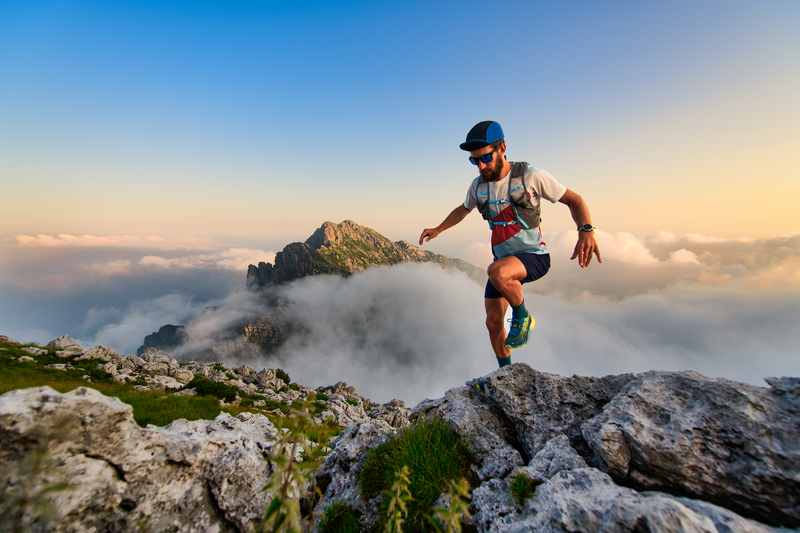
95% of researchers rate our articles as excellent or good
Learn more about the work of our research integrity team to safeguard the quality of each article we publish.
Find out more
REVIEW article
Front. Immunol. , 09 August 2023
Sec. Microbial Immunology
Volume 14 - 2023 | https://doi.org/10.3389/fimmu.2023.1228754
This article is part of the Research Topic Crosstalk Between the GI Redox Environment, Microbiome, and the Immune System View all 5 articles
Frequent use of hormones and drugs may be associated with side-effects. Recent studies have shown that probiotics have effects on the prevention and treatment of immune-related diseases. Limosilactobacillus reuteri (L. reuteri) had regulatory effects on intestinal microbiota, host epithelial cells, immune cells, cytokines, antibodies (Ab), toll-like receptors (TLRs), tryptophan (Try) metabolism, antioxidant enzymes, and expression of related genes, and exhibits antibacterial and anti-inflammatory effects, leading to alleviation of disease symptoms. Although the specific composition of the cell-free supernatant (CFS) of L. reuteri has not been clarified, its efficacy in animal models has drawn increased attention to its potential use. This review summarizes the effects of L. reuteri on intestinal flora and immune regulation, and discusses the feasibility of its application in atopic dermatitis (AD), asthma, necrotizing enterocolitis (NEC), systemic lupus erythematosus (SLE), rheumatoid arthritis (RA), and multiple sclerosis (MS), and provides insights for the prevention and treatment of immune-related diseases.
In recent years, with the development of the concepts of gut-lung axis, gut-brain axis, gut-liver axis, and gut-skin axis, increased attention has been paid to the impact of intestinal flora on health. In particular, the discovery that probiotics can reshape gut flora (1) has triggered a wave of research focused on the gut microbiome. Probiotics colonize and regulate the unbalanced microflora of the host, directly acting on host epithelial and immune cells and regulating the epithelial or immune cells of specific tissues of the host through their metabolites in situ or by entering the circulation and remodeling the microenvironment of the lesion site (2). Unlike drugs, probiotics are associated with less side-effects, such as intestinal ecological disorder and immune dysfunction (3). Various forms of probiotic preparations are available. The employment of heat-killed probiotics, cell-free supernatant (CFS) of probiotics and specific components of purification, or genetic engineering editing of probiotics to obtain certain specific functions and avoid side-effects is an emerging trend in this field (4).
Lactobacillus is one of the earliest discovered probiotics (5) and is most commonly used in foods and dietary supplements (6). It can be isolated from a wide range of sources, including human or animal milk, feces, and fermented foods (7–9). Lactobacillus acidophilus, Lactobacillus rhamnosus (L. rhamnosus), and Limosilactobacillus reuteri (L. reuteri) are currently the most widely used probiotics (10–12). Probiotics have a wide range of functions, including resistance to pathogenic microorganisms, regulation of immunity, regulation of metabolism such as cholesterol and sugar metabolism, antioxidant, and antitumor effects. Notably, the probiotic-related protective mechanisms of lactobacilli on the host are strain-dependent (13).
L. reuteri is one of the most studied strains of the Lactobacillus genus. Its morphology and growth are affected by the host genotype and environmental factors, such as temperature, pH, oxygen concentration, and host dietary components. Genetic and environmental differences lead to phenotypic heterogeneity of L. reuteri strains (14, 15). L. reuteri may play a regulatory role in several systemic diseases through a very intricate immunoregulatory mechanism. First, it colonizes and survives in the gastrointestinal tract by utilizing its acid-base resistant and adhesion properties. Subsequently, it interacts with host intestinal epithelial cells (IECs), by regulating the intestinal flora, to enhance intestinal mucosal barrier, regulate immune cells, inflammatory factors, chemokines, and antibodies, produce indole derivative from tryptophan, secrete exopolysaccharide (EPS) and other bioactive factors, enhance tight junctions (TJs), regulate gene expression, improve antioxidant activity, and further regulate the immune system of the host. As such, L. reuteri has the potential to be used as a new therapeutic or adjunctive therapy in atopic or autoimmune diseases. Previous reviews have focused on the use of L. reuteri in gastrointestinal diseases such as colic and diarrhea. Based on its potential role in immune regulation, this review summarizes the immunomodulatory molecular mechanisms of different strains of L. reuteri. We searched for studies on the application of L. reuteri in disease and found that it was applied to diseases or disease models in multiple systems, with digestive and immune-related diseases being the most common. In these two categories of diseases, the existing reviews and meta-analyses focused on a single L. reuteri strain or a single digestive system disease, and no review summarized the application of different strains of L. reuteri in immune-related diseases. This review summarized the studies on the application of L. reuteri in immune-related diseases. We explored the application value of L. reuteri in these immune-related diseases such as atopic dermatitis, asthma, necrotizing enterocolitis, systemic lupus erythematosus, rheumatoid arthritis, and multiple sclerosis.
For probiotics to exert their beneficial effects on the host organism, some basic characteristics should be fulfilled. First, they need to be resistant to acid and bile salts to survive the passage through the gastrointestinal tract. Second, they should be able to adhere to mucus and IECs to colonize the host. Only after these conditions are met can probiotics exert their anti-infection, anti-inflammatory, and antioxidant properties in the host (16).
The homeostasis of intestinal microorganisms is essential for effective host intestinal barrier function and normal immune responses (17). An imbalance in intestinal microecology decreases the immune tolerance of the host to allergens and causes autoimmune reactions (18). For example, L. reuteri is known to adhere to and aggregate mucus and IECs, hindering the interaction of pathogens with the host, thus constituting a defensive barrier against invading pathogens (19). L. reuteri has also been reported to enrich intestinal microbial diversity and regulate the relative abundance of adminis, mainly manifesting as an increase in the numbers of beneficial genera and decrease in those of harmful genera. For example, studies have shown that following the administration of L. reuteri, the numbers of Lactobacillus and Bifidobacterium were increased (20, 21), whereas those of Escherichia coli (E.coli), Staphylococcus, and Ruminococcus were decreased (22, 23), thus balancing intestinal microecology (Figure 1A). However, no consensus has been reached regarding its influence on microbial composition by heredity, environment, and strain specificity. The antibacterial effect of L. reuteri is achieved through the production of organic acids, which regulate the local pH and facilitate the growth of beneficial bacteria (24), or through the production of peroxides(PEROXs) and reuterin that inhibit the growth of pathogens (25) (Figure 1A). Among these antimicrobial metabolites, reuterin is of the most significance. It metabolizes glycerol to hydroxypropionaldehyde (3-HPA) (26). It’s the chemical nature of reuterin. This process is mediated by glycerol dehydratase and assisted by coenzyme B12 (27, 28). 3-HPA is further converted into acrolein, which exerts cytotoxic effects and inhibits the growth of gram-negative bacilli. This may be the mechanism by which reuterin exerts its antibacterial effect (29, 30). L. reuteri has also been shown to inhibit the formation of bacterial biofilms (Figure 1A). In addition, L. reuteri inhibited the gene expression of invading bacteria, thus limiting their growth and virulence (25). In particular, L. reuteri activated the Wnt/β-catenin pathway, leading to increased expression of antimicrobial peptides (AMPs), thus inhibiting the colonization of C. rodentium (31) (Figure 1A). However, some studies have reported that L. reuteri had no effect on the abundance of E. coli and Group B Streptococcus (GBS) strains (32, 33).
Figure 1 Changes in microbial structure, metabolites, and expression of functional genes. (A) L. reuteri regulated the relative abundance of microorganisms. The antibacterial effect of L. reuteri is achieved through the production of LAC, which regulates the local pH and facilitates the growth of beneficial bacteria, or through the production of PEROXs and bacteriocins. L. reuteri activated the Wnt/β-catenin pathway, leading to increased expression of AMPs, thus inhibiting the colonization of C. rodentium. E.coli, Escherichia coli; LAC, lactic acid; PEROXs, peroxides; AMPs, antimicrobial peptides. (B) L. reuteri increased the levels of amino acid metabolites by regulating the urea, TCA, and methionine cycles, thus enhancing Try metabolism and in turn the anti-inflammatory ability. L. reuteri metabolized dietary Try to produce IAld, which acts as a ligand for the activation of the AHR on the surface of CD4+ T-cells, and induces ILC3s to produce IL-22. This process enhanced host resistance to C. albicans and protected against mucosal inflammation. Similarly, through the activation of Try metabolism and production of ILA that activate AHR and downregulate Thpok, L. reuteri promoted the differentiation of CD4+ T-cells into CD4+CD8αα+ DP IELs. The CSF of L. reuteri includes some of its metabolites and secreted bioactive factors, such as SCFAs, organic acids (such as LAC), hydrogen peroxide, bacteriocin compounds (such as reuterin), and EPS. The carbohydrate and fatty acid metabolites in CFS of L. reuturi AN417 has antibacterial action against oral pathogens. Binding of EPS of L. reuteri I5007 to PRRs was suggested to downregulate inflammatory factors and upregulate claudin-1, occludin, and ZO-1, thus enhancing intestinal barrier function. The CFS of L. reuteri RC-14 combined with that of LGR-1 inhibited the secretion of IL-8 and IP-10. It was shown to inhibit the colonization and growth of C. albicans and occurrence of VVC. TCA, tricarboxylic acid; Try, tryptophan; IAld, indole-3-aldehyde; AHR, aryl hydrocarbon receptor; ILC3s, group 3 innate lymphoid cells; IL-22, interleukin-22; C. albicans, Candida albicans; ILA, indole lactic acid; DP IELs, double-positive intraepithelial lymphocytes; CFS, Cell-free supernatant; EPS, exopolysaccharide; PRRs, pattern recognition receptors; ZO-1, zonula occludens 1; LGR-1, Lacticaseibacillus rhamnosus GR-1; IP-10, interferon-inducible protein-10; VVC, vulvovaginal candidiasis; SCFAs, short-chain fatty acids; LAC, lactic acid. (C) L. reuteri 22 promoted the mRNA expression of LZM and enhanced congenital immunity of intestinal mucosa. L. reuteri DSM 17938 increased the expression of skin epidermal AQP3 and lamininA/B, which strengthens skin barrier function. It also upregulated the expression of genes of opioid peptide, OPRK1, and CD200, which are related to stress and pain in the brain and are involved in anti-inflammatory signaling pathways. L. reuteri -LFCA improved oxidative stress-related indicators by activating Nrf2/HO-1 signaling pathway. The level of MDA was reduced, whereas those of enzymes, such as SOD, GSH-Px, and catalase were increased. L. reuteri DSM 17938 had similar effects. LZM, lysozyme; AQP3, aquaporin 3; kappa-opioid receptor 1, OPRK1; Nrf2/HO-1, nuclear factor E2-related factor 2/Heme oxygenase1; MDA, malondialdehyde; SOD, superoxide dismutase; GSH-Px, glutathione peroxidase.
Tryptophan catabolites: Normal tryptophan (Try) metabolism is an important process in maintaining intestinal mucosal homeostasis. Notably, L. reuteri increased the levels of amino acid metabolites by regulating the urea, tricarboxylic acid, and methionine cycles, thus enhancing Try metabolism and in turn the anti-inflammatory ability in mice (23). Studies have shown that L. reuturi metabolizes dietary Try to produce indole-3-aldehyde (IAld), which acts as a ligand for the activation of the aryl hydrocarbon receptor (AHR) on the surface of CD4+ T-cells (34), and induces group 3 innate lymphoid cells (ILC3s) to produce interleukin-22 (IL-22) (35). This process enhanced host resistance to Candida albicans (C. albicans) and protected against mucosal inflammation (34) (Figure 1B). Similarly, through the activation of Try metabolism and production of indoles that activate AHR and downregulate the transcription factor Thpok, L. reuteri promoted the differentiation of CD4+ T-cells into CD4+CD8αα+ double-positive intraepithelial lymphocytes (DP IELs) (36) (Figure 1B). Therefore, promoting Try catabolism might be one of the mechanisms by which L.reuturi exerts its anti-inflammatory and anti-infection properties.
