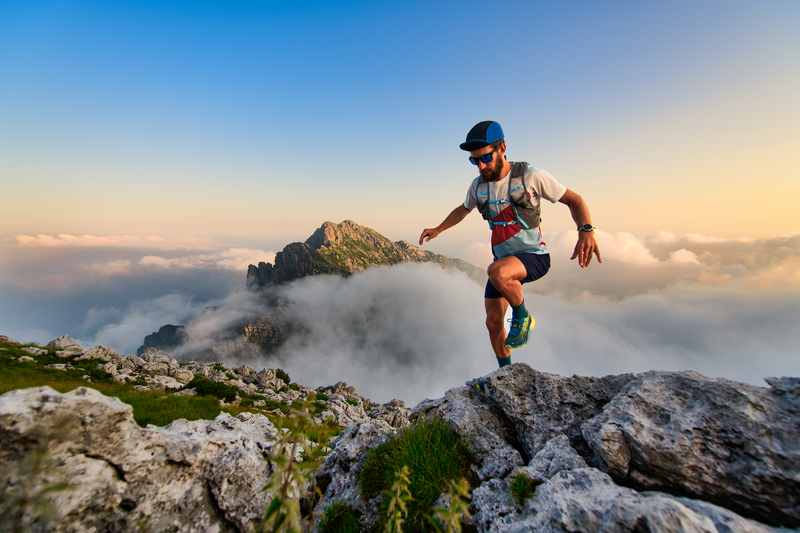
95% of researchers rate our articles as excellent or good
Learn more about the work of our research integrity team to safeguard the quality of each article we publish.
Find out more
ORIGINAL RESEARCH article
Front. Immunol. , 04 September 2023
Sec. Microbial Immunology
Volume 14 - 2023 | https://doi.org/10.3389/fimmu.2023.1229562
This article is part of the Research Topic Community Series in Fighting an Elusive Enemy: Staphylococcus aureus and its Antibiotic Resistance, Immune-Evasion and Toxic Mechanisms: Volume 2 View all 5 articles
Life-threatening toxic shock syndrome is often caused by the superantigen toxic shock syndrome toxin-1 (TSST-1) produced by Staphylococcus aureus. A well-known risk factor is the lack of neutralizing antibodies. To identify determinants of the anti-TSST-1 antibody response, we examined 976 participants of the German population-based epidemiological Study of Health in Pomerania (SHIP-TREND-0). We measured anti-TSST-1 antibody levels, analyzed the colonization with TSST-1-encoding S. aureus strains, and performed a genome-wide association analysis of genetic risk factors. TSST-1-specific serum IgG levels varied over a range of 4.2 logs and were elevated by a factor of 12.3 upon nasal colonization with TSST-1-encoding S. aureus. Moreover, the anti-TSST-1 antibody levels were strongly associated with HLA class II gene loci. HLA-DRB1*03:01 and HLA-DQB1*02:01 were positively, and HLA-DRB1*01:01 as well as HLA-DQB1*05:01 negatively associated with the anti-TSST-1 antibody levels. Thus, both toxin exposure and HLA alleles affect the human antibody response to TSST-1.
Toxic shock syndrome (TSS) is an acute live-threatening toxin-mediated disease caused by the pathobiont Staphylococcus (S.) aureus. It is characterized by fever, scarlet-like rash, multi-organ dysfunction, hypotension, and shock (1, 2). In 1980, the use of highly absorbent tampons in the United States caused an outbreak of menstrual TSS (mTSS) in young women (3). By eliminating highly absorbent materials and providing education on proper usage, the incidence of mTSS dropped from approximately 13.7 in 1980 to 1 per 100,000 menstruating women in 1986 (4, 5). Non-menstrual TSS (nmTSS) is caused by S. aureus infections at other foci and is reported from both males and females. TSS is strongly linked to toxic shock syndrome toxin-1 (TSST-1)-producing S. aureus strains, and a lack of anti-TSST-1 antibodies is a well-known risk factor for TSS (4, 6). However, determinants of low antibody titers against TSST-1 are still largely unknown.
The staphylococcal superantigen (SAg) TSST-1 is the major cause of TSS. The TSST-1-encoding gene tst is detected in 89-100% of mTSS isolates as well as in approximately 50% of nmTSS isolates (4, 7, 8). TSST-1, but not staphylococcal enterotoxins, causes TSS after vaginal application in rabbits (9). Recent data point to a direct activation of vaginal epithelial cells via CD40, which leads to chemokine release and subsequent recruitment of immune cells (10). SAg genes including tst are encoded by mobile genetic elements, rendering the SAg gene profile of S. aureus strains highly variable. The tst gene is located on staphylococcal pathogenicity islands (SaPI) and strongly linked to the wide-spread clonal complex (CC) 30 (1, 11, 12). Accordingly, CC30 isolates account for the majority of mTSS cases and were also frequently isolated from tampons recovered from a cohort of healthy women (13). nmTSS is frequently caused by other S. aureus lineages, which lack tst but harbor other SAg genes, including genes for the staphylococcal enterotoxins SEA, SEB, SEC and SED (1, 4).
SAgs are very potent T cell mitogens, inducing massive T cell proliferation and cytokine release. They circumvent conventional antigen recognition by cross-linking major histocompatibility complex class II (MHC II) molecules on antigen presenting cells (APCs) with T cell receptors (TCRs) on T cells (14). Each SAg binds to a certain subset of TCR Vβ elements, and therefore activate T cells irrespective of their antigen-specificity (15). TSST-1 activates TCR Vβ2-bearing T cells, which account for up to 10% of T cells in human peripheral blood, expanding them in fatal cases to up to 30-70% (16). Superantigenic activation of T cells and APCs (e.g. macrophages) causes strong activation of NFκB and massive cytokine release; predominantly interleukin (IL)-2, tumor necrosis factor (TNF-)α and interferon (IFN-)γ by T cells as well as TNFα, IL-1β and IL-6 by monocytes (2, 14). This causes fever, and generalized capillary leakage and hypotension, ultimately resulting in shock and organ failure.
SAgs are highly immunogenic and antibodies against TSST-1 as well as the staphylococcal enterotoxins are common in the general population (17–19). Seroconversion occurs in the course of S. aureus infection, e.g. sepsis, and endocarditis (20–22). The most frequent route of exposure to TSST-1, however, is nasal colonization. Around 20% of the population are persistently colonized with S. aureus, with the remainder likely being intermittently colonized (23). As CC30 ranks among the top 3 S. aureus lineages in population-based studies around the world, everyone likely gets exposed to the CC30-linked TSST-1 toxin during life (11, 24, 25). TSST-1 binding antibodies correlate with protection against TSS (6, 17, 18, 26). It was estimated that only 9-15% of young US American adults lack sufficient antibody titers against TSST-1 (i.e. have ELISA titers < 100) (27, 28). At the onset of mTSS, however, antibodies against TSST-1 were reported as lacking or low in up to 98% of the patients (29). Around half of these patients do not seroconvert over the course of disease (29). These numbers illustrate that a lack of anti-TSST-1 antibodies is a major risk factor for TSS.
In this study, we aimed to identify genetic determinants and other risk factors for low titers of anti-TSST-1 antibodies by studying a sample of 976 participants of the population-based Study of Health in Pomerania (SHIP-TREND-0). Here, we report that toxin exposure due to nasal colonization with tst-positive S. aureus strains as well as particular HLA alleles determine serum antibody levels against TSST-1.
To identify determinants of the anti-TSST-1 antibody response, we examined a sub-sample of 976 participants in SHIP-TREND-0. For this study sample a complete data set was available, comprising the S. aureus colonization status, anti-TSST-1 antibody profiles, and genome-wide genotyping data for single-nucleotide variants (SNVs) (Supplementary Table 1) (11, 19, 30, 31). Of these individuals, 25.5% (249/976) were intranasally colonized with S. aureus. Spa typing and PCR data were available for 240 of these isolates. The lineage CC30 was most prevalent, accounting for 20.0% (48/240) of the S. aureus isolates. The SAg gene tst was detected in 20.4% (49/240) of the isolates. As previously reported (11), this SaPI-encoded toxin was strongly linked to the lineages CC30 (40/48) and CC395 (5/5), and only rarely detected in other S. aureus CCs if at all (Figure 1 and Supplementary Table 2).
Figure 1 Prevalence of tst is linked to S. aureus clonal complexes. S. aureus nasal colonization was examined in the population-based study SHIP-TREND-0. S. aureus isolates were assigned to clonal complexes (CCs) based on spa typing (11). The gene tst was detected by multiplex PCR (11).
