSs. Mature B cells, plasmablasts and plasma cells have been found in several types of cancer, such as ovarian and prostate cancers (70), NSCLC (71) and renal cell cancer (43). Likewise, IgM+ plasma cells were detected in breast cancer and head and neck squamous carcinoma (72). Regulatory B cells (Breg), a special subset of B cells that play immunosuppressive roles, have also been detected in various types of cancer, such as prostate, gastric, hepatocellular and breast cancer (73–75). On the other hand, naive B cells were described to be very rare in some tumor types such as breast cancer (76), NSCLC (77) and melanoma (78).
The role of B cells in tumor biology has not been studied in depth, but again both pro- and antitumor activities were described for these cells (79, 80). B cells, however, can contribute to cancer eliminatation via tumor-associated antigen presentation or antigen transmission to dendritic cells, together with antibody production and ADCC (antibody-dependent cell-mediated cytotoxicity)/ADCP (antibody-dependent cell-mediated phagocytosis) phenomena. But the potential formation of immune complexes may increase inflammation, angiogenesis, and immunosuppression within tumor microenvironment, resulting in a poor outcome (81).
Most findings related to this question are based on mouse models. Thus, the protumor function of B cells was evidenced in μMT B cell-deficient mice, where reduced tumor growth was observed in MC38 colon carcinoma, B16 melanoma and thymoma (82). In the same line of evidence, another author reported a similar result after IgM antibody depletion in a murine melanoma model and in Rituximab-treated colorectal cancer patients (83). Opposite actions of B cells in tumor development may be partially explained by the existence of a heterogeneous population of varying composition, including subsets with immunosuppressive phenotype. Thus, recent investigations have described a Breg population composed of IL-10+ B cells that coexist with regulatory T cells (Tregs) in human breast and ovarian cancers, which favor the development of metastasis and promote tumor growth (84, 85). Interestingly, TGFβ production by Breg promotes both the polarization of CD4+ T lymphocytes toward Treg and the development of M2 macrophages cells in a mouse model of metastatic breast cancer, resulting in protumor activity. The observed effect in tumor development has been explained by the production of IL-10 by Tregs (86, 87). In addition, M2 macrophages synthesize C1q, a complement component that binds to the Fc portion of anti-tumor antibodies and triggers the classical component cascade in the presence of complement components such as C1r, C1s, C4, C2, C3 and C5 produced by tumor cells. These events result in the generation of some anaphylatoxins (C3a and C5a), which promote inflammation and angiogenesis. Similarly, B cells favor tumor growth by producing VEGF, which induces neovessel formation (88).
In the central areas of TLSs, however, B cells experience antibody class switching and somatic hypermutation, becoming plasma cells that produce tumor-specific antibodies (9) and may exert antitumor actions. In this regard, B lymphocytes in TLSs have been implicated in antitumor responses in patients with melanoma and ovarian cancer (37, 89). Similarly, in hepatocellular cancer, the proportion of B cells in TLSs directly correlate with the number of apoptotic tumor cells, and inversely correlate with the density of proliferating tumor cells (90).
Function of antibodies in tumors
The ability of plasma cells from TLSs to produce specific antibodies (IgG and IgA) against tumor antigens has been demonstrated in multiple cancer types, such as NSCLC, breast cancer, and melanoma (36, 71, 91, 92). These cells may egress the TLSs and migrate into the tumor bed. In cancers with a higher proportion of TLS and plasma cells, specific antibodies can be detected surrounding the tumor, suggesting that such antibodies contribute to antitumor responses (43).
The identification of the antigens specifically recognized by intratumoral B lymphocytes is complex, and only a small number of antigens, mostly intracellular, have been described so far. These antigens are mainly related to proteins overexpressed by the tumor, or to tumor-specific posttranslational modifications or protein mutations. These antibodies may exert their anti-tumor functions by either direct inhibition, CDC (complement-dependent cytotoxicity), ADCC or ADCP. It is noteworthy that the frequent presence of autoantibodies in cancer patients, which seem to either pre-exist or arise by somatic hypermutation phenomena apparently favored under tumor conditions (44, 93). The isotype of autoreactive antibodies has been related to the prognosis of some types of cancer. Thus, in patients with breast cancer, ex vivo IgG autoantibody responses were associated with lower recurrence-free survival and reduced intratumoral CD8+ T-cell infiltrate, whereas IgA responses correlated with germinal center activity and increased number of TLSs (94). However, further research is needed to clarify the relative role of the different antibody isotypes in specific tumor settings.
Similarly, both isotype and tumor type may have an impact on anti-tumor activity of tumor antigen-specific antibodies. Thus, IgA can promote tumor development in mouse models of prostate and liver cancer whereas in human ovarian cancers, this isotype has a protective role. Accordingly, B cells produce IgA that binds to receptors universally expressed by ovarian cancer cells, sensitizing tumor cells to be eliminated by T cells and thus preventing tumor progression (92, 95). In contrast, immunosuppressive B cells in liver cancer are plasma cells expressing IgA, IL-10 and PD-L1. This phenotype depends on TGFβ-receptor (TGFβR) signaling (91). It is plausible that the presence of higher proportions of TReg expressing TGF-β whitin TLSs promote the generation of suppressive B lymphocytes, supporting the notion that TLS composition is determinant for anti-tumor activity.
Regarding IgG, it was described that elevated levels of IgG4 antibodies were associated with a poor prognosis in patients with melanoma (96), whereas in patients with NSCLC they were associated with a better outcome (97). IgG1 potentiates antitumor responses through the induction of T lymphocyte activity. Conversely, this isotype can exert a protumor function through complement activation (98), in particular at the C1q level. As a consequence, the production of C5a and C3a anaphylatoxins increases, fostering inflammation. Furthermore, these factors may promote angiogenesis and have been related to T cell depletion, thus favoring tumor progression (98, 99). In line with these findings, another report shows that in vitro formation of immunocomplexes may contribute to the suppression of cytotoxic activity (100). Hence, it is possible that the presence of immunocomplexes in the TME counteracts anti-tumor immune responses, resulting in the promotion of tumor growth.
Modulation of TLS formation
As mentioned in previous sections, the number, location and composition of TLS, including the type of antibodies produced, may condition tumor progression and are associated with different patient outcomes. The modulation of these characteristics by specific strategies may therefore contribute to optimize antitumor therapy. Several approaches are currently underway to promote the formation of TLSs with anti-tumor action (Figure 4), some of which are detailed below.
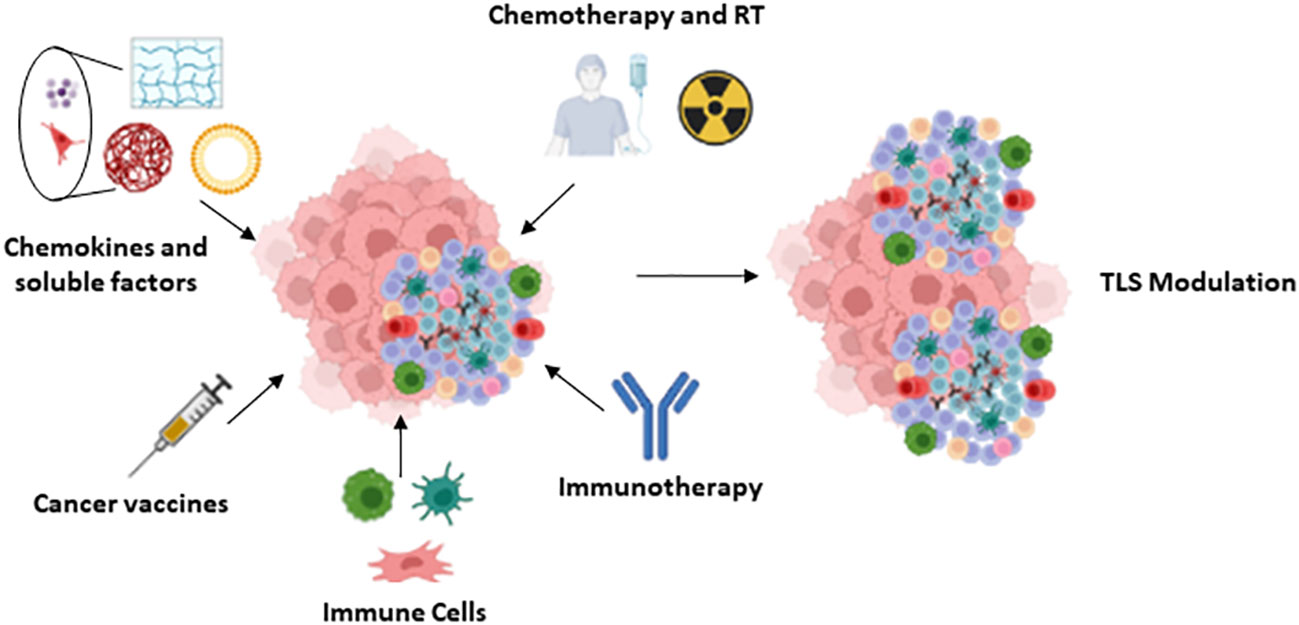
Figure 4 TLS modulation: Summary of the different approaches proposed for the modulation of the TLS.