Cell-free supernatant (CFS): The L. reuturi AN417 strain has no antibacterial action against oral pathogens, whereas its carbohydrate and fatty acid metabolites in CFS do (37) (Figure 1B). Similarly, L. reuturi I5007 exerts regulatory effects on inflammatory factors and TJs (38). Binding of EPS, a possible CFS component, to pattern recognition receptors (PRRs) (39), was suggested to downregulate inflammatory factors and upregulate TJs, such as claudin-1, occludin, and zonula occludens 1 (ZO-1), thus enhancing intestinal barrier function (38) (Figure 1B). Of note, the CFS of L.reuteri RC-14 alone or combined with Lacticaseibacillus rhamnosus GR-1 (LGR-1) was not effective against vulvovaginal candidiasis (VVC), whereas their combined CFS inhibited the secretion of IL-8 and chemotactic factor interferon-inducible protein-10 (IP-10) from human vaginal epithelial VK2/E6E7 cells. Eventually, it was shown to inhibit the colonization and growth of C. albicans and occurrence of VVC (40) (Figure 1B). The CSF of L. reuteri includes some of its metabolites and secreted bioactive factors, such as short-chain fatty acids (SCFAs), organic acids (such as LAC), hydrogen peroxide, bacteriocin compounds (such as reuterin), and EPS (Figure 1B). Several recent studies have demonstrated the effectiveness of CFS. The specific active components and their mechanisms of action can be further explored in the future, potentially enabling major discoveries in the field of microbiome research.
A study found that L. reuteri 22 greatly promoted the mRNA expression of lysozyme (LZM) and enhanced congenital immunity of intestinal mucosa (41) (Figure 1C). In addition, a number of differentially expressed microRNAs (miRNAs) were detected in newborn piglets that were orally administered L. reuteri I5007. These miRNAs were involved in the phosphatidylinositol-3-hydroxykinase (PI3K)-protein kinaseB (AKT) and mitogen-activated protein kinase (MAPK) pathways, playing an important role in the probiotic-host crosstalk. In addition, the expression of ssc-miR-196a/-196b-5p was significantly increased, downregulating the mRNA expression of IL-1β and TNF-α in IPEC-J2 cells (42) (Figure 2G). L. reuteri DSM 17938 was reported to significantly increase the expression of skin epidermal aquaporin 3 (AQP3) and lamininA/B, which strengthens skin barrier function (43) (Figure 1C), and upregulated the expression of genes of opioid peptide, kappa-opioid receptor 1 (OPRK1), and CD200, which are related to stress and pain in the brain and are involved in anti-inflammatory signaling pathways (44) (Figure 1C). In vitro experiments have shown that oral administration of L. reuteri -LFCA improved oxidative stress-related indicators by activating the nuclear factor E2-related factor 2 (Nrf2)/Heme oxygenase1 (HO-1) signaling pathway (45, 46). Moreover, the level of malondialdehyde (MDA) was reduced, whereas those of enzymes, such as superoxide dismutase (SOD), glutathione peroxidase (GSH-Px), and catalase were increased (46) (Figure 1C), thus enhancing the antioxidant capacity of cells. Similar effects of L. reuteri DSM 17938 (Figure 1C)—used as a new treatment for reducing intestinal inflammation and repairing intestinal damage—have been reported (47).
Figure 2 Regulation of the differentiation and function of immune cells. (A) T-cells. L. reuteri 17938 and L. reuteri Fn041 stimulated the production of Foxp3+ Treg cells. Similarly, h-L.reuteri increased the numbers of Treg cells in the spleen and joint drainage lymph nodes. L. reuteri has a structure similar to that of the mouse MOG. It cooperated with erysipelas bacteria to activate CD4+ T-cells, which have the ability to attack MOG, leading to worsening of EAE. h-L. reuteri, heat-killed L. reuteri; MOG, myelin oligodendrocyte glycoprotein; EAE, experimental autoimmune encephalomyelitis. (B) Dendritic cells. L. reuturi ATTC PTA 6475 and its secreted factors induced the expression of CD83 and CD86 on the surface of iDCs and promoted the production of IL-10 by DCs. L. reuteri CO21 inhibited the expression of CD40 and MHCII on the surface of DCs and the activation and proliferation of CD4 + T-cells induced by DCs, thus achieving an anti-inflammatory effect. H-L.reuteri increased the numbers of CD103 + DCs, resulting in a reduction of the level of inflammation and improvement of disease severity. iDCs, immature dendritic cells; IL-10, interleukin-10; DCs, dendritic cells. (C) ILC3s. L. reuteri ATCC PTA 6475 reduced the number of ILC3s. L. reuteri CF48-3A promoted the production of ILC3s and induced the differentiation of IgA+ B–cells, leading to the generation of IgA. However, whether the IgA play a protective role against infection and enhances mucosal immunity or induces autoimmune effects remains unclear. ILC3s, Group 3 innate lymphoid cells. (D) Macrophages and NEs. L. reuteri increased production of NO by activated and increased numbers of macrophages, enhancing their phagocytotic ability. Meanwhile, L. reuteri inhibited the inflammation induced by bacterial infection by regulating the levels of NO. However, L. reuteri reduced the GBS-induced proliferation of NEs. NEs, neutrophils; NO, nitric oxide; GBS, Group B Streptococcus. (E) TLR2. L. reuteri 17938 is a bacterium that following recognition by TLR2 mediates anti-inflammatory immune response in the following ways: First, it led to an increase in the number of Foxp3+ Treg cells and a decrease in that of CD4+CD44+ Teffs. Second, it induced the generation and activation of CD103+ DCs, which were characterized by the expression of co-stimulative markers CD80 and CD86. This DC-induced production of Foxp3+ Treg cells is crucial for maintaining intestinal immune tolerance. Finally, activation of TLR2 mediated the decrease in the levels of IL-1β and IFN-γ.TLR2, Toll-like receptor 2; Teffs, effector T-cells; IFN-γ, interferon-γ. (F) Promotion of synthesis and secretion of Ig. L. reuteri FN041 increased the synthesis of sIgA. L. reuteri CRL1324 not only stimulated the production of IgA, but also that of IgG, which is related to anti-infection functions. An increase in the numbers of lactobacilli and enterococci caused by administration of mixed strains of L. reuteri were correlated with increased levels of IgE. Ig, Immunoglobulin; sIgA, secreted IgA. (G) Regulation of cytokines for improving intestinal mucosal barrier structure and permeability.
The integrated intestinal barrier structure and its normal function constitute a meaningful immune defense barrier against pathogenic microbes. In addition to the above pathways, L. reuteri can also directly enhance intestinal barrier function by acting on intestinal stem cells (ISCs) and TJs of IECs. L. reuteri is known to promote the development and differentiation of IECs, maintaining the integrity of intestinal mucosa. For example, administration of both L. reuteri D8 and L. reuteri 22 increased the number of ISCs, Lgr5+ cells, and promoted the proliferation of IECs by activating the Wnt/β-catenin pathway (31, 41). Of note, L. reuteri 22 inhibited Notch signaling pathway and induced the differentiation of ISCs into mucin-2 (Muc-2)-highly expressing goblet cells (41), whereas L. reuteri D8 induced the differentiation of ISCs into Paneth cells (31) (Figure 3), both of which are significant components of the intestinal barrier. Similarly, L. reuteri I5007 upregulated the expression of TJs, such as occludin, claudin, and ZO-1 of small IECs in piglets, thereby enhancing the intestinal mucosal barrier (38) (Figure 1B). Both L. reuteri DSM17938 and 1563F upregulated the expression of E-cadherin and TJs in infected IECs and competitively inhibited bacterial binding to TJs, thus inhibiting the increased infection-induced intestinal permeability and protecting intestinal barrier function (48) (Figure 3). Meanwhile, use of peptidoglycans on skin epidermis activated TLR2, resulting in the increased expression of TJs and enhanced skin barrier function (38, 39). However, whether peptidoglycans of probiotics can enhance the intestinal mucosal barrier through a similar mechanism of action remains to be clarified. Current animal studies have shown that the upregulation of the expression of TJs in intestinal epithelium can be achieved by inhibiting the TLR4/myeloid differentiation factor 88 (MyD88) signal transduction pathway and downregulating the myosin light chain kinase (MLCK) pathway (46) (Figure 3).
Figure 3 Protection of the integrity of intestinal mucosal barrier. Administration of both L. reuteri D8 and L. reuteri 22 increased the number of ISCs, Lgr5+ cells, and promoted the proliferation of IECs by activating the Wnt/β-catenin pathway. L. reuteri 22 inhibited Notch signaling pathway and induced the differentiation of ISCs into Muc-2-highly expressing goblet cells, whereas L. reuteri D8 induced the differentiation of ISCs into Paneth cells. Both L. reuteri DSM17938 and 1563F upregulated the expression of E-cadherin and TJs in infected IECs and competitively inhibited bacterial binding to TJs, thus inhibiting the increased infection-induced intestinal permeability and protecting intestinal barrier function. Upregulation of the expression of TJs in intestinal epithelium can be achieved by inhibiting the TLR4/MyD88 signal transduction pathway and downregulating the MLCK pathway. ISCs, intestinal stem cells; mucin-2, Muc-2; IECs, intestinal epithelial cells; TJs, tight junctions; TLR4, Toll-like receptor 4; MyD88, myeloid differentiation factor 88; MLCK, myosin light chain kinase.
Various studies have reported that L. reuteri not only promoted the increase of DPIELs in mice (36) but also induced the generation of regulatory T (Treg)-cells. For example, L. reuteri 17938 and L. reuteri Fn041 stimulated the production of Foxp3+ Treg cells in the intestinal mucosa (49–51) (Figure 2A), the reduction of crying in infants with colic was thought to be the therapeutic effect of L. reuteri 17938 (52). Similarly, heat-killed L. reuteri (h-L.reuteri) also induced the generation and peripheral migration of Treg cells and increased the numbers of α4β7+ Treg cells in the spleen and joint drainage lymph nodes (Figure 2A). Notably, despite the immunomodulatory effects reported on mice pretreated with h-L.reuteri, no increase in the number of α4β7+ Tregs in the spleen or symptom improvement was observed in mice with collagen-induced-arthritis (CIA) (53). This might have implications for the timing of clinical application of probiotics in the future. One study hypothesized that miRNAs in breast milk may be involved in immune regulation, based on which to explore whether L. reuteri has a role in miRNA expression. The results showed that miRNA did affect the number of resting Treg cells and activated Treg cells in infants, but L. reuteri did not affect miRNAs expression (54). Studies have shown that prenatal supplementation with L. reuteri induced hypomethylation of CD4+T-cells DNA. Compared to the placebo group, the probiotic group differentially methylated probes (DMPs) were further mapped to genes. Genes associated with chemotaxis, PI3K-Akt, MAPK and TGF-β signalling are activated, which are associated with immune activation and maturation of the baby at birth, and the occurrence of atopic disease (55). Administration of L.reuteri ATCC 55730 didn’t change the CD3+ CD8+ T lymphocytes in the human ileum, but significantly increased the CD4+ T lymphocytes (56). Studies have shown that the activation of T cells promoted the growth of intestinal epithelial cells in the lamina propria of human small intestine (57). The mechanism of L. reuteri maintaining host intestinal health may be related to this. A previous animal study was consistent with this. Poultry colonized with Salmonella enterica serovar Typhimurium showed a significant decrease in colonization after administration of L. reuteri (poultry strain), and an increase in CD4/CD8 ratio was observed in the ileum of poultry. However, in the rat model of enterocolitis, L. reuteri increased both CD4+ cells and CD8+ cells. This is related to the decrease of intestinal mucosal barrier permeability and the enhancement of barrier function. Notably, L. reuteri has a structure similar to that of the mouse myelin oligodendrocyte glycoprotein (MOG) and was shown to cooperate with erysipelas bacteria to activate small intestine CD4+ T-cells, which have the ability to attack MOG, leading to worsening of experimental autoimmune encephalomyelitis (EAE) (58) (Figure 2A).
