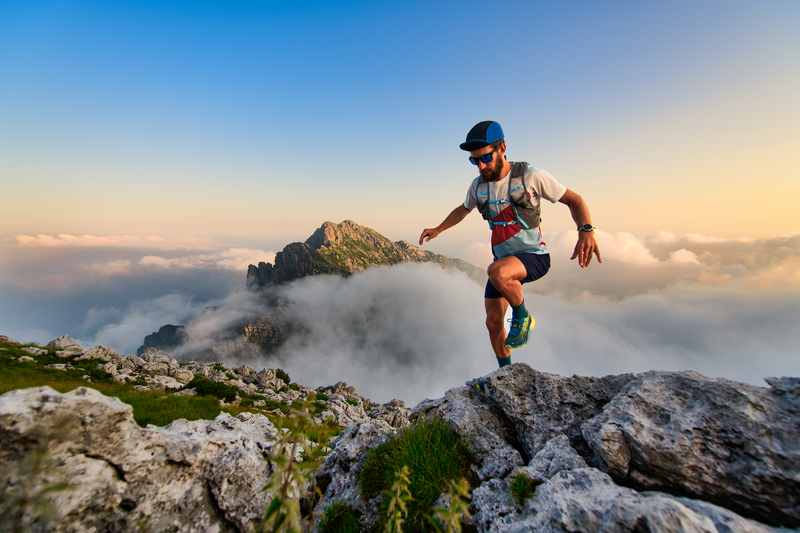
94% of researchers rate our articles as excellent or good
Learn more about the work of our research integrity team to safeguard the quality of each article we publish.
Find out more
MINI REVIEW article
Front. Immunol. , 21 September 2023
Sec. Alloimmunity and Transplantation
Volume 14 - 2023 | https://doi.org/10.3389/fimmu.2023.1231057
This article is part of the Research Topic In-Vitro, In-Vivo, and Ex-Vivo Models of Ischemia-Reperfusion Injury in Lung Transplantation View all 8 articles
Transplantation is an important life-saving therapeutic choice for patients with organ or tissue failure once all other treatment options are exhausted. However, most allografts become damaged over an extended period, and post-transplantation survival is limited. Ischemia reperfusion injury (IRI) tends to be associated with a poor prognosis; resultant severe primary graft dysfunction is the main cause of transplant failure. Targeting the cGAS–STING pathway has recently been shown to be an effective approach for improving transplantation outcomes, when activated or inhibited cGAS–STING pathway, IRI can be alleviated by regulating inflammatory response and programmed cell death. Thus, continuing efforts to develop selective agonists and antagonists may bring great hopes to post-transplant patient. In this mini-review, we reviewed the role of the cGAS–STING pathway in transplantation, and summarized the crosstalk between this pathway and inflammatory response and programmed cell death during IRI, aiming to provide novel insights into the development of therapies to improve patient outcome after transplantation.
The innate immune response mediated by the cyclic guanosine monophosphate-adenosine monophosphate (cGAMP) synthase-stimulator of interferon (IFN) genes (cGAS–STING) pathway has long been the front-line defense against pathogens, such as bacteria, parasites, DNA viruses, or retroviruses (1, 2). However, owing to the sequence-independent identification of double-stranded DNA (dsDNA), relevant research on the cGAS–STING pathway has indicated that cellular function extends beyond resisting the invasion of foreign pathogens, and unnecessary activation by accidental sensing of self-derived DNA or mutations can lead to autoinflammatory diseases (3). For example, STING-associated vasculopathy with onset in infancy (SAVI) commonly develops in patients with gain-of-function mutations in TMEM173 (4, 5). Aicardi-Goutières syndrome mainly presents with an aberrant generation of type I IFN, and accumulation of DNA damage may be an important driver of STING-related inflammatory responses (6, 7).
During organ acquisition, preservation, and transplantation, ischemia-reperfusion injury (IRI) exacerbates damage to donor graft tissues when blood flow is restored after a certain ischemic time. A deficient arterial blood supply invariably leads to a redox imbalance and creates a hypoxic environment in donor graft tissues. Surgical blood reperfusion can lead to severe oxidative damage and an inflammatory response following reoxygenation. This series of events aggravates allograft injury and may lead to primary graft dysfunction, which is associated with high mortality and morbidity (8, 9). The cellular and molecular events that occur during IRI are complex and involve innate immune system activation and programmed cell death (PCD) (10, 11). However, their interplay is still not clearly understood. In the context of limited treatment options, it is urgent to develop less toxic and higher specificity immunosuppressors to better control graft rejection and avoid mortality related to their toxicity (12).
In the present review, we offer an overview of the cGAS–STING pathway and highlight its role in patients who have undergone transplantation. We then summarize the pharmacological basis for targeting the cGAS–STING pathway for treating IRI to explore potential treatment approaches for IRI following transplantation.
Cyclic GMP-AMP synthase (cGAS) serves as a novel cytosolic DNA sensor that stimulates IFN production by binding to abnormal DNA within the cytoplasm and activates STING, this activation then triggers host innate immunity in response to “danger signals” (13, 14). The overview of the cGAS–STING signaling pathway is illustrated in Figure 1. The binding of abnormally accumulated dsDNA to cGAS in the cytoplasm greatly induces a phase transition, during which cGAS is activated. Recognition is independent of a specific sequence (15–18). Owing to the dsDNA-induced oligomerization of cGAS, a dimerized cGAS–dsDNA complex is catalytically formed (19–21). Activated cGAS promotes conformational changes in the catalytic pocket that allow the cyclization of GTP and ATP as substrates for conversion into cGAMP as a second messenger (22, 23).
Important sources of DNA within the cytoplasm include damage-associated DNA released from nuclear and mitochondrial leakage, as well as exogenous pathogen-associated DNA resulting from microbial infection (24, 25). Compared with bacterial cyclic dinucleotides, dsDNA-activated cGAS contains a linear 2’-5’-linked dinucleotide between GMP and AMP that effectively activates human STING (26, 27). Furthermore, higher DNA-binding valences and longer-packed DNA structures facilitate cGAMP production and innate immune signaling (15). Via gap junctions, receptor-based transport, and membrane fusion approaches, activated cGAS can trigger cGAMP transfer from original cells to bystander cells as additional routes to induce downstream signaling cascades (28, 29), which is illustrated in Figure 2. Regarding canonical cGAS–STING signaling, cGAMP is produced as an agonist for STING, and its binding to STING located in the endoplasmic reticulum (ER) induces a 180° rotation for ligand binding with the transmembrane domain as a reference, which unlocks the right-handed cross–over connections.
Figure 2 Intercellular communication in cGAS–STING signaling. (A) Gap junctions, (B) receptor-based transport, and (C) membrane fusion could serve as approaches for intercellular transmission of cGAMP.
Therefore, during the rearrangement of the STING dimer, the ligand-binding pocket is closed. This important conformational transition enables the oligomerization and release of STING from anchoring proteins, which are further translocated by integrating with cytoplasmic coat protein II complex vesicles (30–32). Cytoplasmic coat protein I-mediated retrograde membrane trafficking is also significant for STING activation (33, 34). Under the assistance of ADP-ribosylation factor GTPases and cytoplasmic coat protein II, higher-order complex STING is transferred from the ER through the ER-Golgi intermediate compartment to the Golgi apparatus. There, STING and the transcription factor IFN regulatory factor 3 (IRF3) are phosphorylated by recruited TANK-binding kinase 1 (TBK1), and nuclear factor κB is activated simultaneously (35–38). Phosphorylated IRF3 further oligomerizes and migrates into the nucleus with nuclear factor κB, and both synergistically initiate the expression of type I IFN and inflammatory cytokines, contributing to the innate immune response (39, 40).
