- 1Department of Oncology, The First Affiliated Hospital of Zhengzhou University, Zhengzhou, China
- 2Department of Pathology, The First Affiliated Hospital of Zhengzhou University, Zhengzhou, China
- 3Academy of Medical Science, School of Basic Medical Science, Zhengzhou University, Zhengzhou, China
Immunotherapy has developed rapidly in solid tumors, especially in the areas of blocking inhibitory immune checkpoints and adoptive T-cell transfer for immune regulation. Many patients benefit from immunotherapy. However, the response rate of immunotherapy in the overall population are relatively low, which depends on the characteristics of the tumor and individualized patient differences. Moreover, the occurrence of drug resistance and adverse reactions largely limit the development of immunotherapy. Recently, the emergence of nanodrug delivery systems (NDDS) seems to improve the efficacy of immunotherapy by encapsulating drug carriers in nanoparticles to precisely reach the tumor site with high stability and biocompatibility, prolonging the drug cycle of action and greatly reducing the occurrence of toxic side effects. In this paper, we mainly review the advantages of NDDS and the mechanisms that enhance conventional immunotherapy in solid tumors, and summarize the recent advances in NDDS-based therapeutic strategies, which will provide valuable ideas for the development of novel tumor immunotherapy regimen.
1 Introduction
Since 2013, Chen and Mellman have proposed the concept and critical mechanisms of the tumor immune cycle, suggesting the importance and potential promise of immunotherapy (Figure 1). Specific mechanisms include: a) the release of cell-associated antigens when tumor cells undergo death; b) the capture and presentation of antigens by dendritic cells (DCs); c) the initiation and activation of T cells; d) the recruitment of T cells to the tumor site; e) the infiltration of T cells into the tumor tissue; f) the recognition of corresponding tumor cells by T cells; and g) the killing of tumor cells by T cells via the secretion of granzyme, perforin, and other effectors (1). Immunotherapy has received more attention in recent years due to its robust efficacy and tolerable toxicity. According to the tumor immune cycle, current tumor immunotherapy mainly comprises immune targets antibody, tumor vaccine, cellular immunotherapy, oncolytic virus, and cytokine therapy (2). Chemotherapy drugs are also commonly used to enhance tumor cells immunogenicity, affect the function of immune cells such as DCs, Myeloid-derived suppressor cells (MDSCs), and Regulatory T cells (Tregs) (3). Immune checkpoint inhibitors (ICIs) such as PD-1/PD-L1 and CTLA-4 inhibitors, together with chemotherapy, have received satisfactory results in various clinical trials for most solid tumors (4). However, efficacy is limited by immune-related adverse events, therapeutic resistance, high cost, and limited therapeutic patients (5, 6).
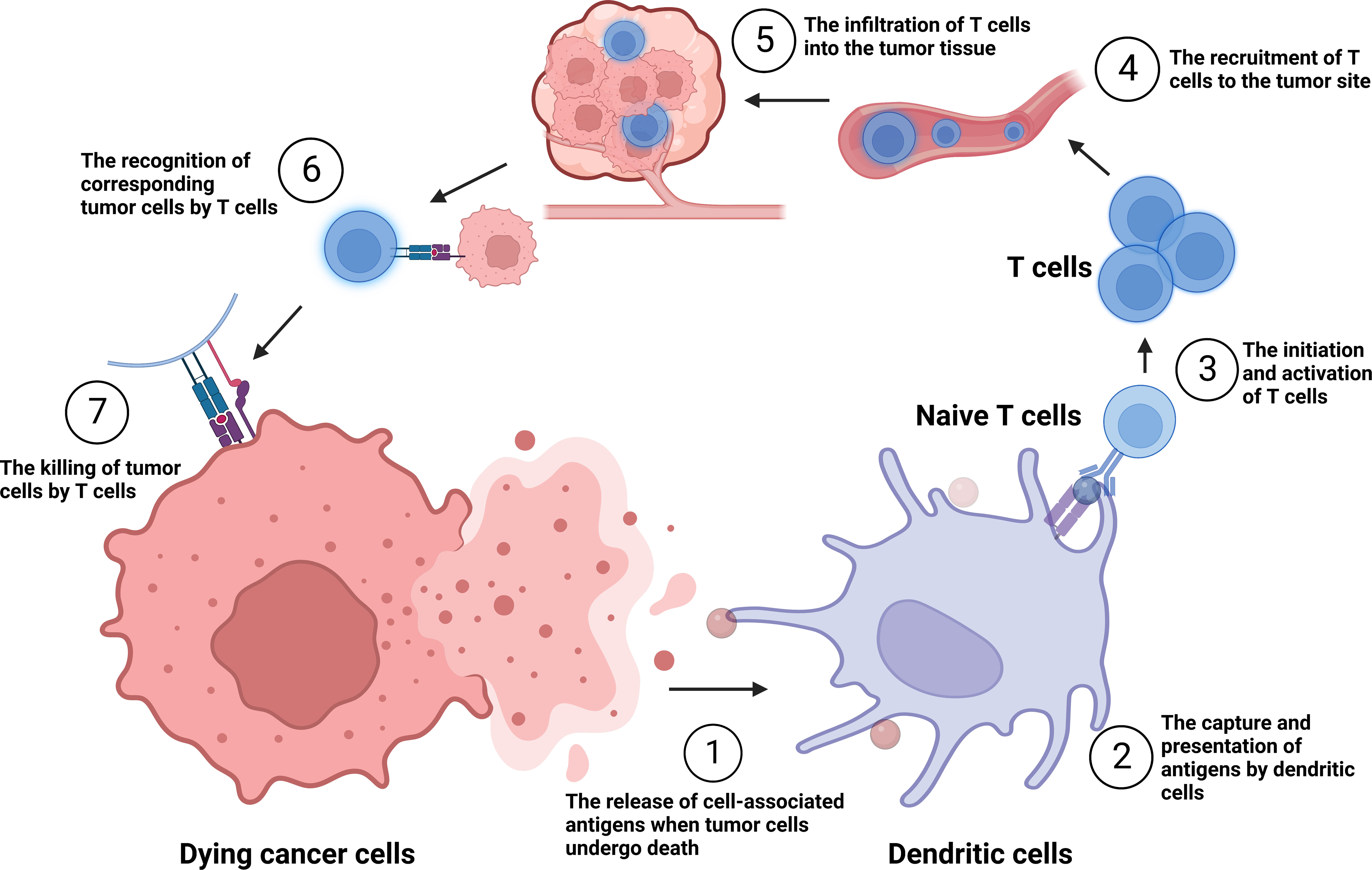
Figure 1 The tumor immune cycle put forward by Chen and Mellman. ①-⑦: the release of cell-associated antigens when tumor cells undergo death; the capture and presentation of antigens by dendritic cells (DCs); the initiation and activation of T cells; the recruitment of T cells to the tumor site; the infiltration of T cells into the tumor tissue; the recognition of corresponding tumor cells by T cells; and the killing of tumor cells by T cells.
Recently, we found that immunogenic cell death (ICD) induction might play a critical factor in improving immunotherapy. ICD releases tumor-associated antigens and endogenous danger signals, further triggering antigen-presenting cells and ultimately activating effective antitumor immune response (7). Several therapies such as chemotherapy, radiotherapy (RT), photothermal therapy (PTT), photodynamic therapy (PDT), and sonodynamic therapy (SDT) and drugs (certain chemotherapeutic drugs, traditional Chinese medicines) have worked to initiate and/or enhance ICD, leading to the activation of tumor-specific immune responses (8, 9). Additionally, the tumor microenvironment (TME) plays a significant role in promoting the tumor immunosuppressive microenvironment (TIME) (10). Based on these points, we need to explore novel methods to overcome current limitations and increase the immunotherapy effect.
One of the novel ways of improving the efficacy of tumor immunotherapy is nanodrug delivery systems (NDDS), which have the features of small size and ease editing. Various smart responsive nanoparticles (NPs) have been designed to deliver drugs accurately to the tumor site, which greatly weakens the “off-target” effect (11–13). Many experiments have shown that NDDS therapy combined with PD-1/PD-L1 antibodies can not only enhance immune efficacy but also control distant metastasis and recurrence of tumors (14). In this article, we mainly discuss the mechanisms of NDDS to enhance immunotherapy and summarize recent NDDS based treatment strategies. We describe the advantages of enhanced anti-tumor effects of NDDS by elucidating the relevant mechanisms. And we summarize the mechanisms of how NDDS-based treatment strategies enhance immunotherapy, including the induction of ICD process and the reprogramming of immunosuppressive microenvironment.
2 The status and limitation of immunotherapy in solid cancers
Tumor immunotherapy comprises various strategies, including ICIs, cellular immunotherapy (15), therapeutic tumor vaccines (16), oncolytic viruses (17), and cytokine immunotherapy (18). ICIs are the most widely used strategy for the treatment of solid tumors. Following the FDA approval of Ipilimumab (a CTLA-4 inhibitor) for advanced melanoma in 2011, ICIs quickly gained approval for treating various cancer types (19), as evidenced by several FDA-approved immune checkpoints such as PD-1 and its receptor PD-L1, CTLA-4, and LAG3. CTLA-4 inhibitors (e.g. Ipilimumab and Tremelimumab), PD-1 inhibitors (e.g. Pembrolizumab, Nivolumab, and Cemiplimab) and PD-L1 inhibitors (e.g. Atezolizumab, Durvalumab, and Avelumab) are widely used alone or in combination to treat different types of cancer, including melanoma, non-small cell lung cancer, bladder cancer, renal cell carcinoma, head and neck squamous cell carcinoma, among others (20). Until last year, the second-generation checkpoint inhibitor Opdualag (targeting LAG-3) was just launched, and it was classified as a first-line treatment for unresectable melanoma along with the PD-1 inhibitor nivolumab. This result is based on a phase 2/3 study in which the median progression-free survival with the combination (10.1 months) was stronger than with Opdivo monotherapy (4.6 months), showing promising clinical response (21). Immunotherapy has emerged as a revolutionary approach to treat cancer, but its efficacy is often limited (22). While mild cases of CRS manifest as fever, fatigue, headache, rash, joint pain, and myalgia, severe cases can lead to an uncontrolled systemic inflammatory response characterized by low blood pressure and high fever (23). Although CAR-T therapy has been successful in treating B-cell lymphomas and leukemias, it has shown limited effectiveness against solid tumors, possibly due to low penetration in the tumor matrix, pressure gradients, and the immunosuppressive microenvironment (24). Other immunotherapies such as therapeutic tumor vaccines, oncolytic viruses, and cytokines face major safety, efficacy, and delivery barriers. One of the major limitations is the low number of patients who respond to ICIs despite their breakthrough success, adding to the issue that patients may gradually develop resistance to immunotherapy (20). Furthermore, a significant proportion of patients experience immune-related toxicity, such as cytokine release syndrome and immune effector cell-associated neurotoxic syndrome (25). Improving drug transport ways to enhance drug concentration and targeting ability is currently recognized as an effective method.
3 The advantage of nanodrug delivery system in solid tumors
NDDS primarily rely on the encapsulation of drugs or biological molecules within particles with a diameter less than 100 nm. Alternatively, NDDS also encompasses materials within the range of 100 nm to 1,000 nm, but possessing nanoparticle behavior. These systems employ distinctive particle properties such as acoustic, electric, optical, magnetic, and thermal attributes to facilitate the targeted transportation of nanoparticles bearing drugs or other biological molecules to specific cells or tissues. FDA-approved NDDS materials primarily include, but are not limited to liposomes, polymers, and inorganic materials (26). More materials of NPs are being developed.
Compared with traditional immunotherapy in solid cancers, NDDS-based immunization strategies provide more advantages (Figure 2). They are listed as follows: a) Enhanced permeability and retention (EPR) effect. EPR effect is a passive targeting process that can be induced by the interaction of endothelial lining gaps extravasation and immune cells in the TME (27–29). However, EPR effects are more pronounced in preclinical studies (small animal xenograft tumor models) and very limited in human tumors, which is a very controversial topic for current research (30). b) Improving solubility of hydrophobic drugs. Most clinical drugs have poor water solubility and biocompatibility. However, NDDS overcomes this obstacle in by creating lipophilic cavities internally to encapsulate drugs, while the external structure of the carrier is hydrophilic and better suited to biological tissues (31, 32). c) Achieving active targeting delivery. NDDS can bind to specific receptors on the cell surface using specific ligands or antibody components, to achieve accumulation in the targeted tissue. The information from the overexpression of some genes, cytokines, and proteins of tumor cells forms the basis for the design of targeted drugs containing intelligent recognition sites. Commonly used targeting molecules include antibody fragments and molecules, folic acid corresponding to ligands (33), proteins (e.g. transferrin) (34), peptide molecules (e.g. RGD and TAT) (35, 36), nucleic acid aptamers (e.g. aptamer) (37) and polysaccharides (e.g. mannose) (38). d) Reducing physiological barriers to drug hindrance. Non-NPs-based drug molecules must undergo phagocytosis by the reticuloendothelial system (RES), cell membrane barrier-permeation and intracellular transport barriers-lysosomal degradation before reaching the target. While most NDDS are designed smaller and multifunctional which can evade these obstacles (39). e) Integration of diagnosis and treatment. Nanomaterials have unique physical properties such as light, heat and magnetism, which offer nanocarriers the potential to be used as diagnostic probes with imaging. Monitoring drug concentration at the target site greatly improves therapeutic efficiency (40). f) Stimulus-responsive intelligent nanodrug delivery systems (41). most tumor microenvironment is characterized by hypoxia, acidity, high expression of enzymes, glutathione and reactive oxygen species, based on which single-factor, or multi-responsive nanomaterial delivery systems can be designed to achieve targeted drug delivery (42). Since FDA approved the first liposome nanodrug - Doxil in 1995, many drugs for treating cancers have been launched one after another (Table 1).