To investigate whether exposure to TSST-1 affects the levels of anti-TSST-1 antibodies, we quantified TSST-1-binding antibody levels and correlated them with current S. aureus nasal carriage. Anti-TSST-1 total IgG (IgGt), IgA, and IgG4 antibodies were measured in serially diluted serum samples over a broad detection range using the xMAP® technology. TSST-1-specific serum IgGt levels varied over a factor of 4.2 logs with no major difference between males and females or age groups (Figure 2 and Supplementary Figure 1). S. aureus carriers exhibited 1.6-fold higher specific IgGt levels than non-carriers (Figure 2, top panel), suggesting that recent exposure increases antibody titers. A broad range of anti-TSST-1 antibodies in non-carriers, however, implies that these individuals have also been exposed to the TSST-1 toxin in the past. As the presence of the gene tst is strongly linked with CC30, we next stratified our analysis by this most common S. aureus lineage. As expected, the difference in antibody titers was much more pronounced when comparing individuals carrying CC30 strains with non-carriers and individuals colonized with other S. aureus CCs (5.8-fold). Finally, individuals carrying a tst-positive S. aureus strain had 12.3 times higher serum IgGt binding to TSST-1 than those who did not (non-carriers and carriers of tst-negative strains) (Figure 2, top panel).
Figure 2 High anti-TSST-1 antibody levels in the general population are linked to S. aureus colonization, in particular colonization with tst-positive S. aureus strains. Anti-TSST-1 IgGt (top panel), IgA (middle panel) and IgG4 (bottom panel) antibody levels were quantified using the xMAP® technology (19, 32). Data were stratified by S. aureus nasal colonization (left panel), colonization with CC30 S. aureus strains (central panel), or colonization with tst-positive S. aureus strains (right panel). CCs were determined by spa typing, the presence of tst was detected by multiplex PCR (11, 33). Box plots display the median along with the 25th and 75th quantiles, whiskers depict the 25th quantile minus 1.5*IQR and 75th quantile plus 1.5*IQR, respectively. Probability density shown as violin plots. Statistics: unpaired Wilcoxon rank sum test; X, fold-change between median values.
The anti-TSST-1 IgA response resembled the IgGt response in many aspects, but IgA levels were generally lower than IgGt levels (Figure 2, middle panel). Again, we observed a broad range of TSST-1 serum IgA antibody titers covering 4.1 logs, IgA levels were by a factor of 5.3 higher in carriers of tst-positive S. aureus strains as compared to non-carriers and carriers of tst-negative strains. We also assessed the IgG4 antibody response as a marker for type 2-driven immune responses. Thirty-seven individuals (3.8%) lacked TSST-1-specific IgG4 and 15.2% (148/976) of the cohort presented with antibody levels below 1,000 AU. Nevertheless, carriage of CC30- or tst-positive strains again strongly affected the anti-TSST-1 IgG4 levels (Figure 2, bottom panel).
There was a fair correlation between IgGt and IgG4 (R² = 0.52) – although some individuals with high IgGt titers lacked IgG4 completely – and between IgGt and IgA (R² = 0.35), as well as a poor correlation between IgA and IgG4 (R² = 0.27) (Figure 3) (34). Previous studies estimated that up to 15% of the population lack protective titers of TSST-1 neutralizing serum antibodies (27, 28). As TSST-1-binding and -neutralizing antibodies correlate well (26), we applied this 15% cut-off to our IgGt, IgA, and IgG4 data sets. Only one out of the 49 carriers of tst-positive strains had an IgGt titer below this 15th percentile. Similarly, only 2 out of 49 carriers of tst-positive strains showed anti-TSST-1 IgA below this threshold. In summary, anti-TSST-1 antibody levels were highly variable. Recent TSST-1 exposure due to nasal colonization with tst-positive S. aureus strains strongly increased the anti-TSST-1 antibody titers.
Figure 3 Moderate correlation of anti-TSST-1 IgGt, IgA and IgG4 antibody levels. Correlation plots of anti-TSST-1 IgGt vs. IgA (A), IgGt vs. IgG4 (B), and IgA vs. lgG4 (C). Colors indicate S. aureus non-carriers (gray), carriers colonized with tst-negative strains (black), and carriers colonized with tst-positive strains (red). Data points are semi-transparent to better visualize areas with high point densities. We performed a 2D kernel density estimation and displayed results as contours. Dashed lines indicate the 15th percentiles in antibody levels, which could indicate a threshold of protection (IgGt: 28,320 AU, IgA: 3,211 AU, IgG4: 931 AU) (27, 28). Diagonal lines with 95% confidence intervals (shaded gray areas) indicate a linear fit based on a simple linear regression model. R², square of the spearman correlation coefficient rho; p, p-value of spearman correlation.
To identify genetic determinants of the anti-TSST-1 antibody response, we performed genome-wide association studies (GWAS) on the phenotypes anti-TSST-1 IgGt-, IgG4-, and IgA levels. We included all SHIP-TREND-0 participants of our study sample with available antibody profiles and genome-wide SNV typing information (n=969). The latter was generated by individual array-based typing using genomic DNA prepared from whole blood and the Illumina Infinium Omni2.5 BeadChip as described before (30, 31). Additional SNV alleles were imputed using the HRC (r1.1 2016) imputed dataset (35). We observed a strong association of the anti-TSST-1 IgGt and IgA levels with the HLA class II genes DQ and DR on chromosome 6 (Figure 4A). In contrast, the anti-TSST-1 IgG4 levels were not associated with the HLA class II complex, and only weakly with other loci.
Figure 4 Manhattan plots demonstrate genome-wide associations of anti-TSST-1 IgGt and IgA antibody levels with HLA-DQ and DR loci. SNVs were plotted on the x-axis according to their position on each chromosome against the association with the respective phenotype (anti-TSST-1 IgGt, IgA, and IgG4 levels) on the y-axis (shown as -log10 p-value) (A). The GWAS are based on 969 SHIP-TREND-0 participants. The dashed line indicates the threshold for genome-wide significance (p=5x10-8). Regional plots of the genomic region on chromosome 6 covering the significant SNVs (32 – 33 Mb) (B).
The identified region on chromosome 6 encodes the α and β chains of the MHC class II molecules HLA-DQ, and -DR, which are involved in antigen presentation to helper T cells (Figure 4B). The β chains of both MHC molecules are highly polymorphic with several thousand reported allelic variants (HLA- DQB1: 2419 alleles, DRB1: 3486 alleles), while the α chains are less variable (DQA1: 556 alleles, DRA: 46 alleles) (https://www.ebi.ac.uk/ipd/imgt/hla/about/statistics/. IPD-IMGT/HLA Database. Version report - 3.51). To reliably identify the involved HLA-DQB1 and -DRB1 alleles, we HLA-typed sub-groups of our cohort by either targeted sequencing of the β chains of the HLA class II gene loci or whole genome sequencing (WGS).
First, we determined the β chains of the HLA class II alleles as well as the HLA class I alleles in a sub-group of 574 SHIP-TREND-0 participants by PCR amplification and subsequent sequencing of the respective genomic regions. Sequencing was performed by the German Bone Marrow Donor Center (Deutsche Knochenmarkspenderdatei, DKMS), which coordinates the largest HLA-typed donor registry in Germany (36). The most frequent HLA class II alleles (frequency ≥ 3%) were correlated with anti-TSST-1 antibody binding. In this screening sample, HLA-DQB1*05:01 and HLA-DRB1*01:01 were negatively associated with the strength of the serum IgGt binding to TSST-1 (Figure 5A). The same alleles were negatively associated with TSST-1-binding IgA antibodies. In contrast, anti-TSST-1 IgG4 levels were not associated with HLA class II alleles. In line with the GWAS results, we observed no correlation of the HLA-DP locus and the HLA class I loci (A, B, and C) with antibody binding to TSST-1 (Figure 5A and Supplementary Figure 2).
Figure 5 HLA class II alleles are associated with low or high anti-TSST-1 IgGt and IgA antibody levels. (A) A sub-cohort of 574 SHIP-TREND-0 participants was HLA-typed by PCR amplification and sequencing of the HLA coding regions (DKMS, light gray). The most frequent HLA class II alleles (frequency ≥ 3%) were correlated with the anti-TSST-1 antibody titers. The findings were validated by deducing HLA types from Illumina whole genome sequencing data using the HLA-HD algorithm (n=402, dark gray). (B) Meta-analysis of the results from both approaches (n=976, black). DNA array-based HLA allele prediction using the Four-digit Multi-ethnic HLA v2 imputation panel (n=965, white). –log10(q-value), negative decadic logarithm of the Benjamini-Hochberg (BH) adjusted p-value.
To validate our findings, we determined the HLA type in the so far untyped SHIP-TREND-0 participants using available WGS data (n=402). Both HLA class I loci and class II loci (including also the α chains) were derived from Illumina WGS data using the HLA-HD algorithm (37). WGS data confirmed the association of anti-TSST-1 IgGt and IgA with HLA-DRB1*01:01, and found a similar trend for HLA-DQB1*05:01 (Figure 5A). In addition, HLA-DQB1*02:01 and HLA-DRB1*03:01 were positively associated with anti-TSST-1 IgA titers.