Few studies have tried to elucidate the role of the cGAS–STING pathway in transplantation models. Some preclinical and clinical studies have demonstrated STING as an effectiveness therapeutic target for graft-versus-host disease following allogeneic hematopoietic stem cell transplantation (41). However, research on solid organ transplantation is still in its infancy, and thus more attention should be paid to this field.
The traditional Chinese herbal medicine ingredient ginsenoside Rb3 could alleviate oxidative stress caused by ischemia-reperfusion damage (42, 43). Li et al. used ginsenoside Rb3 to suppress adhesion molecule expression in endothelial cells (ECs) and improve microcirculation of murine transplanted skin flaps. They confirmed that the protective effect of IRI resulted from the inhibition of STING–IRF3 signaling (44). Besides, Yang et al. demonstrated that tumor necrosis factor-α-induced protein-8 like 2 (TIPE2), a negative immunoregulator for immune homeostasis, showed a positive correlation with apoptosis and TIPE2 expression in the graft, which might activate ferroptosis-mediated transplant rejection. TIPE2−/− mice that had undergone heart transplantation experienced insufficient IFN-γ production through the TBK1 signaling axis and increased expression of glutathione peroxidase 4 compared with wild-type mice. Mechanistically, TIPE2 deficiency may inhibit IFN-γ generation in T cells by suppressing the TBK1 signaling axis, prevent lipid peroxidation, and relieve ferroptotic cell death in an injured allograft (45, 46). Mesenchymal stromal cell therapy combined with low-dose tacrolimus is a feasible and safe therapeutic regimen (47, 48). Surprisingly, Chen et al. revealed that combination treatment using low-dose tacrolimus (FK506) and mesenchymal stem cells is beneficial to graft survival, possibly due to weakened graft inflammation by suppressing IFN-γ production and TBK1/IRF3 phosphorylation (49). The generation of STING-deficient mice through gene deletion is more conducive to improving our understanding of the cGAS–STING pathway and its importance in transplantation, and clinical trials are urgently needed.
SAVI is an autoinflammatory disease arising from gain-of-function mutations in the STING 1 gene from abnormal encoding, leading to the overproduction of type I IFN (5). Three patients diagnosed with SAVI who underwent solid organ transplantation have been reported. The first patient was a 1-year-old infant with SAVI who underwent liver transplantation and immunosuppressive therapy but developed severe multiple biliary cysts and cholangitis in the transplanted liver at the age of 3. Intensive tacrolimus, hydroxychloroquine, prednisolone, and mycophenolate mofetil were administered; however, the patient experienced fatal gastrointestinal bleeding 1 year later (50). The second patient was a 34-year-old woman with SAVI who underwent double-lung transplantation but experienced acute primary graft dysfunction, with acute liver and systemic vasculature complications; she finally died from multiple organ failure (51). The last patient was a 17-year-old girl with SAVI who underwent lung transplantation and developed systemic inflammatory symptoms within 4 months; she was treated with three immunosuppressors, including mycophenolate mofetil, tacrolimus, and prednisolone; however, her symptoms relapsed during prednisolone dose reduction (52). Despite reporting on individual cases, these studies indicated that the abnormal activation of STING in patients receiving transplantation probably led to extremely poor outcomes, even when immunosuppressive therapy was administered. Although the relationship between SAVI and the cGAS–STING pathway is not yet clear, inhibiting STING may be beneficial for improving the outcome of transplant recipients. However, research into this area is still lacking and is considered an important area for future studies.
IRI is a major transplant issue, mostly because there is still no effective treatment plan. Recently, it has been demonstrated that targeting the cGAS–STING pathway may be a feasible approach to improve transplantation outcomes by alleviating IRI. In general, the inflammatory response and PCD following ischemia and reperfusion play vital roles in triggering transplant rejection (53, 54). Both transplantation and non-transplantation models of IRI share these key pathogenic mechanisms to increase the incidence rate and mortality, and the cGAS–STING pathway participates in their regulation (2, 55). This suggests a possible relation between post-transplant IRI and the cGAS–STING pathway. Therefore, we have summarized the regulatory mechanisms of the cGAS–STING pathway in IRI in Figure 3; Table 1, providing new insights for the development of new treatment strategies. Besides, we extracted potential therapeutic agents for treating IRI that may help improve transplant prognosis.
Figure 3 Mechanisms underlying cGAS–STING activity in ischemia–reperfusion injury (IRI) condition. When IRI happens, self-DNA recognition is the primary determinant for cGAS–STING activity. mtDNA leakage, which is executed by RIP3 and PGAM5, is an important source of cGAS stimulation during IRI. But mitophagy mediated by PINK1 and IFI16 help to counteract mtDNA stress. Besides, HDAC3 can upregulate cGAS expression. cGAMP can be transmitted from parenchymal cells to neighboring macrophages through intercellular transmission to activate immune response. PGAM5, phosphoglycerate mutase family member 5; RIP3, receptor-interacting protein 3; IFI16, interferon gamma-inducible protein 16; PINK1, PTEN-induced kinase 1; NLRP3, NOD-like receptor protein 3.
Although it is yet to be fully explored, it would not be surprising to find that the inflammatory response of post-transplant IRI can be triggered by cGAS–STING pathway. Damage-associated molecular patterns (DAMPs) induced by injury are recognized by cGAS, which trigger immune-mediated inflammation. In general, targeting cGAS–STING pathway may help reduce the inflammatory response in transplantation models.
Increased cytosolic DNA can be recognized by the pathogen recognition receptor cGAS and trigger STING activation-induced inflammation, especially for mitochondrial DNA (mtDNA). Elevated mtDNA accumulation caused by IRI is related to delayed graft function (24, 79, 80). Phosphoglycerate mutase 5-mediated Bax dephosphorylation triggers mtDNA release, activating the cGAS–STING pathway and causing acute kidney injury following IRI (56). Furthermore, kidney IRI increases receptor-interacting protein 3 levels to facilitate mtDNA damage and leakage and then activates the cGAS–STING–p65 pathway by promoting cytosolic mtDNA expression and increases the transcription of pro-inflammatory factors (57). In contrast, mixed lineage kinase domain like (MLKL) pseudokinase knockout significantly enhances PTEN-induced kinase 1-mediated mitophagy activation to alleviate oxidative stress in hepatocytes, thereby inhibiting macrophage cGAS–STING activation and liver IRI (58). These make mtDNA an important target for inhibiting cGAS–STING pathway activation. In addition, a flap endonuclease I inhibitor has been shown to inhibit mtDNA fragment release and cGAS–STING pathway activation (81), which should be further verified in transplantation models. Notably, IRI, especially for apoptosis, is often associated with mitochondrial damage and mtDNA release, shaping a positive feedback circuit (82, 83). Thus, combination therapy for inhibiting cGAS–STING signaling and concurrent mtDNA fragment release may have a synergistic effect. Nowadays, some preclinical studies have made progress. STING inhibitor H-515 can prevent extracellular cold-inducible RNA-binding protein, a potent DAMP, from activating STING and causing intestinal and distant organ injuries (59). Moreover, by transcriptionally upregulating cGAS expression, histone deacetylase 3 (HDAC3) activates the cGAS–STING pathway in a p65-dependent manner; tissue inflammation and injury are triggered; accordingly, HDAC3 inhibitors, such as trichostatin A and MS275, can reverse this detrimental effect (60). Post-translational modifications of cGAS play critical roles in regulating its activity and stability (84, 85), and thus regulation of cGAS expression and function may be another intervention approach.