Dendritic cells (DCs) are essential for the balance between autoimmunity and immune tolerance. In vitro experiments have shown that L. reuturi ATTC PTA 6475 and its secreted factors induced the expression of DCs maturation markers CD83 and CD86 on the surface of mouse bone marrow-derived immature dendritic cells (iDCs) and promoted the production of anti-inflammatory cytokine IL-10 by DCs. Mouse colonic organoids and animal models of acute colitis showed similar results (59) (Figure 2B). Similarly, L. reuteri induced the increased expression of IL-10 by DCs in BWF1 mice in vitro experiments (60). In general, L. reuteri and its metabolites have been shown to promote the maturation of DCs and enable their differentiation into anti-inflammatory phenotypes, which is of great significance for maintaining intestinal immune homeostasis. L. reuteri ATCC PTA 4659 also had a protective effect on colitis mice, but it significantly inhibited the growth of CD11b+CD11c+ DCs. It secreted the main inflammatory cytokines of colitis and made the distribution of immune cells tend to that of the control group (61). CD103 (alpha integrin) expressing CD11c+ DCs are essential for maintaining intestinal immune tolerance in mice (62). The lack of these DCs led to a decline in the number of Tregs and is prone to colitis (56). For mice not given L. reuteri 17938, about 20-30% of intestinal DCs expressed CD103, whether or not during NEC. L. reuteri 17938 significantly increased gut CD103+ DCs in WT mice, but this change was not observed in TLR2-/- mice. Thus, L. reuteri DSM 17938 stimulated expansion of CD103+ DCs dependent on TLR2, thereby promoting immune tolerance (49). Oral administration of L. reuteri CO21 (L. reuteri LFCA) in newborn piglets infected with enterotoxigenic Escherichia coli (ETEC) not only inhibited the expression of CD40 and MHCII on the surface of DCs, but also the activation and proliferation of CD4+ T-cells induced by monocyte-derived dendritic cells (moDCs), thus achieving an anti-inflammatory effect (46) (Figure 2B). Moreover, h-L.reuteri was also shown to exert a regulatory effect on DCs by inducing an increase in the numbers of CD103+ DCs in mesenteric lymph nodes (MLNs) in mice, resulting in a reduction of the level of inflammation and improvement of disease severity (59) (Figure 2B). Type I interferon (IFN-I) has been known as the important link of SLE (63). Plasmacytoid dendritic cells (pDCs) produced a large amount of IFN-I (64, 65). pDC has become a key therapeutic target for SLE. Together with autoantibodies, pDCs promoted the production of immunocomplexes. These immunocomplexes were deposited in the kidneys and blood vessels, causing lupus nephritis, vasculitis, and atherosclerosis (66). Increased lupus symptoms and expression of IFN-I genes in spleen and ileum were observed after colonization of L. reuteri in 6-week-old GF B6 mice. This may be the result of increased pDCs in the spleen and mesenteric lymph nodes (67).
ILC3s are mainly found in the intestinal mucosa. Although they can inhibit inflammation, protect intestinal barrier function, and maintain intestinal homeostasis (68, 69), they might also drive proinflammatory responses and cause immunopathological damage under conditions of local biological disorders (70). Of note, administration of L. reuteri ATCC PTA 6475 was reported to reduce the number of ILC3s (Figure 2C), thus reducing tissue damage; however, the specific molecular mechanism remains unexplored (71). Another study found that L. reuteri CF48-3A promoted the production of ILC3s in neonatal mice, and induced the differentiation of IgA+ B–cells, eventually leading to the generation of IgA (72). However, whether the IgA of neonatal mice play a protective role against infection and enhances mucosal immunity or induces autoimmune effects remains unclear (Figure 2C).
Administration of L. reuteri led to an increased production of nitric oxide (NO) by activated and increased numbers of macrophages, enhancing their phagocytotic ability for pathogenic bacteria. Meanwhile, L. reuteri inhibited the inflammation induced by bacterial infection by regulating the levels of NO (73) (Figure 2D). However, L. reuteri reduced the GBS-induced proliferation of NEs (74) (Figure 2D).
Toll-like receptor 2 (TLR2) recognizes the cell wall of gram-positive bacteria (75). L. reuteri 17938 is a gram-positive bacterium that following recognition by TLR2 mediates anti-inflammatory immune response in the following ways: First, it was demonstrated to lead to an increase in the number of Foxp3+ Treg cells and a decrease in that of CD4+CD44+ effector T-cells (Teffs) (49) (Figure 2E). The expression of CD44 on CD4+ T-cells is known to promote the occurrence of type 1 helper (Th1) responses and the generation of inflammatory cytokines (76). Accordingly, the activation and chemotaxis of lymphocytes to inflammatory sites was also found to be closely related to its upregulated expression (77, 78). Second, it induced the generation and activation of CD103+ DCs, which were characterized by the expression of co-stimulative markers CD80 and CD86 (49) that regulate the activity of T-cells (79). Of note, this DC-induced production of Foxp3+ Treg cells is crucial for maintaining intestinal immune tolerance (62) (Figure 2E). Finally, activation of TLR2 was shown to mediate the decrease in the levels of proinflammatory cytokines IL-1β and interferon-γ (IFN-γ) in mice (49) (Figure 2E). Notably, although L. reuteri 17938 has been confirmed to regulate immunity through TLR2-mediated pathways in animal models (49), oral administration of L. reuteri had no effects on the in vivo levels of TLR2 in infants with colic (52). Hence, there is still a long way to go before the mechanism of the interaction between L. reuturi and TLR2 can be transformed from the laboratory to the clinic.
Several studies have demonstrated that L. reuteri inhibited the production of proinflammatory cytokines, such as tumor necrosis factor-α (TNF-α), IL-1β, IL-6, and IL-8 (42, 43, 48, 59, 71), and that of CXCL10, CXCL1, and CCL2 chemokines, which recruit inflammatory cells (80), while it promoted the production of the anti-inflammatory cytokine IL-10 (53, 59), thus stabilizing the expression of TJs and E-cadherin and reinforcing the intestinal barrier function. Likewise, L. reuteri LFCA inhibited the expression of nuclear factor kappa-B (NF-κB)-mediated p65 and phosphorylation of inhibitor of NF-κB(IκB)α(IκBα) and nuclear transfer of p65, thereby inhibiting the transcriptional activation of inflammatory genes, as well as suppressing the production of proinflammatory factors, such as TNF-α, IL-6, and IL-1β (46) (Figure 2G). A significant decrease in the expression of LPS-induced TNF-α and IL-6 was observed in IPEC-J2 porcine IECs treated with L. reuteri I5007. However, this inhibition was dependent on TLR4 and related to LPS and time (38) (Figure 2G). Recent studies have shown that L. reuteri regulates the levels of TNF-α in a strain-dependent manner. For instance, L. reuteri ATCC PTA 6475 inhibited the activation of human monocyte MAPK, which in turn inhibited the activation of transcription factor c-Jun and AP-1, ultimately restraining the production of TNF-α (81) (Figure 2G). Similarly, L. reuteri BM36301 showed a gender preference in regulating the levels of TNF-α, as it significantly reduced the expression of TNF-α in female mice compared with that in male mice (82) (Figure 2G). Moreover, L.reuteri 5289 inhibited the generation of DCs and associated production of IL-12, whereas induced the expression of IL-10, which is associated with prolonged phosphorylation of extracellular regulated protein kinases1/2 (ERK1/2) in the MAPK pathway (83) (Figure 2G). A decrease in the levels of TGF-β in breast milk was observed after mothers in late pregnancy were provided a diet supplemented with L.reuteri. Notably, this might have been related to the decrease in the prevalence of allergy and IgE-related eczema in newborns in the first 2 years of their life (84) (Figure 2G). An engineered L. reuteri strain induced the production of IL-22 in the intestinal tract, hence mediating the expression of lectin regenerating islet-derived 3 gamma (REG3G), which, in turn, inhibited bacterial translocation, playing a protective role in liver (85) (Figure 2G). Notably, gene editing of L. reuteri strains produced better immune protection, providing novel ideas for the application of L. reuteri. Finally, L. reuteri has also been reported to regulate the expression of chemokines. For instance, CCL2 is known to be involved in pathological damages induced by stressor-enhanced infective colitis. Administration of L. reutri inhibited the expression of CCL2, thus reducing the infiltration of monocytes/macrophages in colon and secretion of inflammation-related factors (86).
Administration of L reuteri CF48-3A—derived from human milk—to pregnant C57BL/6(B6) mice induced the synthesis of IgA in mice offspring during their early life through vertical transmission from the vagina and milk. The mechanism by which this intestinal symbiotic microorganisms of maternal origin increased the synthesis of IgA in pups remains unclear; however, researchers found that both T-cells and RORγt+ ILC3s were dispensable in newborn mice (72). Of note, this maternal microbe-promoted synthesized IgA did not provide protection against intestinal infection by common pathogens such as Salmonella typhimurium (S. typhimurium) or enterohemorrhagic E.coli (72), and its function in pups remains unknown. Another study found that administration of L. reuteri FN041 increased the synthesis of secreted IgA (sIgA) in the ileum of mice (Figure 2F), especially in female mice (87). Vaccinating newborn mice with L. reuteri CRL1324 prior to GBS infection not only stimulated the production of IgA, but also that of IgG, which is related to anti-infection functions (74) (Figure 2F). Changes in microbial structure caused by L. reuteri have also been associated with the production of Abs. For example, an increase in the numbers of lactobacilli and enterococci caused by administration of mixed strains of L. reuteri were correlated with increased levels of IgE (88) (Figure 2F). The function of the Abs that promoted by L. reuteri is uncertain. Further studies are needed to determine whether it functions as a protective or an autoimmune factor. Sialylation of IgE is a key pathogenic link in allergies (89). Removal of sialic acid from IgE or de-sialic acid glycoprotein reduced allergic reactions (90). Whether L. reuteri can regulate glycosylation of IgE to improve allergy is the next research direction.
Atopic dermatitis (AD) is associated with an impaired skin barrier, skin microbial dysbiosis, and immune dysfunction (91–96). Skin microorganisms have an important role in the pathogenesis of AD, with increased colonization by Staphylococcus aureus (S.aureus) triggering inflammation or worsening AD (91, 97–100). The pathogenesis of AD has not only been related to skin microorganisms, but also to gut microbiota. Notably, the gut microbiome of infants with AD and their mothers differed markedly from that of healthy mothers and infants in the abundance of specific taxa (101). Accordingly, administration of oral probiotics in infants and children over 1 year old of age exhibited clear efficacy in reducing the incidence of AD and improving AD symptoms (102). Similarly, oral administration of probiotics in young patients with moderate AD significantly decreased the Scoring Atopic Dermatitis Index (SCORAD) values, an index assessing the severity of AD, and led to a reduction in the use of topical glucocorticoids (GCs) (103). Animal studies have shown that administration of probiotics to AD mice resulted in a decrease in the levels of the intestinal inflammatory marker fecal calprotectin (FC), reduced the levels of inflammatory factors, and ameliorated the incidence of skin lesions (104). These findings were also confirmed in clinical studies. After administration of a probiotic mixture containing Lactobacillus and Bifidobacterium for 4 weeks in probiotics group, both the SCORAD index and FC levels were decreased, accompanied by a decrease in the number of microbial species (105). Although a causal relationship between alterations in the composition of the gut microbiome and AD symptoms is not clear, the modulation of gut microbiota by oral or topical skin probiotics was shown to ameliorate AD, thus providing new ideas for probiotic intervention strategies against AD in the future.
The role of L. reuteri in the treatment of AD has only been recently noted. In an animal experiment, L. reuteri Fn041 derived from breast milk was found to ameliorate symptoms, such as skin swelling and inflammatory cell infiltration, in AD mice by modulating the ratio of Th1 and Th2 secreted cytokines, promoting the generation of Treg cells, and modulating the intestinal flora to increase the abundance of Lactobacillus and Akkermansia (50) (Table 1). Another study found that its anti-inflammatory effect was achieved through the activation of retinol metabolism and PPAR signaling pathways in Peyer’s patches. Notably, they argued that administration of late gestational dams with L. reuteri Fn041 and continued supplementation until weaning had better effect than that of supplementation after weaning (Table 1). This result emphasized the role of L. reuteri transmitted vertically by the mother in shaping the intestinal microbiota and strengthening the intestinal barrier of the offspring (107). AD is well-known to be an inflammation-induced disorder mainly mediated by Th2 cells (121, 122). Thymus stromal lymphopoietin (TSLP) is an important cytokine that mediates Th2 inflammatory responses (123, 124). L. reuteri DYNDL22M62 inhibited the production of TSLP and caused a decrease in the levels of Th2 cytokines (108), thus hindering the occurrence and progression of inflammation in AD. These studies showed that L. reuteri DYNDL22M62 metabolized Try into indoles, resulting in an increase in the proportion of intestinal flora that produce indole LAC and increasing the expression of AHR (108), thereby inhibiting intestinal inflammation and regulating immunity (125, 126). Furthermore, L. reuteri DYNDL22M62 reduced the production of IgE and improved AD symptoms (108) (Table 1). Oral administration of L. reuteri ATCC 55730 to children with AD for 8 weeks reduced the production of IL-4 and regulated the levels of cytokines in intestinal and extraintestinal tissues (106) (Table 1). However, the study did not clarify the correlation between the changes in the levels of related cytokines caused by L. reuteri ATCC 55730 and the improvement in AD symptoms. Administration of an oral probiotic mixture significantly reduced SCORAD index and steroid use in children with moderate AD (103). Besides, studies have found that continuous administration of an oral probiotic mixture in pregnant and lactating mothers and infants significantly reduced the incidence of AD in children (127). These findings suggest that the use of L. reuteri mixed with other strains might greater benefit patients with AD. However, currently, no study has compared the efficacy of mixed probiotics containing L. reuteri to that of single probiotics in the prevention and treatment of AD. The current view is that L. reuteri can improve AD, but only few randomized controlled trials (RCTs) have been performed in animals and the clinic, to date. In the future, large sample RCTs should be conducted on the basis of selecting appropriate strains. As the onset of AD is also correlated to imbalances in skin microecology (91, 128), regulating skin microecology might also be a way to prevent and treat AD. However, to date, no study has explored the application of L. reuteri as a human skin probiotic. In the future, the use of emollient cream or slow-release agent containing single or mixed probiotics might be a potential way to optimize the application of probiotics for the prevention and treatment of AD.