Chemotherapy
The impact of radiotherapy and chemotherapy agents on TLS development, as well as the potential role of these treatments on patient clinical outcome were recently investigated with contrasting results. For instance, radiotherapy locally induced the expression of MHC I and co-stimulatory molecules in a mouse model of breast cancer and increased CD8 + T cell infiltration into human tumors, which promoted an anti-tumor response (100). On the contrary, this treatment led to a decrease in the number of TLSs, followed by TLS restoration associated with a Treg enrichment in the TME (101). Similarly, in squamous cell lung cancer neoadjuvant chemotherapy impaired maturation of TLSs and germinal centers (57). Furthermore, in patients with lung squamous cell carcinoma and urothelial cancer, corticosteroid treatment used to control chemotherapy side effects reduced the number of TLSs, consequently leading to worse outcomes (33, 57, 102).
Conversely, some reports describe the induction of TLSs after neoadjuvant chemotherapy or radiotherapy in NSCLC, hepatoblastoma and pancreatic ductal adenocarcinoma, conferring a favorable prognosis (103). Interestingly, after radiotherapy in patients with breast cancer, tumors with higher densities of TLSs experienced an increase in tumor cell apoptosis (104). Other studies have shown that CXCL13 and Tfh and Th1 gene signatures were associated with improved responses in breast cancer patients treated with chemotherapy (105). In this line of evidence, another report based on a immunocompetent mouse model shows that neoadjuvant chemotherapy induces an ICOS-L+ B cell population that expresses the complement receptor CR2, which is associated with the development of TLS and improved disease-free and overall survival (106).
Cyclooxygenase-2 (COX-2) is a key mediator of pro-tumor responses, and is involved in the suppression of anti-tumor immunity, angiogenesis and proliferation of cancer cells (107–109). A study in prostate cancer revealed that COX-2 levels were reduced in the TLSs of patients with spontaneous cancer regression (110). Thus, therapies based on COX-2 blockade could be an attractive strategy to promote TLS-driven tumor immunity.
Mechanisms involved in the modulation of TLS formation by chemotherapy or radiotherapy have not been fully elucidated yet. TLSs experience cell apoptosis and transitory size reduction during radiotherapy. After several days, TLSs tend to normalize; however, the number of apoptotic cells still remains high, and CD8+/TReg ratio decreases during later stages, thus reflecting the immunomodulatory effect of radiation (101). Likewise, chemotherapy-induced tumor apoptosis promote an increase of phosphatidyl serine expression in the TME, which leads to a tolerogenic switch in immune infiltrate and subsequent tumor escape (111). Chemotherapy, however, may induce immunogenic cell death (ICD), that leads to the release of damage-associated molecular patterns (DAMPs) and potentiates immune antitumor response (112).
Immunotherapy
Recently, different forms of immunotherapy have been used successfully to treat cancer. Among other effects, many of these treatments can modulate the formation and composition of TLSs. Thus, in a mouse model of breast and pancreatic tumors, the combination of anti-PDL1 therapies with antiangiogenic treatments can promote the development of TLSs and consequently the reduction of the tumor size. This was explained by the induction of HEV formation followed by TLS development and CD8+ lymphocyte activation, which resulted in tumor elimination (113). In line with these findings, the induction of TLSs was observed after the administration of anti-PD-1 in patients with lung cancer (114). Furthermore, cancer patients who responded to PD-1 and/or CTLA4 blockade had a higher basal number of TLSs with increased B-cell densities than non-responders (5, 7, 115, 116). In line with this, a recent multicohort phase 2 trial of pembrolizumab in combination with low-dose cyclophosphamide in patients with advanced soft-tissue sarcomas indicates that the presence of TLS is related to a better response to this therapy, especially in those patients in whom TLSs are not enriched in TReg (117). Another report demonstrates that an early response to anti-PD-1 antibody pembrolizumab in patients with different tumor types is associated with a gene signature related to Tfh cells (118), which are the main producers of IL-21 in the TME (119, 120). The blockade of this cytokine decreases B cell activation induced by the coadministration of anti-PD-1 and anti-CTLA-4 therapy (121). In addition, Th-1-oriented Tfh cells in TLSs are associated with higher expression of IL-21, and promote immunoglobulin and cytokine production after interaction with B and CD8+ lymphocytes, respectively (103). In line with these findings, a recent article in a mouse model of squamous lung cancer describes how anti-PD-1 increases circulating Tfh (cTfh), the number of TLSs and activation of B cells within these structures. Furthermore, anti-PD-1 induces CCL21 production in tumors, which may mediate cTfh migration to TLSs and subsequent B cell activation (122).
Cancer vaccines can also regulate the formation of TLSs. For example, an intradermal vaccine with an irradiated allogeneic granulocyte colony stimulating factor (GM-CSF), in combination with cyclophosphamide, promoted TLS formation in a cohort of 59 patients with pancreatic ductal adenocarcinoma (123). Furthermore, in patients with cervical neoplasia (CIN2/3), increased TLS development and maturation could be observed in regressing lesions after human papillomavirus vaccination (124). In these patients, vaccination increased the infiltration of CD8+ T cells, which secreted mediators that promoted the development of organized structures with germinal centers and T areas similar to TLSs, associated with HEV. Conversely, in unvaccinated patients the immune cells had a diffuse pattern distribution and were not organized into TLSs.
Chemokines and soluble factors
The development of TLSs in tumors can be fostered by using molecules involved in the formation of these structures. For instance, LTβ receptor (LTβR) ligands promote the development of intra-tumoral TLSs associated with a protective role, and enhance survival in combination with immune checkpoint inhibitors in mice (125). Another report demonstrated that the expression of LTα in a murine model of pancreatic cancer increases CXCL13 and CCL21, resulting in the development of B and T zones within the TLSs. In this model, the expression of both molecules depends on the TNFR1 receptor, thus suggesting that signals through this receptor are sufficient for TLS formation (126). In addition, transgenic expression of IL-6 and its receptor gives rise to the increase of CXCL13 levels, which promote TLS development (127). Furthermore, TLR1, TLR2 and TLR4 ligands were also used for TLS neogenesis in several mice models of cancers (128–130). These molecules belong to the toll like receptor family, and are expressed in the surface of both innate immune cells and some non-immune cells like fibroblasts and epithelial cells. Signals triggered by the activation of these receptors lead to the activation of immune responses through the secretion of cytokines and chemokines. Thus, TLR ligands may represent a promising strategy to promote TLS-based anti tumor immune responses.
LIGHT (tumor necrosis factor superfamily member 14, TNFSF14) is expressed by activated immune cells (131), and binds to lymphotoxin beta receptor (132), which is present in stromal, epithelial and myeloid cells (133). This cytokine is known to play a key role in anti-tumor responses (134). Thus, the combination of LIGHT with an anti-VEGF antibody promotes T cell migration to the tumor, reducing tumor resistance to checkpoint blockade treatments (135). In addition, LIGHT induces the production of pro-inflammatory cytokines and chemokines such as IL-1β, IL-6 and CCL21, which recruit immune cells and enhance TLS development. In this regard, a fusion protein of LIGHT with a vascular targeting moiety (LIGHT-VTP) induces TLS formation in a mouse model of pancreatic cancer (125). Likewise, the combination of LIGHT-VTP with anti-VEGF and immune checkpoint inhibitors increase the amount of T cells and the number of HEVs in glioblastoma and lung metastases (136, 137).
Different approaches have been proposed to selectively deliver soluble factors to the tumor and minimize side effects secondary to systemic administration. One of these strategies is based on the use of collagen matrices containing therapeutic molecules. Successful results were obtained in mice that developed functional TLS in response to artificial collagen matrix with a thymus-derived stromal cell line that expressed LTα (138). The functionality of these TLSs was also proven by transplantating these structures in SCID mice, which developed a secondary immune response (139). Likewise, a collagen sponge scaffold containing gel beads with LTα1β2, CCL19, CCL21, CXCL12, CXCL13, and soluble RANK ligand, was implanted in mouse kidneys, and the presence of TLSs with defined T and B cell areas, follicular dendritic cells, and HEV could be demonstrated (140). An additional option is to use hydrogels, that have the ability to take up water and retain it (141). In vitro models have shown that hydrogel containing stromal cells that express BAFF and IL-4 increase the amount of B cells and promote class-switch events (142). Nanoparticles also represent a useful strategy to induce TLS formation. One of the advantages of this approach is that the release of the molecules contained inside nanoparticles can be tightly controlled, either through chemical properties of nanoparticle components or by cargo liberation under specific environmental conditions: e.g. pH, oxygen tension, protease activity, and so on. In addition, nanoparticles may be directed to specific structures or cell types by the addition of selected targeting molecules. Different types of nanoparticles were developed, such as liposomes, micelles or nanoparticles made from polyesters. These nanoparticles have the capacity to deliver different factors such as cytokines, chemokines, antigens, RNAs, etc (143–145).