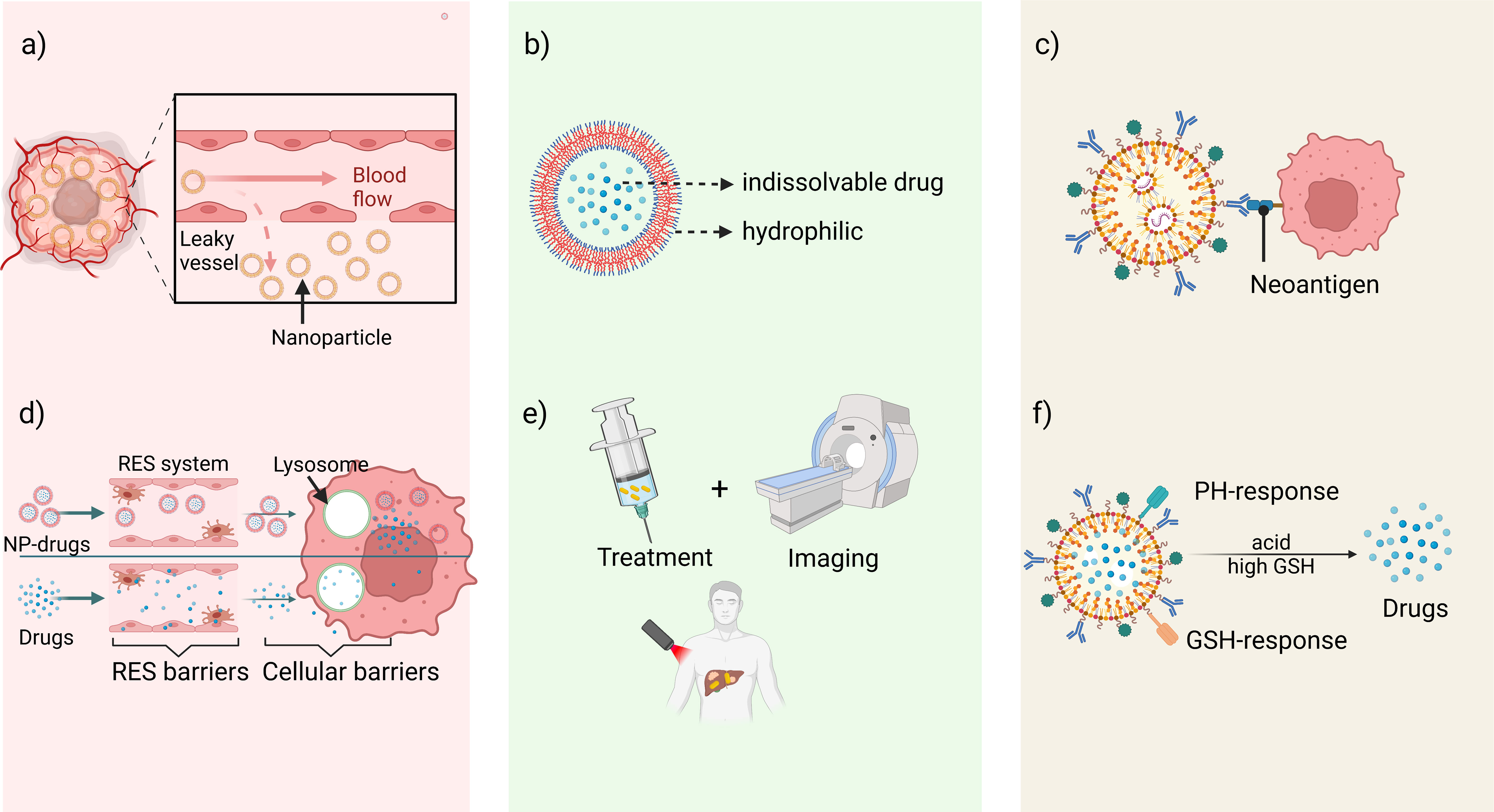
Figure 2 The advantages of NDDS-based immunotherapy. (A) Enhanced permeability and retention (EPR) effect. (B) Improving solubility of hydrophobic drugs. (C) Achieving active targeting delivery by specific antigens. (D) Reducing physiological barriers to drug hindrance. (E) Integration of diagnosis and treatment. (F) Stimulus-responsive intelligent nanodrug delivery systems.
4 The mechanisms of nano-drug delivery system to enhance immunotherapy
NDDS were initially designed to alter the toxicity and pharmacokinetics profiles of chemotherapy agents, allowing for higher drug concentrations to accumulate inside tumors. Recently, with the identification of new immune activation pathways, there has been a surge of interest in using nanomaterials for immunotherapy against solid tumors. The main mechanisms of action involve the induction of ICD and modulation of the immunosuppressive microenvironment.
4.1 NDDS enhances immunotherapy by inducing immunogenic tumor cell death
Cell death stimuli in tumors induce tumor cells to become immunogenic, resulting in the release of molecules that initiate an anti-tumor immune response called ICD process (43). Throughout this process, the tumor cells produce a group of signaling molecules known as damage-associated molecular patterns (DAMPs) (44), including calreticulin (CRT) that exposes on the cell surface, secreted ATP, heat shock proteins (HSP70 and HSP90), and high mobility group protein 1 (HMGB1) (Figure 3). Anthracyclines and oxaliplatin stimulate calreticulin to transfer from the intracellular site to the outer surface of the cell membrane where it is recognized by DCs and presented as tumor antigens, thus initiating the ICD process (45). Other chaperone molecules such as HSP70 and HSP90 can also valgus to the surface of the cell membrane as “eat me” signals (46). The release of ATP serves as a “find me” signal, that attracts mature or immature DCs close to the tumor site, delivering the energy needed for DCs to present antigens (47, 48). HMGB1 acts as the most critical initiator of ICD by binding to Toll-like receptor 4 on DC membranes, thereby activating cytotoxic T cell lymphocytes (CTLs). ICD inducers are commonly categorized into two classes known as type I and type II. Type I ICD inducers initiate the apoptosis of cancer cells proficiently, without affecting the endoplasmic reticulum (ER). These ICD inducers generate a moderate level of ER stress and discharge DAMPs that result in the formulation of immunogenic molecules. Conversely, type II ICD inducers produce more significant ER stress along with elevated levels of reactive oxygen species (ROS), and discharge more DAMPs, ultimately escalating the tumor’s immune response to a greater extent (49, 50). Figure 4 depicts the classification mechanisms of the currently established ICD inducers (51, 52). However, not all clinical drugs or therapeutic strategies can activate ICD, nor can all ICD inducers be efficiently utilized. The use of NDDS provides a new and efficient approach for boosting the existing therapeutic efficacy and reducing the side effects of cancer agents (53). Table 2 outlines the present clinical ICD inducers and immunotherapeutic strategies. In this section, we will discuss how NDDS-based therapeutic strategies can enhance immunotherapy by inducing ICD.
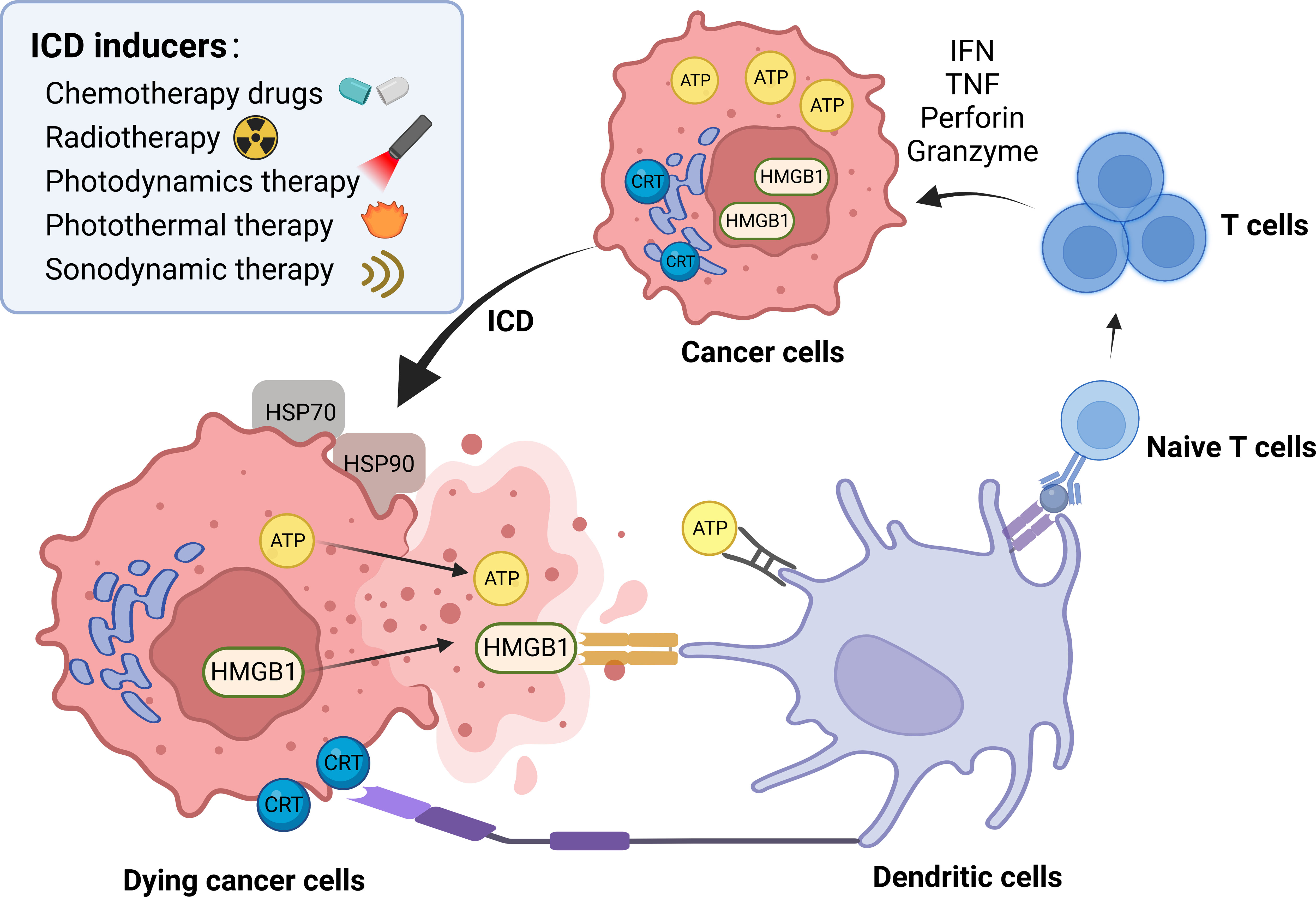
Figure 3 ICD process and common inducers. DAMPs are the main pattern of ICD process, including calreticulin (CRT) that exposes on the cell surface, secreted ATP, heat shock proteins (HSP70 and HSP90), and high mobility group protein 1 (HMGB1). Some chemotherapy drugs, radiotherapy, photodynamic therapy, photothermal therapy and sonodynamic therapy are common ICD inducers. → represents the pointing and promoting effect.
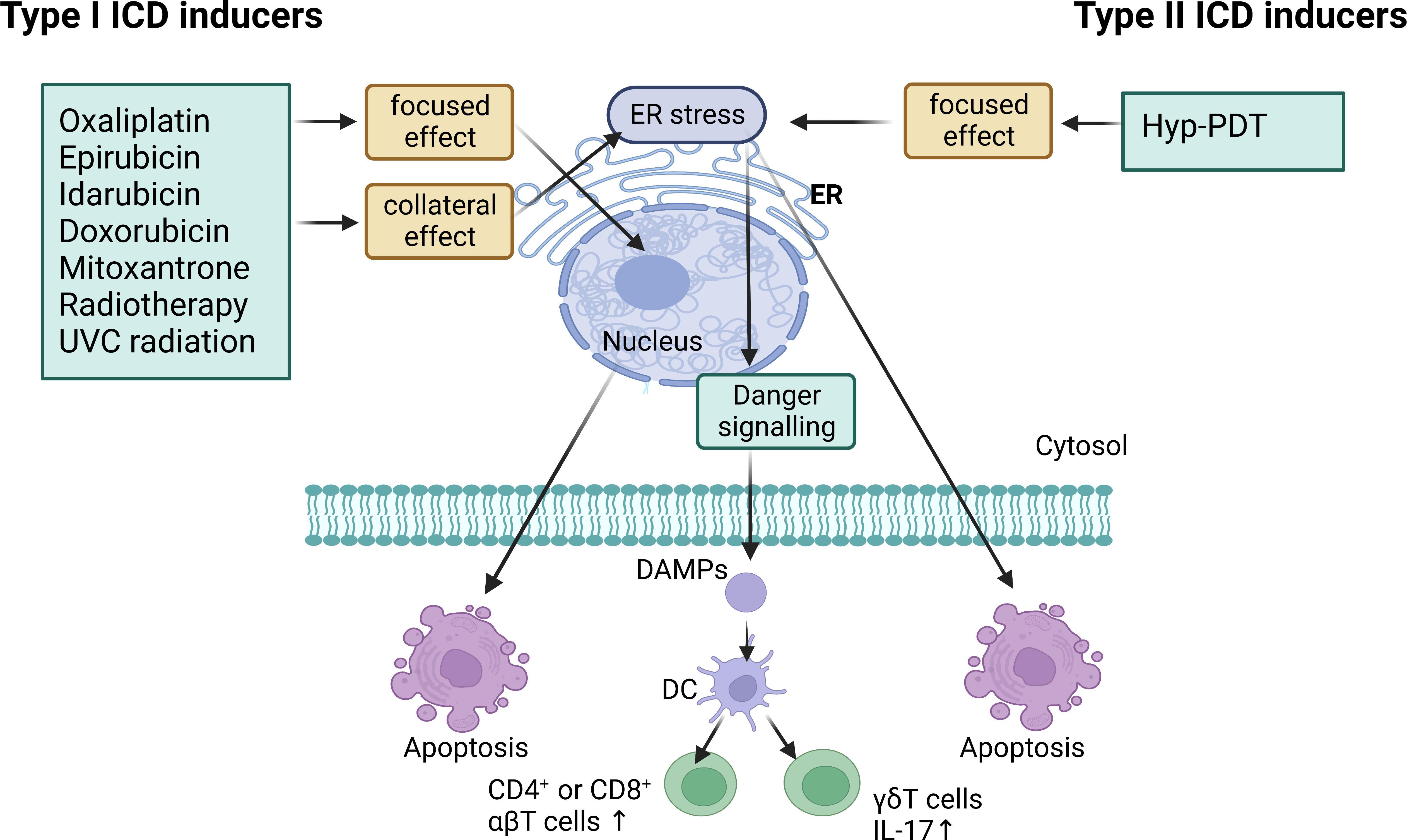
Figure 4 Type I ICD inducers are anticancer drugs that operate on non-ER proteins to induce cell death while also promoting collateral ER stress for danger signaling. Type II ICD inducers are anticancer agents that target the ER for both cell death induction and danger signaling. They both have the ability to induce the emission of damage-associated molecular patterns (DAMPs) which cause the activation of IL-17-producing γδT cells and increased proliferation of CD4+ or CD8+ αβT cells. Hyp-PDT, hypericin-based photodynamic therapy.
4.1.1 Nano-based chemo-immunotherapy
Firstly, compared to non-ICD inducers, ICD inducers demonstrate greater potential in antitumor treatment in clinical settings. Chemotherapy drugs such as doxorubicin, epirubicin, idarubicin, mitoxantrone, bleomycin, bortezomib, cyclophosphamide, and oxaliplatin can induce ICD if used as solitary therapeutic interventions (49). However, these drugs may have weak immunogenicity and lead to adverse effects for many patients. NDDS can ameliorate these problems by extending the functioning cycle of chemotherapy drugs with a reverse-phase protein array effect, minimizing toxic side-effects by utilizing precise targeting characteristics, and augmenting tumor immunity with ICD, thus opening new opportunities for combination chemotherapy and immunotherapy (88, 89).