Recently increasing attention on macrophage-mediated innate immunity as a crucial player in allograft injury (86). Editing macrophage effector function may be an adjuvant therapy to alleviate inflammation. At present, most studies on the regulation of macrophages mediated by the cGAS-STING pathway have set liver IRI as the main research object. Aged mice are more susceptible to aggravated hepatic injury following ischemia-reperfusion, and stronger NLRP3 activation and pro-inflammatory activity of macrophages. Probably because aged parenchyma cells have more extracellular DNA than younger ones, which triggers a stronger STING/TBK1 signaling in macrophages. Consistent with previous studies, older donors are associated with reduced recipient and graft survival rates (61, 87, 88). Knockout of STING in Myelocyte can reduce liver IRI and inflammatory response, indicating that STING activation may promote the proinflammatory response of Monocyte derived macrophages in liver transplantation (62). In addition, Kupffer cells act as tissue-resident macrophages in the liver, playing an important role in in liver IRI as well, but the effect of cGAS-STING pathway toward it is still not clear. However, the promotion of microglial cell M1 polarization can be attenuated by the STING inhibitor C-176 during IRI-induced mtDNA release (63).
It is well known that T cell-mediated immune response is closely related to post-transplant IRI, which is an important factor affecting transplantation prognosis. The cGAS-STING pathway activates adaptive T cell responses by regulating dendritic cells and macrophages (64). By using cGAS-deficient donor tissue, the activation of CD8+ T cells in the graft and the proportion of effector memory lymphocytes in the spleen were reduced, and the graft survival was significantly prolonged, this provided a basis for immunosuppressive therapy targeting T cells (65). In addition, the induction of transplantation tolerance depends on the presence of Treg. Surprisingly, activation of the cGAS-STING pathway can induce an increase in the production of regulatory cytokine IL-10 and promote the inhibitory activity of Treg. Therefore, damage to grafts caused by T-cell-mediated adaptive immunity triggered by the cGAS-STING pathway may be the result of immune imbalance (89). Interestingly, although it has been speculated that STING gain-of-function mutations cause disease through abnormal type I interferon signaling, another study suggests that T cell-mediated adaptive immunity may be the main pathogenic factor, but more researches are needed to confirm it (90).
ECs form the primary barrier between the host and solid organ allografts and are essential for inducing cell-mediated acute rejection following transplantation (91). Mitochondrial exposure may upregulate EC adhesion molecules and enhance inflammatory responses by activating ECs (92). Mitochondrial transplant was able to reduce the risk of primary graft dysfunction in lung transplant recipients during ex-vivo lung perfusion (93). Mitochondrial transplantation therapy has shown promise as a therapy in clinical practice, but there is still a lack of research on the underlying molecular mechanisms. A recent study suggested that exposing murine heart ECs to exogenous mitochondria triggers internalized mitochondria-activated IFI16–STING–NF-κB signaling. Subsequently, STING-dependent mitophagy stabilized the endothelium and weakened apoptotic activity, and activated ECs promoted T-cell-mediated co-stimulation blockade-resistant rejection (94); the cGAS–STING pathway possibly plays a significant role in this.
Communication between the ER and mitochondria bridges ER stress and activates the innate immune system (66). Inhibition of the cGAS–STING pathway suppresses ER stress, thereby attenuating lung IRI (95). Surprisingly, ER stress-induced NLRP3 inflammasome activation is possibly a pivotal driver during post-transplant IRI (67). Moreover, the macrophage TXNIP-mediated CYLD-NRF2-OASL1 axis possesses a regulatory effect, and TXNIP disruption suppresses STING-mediated TBK1 activation and subsequent inflammation (96). However, these regulatory effects on macrophages should be further verified in transplantation models.
The cGAS–STING pathway is also involved in controlling energy metabolism. Activated cGAS–STING signaling is accompanied by systemic and cellular metabolism abnormality, involving increased nutrient metabolism and decreased mitochondrial respiration (68). In adipocytes, TBK1 attenuates AMP-activated protein kinase (AMPK) activation to increase energy reserves but inhibits respiration, and promotion of tissue inflammation can be observed in adipocyte-specific TBK1 knockout models (97). Surprisingly, by activating AMPK signaling, the STING inhibitor C-176 improves intestinal IRI-induced acute lung injury (98). Notably, metabolic disorders are often accompanied with intensive mitochondrial damage (69). This indicates the need to determine whether modulation of cGAS–STING pathway-induced metabolism is beneficial to inflammation regulation.
The cGAS–STING pathway participates in a variety of cell death pathways, including pyroptosis, ferroptosis, necroptosis, apoptosis, and autophagy, but without obvious specificity. During IRI following transplantation, multiple types of PCD may coexist. The cGAS–STING pathway serves as a target to provide further insight into their relation.
A strong STING signaling is associated with apoptosis induction (99, 100). Scutellarin plays a protective role in IRI through downregulation of the NLRP3 inflammasome, and it inhibits Bcl−2/Bax/Caspase−3 and the cGAS–STING pathway to ameliorate graft dysfunction and apoptosis (101, 102). STING antagonists can probably be used in combination with it in transplant models. Additionally, the in vitro upregulation of miR-24-3p relieves cardiomyocyte apoptosis following IRI (71). This protective effect may be due to the targeting of STING by miR-24-3p to salvage the STING–IRF3 activation-mediated inflammatory response and cellular apoptosis (103). Currently, there is a lack of non-invasive biomarkers in clinical practice that can be used to predict transplant prognosis (72). Although significant miRNAs in tissues are hardly as useful as non-invasive biomarkers, they can be used to inspire later research on biological fluids. More studies are necessary to discover the correlation between miRNAs and transplantation prognosis.
Recent studies have indicated that autophagy is involved in IRI regulation. Direct regulation of autophagy alleviates IRI following transplantation (104). Ferritinophagy is a type of autophagy that targets ferritin to maintain balanced intracellular iron levels. NCOA4-mediated ferritinophagy plays a vital role in IRI, and suppression of the cGAS–STING pathway can diminish ferritinophagy, thus ameliorating IRI (105). Activin A, a well-known neuroprotective factor, is also involved in IRI alleviation through inhibition of cGAS/STING-mediated autophagic cell death (73). 25-hydroxycholesterol is an oxidized cholesterol associated with the pathophysiological pathways of cholesterol homeostasis, immune response, or cell survival, it alleviates IRI by inhibiting STING and excessive autophagy-induced cell death (74, 106). Combinations of these agents with STING antagonists may probably help to lower doses of immunosuppressive drugs and reduce toxicity. Interestingly, cGAS-mediated autophagy has been shown to relieve liver IRI, with this novel protective effect being independent of STING (75). The cGAS–STING pathway participates in the regulation of autophagic cell death in a bidirectional manner. These conflicting results may be related to the existence of multiple noncanonical cascades (76). Thus, future studies should aim to validate the precise mechanism involved in selective activation and understand whether it is equally applicable to other pathogenic process.
Ferroptosis is triggered under conditions of excessive oxidative stress, such as IRI (107, 108). Ferroptotic cell death contributes to inflammatory responses following transplantation (109). Mechanistically, this PCD is induced by activation of the cGAS–STING pathway via lipid peroxidation, this damage can be reversed by the anti-lipid peroxidation drug liproxstatin-1 (110). Surprisingly, lipid peroxidation induced by cellular stress also specifically weakens the STING pathway (70). Thus, more accurate experiments are required to explain their relationship. Additionally, Ubiquitin-specific protease 7 inhibition could reverse ferroptosis-induced IRI, probably because of the suppression of TBK1 degradation and DNA methyltransferase 1 (DNMT1)-mediated methylation of FMRP translational regulator 1 (77, 111).