To increase the statistical power, we next combined the HLA typing data from the two groups, HLA typed by DKMS or WGS, in a meta-analysis based on the summary statistics from the single variant association analyses (Figure 5B) (38). This approach confirmed the results and identified additional associations of HLA alleles with anti-TSST-1 antibody levels. Again, HLA-DQB1*05:01 and DRB1*01:01 were negatively associated with the strength of the serum IgGt reaction to TSST-1. In addition, HLA-DQB1*02:01 and HLA-DRB1*03:01 now also exhibited a significant positive association with TSST-1-binding IgGt antibodies. The very same alleles were positively or negatively associated with TSST-1-binding IgA antibodies. In addition, the alleles HLA-DQB1*06:03 and HLA-DRB1*13:01 were positively associated with IgA levels and showed a similar trend for IgGt. In contrast, anti-TSST-1 IgG4 levels were associated with a different set of HLA-DQ and -DR alleles, albeit weakly.
HLA sequencing data or WGS data are rarely available for population-based cohort studies similar to SHIP. Hence, we explored whether the HLA type can be reliably deduced from array-based genotyping data for all individuals with available genotyping data and anti-TSST-1 antibody profiles (n=965). We determined the HLA type, covering both α and β chains for HLA class II, by applying the recently published Four-digit Multi-ethnic HLA v2 (2022) algorithm (39) to genotyping data that had been obtained with the Illumina Infinium HumanOmni2.5 BeadChip. Again, we retrieved exactly the same panel of positively and negatively associated HLA-DQB1 and -DRB1 alleles. We also observed an association of anti TSST-1 IgGt and -IgA with DQA1 alleles (Supplementary Figure 3). The log10 p-values were highly concordant between the array-based and the sequencing-based HLA data (Figure 5B). A comparison of the imputed HLA alleles with the sequence-based HLA typing (DKMS) confirmed 98.97% and 98.67% accuracy in HLA class I and II allele prediction based on the four-digit code. Hence, reliable prediction of HLA alleles from genotyping data is feasible, making HLA analysis easily available to numerous population-based studies.
To summarize, both a sequencing-based meta-analysis and an array-based genotyping approach revealed that HLA-DQB1*02:01 and HLA-DRB1*03:01 were positively, and HLA-DQB1*05:01 and HLA-DRB1*01:01 negatively associated with anti-TSST-1 IgGt and IgA serum levels.
Due to the high polymorphism of the HLA locus, most individuals are heterozygous for the genes encoding the HLA type I and II molecules. Since HLA alleles are co-dominantly expressed, most individuals will express two HLA-DR and -DQ alleles and thus could potentially harbor combinations of up to four positively and/or negatively associated alleles. To estimate a possible dosage effect, we assigned each positively and negatively associated allele with an arbitrary value of 1 and -1, respectively, and calculated the sum of all alleles that were significantly associated with TSST-1-specific IgGt and IgA responses for each individual (Supplementary Table 3). The obtained dosage score correlated with the IgGt and IgA responses to TSST-1, showing increasing median antibody levels with increasing dosage score (Figure 6 and Supplementary Figure 4). We focused on dosage groups with ≥ 20 individuals (dosage groups -2 to 4) and conducted a regression analyses based on the median antibody levels. This confirmed a cumulative effect of the number of positively or negatively associated HLA alleles on the strength of the anti-TSST-1 IgGt and IgA response. The maximal effect size was a 4.9-fold increase in median antibody levels between the dosage groups -2 and 4 for IgGt and a 4.5-fold increase for IgA. Since HLA-DQ and -DR were only moderately correlated with IgG4 binding to TSST-1, there was no clear dosage effect for this antibody class (data not shown).
Figure 6 Cumulative effect of positively and negatively associated HLA alleles on anti-TSST-1 IgGt and IgA levels. Allele dosage effects of positively and negatively associated HLA-DQB1 and -DRB1 alleles on anti-TSST-1 IgGt (A) and IgA (B) were assessed by assigning positively associated alleles the arbitrary value of 1 (IgGt: DQB1*02:01, DRB1*03:01; IgA: additionally, DQB1*06:03 and DRB1*13:01) and negatively associated alleles the value of -1 (IgGt and IgA: DQB1*05:01, DRB1*01:01). The analysed subsample comprised all DKMS- and WGS-based HLA typed individuals (n=976). The allele dosage was defined as the sum of those values. Only allele dosage groups with case numbers ≥ 20 were included in the correlation analysis. Box plots display the median along with the 25th and 75th quantiles, whiskers depict the 25th quantile minus 1.5*IQR and 75th quantile plus 1.5*IQR, respectively. Probability density shown as violin plots. Statistics: p-value and adjusted R squared by simple linear regression model of median log10-transformed anti-TSST-1 antibody levels and allele dosage categories.
If we assume that 15% of the population are at an increased risk of TSS because of low toxin-specific antibody levels, 25.6% (50/195) of those with predominantly negative HLA alleles but only 8.7% (28/323) of those with predominantly positive HLA alleles belong to this vulnerable group. In summary, we demonstrated a cumulative effect of positively and negatively associated HLA alleles on anti-TSST-1 IgGt and IgA levels.
Most adults have protective titers of anti-TSST-1 antibodies, but up to 15% of the population is considered at risk for TSS. We have identified determinants of the anti-TSST-1 antibody response in the population-based study SHIP-TREND-0. Anti-TSST-1 IgGt antibody levels were highly variable, with 4.2 orders of magnitude difference between low and high responders. Recent exposure by nasal colonization with tst-positive S. aureus strains enhanced antibody levels by a factor of 12.3. In addition, we observed a strong genetic correlation with HLA class II alleles. HLA-DRB1*03:01 and HLA-DQB1*02:01 were positively, and HLA-DRB1*01:01 and HLA-DQB1*05:01 negatively associated with anti-TSST-1 antibody levels. Antibody levels differed by a factor of 4.9 between individuals harboring negatively-associated HLA-DR and -DQ alleles and those with 4 positively associated alleles. Hence, both toxin exposure and HLA alleles have a strong impact on the antibody response to TSST-1.
Using a sensitive Luminex® assay, we were able to measure anti-TSST-1 serum antibodies in every study participant, with highly variable titers between individuals. In contrast, previous studies employing ELISA or RIA failed to detect anti-TSST-1 antibodies in approximately 8% of the young general population (<30 years) (27, 40). The higher sensitivity of our analyses is a feature of the Luminex® system. By measuring each serum in seven dilutions, we achieved a dynamic range of 5 orders of magnitude. We calculated the relative specific antibody levels (AU) with the new data analysis tool xMAPr (32). In this study, we determined only TSST-1-binding, however, binding and neutralizing antibody titers against TSST-1 are strongly correlated. In detail, we observed a strict correlation between antibody binding as determined by Luminex® assay and TSST-1 neutralizing capacity (inhibition of TSST-1-induced T cell proliferation) in a cohort of healthy S. aureus carriers and non-carriers (26). Mouse studies corroborate this and show that SAg binding (SEA, SEB, SEC, TSST-1, as determined by ELISA) strictly correlates with protection (41).
TSST-1-neutralizing antibodies protect against TSS, while low antibody titers put individuals at risk (27, 29, 42). There is anecdotal evidence that the application of intravenous Ig preparations, which are prepared from large human plasma pools and contain TSST-1-neutralizing antibodies, can decrease mortality in staphylococcal TSS (42, 43). ELISA-based studies from the 1980s suggest that serum antibody titers below 100 will not provide sufficient protection (27, 29, 44). These studies estimated that up to 15% of the general adult population lack sufficient antibody titers. Assuming a similar seroprevalence, this would mean that up to 146 individuals in our study sample were at an increased risk of TSS. This corresponds to a cut-off of 28,320 AU for anti-TSST-1 IgGt, which is 10 times lower than the median IgGt level.
Anti-TSST-1 antibodies are generated by direct exposure to the toxin during colonization or infection (20–22). The most frequent route of TSST-1 exposure is nasal (and vaginal) colonization. In our study, 5.02% of the participants were intranasally colonized with tst-positive strains, which is comparable to data from North America (40). Vaginal colonization with TSST-1-producing S. aureus reportedly ranged between 1-3% (40, 45, 46). Moreover, TSST-1-producing S. aureus was isolated from 4% of tampons retrieved from healthy menstruating women (13). The relatively low frequency of colonizing tst-encoding S. aureus strains as compared to 100% seroconversion to TSST-1 suggests frequent fluctuation of S. aureus strains in the general population. Nevertheless, in some adults the immune system was probably never directly confronted with TSST-1. Antibody binding, especially at low levels, could be due to cross reactivity with other SAgs, despite the limited sequence similarity (33-39%; data not shown) (22, 41, 47, 48). Moreover, environmental and behavioral factors could influence seroconversion (28). Thus, individuals with very low or lacking anti-TSST-1 antibodies have either never encountered TSST-1 or were inherently incapable of developing a TSST-1 antibody response. This is consistent with the results of the first clinical TSST-1 vaccine trial. 46 individuals were immunized (with adjuvant) with a recombinant TSST-1 toxoid (dose range: 100 ng - 30 µg). Only two vaccinees failed to produce specific antibodies; both had received a very low antigen dose (100 ng or 300 ng) (49, 50). This illustrates that upon exposure with significant amounts of TSST-1, almost everyone generates antibodies.