Nanoparticles contribute to both ICD induction and microenvironment reprogramming. For example, Fluorouracil (5-Fu) and oxaliplatin (OxP) are two commonly used chemotherapeutic agents for CRC and HCC and both are ICD inducers (51, 90). FOLFOX (Folinic acid + 5-Fu + OxP) is a standard chemotherapy regimen for advanced CRC and HCC patients. Jianfeng Guo et al. designed two nano-formulations to treat in situ CRC and HCC mice with or without PD-L1 antagonists. One Nano-formulation was nano-FdUMP (5-Fu active metabolite), which induced ROS formation and significantly improved the efficacy of the second nanoformulation, nano-Folox, in inducing ICD. However, nano-FdUMP could not induce ICD on its own but achieved an efficient chemo-immunotherapeutic response in combination with nano-Folox. The combination of both nanoformulations transformed the ‘cold’ TME into a ‘hot’ one, supported by increased numbers of CD8+ T cells, CD4+ T cells, dendritic cells, IFN-γ, TNF-α, and IL-12 and reduced numbers of MDSCs, Tregs, tumor-associated macrophages (M2), IL-4, IL-6, and IL-10. With the immunosuppressive TME reprogrammed, the anti-PD-L1 monoclonal antibody showed improved efficacy against microsatellite stable CRC liver metastasis (54). Bing Feng’s team designed a binary cooperative prodrug nanoparticle (BCPN) that, when triggered by an acidic environment, OxP and NLG919 (an IDO-1 inhibitor that regulates immunosuppression) were activated and released, achieving a stronger EPR effect, promoting T lymphocyte infiltration by stimulating ICD recruitment, and reducing tumor burdens (breast and colorectal cancer) (91). 3-(2-nitrophenyl) propionic acid-paclitaxel nanoparticles (NPPA-PTX NPs) work as an ICD inducer in MDA-MB-231 and 4T1 cell lines by upregulating HMGB1 and CRT. The combination of NPPA-PTX nanoparticles and anti-PD-L1 monoclonal antibody enhances the antitumor response by recruiting infiltrating CD8+, CD3+, CD4+ T cells and increasing IFN-γ and TNF-α levels (92). While irinotecan is a weakly alkaline drug that neutralizes the acidic lysosomal environment in pancreatic ductal adenocarcinoma (PDAC) cells. Based on this, Mesoporous silica nanoparticles delivery system amplifies this advantage, causing a series of endoplasmic reticulum response, immunogenic cell death, and PD-L1 expression. This effect was observed in an orthotopic Kras-dependent pancreatic cancer model. When combined with anti-PD-L1 therapy, the use of silicosomes showed better chemo-immunotherapy response compared to free or liposomal drugs such as Onivyde (57). Chemotherapeutic agents may also induce immunogenic cell death through autophagy process to mediate antitumor immunotherapy. A recent study of STF@AHPP nanoparticles, combining epirubicin with an autophagy inducer STF-62247 have shown a stronger immune activation ability than epirubicin alone in mice with CT26 tumors. This provides a new approach to combining chemo-immunotherapy and autophagy agonist (61).
Of course, in addition to the regular chemotherapeutic agents, there are new drugs that can also induce ICD and contribute to chemo-immunotherapy. Ginsenoside Rg3 and quercetin (QTN) are examples of a novel ICD inducer and a ROS generator, which enhance the Rg3-mediated ICD. A folate-targeted PEGylated cyclodextrin-based nanoparticle that delivers Rg3 and QTN was developed by Dandan Sun et al. This co-delivery formulation, when combined with anti-PD-L1 antibody, achieved chemo-immunotherapy in colorectal cancer patients who were insensitive to ICIs (55). Chinese herbal medicine-Icariin was encapsulated by polylactic acid-glycolic acid and injected into gastric cancer patients as PLGA@Icaritin NPs. PLGA@Icaritin may also contribute to the production of dozens of ROS, which can result in a significant loss of mitochondrial membrane potential and overproduction of oxidized mitochondrial DNA (Ox-mitoDNA). This leads to the release of DAMPs and ultimately results in ICD. In vivo studies have shown that PLGA@Icaritin nanoparticles enhance anti-tumor immunity by recruiting infiltrating CD4+ cells, CD8+ T cells, and immune factors such as IFN-γ, TNF-α, and IL-1 (93).
4.1.2 Nano-based RT/PDT/PTT/SDT
Ablative treatments for cancer, including radiotherapy (RT) (94), photodynamics therapy (PDT) (95), photothermal therapy (PTT) (96) and sonodynamic therapy (SDT) (97) are effective methods for inducing ICD in various solid tumors. Often acting as type II ICD inducers, ablative therapies generate ROS and stimulate ER stress response (98). Different nanomaterials can be employed to transport medications, vaccines and other compounds to the tumor location for augmented drug accumulation in the radiation treatment zone, resulting in elevated sensitivity of RT/PDT/PTT/SDT. At the same time, NDDS with acoustic, optical, thermal, and magnetic properties can be used to realize imaging tacking in vivo, providing convenience for the precision of immunotherapy (76, 99, 100).
RT has been extensively used in clinical practice as a local therapy for more than a century. Although RT mainly targets the DNA of tumor cells, the induction of ICD provides a new possibility for immunotherapy (101). In fact, RT is a dose-dependent therapy. Large doses of radiotherapy primarily trigger ICD, release tumor-specific antigens, activate immune cells, and enhance the density of tumor-infiltrating lymphocytes (TILs). Low doses of RT primarily recruit immune cells, regulate the inflammatory microenvironment, and produce an abscopal effect (102). These mechanisms are the fundamental basis for Nano-based RT-enhanced immunotherapy. The abscopal effect, a systemic inhibitory effect on metastatic tumors beyond the radiation field, was first discovered in 1953 as a result of radiation therapy (103). To maximize the benefits of RT in systemic therapy, scientists are actively exploring drugs and delivery methods that are compatible with RT. For example, cisplatin is said to increase the CD8+ T cells in RT plus anti-PD-1 treated tumors. Ying Wang et al. then explored that cisplatin (CDDP) loaded complex nanoparticles consisting of poly (L-glutamic acid)-graft-methoxy poly (ethylene glycol) (CDDP-NPs) have a stronger inhibitory effect on RT and amplify RT-induced ICD in models of Lewis lung carcinoma. Furthermore, CDDP-NPs can significantly improve the abscopal effect of RT plus anti-PD-1 in the treatment of non-radiotherapy tumors (64). This promising combination strategy was reported by another study. The efficacy of radioimmunotherapy for colorectal cancer is limited. While “carrier free” coordination polymer nanorods (ZGd-NRs) (composed of zoledronic acid and gadolinium) have been shown to deposit X-rays, enhance ROS induced by RT, and induce ICD characterized by increased CRT, HMGB1, and ATP expression in CT26 cell lines. Additionally, ZGd-NRs improves the immune-suppressive microenvironment by inhibiting TAMs and promoting DC maturation and ZGd-NRs sensitized radiotherapy can significantly suppress distant tumor growth. This effect is further amplified by PD-L1 blockers (66). In clinical practice, RT-induced ICD has been limited to only inducing modest levels of ICD. Therefore, Wang et al. constructed a Cu-based mixed-valent (Cu+/Cu2+) nanoscale coordination polymer (Cu-NCPs) that can generate hydroxyl radicals and deplete GSH, thus enhancing the generation of RT-induced ICD (104). In recent years, Gold nanoparticles (AuNPs) have been used extensively in radiotherapy sensitization studies, which can catalyze the generation of free radicals and very low-energy electrons to enhance the sensitivity of DNA to ionizing radiation on the one hand, and enhance radiation damage effects through oxidative stress, cell cycle blockade, and inhibition of DNA repair on the other hand (105–107). It’s true that AuNPs can enhance the efficacy of cancer radiotherapy by absorbing radiation energy locally in tumors, while protecting surrounding normal tissues from radiation toxicity. Branislava Janic et al. found that 14-nm AuNPs significantly enhance RT-induced ICD and increase macrophage infiltration in tumor tissue. The delayed tumor growth and improved overall survival revealed additional underlying immunological mechanisms and provided a platform for studying a multimodal approach to RT in triple negative breast cancer (65).
PDT is a therapeutic modality based on photosensitizers (PS) and oxygen within the tumor tissue. After selective accumulation of PS in the tumor, appropriate wavelengths of visible light are used to excite the PS. This leads to the production of ROS within the tumor, resulting in cell death and destruction of the tumor tissue (108). This non-invasive treatment produces fewer side effects. To enhance the efficacy of PDT, emerging strategies include hypoxic reversal nanomedicine and multi-functional photosensitizers (109). For instance, Huang Cong et al. designed a nanoparticle CaO2@CuS-MnO2@HA (CCMH) for breast cancer therapy. CaO2 reacts with H2O to produce a large amount of oxygen, which promotes CuS-mediated PDT, leading to ICD. In vivo experiments combining anti-PD-L1 and CCMH effectively increased the matured DCs, M1 macrophages, and CD8+ T cells in tumor tissue, indicating it a promising treatment (110). For another, a rational design of photosensitizer-based nanoplatform (CAM-NPs) was constructed with the help of human serum albumin (HSA), composed of chlorin e6 (Ce6, a photosensitizer), axitinib (AXT, a tyrosine kinase inhibitor) and dextro-1-methyl tryptophan (1MT, an IDO inhibitor). CAM-NPs treated cells after laser irradiation both secreted significant ICD biomarkers (CRT, ATP and HMGB1) and reverse the hypoxic situation, which proved its excellent performance for immunotherapy (111). The regulation of TME greatly enhances immunotherapy. LIC, a multifunctional nanodrug encapsulating IPI-549 (a PI3Kγ inhibitor to inhibit MDSCs) and Ce6, can penetrate CT26 cells to induce ROS and ICD production. Additionally, LIC reprograms the TIME by decreasing the amount of MDSCs, Tregs, and M2-TAMs as well as increasing the maturity of DC and the infiltration of CD8+ T cells (112). Smart nanoparticles provide convenience to drug delivery. PH-dependent smart nanomaterials (M(a)D@PI-PEG-RGD) are proportionally loaded with doxorubicin, NH4HCO3, and PS while highly targeting the RGD modification. The decomposition of NH4HCO3 stimulated by PH into bubbles hastens the doxorubicin’s release. Additionally, the combination of M(a)D@PI-PEG-RGD and PDT significantly inhibits tumor growth, it provides exploration value for immunotherapy research (113). More importantly, photosensitizers with fluorescent emission enable molecular image-guided PDT/immunotherapy. This integrated approach to diagnosis and treatment has drawn a lot of attention for its time and cost-effectiveness. Liu Qiang et al. designed self-assembled nanomaterials that encapsulated photosensitized agent BDP-I-N and anti-PD-L1 drugs. Immunotherapy guidance can be done through real-time imaging of PD-L1 immune checkpoint in the NIR II window (1000-1700nm). These NPs produced singlet oxygen to induce tumor elimination, and no evident toxicity was observed in mice with MC38 tumors (114). Several powerful fluorescent photosensitizers, such as porphyrins (115), aggregation-induced emission (AIE) (116), and iodinated cyanine dyes (117), have demonstrated satisfactory performance in mouse models. Multimodal therapy is necessary when needed. For instance, a multifunctional nanoplatform that utilizes single aggregation-induced emission luminogen (AIEgen) and EPR effects is capable of performing image-guided surgery-PTT/PDT, while the use of PD-L1 antibodies produces a satisfactory immunotherapy effect. During the treatment, AIEgen generates ROS, which helps to facilitate the ICD process (118). More recently, an interesting research found that PDT-induced P53 can reprogram M2 TAM into M1 TAM, providing us with new ideas for PDT-enhanced tumor immunity (119).
PTT is a method of selectively ablating tumors through the conversion of near-infrared (NIR) laser energy into heat therapy using a photothermal agent. However, tumor recurrence and metastasis are often associated with unequal heat distribution on the tumor surface and tumor immunosuppressive microenvironment (78). NDDS use their advantages to break these limitations. Zhaowei Li et al. designed a multifunctional biomimetic nanoplatform called AuDRM with both pH and temperature stimulation response characteristics. Their results showed that laser irradiation can induce ICD through PTT by increasing the tumor temperature. In addition, the release of R837, an immunostimulant, and tumor antigen from the nanomaterial enhanced immunotherapeutic effects and long-term immunological memory. Furthermore, the shedding of pH-sensitive membranes increases exposure to gold nanoparticles, effectively releasing R837 to enhance immunotherapy (79). HA-AuNR/M-M2pep NP, which is a gold nanorod modified with hyaluronic acid (HA), can cleave the MMP2-sensitive peptide on the surface of tumor cells, leading to the release of M2pep, the selective consumption of M2-TAM, the recruitment of TILs, the activation of T cells, and the secretion of anti-tumor cytokines (like IFN-γ and TNF-α). Moreover, HA exhibits excellent biocompatibility, biodegradability, and CD44 receptor binding affinity. HA-AuNR accurately targets tumor tissues (because the tumor cells express CD44 at a high level) under NIR laser for PTT, stimulating the ICD of tumor cells and anti-tumor immunity (77). This EPR effect is effective in several cancer models. After HA modification, black phosphorus (BP) acts as a PTT/PDT reagent exhibiting strong electrical conductivity, strong optical properties, and low toxicity, boosting the EPR effect at tumor sites. Regarding in vitro and in vivo experiments based on 4T1 cell lines, the HA-BP granules exhibited exceptional imaging capability and PTT treatment efficiency. Additionally, HA-BP can induce M2-to-M1 polarization of macrophages, leading to the induction of ICD (70). Nevertheless, obtaining complete tumor remission through PTT/PDT alone is challenging. Hence, the combination of nano-based PTT/PDT with other treatments, mainly immunotherapy, is regarded as having better clinical prospects. For example, Liangjie Jin’s team has designed a corn-like Au/Ag nanorod (NR) that can provoke tumor ICD at the NIR-II window (1064 nm), increase tumor T cells infiltration significantly, and change ‘cold’ tumors into ‘hot’ ones. The combination of CTLA4 antibody and Au/Ag-NRs-based PTT/PDT can generate a robust immune memory effect, preventing the recurrence of breast tumors and distant metastasis (120). The similar combination also proved perfect clinical effect in TNBC (80).