Immune cells
Some studies support the application of modified immune cells to induce the generation of TLSs within tumors. For instance, in a mouse model of sarcoma, the use of dendritic cells expressing T-bet promotes lymphocyte infiltration, Th1 responses and the development of TLSs, resulting in a reduction of tumor growth. This effect is mediated by the secretion of IL-36γ from Tbet-expressing dendritic cells (146, 147). This cytokine induces the expression of adhesion molecules and chemokines such as IL-8, CCL2 and CCL20 in endothelial cells, promoting T cell migration (148). A recent study in human colon cancer has shown that tumor IL-36γ associates with increased amount of central memory CD4+ T cells and a higher TLS number (149). In line with these results, DCs expressing chemokines involved in secondary lymphoid tissue development rapidly lead to increased T cell infiltration and enhance intratumor immune responses when injected into melanoma tumors (150). In addition, dendritic cells promote LTβR-dependent HEV differentiation and lymphocyte recruitment in the lymph nodes (151), and constitute a source of chemokines (CXCL12, CCL20/21,CCL19) involved in TLS development in the lungs of influenza virus-infected mice (152). In human lung cancer, autologous dendritic cells expressing CCL21 reduce tumor size, and increase the expression of some cytokines and chemokines like IFNγ, IL-12, and CXCL10 among others (153, 154). Several clinical trials are currently ongoing to evaluate the safety of a vaccine based on CCL21-expressing dendritic cells in different cancer types (155, 156).
Other cell types may be utilized to promote TLS formation. Human mesenchymal stem cells stimulated with TNF-α and IL-1β express higher levels of CCL19, VCAM-1 and ICAM-1, and induce CD4+T cell proliferation. These cells may act as LTo, generating the inflammatory environment required for TLS formation (157). Furthermore, intratumoral inoculation of stimulated LIGHT+ macrophages is sufficient to promote the development of TLS in a mouse model (125). These macrophages express high levels of CCL21 and TNFα, both of which enhance TLS formation in mice. Finally, in a model of infection with Salmonella, resident macrophages expressing CXCL13 and CX3CR1 promote the infiltration of B and CD4+ T cells in areas of infection, where they are activated, giving rise to TLS induction (158). Although these studies prove the involvement of these cell types in TLS generation, further studies are required to establish their suitability in a clinical setting.
Concluding remarks
TLSs are structures where effective adaptive immune responses against tumors take place. In many cases, their presence is associated with improved response to therapy. Furthermore, most antineoplastic agents modulate TLS development, suggesting a role for these structures in the antitumor activity of these treatments. As such, TLSs have arisen as potential targets for the design of novel therapeutic approaches against cancer. However, TLSs may also be associated with poorer tumor progression and patient prognosis, so that the use of these structures as a therapeutic tool may have unintended deleterious effects. The factors involved in the differential role of TLSs are not fully elucidated, and include tumor type, TLS location within the tumor, specific cellular composition, and so on. The characterization of the specific mediators that regulate these characteristics is, therefore, a prerequisite for the optimization of treatments aimed at enhancing TLS-dependent anti-tumor immune response in an efficient and safe manner. Likewise, a careful selection of the target tumor type is necessary for the successful implementation of this strategy. The use of combinations of soluble factors that promote both TLS development and suitable cell composition, or the employment of selected immune cells modified to produce specific cytokines or chemokines, may contribute to the formation of TLSs with effective anti-tumor activity. In addition, the optimization of transporter systems that enable the delivery of mediators selectively to the tumor after systemic administration may facilitate their use in these patients, especially in barely accessible tumors and metastatic disease.
Author contributions
LE-P, NR-L, and AA had scientific discussion for this work and wrote the manuscript. All authors contributed to the article and approved the submitted version.
Funding
This work was supported by Fondo de Investigaciones Sanitarias from Instituto de Salud Carlos III co-funded by the “Fondo Europeo de Desarrollo Regional FEDER” grants PI22/01542 and CIBER Cardiovascular to AA, and by the CRIS Clinical Talent Programme 2022 from ‘Fundación CRIS contra el Cancer’, and grants from ISCIII (PI21/01111) and Mutua Madrileña (AP176822021) to NR-L.
Acknowledgments
The authors want to thank L Baron for editing of the manuscript. Figures have been created with BioRender.com.
Conflict of interest
The authors declare that the research was conducted in the absence of any commercial or financial relationships that could be construed as a potential conflict of interest.
Publisher’s note
All claims expressed in this article are solely those of the authors and do not necessarily represent those of their affiliated organizations, or those of the publisher, the editors and the reviewers. Any product that may be evaluated in this article, or claim that may be made by its manufacturer, is not guaranteed or endorsed by the publisher.
References
1. Anderson NM, Simon MC. The tumor microenvironment. Curr Biol CB (2020) 30:R921–5. doi: 10.1016/j.cub.2020.06.081
2. Sautès-Fridman C, Verneau J, Sun C-M, Moreira M, Chen TW-W, Meylan M, et al. Tertiary Lymphoid Structures and B cells: Clinical impact and therapeutic modulation in cancer. Semin Immunol (2020) 48:101406. doi: 10.1016/j.smim.2020.101406
3. Dieu-Nosjean M-C, Giraldo NA, Kaplon H, Germain C, Fridman WH, Sautès-Fridman C. Tertiary lymphoid structures, drivers of the anti-tumor responses in human cancers. Immunol Rev (2016) 271:260–75. doi: 10.1111/imr.12405
4. Sautès-Fridman C, Lawand M, Giraldo NA, Kaplon H, Germain C, Fridman WH, et al. Tertiary lymphoid structures in cancers: prognostic value, regulation, and manipulation for therapeutic intervention. Front Immunol (2016) 7:407. doi: 10.3389/fimmu.2016.00407
5. Helmink BA, Reddy SM, Gao J, Zhang S, Basar R, Thakur R, et al. B cells and tertiary lymphoid structures promote immunotherapy response. Nature (2020) 577:549–55. doi: 10.1038/s41586-019-1922-8
6. Cabrita R, Lauss M, Sanna A, Donia M, Skaarup Larsen M, Mitra S, et al. Tertiary lymphoid structures improve immunotherapy and survival in melanoma. Nature (2020) 577:561–5. doi: 10.1038/s41586-019-1914-8
7. Petitprez F, de Reyniès A, Keung EZ, Chen TW-W, Sun C-M, Calderaro J, et al. B cells are associated with survival and immunotherapy response in sarcoma. Nature (2020) 577:556–60. doi: 10.1038/s41586-019-1906-8
8. Germain C, Devi-Marulkar P, Knockaert S, Biton J, Kaplon H, Letaïef L, et al. Tertiary lymphoid structure-B cells narrow regulatory T cells impact in lung cancer patients. Front Immunol (2021) 12:626776. doi: 10.3389/fimmu.2021.626776