Recent exposure by nasal S. aureus colonization strongly enhanced anti-TSST-1 antibody titers. On average, antibody levels were 12.3-fold higher in carriers of tst-positive S. aureus strains than in the rest of the cohort comprising noncarriers and carriers of tst-negative strains. In an earlier study, 98% of menstruating women colonized with tst-positive strains had measurable anti-TSST-1 antibodies as compared to 84% in noncarriers or carriers of tst-negative strains (40). In line with this, our group previously reported that S. aureus carriers mount a strong antibody response against the SAgs produced by their colonizing strain (17). This suggests that colonization with a tst-positive S. aureus strain, either per se or due to minor subclinical infections, induces an anti-toxin antibody response. Among the 49 carriers of tst-positive S. aureus strains, only one had IgGt and two had IgA levels below the 15% percentile, placing them at increased risk of developing TSS. This implies that given sufficient exposure to TSST-1 almost all adults can generate specific antibodies against the toxin. Whether the antibodies induced by intermittent colonization are stable over time, remains to be clarified as longitudinal data on anti-S. aureus antibodies are not available in the SHIP-TREND cohort. Such data are generally scarce (26). The determination of the stability of anti-S. aureus toxin antibodies is an important topic to be addressed in future studies, since there are examples for both extremely stable humoral immune memory in humans and a rapid decline of antibody titers after cessation of exposure (51, 52).
Our GWAS revealed a robust association of anti-TSST-1 IgGt and IgA levels with HLA-class II but not with HLA-class I alleles. HLA typing demonstrated that HLA-DRB1*03:01 and HLA-DQB1*02:01 were positively, and HLA-DRB1*01:01 and HLA-DQB1*05:01 negatively associated with the anti-TSST-1 IgGt serum levels. The effect size was 4.9-fold when comparing individuals harboring all four positively associated alleles and those with a cumulative allele dosage of -2. Hence, HLA alleles have a smaller impact than recent TSST-1 exposure. If we assume that 15% of the adult population has antibody levels too low to protect against TSS, 25.6% (50/195) of those with predominantly negative HLA alleles versus 8.7% (28/323) of those with predominantly positive HLA alleles belong to this vulnerable group. Whether the reported negatively-associated alleles are enriched in TSS patients will be in the focus of future projects. The selective association of HLA class II with the TSST-1-specific antibody response can be explained by their behavior as a conventional protein antigen. HLA-alleles that present a broader spectrum of TSST-1 peptide epitopes could potentially activate more T helper cells resulting in more effective help to TSST-1-specific B cells and higher anti-TSST-1 antibody titers.
The determinants of the IgG4 response to TSST-1 differed from those influencing the IgGt and IgA response. While the TSST-1-specific IgG4 titers were also associated with colonization by tst-positive S. aureus strains, some individuals had no measurable toxin-binding IgG4 at all. Second, serum IgG4 was only weakly affected by the HLA-II polymorphism. The distinct behavior of the IgG4 response to TSST-1 is not surprising, as function and regulation of IgG4 are special (53). IgG4 is not usually associated with antimicrobial defense but rather with immune responses to worms and with allergies. There is further a broad spectrum of rare IgG4-related diseases characterized by inflammatory dysregulation (53–55). IgG4 acts mainly by competing with other Ig classes for epitope binding and has little inflammatory effect itself (53). IgG4 release is regulated similarly to IgE. Both Ig subclasses are generated by B cells under the influence of IL-4 and IL-13, which requires the help of Th2 cells, more specifically follicular T helper cells type 2 (56). Since IgG4 production additionally requires IL-10 derived, for example, from regulatory T cells, this is referred to as a modified Th2 response (57–59). Further, HLA alleles play a role in IgG4 production. There is a strong, cross-disease association with HLA-DQB1*05 (60). This HLA allele apparently predisposes to IgG4 generation, but, as shown in this study, it antagonizes the humoral immune response to anti-TSST-1. We conclude that two independent conditions must be met for the development of TSST-1-specific IgG4 (i): exposure to TSST-1 and (ii) a genetic predisposition favoring a modified type 2 response and a class switch to IgG4.
To which extent variations in total antibody levels in the blood may have biased the TSST-1-specific antibody titers could not be measured in this study, because data on total IgG, IgA, and IgG4 subclasses are not available in the SHIP-TREND-0 cohort. However, variations in total IgG and IgA serum levels are small (reference ranges IgG: 7,0 – 16.0 g/L; IgA: 0.7 – 4.0 g/L) in comparison with the large differences in anti-TSST-1 IgGt and IgA antibody levels, spanning 4-5 orders of magnitude and therefore cannot explain them (61, 62). Total IgG4 serum concentrations, however, are more variable (reference range: 0.004–2.68 g/L). In line with this, many individuals in our cohort had no measurable or very low TSST-1-specific IgG4 titers. Thus, in the case of IgG4, factors other than antigen exposure and HLA class II polymorphism also have an important influence.
Besides influencing anti-TSST-1 antibody titers, HLA class II polymorphisms can also directly impact on TSST-1 binding and thus SAg-driven pathogenesis. Of note, SAg-mediated cross-linking of MHC class II and TCR is not occurring simultaneously. It is initiated by high affinity binding of these toxins to HLA class II molecules on the APC surface, followed successive engagement and cross-linking of multiple TCR molecules (63). Several in vitro studies demonstrated that the binding of SAgs to HLA class II is influenced by HLA class II loci and polymorphism. TSST-1 binds effectively to HLA-DR, but not HLA-DP (64, 65). In addition, HLA class II alleles differ strongly in their capacity to induce T cell proliferation and cytokine secretion upon SAg stimulation (66). Interestingly, there are also qualitative differences in the SAg-induced T cell response, as reflected by different cytokine patterns and modified TCR Vβ profiles (66). The influence of HLA-DR polymorphisms on the immune response to TSST-1 could be confirmed in HLA transgenic mice (67). Moreover, HLA class II haplotypes influence the outcome of invasive group A streptococcal infections (68). HLA class II alleles that were associated with protection from severe systemic manifestations of disease presented streptococcal SAgs in a manner that resulted in significantly reduced cytokine and proliferative responses (68). On top, TSST-1-induced T cell activation is also affected by the bound peptide antigen, because this toxin forms a wedge between HLA class II and TCR and directly contacts the bound peptide antigen (69, 70). Together, the overall avidity of the HLA:peptide - TCR - TSST-1 complex likely determines the strength and also quality of the TSST-1-driven immune response.
The HLA-alleles are strongly associated with numerous diseases. However, in many population-based studies, HLA-haplotypes have not been determined due to technical difficulties in resolving the extreme polymorphism of the HLA gene locus. Our study shows how this gap may be closed by imputation in many cases.
The classical HLA class I and class II molecules mediate the antigen-specific activation of T cells, which in turn orchestrate the adaptive immune responses. Since the locus is highly polymorphic and HLA alleles present distinct spectra of antigenic peptide epitopes to T cells, the HLA haplotypes impact on a variety of diseases with large effect sizes, in particular, autoimmune disorders, immune deficiencies, inflammatory diseases, and infectious diseases (71). Nevertheless, in GWASs, the HLA gene locus is often excluded because it is one of the most challenging genome regions to study due to its extreme polymorphism, its complex relationship with natural selection, and its unique long-range linkage disequilibrium structure (72). On top, the genetic diversity at the HLA gene locus is highly population-specific, necessitating balanced global reference panels. PCR-based methods are still the gold standard in HLA typing (36), but recently developed algorithms, such as “HLA-HD”, now also enable high- to full-resolution HLA genotyping based on NGS data (37, 73, 74).
Since to date HLA sequencing data or NGS data are often not available in population-based studies, we explored whether the HLA type can also be reliably imputed in silico from array-based genotyping data. Therefore, in addition to the HLA typing by direct sequencing, we imputed the genotype information (based on Illumina Infinium HumanOmni2.5 BeadChip genotyping data) on the Michigan Imputation server (MIS) to the four-digit Multi-ethnic HLA v2 (2022) panel for the set of 976 samples (39, 72). A comparison of the imputed HLA alleles with the sequence-based HLA typing (DKMS) confirmed 98.97% and 98.67% accuracy in HLA class I and II allele prediction, respectively, based on the four-digit code. In line with our data, Luo et al. reported 97.8% accurate imputation at G-group resolution in European populations using the same reference panel (39). Thus, reliable prediction of HLA alleles from genotyping data is feasible and as accurate as NGS-based HLA typing. This means that now HLA alleles can be reliably determined in the millions of individuals that have been genotyped on microarrays, promising new insights into the genetics of infectious diseases, autoimmune disorders, immune deficiencies, and inflammatory disorders.