SDT is an emerging non-invasive and deep tissue penetrating therapeutic method with great potential in the treatment of tumors. SDT mainly kills tumor cells by producing ROS through ultrasound waves (121). Currently, small molecule sonosensitizers face issues such as poor stability and ROS generation performance. To improve the therapeutic effects of sonosensitizers, researchers have developed several nano-sonosensitizers, including Au-MnO and Au-TiO2 (122–124). Nevertheless, these sonosensitizers have low efficiency in killing tumor cells, exhibit poor tissue selectivity due to their sustained pharmacological activity, and may cause off-target toxicity. Future explorations should focus on multifunctional nanomaterials and novel sonosensitizers. IRO@FA NPs, a complex sonosensitizer nanoparticle, has a perfluorohexane core and shells of IR780, PLGA, PEG, and FA (PLGA: poly (lactic-co-glycolic) acid; PEG: polyethylene glycol; FA: folate). IRO@FA NPs generate enough ROS upon ultrasound irradiation and produce DAMPs, inducing ICD in ID8 ovarian cancer cells. In vivo results revealed that IRO@FA NP-mediated SDT caused the infiltration of CD3+ T and CD8+ T cells, leading to a significant up-regulation of PD-L1 expression, potentially assisting ICI treatment (81). Xuan Tan’s research team designed a novel core-shell transformable nano sonosensitizer, TiO2@CaP. TiO2@CaP significantly enhanced ICD, T-cell recruitment and infiltration, and transforms immunogenic ‘cold’ tumors into ‘hot’ tumors. In combination with anti-PD-1, TiO2@CaP-mediated sonodynamic therapy suppressed lung metastases and untreated distant tumor growth (125).
In conclusion, nanomaterials play a powerful role as novel drug delivery systems in ablative therapy (RT, PDT, PTT and SDT etc.). Taking full advantage of the physical properties and easy design properties of nanomaterials can enhance ICD production and significantly increase the immune response. Finally, the combination of ICIs can further enhance the efficacy of systemic therapy and reduce the toxic side effects, tumor recurrence and metastasis.
4.1.3 Nano-based gene editing
Gene editing technology permits precise regulation of target gene expression in immune cells, boosting the immune response. The nano drug delivery system is the current most promising carrier, circumventing the instability and large size of gene editing products and accurately delivering target genes to cells (126). Clustered regularly interspaced short palindromic repeat-associated nuclease 9 (CRISPR/cas9) and small interfering RNA (siRNA) frequently intercept the expression of immune-regulating genes. Initially, IDO1 siRNA-based NPs reversed IDO1-induced immunosuppression and strengthened the ICD-induced immune response. This tumor-fighting approach demonstrated efficacy in colorectal and orthotopic pancreatic tumor models (84). Menghao Shi et al. designed a novel charge-switchable, acid-triggered nanoparticle incorporating IDO1 siRNA and mitoxantrone in 2022. Under the acidity conditions of TME, the drug is released efficiently, which promotes the maturation of DC cells, improves the infiltration of CTLs and down-regulates the number of Tregs, and finally causes strong anti-tumor immune response (85). Conversely, SDT sound-sensitive agents have the potential to generate ROS and contribute to ICD. However, the naturally occurring REDOX regulatory pathway in tumor cells can weaken the effect of ROS and other harmful substances and thus develop resistance to SDT therapy. The classical deoxidation signaling pathway mediated by nuclear factor (erythroid-derived 2)-like 2 (Nrf2) was discovered to cause tumor cell resistance to PDT or SDT through persistent ROS consumption (127). Therefore, we hypothesize that Nrf2 knockout can reverse this resistance phenomenon. In 2021, TIR@siRNA was designed to deliver Nrf2-siRNA into the cytoplasm of CT26 cells, inducing the downregulation of Nrf2, DNA damage, and cell apoptosis, significantly improving SDT cytotoxicity and ICD induction. In vivo experiments, the use of TIR@siRNA greatly enhanced the antitumor effect of SDT and even stimulated the tumor immune response to enhance PD-L1 therapy in patients (86). In several laboratories, nanomaterials have been employed in the most popular chemo-immunotherapy regimen for many solid tumors to deliver PD-L1 knockout genes and chemotherapy drugs, demonstrating satisfactory efficacy. Ling Xiang et al. examined a type of nanoscale coordination polymer particles that, through the property of low-pH spurt inducing excessive osmotic pressure in endo/lysosomes, effectively released PD-L1 siRNA, carboplatin, and digitoxin into the cytoplasm. The expression of ICD markers (CRT, Hsp70) and the percentages of CD3ϵ+CD4+ Helper T cells (Ths) and CD3ϵ+CD8a+ Cytotoxic T cells (Tcs) were notably increased with triple therapy, suggesting a possible combination therapy for advanced and aggressive tumors (87).
4.2 NDDS enhances immunotherapy by regulating TIME in an ICD-independent way
The TIME is a complex milieu of competing immune promotion and suppression. In many tumors, the recruitment of immunosuppressive cells such as MDSCs, TAMs, and Tregs while diminishing the proportion of essential immune cells such as CTLs, NKs, and DCs creates a TIME that ultimately leads to resistance to immunotherapy (128). Furthermore, hypoxia and acidic environments are also major obstacles to immunotherapy. We have already discussed several NDDS-based strategies to improve immunotherapy primarily by inducing ICD, but also by improving the immune microenvironment. In fact, reversing the immunosuppressive microenvironment is indeed very important for immunotherapy. Next, we will discuss NDDS-based strategies for reversing immunosuppression independent of ICD process. It mainly includes: 1) enhance the infiltration of immune cells; 2) reduce the number and function of immunosuppressive cells; 3) improve hypoxic environment; 4) activate important immune-related signaling pathways (Figure 5).
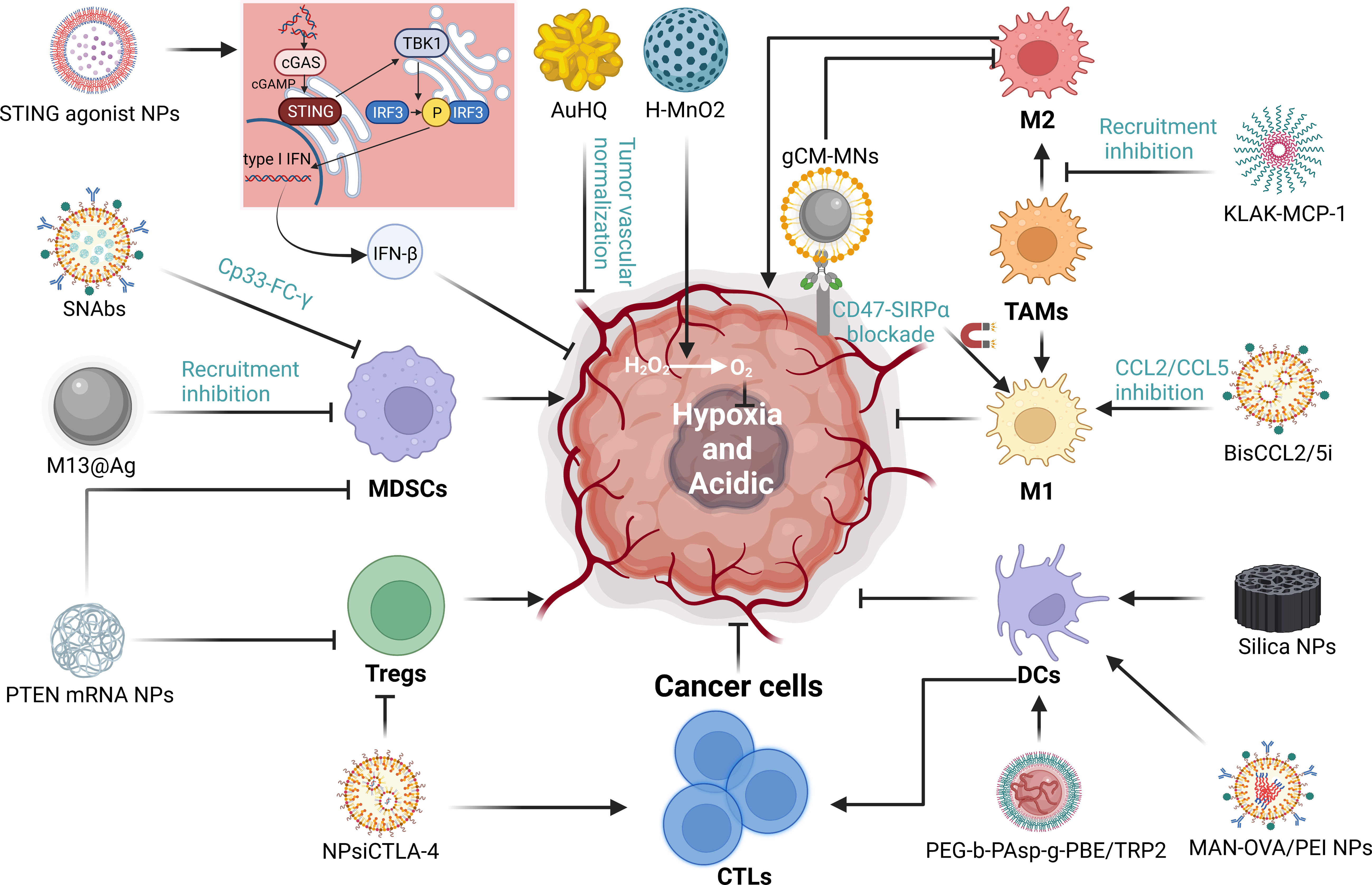
Figure 5 The mechanisms of NDDS regulating tumor immune microenvironment (TIME). We summarized the mechanisms by which nanoparticles improve the immunosuppressive microenvironment through targeting immune cells, hypoxia and and acidity environment and significant signaling. “→” represents the pointing and promoting effect. “⊥” represents the inhibiting effect.
4.2.1 Immune cells
MDSCs are heterogeneous and immature cells of bone marrow origin that can be found in almost all types of tumors and malignant or pathological conditions (e.g., infections, autoimmune diseases, and trauma). Within tumors, MDSCs are triggered by pro-inflammatory mediators that inhibit T-cell activation and encourage tumor immune tolerance and growth (129). Nanodelivery systems can induce significant changes in the number and function of MDSCs, reducing their immunosuppressive effects. Some studies suggest that the loss of phosphatase and tensin homolog (PTEN) leads to the accumulation of MDSCs and Tregs (130). In one study, a polymeric nanoparticle encapsulating PTEN mRNA showed promise in both melanoma and prostate cancer models, where it was able to restore PTEN function in cancer cells, leading to the observed reduction in MDSCs and Tregs numbers (131). Recent studies have indicated that microbiota influences tumor immunology. Specifically, Fusobacterium nucleatum (Fn), commonly found in colorectal cancer, selectively amplifies MDSCs (132). To inhibit Fn function, silver nanoparticles (AgNP) were electrostatically assembled around the Fn-binding M13 phage surface capsid protein (M13@Ag) to achieve a specific clearance of Fn, ultimately reducing MDSC numbers. Additionally, M13@Ag nanoparticles also stimulated antigen-presenting cells (APCs) to further activate the host immune response (133). Impressively, circulating MDSCs in a TNBC mouse model were completely depleted by Synthetic Nanoparticle Antibodies (SNAbs). SNAbs are engineered with cp33 peptide, a human IgG1 Fc mimicking ligand that binds to FC-γ receptors (FC-γ rs) on immune effector cells and can precisely target MDSCs (134).
TAMs, one of the most abundant immune cell populations in the TME, display heterogeneity and differentiation plasticity, and can transit between anti-tumor and pro-tumor states. M1 TAMs are known to be anti-tumoral while M2 TAMs are pro-tumoral (135). TAMs play a significant role in various malignant solid tumors such as breast, prostate, liver, lung, ovarian, gastric, pancreatic, and colorectal cancers. Notably, the presence of M2 is associated with a poorer prognosis for tumors (136–140). Numerous studies found that nanoparticles can efficiently limit TAMs survival and recruitment, and repolarize M2 into the M1 (141). For instance, a lipid NPs encapsulating mRNA encoding CCL2/CCL5 inhibitor reversed immune suppression in liver cancer by inducing M1 polarization. Moreover, in conjunction with anti-PD-L1, it significantly improved the survival in mice with primary hepatocellular, colorectal, and pancreatic cancers with liver metastasis (142). Gene editing nanoparticles also offer novel ideas (143). Cancer cells express CD47, which binds to a signal-regulatory protein α (SiRP-α) receptor on macrophages to protect them from phagocytosis (144, 145). Lang Rao’s team designed genetically edited cell membrane-coated magnetic nanoparticles (gCM-MNs). The gCM shell overexpresses the SIRPα variant at the gene level with a 50,000-fold enhanced affinity for CD47, effectively blocking the CD47-SIRPα signaling pathway, while the MN nucleus promotes M2 to M1 repolarization, restores macrophage phagocytosis of tumor cells and triggers antitumor T cells immunity (146). Recently, a KLAK-MCP-1 micelle composed of CCR2-targeted peptide sequence and apoptosis-inducing KLAK peptide inhibited melanoma growth in B16F10 mouse models by inhibiting MCP-1/CCR2 axis and decreasing the recruitment of TAMs (147). Although numerous studies have demonstrated that nanoparticles can enhance anti-tumor efficacy, their use has not yet been widely used in clinical settings, and further clinical trials are necessary to establish their safety and efficacy in humans.
Tregs can suppress aberrant immune responses against autoantigens and anti-tumor immunity (148). Tregs infiltrating tumors have been detected in different types of cancer, such as pancreatic, liver, gastrointestinal, and lung cancers. Their abundance is often related to a poor clinical prognosis (149, 150). Recent studies have provided increasing evidence that blocking or eliminating Tregs cells promotes anti-tumor immune responses (151, 152). Immunotherapy can successfully target Tregs through specific molecules like CTLA-4, GITR, CCR4, PD-1, OX-40, and LAG3 (148). In a subset of cancer patients with a poor prognosis, the inhibition of PD-1 and CTLA-4 has increased their progression-free survival (153). Nanoparticles are being developed using NDDS technology to precisely target Tregs. Early in 2016, Li et al. have designed a nanoparticle (NPsiCTLA-4) that could distribute the CTLA-4-siRNA to the CD4+ and CD8+ T cell subsets present in the tumor microenvironment. Their study demonstrated a significant increase in the percentage of CD8+ T cells, which led to a decrease in the number of Tregs in tumor-infiltrating lymphocytes (TIL) (154). Future investigations on immunotherapy approaches to target Tregs with nanoparticles hold significant potential.