Both pyroptosis and necroptosis are inflammatory forms of cell death (112, 113). The cGAS–STING–IFN pathway is responsible for maintaining mixed lineage kinase domain-like pseudokinase (MLKL) expression, which is a key component for initiating necroptosis (114). Interestingly, mtDNA released from ECs during intestinal IRI activates the STING pathway and triggers necroptosis through collaborative IFN and tumor necrosis factor-α signaling (115). The cGAS–STING–NLRP3 axis has been demonstrated to be the default mode of inflammatory body activation and pyroptosis. STING activation induces lysosomal cell death and triggers the classic mode of NLRP3 activation (78). STING deficiency in macrophages can inhibit pyroptosis and the subsequent intense inflammatory response during liver IRI; this protective effect is probably due to reduced calcium-dependent caspase 1-GSDMD processing in macrophages (116). However, these results need to be confirmed and complemented in transplantation models.
At present, immunosuppressors effectively control transplant rejection; however, considerable issues, such as opportunistic infections, higher occurrence of malignancy, and drug toxicity, have been linked to their use. Compared to immunosuppressor, the breadth of the cGAS-STING pathway in inflammation and PCD is its most powerful advantage, so it has the potential to serve as a multifunctional therapeutic target. Because the mechanism of IRI after transplantation is very complex, single immunosuppressor is limited and combinations are required to achieve the desired therapeutic effect. Superimposed drug toxicity inevitably deteriorates the prognosis. Among them, the most serious side effect of Immunosuppressive drug in transplantation is the severe infection caused by the excessively low immunity of the body (117). The activation of the cGAS-STING pathway has a highly collaborative characteristic, and partial rather than complete blockade seems sufficient to produce anti-inflammatory effects, and proper activity of the cGAS-STING pathway is allowed under obvious infection conditions. Therefore, it is feasible to retain necessary ability of anti-infection while achieve anti-inflammatory, and achieving this balance can help improve prognosis. In order to achieve this balance, a key aspect in the future is to better understand the minimum level of inhibition required for therapeutic benefits. In addition, personalized treatment is the biggest problem faced by the clinical application of immunosuppressors. A lot of efforts are being made to develop small molecule inhibitors targeting the cGAS-STING pathway, and precise treatment based on this pathway may become an important component of future clinical organ transplantation (118).
IRI is currently a serious complication after transplantation, mainly because there is still no effective therapy to manage it. Attempting to utilize the cGAS–STING pathway as a potential target can provide new insights and help develop treatment approaches for post-transplant IRI. So far, many drugs targeting the cGAS–STING pathway have played therapeutic roles in IRI based on the mechanisms of inflammation and PCD. The next step may include further analysis of the results of these agents in transplantation models and exploring more convincing evidence to elucidate their clinical translation value. In addition, the detailed molecular mechanism of the cGAS–STING pathway is not yet clear, and preventing unexpected and adverse cascade reactions are also issues that need to be addressed.
Conception and design: ZC and WH. Administrative support: WH. Collection and assembly of data: ZC, YL, and ZL. Data analysis and interpretation: ZC and YL. Drafting the article or revising it critically for important intellectual content: All authors. Final approval of manuscript: All authors. Accountable for all aspects of the work in ensuring that questions related to the accuracy or integrity of any part of the work are appropriately investigated and resolved: All authors.
The authors declare that the research was conducted in the absence of any commercial or financial relationships that could be construed as a potential conflict of interest.
All claims expressed in this article are solely those of the authors and do not necessarily represent those of their affiliated organizations, or those of the publisher, the editors and the reviewers. Any product that may be evaluated in this article, or claim that may be made by its manufacturer, is not guaranteed or endorsed by the publisher.
IRI, Ischemia-reperfusion injury; cGAS-STING, cyclic guanosine monophosphate-adenosine monophosphate synthase-stimulator of interferon genes; cGAMP, cyclic guanosine monophosphate-adenosine monophosphate; cGAS, cyclic GMP-AMP synthase; IFN, interferon; dsDNA, double-stranded DNA; STING, synthase-stimulator of interferon genes; PCD, programmed cell death; ER, endoplasmic reticulum; IRF3, IFN regulatory factor 3; TBK1, TANK-binding kinase 1; SAVI, STING-associated vasculopathy with onset in infancy; EC, endothelial cell.
1. Cheng Z, Dai T, He X, Zhang Z, Xie F, Wang S, et al. The interactions between cGAS-STING pathway and pathogens. Signal Transduct Target Ther (2020) 5:91. doi: 10.1038/s41392-020-0198-7
2. Zhang X, Bai XC, Chen ZJ. Structures and mechanisms in the cGAS-STING innate immunity pathway. Immunity (2020) 53:43–53. doi: 10.1016/j.immuni.2020.05.013
3. Harapas CR, Idiiatullina E, Al-Azab M, Hrovat-Schaale K, Reygaerts T, Steiner A, et al. Organellar homeostasis and innate immune sensing. Nat Rev Immunol (2022) 22:535–49. doi: 10.1038/s41577-022-00682-8
4. Jeremiah N, Neven B, Gentili M, Callebaut I, Maschalidi S, Stolzenberg MC, et al. Inherited STING-activating mutation underlies a familial inflammatory syndrome with lupus-like manifestations. J Clin Invest (2014) 124:5516–20. doi: 10.1172/JCI79100
5. Liu Y, Jesus AA, Marrero B, Yang D, Ramsey SE, Sanchez GA, et al. Activated STING in a vascular and pulmonary syndrome. N Engl J Med (2014) 371:507–18. doi: 10.1056/NEJMoa1312625
6. Giordano AMS, Luciani M, Gatto F, Abou Alezz M, Beghè C, Della Volpe L, et al. DNA damage contributes to neurotoxic inflammation in Aicardi-Goutières syndrome astrocytes. J Exp Med (2022) 219:e20211121. doi: 10.1084/jem.20211121
7. Crow YJ. Type I interferonopathies: mendelian type I interferon up-regulation. Curr Opin Immunol (2015) 32:7–12. doi: 10.1016/j.coi.2014.10.005