Our study population is generally very homogeneous, which is a limitation of the work we present in this manuscript. Other populations could differ (i) in molecular epidemiology of endemic S. aureus, (ii) HLA-haplotype distribution and (iii) numerous other genes and environmental factors that influence the immune response. Variability of HLA alleles across different ethnicities is well-documented (75). The outcome of the analysis could therefore differ between groups of different ancestry. In particular, there may be additional associations between HLA and TSST-1 antibodies that were undetectable in our cohort because of low allele frequency. A Japanese study reported a similar prevalence of TSST-1 producing S. aureus in Japan and the US, while antibody levels in the Japanese cohort were much lower. Notably, both genetic and environmental factors appeared to be important in promoting the development of anti-TSST-1 antibodies, as antibody titers in Japanese women living in Tokyo, were higher than in those living in the United States, but still lower than titers in Caucasian US women (28).
Our research has implications for S. aureus vaccine development. We found that only very few individuals may be inherently incapable of developing a TSST-1 antibody response. Nevertheless, a substantial fraction of the adult population has low specific antibody titers and would benefit from an antibody boost induced by a TSST-1 vaccine. This would not only protect them from TSS, but also from other staphylococcal diseases with SAg involvement, e.g., sepsis, and osteomyelitis.
The Study of Health in Pomerania (SHIP) is a longitudinal population-based study which aims to determine the incidence and prevalence of common risk factors, subclinical disorders and clinical disease (30, 31). The SHIP-TREND cohort only includes individuals of European ancestry. The detailed study design has been published previously (30, 31). SHIP-TREND-0 is the baseline assessment of the 2nd SHIP cohort with proband recruitment (N = 4,420) conducted in the Northeast of Germany between 2008 and 2012. In depth OMICs data (whole genome sequencing, whole-blood-transcriptome and methylome, plasma circulating miRNome, plasma proteome, plasma, urine and saliva metabolome as well as stool and saliva microbiome) as well as S. aureus antibody profiles, however, are only available for a subcohort of 996 subjects (19, 30). Further 20 individuals had to be excluded from our analyses because information on the colonizing S. aureus strain were not available (n=11), HLA typing via DMKS was unsuccessful and WGS not available (n=7), or smoking status was not available (n=2). The study protocol of SHIP-TREND-0 was approved by the local ethics committee of the University of Greifswald (registration no. BB39/08) with all participants giving informed written consent.
Nasal S. aureus colonization was determined by swabbing both nasal vestibules with a rayon swab (BBL CultureSwab Liquid Stuart; BD, USA) and subsequent cultivation of retrieved bacteria in phenol red mannitol salt broth and on mannitol salt agar (BD, Heidelberg, Germany) as described in detail elsewhere (11).
spa genotyping of S. aureus strains was performed according to published protocols using the primers spa-1113f and spa-1514r (76). Closely related spa types (costs ≤ 3) were grouped into spa clonal complexes (spa CCs) using the BURP algorithm (77). Spa CCs were subsequently assigned to multilocus sequence type (MLST) CCs using the SpaServer database (www.spaserver.ridom.de). The gene tst gene was detected by multiplex-PCR as previously reported (33).
Anti-TSST-1 (locus ID: SA1819) total IgG (IgGt), and IgA antibodies were quantified using a bead-based suspension array (xMAP® technology, Luminex®) as described in detail elsewhere (19, 32). TSST-1-specific IgG4 was quantified with an R-Phycoerythrin-labelled anti-human IgG4 (Southern Biotech, order number 9200-09). An arbitrary antibody binding value (AU) was calculated from the dilution series based on curve fitting algorithms using the xMAPr analysis tool (32). If curve fitting failed, an imputation was performed based on a LOESS fit over all measurements of a single dilution (19).
HLA typing of 574 SHIP-TREND-0 samples for HLA-A, B, C, DPB1, DQB1, and DRB1 was performed at the DKMS (Deutsche Knochenmarkspenderdatei) Life Science Lab Dresden (36). The respective genomic regions were amplified by PCR and subsequently sequenced. Allele codes were allocated by either G groups or Multiple Allele Code (MAC) using IPD-IMGT/HLA database version 3.36.0 (https://www.ebi.ac.uk/ipd/imgt/hla/release/v336/).
HLA typing based on WGS results for 408 SHIP-TREND samples was performed using HLA-HD version 1.5.0 with default parameters (37, 78). The software deduces HLA-A, B, C, DPA1, DPB1, DQA1, DQB1, DRA, and DRB1 alleles along with some non-classical HLA genes. HLA-HD dictionary was updated to IPD-IMGT/HLA database version 3.36.0 to match the database used by the DKMS. Typing was done on paired-end whole genome sequencing fastq data. HLA-HD allocated the alleles predominately in 4- or 6-digit field codes.
Genotyping of 983 SHIP-TREND-0 sample was initially performed using the Illumina Infinium HumanOmni2.5 BeadChip. Pre-imputation data generation and quality control of genotyping results was conducted according to the guidelines provided by the imputation service. Genotype information was imputed on the Michigan Imputation server (MIS) to the four-digit Multi-ethnic HLA v2 (2022) panel which is based on IPD-IMGT/HLA database version 3.32.0 (https://www.ebi.ac.uk/ipd/imgt/hla/release/v332/) (39). This panel provides information on HLA-A, B, C, DPA1, DPB1, DQA1, DQB1, and DRB1 alleles.
DKMS-allocated MAC designations were converted to G groups using the MAC Service 2.12.1-SNAPSHOT UI provided by the National Marrow Donor Program (https://hml.nmdp.org/MacUI/). All allele codes derived by the abovementioned methods were restricted to a two-field code (four-digit) and assigned to the associated G groups.
For each HLA typing method (WGS-based, DKMS, and imputation-based) we conducted linear regression analyses and implemented an additive genetic model to evaluate the association of log10-transformed anti-TSST-1 antibody levels on the HLA allele occurrence (0, 1, or 2 copies of the allele). Only individuals with complete data on HLA typing, and anti-TSST-1 antibody levels were included. We adjusted for the covariates age (years), sex, body-mass index (BMI, kg/m2), S. aureus colonization status, and smoking status (current, former and non-smoker). These confounders were identified in a previous study investigating anti-S. aureus serum IgGt levels against a panel of 79 S. aureus antigens (19).
We used the R package metaphor (v3.8.1) to perform a meta-analysis on the summary statistics from the single variant association analyses for WGS- and DKMS-based HLA typing (38). We applied the implemented fixed-effects model with weighted estimation.
A dosage effect was determined by assigning protective HLA-DQB1 and -DRB1 alleles (as determined by the abovementioned HLA typing approaches) with an arbitrary value of 1 and risk alleles with a value of -1 and summing up the protective and risk alleles on both chromosomes for each individual.
All statistical testing and data visualization was performed in R (v4.2.0; https://www.R-project.org/) in combination with the tidyverse package (v2.0.0) (79), rstatix (v0.7.2; https://CRAN.R-project.org/package=rstatix), metaphor (v3.8.1) (80), and plotgardener (v1.4.2) (81).
The data analyzed in this study is subject to the following licenses/restrictions: The data protection policy of the Study of Health in Pomerania (SHIP) does not permit open access to SHIP data or transfer to journals. Data can be accessed for reuse and quality control/assurance via SHIP’s standardized data usage application procedure. Requests to access these datasets should be directed to https://transfer.ship-med.uni-greifswald.de. Summary statistics of all data are provided in Supplementary Figure 4.
The studies involving human participants were reviewed and approved by the Clinical Ethics committee of the University Medicine Greifswald, Greifswald, Germany. The patients/participants provided their written informed consent to participate in this study.
Conceptualization, UV, SH, and BB. Methodology, SW. Software: SW. Formal Analysis, SW, SM. Investigation, SW, TM, CM, CC. Resources, MD, SF, HG, GH, CK, MN, NF, SS, HV, UV, BB. Data Curation, SW. Writing – Original Draft, FS, SH, SW. Writing – Review & Editing, all authors. Visualization, SW, SM. Supervision, UV, SH, and BB. Funding Acquisition, UV, BB, SF, MD, NF, GH, MN, HG. All authors contributed to the article and approved the submitted version.
This study was supported by grants of the DFG (Deutsche Forschungsgemeinschaft) with the CRC TR 34 (project C16; UV, BB, TM) and by the European Social Fund, Card-ii-Omics, ESF/14-BMA55-0037/16 (UV, BB, SH, SM, SF). SHIP is part of the Community Medicine Research net of the University of Greifswald, Germany, which is funded by the Federal Ministry of Education and Research (grants no. 01ZZ9603, 01ZZ0103, and 01ZZ0403), the Ministry of Cultural Affairs as well as the Social Ministry of the Federal State of Mecklenburg-West Pomerania, and the network ‘Greifswald Approach to Individualized Medicine (GANI_MED)’ funded by the Federal Ministry of Education and Research (grant 03IS2061A). Genomewide data have been supported by the Federal Ministry of Education and Research (grant no. 03ZIK012) and a joint grant from Siemens Healthineers, Erlangen, Germany and the Federal State of Mecklenburg-West Pomerania. The genome-sequencing in this study was conducted by the Helmholtz Zentrum München (Deutsches Forschungszentrum für Gesundheit und Umwelt) with funds from the DZHK OMICs initiative (81X1600102; MD, NF, GH, MN, UV, SW). The genome-sequencing in this study was further supported by the EU-JPND funding for the BRIDGET project (01ED1615; HG).