Asthma is a chronic airway inflammatory disease (129). Both Th2 cytokine-mediated inflammation and increased levels of IgE are important links in the pathogenesis of asthma (130, 131). Notably, seasonal exacerbation of asthma has been reported to be synchronous in time with changes in the composition of respiratory tract microbiota (132). Further, Streptococcus, Haemophilus, and Moraxella are believed to cause the deterioration of airway inflammation (133–135). Inhaled GCs are commonly used for the treatment of asthma. The application of these GCs leads to changes in the composition of airway microbiota, which have been correlated with the number of eosinophils and neutrophils in the sputum (136). In recent years, the discovery of the gut-lung axis also provides a possibility to explain the relationship between intestinal flora and lung health and disease. Accordingly, a possible mechanism is an intestinal ecological imbalance. Microorganisms and their metabolites might be transferred from the damaged intestinal barrier to the lung through blood or lymph circulation, causing lung inflammation and asthma-related symptoms (137–140). Additionally, intestinal microflora is crucial in the immune development of infants (141). Of note, retardation in intestinal microbial growth caused immune maturation disorder, affecting the immune responses of the lung, causing intolerance or overreaction to allergens (142). The lack of microbiota in the first year of life of infants born to asthmatic mothers confirmed this finding, as indicated by the increased risk of asthma in these children at the age of 5 (142).
As a dietary supplement, probiotics reshape the composition of intestinal microorganisms and might thus become potential candidates for early intervention in patients with asthma. Probiotics have been reported to not only have a positive impact on the health of newborns, but also on tackling allergic diseases of children over 14 years old (143). Although the conditions under which probiotics play their ideal role remain unclear, their administration has been shown to result in improvement of asthma-related parameters and symptoms in patients participating in clinical trials. For example, probiotics improved the anti-inflammation lipid depletion in the placebo group (141), significantly reduced the alveolar NO concentration of patients (P = 0.038), greatly relieved their symptoms (P = 0.001), and improved their score in the asthma control test (ACT) (P = 0.023). In addition, they increased the diversity of microorganisms, gene expression in potentially beneficial bacteria, such as Bifidobacteria, and the production of microbial active metabolites (144). Animal studies showed that probiotics reduced the levels of Th2-secreted cytokines IL-4, IL-5, IL-13, whereas increased those of the Th1-secreted cytokine, IFN-γ. They also inhibited the aggregation and inflow of eosinophils in the lung, and reduced the serum levels of IgE and airway hyper-responsiveness (145). Apart from this, they inhibited the deposition of lung collagen and proliferation of goblet cells, and reduced airway remodeling (146). The role of probiotics in regulating the levels of serum cytokines, lowering of the levels of IgE, and reducing eosinophil infiltration in patients with asthma was also confirmed in another clinical study (147). In this study, although probiotics had a positive effect on asthma-related immune biomarkers, the clinical symptoms of asthma were not improved (147). The inconsistent effect of probiotics on asthma-related parameters and clinical symptoms could be relevant to the dosage of probiotics. Hence, designing experiments where different dosages will be tested are necessary to clarify whether a correlation exists between dosage and effect. Thus, at present, probiotics should be carefully used in the prevention and treatment of asthma, especially in infants, considering all safety precautions. A large number of samples and the selection of appropriate strains and combinations are needed to further clarify whether probiotics are efficacious in the prevention and treatment of asthma and whether they can replace asthma drugs.
Owing to their great variety, some probiotics might become harmful under certain conditions. Thus, selecting the appropriate probiotic strains for targeting a specific disease is crucial. Some studies have compared the effects of L. reuteri, L. rhamnosus, and other strains on mice with asthma induced by the house dust mite (HDM), and observed that L. reuteri exhibited the best effect. This confirmed the core position of L. reuteri in regulating intestinal flora to affect lung immunity. Briefly, L. reuteri improved the airway inflammation index and decreased the total serum levels of IgE, HDM-IgG1, and Th2 cytokines, suppressing airway inflammation. Apart from this, it increased the abundance of Lactobacillus and Enterococci in the intestine and affected the production of total IgE and IL-13. Moreover, it increased the production of butyric acid, a microbial metabolite, by reshaping the intestinal microbial structure (88) (Table 1). The findings of this study were consistent with those in which oral L. reuteri inhibited eosinophil infiltration in the respiratory tract of mice, reducing the levels of cytokines, such as TNF, monocyte chemoattractant protein-1 (MCP-1), IL-5, and IL-13 in the lung, as well as reducing airway hyper-responsiveness (109) (Table 1). A clinical study of 30 patients aged 6–17 years—where patients were divided into 2 groups, those that were administered beclomethasone only, and those that were administered beclomethasone and L. reuteri DSM 17938 108 colony forming units/day (CFU/d)—revealed that the asthma-related symptoms in the probiotics group were improved and the score of ACT and peak expiratory flow (PEF) were increased after 2 months (110) (Table 1). Similarly, L. reuteri CCFM1040 also improved ACT and led to an improved control of asthma symptoms (112) (Table 1). In contrast, a clinical study found that the use of L. reuteri DSM 17938 109 CFU/d in adults with an average age of 27 years did not improve respiratory inflammation and symptoms, skin allergy, or T-cell responses (111) (Table 1). The discrepant effects of L. reuteri DSM 17938 in these two clinical trials might have been affected by age and dosage. Of note, the form of L. reuteri also affected its effect on asthma. For example, live L. reuteri exhibited an anti-inflammatory role and reduced airway hyper-responsiveness, whereas inactivated L. reuteri did not have this effect (109). In general, the administration of L. reuteri has shown beneficial effects on relieving asthma symptoms, and thus might become a potential candidate for the adjuvant treatment of asthma. Identification of the ideal strain, dosage, duration, and specific mechanism of action of L. reuteri in the prevention and treatment of asthma is warranted in the future.
Necrotizing enterocolitis (NEC) is a fatal acute inflammatory disease of the intestine that tends to occur in newborns, especially preterm infants (148), with a high incidence rate (149, 150). The risk factors of NEC include premature delivery, no breastfeeding (151), increased antibiotic exposure (152), and intestinal microbial ecological imbalance (153). Notably, 16S rRNA gene sequencing analysis found that the abundance of Proteus was increased in infants with NEC, whereas that of Bacteroides and Firmicutes was decreased (154). Some studies also found that the increase in the numbers of Clostridium (155, 156) or Klebsiella (157, 158) occurred before the diagnosis of NEC in premature infants. Changes in the abundance of Lactobacillus, Staphylococcus (159), and Streptococcus (160) have also been implicated in the occurrence and development of NEC. Although, these changes in the composition of intestinal microorganisms among children with NEC exhibit individual differences (159), their common feature is the low diversity of microorganisms (156, 159). Therefore, identifying specific microorganisms as biomarkers for the early diagnosis of NEC and applying prompt therapeutic approaches to reduce the incidence and severity of NEC, is imperative. Compared with the control group, sterile mice receiving fecal flora transplantation from patients with NEC showed more severe intestinal pathological damage (154). Of note, in another study, premature and cesarean piglets that received fecal filtrate transplantation from healthy piglets did not develop NEC (161). These evidence revealed that using intestinal microbiota as a therapeutic modality is of great significance in the prevention and treatment of NEC, and highlighted the potential use of probiotics as the next generation of promising therapies for NEC.
There is evidence to support that administration of single strains or combinations of multiple strains of Lactobacillus or Bifidobacterium has more advantages than that of other strains or combinations in reducing the incidence rate of NEC in preterm infants, by improving severity and reducing mortality (162, 163). However, it should be noted that the pathogenic or protective effect of probiotics on NEC is strain-dependent. Different strains of the same species might have distinct effects on NEC. For example, Enterococcus faecalis (E. faecalis) 224 improved the intestinal pathological damage of NEC rats, whereas BB70 and BB24 worsened NEC (164). Of note, the use of probiotics not only inhibited the growth of pathogenic and opportunistic pathogens (165), but also reduced the feeding intolerance of premature infants, which is of great significance to the nutrition and subsequent growth and development of the nervous system (163). In general, oral probiotics prevented the occurrence of NEC and improved the condition especially in terms of prevention in animal models and clinical trials, albeit not without certain risks. Although probiotics promote the health of the body, premature infants might be sensitive to them as their immune system is not yet mature. Further research is needed to evaluate the short-term and long-term effects and potential safety risks of the administration of probiotics to premature infants.
As a member of Lactobacillus, L. reuteri was studied for its prophylactic and therapeutic effect in NEC. Notably, TLR4, TLR2, and NF-κB are highly expressed in the intestine of experimental NEC animal models, mediating intestinal injury, as confirmed in many experiments (151, 166–168). Both L. reuteri DSM 17938 and ATCC PTA 4659 downregulated the expression of TLR4 and NF-κB and that of the pro-inflammatory cytokines IL-6, TNF-α, and IL-1 β, whereas upregulated the expression of the anti-inflammatory cytokine IL-10, inhibiting the occurrence and severity of NEC in neonatal rats. Among them, L. reuteri DSM 17938 was found to be more effective (114) (Table 1). Mechanistically, the improvement of experimental NEC by L. reuteri DSM 17938 involved the regulation of immune cells. In particular, L. reuteri DSM 17938 reduced the numbers of CD4+ effector T-cells, whereas increased those of Foxp3+ Tregs (49, 51) and tolerogenic dendritic cells (tolDC) in intestinal mucosa. These phenomena were observed in wild-type (WT) mice, but not in TLR2-/- mice, suggesting that the anti-inflammatory protective effect of L. reuteri DSM 17938 might be related to the TLR2 signaling pathway (49) (Table 1). Moreover, L. reuteri DSM 17938 reduced intestinal inflammation by inhibiting the oxidative stress reaction and enhancing the intestinal antioxidant capacity of NEC mice (47) (Table 1). However, to date, there have only been a few clinical studies in humans. A retrospective cohort study analyzed the medical data of 311 extremely low-birth weight infants (ELBWI) with birth weight ≤ 1000 g, 232 of whom did not use probiotics, and 79 of whom that received 0.1 mL (108 CFU/0.18 mL) of probiotics containing L. reuteri every day after birth. The incidence of NEC was decreased from 15.1% (35/232) to 2.5% (2/79) (169) (Table 1). In another RCT, L. reuteri DSM 17938 was administered to 400 very low-birth weight infants (VLBWI) (≤ 1500 g) at 108 CFU/d. Although no differences were detected in the incidence and mortality of NEC between the intervention and placebo groups, probiotics significantly reduced the incidence of delayed sepsis and feeding intolerance, and shortened the length of hospital stay (Table 1). However, this trial did not further randomize premature infants with weight ≤ 1000 g (113). The lower weight group was more likely to present positive results, which might be the reason for the differences observed in the experimental results. Current evidence has supported that the application of L. reuteri in animal models reduced the incidence rate of NEC, suggesting its potential protection against NEC in clinical trials. However, large sample, multicenter RCTs need to be conducted in the future to verify the preventive effect of L. reuteri on NEC pathogenesis in preterm infants. As the combination of Lactobacillus and Bifidobacterium is currently the recommended combination of probiotics for the prevention and treatment of NEC, the experimental combination of L. reuteri and Bifidobacterium can be tested in the future to verify its efficacy against NEC. Further understanding of the pathogenesis of NEC and exploration of whether L. reuteri can act on key targets involved in its pathogenesis is the direction of our efforts.
Systemic lupus erythematosus (SLE) is a complex autoimmune disease. Recent studies have observed changes in the composition of the intestinal microbiome and its metabolic functions in SLE (170–174). Sequencing analysis of intestinal microorganisms in patients with SLE showed low diversity and unbalanced distribution of species ratio. The metagenome-wide association study (MWAS) and the genome-wide association study (GWAS) showed that the abundance of Streptococcus intermedius and Streptococcus anginosus increased in the gut of SLE patients (175). The abundance of certain species increased in untreated SLE patients, including the Clostridium species ATCC BAA-442 as well as Atopobium rimae, Shuttleworthia satelles, Actinomyces massiliensis, Bacteroides fragilis, and Clostridium leptum. After treatment, these microorganisms decreased. The proinflammatory ability of some microbial peptide structures of SLE-enriched species was also confirmed by functional validation assays (176). An animal trial in 2020 found that the transfer of the gut microbiota of triplecongenic (TC) lupus-prone mice to germfree congenic C57BL/6(B6) mice reduced autoimmune severity. Metabolomics results showed that the distribution of tryptophan metabolites in feces of TC mice changed. Further study showed that high dietary tryptophan could aggravate the pathology of TC mice and the reduction of dietary tryptophan changed the intestinal microbiota of TC mice and B6 mice, suggesting that tryptophan metabolism might lead to immune activation of SLE by changing the microbiota structure (176). Another animal study in 2022 found defective TCR signaling proliferation of segmented filamentous bacteria in the gut of B6SKG mice, driving Th17 cell differentiation and causing autoimmunity in SLE (177). The intestinal microecology of patients with SLE is out of balance, and characterized by decreased microbial diversity, which is particularly noticeable in active patients (178). Changes in the content of specific species, such as increased numbers of Streptococcus, Campylobacter, and Velocilla, promote the arrival of the active phase of lupus, whereas increased numbers of Bifidobacterium prolong the remission phase of SLE (179). Patients with SLE can be distinguished from those with rheumatoid arthritis (RA) and healthy people by analyzing the differences in the composition and metabolic functions of intestinal microorganisms. These criteria can also further distinguish the active from the remission period of SLE (179). Transplantation of the fecal flora of SLE mice or patients to sterile mice significantly increased the level of anti-dsDNA, stimulated the inflammatory immune response, and increased the expression of SLE susceptibility genes (171, 180). After receiving Ruminococcus(RG) transplantation from patients with lupus, C57BL/6 mice had increased levels of anti-RG antibodies and intestinal permeability, especially female mice (157).In addition, transplantation of fecal flora from SLE patients also affected the metabolism of histidine in sterile mice, resulting in increased production of histamine, which might also be involved in the pathogenesis of SLE (171). In conclusion, the administration of probiotics for the treatment of microbial ecological imbalance in patients with SLE might be a new treatment direction that can bring hope to patients with SLE.