8. Chouchani ET, Pell VR, James AM, Work LM, Saeb-Parsy K, Frezza C, et al. A unifying mechanism for mitochondrial superoxide production during ischemia-reperfusion injury. Cell Metab (2016) 23:254–63. doi: 10.1016/j.cmet.2015.12.009
9. Roesel MJ, Sharma NS, Schroeter A, Matsunaga T, Xiao Y, Zhou H, et al. Primary graft dysfunction: the role of aging in lung ischemia-reperfusion injury. Front Immunol (2022) 13:891564. doi: 10.3389/fimmu.2022.891564
10. Tang Q, Dong C, Sun Q. Immune response associated with ischemia and reperfusion injury during organ transplantation. Inflamm Res (2022) 71:1463–76. doi: 10.1007/s00011-022-01651-6
11. Linkermann A, Hackl MJ, Kunzendorf U, Walczak H, Krautwald S, Jevnikar AM. Necroptosis in immunity and ischemia-reperfusion injury. Am J Transplant (2013) 13:2797–804. doi: 10.1111/ajt.12448
12. Parlakpinar H, Gunata M. Transplantation and immunosuppression: a review of novel transplant-related immunosuppressant drugs. Immunopharmacol Immunotoxicol (2021) 43:651–65. doi: 10.1080/08923973.2021.1966033
13. Sun L, Wu J, Du F, Chen X, Chen ZJ. Cyclic GMP-AMP synthase is a cytosolic DNA sensor that activates the type I interferon pathway. Science (2013) 339:786–91. doi: 10.1126/science.1232458
14. Sun Z, Hornung V. cGAS-STING signaling. Curr Biol (2022) 32:R730–4. doi: 10.1016/j.cub.2022.05.027
15. Du M, Chen ZJ. DNA-induced liquid phase condensation of cGAS activates innate immune signaling. Science (2018) 361:704–9. doi: 10.1126/science.aat1022
16. Xie W, Lama L, Adura C, Tomita D, Glickman JF, Tuschl T, et al. Human cGAS catalytic domain has an additional DNA-binding interface that enhances enzymatic activity and liquid-phase condensation. Proc Natl Acad Sci USA (2019) 116:11946–55. doi: 10.1073/pnas.1905013116
17. Civril F, Deimling T, de Oliveira Mann CC, Ablasser A, Moldt M, Witte G, et al. Structural mechanism of cytosolic DNA sensing by cGAS. Nature (2013) 498:332–7. doi: 10.1038/nature12305
18. Yang H, Wang H, Ren J, Chen Q, Chen ZJ. cGAS is essential for cellular senescence. Proc Natl Acad Sci U.S.A. (2017) 114:E4612–20. doi: 10.1073/pnas.1705499114
19. Li X, Shu C, Yi G, Chaton CT, Shelton CL, Diao J, et al. Cyclic GMP-AMP synthase is activated by double-stranded DNA-induced oligomerization. Immunity (2013) 39:1019–31. doi: 10.1016/j.immuni.2013.10.019
20. Kranzusch PJ, Lee ASY, Wilson SC, Solovykh MS, Vance RE, Berger JM, et al. Structure-guided reprogramming of human cGAS dinucleotide linkage specificity. Cell (2014) 158:1011–21. doi: 10.1016/j.cell.2014.07.028
21. Kranzusch PJ, Lee AS, Berger JM, Doudna JA. Structure of human cGAS reveals a conserved family of second-messenger enzymes in innate immunity. Cell Rep (2013) 3:1362–8. doi: 10.1016/j.celrep.2013.05.008
22. Gao P, Ascano M, Wu Y, Barchet W, Gaffney BL, Zillinger T, et al. Cyclic [G(2’,5’)pA(3’,5’)p] is the metazoan second messenger produced by DNA-activated cyclic GMP-AMP synthase. Cell (2013) 153:1094–107. doi: 10.1016/j.cell.2013.04.046
23. Wu J, Sun L, Chen X, Du F, Shi H, Chen C, et al. Cyclic GMP-AMP is an endogenous second messenger in innate immune signaling by cytosolic DNA. Science (2013) 339:826–30. doi: 10.1126/science.1229963
24. Ablasser A, Chen ZJ. cGAS in action: Expanding roles in immunity and inflammation. Science (2019) 363:eaat8657. doi: 10.1126/science.aat8657
25. Landman SL, Ressing ME, van der Veen AG. Balancing STING in antimicrobial defense and autoinflammation. Cytokine Growth Factor Rev (2020) 55:1–14. doi: 10.1016/j.cytogfr.2020.06.004
26. Ablasser A, Goldeck M, Cavlar T, Deimling T, Witte G, Röhl I, et al. cGAS produces a 2’-5’-linked cyclic dinucleotide second messenger that activates STING. Nature (2013) 498:380–4. doi: 10.1038/nature12306
27. Zhang X, Shi H, Wu J, Zhang X, Sun L, Chen C, et al. Cyclic GMP-AMP containing mixed phosphodiester linkages is an endogenous high-affinity ligand for STING. Mol Cell (2013) 51:226–35. doi: 10.1016/j.molcel.2013.05.022
28. Zhou C, Chen X, Planells-Cases R, Chu J, Wang L, Cao L, et al. Transfer of cGAMP into Bystander Cells via LRRC8 Volume-Regulated Anion Channels Augments STING-Mediated Interferon Responses and Anti-viral Immunity. Immunity (2020) 52:767–81.e6. doi: 10.1016/j.immuni.2020.03.016
29. Ablasser A, Schmid-Burgk JL, Hemmerling I, Horvath GL, Schmidt T, Latz E, et al. Cell intrinsic immunity spreads to bystander cells via the intercellular transfer of cGAMP. Nature (2013) 503:530–4. doi: 10.1038/nature12640
30. Dobbs N, Burnaevskiy N, Chen D, Gonugunta VK, Alto NM, Yan N. STING activation by translocation from the ER is associated with infection and autoinflammatory disease. Cell Host Microbe (2015) 18:157–68. doi: 10.1016/j.chom.2015.07.001
31. Zhang X, Wu J, Du F, Xu H, Sun L, Chen Z, et al. The cytosolic DNA sensor cGAS forms an oligomeric complex with DNA and undergoes switch-like conformational changes in the activation loop. Cell Rep (2014) 6:421–30. doi: 10.1016/j.celrep.2014.01.003
32. Mukai K, Konno H, Akiba T, Uemura T, Waguri S, Kobayashi T, et al. Activation of STING requires palmitoylation at the Golgi. Nat Commun (2016) 7:11932. doi: 10.1038/ncomms11932
33. Mukai K, Ogawa E, Uematsu R, Kuchitsu Y, Kiku F, Uemura T, et al. Homeostatic regulation of STING by retrograde membrane traffic to the ER. Nat Commun (2021) 12:61. doi: 10.1038/s41467-020-20234-9
34. Steiner A, Hrovat-Schaale K, Prigione I, Yu CH, Laohamonthonkul P, Harapas CR, et al. Deficiency in coatomer complex I causes aberrant activation of STING signalling. Nat Commun (2022) 13:2321. doi: 10.1038/s41467-022-29946-6
35. Zhang C, Shang G, Gui X, Zhang X, Bai XC, Chen ZJ. Structural basis of STING binding with and phosphorylation by TBK1. Nature (2019) 567:394–8. doi: 10.1038/s41586-019-1000-2
36. Liu S, Cai X, Wu J, Cong Q, Chen X, Li T, et al. Phosphorylation of innate immune adaptor proteins MAVS, STING, and TRIF induces IRF3 activation. Science (2015) 347:aaa2630. doi: 10.1126/science.aaa2630
37. Zhao B, Shu C, Gao X, Sankaran B, Du F, Shelton CL, et al. Structural basis for concerted recruitment and activation of IRF-3 by innate immune adaptor proteins. Proc Natl Acad Sci USA (2016) 113:E3403–12. doi: 10.1073/pnas.1603269113
38. Ma X, Helgason E, Phung QT, Quan CL, Iyer RS, Lee MW, et al. Molecular basis of Tank-binding kinase 1 activation by transautophosphorylation. Proc Natl Acad Sci USA (2012) 109:9378–83. doi: 10.1073/pnas.1121552109
39. Agalioti T, Lomvardas S, Parekh B, Yie J, Maniatis T, Thanos D. Ordered recruitment of chromatin modifying and general transcription factors to the IFN-beta promoter. Cell (2000) 103:667–78. doi: 10.1016/s0092-8674(00)00169-0