DCs play a crucial role in regulating the adaptive immune response and are vital for T-cell-mediated immunity against cancer. Tumor-associated conventional dendritic cells (cDCs) are responsible for endocytosing dead tumor cells or cellular debris and transporting cancer-associated antigens to draining lymph nodes, where T-cell initiation and activation occur (155, 156). DC vaccines are often used to enhance the anti-tumor ability of the immune system by increasing the number of DCS in TME and promoting DCs antigen presentation. Nevertheless, various technical problems impede the delivery of vaccine antigens to DCs, which limit the effectiveness of therapeutic interventions due to antigen uptake and presentation insufficiency by antigen-presenting cells (157–159). NPs exhibit tremendous potential as delivery systems for cancer vaccines by facilitating the co-delivery of tumor-associated antigens and adjuvants to DCs. Mesoporous silica nanoparticles with extra-large pores have been shown to stimulate DC activation and enhance antigen presentation, increase the secretion of pro-inflammatory cytokines, and inhibit tumor growth in both in vitro and in vivo studies (158). Pei et al. designed Mannose-functionalized antigen nanoparticles (MAN-OVA/PEI NPs) with the ability to escape the endosome for targeting DCs. Their study shows that MAN-OVA/PEI NPs significantly improved antigen uptake by DCs, caused cytoplasmic antigen release, and stimulated cytokine production and DC maturation considerably in vitro (159). Wang et al. developed a PBE (Phenyl Borate)-modified TRP2 nanovaccine that infiltrates the lymph nodes, absorbed by DCs, and triggers DCs maturation. Unlike conventional cancer vaccines, the TRP2 nanovaccine also overcomes standard cancer vaccines’ challenges and stimulates the body’s most potent T-cell immune response to melanoma without an external adjuvant or antitumor activity (160).
4.2.2 Targeting Hypoxia and acidity
The tumor microenvironment is characterized by hypoxia and acidity, which are attributed to the rapid proliferation of tumor cells, increased metabolic rate, and inadequate blood supply (161, 162). Hypoxia frequently leads to therapeutic resistance, including immunotherapy (163),chemotherapy (164), RT (165), PDT (166) etc. Multifunctional nanomaterials can enhance oxygen-dependent therapies such as RT, PDT and SDT by improving the anoxic and acidic microenvironment of tumors, which have been discussed in section 4.1. MnO2-based nanomaterials to improve hypoxia are the most frequently used in research (167, 168). However, in some tumors, increasing the O2 concentration alone is not enough, because the antioxidant substances (e.g., GSH) present in cancer cells also consume oxygen. Therefore, on the one hand, nanoparticles made of MnO2 can neutralize GSH with their own oxidative properties, and on the other hand, co-delivery of Bcl-2 inhibitors can contribute to reduce GSH (169). Lastly, tumor vascular normalization has emerged as a novel strategy for enhancing tumor immunity by reducing hypoxia and increasing perfusion (170). Wang and colleagues developed a protocol to reduce the expression of angiopoietin-2, VEGF, and bFGF in human umbilical vein endothelial cells using 8-hydroxyquinoline-modified gold nanomaterials (AuHQ), which inhibited the production of ROS in tumor cells by chelating iron ions. In vivo, AuHQ regulates tumor leakage, reduces tumor hypoxia, and increases blood perfusion, thereby inducing normalization of tumor vasculature (171). Despite the challenges faced by nanotechnologies regarding their safety and the stability of their effects, they possess great potential and merit continued attention and exploration.
4.2.3 Significant signaling
Stimulator of interferon genes (STING) signaling is a promising target for tumor immunotherapy. STING was discovered as a crucial molecule in the innate immune response in 2008 (172). The cGAS-STING pathway resides in the endoplasmic reticulum. Upon receiving exogenous DNA, cGAS produces cyclic dinucleotides (CDNs) that activate STING. This leads to the formation of a tetramer that recruits TANK-binding kinase 1 (TBK1) proteins to Golgi, causing TBK1 to phosphorylate IRF3 and inducing the production of type I interferon (especially IFN-β). This process also activates multiple pro-inflammatory factors and chemokines (such as CXCL10 and CCL5), which attract T cells and natural killer cells (173). As a result, STING activators have been developed to regulate the immune microenvironment and achieve anti-cancer effects. At present, cyclic dinucleotides (ADU-S100, MK-1454) (174, 175) and macrocyclic bridge drugs (E7766) (NCT04144140) have entered clinical studies, but they are associated with significant transportation issues and side effects. This problem can be solved by NDDS technology (176). Compared with non-NPs administration, nanomaterial encapsulated drugs increased the half-life by 40 times, took advantage of EPR effect to accumulate drug concentration in the tumor, and increased the number of immune cells (CD4+ and CD8+ T cells) by 20 times. In addition, combined PD-1/PD-L1 drugs significantly reduced tumor burden in melanoma and breast cancer (177). Not only that, multifunctional nanomaterials and new therapeutic strategies are also under investigation. For example, Su Ting et al. designed a PH-responsive nanovaccine that delivers both tumor-specific antigen and STING agonist, selectively generating IFN response, enhancing DCs antigen presentation and sustained T cells response (178). Notably, Mn2+ is said to increase the STING agonist effect by 12-77 times. Their self-assembled nanoparticles effectively deliver them to tumor tissues, exerting powerful anti-tumor immunity, suggesting the potential of combining nanomedical and metal immunotherapy (179). More recently, some researchers have abandoned STING agonists because of its poor efficacy. Instead, a method was proposed to activate STING pathway in situ using nanoparticles to deliver DNA-targeted chemotherapy drugs (SN38-NPs). SN38-NPs causes DNA damage and leakage within tumor cells, which is transmitted from tumor cells to DCs via DNA-containing exosomes and subsequently activates the STING pathway. This therapy significantly reduced the toxicity of free SN38 and increased the rate of tumor suppression (80%) (180). Nanomaterial delivery systems have broad clinical application prospects for the activation of signaling pathways.
6 Conclusion and prospects
For the treatment of solid tumors, immune checkpoint inhibitors, targeted drugs, chemotherapy drugs, ablative surgery and their combination therapy are the current mainstream. Among them, immunotherapy is the paramount. However, treatment resistance and severe side effects often occur. To address these issues, we have found that changing drug delivery routes is effective. For example, nano-drug delivery systems can enhance immune efficacy through a variety of advantages, including enhancing drug permeability and retention in tumors, improving the solubility of hydrophobic drugs, targeting drug delivery, reducing physiological barriers to drug delivery, achieving integration of diagnosis and treatment, and providing multi-functional intelligent nanoplatforms. A number of preclinical studies have found that nanoparticles enhance immune efficacy mainly by inducing tumor immunogenic cell death and improving the immunosuppressive microenvironment. Moreover, most nanoparticles-based therapies combined with immune checkpoint inhibitors tend to have better anti-tumor effects in mice. However, unfortunately, most of these successful cases have only been realized in animal trials and rarely applied to the clinic. In the future, the fine application of nanomedicine delivery systems in the clinic still faces great challenges.
Author contributions
YQ and HH designed and reviewed the article; JZ and SW were responsible for writing the article and drawing pictures; JZ was also responsible for the revision of the article. DZ summarized the tables, and XH and XW were responsible for reviewing the article. All authors contributed to the article and approved the submitted version.
Funding
This research was funded by National Natural Science Foundation of China, grant number no. 8227 3381.
Acknowledgments
We thank Peiming Yan for his support in polishing this article. The figures were created with BioRender.com.
Conflict of interest
The authors declare that the research was conducted in the absence of any commercial or financial relationships that could be construed as a potential conflict of interest.
Publisher’s note
All claims expressed in this article are solely those of the authors and do not necessarily represent those of their affiliated organizations, or those of the publisher, the editors and the reviewers. Any product that may be evaluated in this article, or claim that may be made by its manufacturer, is not guaranteed or endorsed by the publisher.
References
1. Chen DS, Mellman I. Oncology meets immunology: the cancer-immunity cycle. Immunity (2013) 39(1):1–10. doi: 10.1016/j.immuni.2013.07.012
2. Pan C, Liu H, Robins E, Song W, Liu D, Li Z, et al. Next-generation immuno-oncology agents: current momentum shifts in cancer immunotherapy. J Hematol Oncol (2020) 13(1):29. doi: 10.1186/s13045-020-00862-w
3. Ghansah T, Vohra N, Kinney K, Weber A, Kodumudi K, Springett G, et al. Dendritic cell immunotherapy combined with gemcitabine chemotherapy enhances survival in a murine model of pancreatic carcinoma. Cancer immunology immunotherapy CII (2013) 62(6):1083–91. doi: 10.1007/s00262-013-1407-9
4. Heinhuis KM, Ros W, Kok M, Steeghs N, Beijnen JH, Schellens JHM. Enhancing antitumor response by combining immune checkpoint inhibitors with chemotherapy in solid tumors. Ann Oncol (2019) 30(2):219–35. doi: 10.1093/annonc/mdy551
5. Ramos-Casals M, Brahmer JR, Callahan MK, Flores-Chávez A, Keegan N, Khamashta MA, et al. Immune-related adverse events of checkpoint inhibitors. Nat Rev Dis Primers (2020) 6(1):38. doi: 10.1038/s41572-020-0160-6
6. Yap TA, Parkes EE, Peng W, Moyers JT, Curran MA, Tawbi HA. Development of immunotherapy combination strategies in cancer. Cancer Discovery (2021) 11(6):1368–97. doi: 10.1158/2159-8290.Cd-20-1209
7. Jiang M, Zeng J, Zhao L, Zhang M, Ma J, Guan X, et al. Chemotherapeutic drug-induced immunogenic cell death for nanomedicine-based cancer chemo-immunotherapy. Nanoscale (2021) 13(41):17218–35. doi: 10.1039/d1nr05512g
8. Duan X, Chan C, Lin W. Nanoparticle-mediated immunogenic cell death enables and potentiates cancer immunotherapy. Angewandte Chemie (International ed English) (2019) 58(3):670–80. doi: 10.1002/anie.201804882
9. Jubair L, Lam AK, Fallaha S, McMillan NAJ. Crispr/cas9-loaded stealth liposomes effectively cleared established Hpv16-driven tumours in syngeneic mice. PloS One (2021) 16(1):e0223288. doi: 10.1371/journal.pone.0223288
10. Tie Y, Tang F, Wei YQ, Wei XW. Immunosuppressive cells in cancer: mechanisms and potential therapeutic targets. J Hematol Oncol (2022) 15(1):61. doi: 10.1186/s13045-022-01282-8
11. Simon L, Marcotte N, Devoisselle JM, Begu S, Lapinte V. Recent advances and prospects in nano drug delivery systems using lipopolyoxazolines. Int J pharmaceutics (2020) 585:119536. doi: 10.1016/j.ijpharm.2020.119536
12. Han Z, Liu G. Cest Mri trackable nanoparticle drug delivery systems. Biomed materials (Bristol England) (2021) 16(2):024103. doi: 10.1088/1748-605X/abdd70
13. Zou T, Lu W, Mezhuev Y, Lan M, Li L, Liu F, et al. A review of nanoparticle drug delivery systems responsive to endogenous breast cancer microenvironment. Eur J pharmaceutics biopharmaceutics (2021) 166:30–43. doi: 10.1016/j.ejpb.2021.05.029
14. Li JH, Huang LJ, Zhou HL, Shan YM, Chen FM, Lehto VP, et al. Engineered nanomedicines block the Pd-1/Pd-L1 axis for potentiated cancer immunotherapy. Acta pharmacologica Sin (2022) 43(11):2749–58. doi: 10.1038/s41401-022-00910-w
15. Met Ö, Jensen KM, Chamberlain CA, Donia M, Svane IM. Principles of adoptive T cell therapy in cancer. Semin immunopathology (2019) 41(1):49–58. doi: 10.1007/s00281-018-0703-z
16. Enokida T, Moreira A, Bhardwaj N. Vaccines for immunoprevention of cancer. J Clin Invest (2021) 131(9):e146956. doi: 10.1172/jci146956
17. Fukuhara H, Ino Y, Todo T. Oncolytic virus therapy: A new era of cancer treatment at dawn. Cancer Sci (2016) 107(10):1373–9. doi: 10.1111/cas.13027
18. Bonati L, Tang L. Cytokine engineering for targeted cancer immunotherapy. Curr Opin Chem Biol (2021) 62:43–52. doi: 10.1016/j.cbpa.2021.01.007
19. Graziani G, Tentori L, Navarra P. Ipilimumab: A novel immunostimulatory monoclonal antibody for the treatment of cancer. Pharmacol Res (2012) 65(1):9–22. doi: 10.1016/j.phrs.2011.09.002
20. Bagchi S, Yuan R, Engleman EG. Immune checkpoint inhibitors for the treatment of cancer: clinical impact and mechanisms of response and resistance. Annu Rev Pathol (2021) 16:223–49. doi: 10.1146/annurev-pathol-042020-042741
21. Fda approves Anti-lag3 checkpoint. Nat Biotechnol (2022) 40(5):625. doi: 10.1038/s41587-022-01331-0
22. Hiam-Galvez KJ, Allen BM, Spitzer MH. Systemic immunity in cancer. Nat Rev Cancer (2021) 21(6):345–59. doi: 10.1038/s41568-021-00347-z
23. Shimabukuro-Vornhagen A, Gödel P, Subklewe M, Stemmler HJ, Schlößer HA, Schlaak M, et al. Cytokine release syndrome. J immunotherapy Cancer (2018) 6(1):56. doi: 10.1186/s40425-018-0343-9
24. Morse MA, Gwin WR 3rd, Mitchell DA. Vaccine therapies for cancer: then and now. Targeted Oncol (2021) 16(2):121–52. doi: 10.1007/s11523-020-00788-w
25. Morris EC, Neelapu SS, Giavridis T, Sadelain M. Cytokine release syndrome and associated neurotoxicity in cancer immunotherapy. Nat Rev Immunol (2022) 22(2):85–96. doi: 10.1038/s41577-021-00547-6
26. Mitchell MJ, Billingsley MM, Haley RM, Wechsler ME, Peppas NA, Langer R. Engineering precision nanoparticles for drug delivery. Nat Rev Drug Discovery (2021) 20(2):101–24. doi: 10.1038/s41573-020-0090-8
27. Sindhwani S, Syed AM, Ngai J, Kingston BR, Maiorino L, Rothschild J, et al. The entry of nanoparticles into solid tumours. Nat materials (2020) 19(5):566–75. doi: 10.1038/s41563-019-0566-2
28. Miller MA, Zheng YR, Gadde S, Pfirschke C, Zope H, Engblom C, et al. Tumour-associated macrophages act as a slow-release reservoir of Nano-therapeutic Pt(Iv) pro-drug. Nat Commun (2015) 6:8692. doi: 10.1038/ncomms9692
29. Miller MA, Gadde S, Pfirschke C, Engblom C, Sprachman MM, Kohler RH, et al. Predicting therapeutic nanomedicine efficacy using a companion magnetic resonance imaging nanoparticle. Sci Trans Med (2015) 7(314):314ra183. doi: 10.1126/scitranslmed.aac6522
30. Petersen GH, Alzghari SK, Chee W, Sankari SS, La-Beck NM. Meta-analysis of clinical and preclinical studies comparing the anticancer efficacy of liposomal versus conventional non-liposomal doxorubicin. J Controlled release (2016) 232:255–64. doi: 10.1016/j.jconrel.2016.04.028
31. Mundargi RC, Babu VR, Rangaswamy V, Patel P, Aminabhavi TM. Nano/micro technologies for delivering macromolecular therapeutics using Poly(D,L-lactide-co-glycolide) and its derivatives. J Controlled release (2008) 125(3):193–209. doi: 10.1016/j.jconrel.2007.09.013
32. Rabinow BE. Nanosuspensions in drug delivery. Nat Rev Drug Discovery (2004) 3(9):785–96. doi: 10.1038/nrd1494
33. Buskaran K, Hussein MZ, Moklas MAM, Masarudin MJ, Fakurazi S. Graphene oxide loaded with protocatechuic acid and chlorogenic acid dual drug nanodelivery system for human hepatocellular carcinoma therapeutic application. Int J Mol Sci (2021) 22(11):5786. doi: 10.3390/ijms22115786
34. Sun J, Li Y, Wang X, Fei W, Guo J, Wang C. Entropy-driven quick loading of functional proteins in nanohydrogels for highly efficient tumor targeting therapy. ACS Appl materials interfaces (2021) 13(11):12888–98. doi: 10.1021/acsami.0c23124
35. Lin W, Ma G, Yuan Z, Qian H, Xu L, Sidransky E, et al. Development of zwitterionic polypeptide nanoformulation with high doxorubicin loading content for targeted drug delivery. Langmuir ACS J surfaces colloids (2019) 35(5):1273–83. doi: 10.1021/acs.langmuir.8b00851
36. Murugan C, Venkatesan S, Kannan S. Cancer therapeutic proficiency of dual-targeted mesoporous silica nanocomposite endorses combination drug delivery. ACS omega (2017) 2(11):7959–75. doi: 10.1021/acsomega.7b00978
37. Guo Y, Hu Y, Zheng X, Cao X, Li Q, Wei Z, et al. Self-assembled peptide nanoparticles with endosome escaping permits for co-drug delivery. Talanta (2021) 221:121572. doi: 10.1016/j.talanta.2020.121572
38. Assali M, Kittana N, Dayyeh S, Khiar N. Dual covalent functionalization of single-walled carbon nanotubes for effective targeted cancer therapy. Nanotechnology (2021) 32(20):205101. doi: 10.1088/1361-6528/abe48c
39. Liu L, Ye Q, Lu M, Chen ST, Tseng HW, Lo YC, et al. A new approach to deliver anti-cancer nanodrugs with reduced off-target toxicities and improved efficiency by temporarily blunting the reticuloendothelial system with intralipid. Sci Rep (2017) 7(1):16106. doi: 10.1038/s41598-017-16293-6
40. Bao G, Mitragotri S, Tong S. Multifunctional nanoparticles for drug delivery and molecular imaging. Annu Rev Biomed Eng (2013) 15:253–82. doi: 10.1146/annurev-bioeng-071812-152409
41. Sethuraman V, Janakiraman K, Krishnaswami V, Kandasamy R. Recent progress in stimuli-responsive intelligent nano scale drug delivery systems: A special focus towards Ph-sensitive systems. Curr Drug Targets (2021) 22(8):947–66. doi: 10.2174/1389450122999210128180058
42. Hu Chuan SY, Huile G. Advances in research on tumor microenvironment-response nano drug delivery systems for tumor immunotherapy. Prog Pharm Sci (2022) 46(07):485–94.