The number of probiotics used in SLE-related experiments has been limited, with Lactobacillus being the most commonly used genus. Notably, SLE affects multiple systems, and is often associated with cardiovascular and renal diseases (181–183). In the case of SLE-related renal injury, the use of probiotics reduced the levels of serum anti-dsDNA in female mice of a hypertensive lupus model. In addition, the level of inflammation in renal tissues was decreased, mainly manifested by the decrease in the numbers of Th1 and Th17 cells and levels of proinflammatory cytokines. Moreover, long-term use of probiotics alleviated renal injury and improved renal function, by inhibiting the activity of NADPH oxidases (NOX), thereby reducing the level of oxidative stress (184). In the case of SLE-related cardiomyopathy, administration of probiotics inhibited cardiac fibrosis and anticardiomyocyte apoptosis, while led to the significant thickening of the ventricular wall and the restoration of the structure of mildly changed cardiomyocytes to a certain extent. These findings were further confirmed by the decrease in the number of apoptotic cardiomyocytes (185). Regarding SLE-related vascular diseases, probiotics might play a role in preventing endothelial dysfunction. For example, the sensitivity of mouse aorta to acetylcholine, which depends on the levels of NO produced by endothelial cells, was increased after administration of probiotic (186). The excess of reactive oxygen species (ROS) is a pathogenic factor for cardiovascular diseases (187). Moreover, the excessive production of ROS is also known to be combined with increased levels of NO and inactivate vasodilator NO (188). Probiotics inhibited the activity of nitrogen oxides and reduced the production of ROS, thus maintaining the balance of ROS/NO and restoring the normal function of vascular endothelium (186). Based on their protective effect on endothelial dysfunction, probiotics might also have potential effects on preventing hypertension (186). Apart from their positive protective effect on SLE-related multisystem complications, combinations of probiotics and drugs were also reported to enhance the efficacy of drugs. A study found that probiotics combined with prednisolone pretreatment or -treatment reduced the serum levels of antinuclear antibody (ANA), anti-dsDNA antibody, and anti-RNP antibody in SLE mice (189). These autoantibodies are key pathogenic factors of SLE-related organ or tissue damage (190–193). In addition, a Th17/Treg imbalance is an important marker of inflammation progression during the pathogenesis of SLE (194–196). Hence, regulation of the Th17/Treg balance is of great significance for the protection from SLE-related complications (184, 197, 198). Tacrolimus (Tac) is an immunosuppressive agent commonly used in the treatment of SLE (199–201), and also known to inhibit Treg cells (202). A study found that compared with using Tac or probiotics alone, the combination of Tac and probiotics reduced the serum levels of autoimmune antibodies in MRL/lpr mice. In addition, both in vivo and in vitro experiments revealed an increase in the numbers of Treg cells and a decrease in those of Th17 cells. Based on this, the authors of the study suggested that probiotics could be used as the synergist of Tac for the regulation of the Th17/Treg balance in the SLE mice model (198). Various animal experiments have shown that probiotics might have anti-inflammatory and potential immunomodulatory effects in the prevention and treatment of SLE-related nephritis, cardiovascular diseases, and other complications. Additionally, the synergistic effect of probiotics combined with SLE therapeutic drugs was observed in a lupus mouse model. Therefore, the use of probiotics as an adjunct of drugs might become a promising therapeutic approach in clinical practice.
Research on the effect of L. reuteri in SLE has been limited, and showed contradictory results. Pretreatment with L. reuteri DSM 17509 prolonged the remission period of lupus, delayed its onset, increased the survival rate of NZB/WF1 mice, while it increased the production of IL-10 by DCs in lupus mice (60) (Table 1), indicating the preventive and therapeutic effects of L. reuteri in SLE. L. reuteri was also demonstrated to exert its protective effect on lupus-associated cardiovascular disease. Administration of heat-inactivated L. reuteri (h-L. reuteri) GMNL-263 prevented cardiac remodeling and inhibited the apoptosis of myocardial cells in NZB/WF1 mice, which were characterized by a decrease in the number of TUNEL-positive cells, related components of the Fas death receptor, and number of apoptotic cells in their heart tissues (116) (Table 1). Moreover, supplementation with L. reuteri GMNL-89 or L. reuteri GMNL-263 alleviated liver inflammation and inhibited hepatocyte apoptosis in NZB/WF1 mice, as indicated by the improvement in liver inflammation and apoptosis indicators. Lower levels of liver proinflammatory cytokines IL-1 β, IL-6, and TNF-α were linked to the inhibition of the MAPK and NF-κB signaling pathways by L. reuteri (117) (Table 1). However, Zegara-Ruiz et al. put forward an opposing opinion in 2019. They used 2 models, one of a spontaneous lupus TLR7.1 Tg C57BL/6 mouse model, and the other of an induced lupus model using the TLR agonist imiquimod (IMQ); both of them were TLR7-dependent lupus models (67). They found that the abundance of L. reuteri was increased in the intestines of these 2 model mice, while the intestinal mucosal barrier was damaged, allowing the translocation of L. reuteri outside of the intestine. They also found that in specific pathogen-free (SPF) and germ-free (GF) lupus mice, L. reuteri stimulated the autoimmune reaction, which led to the damage of intestinal barrier integrity, anemia, renal tissue pathological damage, and multisystem involvement. The key features in these processes were the accumulation of plasmacytoid dendritic cells (pDCs) and the increased levels of type I IFN (67, 115) (Table 1). First, comparing this with the previous studies, it is obvious that different animal models were used. Second, the pathogenic effect of L. reuteri in the latter study was under SPF or GF conditions, and thus is not comparable to the effect of L. reuteri in the real complex intestinal environment. Such genetic and environmental differences affect the host-microorganism crosstalk, which have a complex relationship from the onset of the disease. Finally, the authors did not clarify the strain of L. reuteri used in the last study. As mentioned, differences in treatment outcomes might be caused by the different characteristics of L. reuteri strains. In the future, large sample studies based on a unified SLE model need to be conducted to clarify the role of L. reuteri in the pathogenesis of SLE.
Immune dysfunction and tissue inflammation are important features of RA (203, 204). The pathogenesis of RA has been correlated to imbalances in the content of oral and intestinal microorganisms. Porphyromonas gingivalis (P. gingivalis) is the pathogenic bacteria causing periodontitis. The pathological and clinical symptoms of periodontitis and RA are similar to some extent, and the risk of RA in patients with periodontitis was found to be markedly increased (205). A significant decrease in microbial diversity was observed in the non-diseased areas of periodontitis in patients at high risk of RA (206). In addition, the oral microbial composition of RA high-risk patients and patients with early RA was found to be similar, with the proportion of Prevotella being significantly higher than that in healthy people (207, 208). These findings suggest that the change in the composition of the microbial community might have preceded the occurrence of RA. The involvement of intestinal microbiota in the occurrence and progression of RA has also been confirmed in an animal experiment. This study used broad-spectrum antibiotics to deplete the microorganisms of collagen-induced arthritis (CIA) model mice, and found that the severity of arthritis was significantly improved and the production of intestinal proinflammatory cytokines was delayed, whereas the serum levels of proinflammatory cytokines and anti-CII antibodies were significantly decreased (209). Notably, the activity of RA was also correlated to the microbial composition (210). Although changes in the composition of oral and intestinal microorganisms might be involved in the pathogenesis of RA, their causal relationship and whether these changes are related to the production of autoantibodies remain unclear. Nevertheless, the association between microbial ecological imbalance and disease severity observed in RA animals and clinical trials has provides us with a new direction for microbial interventions in RA.
Probiotics have the ability to reduce the level of inflammation and oxidative stress in patients with RA. In a recent study, 21 patients received a probiotics mixture every day for 2 consecutive months. The level of inflammation, white blood cell count, levels of proinflammatory cytokines TNF-α and IL-6, and levels of NOx were decreased, whereas the level of sulfhydryl (SH) and total radiotrapping antioxidant parameters (TRAP) were increased in the intervention group, indicating a decrease in the level of oxidative stress and an increase in the level of antioxidant parameters (211). This suggests that probiotics regulate the levels of inflammatory and oxidative stress indicators. Further studies should be conducted on the relationship between the probiotic-induced improvement of these indicators and the incidence rate, clinical symptoms, and prognosis of RA. A healthy diet might have a protective effect on RA (212, 213). Based on this, researchers combined probiotics with food ingredients with anti-inflammatory and antioxidant properties to explore their combined efficacy against RA. For example, a study investigated the effect of an anti-inflammatory diet containing probiotics and some food ingredients with anti-inflammatory effects in patients with RA and found that the 28 joints-Erythrocyte Seduction Rate (DAS28-ESR) and levels of 28 joints-C-reactive protein (DAS28-CRP), which reflect the activity of RA, were significantly reduced and the proportion of joint tenderness relief was higher than that of the control group (115). In another study, 50 patients with RA consumed an anti-inflammatory diet supplemented with probiotics. After 10 weeks, the levels of triglycerides (TG), high density lipoprotein cholesterol (HDL-C), apolipoprotein-B100/A1, fatty acids, and other blood lipid indicators of patients exhibited a trend towards the direction of improved prognosis (214). Based on these two studies, and despite the inability to determine whether the decline in RA activity and improvement of blood lipid status were the result of specific dietary ingredients or probiotics, it was concluded that the combination of probiotics and anti-inflammatory foods might have synergistic effects, providing potential reference significance for formulating a daily dietary strategy for patients with RA.
As L. reuteri regulates immune function through a variety of mechanisms, researchers have attempted to use it for the treatment of RA. In one study, where mice were administered h-L. reuteri MM2-3 before and after CIA induction, it was observed that h-L. reuteri pretreatment reduced the severity and incidence of CIA in mice. In addition, they also observed that the serum levels of type II collagen-specific immunoglobulin G (CII-IgG) and proinflammatory cytokines IL-6 and CXCL1 were decreased, whereas those of the anti-inflammatory cytokine IL-10 were increased. The improvement of CIA by h-L. reuteri pretreatment might be related to the reduction in the level of IL-6 and stimulation of production of CD103+ DCs, which, in turn, promote the production and peripheral migration of Tregs. However, it is noteworthy that although CIA did not occur in mice pretreated with h-L. reuteri, pretreatment had no effect on mice with developing CIA (53) (Table 1). Another study found that pretreatment with a probiotic mixture containing L. reuteri CCFM 8631 and L. reuteri CCFM 14 before the occurrence of CIA reduced the swelling and pathological damage in the joints of rats, suggesting its potential use for the prevention of CIA. In addition to the changes in the levels of proinflammatory cytokines and collagen-specific antibodies, this study also observed the levels of Th1-secreted cytokine IFN-γ and IL-12, which promotes Th1 cell differentiation, were decreased. In addition, the proportion of SCFA-producing microbial communities was increased. Notably, the authors found that the levels of the anti-inflammatory cytokine IL-10 were significantly decreased after treatment with L. reuteri, which was in contradiction with the changes in the levels of IL-10 observed in the former study. They hence assumed that IL-10 secreted by Tregs exerts anti-inflammatory and immunosuppressive effects, whereas IL-10 secreted by non-T-cells might aggravate the production of autoantibodies in patients with RA. In this study, the high levels of IL-10 in CIA rats were mainly produced by non-T-cells, with L. reuteri playing a protective role by regulating the number of non-T-cells and reducing the levels of destructive IL-10 (118) (Table 1). Recent research has suggested that L. reuteri can prevent CIA; however, the treatment effect on CIA remains unclear. LrS235, a genetically engineered L. reuteri secreting Kv1.3 potassium blocker ShK-235, significantly reduced clinical symptoms and joint inflammation in RA rats. This may be related to functional ShK-235 inhibiting T effector memory (TEM) cells (215). Although the functional substances produced by such bioengineering benefit the body, we should also pay attention to its immunogenicity. As RA is a chronic disease, the management of daily lifestyle and diet cannot be ignored. Based on recent developments in this field, adding L. reuteri to the daily diet might be a new strategy for the prevention of RA in the future. However, these findings need to be further explored and verified in the clinical setting.