40. Abe T, Barber GN. Cytosolic-DNA-mediated, STING-dependent proinflammatory gene induction necessitates canonical NF-κB activation through TBK1. J Virol (2014) 88:5328–41. doi: 10.1128/JVI.00037-14
41. Bader CS, Jin L, Levy RB. STING and transplantation: can targeting this pathway improve outcomes? Blood (2021) 137:1871–8. doi: 10.1182/blood.2020008911
42. Chen X, Wang Q, Shao M, Ma L, Guo D, Wu Y, et al. Ginsenoside Rb3 regulates energy metabolism and apoptosis in cardiomyocytes via activating PPARα pathway. BioMed Pharmacother (2019) 120:109487. doi: 10.1016/j.biopha.2019.109487
43. Sun J, Yu X, Huangpu H, Yao F. Ginsenoside Rb3 protects cardiomyocytes against hypoxia/reoxygenation injury via activating the antioxidation signaling pathway of PERK/Nrf2/HMOX1. BioMed Pharmacother (2019) 109:254–61. doi: 10.1016/j.biopha.2018.09.002
44. Li Y, Liu H, Zeng Z, Lin H, Chen X, Yuan X, et al. Ginsenoside Rb3 attenuates skin flap ischemia-reperfusion damage by inhibiting STING-IRF3 signaling. J Mol Histol (2022) 53:763–72. doi: 10.1007/s10735-022-10081-x
45. Yang Y, Ma Y, Yu S, Lin Z, Yan C, Wang Y, et al. TIPE2 knockout reduces myocardial cell damage by inhibiting IFN-γ-mediated ferroptosis. Biochim Biophys Acta Mol Basis Dis (2023) 1869:166566. doi: 10.1016/j.bbadis.2022.166566
46. Wang Q, Wei C, Ma L, Wang X, Li L, Zhou Q, et al. Inflammatory cytokine TNF-α promotes corneal endothelium apoptosis via upregulating TIPE2 transcription during corneal graft rejection. Graefes Arch Clin Exp Ophthalmol (2018) 256:709–15. doi: 10.1007/s00417-018-3913-0
47. Almansoori AA, Khentii N, Kim B, Kim SM, Lee JH. Mesenchymal stem cell therapy in submandibular salivary gland allotransplantation: experimental study. Transplantation (2019) 103:1111–20. doi: 10.1097/TP.0000000000002612
48. Dreyer GJ, Groeneweg KE, Heidt S, Roelen DL, van Pel M, Roelofs H, et al. Human leukocyte antigen selected allogeneic mesenchymal stromal cell therapy in renal transplantation: The Neptune study, a phase I single-center study. Am J Transplant (2020) 20:2905–15. doi: 10.1111/ajt.15910
49. Chen Y, Yan G, Ma Y, Zhong M, Yang Y, Guo J, et al. Combination of mesenchymal stem cells and FK506 prolongs heart allograft survival by inhibiting TBK1/IRF3-regulated-IFN-γ production. Immunol Lett (2021) 238:21–8. doi: 10.1016/j.imlet.2021.06.007
50. Ishikawa T, Tamura E, Kasahara M, Uchida H, Higuchi M, Kobayashi H, et al. Severe liver disorder following liver transplantation in STING-associated vasculopathy with onset in infancy. J Clin Immunol (2021) 41:967–74. doi: 10.1007/s10875-021-00977-w
51. Picard C, Thouvenin G, Kannengiesser C, Dubus JC, Jeremiah N, Rieux-Laucat F, et al. Severe pulmonary fibrosis as the first manifestation of interferonopathy (TMEM173 mutation). Chest (2016) 150:e65–71. doi: 10.1016/j.chest.2016.02.682
52. Kim D, Song KB, Choi EJ, Yu J. A 17-year-old girl diagnosed with STING-associated vasculopathy with onset in infancy (SAVI) after lung transplantation. Chest (2022) 162:e249–52. doi: 10.1016/j.chest.2022.05.025
53. Li Q, Lan P. Activation of immune signals during organ transplantation. Signal Transduct Target Ther (2023) 8:110. doi: 10.1038/s41392-023-01377-9
54. Capuzzimati M, Hough O, Liu M. Cell death and ischemia-reperfusion injury in lung transplantation. J Heart Lung Transplant (2022) 41:1003–13. doi: 10.1016/j.healun.2022.05.013
55. Murthy AMV, Robinson N, Kumar S. Crosstalk between cGAS-STING signaling and cell death. Cell Death Differ (2020) 27:2989–3003. doi: 10.1038/s41418-020-00624-8
56. Li J, Sun X, Yang N, Ni J, Xie H, Guo H, et al. Phosphoglycerate mutase 5 initiates inflammation in acute kidney injury by triggering mitochondrial DNA release by dephosphorylating the pro-apoptotic protein Bax. Kidney Int (2023) 103:115–33. doi: 10.1016/j.kint.2022.08.022
57. Feng Y, Imam Aliagan A, Tombo N, Draeger D, Bopassa JC. RIP3 Translocation into Mitochondria Promotes Mitofilin Degradation to Increase Inflammation and Kidney Injury after Renal Ischemia-Reperfusion. Cells (2022) 11:1894. doi: 10.3390/cells11121894
58. Xu J, Wu D, Zhou S, Hu H, Li F, Guan Z, et al. MLKL deficiency attenuated hepatocyte oxidative DNA damage by activating mitophagy to suppress macrophage cGAS-STING signaling during liver ischemia and reperfusion injury. Cell Death Discov (2023) 9:58. doi: 10.1038/s41420-023-01357-6
59. Kobritz M, Borjas T, Patel V, Coppa G, Aziz M, Wang P. H151, A SMALL MOLECULE INHIBITOR OF STING AS A NOVEL THERAPEUTIC IN INTESTINAL ISCHEMIA-REPERFUSION INJURY. Shock (2022) 58:241–50. doi: 10.1097/SHK.0000000000001968
60. Liao Y, Cheng J, Kong X, Li S, Li X, Zhang M, et al. HDAC3 inhibition ameliorates ischemia/reperfusion-induced brain injury by regulating the microglial cGAS-STING pathway. Theranostics (2020) 10:9644–62. doi: 10.7150/thno.47651
61. Zhong W, Rao Z, Rao J, Han G, Wang P, Jiang T, et al. Aging aggravated liver ischemia and reperfusion injury by promoting STING-mediated NLRP3 activation in macrophages. Aging Cell (2020) 19:e13186. doi: 10.1111/acel.13186
62. Jiao J, Jiang Y, Qian Y, Liu G, Xu M, Wang F, et al. Expression of STING is increased in monocyte-derived macrophages and contributes to liver inflammation in hepatic ischemia-reperfusion injury. Am J Pathol (2022) 192:1745–62. doi: 10.1016/j.ajpath.2022.09.002
63. Kong L, Li W, Chang E, Wang W, Shen N, Xu X, et al. mtDNA-STING axis mediates microglial polarization via IRF3/NF-κB signaling after ischemic stroke. Front Immunol (2022) 13:860977. doi: 10.3389/fimmu.2022.860977
64. Corrales L, McWhirter SM, Dubensky TW Jr, Gajewski TF. The host STING pathway at the interface of cancer and immunity. J Clin Invest (2016) 126:2404–11. doi: 10.1172/JCI86892
65. Li S, Xu H, Song M, Shaw BI, Li QJ, Kirk AD. IFI16-STING-NF-κB signaling controls exogenous mitochondrion-induced endothelial activation. Am J Transplant (2022) 22:1578–92. doi: 10.1111/ajt.17034
66. Huang R, Shi Q, Zhang S, Lin H, Han C, Qian X, et al. Inhibition of the cGAS-STING Pathway Attenuates Lung Ischemia/Reperfusion Injury via Regulating Endoplasmic Reticulum Stress in Alveolar Epithelial Type II Cells of Rats. J Inflamm Res (2022) 15:5103–19. doi: 10.2147/JIR.S365970
67. Zhan Y, Xu D, Tian Y, Qu X, Sheng M, Lin Y, et al. Novel role of macrophage TXNIP-mediated CYLD-NRF2-OASL1 axis in stress-induced liver inflammation and cell death. JHEP Rep (2022) 4:100532. doi: 10.1016/j.jhepr.2022.100532