43. Krysko DV, Garg AD, Kaczmarek A, Krysko O, Agostinis P, Vandenabeele P. Immunogenic cell death and damps in cancer therapy. Nat Rev Cancer (2012) 12(12):860–75. doi: 10.1038/nrc3380
44. Ahmed A, Tait SWG. Targeting immunogenic cell death in cancer. Mol Oncol (2020) 14(12):2994–3006. doi: 10.1002/1878-0261.12851
45. Panaretakis T, Kepp O, Brockmeier U, Tesniere A, Bjorklund AC, Chapman DC, et al. Mechanisms of pre-apoptotic calreticulin exposure in immunogenic cell death. EMBO J (2009) 28(5):578–90. doi: 10.1038/emboj.2009.1
46. Garg AD, Martin S, Golab J, Agostinis P. Danger signalling during cancer cell death: origins, plasticity and regulation. Cell Death differentiation (2014) 21(1):26–38. doi: 10.1038/cdd.2013.48
47. Elliott MR, Chekeni FB, Trampont PC, Lazarowski ER, Kadl A, Walk SF, et al. Nucleotides released by apoptotic cells act as a find-me signal to promote phagocytic clearance. Nature (2009) 461(7261):282–6. doi: 10.1038/nature08296
48. Martins I, Wang Y, Michaud M, Ma Y, Sukkurwala AQ, Shen S, et al. Molecular mechanisms of Atp secretion during immunogenic cell death. Cell Death differentiation (2014) 21(1):79–91. doi: 10.1038/cdd.2013.75
49. Pol J, Vacchelli E, Aranda F, Castoldi F, Eggermont A, Cremer I, et al. Trial watch: immunogenic cell death inducers for anticancer chemotherapy. Oncoimmunology (2015) 4(4):e1008866. doi: 10.1080/2162402x.2015.1008866
50. Rufo N, Garg AD, Agostinis P. The unfolded protein response in immunogenic cell death and cancer immunotherapy. Trends Cancer (2017) 3(9):643–58. doi: 10.1016/j.trecan.2017.07.002
51. Wang Q, Ju X, Wang J, Fan Y, Ren M, Zhang H. Immunogenic cell death in anticancer chemotherapy and its impact on clinical studies. Cancer Lett (2018) 438:17–23. doi: 10.1016/j.canlet.2018.08.028
52. Garg AD, Galluzzi L, Apetoh L, Baert T, Birge RB, Bravo-San Pedro JM, et al. Molecular and translational classifications of damps in immunogenic cell death. Front Immunol (2015) 6:588. doi: 10.3389/fimmu.2015.00588
53. Guo J, Huang L. Membrane-core nanoparticles for cancer nanomedicine. Advanced Drug delivery Rev (2020) 156:23–39. doi: 10.1016/j.addr.2020.05.005
54. Guo J, Yu Z, Sun D, Zou Y, Liu Y, Huang L. Two nanoformulations induce reactive oxygen species and immunogenetic cell death for synergistic chemo-immunotherapy eradicating colorectal cancer and hepatocellular carcinoma. Mol Cancer (2021) 20(1):10. doi: 10.1186/s12943-020-01297-0
55. Sun D, Zou Y, Song L, Han S, Yang H, Chu D, et al. A cyclodextrin-based nanoformulation achieves co-delivery of ginsenoside Rg3 and quercetin for chemo-immunotherapy in colorectal cancer. Acta Pharm Sin B (2022) 12(1):378–93. doi: 10.1016/j.apsb.2021.06.005
56. Zhang Q, Kuang G, Yu Y, Ding X, Ren H, Sun W, et al. Hierarchical microparticles delivering oxaliplatin and Nlg919 nanoprodrugs for local chemo-immunotherapy. ACS Appl materials interfaces (2022) 14(43):48527–39. doi: 10.1021/acsami.2c16564
57. Liu X, Jiang J, Liao YP, Tang I, Zheng E, Qiu W, et al. Combination chemo-immunotherapy for pancreatic cancer using the immunogenic effects of an irinotecan silicasome nanocarrier plus anti-Pd-1. Advanced Sci (Weinheim Baden-Wurttemberg Germany) (2021) 8(6):2002147. doi: 10.1002/advs.202002147
58. Zhu W, Bai Y, Zhang N, Yan J, Chen J, He Z, et al. A tumor extracellular Ph-sensitive Pd-L1 binding peptide nanoparticle for chemo-immunotherapy of cancer. J materials Chem B (2021) 9(20):4201–10. doi: 10.1039/d1tb00537e
59. Liu D, Li K, Gong L, Fu L, Yang D. Charge reversal yolk-shell liposome co-loaded Jq1 and doxorubicin with high drug loading and optimal ratio for synergistically enhanced tumor chemo-immunotherapy via blockade Pd-L1 pathway. Int J pharmaceutics (2023) 635:122728. doi: 10.1016/j.ijpharm.2023.122728
60. Zhang J, Sun X, Zhao X, Yang C, Shi M, Zhang B, et al. Combining immune checkpoint blockade with Atp-based immunogenic cell death amplifier for cancer chemo-immunotherapy. Acta Pharm Sin B (2022) 12(9):3694–709. doi: 10.1016/j.apsb.2022.05.008
61. Long X, Wang H, Yan J, Li Y, Dong X, Tian S, et al. Tailor-made autophagy cascade amplification polymeric nanoparticles for enhanced tumor immunotherapy. Small (Weinheim an der Bergstrasse Germany) (2023) 19:e2207898. doi: 10.1002/smll.202207898
62. Wang R, Xu X, Li D, Zhang W, Shi X, Xu H, et al. Smart Ph-responsive polyhydralazine/bortezomib nanoparticles for remodeling tumor microenvironment and enhancing chemotherapy. Biomaterials (2022) 288:121737. doi: 10.1016/j.biomaterials.2022.121737
63. Xu X, Wang R, Li D, Xiang J, Zhang W, Shi X, et al. Guanidine-modified nanoparticles as robust Btz delivery carriers and activators of immune responses. J Controlled release (2023) 357:310–8. doi: 10.1016/j.jconrel.2023.04.004
64. Wang Y, Shen N, Wang Y, Li M, Zhang W, Fan L, et al. Cisplatin nanoparticles boost abscopal effect of radiation plus anti-Pd1 therapy. Biomaterials Sci (2021) 9(8):3019–27. doi: 10.1039/d1bm00112d
65. Janic B, Brown SL, Neff R, Liu F, Mao G, Chen Y, et al. Therapeutic enhancement of radiation and immunomodulation by gold nanoparticles in triple negative breast cancer. Cancer Biol Ther (2021) 22(2):124–35. doi: 10.1080/15384047.2020.1861923
66. Huang Z, Yao D, Ye Q, Jiang H, Gu R, Ji C, et al. Zoledronic acid-gadolinium coordination polymer nanorods for improved tumor radioimmunotherapy by synergetically inducing immunogenic cell death and reprogramming the immunosuppressive microenvironment. ACS nano (2021) 15(5):8450–65. doi: 10.1021/acsnano.0c10764
67. Huang Z, Chen Y, Zhang J, Li W, Shi M, Qiao M, et al. Laser/gsh-activatable oxaliplatin/phthalocyanine-based coordination polymer nanoparticles combining chemophotodynamic therapy to improve cancer immunotherapy. ACS Appl materials interfaces (2021) 13(33):39934–48. doi: 10.1021/acsami.1c11327
68. Pan P, Dong X, Chen Y, Ye JJ, Sun YX, Zhang XZ. A heterogenic membrane-based biomimetic hybrid nanoplatform for combining radiotherapy and immunotherapy against breast cancer. Biomaterials (2022) 289:121810. doi: 10.1016/j.biomaterials.2022.121810
69. Zhu X, Wang X, Li B, Zhang Y, Chen Y, Zhang W, et al. A three-in-one assembled nanoparticle containing peptide-radio-sensitizer conjugate and Tlr7/8 agonist can initiate the cancer-immunity cycle to trigger antitumor immune response. Small (Weinheim an der Bergstrasse Germany) (2022) 18(20):e2107001. doi: 10.1002/smll.202107001
70. Zhang X, Tang J, Li C, Lu Y, Cheng L, Liu J. A targeting black phosphorus nanoparticle based immune cells nano-regulator for photodynamic/photothermal and photo-immunotherapy. Bioactive materials (2021) 6(2):472–89. doi: 10.1016/j.bioactmat.2020.08.024
71. Xie BR, Yu Y, Liu XH, Zeng JY, Zou MZ, Li CX, et al. A near infrared ratiometric platform based Π-extended porphyrin metal-organic framework for O(2) imaging and cancer therapy. Biomaterials (2021) 272:120782. doi: 10.1016/j.biomaterials.2021.120782
72. Liang R, Liu L, He H, Chen Z, Han Z, Luo Z, et al. Oxygen-boosted immunogenic photodynamic therapy with gold nanocages@Manganese dioxide to inhibit tumor growth and metastases. Biomaterials (2018) 177:149–60. doi: 10.1016/j.biomaterials.2018.05.051
73. Liu D, Huang H, Zhao B, Guo W. Natural melanin-based nanoparticles with combined chemo/photothermal/photodynamic effect induce immunogenic cell death (Icd) on tumor. Front bioengineering Biotechnol (2021) 9:635858. doi: 10.3389/fbioe.2021.635858
74. Sun Q, Yang Z, Lin M, Peng Y, Wang R, Du Y, et al. Phototherapy and anti-Gitr antibody-based therapy synergistically reinvigorate immunogenic cell death and reject established cancers. Biomaterials (2021) 269:120648. doi: 10.1016/j.biomaterials.2020.120648
75. Liu H, Xu C, Meng M, Li S, Sheng S, Zhang S, et al. Metal-organic framework-mediated multifunctional nanoparticles for combined chemo-photothermal therapy and enhanced immunotherapy against colorectal cancer. Acta biomaterialia (2022) 144:132–41. doi: 10.1016/j.actbio.2022.03.023
76. Jiao X, Sun L, Zhang W, Ren J, Zhang L, Cao Y, et al. Engineering oxygen-deficient Zro(2-X) nanoplatform as therapy-activated "Immunogenic cell death (Icd)" Inducer to synergize photothermal-augmented sonodynamic tumor elimination in Nir-Ii biological window. Biomaterials (2021) 272:120787. doi: 10.1016/j.biomaterials.2021.120787
77. Tian D, Qin F, Zhao H, Zhang C, Wang H, Liu N, et al. Bio-responsive nanoparticle for tumor targeting and enhanced photo-immunotherapy. Colloids surfaces B Biointerfaces (2021) 202:111681. doi: 10.1016/j.colsurfb.2021.111681
78. Zhang X, Li X, Sun S, Wang P, Ma X, Hou R, et al. Anti-tumor metastasis via platelet inhibitor combined with photothermal therapy under activatable fluorescence/magnetic resonance bimodal imaging guidance. ACS Appl materials interfaces (2021) 13(17):19679–94. doi: 10.1021/acsami.1c02302
79. Li Z, Rong L. A homotypic membrane-camouflaged biomimetic nanoplatform with gold nanocrystals for synergistic photothermal/starvation/immunotherapy. ACS Appl materials interfaces (2021) 13(20):23469–80. doi: 10.1021/acsami.1c04305
80. Yasothamani V, Karthikeyan L, Shyamsivappan S, Haldorai Y, Seetha D, Vivek R. Synergistic effect of photothermally targeted Nir-responsive nanomedicine-induced immunogenic cell death for effective triple negative breast cancer therapy. Biomacromolecules (2021) 22(6):2472–90. doi: 10.1021/acs.biomac.1c00244
81. Zheng J, Sun Y, Long T, Yuan D, Yue S, Zhang N, et al. Sonosensitizer nanoplatform-mediated sonodynamic therapy induced immunogenic cell death and tumor immune microenvironment variation. Drug delivery (2022) 29(1):1164–75. doi: 10.1080/10717544.2022.2058653
82. Zhang Y, Qiu N, Zhang Y, Yan H, Ji J, Xi Y, et al. Oxygen-carrying nanoparticle-based chemo-sonodynamic therapy for tumor suppression and autoimmunity activation. Biomaterials Sci (2021) 9(11):3989–4004. doi: 10.1039/d1bm00198a
83. Chen H, Liu L, Ma A, Yin T, Chen Z, Liang R, et al. Noninvasively immunogenic sonodynamic therapy with manganese protoporphyrin liposomes against triple-negative breast cancer. Biomaterials (2021) 269:120639. doi: 10.1016/j.biomaterials.2020.120639
84. Huang H, Jiang CT, Shen S, Liu A, Gan YJ, Tong QS, et al. Nanoenabled reversal of ido1-mediated immunosuppression synergizes with immunogenic chemotherapy for improved cancer therapy. Nano Lett (2019) 19(8):5356–65. doi: 10.1021/acs.nanolett.9b01807
85. Shi M, Zhang J, Wang Y, Han Y, Zhao X, Hu H, et al. Blockage of the ido1 pathway by charge-switchable nanoparticles amplifies immunogenic cell death for enhanced cancer immunotherapy. Acta biomaterialia (2022) 150:353–66. doi: 10.1016/j.actbio.2022.07.022
86. Wan G, Chen X, Wang H, Hou S, Wang Q, Cheng Y, et al. Gene augmented nuclear-targeting sonodynamic therapy via Nrf2 pathway-based redox balance adjustment boosts peptide-based anti-Pd-L1 therapy on colorectal cancer. J nanobiotechnology (2021) 19(1):347. doi: 10.1186/s12951-021-01094-x
87. Ling X, Han W, Jiang X, Chen X, Rodriguez M, Zhu P, et al. Point-source burst of coordination polymer nanoparticles for Tri-modality cancer therapy. Biomaterials (2021) 270:120690. doi: 10.1016/j.biomaterials.2021.120690