The authors acknowledge the investigative group of the SHIP study, the study staff, and all study participants. We thank Veronika Balau, Annette Barwich, André Goehler, Katrin Kühn, Katrin Schulz, and Ivo Steinmetz for supporting the analysis of the S. aureus colonization status in SHIP-TREND-0. We thank Dorothee Grumann, Julia Kolata, Paula Döring, Erika Friebe, Fawaz Al’Sholui, Sophie Nowotny, Sebastian R. Schulz, Patricia Trübe, and Chi Hai Vu for their contributions to the S. aureus strain characterization. We thank Anja Wiechert for technical assistance. We thank Nicole Haasler, Kristin Henselin, Dörte Radke, and Susanne Westphal for coordinating data acquisition, quality control and transfer in the SHIP study. We thank Thomas Schäfer, Nicole Gruber and the DKMS Life Science Lab GmbH for their support in the PCR-based HLA typing and interpretation of HLA alleles.
Author HG has received travel grants and speaker’s honoraria from Fresenius Medical Care, Neuraxpharm, Servier and Janssen Cilag as well as research funding from Fresenius Medical Care.
The remaining authors declare that the research was conducted in the absence of any commercial or financial relationships that could be construed as a potential conflict of interest.
All claims expressed in this article are solely those of the authors and do not necessarily represent those of their affiliated organizations, or those of the publisher, the editors and the reviewers. Any product that may be evaluated in this article, or claim that may be made by its manufacturer, is not guaranteed or endorsed by the publisher.
The Supplementary Material for this article can be found online at: https://www.frontiersin.org/articles/10.3389/fimmu.2023.1229562/full#supplementary-material
1. Sharma H, Smith D, Turner CE, Game L, Pichon B, Hope R, et al. Clinical and molecular epidemiology of staphylococcal toxic shock syndrome in the United Kingdom. Emerg Infect Dis (2018) 24:258–66. doi: 10.3201/eid2402.170606
2. Lappin E, Ferguson AJ. Gram-positive toxic shock syndromes. Lancet Infect Dis (2009) 9:281–90. doi: 10.1016/S1473-3099(09)70066-0
3. Shands KN, Schmid GP, Dan BB, Blum D, Guidotti RJ, Hargrett NT, et al. Toxic-shock syndrome in menstruating women: association with tampon use and Staphylococcus aureus and clinical features in 52 cases. N Engl J Med (1980) 303:1436–42. doi: 10.1056/NEJM198012183032502
4. DeVries AS, Lesher L, Schlievert PM, Rogers T, Villaume LG, Danila R, et al. Staphylococcal toxic shock syndrome 2000-2006: epidemiology, clinical features, and molecular characteristics. PloS One (2011) 6:e22997. doi: 10.1371/journal.pone.0022997
5. Osterholm MT, Forfang JC. Toxic-shock syndrome in Minnesota: results of an active-passive surveillance system. J Infect Dis (1982) 145:458–64. doi: 10.1093/infdis/145.4.458
6. Bonventre PF, Linnemann C, Weckbach LS, Staneck JL, Buncher CR, Vigdorth E, et al. Antibody responses to toxic-shock-syndrome (TSS) toxin by patients with TSS and by healthy staphylococcal carriers. J Infect Dis (1984) 150:662–6. doi: 10.1093/infdis/150.5.662
7. Bohach GA, Kreiswirth BN, Novick RP, Schlievert PM. Analysis of toxic shock syndrome isolates producing staphylococcal enterotoxins B and C1 with use of southern hybridization and immunologic assays. Rev Infect Dis (1989) 11 Suppl 1:75–81. doi: 10.1093/clinids/11.supplement_1.s75
8. Bohach GA, Fast DJ, Nelson RD, Schlievert PM. Staphylococcal and streptococcal pyrogenic toxins involved in toxic shock syndrome and related illnesses. Crit Rev Microbiol (1990) 17:251–72. doi: 10.3109/10408419009105728
9. Schlievert PM, Jablonski LM, Roggiani M, Sadler I, Callantine S, Mitchell DT, et al. Pyrogenic toxin superantigen site specificity in toxic shock syndrome and food poisoning in animals. Infect Immun (2000) 68:3630–4. doi: 10.1128/IAI.68.6.3630-3634.2000
10. Schlievert PM, Cahill MP, Hostager BS, Brosnahan AJ, Klingelhutz AJ, Gourronc FA, et al. Staphylococcal superantigens stimulate epithelial cells through CD40 to produce chemokines. mBio (2019) 10(2):e00214–19. doi: 10.1128/mBio.00214-19
11. Holtfreter S, Grumann D, Balau V, Barwich A, Kolata J, Goehler A, et al. Molecular epidemiology of staphylococcus aureus in the general population in northeast Germany: results of the study of health in pomerania (SHIP-TREND-0). J Clin Microbiol (2016) 54:2774–85. doi: 10.1128/JCM.00312-16
12. Musser JM, Schlievert PM, Chow AW, Ewan P, Kreiswirth BN, Rosdahl VT, et al. A single clone of Staphylococcus aureus causes the majority of cases of toxic shock syndrome. Proc Natl Acad Sci U.S.A. (1990) 87:225–9. doi: 10.1073/pnas.87.1.225
13. Chiaruzzi M, Barbry A, Muggeo A, Tristan A, Jacquemond I, Badiou C, et al. Vaginal tampon colonization by staphylococcus aureus in healthy women. Appl Environ Microbiol (2020) 86:e01249–20. doi: 10.1128/AEM.01249-20
14. Abdurrahman G, Schmiedeke F, Bachert C, Bröker BM, Holtfreter S. Allergy—A new role for T cell superantigens of staphylococcus aureus? Toxins (Basel) (2020) 12:176. doi: 10.3390/toxins12030176
15. Petersson K, Pettersson H, Skartved NJ, Walse B, Forsberg G. Staphylococcal enterotoxin H induces V -specific expansion of T cells. J Immunol (2003) 170:4148–54. doi: 10.4049/jimmunol.170.8.4148
16. Choi Y, Lafferty JA, Clements JR, Todd JK, Gelfand EW, Kappler J, et al. Selective expansion of T cells expressing V beta 2 in toxic shock syndrome. J Exp Med (1990) 172:981–4. doi: 10.1084/jem.172.3.981
17. Holtfreter S, Roschack K, Eichler P, Eske K, Holtfreter B, Kohler C, et al. Staphylococcus aureus carriers neutralize superantigens by antibodies specific for their colonizing strain: a potential explanation for their improved prognosis in severe sepsis. J Infect Dis (2006) 193:1275–8. doi: 10.1086/503048
18. Holtfreter S, Bröker BM. Staphylococcal superantigens: do they play a role in sepsis? Arch Immunol Ther Exp (Warsz) (2005) 53:13–27.