9. Fridman WH, Meylan M, Petitprez F, Sun C-M, Italiano A, Sautès-Fridman C. B cells and tertiary lymphoid structures as determinants of tumour immune contexture and clinical outcome. Nat Rev Clin Oncol (2022) 19:441–57. doi: 10.1038/s41571-022-00619-z
10. Neyt K, Perros F, GeurtsvanKessel CH, Hammad H, Lambrecht BN. Tertiary lymphoid organs in infection and autoimmunity. Trends Immunol (2012) 33:297–305. doi: 10.1016/j.it.2012.04.006
11. Manzo A, Bombardieri M, Humby F, Pitzalis C. Secondary and ectopic lymphoid tissue responses in rheumatoid arthritis: from inflammation to autoimmunity and tissue damage/remodeling. Immunol Rev (2010) 233:267–85. doi: 10.1111/j.0105-2896.2009.00861.x
12. Pipi E, Nayar S, Gardner DH, ColaFrancesco S, Smith C, Barone F. Tertiary lymphoid structures: autoimmunity goes local. Front Immunol (2018) 9:1952. doi: 10.3389/fimmu.2018.01952
13. Thaunat O, Patey N, Caligiuri G, Gautreau C, Mamani-Matsuda M, Mekki Y, et al. Chronic rejection triggers the development of an aggressive intragraft immune response through recapitulation of lymphoid organogenesis. J Immunol Baltim Md 1950 (2010) 185:717–28. doi: 10.4049/jimmunol.0903589
14. Colbeck EJ, Ager A, Gallimore A, Jones GW. Tertiary lymphoid structures in cancer: drivers of antitumor immunity, immunosuppression, or bystander sentinels in disease? Front Immunol (2017) 8:1830. doi: 10.3389/fimmu.2017.01830
15. Dieu-Nosjean M-C, Antoine M, Danel C, Heudes D, Wislez M, Poulot V, et al. Long-term survival for patients with non-small-cell lung cancer with intratumoral lymphoid structures. J Clin Oncol Off J Am Soc Clin Oncol (2008) 26:4410–7. doi: 10.1200/JCO.2007.15.0284
16. de Chaisemartin L, Goc J, Damotte D, Validire P, Magdeleinat P, Alifano M, et al. Characterization of chemokines and adhesion molecules associated with T cell presence in tertiary lymphoid structures in human lung cancer. Cancer Res (2011) 71:6391–9. doi: 10.1158/0008-5472.CAN-11-0952
17. Goc J, Germain C, Vo-Bourgais TKD, Lupo A, Klein C, Knockaert S, et al. Dendritic cells in tumor-associated tertiary lymphoid structures signal a Th1 cytotoxic immune contexture and license the positive prognostic value of infiltrating CD8+ T cells. Cancer Res (2014) 74:705–15. doi: 10.1158/0008-5472.CAN-13-1342
18. Bergomas F, Grizzi F, Doni A, Pesce S, Laghi L, Allavena P, et al. Tertiary intratumor lymphoid tissue in colo-rectal cancer. Cancers (2011) 4:1–10. doi: 10.3390/cancers4010001
19. Coppola D, Nebozhyn M, Khalil F, Dai H, Yeatman T, Loboda A, et al. Unique ectopic lymph node-like structures present in human primary colorectal carcinoma are identified by immune gene array profiling. Am J Pathol (2011) 179:37–45. doi: 10.1016/j.ajpath.2011.03.007
20. Sautès-Fridman C, Petitprez F, Calderaro J, Fridman WH. Tertiary lymphoid structures in the era of cancer immunotherapy. Nat Rev Cancer (2019) 19:307–25. doi: 10.1038/s41568-019-0144-6
21. Posch F, Silina K, Leibl S, Mündlein A, Moch H, Siebenhüner A, et al. Maturation of tertiary lymphoid structures and recurrence of stage II and III colorectal cancer. Oncoimmunology (2018) 7:e1378844. doi: 10.1080/2162402X.2017.1378844
22. Tang J, Ramis-Cabrer D, Curull V, Wang X, Mateu-Jiménez M, Pijuan L, et al. B cells and tertiary lymphoid structures influence survival in lung cancer patients with resectable tumors. Cancers (2020) 12:2644. doi: 10.3390/cancers12092644
23. Hill DG, Yu L, Gao H, Balic JJ, West A, Oshima H, et al. Hyperactive gp130/STAT3-driven gastric tumourigenesis promotes submucosal tertiary lymphoid structure development. Int J Cancer (2018) 143:167–78. doi: 10.1002/ijc.31298
24. Calderaro J, Petitprez F, Becht E, Laurent A, Hirsch TZ, Rousseau B, et al. Intra-tumoral tertiary lymphoid structures are associated with a low risk of early recurrence of hepatocellular carcinoma. J Hepatol (2019) 70:58–65. doi: 10.1016/j.jhep.2018.09.003
25. Messina JL, Fenstermacher DA, Eschrich S, Qu X, Berglund AE, Lloyd MC, et al. 12-Chemokine gene signature identifies lymph node-like structures in melanoma: potential for patient selection for immunotherapy? Sci Rep (2012) 2:765. doi: 10.1038/srep00765
26. Bery AI, Shepherd HM, Li W, Krupnick AS, Gelman AE, Kreisel D. Role of tertiary lymphoid organs in the regulation of immune responses in the periphery. Cell Mol Life Sci CMLS (2022) 79:359. doi: 10.1007/s00018-022-04388-x
27. Colvin KL, Cripe PJ, Ivy DD, Stenmark KR, Yeager ME. Bronchus-associated lymphoid tissue in pulmonary hypertension produces pathologic autoantibodies. Am J Respir Crit Care Med (2013) 188:1126–36. doi: 10.1164/rccm.201302-0403OC
28. Eddens T, Elsegeiny W, Garcia-Hernadez M de la L, Castillo P, Trevejo-Nunez G, Serody K, et al. Pneumocystis-driven inducible bronchus-associated lymphoid tissue formation requires th2 and Th17 immunity. Cell Rep (2017) 18:3078–90. doi: 10.1016/j.celrep.2017.03.016
29. Neyt K, GeurtsvanKessel CH, Deswarte K, Hammad H, Lambrecht BN. Early IL-1 Signaling Promotes iBALT Induction after Influenza Virus Infection. Front Immunol (2016) 7:312. doi: 10.3389/fimmu.2016.00312
30. Wiley JA, Richert LE, Swain SD, Harmsen A, Barnard DL, Randall TD, et al. Inducible bronchus-associated lymphoid tissue elicited by a protein cage nanoparticle enhances protection in mice against diverse respiratory viruses. PloS One (2009) 4:e7142. doi: 10.1371/journal.pone.0007142
31. Dominiak A, Chełstowska B, Olejarz W, Nowicka G. Communication in the cancer microenvironment as a target for therapeutic interventions. Cancers (2020) 12:1232. doi: 10.3390/cancers12051232
32. Schlößer HA, Thelen M, Lechner A, Wennhold K, Garcia-Marquez MA, Rothschild SI, et al. B cells in esophago-gastric adenocarcinoma are highly differentiated, organize in tertiary lymphoid structures and produce tumor-specific antibodies. Oncoimmunology (2019) 8:e1512458. doi: 10.1080/2162402X.2018.1512458
33. Cipponi A, Mercier M, Seremet T, Baurain J-F, Théate I, van den Oord J, et al. Neogenesis of lymphoid structures and antibody responses occur in human melanoma metastases. Cancer Res (2012) 72:3997–4007. doi: 10.1158/0008-5472.CAN-12-1377
34. Baratin M, Simon L, Jorquera A, Ghigo C, Dembele D, Nowak J, et al. T cell zone resident macrophages silently dispose of apoptotic cells in the lymph node. Immunity (2017) 47:349–362.e5. doi: 10.1016/j.immuni.2017.07.019
35. Martinet L, Garrido I, Girard J-P. Tumor high endothelial venules (HEVs) predict lymphocyte infiltration and favorable prognosis in breast cancer. Oncoimmunology (2012) 1:789–90. doi: 10.4161/onci.19787
36. Garaud S, Buisseret L, Solinas C, Gu-Trantien C, de Wind A, Van den Eynden G, et al. Tumor infiltrating B-cells signal functional humoral immune responses in breast cancer. JCI Insight (2019) 5:e129641. doi: 10.1172/jci.insight.129641
37. Nielsen JS, Sahota RA, Milne K, Kost SE, Nesslinger NJ, Watson PH, et al. CD20+ tumor-infiltrating lymphocytes have an atypical CD27- memory phenotype and together with CD8+ T cells promote favorable prognosis in ovarian cancer. Clin Cancer Res Off J Am Assoc Cancer Res (2012) 18:3281–92. doi: 10.1158/1078-0432.CCR-12-0234
38. Chen L, Oke T, Siegel N, Cojocaru G, Tam AJ, Blosser RL, et al. The immunosuppressive niche of soft-tissue sarcomas is sustained by tumor-associated macrophages and characterized by intratumoral tertiary lymphoid structures. Clin Cancer Res Off J Am Assoc Cancer Res (2020) 26:4018–30. doi: 10.1158/1078-0432.CCR-19-3416