88. Zhao X, Yang K, Zhao R, Ji T, Wang X, Yang X, et al. Inducing enhanced immunogenic cell death with nanocarrier-based drug delivery systems for pancreatic cancer therapy. Biomaterials (2016) 102:187–97. doi: 10.1016/j.biomaterials.2016.06.032
89. Qi J, Jin F, Xu X, Du Y. Combination cancer immunotherapy of nanoparticle-based immunogenic cell death inducers and immune checkpoint inhibitors. Int J nanomedicine (2021) 16:1435–56. doi: 10.2147/ijn.S285999
90. Jie Y, Yang X, Chen W. Pulsatilla decoction combined with 5-fluorouracil triggers immunogenic cell death in colorectal cancer cells. Cancer biotherapy radiopharmaceuticals (2022) 37(10):945–54. doi: 10.1089/cbr.2020.4369
91. Feng B, Zhou F, Hou B, Wang D, Wang T, Fu Y, et al. Binary cooperative prodrug nanoparticles improve immunotherapy by synergistically modulating immune tumor microenvironment. Advanced materials (Deerfield Beach Fla) (2018) 30(38):e1803001. doi: 10.1002/adma.201803001
92. Duan XC, Peng LY, Yao X, Xu MQ, Li H, Zhang SQ, et al. The synergistic antitumor activity of 3-(2-Nitrophenyl) propionic acid-paclitaxel nanoparticles (Nppa-ptx nps) and anti-Pd-L1 antibody inducing immunogenic cell death. Drug delivery (2021) 28(1):800–13. doi: 10.1080/10717544.2021.1909180
93. Xiao Y, Yao W, Lin M, Huang W, Li B, Peng B, et al. Icaritin-loaded Plga nanoparticles activate immunogenic cell death and facilitate tumor recruitment in mice with gastric cancer. Drug delivery (2022) 29(1):1712–25. doi: 10.1080/10717544.2022.2079769
94. Golden EB, Frances D, Pellicciotta I, Demaria S, Helen Barcellos-Hoff M, Formenti SC. Radiation fosters dose-dependent and chemotherapy-induced immunogenic cell death. Oncoimmunology (2014) 3:e28518. doi: 10.4161/onci.28518
95. Alzeibak R, Mishchenko TA, Shilyagina NY, Balalaeva IV, Vedunova MV, Krysko DV. Targeting immunogenic cancer cell death by photodynamic therapy: past, present and future. J immunotherapy Cancer (2021) 9(1):e001926. doi: 10.1136/jitc-2020-001926
96. Tang H, Xu X, Chen Y, Xin H, Wan T, Li B, et al. Reprogramming the tumor microenvironment through second-near-infrared-window photothermal genome editing of Pd-L1 mediated by supramolecular gold nanorods for enhanced cancer immunotherapy. Advanced materials (Deerfield Beach Fla) (2021) 33(12):e2006003. doi: 10.1002/adma.202006003
97. Zheng J, Sun J, Chen J, Zhu S, Chen S, Liu Y, et al. Oxygen and oxaliplatin-loaded nanoparticles combined with photo-sonodynamic inducing enhanced immunogenic cell death in syngeneic mouse models of ovarian cancer. J Controlled release (2021) 332:448–59. doi: 10.1016/j.jconrel.2021.02.032
98. Adkins I, Fucikova J, Garg AD, Agostinis P, Špíšek R. Physical modalities inducing immunogenic tumor cell death for cancer immunotherapy. Oncoimmunology (2014) 3(12):e968434. doi: 10.4161/21624011.2014.968434
99. Mi P. Stimuli-responsive nanocarriers for drug delivery, tumor imaging, therapy and theranostics. Theranostics (2020) 10(10):4557–88. doi: 10.7150/thno.38069
100. Day NB, Wixson WC, Shields CWT. Magnetic systems for cancer immunotherapy. Acta Pharm Sin B (2021) 11(8):2172–96. doi: 10.1016/j.apsb.2021.03.023
101. Wu Q, Allouch A, Martins I, Brenner C, Modjtahedi N, Deutsch E, et al. Modulating both tumor cell death and innate immunity is essential for improving radiation therapy effectiveness. Front Immunol (2017) 8:613. doi: 10.3389/fimmu.2017.00613
102. Rückert M, Deloch L, Fietkau R, Frey B, Hecht M, Gaipl US. Immune modulatory effects of radiotherapy as basis for well-reasoned radioimmunotherapies. Strahlentherapie und Onkologie Organ der Deutschen Rontgengesellschaft [et al] (2018) 194(6):509–19. doi: 10.1007/s00066-018-1287-1
103. Mole RH. Whole body irradiation; radiobiology or medicine? Br J Radiol (1953) 26(305):234–41. doi: 10.1259/0007-1285-26-305-234
104. Wang Y, Ding Y, Yao D, Dong H, Ji C, Wu J, et al. Copper-based nanoscale coordination polymers augmented tumor radioimmunotherapy for immunogenic cell death induction and T-cell infiltration. Small (Weinheim an der Bergstrasse Germany) (2021) 17(8):e2006231. doi: 10.1002/smll.202006231
105. Yu M, Zheng J. Clearance pathways and tumor targeting of imaging nanoparticles. ACS nano (2015) 9(7):6655–74. doi: 10.1021/acsnano.5b01320
106. Zhou P, Jia S, Pan D, Wang L, Gao J, Lu J, et al. Reversible regulation of catalytic activity of gold nanoparticles with DNA nanomachines. Sci Rep (2015) 5:14402. doi: 10.1038/srep14402
107. Yao X, Huang C, Chen X, Yi Z, Sanche L. Chemical radiosensitivity of DNA induced by gold nanoparticles. J Biomed nanotechnology (2015) 11(3):478–85. doi: 10.1166/jbn.2015.1922
108. Huis In 't Veld RV, Heuts J, Ma S, Cruz LJ, Ossendorp FA, Jager MJ. Current challenges and opportunities of photodynamic therapy against cancer. Pharmaceutics (2023) 15(2):330. doi: 10.3390/pharmaceutics15020330
109. Ji B, Wei M, Yang B. Recent advances in nanomedicines for photodynamic therapy (Pdt)-driven cancer immunotherapy. Theranostics (2022) 12(1):434–58. doi: 10.7150/thno.67300
110. Huang C, Lin B, Chen C, Wang H, Lin X, Liu J, et al. Synergistic reinforcing of immunogenic cell death and transforming tumor-associated macrophages via a multifunctional cascade bioreactor for optimizing cancer immunotherapy. Advanced materials (Deerfield Beach Fla) (2022) 34(51):e2207593. doi: 10.1002/adma.202207593
111. Zhou Y, Ren X, Hou Z, Wang N, Jiang Y, Luan Y. Engineering a photosensitizer nanoplatform for amplified photodynamic immunotherapy via tumor microenvironment modulation. Nanoscale horizons (2021) 6(2):120–31. doi: 10.1039/d0nh00480d
112. Ding D, Zhong H, Liang R, Lan T, Zhu X, Huang S, et al. Multifunctional nanodrug mediates synergistic photodynamic therapy and Mdscs-targeting immunotherapy of colon cancer. Advanced Sci (Weinheim Baden-Wurttemberg Germany) (2021) 8(14):e2100712. doi: 10.1002/advs.202100712
113. Zhang Z, Huang C, Zhang L, Guo Q, Qin Y, Fan F, et al. Ph-sensitive and bubble-generating mesoporous silica-based nanoparticles for enhanced tumor combination therapy. Acta Pharm Sin B (2021) 11(2):520–33. doi: 10.1016/j.apsb.2020.08.013
114. Liu Q, Tian J, Tian Y, Sun Q, Sun D, Wang F, et al. Near-infrared-Ii nanoparticles for cancer imaging of immune checkpoint programmed death-ligand 1 and photodynamic/immune therapy. ACS nano (2021) 15(1):515–25. doi: 10.1021/acsnano.0c05317
115. Hao K, Lin L, Sun P, Hu Y, Atsushi M, Guo Z, et al. Cationic flexible organic framework for combination of photodynamic therapy and genetic immunotherapy against tumors. Small (Weinheim an der Bergstrasse Germany) (2021) 17(19):e2008125. doi: 10.1002/smll.202008125
116. Cheng G, Zong W, Guo H, Li F, Zhang X, Yu P, et al. Programmed size-changeable nanotheranostic agents for enhanced imaging-guided chemo/photodynamic combination therapy and fast elimination. Advanced materials (Deerfield Beach Fla) (2021) 33(21):e2100398. doi: 10.1002/adma.202100398
117. Dong Y, Zhou L, Shen Z, Ma Q, Zhao Y, Sun Y, et al. Iodinated cyanine dye-based nanosystem for synergistic phototherapy and hypoxia-activated bioreductive therapy. Drug delivery (2022) 29(1):238–53. doi: 10.1080/10717544.2021.2023701
118. Jiang R, Dai J, Dong X, Wang Q, Meng Z, Guo J, et al. Improving image-guided surgical and immunological tumor treatment efficacy by photothermal and photodynamic therapies based on a multifunctional Nir aiegen. Advanced materials (Deerfield Beach Fla) (2021) 33(22):e2101158. doi: 10.1002/adma.202101158
119. Cheng H, Fan X, Ye E, Chen H, Yang J, Ke L, et al. Dual tumor microenvironment remodeling by glucose-contained radical copolymer for Mri-guided photoimmunotherapy. Advanced materials (Deerfield Beach Fla) (2022) 34(25):e2107674. doi: 10.1002/adma.202107674
120. Jin L, Shen S, Huang Y, Li D, Yang X. Corn-like Au/Ag nanorod-mediated Nir-Ii photothermal/photodynamic therapy potentiates immune checkpoint antibody efficacy by reprogramming the cold tumor microenvironment. Biomaterials (2021) 268:120582. doi: 10.1016/j.biomaterials.2020.120582
121. Son S, Kim JH, Wang X, Zhang C, Yoon SA, Shin J, et al. Multifunctional sonosensitizers in sonodynamic cancer therapy. Chem Soc Rev (2020) 49(11):3244–61. doi: 10.1039/c9cs00648f
122. Deepagan VG, You DG, Um W, Ko H, Kwon S, Choi KY, et al. Long-circulating Au-Tio(2) nanocomposite as a sonosensitizer for Ros-mediated eradication of cancer. Nano Lett (2016) 16(10):6257–64. doi: 10.1021/acs.nanolett.6b02547
123. Lin X, Liu S, Zhang X, Zhu R, Chen S, Chen X, et al. An ultrasound activated vesicle of Janus Au-Mno nanoparticles for promoted tumor penetration and sono-chemodynamic therapy of orthotopic liver cancer. Angewandte Chemie (International ed English) (2020) 59(4):1682–8. doi: 10.1002/anie.201912768
124. Soratijahromi E, Mohammadi S, Dehdari Vais R, Azarpira N, Sattarahmady N. Photothermal/sonodynamic therapy of melanoma tumor by a gold/manganese dioxide nanocomposite: in vitro and in vivo studies. Photodiagnosis Photodyn Ther (2020) 31:101846. doi: 10.1016/j.pdpdt.2020.101846
125. Tan X, Huang J, Wang Y, He S, Jia L, Zhu Y, et al. Transformable nanosensitizer with tumor microenvironment-activated sonodynamic process and calcium release for enhanced cancer immunotherapy. Angewandte Chemie (International ed English) (2021) 60(25):14051–9. doi: 10.1002/anie.202102703
126. Mukalel AJ, Riley RS, Zhang R, Mitchell MJ. Nanoparticles for nucleic acid delivery: applications in cancer immunotherapy. Cancer Lett (2019) 458:102–12. doi: 10.1016/j.canlet.2019.04.040
127. Ishikawa T, Kajimoto Y, Sun W, Nakagawa H, Inoue Y, Ikegami Y, et al. Role of Nrf2 in cancer photodynamic therapy: regulation of human Abc transporter Abcg2. J Pharm Sci (2013) 102(9):3058–69. doi: 10.1002/jps.23563
128. Oya Y, Hayakawa Y, Koike K. Tumor microenvironment in gastric cancers. Cancer Sci (2020) 111(8):2696–707. doi: 10.1111/cas.14521
129. Parker KH, Beury DW, Ostrand-Rosenberg S. Myeloid-derived suppressor cells: critical cells driving immune suppression in the tumor microenvironment. Adv Cancer Res (2015) 128:95–139. doi: 10.1016/bs.acr.2015.04.002
130. Yang R, Cai TT, Wu XJ, Liu YN, He J, Zhang XS, et al. Tumour Yap1 and Pten expression correlates with tumour-associated myeloid suppressor cell expansion and reduced survival in colorectal cancer. Immunology (2018) 155(2):263–72. doi: 10.1111/imm.12949
131. Lin YX, Wang Y, Ding J, Jiang A, Wang J, Yu M, et al. Reactivation of the tumor suppressor Pten by Mrna nanoparticles enhances antitumor immunity in preclinical models. Sci Trans Med (2021), 13(599). doi: 10.1126/scitranslmed.aba9772
132. Kostic AD, Chun E, Robertson L, Glickman JN, Gallini CA, Michaud M, et al. Fusobacterium nucleatum potentiates intestinal tumorigenesis and modulates the tumor-immune microenvironment. Cell Host Microbe (2013) 14(2):207–15. doi: 10.1016/j.chom.2013.07.007
133. Dong X, Pan P, Zheng DW, Bao P, Zeng X, Zhang XZ. Bioinorganic hybrid bacteriophage for modulation of intestinal microbiota to remodel tumor-immune microenvironment against colorectal cancer. Sci Adv (2020) 6(20):eaba1590. doi: 10.1126/sciadv.aba1590
134. Liu J, Toy R, Vantucci C, Pradhan P, Zhang Z, Kuo KM, et al. Bifunctional Janus particles as multivalent synthetic nanoparticle antibodies (Snabs) for selective depletion of target cells. Nano Lett (2021) 21(1):875–86. doi: 10.1021/acs.nanolett.0c04833