68. Yang M, Ma YX, Zhi Y, Wang HB, Zhao L, Wang PS, et al. Inhibitors of IFN gene stimulators (STING) improve intestinal ischemia-reperfusion-induced acute lung injury by activating AMPK signaling. Eur J Med Res (2022) 27:79. doi: 10.1186/s40001-022-00703-1
69. Wu J, Liu Q, Zhang X, Wu X, Zhao Y, Ren J. STING-dependent induction of lipid peroxidation mediates intestinal ischemia-reperfusion injury. Free Radic Biol Med (2021) 163:135–40. doi: 10.1016/j.freeradbiomed.2020.12.010
70. Li JK, Song ZP, Hou XZ. Scutellarin ameliorates ischemia/reperfusion injury−induced cardiomyocyte apoptosis and cardiac dysfunction via inhibition of the cGAS−STING pathway. Exp Ther Med (2023) 25:155. doi: 10.3892/etm.2023.11854
71. Shen A, Zheng D, Luo Y, Mou T, Chen Q, Huang Z, et al. MicroRNA-24-3p alleviates hepatic ischemia and reperfusion injury in mice through the repression of STING signaling. Biochem Biophys Res Commun (2020) 522:47–52. doi: 10.1016/j.bbrc.2019.10.182
72. Li B, Wang W, Li Y, Wang S, Liu H, Xia Z, et al. cGAS-STING pathway aggravates early cerebral ischemia-reperfusion injury in mice by activating NCOA4-mediated ferritinophagy. Exp Neurol (2023) 359:114269. doi: 10.1016/j.expneurol.2022.114269
73. Liu M, Li Y, Han S, Wang H, Li J. Activin A alleviates neuronal injury through inhibiting cGAS-STING-mediated autophagy in mice with ischemic stroke. J Cereb Blood Flow Metab (2023) 43:736–48. doi: 10.1177/0271678X221147056
74. Lin F, Yao X, Kong C, Liu X, Zhao Z, Rao S, et al. 25-Hydroxycholesterol protecting from cerebral ischemia-reperfusion injury through the inhibition of STING activity. Aging (Albany NY) (2021) 13:20149–63. doi: 10.18632/aging.203337
75. Lei Z, Deng M, Yi Z, Sun Q, Shapiro RA, Xu H, et al. cGAS-mediated autophagy protects the liver from ischemia-reperfusion injury independently of STING. Am J Physiol Gastrointest Liver Physiol (2018) 314:G655–67. doi: 10.1152/ajpgi.00326.2017
76. Tang LJ, Zhou YJ, Xiong XM, Li NS, Zhang JJ, Luo XJ, et al. Ubiquitin-specific protease 7 promotes ferroptosis via activation of the p53/TfR1 pathway in the rat hearts after ischemia/reperfusion. Free Radic Biol Med (2021) 162:339–52. doi: 10.1016/j.freeradbiomed.2020.10.307
77. Zhang X, Wu J, Liu Q, Li X, Li S, Chen J, et al. mtDNA-STING pathway promotes necroptosis-dependent enterocyte injury in intestinal ischemia reperfusion. Cell Death Dis (2020) 11:1050. doi: 10.1038/s41419-020-03239-6
78. Wu XY, Chen YJ, Liu CA, Gong JH, Xu XS. STING induces liver ischemia-reperfusion injury by promoting calcium-dependent caspase 1-GSDMD processing in macrophages. Oxid Med Cell Longev (2022) 2022:8123157. doi: 10.1155/2022/8123157
79. Mallavia B, Liu F, Lefrançais E, Cleary SJ, Kwaan N, Tian JJ, et al. Mitochondrial DNA stimulates TLR9-dependent neutrophil extracellular trap formation in primary graft dysfunction. Am J Respir Cell Mol Biol (2020) 62:364–72. doi: 10.1165/rcmb.2019-0140OC
80. Xian H, Watari K, Sanchez-Lopez E, Offenberger J, Onyuru J, Sampath H, et al. Oxidized DNA fragments exit mitochondria via mPTP- and VDAC-dependent channels to activate NLRP3 inflammasome and interferon signaling. Immunity (2022) 55:1370–85.e8. doi: 10.1016/j.immuni.2022.06.007
81. McArthur K, Whitehead LW, Heddleston JM, Li L, Padman BS, Oorschot V. BAK/BAX macropores facilitate mitochondrial herniation and mtDNA efflux during apoptosis. Science (2018) 359:eaao6047. doi: 10.1126/science.aao6047
82. Riley JS, Quarato G, Cloix C, Lopez J, O’Prey J, Pearson M. Mitochondrial inner membrane permeabilisation enables mtDNA release during apoptosis. EMBO J (2018) 37:e99238. doi: 10.15252/embj.201899238
83. Konno H, Konno K, Barber GN. Cyclic dinucleotides trigger ULK1 (ATG1) phosphorylation of STING to prevent sustained innate immune signaling. Cell (2013) 155:688–98. doi: 10.1016/j.cell.2013.09.049
84. Xia P, Ye B, Wang S, Zhu X, Du Y, Xiong Z, et al. Glutamylation of the DNA sensor cGAS regulates its binding and synthase activity in antiviral immunity. Nat Immunol (2016) 17:369–78. doi: 10.1038/ni.3356
85. Panzer SE. Macrophages in transplantation: A matter of plasticity, polarization, and diversity. Transplantation (2022) 106:257–67. doi: 10.1097/TP.0000000000003804
86. Lan YY, Heather JM, Eisenhaure T, Garris CS, Lieb D, Raychowdhury R, et al. Extranuclear DNA accumulates in aged cells and contributes to senescence and inflammation. Aging Cell (2019) 18:e12901. doi: 10.1111/acel.12901
87. Kanneganti M, Olthoff KM, Bittermann T. Impact of older donor age on recipient and graft survival after LDLT: the US experience. Transplantation (2023) 107:162–71. doi: 10.1097/TP.0000000000004289
88. Wu Z, Miao X, Jiang Y, Kong D, Liu H, Xie W, et al. Cardiomyocytic cyclic GMP-AMP synthase is critical for the induction of experimental cardiac graft rejection. J Thorac Cardiovasc Surg (2023), S0022–522300199-X. doi: 10.1016/j.jtcvs.2023.03.005
89. Field CS, Baixauli F, Kyle RL, Puleston DJ, Cameron AM, Sanin DE, et al. Mitochondrial integrity regulated by lipid metabolism is a cell-intrinsic checkpoint for treg suppressive function. Cell Metab (2020) 31:422–437.e5. doi: 10.1016/j.cmet.2019.11.021
90. Luksch H, Stinson WA, Platt DJ, Qian W, Kalugotla G, Miner CA, et al. STING-associated lung disease in mice relies on T cells but not type I interferon. J Allergy Clin Immunol (2019) 144:254–266.e8. doi: 10.1016/j.jaci.2019.01.044
91. Kariya T, Ueta H, Xu XD, Koga D, Ezaki T, Yu E, et al. Direct evidence for activated CD8+ T cell transmigration across portal vein endothelial cells in liver graft rejection. J Gastroenterol (2016) 51:985–98. doi: 10.1007/s00535-016-1169-1
92. Lin L, Xu H, Bishawi M, Feng F, Samy K, Truskey G, et al. Circulating mitochondria in organ donors promote allograft rejection. Am J Transplant (2019) 19:1917–29. doi: 10.1111/ajt.15309
93. Cloer CM, Givens CS, Buie LK, Rochelle LK, Lin YT, Popa S, et al. Mitochondrial transplant after ischemia reperfusion promotes cellular salvage and improves lung function during ex-vivo lung perfusion. J Heart Lung Transplant (2023) 42:575–84. doi: 10.1016/j.healun.2023.01.002
94. Namgaladze D, Khodzhaeva V, Brüne B. ER-mitochondria communication in cells of the innate immune system. Cells (2019) 8:1088. doi: 10.3390/cells8091088
95. Pereira AC, De Pascale J, Resende R, Cardoso S, Ferreira I, Neves BM, et al. ER-mitochondria communication is involved in NLRP3 inflammasome activation under stress conditions in the innate immune system. Cell Mol Life Sci (2022) 79:213. doi: 10.1007/s00018-022-04211-7