39. Ladányi A, Kiss J, Somlai B, Gilde K, Fejos Z, Mohos A, et al. Density of DC-LAMP(+) mature dendritic cells in combination with activated T lymphocytes infiltrating primary cutaneous melanoma is a strong independent prognostic factor. Cancer Immunol Immunother CII (2007) 56:1459–69. doi: 10.1007/s00262-007-0286-3
40. Wirsing AM, Ervik IK, Seppola M, Uhlin-Hansen L, Steigen SE, Hadler-Olsen E. Presence of high-endothelial venules correlates with a favorable immune microenvironment in oral squamous cell carcinoma. Mod Pathol Off J U S Can Acad Pathol Inc (2018) 31:910–22. doi: 10.1038/s41379-018-0019-5
41. Finkin S, Yuan D, Stein I, Taniguchi K, Weber A, Unger K, et al. Ectopic lymphoid structures function as microniches for tumor progenitor cells in hepatocellular carcinoma. Nat Immunol (2015) 16:1235–44. doi: 10.1038/ni.3290
42. Hiraoka N, Ino Y, Yamazaki-Itoh R, Kanai Y, Kosuge T, Shimada K. Intratumoral tertiary lymphoid organ is a favourable prognosticator in patients with pancreatic cancer. Br J Cancer (2015) 112:1782–90. doi: 10.1038/bjc.2015.145
43. Meylan M, Petitprez F, Becht E, Bougoüin A, Pupier G, Calvez A, et al. Tertiary lymphoid structures generate and propagate anti-tumor antibody-producing plasma cells in renal cell cancer. Immunity (2022) 55:527–541.e5. doi: 10.1016/j.immuni.2022.02.001
44. Laumont CM, Nelson BH. B cells in the tumor microenvironment: Multi-faceted organizers, regulators, and effectors of anti-tumor immunity. Cancer Cell (2023) 41:466–89. doi: 10.1016/j.ccell.2023.02.017
45. Cupedo T, Kraal G, Mebius RE. The role of CD45+CD4+CD3- cells in lymphoid organ development. Immunol Rev (2002) 189:41–50. doi: 10.1034/j.1600-065x.2002.18905.x
46. van de Pavert SA. Lymphoid Tissue inducer (LTi) cell ontogeny and functioning in embryo and adult. BioMed J (2021) 44:123–32. doi: 10.1016/j.bj.2020.12.003
47. Schumacher TN, Thommen DS. Tertiary lymphoid structures in cancer. Science (2022) 375:eabf9419. doi: 10.1126/science.abf9419
48. Luther SA, Bidgol A, Hargreaves DC, Schmidt A, Xu Y, Paniyadi J, et al. Differing activities of homeostatic chemokines CCL19, CCL21, and CXCL12 in lymphocyte and dendritic cell recruitment and lymphoid neogenesis. J Immunol Baltim Md 1950 (2002) 169:424–33. doi: 10.4049/jimmunol.169.1.424
49. Luther SA, Ansel KM, Cyster JG. Overlapping roles of CXCL13, interleukin 7 receptor alpha, and CCR7 ligands in lymph node development. J Exp Med (2003) 197:1191–8. doi: 10.1084/jem.20021294
50. De Togni P, Goellner J, Ruddle NH, Streeter PR, Fick A, Mariathasan S, et al. Abnormal development of peripheral lymphoid organs in mice deficient in lymphotoxin. Science (1994) 264:703–7. doi: 10.1126/science.8171322
51. Peters A, Pitcher LA, Sullivan JM, Mitsdoerffer M, Acton SE, Franz B, et al. Th17 cells induce ectopic lymphoid follicles in central nervous system tissue inflammation. Immunity (2011) 35:986–96. doi: 10.1016/j.immuni.2011.10.015
52. Guedj K, Khallou-Laschet J, Clement M, Morvan M, Gaston A-T, Fornasa G, et al. M1 macrophages act as LTβR-independent lymphoid tissue inducer cells during atherosclerosis-related lymphoid neogenesis. Cardiovasc Res (2014) 101:434–43. doi: 10.1093/cvr/cvt263
53. Mebius RE. Organogenesis of lymphoid tissues. Nat Rev Immunol (2003) 3:292–303. doi: 10.1038/nri1054
54. Takatori H, Kanno Y, Watford WT, Tato CM, Weiss G, Ivanov II, et al. Lymphoid tissue inducer-like cells are an innate source of IL-17 and IL-22. J Exp Med (2009) 206:35–41. doi: 10.1084/jem.20072713
55. Barone F, Gardner DH, Nayar S, Steinthal N, Buckley CD, Luther SA. Stromal fibroblasts in tertiary lymphoid structures: A novel target in chronic inflammation. Front Immunol (2016) 7:477. doi: 10.3389/fimmu.2016.00477
56. Wang B, Liu J, Han Y, Deng Y, Li J, Jiang Y. The presence of tertiary lymphoid structures provides new insight into the clinicopathological features and prognosis of patients with breast cancer. Front Immunol (2022) 13:868155. doi: 10.3389/fimmu.2022.868155
57. Siliņa K, Soltermann A, Attar FM, Casanova R, Uckeley ZM, Thut H, et al. Germinal centers determine the prognostic relevance of tertiary lymphoid structures and are impaired by corticosteroids in lung squamous cell carcinoma. Cancer Res (2018) 78:1308–20. doi: 10.1158/0008-5472.CAN-17-1987
58. Curiel TJ, Coukos G, Zou L, Alvarez X, Cheng P, Mottram P, et al. Specific recruitment of regulatory T cells in ovarian carcinoma fosters immune privilege and predicts reduced survival. Nat Med (2004) 10:942–9. doi: 10.1038/nm1093
59. Lin Q, Tao P, Wang J, Ma L, Jiang Q, Li J, et al. Tumor-associated tertiary lymphoid structure predicts postoperative outcomes in patients with primary gastrointestinal stromal tumors. Oncoimmunology (2020) 9:1747339. doi: 10.1080/2162402X.2020.1747339
60. Schrama D, thor Straten P, Fischer WH, McLellan AD, Bröcker EB, Reisfeld RA, et al. Targeting of lymphotoxin-alpha to the tumor elicits an efficient immune response associated with induction of peripheral lymphoid-like tissue. Immunity (2001) 14:111–21. doi: 10.1016/s1074-7613(01)00094-2
61. Asam S, Nayar S, Gardner D, Barone F. Stromal cells in tertiary lymphoid structures: Architects of autoimmunity. Immunol Rev (2021) 302:184–95. doi: 10.1111/imr.12987
62. McMullen TPW, Lai R, Dabbagh L, Wallace TM, de Gara CJ. Survival in rectal cancer is predicted by T cell infiltration of tumour-associated lymphoid nodules. Clin Exp Immunol (2010) 161:81–8. doi: 10.1111/j.1365-2249.2010.04147.x
63. Gao Q, Qiu S-J, Fan J, Zhou J, Wang X-Y, Xiao Y-S, et al. Intratumoral balance of regulatory and cytotoxic T cells is associated with prognosis of hepatocellular carcinoma after resection. J Clin Oncol Off J Am Soc Clin Oncol (2007) 25:2586–93. doi: 10.1200/JCO.2006.09.4565
64. Bates GJ, Fox SB, Han C, Leek RD, Garcia JF, Harris AL, et al. Quantification of regulatory T cells enables the identification of high-risk breast cancer patients and those at risk of late relapse. J Clin Oncol Off J Am Soc Clin Oncol (2006) 24:5373–80. doi: 10.1200/JCO.2006.05.9584
65. Joshi NS, Akama-Garren EH, Lu Y, Lee D-Y, Chang GP, Li A, et al. Regulatory T cells in tumor-associated tertiary lymphoid structures suppress anti-tumor T cell responses. Immunity (2015) 43:579–90. doi: 10.1016/j.immuni.2015.08.006
66. Ding G-Y, Ma J-Q, Yun J-P, Chen X, Ling Y, Zhang S, et al. Distribution and density of tertiary lymphoid structures predict clinical outcome in intrahepatic cholangiocarcinoma. J Hepatol (2022) 76:608–18. doi: 10.1016/j.jhep.2021.10.030
67. Werner F, Wagner C, Simon M, Glatz K, Mertz KD, Läubli H, et al. A standardized analysis of tertiary lymphoid structures in human melanoma: disease progression- and tumor site-associated changes with germinal center alteration. Front Immunol (2021) 12:675146. doi: 10.3389/fimmu.2021.675146
68. Bento DC, Jones E, Junaid S, Tull J, Williams GT, Godkin A, et al. High endothelial venules are rare in colorectal cancers but accumulate in extra-tumoral areas with disease progression. Oncoimmunology (2015) 4:e974374. doi: 10.4161/2162402X.2014.974374
69. Li H, Liu H, Fu H, Li J, Xu L, Wang G, et al. Peritumoral tertiary lymphoid structures correlate with protective immunity and improved prognosis in patients with hepatocellular carcinoma. Front Immunol (2021) 12:648812. doi: 10.3389/fimmu.2021.648812