Multiple sclerosis (MS) is an autoimmune disease characterized by central nervous system (CNS) demyelination, axonal injury, and neurodegeneration as the main pathological changes (216, 217). The participation of intestinal microbiota in the pathogenesis of MS has been preliminarily verified in an animal model of experimental autoimmune encephalopathy (EAE). The diversity of intestinal microflora, number of Lactobacillus, Bacteroides, and Prevotellaceae, and antioxidant level of intestinal microflora were increased in EAE mice fed an intermittent diet. Notably, transplantation of their fecal matter to EAE mice fed a normal diet resulted in the relief of symptoms (218). Some microorganisms that mediate the intensification or protection of EAE have also been observed in human intestines. For example, an increase in the abundance of Anaerotruncus colihomis was reported to improve EAE, and has been demonstrated to exert potential beneficial effects on patients with MS, such as reducing the incidence of disability (219, 220). The clinical classification of MS includes relapsing remitting (RR), secondary progressive (SP), primary progressive (PP), and progressive relapsing (PR) disease. However, these different phenotypes do not correlate with increasing imbalances in the composition of microorganisms (221, 222). For instance, compared with RRMS, the proportion of Enteroberiaceae and Clostridium in progressive MS was increased, whereas that of Blautia and Agathobaculum was decreased. Clostridium has been associated with higher disability and fatigue rates (220). The microflora of different types of MS have characteristic community characteristics, and can thus serve as biomarkers for determining the stage and severity of the disease (221). Clarifying the microbial composition of several types of MS might have great guiding significance for early clinical diagnosis and intervention. Specific microorganism might also serve as indicators of MS activity. Studies have shown that the presence of two of five microorganisms, namely Odoribacter and Butyricicoccus, has been significantly related to the risk of poor imaging performance of brain MRI (223). The pathogenesis of microbial-mediated MS might be related to the low level of butyrate and indolelactate (224), which is the intermediate product of indole propionate in Try metabolism (225). A decrease in the number of indolelactate-producing microbiota might lead to a decrease in the serum levels of indolelactate and indolepropionate (224). Indolepropionate is considered to be an antioxidant with significant neuroprotective effects (226). Accordingly, the inflammatory state in the body might be related to the decline in the serum levels of these metabolites (224). In general, we should focus not only on the microorganism itself that might serve as a biomarker and intervention target of MS pathogenicity, but also in its metabolites.
The outcomes of clinical trials on the use of probiotics in patients with MS have been positive. A study found that oral administration of 3600 billion CFU/d of a probiotics mixture containing 8 strains for 2 consecutive months not only reduced the number of intermediate monocytes (CD14highCD16low), but also decreased the expression of human leukocyte antigen DR (HLA-DR) in DCs of patients with RRMS. In addition, a reduction in the number of inflammatory monocytes (CD14low CD16high) and expression of costimulatory marker CD80 of classical monocytes was observed in the healthy control group. Conversely, after discontinuation of the use of probiotics, the number of inflammatory monocytes was increased, whereas that of Tregs was decreased (227). This showed that the probiotics mixture regulated the innate immune cells of patients with MS and healthy controls, and promoted anti-inflammatory reactions in vivo (227). Another study found that the serum levels of the proinflammatory cytokine IL-6 were decreased, whereas that of the brain-derived neurotrophic factor (BDNF) were significantly increased after the use of multiple probiotics (228). BDNF is a neuroregulatory protein that plays an important role in maintaining the survival and differentiation of neurons and can improve psychological symptoms, such as depression and anxiety (229). By using the mental health questionnaire to determine the depression score, fatigue degree, and pain assessment, the mental health of patients with MS was shown to be improved after the use of probiotics (228). Results from current clinical trials have indicated that probiotics might be used as potential candidates for the treatment of MS. In the future, more trials should be carried out on patients with different MS types on the basis of acquiring unified inclusion criteria to verify whether the use of probiotics can really benefit patients with MS.
Trp metabolites combined with AHR have been reported to exert immunoregulatory and anti-inflammatory effects and enhance gastrointestinal barrier function (225). Ampicillin (Amp)-sensitive vancomycin (Vanco)-resistant bacteria catalyze the binding of Trp to AHR ligands (34). L. reuteri is an Amp-sensitive Vanco-resistant bacterium, which metabolizes Trp to AHR agonists, acts on AHR of astrocytes, reduces inflammation of the central nervous system, and plays a protective role on EAE (119) (Table 1). Further supporting evidence have suggested that L. reuteri DSM 17938 improved the immune response of EAE by regulating the relative abundance of “beneficial” bacteria, such as Bifidobacterium, Prevotella, and Lactobacillus and that of harmful bacteria, such as Anaeroplasma, Rikenellaceae, and Clostridium, and restoring the low diversity of intestinal microorganisms. In another study, the numbers of Th1 and Th17 cells and levels of their cytokines were decreased in EAE mice (120) (Table 1). With respect to the role of L. reuteri in EAE, Miyauchi et al. reached a diametrically opposite conclusion in their subsequent study. They assumed that L. reuteri as a host symbiotic bacteria would aggravate the disease of genetically susceptible EAE mice and change their systemic metabolism. They accordingly found that L. reuteri and Erysipelaceae bacteria co-colonized and synergistically induced pathogenicity in EAE mice. This might be due to the similarity of L. reuteri to the myelin oligodendrocyte glycoprotein (MOG), which activates the MOG-specific CD4+ T-cells in the small intestine, causing an attack by autoimmune T-cells and worsening of spinal inflammation (58) (Table 1). L. reuteri is considered to be a strain that can interact with the genetically susceptible host to aggravate the symptoms of EAE (230). However, this contradictory conclusion might be related to difference among L. reuteri strains. Concomitantly, we should also note that L. reuteri can exert a synergistic effect with other microorganisms, potentially either benefiting health or causing disease. Therefore, we cannot ignore the “assistant” role of L. reuteri. Further research on MS-related intestinal microorganisms might help to identify more relevant species. At present, L. reuteri has only been applied to an animal model of MS, and has not been tested in clinical trials. Whether it can improve EAE remains undetermined and requires further research in clinical trials. Hence, there is still a long way to go before it becomes a new candidate for the future prevention and treatment of MS.
With the development of multiomics, the integration of metagenomics, macrotranscriptomics, metabolomics, and immunological data provides great assistance to the study of the mechanism of microbe-host crosstalk. The ability of probiotics to adhere to and colonize the gut may underlie a variety of mechanisms. In the complex immune regulation mechanism, L. reuteri and its metabolites play a central role in protecting intestinal barrier function and regulating immune cells. Multiple results have shown that L. reuteri affects the number and differentiation of DCs, which in turn affects the differentiation of T cells and the proportion of its subsets, playing a role in the regulation of the host immune system. However, due to the influence of host genetics, epigenetics and environment, especially diet, Probiotics have differentiated immune regulation in individuals. These insights highlight the importance of studying the level of probiotic strains and differences in individual physiological characteristics. The analysis of the molecular structure of probiotics and host cell surface receptors will help us to further understand its mechanism of action, and more meaningful conclusions will be achieved in the future by unifying experimental models and standardized samples.
The results of some animal and clinical studies indicated the encouraging application prospect of L. reuteri. It is undeniable that the research on probiotics had obvious heterogeneity, including the animals or people included in the study, the choice of the control group, the type and combination of probiotics selected, the intervention dose, the duration of the intervention or experiment, the environment and diets. These are reasons for contradictory or ambiguous conclusions. In addition, the efficacy of probiotics were evaluated based on clinical data, which were collected and analyzed differently. For example, many reports had obvious subjectivity in the evaluation of emotional or social function. In the future, we need to adopt high-quality research methods and carry out research on the basis of unified evaluation criteria.
With the in-depth study of probiotics, more details of the application of L. reuteri have been derived. No study has explored its metabolism in vivo, which is closely related to its safety; The biofilm state of L. reuteri optimizes the traditional form of drug delivery, but also requires close monitoring and control to avoid the excessive proliferation of L. reuteri; At present, there are two kinds of understanding on the choice of single strain or multi-strain combination, one is that microbiota have a complex network of relationships, with interconnected components that interact with each other to jointly maintain the microecological balance. Therefore, the synergistic effect of multiple strains may be more conducive to the inflammatory response and the stability of the immune system. The other believes that a single strain exhibiting a definite effect was more effective. This is because mixed strains might get out of control due to the inconsistent reproduction speed of each strain, thus disturbing the balance and hindering the control of microecology.
Generally speaking, we need to study further the metabolic pathway of L. reuteri in human body, determine the precise efficacy of different strains, comprehensively consider the safety and efficacy of the strain, carefully select the best strain or its combination, adopt the appropriate dose at the right time and maintain it for enough time. This may provide guidance for the clinical application of L. reuteri in the future.
ZL and AC designed and wrote the manuscript. RY is responsible for the concept development, funding acquisition, review and revision. AX, XL and SJ searched the literature. All authors approved the manuscript for public. All authors contributed to the article and approved the submitted version.
This work was supported by the Jiangsu Provincial Department of Science and Technology (No. BE2022698), the Wuxi Municipal Science and Technology Bureau (No.Y20222003), the Wuxi Municipal Medical Innovation Team (No.CXTD2021013), the Wuxi Commission of Health and Family Planning (Nos. SW202201 and M202171) and the Wuxi Young and Middle-aged Medical Talents Project (No. BJ2020075).
The authors declare that the research was conducted in the absence of any commercial or financial relationships that could be construed as a potential conflict of interest.
All claims expressed in this article are solely those of the authors and do not necessarily represent those of their affiliated organizations, or those of the publisher, the editors and the reviewers. Any product that may be evaluated in this article, or claim that may be made by its manufacturer, is not guaranteed or endorsed by the publisher.