19. Meyer TC, Michalik S, Holtfreter S, Weiss S, Friedrich N, Völzke H, et al. A comprehensive view on the human antibody repertoire against staphylococcus aureus antigens in the general population. Front Immunol (2021) 12:651619. doi: 10.3389/fimmu.2021.651619
20. Grumann D, Ruotsalainen E, Kolata J, Kuusela P, Järvinen A, Kontinen VP, et al. Characterization of infecting strains and superantigen-neutralizing antibodies in Staphylococcus aureus bacteremia. Clin Vaccine Immunol (2011) 18:487–93. doi: 10.1128/CVI.00329-10
21. Verkaik NJ, Dauwalder O, Antri K, Boubekri I, de Vogel CP, Badiou C, et al. Immunogenicity of toxins during Staphylococcus aureus infection. Clin Infect Dis (2010) 50:61–8. doi: 10.1086/648673
22. Kanclerski K, Söderquist B, Kjellgren M, Holmberg H, Möllby R. Serum antibody response to Staphylococcus aureus enterotoxins and TSST-1 in patients with septicaemia. J Med Microbiol (1996) 44:171–7. doi: 10.1099/00222615-44-3-171
23. van Belkum A, Verkaik NJ, de Vogel CP, Boelens HA, Verveer J, Nouwen JL, et al. Reclassification of Staphylococcus aureus nasal carriage types. J Infect Dis (2009) 199:1820–6. doi: 10.1086/599119
24. Sangvik M, Olsen RS, Olsen K, Simonsen GS, Furberg A-S, Sollid JU. Age- and gender-associated Staphylococcus aureus spa types found among nasal carriers in a general population: the Tromso Staph and Skin Study. J Clin Microbiol (2011) 49:4213–8. doi: 10.1128/JCM.05290-11
25. Ruimy R, Armand-Lefevre L, Barbier F, Ruppé E, Cocojaru R, Mesli Y, et al. Comparisons between Geographically Diverse Samples of Carried Staphylococcus aureus. J Bacteriol (2009) 191:5577–83. doi: 10.1128/JB.00493-09
26. Verkaik NJ, de Vogel CP, Boelens HA, Grumann D, Hoogenboezem T, Vink C, et al. Anti-staphylococcal humoral immune response in persistent nasal carriers and noncarriers of Staphylococcus aureus. J Infect Dis (2009) 199:625–32. doi: 10.1086/596743
27. Vergeront JM, Stolz SJ, Crass BA, Nelson DB, Davis JP, Bergdoll MS. Prevalence of serum antibody to staphylococcal enterotoxin F among Wisconsin residents: implications for toxic-shock syndrome. J Infect Dis (1983) 148:692–8. doi: 10.1093/infdis/148.4.692
28. Parsonnet J, Goering RV, Hansmann MA, Jones MB, Ohtagaki K, Davis CC, et al. Prevalence of toxic shock syndrome toxin 1 (TSST-1)-producing strains of Staphylococcus aureus and antibody to TSST-1 among healthy Japanese women. J Clin Microbiol (2008) 46:2731–8. doi: 10.1128/jcm.00228-08
29. Stolz SJ, Davis JP, Vergeront JM, Crass BA, Chesney PJ, Wand PJ, et al. Development of serum antibody to toxic shock toxin among individuals with toxic shock syndrome in Wisconsin. J Infect Dis (1985) 151:883–9. doi: 10.1093/infdis/151.5.883
30. Völzke H, Schössow J, Schmidt CO, Jürgens C, Richter A, Werner A, et al. Cohort profile update: the study of health in pomerania (SHIP). Int J Epidemiol (2022) 51:e372–83. doi: 10.1093/ije/dyac034
31. Völzke H, Alte D, Schmidt CO, Radke D, Lorbeer R, Friedrich N, et al. Cohort profile: the study of health in Pomerania. Int J Epidemiol (2011) 40:294–307. doi: 10.1093/ije/dyp394
32. Meyer TC, Schmidt F, Steinke J, Bröker BM, Völker U, Michalik S. Technical report: xMAPr - High-dynamic-range (HDR) quantification of antigen-specific antibody binding. J Proteomics (2020) 212:103577. doi: 10.1016/j.jprot.2019.103577
33. Holtfreter S, Grumann D, Schmudde M, Nguyen HT, Eichler P, Strommenger B, et al. Clonal distribution of superantigen genes in clinical Staphylococcus aureus isolates. J Clin Microbiol (2007) 45:2669–80. doi: 10.1128/JCM.00204-07
34. Akoglu H. User’s guide to correlation coefficients. Turk J Emerg Med (2018) 18:91–3. doi: 10.1016/j.tjem.2018.08.001
35. McCarthy S, Das S, Kretzschmar W, Delaneau O, Wood AR, Teumer A, et al. A reference panel of 64,976 haplotypes for genotype imputation. Nat Genet (2016) 48:1279–83. doi: 10.1038/ng.3643
36. Lange V, Böhme I, Hofmann J, Lang K, Sauter J, Schöne B, et al. Cost-efficient high-throughput HLA typing by MiSeq amplicon sequencing. BMC Genomics (2014) 15:63. doi: 10.1186/1471-2164-15-63
37. Kawaguchi S, Higasa K, Shimizu M, Yamada R, Matsuda F. HLA-HD: An accurate HLA typing algorithm for next-generation sequencing data. Hum Mutat (2017) 38:788–97. doi: 10.1002/humu.23230
38. Willer CJ, Li Y, Abecasis GR. METAL: fast and efficient meta-analysis of genomewide association scans. Bioinformatics (2010) 26:2190–1. doi: 10.1093/bioinformatics/btq340
39. Luo Y, Kanai M, Choi W, Li X, Sakaue S, Yamamoto K, et al. A high-resolution HLA reference panel capturing global population diversity enables multi-ancestry fine-mapping in HIV host response. Nat Genet (2021) 53:1504–16. doi: 10.1038/s41588-021-00935-7
40. Parsonnet J, Hansmann MA, Delaney ML, Modern PA, Dubois AM, Wieland-Alter W, et al. Prevalence of toxic shock syndrome toxin 1-producing Staphylococcus aureus and the presence of antibodies to this superantigen in menstruating women. J Clin Microbiol (2005) 43:4628–34. doi: 10.1128/JCM.43.9.4628-4634.2005
41. Bavari S, Ulrich RG, LeClaire RD. Cross-reactive antibodies prevent the lethal effects of Staphylococcus aureus superantigens. J Infect Dis (1999) 180:1365–9. doi: 10.1086/314977
42. McCormick JK, Yarwood JM, Schlievert PM. Toxic shock syndrome and bacterial superantigens: an update. Annu Rev Microbiol (2001) 55:77–104. doi: 10.1146/annurev.micro.55.1.77
43. Holtfreter S, Bauer K, Thomas D, Feig C, Lorenz V, Roschack K, et al. egc-Encoded superantigens from Staphylococcus aureus are neutralized by human sera much less efficiently than are classical staphylococcal enterotoxins or toxic shock syndrome toxin. Infect Immun (2004) 72:4061–71. doi: 10.1128/IAI.72.7.4061-4071.2004
44. Bergdoll MS. A new Staphylococcal Enterotoxin, Enterotoxin F, associated with Toxic-shock-Syndrome Staphylococcus aureus isolates. Lancet (1981) 317:1017–21. doi: 10.1016/s0140-6736(81)92186-3
45. Chow AW, Bartlett KH, Percival-Smith R, Morrison BJ. Vaginal colonization with Staphylococcus aureus, positive for toxic-shock marker protein, and Escherichia coli in healthy women. J Infect Dis (1984) 150:80–4. doi: 10.1093/infdis/150.1.80
46. Linnemann CC, Staneck JL, Hornstein S, Barden TP, Rauh JL, Bonventre PF, et al. The epidemiology of genital colonization with Staphylococcus aureus. Ann Intern Med (1982) 96:940–4. doi: 10.7326/0003-4819-96-6-940
47. Pang LT, Kum WW, Chow AW. Inhibition of staphylococcal enterotoxin B-induced lymphocyte proliferation and tumor necrosis factor alpha secretion by MAb5, an anti-toxic shock syndrome toxin 1 monoclonal antibody. Infect Immun (2000) 68:3261–8. doi: 10.1128/IAI.68.6.3261-3268.2000
48. Kum WW, Chow AW. Inhibition of staphylococcal enterotoxin A-induced superantigenic and lethal activities by a monoclonal antibody to toxic shock syndrome toxin-1. J Infect Dis (2001) 183:1739–48. doi: 10.1086/320732
49. Schwameis M, Roppenser B, Firbas C, Gruener CS, Model N, Stich N, et al. Safety, tolerability, and immunogenicity of a recombinant toxic shock syndrome toxin (rTSST)-1 variant vaccine: a randomised, double-blind, adjuvant-controlled, dose escalation first-in-man trial. Lancet Infect Dis (2016) 16:1036–44. doi: 10.1016/S1473-3099(16)30115-3
50. Roetzer A, Stich N, Model N, Schwameis M, Firbas C, Jilma B, et al. High titer persistent neutralizing antibodies induced by TSST-1 variant vaccine against toxic shock cytokine storm. Toxins (Basel) (2020) 12. doi: 10.3390/toxins12100640
51. Amanna IJ, Carlson NE, Slifka MK. Duration of humoral immunity to common viral and vaccine antigens. N Engl J Med (2007) 357:1903–15. doi: 10.1056/NEJMoa066092
52. Goel RR, Painter MM, Lundgreen KA, Apostolidis SA, Baxter AE, Giles JR, et al. Efficient recall of Omicron-reactive B cell memory after a third dose of SARS-CoV-2 mRNA vaccine. Cell (2022) 185:1875–1887.e8. doi: 10.1016/j.cell.2022.04.009
53. Aalberse RC, Stapel SO, Schuurman J, Rispens T. Immunoglobulin G4: an odd antibody. Clin Exp Allergy: J Br Soc Allergy Clin Immunol (2009) 39:469–77. doi: 10.1111/j.1365-2222.2009.03207.x