70. Weiner AB, Vidotto T, Liu Y, Mendes AA, Salles DC, Faisal FA, et al. Plasma cells are enriched in localized prostate cancer in Black men and are associated with improved outcomes. Nat Commun (2021) 12:935. doi: 10.1038/s41467-021-21245-w
71. Germain C, Gnjatic S, Tamzalit F, Knockaert S, Remark R, Goc J, et al. Presence of B cells in tertiary lymphoid structures is associated with a protective immunity in patients with lung cancer. Am J Respir Crit Care Med (2014) 189:832–44. doi: 10.1164/rccm.201309-1611OC
72. Hu Q, Hong Y, Qi P, Lu G, Mai X, Xu S, et al. Atlas of breast cancer infiltrated B-lymphocytes revealed by paired single-cell RNA-sequencing and antigen receptor profiling. Nat Commun (2021) 12:2186. doi: 10.1038/s41467-021-22300-2
73. Lee-Chang C, Bodogai M, Martin-Montalvo A, Wejksza K, Sanghvi M, Moaddel R, et al. Inhibition of breast cancer metastasis by resveratrol-mediated inactivation of tumor-evoked regulatory B cells. J Immunol Baltim Md 1950 (2013) 191:4141–51. doi: 10.4049/jimmunol.1300606
74. Roya N, Fatemeh T, Faramarz M-A, Milad S-G, Mohammad-Javad S, Najmeh S-V, et al. Frequency of IL-10+CD19+ B cells in patients with prostate cancer compared to patients with benign prostatic hyperplasia. Afr Health Sci (2020) 20:1264–72. doi: 10.4314/ahs.v20i3.31
75. Wang WW, Yuan XL, Chen H, Xie GH, Ma YH, Zheng YX, et al. CD19+CD24hiCD38hiBregs involved in downregulate helper T cells and upregulate regulatory T cells in gastric cancer. Oncotarget (2015) 6:33486–99. doi: 10.18632/oncotarget.5588
76. Jiang J, Pan W, Xu Y, Ni C, Xue D, Chen Z, et al. Tumour-infiltrating immune cell-based subtyping and signature gene analysis in breast cancer based on gene expression profiles. J Cancer (2020) 11:1568–83. doi: 10.7150/jca.37637
77. Chen J, Tan Y, Sun F, Hou L, Zhang C, Ge T, et al. Single-cell transcriptome and antigen-immunoglobin analysis reveals the diversity of B cells in non-small cell lung cancer. Genome Biol (2020) 21:152. doi: 10.1186/s13059-020-02064-6
78. Griss J, Bauer W, Wagner C, Simon M, Chen M, Grabmeier-Pfistershammer K, et al. B cells sustain inflammation and predict response to immune checkpoint blockade in human melanoma. Nat Commun (2019) 10:4186. doi: 10.1038/s41467-019-12160-2
79. DiLillo DJ, Yanaba K, Tedder TF. B cells are required for optimal CD4+ and CD8+ T cell tumor immunity: therapeutic B cell depletion enhances B16 melanoma growth in mice. J Immunol Baltim Md 1950 (2010) 184:4006–16. doi: 10.4049/jimmunol.0903009
80. DeNardo DG, Andreu P, Coussens LM. Interactions between lymphocytes and myeloid cells regulate pro- versus anti-tumor immunity. Cancer Metastasis Rev (2010) 29:309–16. doi: 10.1007/s10555-010-9223-6
81. Fridman WH, Petitprez F, Meylan M, Chen TW-W, Sun C-M, Roumenina LT, et al. B cells and cancer: To B or not to B? J Exp Med (2021) 218:e20200851. doi: 10.1084/jem.20200851
82. Qin Z, Richter G, Schüler T, Ibe S, Cao X, Blankenstein T. B cells inhibit induction of T cell-dependent tumor immunity. Nat Med (1998) 4:627–30. doi: 10.1038/nm0598-627
83. Barbera-Guillem E, Nelson MB, Barr B, Nyhus JK, May KF, Feng L, et al. B lymphocyte pathology in human colorectal cancer. Experimental and clinical therapeutic effects of partial B cell depletion. Cancer Immunol Immunother CII (2000) 48:541–9. doi: 10.1007/pl00006672
84. Wei X, Jin Y, Tian Y, Zhang H, Wu J, Lu W, et al. Regulatory B cells contribute to the impaired antitumor immunity in ovarian cancer patients. Tumour Biol J Int Soc Oncodevelopmental Biol Med (2016) 37:6581–8. doi: 10.1007/s13277-015-4538-0
85. Ishigami E, Sakakibara M, Sakakibara J, Masuda T, Fujimoto H, Hayama S, et al. Coexistence of regulatory B cells and regulatory T cells in tumor-infiltrating lymphocyte aggregates is a prognostic factor in patients with breast cancer. Breast Cancer Tokyo Jpn (2019) 26:180–9. doi: 10.1007/s12282-018-0910-4
86. Olkhanud PB, Damdinsuren B, Bodogai M, Gress RE, Sen R, Wejksza K, et al. Tumor-evoked regulatory B cells promote breast cancer metastasis by converting resting CD4+ T cells to T-regulatory cells. Cancer Res (2011) 71:3505–15. doi: 10.1158/0008-5472.CAN-10-4316
87. Mantovani A, Sozzani S, Locati M, Allavena P, Sica A. Macrophage polarization: tumor-associated macrophages as a paradigm for polarized M2 mononuclear phagocytes. Trends Immunol (2002) 23:549–55. doi: 10.1016/s1471-4906(02)02302-5
88. Carmeliet P. VEGF as a key mediator of angiogenesis in cancer. Oncology (2005) 69 Suppl 3:4–10. doi: 10.1159/000088478
89. Ladányi A, Kiss J, Mohos A, Somlai B, Liszkay G, Gilde K, et al. Prognostic impact of B-cell density in cutaneous melanoma. Cancer Immunol Immunother CII (2011) 60:1729–38. doi: 10.1007/s00262-011-1071-x
90. Garnelo M, Tan A, Her Z, Yeong J, Lim CJ, Chen J, et al. Interaction between tumour-infiltrating B cells and T cells controls the progression of hepatocellular carcinoma. Gut (2017) 66:342–51. doi: 10.1136/gutjnl-2015-310814
91. Montfort A, Pearce O, Maniati E, Vincent BG, Bixby L, Böhm S, et al. A strong B-cell response is part of the immune landscape in human high-grade serous ovarian metastases. Clin Cancer Res Off J Am Assoc Cancer Res (2017) 23:250–62. doi: 10.1158/1078-0432.CCR-16-0081
92. Biswas S, Mandal G, Payne KK, Anadon CM, Gatenbee CD, Chaurio RA, et al. IgA transcytosis and antigen recognition govern ovarian cancer immunity. Nature (2021) 591:464–70. doi: 10.1038/s41586-020-03144-0
93. Mazor RD, Nathan N, Gilboa A, Stoler-Barak L, Moss L, Solomonov I, et al. Tumor-reactive antibodies evolve from non-binding and autoreactive precursors. Cell (2022) 185:1208–1222.e21. doi: 10.1016/j.cell.2022.02.012
94. Garaud S, Zayakin P, Buisseret L, Rulle U, Silina K, de Wind A, et al. Antigen specificity and clinical significance of igG and igA autoantibodies produced in situ by tumor-infiltrating B cells in breast cancer. Front Immunol (2018) 9:2660. doi: 10.3389/fimmu.2018.02660
95. Shalapour S, Lin X-J, Bastian IN, Brain J, Burt AD, Aksenov AA, et al. Inflammation-induced IgA+ cells dismantle anti-liver cancer immunity. Nature (2017) 551:340–5. doi: 10.1038/nature24302
96. Karagiannis P, Gilbert AE, Josephs DH, Ali N, Dodev T, Saul L, et al. IgG4 subclass antibodies impair antitumor immunity in melanoma. J Clin Invest (2013) 123:1457–74. doi: 10.1172/JCI65579
97. Fujimoto M, Yoshizawa A, Sumiyoshi S, Sonobe M, Kobayashi M, Koyanagi I, et al. Stromal plasma cells expressing immunoglobulin G4 subclass in non-small cell lung cancer. Hum Pathol (2013) 44:1569–76. doi: 10.1016/j.humpath.2013.01.002
98. Roumenina LT, Daugan MV, Noé R, Petitprez F, Vano YA, Sanchez-Salas R, et al. Tumor cells hijack macrophage-produced complement C1q to promote tumor growth. Cancer Immunol Res (2019) 7:1091–105. doi: 10.1158/2326-6066.CIR-18-0891
99. Bulla R, Tripodo C, Rami D, Ling GS, Agostinis C, Guarnotta C, et al. C1q acts in the tumour microenvironment as a cancer-promoting factor independently of complement activation. Nat Commun (2016) 7:10346. doi: 10.1038/ncomms10346
100. Charab W, Rosenberger MG, Shivram H, Mirazee JM, Donkor M, Shekhar SR, et al. IgG immune complexes inhibit naïve T cell proliferation and suppress effector function in cytotoxic T cells. Front Immunol (2021) 12:713704. doi: 10.3389/fimmu.2021.713704