96. Hasan M, Gonugunta VK, Dobbs N, Ali A, Palchik G, Calvaruso MA. Chronic innate immune activation of TBK1 suppresses mTORC1 activity and dysregulates cellular metabolism. Proc Natl Acad Sci USA (2017) 114:746–51. doi: 10.1073/pnas.1611113114
97. Zhao P, Wong KI, Sun X, Reilly SM, Uhm M, Liao Z. TBK1 at the crossroads of inflammation and energy homeostasis in adipose tissue. Cell (2018) 172:731–43.e12. doi: 10.1016/j.cell.2018.01.007
98. Sanz MN, Farine E, Niederberger P, Méndez-Carmona N, Wyss RK, Arnold M, et al. Cardioprotective reperfusion strategies differentially affect mitochondria: Studies in an isolated rat heart model of donation after circulatory death (DCD). Am J Transplant (2019) 19:331–44. doi: 10.1111/ajt.15024
99. Tang CH, Zundell JA, Ranatunga S, Lin C, Nefedova Y, Del Valle JR. Agonist-mediated activation of STING induces apoptosis in Malignant B cells. Cancer Res (2016) 76:2137–52. doi: 10.1158/0008-5472.CAN-15-1885
100. Gulen MF, Koch U, Haag SM, Schuler F, Apetoh L, Villunger A. Signalling strength determines proapoptotic functions of STING. Nat Commun (2017) 8:427. doi: 10.1038/s41467-017-00573-w
101. Xu LJ, Chen RC, Ma XY, Zhu Y, Sun GB, Sun XB. Scutellarin protects against myocardial ischemia-reperfusion injury by suppressing NLRP3 inflammasome activation. Phytomedicine (2020) 68:153169. doi: 10.1016/j.phymed.2020.153169
102. Xiao X, Lu Z, Lin V, May A, Shaw DH, Wang Z, et al. MicroRNA miR-24-3p reduces apoptosis and regulates keap1-nrf2 pathway in mouse cardiomyocytes responding to ischemia/reperfusion injury. Oxid Med Cell Longev (2018) 2018:7042105. doi: 10.1155/2018/7042105
103. Bardhi E, McDaniels J, Rousselle T, Maluf DG, Mas VR. Nucleic acid biomarkers to assess graft injury after liver transplantation. JHEP Rep (2022) 4:100439. doi: 10.1016/j.jhepr.2022.100439
104. Mao B, Yuan W, Wu F, Yan Y, Wang B. Autophagy in hepatic ischemia-reperfusion injury. Cell Death Discov (2023) 9:115. doi: 10.1038/s41420-023-01387-0
105. Cao Q, Liu Z, Xiong Y, Zhong Z, Ye Q. Multiple roles of 25-hydroxycholesterol in lipid metabolism, antivirus process, inflammatory response, and cell survival. Oxid Med Cell Longev (2020) 2020:8893305. doi: 10.1155/2020/8893305
106. Chen C, Xu P. Cellular functions of cGAS-STING signaling. Trends Cell Biol (2022) 33:630–48. doi: 10.1016/j.tcb.2022.11.001
107. Li C, Zhang Y, Liu J, Kang R, Klionsky DJ, Tang D. Mitochondrial DNA stress triggers autophagy-dependent ferroptotic death. Autophagy (2021) 17:948–60. doi: 10.1080/15548627.2020.1739447
108. Yu Y, Yan Y, Niu F, Wang Y, Chen X, Su G, et al. Ferroptosis: a cell death connecting oxidative stress, inflammation and cardiovascular diseases. Cell Death Discov (2021) 7:193. doi: 10.1038/s41420-021-00579-w
109. Li W, Feng G, Gauthier JM, Lokshina I, Higashikubo R, Evans S, et al. Ferroptotic cell death and TLR4/Trif signaling initiate neutrophil recruitment after heart transplantation. J Clin Invest (2019) 129:2293–304. doi: 10.1172/JCI126428
110. Jia M, Qin D, Zhao C, Chai L, Yu Z, Wang W, et al. Redox homeostasis maintained by GPX4 facilitates STING activation. Nat Immunol (2020) 21:727–35. doi: 10.1038/s41590-020-0699-0
111. Dong B, Ding C, Xiang H, Zheng J, Li X, Xue W, et al. USP7 accelerates FMR1-mediated ferroptosis by facilitating TBK1 ubiquitination and DNMT1 deubiquitination after renal ischemia-reperfusion injury. Inflamm Res (2022) 71:1519–33. doi: 10.1007/s00011-022-01648-1
112. Lukenaite B, Griciune E, Leber B, Strupas K, Stiegler P, Schemmer P. Necroptosis in solid organ transplantation: A literature overview. Int J Mol Sci (2022) 23:3677. doi: 10.3390/ijms23073677
113. Lucas-Ruiz F, Peñín-Franch A, Pons JA, Ramírez P, Pelegrín P, Cuevas S, et al. Emerging role of NLRP3 inflammasome and pyroptosis in liver transplantation. Int J Mol Sci (2022) 23:14396. doi: 10.3390/ijms232214396
114. Sarhan J, Liu BC, Muendlein HI, Weindel CG, Smirnova I, Tang AY. Constitutive interferon signaling maintains critical threshold of MLKL expression to license necroptosis. Cell Death Differ (2019) 26:332–47. doi: 10.1038/s41418-018-0122-7
115. Gaidt MM, Ebert TS, Chauhan D, Ramshorn K, Pinci F, Zuber S. The DNA inflammasome in human myeloid cells is initiated by a STING-cell death program upstream of NLRP3. Cell (2017) 171:1110–24.e18. doi: 10.1016/j.cell.2017.09.039
116. Roberts MB, Fishman JA. Immunosuppressive agents and infectious risk in transplantation: managing the “Net state of immunosuppression”. Clin Infect Dis (2021) 73:e1302–17. doi: 10.1093/cid/ciaa1189
117. Jansen MPB, Pulskens WPC, Uil M, Claessen N, Nieuwenhuizen G, Standaar D, et al. Urinary mitochondrial DNA associates with delayed graft function following renal transplantation. Nephrol Dial Transplant (2020) 35:1320–7. doi: 10.1093/ndt/gfy372
Keywords: allografts, cGAS-STING, ischemia-reperfusion injury, programmed cell death, transplantation, inflammation
Citation: Chen Z, Liu Y, Lin Z and Huang W (2023) cGAS–STING pathway in ischemia-reperfusion injury: a potential target to improve transplantation outcomes. Front. Immunol. 14:1231057. doi: 10.3389/fimmu.2023.1231057
Received: 30 May 2023; Accepted: 04 September 2023;
Published: 21 September 2023.
Edited by:
Stanislaw Stepkowski, University of Toledo, United StatesReviewed by:
Wang Qi, First Affiliated Hospital of Anhui Medical University, ChinaCopyright © 2023 Chen, Liu, Lin and Huang. This is an open-access article distributed under the terms of the Creative Commons Attribution License (CC BY). The use, distribution or reproduction in other forums is permitted, provided the original author(s) and the copyright owner(s) are credited and that the original publication in this journal is cited, in accordance with accepted academic practice. No use, distribution or reproduction is permitted which does not comply with these terms.
*Correspondence: Weizhe Huang, aHVhbmd3ZWl6aGUxOTgwQDE2My5jb20=
Disclaimer: All claims expressed in this article are solely those of the authors and do not necessarily represent those of their affiliated organizations, or those of the publisher, the editors and the reviewers. Any product that may be evaluated in this article or claim that may be made by its manufacturer is not guaranteed or endorsed by the publisher.
Research integrity at Frontiers
Learn more about the work of our research integrity team to safeguard the quality of each article we publish.