1. Kim SK, Guevarra RB, Kim YT, Kwon J, Kim H, Cho JH, et al. Role of probiotics in human gut microbiome-associated diseases. J Microbiol Biotechnol (2019) 29(9):1335–40. doi: 10.4014/jmb.1906.06064
2. Wieers G, Belkhir L, Enaud R, Leclercq S, Philippart de Foy JM, Dequenne I, et al. How probiotics affect the microbiota. Front Cell Infect Microbiol (2019) 9:454. doi: 10.3389/fcimb.2019.00454
3. Jardou M, Brossier C, Guiyedi K, Faucher Q, Lawson R. Pharmacological hypothesis: A recombinant probiotic for taming bacterial beta-glucuronidase in drug-induced enteropathy. Pharmacol Res Perspect (2022) 10(5):e00998. doi: 10.1002/prp2.998
4. Pique N, Berlanga M, Minana-Galbis D. Health benefits of heat-killed (Tyndallized) probiotics: an overview. Int J Mol Sci (2019) 20(10):2534. doi: 10.3390/ijms20102534
5. Krawczyk RT, Banaszkiewicz A. Dr. Jozef Brudzinski - the true 'Father of probiotics'. Benef Microbes (2021) 12(3):211–3. doi: 10.3920/BM2020.0201
6. Gangaiah D, Mane SP, Tawari NR, Lakshmanan N, Ryan V, Volland A, et al. In silico, in vitro and in vivo safety evaluation of Limosilactobacillus reuteri strains ATCC PTA-126787 & ATCC PTA-126788 for potential probiotic applications. PloS One (2022) 17(1):e0262663. doi: 10.1371/journal.pone.0262663
7. Anjum J, Nazir S, Tariq M, Barrett K, Zaidi A. Lactobacillus commensals autochthonous to human milk have the hallmarks of potent probiotics. Microbiol (Reading). (2020) 166(10):966–80. doi: 10.1099/mic.0.000966
8. Bindu A, Lakshmidevi N. Identification and in vitro evaluation of probiotic attributes of lactic acid bacteria isolated from fermented food sources. Arch Microbiol (2021) 203(2):579–95. doi: 10.1007/s00203-020-02037-0
9. Jomehzadeh N, Javaherizadeh H, Amin M, Saki M, Al-Ouqaili MTS, Hamidi H, et al. Isolation and identification of potential probiotic Lactobacillus species from feces of infants in southwest Iran. Int J Infect Dis (2020) 96:524–30. doi: 10.1016/j.ijid.2020.05.034
10. Bull M, Plummer S, Marchesi J, Mahenthiralingam E. The life history of Lactobacillus acidophilus as a probiotic: a tale of revisionary taxonomy, misidentification and commercial success. FEMS Microbiol Lett (2013) 349(2):77–87. doi: 10.1111/1574-6968.12293
11. Kubota M, Ito K, Tomimoto K, Kanazaki M, Tsukiyama K, Kubota A, et al. Lactobacillus reuteri DSM 17938 and magnesium oxide in children with functional chronic constipation: A double-blind and randomized clinical trial. Nutrients (2020) 12(1):225. doi: 10.3390/nu12010225
12. Korpela K, Salonen A, Vepsalainen O, Suomalainen M, Kolmeder C, Varjosalo M, et al. Probiotic supplementation restores normal microbiota composition and function in antibiotic-treated and in caesarean-born infants. Microbiome (2018) 6(1):182. doi: 10.1186/s40168-018-0567-4
13. Slattery C, Cotter PD, O'Toole PW. Analysis of health benefits conferred by lactobacillus species from Kefir. Nutrients (2019) 11(6):1252. doi: 10.3390/nu11061252
14. Rao NS, Lundberg L, Palmkron S, Hakansson S, Bergenstahl B, Carlquist M. Flow cytometric analysis reveals culture condition dependent variations in phenotypic heterogeneity of Limosilactobacillus reuteri. Sci Rep (2021) 11(1):23567. doi: 10.1038/s41598-021-02919-3
15. Wegmann U, MacKenzie DA, Zheng J, Goesmann A, Roos S, Swarbreck D, et al. The pan-genome of Lactobacillus reuteri strains originating from the pig gastrointestinal tract. BMC Genomics (2015) 16:1023. doi: 10.1186/s12864-015-2216-7
16. Shokryazdan P, Faseleh Jahromi M, Liang JB, Ho YW. Probiotics: from isolation to application. J Am Coll Nutr (2017) 36(8):666–76. doi: 10.1080/07315724.2017.1337529
17. Espirito Santo C, Caseiro C, Martins MJ, Monteiro R, Brandao I. Gut microbiota, in the halfway between nutrition and lung function. Nutrients (2021) 13(5):1716. doi: 10.3390/nu13051716
18. Liu AH. Revisiting the hygiene hypothesis for allergy and asthma. J Allergy Clin Immunol (2015) 136(4):860–5. doi: 10.1016/j.jaci.2015.08.012
19. MacKenzie DA, Jeffers F, Parker ML, Vibert-Vallet A, Bongaerts RJ, Roos S, et al. Strain-specific diversity of mucus-binding proteins in the adhesion and aggregation properties of Lactobacillus reuteri. Microbiol (Reading) (2010) 156(Pt 11):3368–78. doi: 10.1099/mic.0.043265-0
20. Liu M, Hu R, Guo Y, Sun W, Li J, Fan M, et al. [Influence of Lactobacillus reuteri SL001 on intestinal microbiota in AD model mice and C57BL/6 mice]. Sheng Wu Gong Cheng Xue Bao. (2020) 36(9):1887–98. doi: 10.13345/j.cjb.200024
21. Garg S, Singh TP, Malik RK. In vivo implications of potential probiotic lactobacillus reuteri LR6 on the gut and immunological parameters as an adjuvant against protein energy malnutrition. Probiotics Antimicrob Proteins. (2020) 12(2):517–34. doi: 10.1007/s12602-019-09563-4
22. Yang J, Wang C, Liu L, Zhang M. Lactobacillus reuteri KT260178 supplementation reduced morbidity of piglets through its targeted colonization, improvement of cecal microbiota profile, and immune functions. Probiotics Antimicrob Proteins. (2020) 12(1):194–203. doi: 10.1007/s12602-019-9514-3
23. Liu Y, Tian X, He B, Hoang TK, Taylor CM, Blanchard E, et al. Lactobacillus reuteri DSM 17938 feeding of healthy newborn mice regulates immune responses while modulating gut microbiota and boosting beneficial metabolites. Am J Physiol Gastrointest Liver Physiol (2019) 317(6):G824–G38. doi: 10.1152/ajpgi.00107.2019
24. Hou C, Liu H, Zhang J, Zhang S, Yang F, Zeng X, et al. Intestinal microbiota succession and immunomodulatory consequences after introduction of Lactobacillus reuteri I5007 in neonatal piglets. PloS One (2015) 10(3):e0119505. doi: 10.1371/journal.pone.0119505
25. Wasfi R, Abd El-Rahman OA, Zafer MM, Ashour HM. Probiotic Lactobacillus sp. inhibit growth, biofilm formation and gene expression of caries-inducing Streptococcus mutans. J Cell Mol Med (2018) 22(3):1972–83. doi: 10.1111/jcmm.13496
26. Talarico TL, Dobrogosz WJ. Chemical characterization of an antimicrobial substance produced by Lactobacillus reuteri. Antimicrob Agents Chemother (1989) 33(5):674–9. doi: 10.1128/AAC.33.5.674
27. Talarico TL, Dobrogosz WJ. Purification and characterization of glycerol dehydratase from lactobacillus reuteri. Appl Environ Microbiol (1990) 56(4):1195–7. doi: 10.1128/aem.56.4.1195-1197.1990
28. Chen G, Chen J. A novel cell modification method used in biotransformation of glycerol to 3-HPA by Lactobacillus reuteri. Appl Microbiol Biotechnol (2013) 97(10):4325–32. doi: 10.1007/s00253-013-4723-2
29. Stevens JF, Maier CS. Acrolein: sources, metabolism, and biomolecular interactions relevant to human health and disease. Mol Nutr Food Res (2008) 52(1):7–25. doi: 10.1002/mnfr.200700412
30. Engels C, Schwab C, Zhang J, Stevens MJ, Bieri C, Ebert MO, et al. Acrolein contributes strongly to antimicrobial and heterocyclic amine transformation activities of reuterin. Sci Rep (2016) 6:36246. doi: 10.1038/srep36246
31. Wu H, Xie S, Miao J, Li Y, Wang Z, Wang M, et al. Lactobacillus reuteri maintains intestinal epithelial regeneration and repairs damaged intestinal mucosa. Gut Microbes (2020) 11(4):997–1014. doi: 10.1080/19490976.2020.1734423
32. Nation ML, Dunne EM, Joseph SJ, Mensah FK, Sung V, Satzke C, et al. Impact of Lactobacillus reuteri colonization on gut microbiota, inflammation, and crying time in infant colic. Sci Rep (2017) 7(1):15047. doi: 10.1038/s41598-017-15404-7
33. Husain S, Allotey J, Drymoussi Z, Wilks M, Fernandez-Felix BM, Whiley A, et al. Effects of oral probiotic supplements on vaginal microbiota during pregnancy: a randomised, double-blind, placebo-controlled trial with microbiome analysis. BJOG (2020) 127(2):275–84. doi: 10.1111/1471-0528.15675
34. Zelante T, Iannitti RG, Cunha C, De Luca A, Giovannini G, Pieraccini G, et al. Tryptophan catabolites from microbiota engage aryl hydrocarbon receptor and balance mucosal reactivity via interleukin-22. Immunity (2013) 39(2):372–85. doi: 10.1016/j.immuni.2013.08.003
35. Spits H, Artis D, Colonna M, Diefenbach A, Di Santo JP, Eberl G, et al. Innate lymphoid cells–a proposal for uniform nomenclature. Nat Rev Immunol (2013) 13(2):145–9. doi: 10.1038/nri3365
36. Cervantes-Barragan L, Chai JN, Tianero MD, Di Luccia B, Ahern PP, Merriman J, et al. Lactobacillus reuteri induces gut intraepithelial CD4(+)CD8alphaalpha(+) T cells. Science (2017) 357(6353):806–10. doi: 10.1126/science.aah5825
37. Yang KM, Kim JS, Kim HS, Kim YY, Oh JK, Jung HW, et al. Lactobacillus reuteri AN417 cell-free culture supernatant as a novel antibacterial agent targeting oral pathogenic bacteria. Sci Rep (2021) 11(1):1631. doi: 10.1038/s41598-020-80921-x
38. Yang F, Wang A, Zeng X, Hou C, Liu H, Qiao S. Lactobacillus reuteri I5007 modulates tight junction protein expression in IPEC-J2 cells with LPS stimulation and in newborn piglets under normal conditions. BMC Microbiol (2015) 15:32. doi: 10.1186/s12866-015-0372-1
39. Yuki T, Yoshida H, Akazawa Y, Komiya A, Sugiyama Y, Inoue S. Activation of TLR2 enhances tight junction barrier in epidermal keratinocytes. J Immunol (Baltimore Md 1950). (2011) 187(6):3230–7. doi: 10.4049/jimmunol.1100058
40. Martinez RC, Seney SL, Summers KL, Nomizo A, De Martinis EC, Reid G. Effect of Lactobacillus rhamnosus GR-1 and Lactobacillus reuteri RC-14 on the ability of Candida albicans to infect cells and induce inflammation. Microbiol Immunol (2009) 53(9):487–95. doi: 10.1111/j.1348-0421.2009.00154.x
41. Xie S, Zhao S, Jiang L, Lu L, Yang Q, Yu Q. Lactobacillus reuteri Stimulates Intestinal Epithelial Proliferation and Induces Differentiation into Goblet Cells in Young Chickens. J Agric Food Chem (2019) 67(49):13758–66. doi: 10.1021/acs.jafc.9b06256
42. Wang Q, Sun Q, Wang J, Qiu X, Qi R, Huang J. Identification of differentially expressed miRNAs after Lactobacillus reuteri treatment in the ileum mucosa of piglets. Genes Genomics (2020) 42(11):1327–38. doi: 10.1007/s13258-020-00998-6
43. Khmaladze I, Butler E, Fabre S, Gillbro JM. Lactobacillus reuteri DSM 17938-A comparative study on the effect of probiotics and lysates on human skin. Exp Dermatol (2019) 28(7):822–8. doi: 10.1111/exd.13950
44. Park ES, Freeborn J, Venna VR, Roos S, Rhoads JM, Liu Y. Lactobacillus reuteri effects on maternal separation stress in newborn mice. Pediatr Res (2021) 90(5):980–8. doi: 10.1038/s41390-021-01374-0
45. Gupta N, Ferreira J, Hong CHL, Tan KS. Lactobacillus reuteri DSM 17938 and ATCC PTA 5289 ameliorates chemotherapy-induced oral mucositis. Sci Rep (2020) 10(1):16189. doi: 10.1038/s41598-020-73292-w
46. Xie W, Song L, Wang X, Xu Y, Liu Z, Zhao D, et al. A bovine lactoferricin-lactoferrampin-encoding Lactobacillus reuteri CO21 regulates the intestinal mucosal immunity and enhances the protection of piglets against enterotoxigenic Escherichia coli K88 challenge. Gut Microbes (2021) 13(1):1956281. doi: 10.1080/19490976.2021.1956281
47. Tang J, Guo C, Gong F. [Protective effect of Lactobacillus reuteri against oxidative stress in neonatal mice with necrotizing enterocolitis]. Nan Fang Yi Ke Da Xue Xue Bao. (2019) 39(10):1221–6. doi: 10.12122/j.issn.1673-4254.2019.10.14
48. Karimi S, Jonsson H, Lundh T, Roos S. Lactobacillus reuteri strains protect epithelial barrier integrity of IPEC-J2 monolayers from the detrimental effect of enterotoxigenic Escherichia coli. Physiol Rep (2018) 6(2):e13514. doi: 10.14814/phy2.13514
49. Hoang TK, He B, Wang T, Tran DQ, Rhoads JM, Liu Y. Protective effect of Lactobacillus reuteri DSM 17938 against experimental necrotizing enterocolitis is mediated by Toll-like receptor 2. Am J Physiol Gastrointest Liver Physiol (2018) 315(2):G231–G40. doi: 10.1152/ajpgi.00084.2017
50. Zhao Y, Qi C, Li X, Lu M, Zhang H, Zhou J, et al. Prevention of atopic dermatitis in mice by lactobacillus reuteri Fn041 through induction of regulatory T cells and modulation of the gut microbiota. Mol Nutr Food Res (2022) 66(6):e2100699. doi: 10.1002/mnfr.202100699
51. Liu Y, Tran DQ, Fatheree NY, Marc Rhoads J. Lactobacillus reuteri DSM 17938 differentially modulates effector memory T cells and Foxp3+ regulatory T cells in a mouse model of necrotizing enterocolitis. Am J Physiol Gastrointest Liver Physiol (2014) 307(2):G177–86. doi: 10.1152/ajpgi.00038.2014