101. Boivin G, Kalambaden P, Faget J, Rusakiewicz S, Montay-Gruel P, Meylan E, et al. Cellular composition and contribution of tertiary lymphoid structures to tumor immune infiltration and modulation by radiation therapy. Front Oncol (2018) 8:256. doi: 10.3389/fonc.2018.00256
102. van Dijk N, Gil-Jimenez A, Silina K, Hendricksen K, Smit LA, de Feijter JM, et al. Preoperative ipilimumab plus nivolumab in locoregionally advanced urothelial cancer: the NABUCCO trial. Nat Med (2020) 26:1839–44. doi: 10.1038/s41591-020-1085-z
103. Noël G, Fontsa ML, Garaud S, De Silva P, de Wind A, Van den Eynden GG, et al. Functional Th1-oriented T follicular helper cells that infiltrate human breast cancer promote effective adaptive immunity. J Clin Invest (2021) 131:e139905. doi: 10.1172/JCI139905
104. Li JJ, Tsang JY, Tse GM. Tumor microenvironment in breast cancer-updates on therapeutic implications and pathologic assessment. Cancers (2021) 13:4233. doi: 10.3390/cancers13164233
105. Gu-Trantien C, Loi S, Garaud S, Equeter C, Libin M, de Wind A, et al. CD4+ follicular helper T cell infiltration predicts breast cancer survival. J Clin Invest (2013) 123:2873–92. doi: 10.1172/JCI67428
106. Lu Y, Zhao Q, Liao J-Y, Song E, Xia Q, Pan J, et al. Complement signals determine opposite effects of B cells in chemotherapy-induced immunity. Cell (2020) 180:1081–1097.e24. doi: 10.1016/j.cell.2020.02.015
107. Aparicio Gallego G, Díaz Prado S, Jiménez Fonseca P, García Campelo R, Cassinello Espinosa J, Antón Aparicio LM. Cyclooxygenase-2 (COX-2): a molecular target in prostate cancer. Clin Transl Oncol Off Publ Fed Span Oncol Soc Natl Cancer Inst Mex (2007) 9:694–702. doi: 10.1007/s12094-007-0126-0
108. Zelenay S, van der Veen AG, Böttcher JP, Snelgrove KJ, Rogers N, Acton SE, et al. Cyclooxygenase-dependent tumor growth through evasion of immunity. Cell (2015) 162:1257–70. doi: 10.1016/j.cell.2015.08.015
109. Zelenay S, Reis e Sousa C. Reducing prostaglandin E2 production to raise cancer immunogenicity. Oncoimmunology (2016) 5:e1123370. doi: 10.1080/2162402X.2015.1123370
110. García-Hernández M de la L, Uribe-Uribe NO, Espinosa-González R, Kast WM, Khader SA, Rangel-Moreno J. A unique cellular and molecular microenvironment is present in tertiary lymphoid organs of patients with spontaneous prostate cancer regression. Front Immunol (2017) 8:563. doi: 10.3389/fimmu.2017.00563
111. Kumar S, Calianese D, Birge RB. Efferocytosis of dying cells differentially modulate immunological outcomes in tumor microenvironment. Immunol Rev (2017) 280:149–64. doi: 10.1111/imr.12587
112. Green DR, Ferguson T, Zitvogel L, Kroemer G. Immunogenic and tolerogenic cell death. Nat Rev Immunol (2009) 9:353–63. doi: 10.1038/nri2545
113. Allen E, Jabouille A, Rivera LB, Lodewijckx I, Missiaen R, Steri V, et al. Combined antiangiogenic and anti-PD-L1 therapy stimulates tumor immunity through HEV formation. Sci Transl Med (2017) 9:eaak9679. doi: 10.1126/scitranslmed.aak9679
114. Cottrell TR, Thompson ED, Forde PM, Stein JE, Duffield AS, Anagnostou V, et al. Pathologic features of response to neoadjuvant anti-PD-1 in resected non-small-cell lung carcinoma: a proposal for quantitative immune-related pathologic response criteria (irPRC). Ann Oncol Off J Eur Soc Med Oncol (2018) 29:1853–60. doi: 10.1093/annonc/mdy218
115. Vanhersecke L, Brunet M, Guégan J-P, Rey C, Bougouin A, Cousin S, et al. Mature tertiary lymphoid structures predict immune checkpoint inhibitor efficacy in solid tumors independently of PD-L1 expression. Nat Cancer (2021) 2:794–802. doi: 10.1038/s43018-021-00232-6
116. Gao J, Navai N, Alhalabi O, Siefker-Radtke A, Campbell MT, Tidwell RS, et al. Neoadjuvant PD-L1 plus CTLA-4 blockade in patients with cisplatin-ineligible operable high-risk urothelial carcinoma. Nat Med (2020) 26:1845–51. doi: 10.1038/s41591-020-1086-y
117. Italiano A, Bessede A, Pulido M, Bompas E, Piperno-Neumann S, Chevreau C, et al. Pembrolizumab in soft-tissue sarcomas with tertiary lymphoid structures: a phase 2 PEMBROSARC trial cohort. Nat Med (2022) 28:1199–206. doi: 10.1038/s41591-022-01821-3
118. Cindy Yang SY, Lien SC, Wang BX, Clouthier DL, Hanna Y, Cirlan I, et al. Pan-cancer analysis of longitudinal metastatic tumors reveals genomic alterations and immune landscape dynamics associated with pembrolizumab sensitivity. Nat Commun (2021) 12:5137. doi: 10.1038/s41467-021-25432-7
119. Hui Z, Zhang J, Ren Y, Li X, Yan C, Yu W, et al. Single-cell profiling of immune cells after neoadjuvant pembrolizumab and chemotherapy in IIIA non-small cell lung cancer (NSCLC). Cell Death Dis (2022) 13:607. doi: 10.1038/s41419-022-05057-4
120. Cui C, Wang J, Fagerberg E, Chen P-M, Connolly KA, Damo M, et al. Neoantigen-driven B cell and CD4 T follicular helper cell collaboration promotes anti-tumor CD8 T cell responses. Cell (2021) 184:6101–6118.e13. doi: 10.1016/j.cell.2021.11.007
121. Hollern DP, Xu N, Thennavan A, Glodowski C, Garcia-Recio S, Mott KR, et al. B cells and T follicular helper cells mediate response to checkpoint inhibitors in high mutation burden mouse models of breast cancer. Cell (2019) 179:1191–1206.e21. doi: 10.1016/j.cell.2019.10.028
122. Sánchez-Alonso S, Setti-Jerez G, Arroyo M, Hernández T, Martos MI, Sánchez-Torres JM, et al. A new role for circulating T follicular helper cells in humoral response to anti-PD-1 therapy. J Immunother Cancer (2020) 8:e001187. doi: 10.1136/jitc-2020-001187
123. Lutz ER, Wu AA, Bigelow E, Sharma R, Mo G, Soares K, et al. Immunotherapy converts nonimmunogenic pancreatic tumors into immunogenic foci of immune regulation. Cancer Immunol Res (2014) 2:616–31. doi: 10.1158/2326-6066.CIR-14-0027
124. Maldonado L, Teague JE, Morrow MP, Jotova I, Wu TC, Wang C, et al. Intramuscular therapeutic vaccination targeting HPV16 induces T cell responses that localize in mucosal lesions. Sci Transl Med (2014) 6:221ra13. doi: 10.1126/scitranslmed.3007323
125. Johansson-Percival A, He B, Li Z-J, Kjellén A, Russell K, Li J, et al. De novo induction of intratumoral lymphoid structures and vessel norMalization enhances immunotherapy in resistant tumors. Nat Immunol (2017) 18:1207–17. doi: 10.1038/ni.3836
126. Hjelmström P, Fjell J, Nakagawa T, Sacca R, Cuff CA, Ruddle NH. Lymphoid tissue homing chemokines are expressed in chronic inflammation. Am J Pathol (2000) 156:1133–8. doi: 10.1016/S0002-9440(10)64981-4
127. Carragher DM, Rangel-Moreno J, Randall TD. Ectopic lymphoid tissues and local immunity. Semin Immunol (2008) 20:26–42. doi: 10.1016/j.smim.2007.12.004
128. Sharma N, Vacher J, Allison JP. TLR1/2 ligand enhances antitumor efficacy of CTLA-4 blockade by increasing intratumoral Treg depletion. Proc Natl Acad Sci U S A. (2019) 116:10453–62. doi: 10.1073/pnas.1819004116
129. Stier S, Maletzki C, Klier U, Linnebacher M. Combinations of TLR ligands: a promising approach in cancer immunotherapy. Clin Dev Immunol (2013) 2013:271246. doi: 10.1155/2013/271246
130. Robinet M, Villeret B, Maillard S, Cron MA, Berrih-Aknin S, Le Panse R. Use of toll-like receptor agonists to induce ectopic lymphoid structures in myasthenia gravis mouse models. Front Immunol (2017) 8:1029. doi: 10.3389/fimmu.2017.01029
131. Wang J, Lo JC, Foster A, Yu P, Chen HM, Wang Y, et al. The regulation of T cell homeostasis and autoimmunity by T cell-derived LIGHT. J Clin Invest (2001) 108:1771–80. doi: 10.1172/JCI13827