54. Perugino CA, Stone JH. IgG4-related disease: an update on pathophysiology and implications for clinical care. Nat Rev Rheumatol (2020) 16:702–14. doi: 10.1038/s41584-020-0500-7
55. Durham SR, Shamji MH. Allergen immunotherapy: past, present and future. Nat Rev Immunol (2022) 23:1–12. doi: 10.1038/s41577-022-00786-1
56. Akiyama M, Yasuoka H, Yamaoka K, Suzuki K, Kaneko Y, Kondo H, et al. Enhanced IgG4 production by follicular helper 2 T cells and the involvement of follicular helper 1 T cells in the pathogenesis of IgG4-related disease. Arthritis Res Ther (2016) 18:167. doi: 10.1186/s13075-016-1064-4
57. Jeannin P, Lecoanet S, Delneste Y, Gauchat JF, Bonnefoy JY. IgE versus IgG4 production can be differentially regulated by IL-10. J Immunol (1998) 160:3555–61. doi: 10.4049/jimmunol.160.7.3555
58. Platts-Mills T, Vaughan J, Squillace S, Woodfolk J, Sporik R. Sensitisation, asthma, and a modified Th2 response in children exposed to cat allergen: a population-based cross-sectional study. Lancet (2001) 357:752–6. doi: 10.1016/S0140-6736(00)04168-4
59. Satoguina JS, Weyand E, Larbi J, Hoerauf A. T regulatory-1 cells induce IgG4 production by B cells: role of IL-10. J Immunol (2005) 174:4718–26. doi: 10.4049/jimmunol.174.8.4718
60. Panhuber A, Lamorte G, Bruno V, Cetin H, Bauer W, Höftberger R, et al. A systematic review and meta-analysis of HLA class II associations in patients with IgG4 autoimmunity. Sci Rep (2022) 12:9229. doi: 10.1038/s41598-022-13042-2
61. Rasmussen KF, Sprogøe U, Nielsen C, Shalom D-B, Assing K. Time-related variation in IgG subclass concentrations in a group of healthy Danish adults. Immun Inflammation Dis (2021) 9:1009–15. doi: 10.1002/iid3.464
62. Jazayeri MH, Pourfathollah AA, Rasaee MJ, Porpak Z, Jafari ME. The concentration of total serum IgG and IgM in sera of healthy individuals varies at different age intervals. Biomed. Aging Pathol (2013) 3:241–5. doi: 10.1016/j.biomag.2013.09.002
63. Fraser JD, Proft T. The bacterial superantigen and superantigen-like proteins. Immunol Rev (2008) 225:226–43. doi: 10.1111/j.1600-065X.2008.00681.x
64. Scholl P, Diez A, Mourad W, Parsonnet J, Geha RS, Chatila T. Toxic shock syndrome toxin 1 binds to major histocompatibility complex class II molecules. Proc Natl Acad Sci U.S.A. (1989) 86:4210–4. doi: 10.1073/pnas.86.11.4210
65. Karp DR, Teletski CL, Scholl P, Geha R, Long EO. The alpha 1 domain of the HLA-DR molecule is essential for high-affinity binding of the toxic shock syndrome toxin-1. Nature (1990) 346:474–6. doi: 10.1038/346474a0
66. Llewelyn M, Sriskandan S, Peakman M, Ambrozak DR, Douek DC, Kwok WW, et al. HLA class II polymorphisms determine responses to bacterial superantigens. J Immunol (2004) 172:1719–26. doi: 10.4049/jimmunol.172.3.1719
67. Krogman A, Tilahun A, David CS, Chowdhary VR, Alexander MP, Rajagopalan G. HLA-DR polymorphisms influence in vivo responses to staphylococcal toxic shock syndrome toxin-1 in a transgenic mouse model. HLA (2017) 89:20–8. doi: 10.1111/tan.12930
68. Kotb M, Norrby-Teglund A, McGeer A, El-Sherbini H, Dorak MT, Khurshid A, et al. An immunogenetic and molecular basis for differences in outcomes of invasive group A streptococcal infections. Nat Med (2002) 8:1398–404. doi: 10.1038/nm1202-800
69. Kim J, Urban RG, Strominger JL, Wiley DC. Toxic shock syndrome toxin-1 complexed with a class II major histocompatibility molecule HLA-DR1. Science (1994) 266:1870–4. doi: 10.1126/science.7997880
70. Wen R, Cole GA, Surman S, Blackman MA, Woodland DL. Major histocompatibility complex class II-associated peptides control the presentation of bacterial superantigens to T cells. J Exp Med (1996) 183:1083–92. doi: 10.1084/jem.183.3.1083
71. Dendrou CA, Petersen J, Rossjohn J, Fugger L. HLA variation and disease. Nat Rev Immunol (2018) 18:325–39. doi: 10.1038/nri.2017.143
72. Sakaue S, Gurajala S, Curtis M, Luo Y, Choi W, Ishigaki K, et al. Tutorial: a statistical genetics guide to identifying HLA alleles driving complex disease. Nat Protoc (2023). doi: 10.1038/s41596-023-00853-4
73. Liu P, Yao M, Gong Y, Song Y, Chen Y, Ye Y, et al. Benchmarking the human leukocyte antigen typing performance of three assays and seven next-generation sequencing-based algorithms. Front Immunol (2021) 12:652258. doi: 10.3389/fimmu.2021.652258
74. Xin H, Li J, Sun H, Zhao N, Yao B, Zhong W, et al. Benchmarking of 5 algorithms for high-resolution genotyping of human leukocyte antigen class I genes from blood and tissue samples. Ann Transl Med (2022) 10:633. doi: 10.21037/atm-22-875
75. Hurley CK, Kempenich J, Wadsworth K, Sauter J, Hofmann JA, Schefzyk D, et al. Common, intermediate and well-documented HLA alleles in world populations: CIWD version 3.0.0. HLA (2020) 95:516–31. doi: 10.1111/tan.13811
76. Harmsen D, Claus H, Witte W, Rothgänger J, Claus H, Turnwald D, et al. Typing of Methicillin-Resistant Staphylococcus aureus in a University Hospital Setting by Using Novel Software for spa Repeat Determination and Database Management. J Clin Microbiol (2003) 41:5442–8. doi: 10.1128/JCM.41.12.5442-5448.2003
77. Mellmann A, WeNiger T, Berssenbrügge C, Rothgänger J, Sammeth M, Stoye J, et al. Based Upon Repeat Pattern (BURP): an algorithm to characterize the long-term evolution of Staphylococcus aureus populations based on spa polymorphisms. BMC Microbiol (2007) 7:98. doi: 10.1186/1471-2180-7-98
78. Kawaguchi S, Higasa K, Yamada R, Matsuda F. Comprehensive HLA typing from a current allele database using next-generation sequencing data. Methods Mol Biol (2018) 1802:225–33. doi: 10.1007/978-1-4939-8546-3_16
79. Wickham H, Averick M, Bryan J, Chang W, McGowan LD, François R, et al. Welcome to the tidyverse. J Open Source Software (2019) 4:1686. doi: 10.21105/joss.01686
80. Viechtbauer W. Conducting meta-analyses in R with the metafor package. J Stat Software (2010) 36:1–48. doi: 10.18637/jss.v036.i03
Keywords: Staphylococcus aureus, toxic shock syndrome, TSST-1, superantigen, GWA, HLA, MHC, antibody
Citation: Weiss S, Holtfreter S, Meyer TC, Schmiedeke F, Cammann C, Dörr M, Felix SB, Grabe HJ, Homuth G, Kohler C, Mahncke C, Michalik S, Nauck M, Friedrich N, Samietz S, Völzke H, Völker U and Bröker BM (2023) Toxin exposure and HLA alleles determine serum antibody binding to toxic shock syndrome toxin 1 (TSST-1) of Staphylococcus aureus. Front. Immunol. 14:1229562. doi: 10.3389/fimmu.2023.1229562
Received: 26 May 2023; Accepted: 31 July 2023;
Published: 04 September 2023.
Edited by:
Isabelle Bekeredjian-Ding, Paul-Ehrlich-Institut (PEI), GermanyReviewed by:
Davide Firinu, University of Cagliari, ItalyCopyright © 2023 Weiss, Holtfreter, Meyer, Schmiedeke, Cammann, Dörr, Felix, Grabe, Homuth, Kohler, Mahncke, Michalik, Nauck, Friedrich, Samietz, Völzke, Völker and Bröker. This is an open-access article distributed under the terms of the Creative Commons Attribution License (CC BY). The use, distribution or reproduction in other forums is permitted, provided the original author(s) and the copyright owner(s) are credited and that the original publication in this journal is cited, in accordance with accepted academic practice. No use, distribution or reproduction is permitted which does not comply with these terms.
*Correspondence: Barbara M. Bröker, QmFyYmFyYS5icm9la2VyQG1lZC51bmktZ3JlaWZzd2FsZC5kZQ==; Uwe Völker, dm9lbGtlckB1bmktZ3JlaWZzd2FsZC5kZQ==
†These authors share first authorship
‡These authors share last authorship
Disclaimer: All claims expressed in this article are solely those of the authors and do not necessarily represent those of their affiliated organizations, or those of the publisher, the editors and the reviewers. Any product that may be evaluated in this article or claim that may be made by its manufacturer is not guaranteed or endorsed by the publisher.
Research integrity at Frontiers
Learn more about the work of our research integrity team to safeguard the quality of each article we publish.