135. Mantovani A, Marchesi F, Malesci A, Laghi L, Allavena P. Tumour-associated macrophages as treatment targets in oncology. Nat Rev Clin Oncol (2017) 14(7):399–416. doi: 10.1038/nrclinonc.2016.217
136. Ngambenjawong C, Gustafson HH, Pun SH. Progress in tumor-associated macrophage (Tam)-targeted therapeutics. Advanced Drug delivery Rev (2017) 114:206–21. doi: 10.1016/j.addr.2017.04.010
137. Wang N, Wang S, Wang X, Zheng Y, Yang B, Zhang J, et al. Research trends in pharmacological modulation of tumor-associated macrophages. Clin Trans Med (2021) 11(1):e288. doi: 10.1002/ctm2.288
138. Weber C, Telerman SB, Reimer AS, Sequeira I, Liakath-Ali K, Arwert EN, et al. Macrophage infiltration and alternative activation during wound healing promote Mek1-induced skin carcinogenesis. Cancer Res (2016) 76(4):805–17. doi: 10.1158/0008-5472.Can-14-3676
139. Leek RD, Harris AL. Tumor-associated macrophages in breast cancer. J mammary gland Biol neoplasia (2002) 7(2):177–89. doi: 10.1023/a:1020304003704
140. Lin EY, Nguyen AV, Russell RG, Pollard JW. Colony-stimulating factor 1 promotes progression of mammary tumors to malignancy. J Exp Med (2001) 193(6):727–40. doi: 10.1084/jem.193.6.727
141. Kumari N, Choi SH. Tumor-associated macrophages in cancer: recent advancements in cancer nanoimmunotherapies. J Exp Clin Cancer Res CR (2022) 41(1):68. doi: 10.1186/s13046-022-02272-x
142. Wang Y, Tiruthani K, Li S, Hu M, Zhong G, Tang Y, et al. Mrna delivery of a bispecific single-domain antibody to polarize tumor-associated macrophages and synergize immunotherapy against liver malignancies. Advanced materials (Deerfield Beach Fla) (2021) 33(23):e2007603. doi: 10.1002/adma.202007603
143. Zins K, Abraham D. Cancer immunotherapy: targeting tumor-associated macrophages by gene silencing. Methods Mol Biol (Clifton NJ) (2020) 2115:289–325. doi: 10.1007/978-1-0716-0290-4_17
144. Kershaw MH, Smyth MJ. Immunology. Making macrophages eat cancer. Sci (New York NY) (2013) 341(6141):41–2. doi: 10.1126/science.1241716
145. Majeti R, Chao MP, Alizadeh AA, Pang WW, Jaiswal S, Gibbs KD Jr., et al. Cd47 is an adverse prognostic factor and therapeutic antibody target on human acute myeloid leukemia stem cells. Cell (2009) 138(2):286–99. doi: 10.1016/j.cell.2009.05.045
146. Rao L, Zhao SK, Wen C, Tian R, Lin L, Cai B, et al. Activating macrophage-mediated cancer immunotherapy by genetically edited nanoparticles. Advanced materials (Deerfield Beach Fla) (2020) 32(47):e2004853. doi: 10.1002/adma.202004853
147. Trac N, Chen LY, Zhang A, Liao CP, Poon C, Wang J, et al. Ccr2-targeted micelles for anti-cancer peptide delivery and immune stimulation. J Controlled release (2021) 329:614–23. doi: 10.1016/j.jconrel.2020.09.054
148. Tanaka A, Sakaguchi S. Regulatory T cells in cancer immunotherapy. Cell Res (2017) 27(1):109–18. doi: 10.1038/cr.2016.151
149. Shimizu J, Yamazaki S, Sakaguchi S. Induction of tumor immunity by removing Cd25+Cd4+ T cells: a common basis between tumor immunity and autoimmunity. J Immunol (Baltimore Md 1950) (1999) 163(10):5211–8. doi: 10.4049/jimmunol.163.10.5211
150. Nishikawa H, Sakaguchi S. Regulatory T cells in tumor immunity. Int J Cancer (2010) 127(4):759–67. doi: 10.1002/ijc.25429
151. Hodi FS, O'Day SJ, McDermott DF, Weber RW, Sosman JA, Haanen JB, et al. Improved survival with ipilimumab in patients with metastatic melanoma. New Engl J Med (2010) 363(8):711–23. doi: 10.1056/NEJMoa1003466
152. Van Damme H, Dombrecht B, Kiss M, Roose H, Allen E, Van Overmeire E, et al. Therapeutic depletion of Ccr8(+) tumor-infiltrating regulatory T cells elicits antitumor immunity and synergizes with anti-Pd-1 therapy. J immunotherapy Cancer (2021) 9(2):e001749. doi: 10.1136/jitc-2020-001749
153. Marangoni F, Zhakyp A, Corsini M, Geels SN, Carrizosa E, Thelen M, et al. Expansion of tumor-associated treg cells upon disruption of a Ctla-4-dependent feedback loop. Cell (2021) 184(15):3998–4015.e19. doi: 10.1016/j.cell.2021.05.027
154. Li SY, Liu Y, Xu CF, Shen S, Sun R, Du XJ, et al. Restoring anti-tumor functions of T cells via nanoparticle-mediated immune checkpoint modulation. J Controlled release (2016) 231:17–28. doi: 10.1016/j.jconrel.2016.01.044
155. Gardner A, Ruffell B. Dendritic cells and cancer immunity. Trends Immunol (2016) 37(12):855–65. doi: 10.1016/j.it.2016.09.006
156. Sánchez-Paulete AR, Teijeira A, Cueto FJ, Garasa S, Pérez-Gracia JL, Sánchez-Arráez A, et al. Antigen cross-presentation and T-cell cross-priming in cancer immunology and immunotherapy. Ann Oncol (2017) 28(suppl_12):xii44–55. doi: 10.1093/annonc/mdx237
157. Kranz LM, Diken M, Haas H, Kreiter S, Loquai C, Reuter KC, et al. Systemic Rna delivery to dendritic cells exploits antiviral defence for cancer immunotherapy. Nature (2016) 534(7607):396–401. doi: 10.1038/nature18300
158. Cha BG, Jeong JH, Kim J. Extra-large pore mesoporous silica nanoparticles enabling co-delivery of high amounts of protein antigen and toll-like receptor 9 agonist for enhanced cancer vaccine efficacy. ACS Cent Sci (2018) 4(4):484–92. doi: 10.1021/acscentsci.8b00035
159. Pei M, Xu R, Zhang C, Wang X, Li C, Hu Y. Mannose-functionalized antigen nanoparticles for targeted dendritic cells, accelerated endosomal escape and enhanced Mhc-I antigen presentation. Colloids surfaces B Biointerfaces (2021) 197:111378. doi: 10.1016/j.colsurfb.2020.111378
160. Wang Q, Dong Z, Lou F, Yin Y, Zhang J, Wen H, et al. Phenylboronic ester-modified polymeric nanoparticles for promoting Trp2 peptide antigen delivery in cancer immunotherapy. Drug delivery (2022) 29(1):2029–43. doi: 10.1080/10717544.2022.2086941
161. Kato Y, Ozawa S, Miyamoto C, Maehata Y, Suzuki A, Maeda T, et al. Acidic extracellular microenvironment and cancer. Cancer Cell Int (2013) 13(1):89. doi: 10.1186/1475-2867-13-89
162. Jain RK, Martin JD, Stylianopoulos T. The role of mechanical forces in tumor growth and therapy. Annu Rev Biomed Eng (2014) 16:321–46. doi: 10.1146/annurev-bioeng-071813-105259
163. Wang B, Zhao Q, Zhang Y, Liu Z, Zheng Z, Liu S, et al. Targeting hypoxia in the tumor microenvironment: A potential strategy to improve cancer immunotherapy. J Exp Clin Cancer Res CR (2021) 40(1):24. doi: 10.1186/s13046-020-01820-7
164. Ghattass K, Assah R, El-Sabban M, Gali-Muhtasib H. Targeting hypoxia for sensitization of tumors to radio- and chemotherapy. Curr Cancer Drug Targets (2013) 13(6):670–85. doi: 10.2174/15680096113139990004
165. Kabakov AE, Yakimova AO. Hypoxia-induced cancer cell responses driving radioresistance of hypoxic tumors: approaches to targeting and radiosensitizing. Cancers (2021) 13(5):1102. doi: 10.3390/cancers13051102
166. Khan MS, Hwang J, Lee K, Choi Y, Kim K, Koo HJ, et al. Oxygen-carrying micro/nanobubbles: composition, synthesis techniques and potential prospects in photo-triggered theranostics. Molecules (Basel Switzerland) (2018) 23(9). doi: 10.3390/molecules23092210
167. Yang G, Ji J, Liu Z. Multifunctional Mno(2) nanoparticles for tumor microenvironment modulation and cancer therapy. Wiley Interdiscip Rev Nanomedicine nanobiotechnology (2021) 13(6):e1720. doi: 10.1002/wnan.1720
168. Yang G, Xu L, Chao Y, Xu J, Sun X, Wu Y, et al. Hollow Mno(2) as a tumor-microenvironment-responsive biodegradable nano-platform for combination therapy favoring antitumor immune responses. Nat Commun (2017) 8(1):902. doi: 10.1038/s41467-017-01050-0
169. Shi L, Hu F, Duan Y, Wu W, Dong J, Meng X, et al. Hybrid nanospheres to overcome hypoxia and intrinsic oxidative resistance for enhanced photodynamic therapy. ACS nano (2020) 14(2):2183–90. doi: 10.1021/acsnano.9b09032
170. Martin JD, Fukumura D, Duda DG, Boucher Y, Jain RK. Reengineering the tumor microenvironment to alleviate hypoxia and overcome cancer heterogeneity. Cold Spring Harbor Perspect Med (2016), 6(12). doi: 10.1101/cshperspect.a027094
171. Wang X, Niu X, Zhang X, Zhang Z, Gao X, Wang W, et al. Construction of an auhq nano-sensitizer for enhanced radiotherapy efficacy through remolding tumor vasculature. J materials Chem B (2021) 9(21):4365–79. doi: 10.1039/d1tb00515d
172. Ishikawa H, Barber GN. Sting is an endoplasmic reticulum adaptor that facilitates innate immune signalling. Nature (2008) 455(7213):674–8. doi: 10.1038/nature07317
173. Barber GN. Sting: infection, inflammation and cancer. Nat Rev Immunol (2015) 15(12):760–70. doi: 10.1038/nri3921
174. Meric-Bernstam F, Sweis RF, Hodi FS, Messersmith WA, Andtbacka RHI, Ingham M, et al. Phase I dose-escalation trial of Miw815 (Adu-S100), an intratumoral sting agonist, in patients with advanced/metastatic solid tumors or lymphomas. Clin Cancer Res (2022) 28(4):677–88. doi: 10.1158/1078-0432.Ccr-21-1963
175. McIntosh JA, Liu Z, Andresen BM, Marzijarani NS, Moore JC, Marshall NM, et al. A kinase-Cgas cascade to synthesize a therapeutic sting activator. Nature (2022) 603(7901):439–44. doi: 10.1038/s41586-022-04422-9
176. Guo J, Huang L. Nanodelivery of Cgas-sting activators for tumor immunotherapy. Trends Pharmacol Sci (2022) 43(11):957–72. doi: 10.1016/j.tips.2022.08.006
177. Wehbe M, Wang-Bishop L, Becker KW, Shae D, Baljon JJ, He X, et al. Nanoparticle delivery improves the pharmacokinetic properties of cyclic dinucleotide sting agonists to open a therapeutic window for intravenous administration. J Controlled release (2021) 330:1118–29. doi: 10.1016/j.jconrel.2020.11.017
178. Su T, Cheng F, Qi J, Zhang Y, Zhou S, Mei L, et al. Responsive multivesicular polymeric nanovaccines that codeliver sting agonists and neoantigens for combination tumor immunotherapy. Advanced Sci (Weinheim Baden-Wurttemberg Germany) (2022) 9(23):e2201895. doi: 10.1002/advs.202201895
179. Sun X, Zhang Y, Li J, Park KS, Han K, Zhou X, et al. Amplifying sting activation by cyclic dinucleotide-manganese particles for local and systemic cancer metalloimmunotherapy. Nat nanotechnology (2021) 16(11):1260–70. doi: 10.1038/s41565-021-00962-9
Keywords: nanodrug delivery systems, nanoparticles, immunogenic cell death, immunotherapy, tumor microenvironment, solid tumors, EPR effect
Citation: Zhang J, Wang S, Zhang D, He X, Wang X, Han H and Qin Y (2023) Nanoparticle-based drug delivery systems to enhance cancer immunotherapy in solid tumors. Front. Immunol. 14:1230893. doi: 10.3389/fimmu.2023.1230893
Received: 29 May 2023; Accepted: 19 July 2023;
Published: 03 August 2023.
Edited by:
Fernando Torres Andón, Institute of Biomedical Research of A Coruña (INIBIC), SpainReviewed by:
Jun Ye, Chinese Academy of Medical Sciences and Peking Union Medical College, ChinaHongwei Cheng, University of Macau, China
Gang Chen, University of Health and Rehabilitation Sciences, China
Copyright © 2023 Zhang, Wang, Zhang, He, Wang, Han and Qin. This is an open-access article distributed under the terms of the Creative Commons Attribution License (CC BY). The use, distribution or reproduction in other forums is permitted, provided the original author(s) and the copyright owner(s) are credited and that the original publication in this journal is cited, in accordance with accepted academic practice. No use, distribution or reproduction is permitted which does not comply with these terms.
*Correspondence: Huiqiong Han, aGhxODgzMjNAMTYzLmNvbQ==; Yanru Qin, eWFucnVxaW5AMTYzLmNvbQ==