132. Rooney IA, Butrovich KD, Glass AA, Borboroglu S, Benedict CA, Whitbeck JC, et al. The lymphotoxin-beta receptor is necessary and sufficient for LIGHT-mediated apoptosis of tumor cells. J Biol Chem (2000) 275:14307–15. doi: 10.1074/jbc.275.19.14307
133. Ware CF. Targeting lymphocyte activation through the lymphotoxin and LIGHT pathways. Immunol Rev (2008) 223:186–201. doi: 10.1111/j.1600-065X.2008.00629.x
134. Skeate JG, Otsmaa ME, Prins R, Fernandez DJ, Da Silva DM, Kast WM. TNFSF14: LIGHTing the way for effective cancer immunotherapy. Front Immunol (2020) 11:922. doi: 10.3389/fimmu.2020.00922
135. Tang H, Wang Y, Chlewicki LK, Zhang Y, Guo J, Liang W, et al. Facilitating T cell infiltration in tumor microenvironment overcomes resistance to PD-L1 blockade. Cancer Cell (2016) 29:285–96. doi: 10.1016/j.ccell.2016.02.004
136. He B, Jabouille A, Steri V, Johansson-Percival A, Michael IP, Kotamraju VR, et al. Vascular targeting of LIGHT normalizes blood vessels in primary brain cancer and induces intratumoural high endothelial venules. J Pathol (2018) 245:209–21. doi: 10.1002/path.5080
137. He B, Johansson-Percival A, Backhouse J, Li J, Lee GYF, Hamzah J, et al. Remodeling of metastatic vasculature reduces lung colonization and sensitizes overt metastases to immunotherapy. Cell Rep (2020) 30:714–724.e5. doi: 10.1016/j.celrep.2019.12.013
138. Suematsu S, Watanabe T. Generation of a synthetic lymphoid tissue-like organoid in mice. Nat Biotechnol (2004) 22:1539–45. doi: 10.1038/nbt1039
139. Okamoto N, Chihara R, Shimizu C, Nishimoto S, Watanabe T. Artificial lymph nodes induce potent secondary immune responses in naive and immunodeficient mice. J Clin Invest (2007) 117:997–1007. doi: 10.1172/JCI30379
140. Kobayashi Y, Watanabe T. Gel-trapped lymphorganogenic chemokines trigger artificial tertiary lymphoid organs and mount adaptive immune responses. In Vivo. Front Immunol (2016) 7:316. doi: 10.3389/fimmu.2016.00316
141. Bashir S, Hina M, Iqbal J, Rajpar AH, Mujtaba MA, Alghamdi NA, et al. Fundamental concepts of hydrogels: synthesis, properties, and their applications. Polymers (2020) 12:2702. doi: 10.3390/polym12112702
142. Purwada A, Singh A. Immuno-engineered organoids for regulating the kinetics of B-cell development and antibody production. Nat Protoc (2017) 12:168–82. doi: 10.1038/nprot.2016.157
143. Ishii S, Kaneko J, Nagasaki Y. Development of a long-acting, protein-loaded, redox-active, injectable gel formed by a polyion complex for local protein therapeutics. Biomaterials (2016) 84:210–8. doi: 10.1016/j.biomaterials.2016.01.029
144. Li H, Li Y, Wang X, Hou Y, Hong X, Gong T, et al. Rational design of polymeric hybrid micelles to overcome lymphatic and intracellular delivery barriers in cancer immunotherapy. Theranostics (2017) 7:4383–98. doi: 10.7150/thno.20745
145. Liu L, Yi H, Wang C, He H, Li P, Pan H, et al. Integrated nanovaccine with microRNA-148a inhibition reprograms tumor-associated dendritic cells by modulating miR-148a/DNMT1/SOCS1 axis. J Immunol Baltim Md 1950 (2016) 197:1231–41. doi: 10.4049/jimmunol.1600182
146. Chen L, Taylor JL, Sabins NC, Lowe DB, Qu Y, You Z, et al. Extranodal induction of therapeutic immunity in the tumor microenvironment after intratumoral delivery of Tbet gene-modified dendritic cells. Cancer Gene Ther (2013) 20:469–77. doi: 10.1038/cgt.2013.42
147. Weinstein AM, Chen L, Brzana EA, Patil PR, Taylor JL, Fabian KL, et al. Tbet and IL-36γ cooperate in therapeutic DC-mediated promotion of ectopic lymphoid organogenesis in the tumor microenvironment. Oncoimmunology (2017) 6:e1322238. doi: 10.1080/2162402X.2017.1322238
148. Bridgewood C, Stacey M, Alase A, Lagos D, Graham A, Wittmann M. IL-36γ has proinflammatory effects on human endothelial cells. Exp Dermatol (2017) 26:402–8. doi: 10.1111/exd.13228
149. Weinstein AM, Giraldo NA, Petitprez F, Julie C, Lacroix L, Peschaud F, et al. Association of IL-36γ with tertiary lymphoid structures and inflammatory immune infiltrates in human colorectal cancer. Cancer Immunol Immunother CII (2019) 68:109–20. doi: 10.1007/s00262-018-2259-0
150. Kirk CJ, Hartigan-O’Connor D, Mulé JJ. The dynamics of the T-cell antitumor response: chemokine-secreting dendritic cells can prime tumor-reactive T cells extranodally. Cancer Res (2001) 61:8794–802.
151. Moussion C, Girard J-P. Dendritic cells control lymphocyte entry to lymph nodes through high endothelial venules. Nature (2011) 479:542–6. doi: 10.1038/nature10540
152. GeurtsvanKessel CH, Willart MAM, Bergen IM, van Rijt LS, Muskens F, Elewaut D, et al. Dendritic cells are crucial for maintenance of tertiary lymphoid structures in the lung of influenza virus-infected mice. J Exp Med (2009) 206:2339–49. doi: 10.1084/jem.20090410
153. Lee JM, Lee M-H, Garon E, Goldman JW, Salehi-Rad R, Baratelli FE, et al. Phase I trial of intratumoral injection of CCL21 gene-modified dendritic cells in lung cancer elicits tumor-specific immune responses and CD8+ T-cell infiltration. Clin Cancer Res Off J Am Assoc Cancer Res (2017) 23:4556–68. doi: 10.1158/1078-0432.CCR-16-2821
154. Yang S-C, Batra RK, Hillinger S, Reckamp KL, Strieter RM, Dubinett SM, et al. Intrapulmonary administration of CCL21 gene-modified dendritic cells reduces tumor burden in spontaneous murine bronchoalveolar cell carcinoma. Cancer Res (2006) 66:3205–13. doi: 10.1158/0008-5472.CAN-05-3619
155. Jonsson Comprehensive Cancer Center. A phase I trial of intratumoral administration of CCL21-gene modified dendritic cell (Ad-CCL21-DC) combined with intravenous pembrolizumab for advanced NSCLC (2022). Available at: https://clinicaltrials.gov/ct2/show/NCT03546361 (Accessed May 21, 2023).
156. VA Office of Research and Development. Intratumoral genetic therapy for lung cancer (2018). Available at: https://clinicaltrials.gov/ct2/show/NCT01574222 (Accessed May 21, 2023).
157. Dorraji SE, Hovd A-MK, Kanapathippillai P, Bakland G, Eilertsen GØ, Figenschau SL, et al. Mesenchymal stem cells and T cells in the formation of Tertiary Lymphoid Structures in Lupus Nephritis. Sci Rep (2018) 8:7861. doi: 10.1038/s41598-018-26265-z
Keywords: tertiary lymphoid structures, B cells, adaptive anti-tumor response, TLS modulation, immunotherapy
Citation: Esparcia-Pinedo L, Romero-Laorden N and Alfranca A (2023) Tertiary lymphoid structures and B lymphocytes: a promising therapeutic strategy to fight cancer. Front. Immunol. 14:1231315. doi: 10.3389/fimmu.2023.1231315
Received: 30 May 2023; Accepted: 24 July 2023;
Published: 09 August 2023.
Edited by:
Juan M. Zapata, Consejo Superior de Investigaciones Científicas (CSIC), SpainReviewed by:
J. David Peske, Johns Hopkins Medicine, United StatesHélène Kaplon, Institut de Recherche International Servier, France
Copyright © 2023 Esparcia-Pinedo, Romero-Laorden and Alfranca. This is an open-access article distributed under the terms of the Creative Commons Attribution License (CC BY). The use, distribution or reproduction in other forums is permitted, provided the original author(s) and the copyright owner(s) are credited and that the original publication in this journal is cited, in accordance with accepted academic practice. No use, distribution or reproduction is permitted which does not comply with these terms.
*Correspondence: Arantzazu Alfranca, mariaaranzazu.alfranca@salud.madrid.org