52. Savino F, Galliano I, Garro M, Savino A, Dapra V, Montanari P, et al. Regulatory T cells and Toll-like receptor 2 and 4 mRNA expression in infants with colic treated with Lactobacillus reuteri DSM17938. Benef Microbes (2018) 9(6):917–25. doi: 10.3920/BM2017.0194
53. Jia H, Ren S, Wang X. Heat-killed probiotic regulates the body's regulatory immunity to attenuate subsequent experimental autoimmune arthritis. Immunol Lett (2019) 216:89–96. doi: 10.1016/j.imlet.2019.10.009
54. Ahlberg E, Marti M, Govindaraj D, Severin E, Duchen K, Jenmalm MC, et al. Immune-related microRNAs in breast milk and their relation to regulatory T cells in breastfed children. Pediatr Allergy Immunol (2023) 34(4):e13952. doi: 10.1111/pai.13952
55. Forsberg A, HuOman J, Soderholm S, Bhai Mehta R, Nilsson L, Abrahamsson TR, et al. Pre- and postnatal Lactobacillus reuteri treatment alters DNA methylation of infant T helper cells. Pediatr Allergy Immunol (2020) 31(5):544–53. doi: 10.1111/pai.13240
56. Valeur N, Engel P, Carbajal N, Connolly E, Ladefoged K. Colonization and immunomodulation by Lactobacillus reuteri ATCC 55730 in the human gastrointestinal tract. Appl Environ Microbiol (2004) 70(2):1176–81. doi: 10.1128/AEM.70.2.1176-1181.2004
57. Ferreira RC, Forsyth LE, Richman PI, Wells C, Spencer J, MacDonald TT. Changes in the rate of crypt epithelial cell proliferation and mucosal morphology induced by a T-cell-mediated response in human small intestine. Gastroenterology (1990) 98(5 Pt 1):1255–63. doi: 10.1016/0016-5085(90)90342-X
58. Miyauchi E, Kim SW, Suda W, Kawasumi M, Onawa S, Taguchi-Atarashi N, et al. Gut microorganisms act together to exacerbate inflammation in spinal cords. Nature (2020) 585(7823):102–6. doi: 10.1038/s41586-020-2634-9
59. Engevik MA, Ruan W, Esparza M, Fultz R, Shi Z, Engevik KA, et al. Immunomodulation of dendritic cells by Lactobacillus reuteri surface components and metabolites. Physiol Rep (2021) 9(2):e14719. doi: 10.14814/phy2.14719
60. Manirarora JN, Kosiewicz MM, Alard P. Feeding lactobacilli impacts lupus progression in (NZBxNZW)F1 lupus-prone mice by enhancing immunoregulation. Autoimmunity (2020) 53(6):323–32. doi: 10.1080/08916934.2020.1777282
61. Liu HY, Gu F, Zhu C, Yuan L, Zhu C, Zhu M, et al. Epithelial heat shock proteins mediate the protective effects of limosilactobacillus reuteri in dextran sulfate sodium-induced colitis. Front Immunol (2022) 13:865982. doi: 10.3389/fimmu.2022.865982
62. Coombes JL, Siddiqui KR, Arancibia-Carcamo CV, Hall J, Sun CM, Belkaid Y, et al. A functionally specialized population of mucosal CD103+ DCs induces Foxp3+ regulatory T cells via a TGF-beta and retinoic acid-dependent mechanism. J Exp Med (2007) 204(8):1757–64. doi: 10.1084/jem.20070590
63. Eloranta ML, Ronnblom L. Cause and consequences of the activated type I interferon system in SLE. J Mol Med (Berl). (2016) 94(10):1103–10. doi: 10.1007/s00109-016-1421-4
64. Kim JM, Park SH, Kim HY, Kwok SK. A plasmacytoid dendritic cells-type I interferon axis is critically implicated in the pathogenesis of systemic lupus erythematosus. Int J Mol Sci (2015) 16(6):14158–70. doi: 10.3390/ijms160614158
65. Davison LM, Jorgensen TN. New treatments for systemic lupus erythematosus on the horizon: targeting plasmacytoid dendritic cells to inhibit cytokine production. J Clin Cell Immunol (2017) 8(6):534. doi: 10.4172/2155-9899.1000534
66. Crow MK. Type I interferon in the pathogenesis of lupus. J Immunol (Baltimore Md 1950). (2014) 192(12):5459–68. doi: 10.4049/jimmunol.1002795
67. Zegarra-Ruiz DF, El Beidaq A, Iniguez AJ, Lubrano Di Ricco M, Manfredo Vieira S, Ruff WE, et al. A diet-sensitive commensal lactobacillus strain mediates TLR7-dependent systemic autoimmunity. Cell Host Microbe (2019) 25(1):113–27 e6. doi: 10.1016/j.chom.2018.11.009
68. Jarade A, Di Santo JP, Serafini N. Group 3 innate lymphoid cells mediate host defense against attaching and effacing pathogens. Curr Opin Microbiol (2021) 63:83–91. doi: 10.1016/j.mib.2021.06.005
69. Wen Z, Chen D, Bian J. [Role of group 3 innate lymphoid cells in intestinal barrier]. Zhonghua Wei Zhong Bing Ji Jiu Yi Xue. (2019) 31(2):252–6. doi: 10.3760/cma.j.issn.2095-4352.2019.02.028
70. Song D, Lai L, Ran Z. Metabolic regulation of group 3 innate lymphoid cells and their role in inflammatory bowel disease. Front Immunol (2020) 11:580467. doi: 10.3389/fimmu.2020.580467
71. Wang T, Zheng N, Luo Q, Jiang L, He B, Yuan X, et al. Probiotics lactobacillus reuteri abrogates immune checkpoint blockade-associated colitis by inhibiting group 3 innate lymphoid cells. Front Immunol (2019) 10:1235. doi: 10.3389/fimmu.2019.01235
72. Mu Q, Swartwout BK, Edwards M, Zhu J, Lee G, Eden K, et al. Regulation of neonatal IgA production by the maternal microbiota. Proc Natl Acad Sci U.S.A. (2021) 118(9):e2015691118. doi: 10.1073/pnas.2015691118
73. Jiang P, Yang W, Jin Y, Huang H, Shi C, Jiang Y, et al. Lactobacillus reuteri protects mice against Salmonella typhimurium challenge by activating macrophages to produce nitric oxide. Microb Pathog (2019) 137:103754. doi: 10.1016/j.micpath.2019.103754
74. De Gregorio PR, Juarez Tomas MS, Nader-Macias ME. Immunomodulation of lactobacillus reuteri CRL1324 on group B streptococcus vaginal colonization in a murine experimental model. Am J Reprod Immunol (New York NY 1989). (2016) 75(1):23–35. doi: 10.1111/aji.12445
75. Cario E, Gerken G, Podolsky DK. Toll-like receptor 2 controls mucosal inflammation by regulating epithelial barrier function. Gastroenterology (2007) 132(4):1359–74. doi: 10.1053/j.gastro.2007.02.056
76. Collins CB, Ho J, Wilson TE, Wermers JD, Tlaxca JL, Lawrence MB, et al. CD44 deficiency attenuates chronic murine ileitis. Gastroenterology (2008) 135(6):1993–2002. doi: 10.1053/j.gastro.2008.08.053
77. Baaten BJ, Li CR, Bradley LM. Multifaceted regulation of T cells by CD44. Communicative Integr Biol (2010) 3(6):508–12. doi: 10.4161/cib.3.6.13495
78. Lee WT, Vitetta ES. The differential expression of homing and adhesion molecules on virgin and memory T cells in the mouse. Cell Immunol (1991) 132(1):215–22. doi: 10.1016/0008-8749(91)90020-C
79. Kornete M, Piccirillo CA. Functional crosstalk between dendritic cells and Foxp3(+) regulatory T cells in the maintenance of immune tolerance. Front Immunol (2012) 3:165. doi: 10.3389/fimmu.2012.00165
80. Gabryszewski SJ, Bachar O, Dyer KD, Percopo CM, Killoran KE, Domachowske JB, et al. Lactobacillus-mediated priming of the respiratory mucosa protects against lethal pneumovirus infection. J Immunol (Baltimore Md 1950). (2011) 186(2):1151–61. doi: 10.4049/jimmunol.1001751
81. Lin YP, Thibodeaux CH, Pena JA, Ferry GD, Versalovic J. Probiotic Lactobacillus reuteri suppress proinflammatory cytokines via c-Jun. Inflammatory bowel diseases. (2008) 14(8):1068–83. doi: 10.1002/ibd.20448
82. Lee J, Yang W, Hostetler A, Schultz N, Suckow MA, Stewart KL, et al. Characterization of the anti-inflammatory Lactobacillus reuteri BM36301 and its probiotic benefits on aged mice. BMC Microbiol (2016) 16:69. doi: 10.1186/s12866-016-0686-7
83. Amar Y, Rizzello V, Cavaliere R, Campana S, De Pasquale C, Barberi C, et al. Divergent signaling pathways regulate IL-12 production induced by different species of Lactobacilli in human dendritic cells. Immunol Lett (2015) 166(1):6–12. doi: 10.1016/j.imlet.2015.05.001
84. Bottcher MF, Abrahamsson TR, Fredriksson M, Jakobsson T, Bjorksten B. Low breast milk TGF-beta2 is induced by Lactobacillus reuteri supplementation and associates with reduced risk of sensitization during infancy. Pediatr Allergy Immunol (2008) 19(6):497–504. doi: 10.1111/j.1399-3038.2007.00687.x
85. Hendrikx T, Duan Y, Wang Y, Oh JH, Alexander LM, Huang W, et al. Bacteria engineered to produce IL-22 in intestine induce expression of REG3G to reduce ethanol-induced liver disease in mice. Gut (2019) 68(8):1504–15. doi: 10.1136/gutjnl-2018-317232
86. Mackos AR, Galley JD, Eubank TD, Easterling RS, Parry NM, Fox JG, et al. Social stress-enhanced severity of Citrobacter rodentium-induced colitis is CCL2-dependent and attenuated by probiotic Lactobacillus reuteri. Mucosal Immunol (2016) 9(2):515–26. doi: 10.1038/mi.2015.81
87. Qi C, Ding M, Li S, Zhou Q, Li D, Yu R, et al. Sex-dependent modulation of immune development in mice by secretory IgA-coated Lactobacillus reuteri isolated from breast milk. J Dairy Sci (2021) 104(4):3863–75. doi: 10.3168/jds.2020-19437
88. Li L, Fang Z, Liu X, Hu W, Lu W, Lee YK, et al. Lactobacillus reuteri attenuated allergic inflammation induced by HDM in the mouse and modulated gut microbes. PloS One (2020) 15(4):e0231865. doi: 10.1371/journal.pone.0231865
89. Gao G, Li C, Fan W, Zhang M, Li X, Chen W, et al. Brilliant glycans and glycosylation: Seq and ye shall find. Int J Biol macromolecules. (2021) 189:279–91. doi: 10.1016/j.ijbiomac.2021.08.054
90. Shade KC, Conroy ME, Washburn N, Kitaoka M, Huynh DJ, Laprise E, et al. Sialylation of immunoglobulin E is a determinant of allergic pathogenicity. Nature (2020) 582(7811):265–70. doi: 10.1038/s41586-020-2311-z
91. Fyhrquist N, Muirhead G, Prast-Nielsen S, Jeanmougin M, Olah P, Skoog T, et al. Microbe-host interplay in atopic dermatitis and psoriasis. Nat Commun (2019) 10(1):4703. doi: 10.1038/s41467-019-12253-y
92. Lipsky ZW, Patsy M, Marques CNH, German GK. Mechanisms and implications of bacterial invasion across the human skin barrier. Microbiol Spectr. (2022) 10(3):e0274421. doi: 10.1128/spectrum.02744-21
93. Mizutani Y, Takagi N, Nagata H, Inoue S. Interferon-gamma downregulates tight junction function, which is rescued by interleukin-17A. Exp Dermatol (2021) 30(12):1754–63. doi: 10.1111/exd.14425
94. Margolis DJ. Atopic dermatitis: filaggrin and skin barrier dysfunction. Br J Dermatol (2022) 186(3):396. doi: 10.1111/bjd.20946
95. Dainichi T, Kitoh A, Otsuka A, Nakajima S, Nomura T, Kaplan DH, et al. The epithelial immune microenvironment (EIME) in atopic dermatitis and psoriasis. Nat Immunol (2018) 19(12):1286–98. doi: 10.1038/s41590-018-0256-2
96. Akdis CA. Does the epithelial barrier hypothesis explain the increase in allergy, autoimmunity and other chronic conditions? Nat Rev Immunol (2021) 21(11):739–51. doi: 10.1038/s41577-021-00538-7
97. Masiuk H, Wcislek A, Jursa-Kulesza J. Determination of nasal carriage and skin colonization, antimicrobial susceptibility and genetic relatedness of Staphylococcus aureus isolated from patients with atopic dermatitis in Szczecin, Poland. BMC Infect Dis (2021) 21(1):701. doi: 10.1186/s12879-021-06382-3
98. Luu LA, Flowers RH, Gao Y, Wu M, Gasperino S, Kellams AL, et al. Apple cider vinegar soaks do not alter the skin bacterial microbiome in atopic dermatitis. PloS One (2021) 16(6):e0252272. doi: 10.1371/journal.pone.0252272
99. Bjerre RD, Holm JB, Palleja A, Solberg J, Skov L, Johansen JD. Skin dysbiosis in the microbiome in atopic dermatitis is site-specific and involves bacteria, fungus and virus. BMC Microbiol (2021) 21(1):256. doi: 10.1186/s12866-021-02302-2
100. Towell AM, Feuillie C, Vitry P, Da Costa TM, Mathelie-Guinlet M, Kezic S, et al. Staphylococcus aureus binds to the N-terminal region of corneodesmosin to adhere to the stratum corneum in atopic dermatitis. Proc Natl Acad Sci U.S.A. (2021) 118(1):e2014444118. doi: 10.1073/pnas.2014444118
101. Sung M, Choi Y, Park H, Huh CS. Gut microbiome characteristics in mothers and infants according to the presence of atopic dermatitis. BioMed Res Int (2022) 2022:8145462. doi: 10.1155/2022/8145462
102. Jiang W, Ni B, Liu Z, Liu X, Xie W, Wu IXY, et al. The role of probiotics in the prevention and treatment of atopic dermatitis in children: an updated systematic review and meta-analysis of randomized controlled trials. Paediatr Drugs (2020) 22(5):535–49. doi: 10.1007/s40272-020-00410-6
103. Navarro-Lopez V, Ramirez-Bosca A, Ramon-Vidal D, Ruzafa-Costas B, Genoves-Martinez S, Chenoll-Cuadros E, et al. Effect of oral administration of a mixture of probiotic strains on SCORAD index and use of topical steroids in young patients with moderate atopic dermatitis: A randomized clinical trial. JAMA Dermatol (2018) 154(1):37–43. doi: 10.1001/jamadermatol.2017.3647
104. Kim MJ, Kim JY, Kang M, Won MH, Hong SH, Her Y. Reduced fecal calprotectin and inflammation in a murine model of atopic dermatitis following probiotic treatment. Int J Mol Sci (2020) 21(11):3968. doi: 10.3390/ijms21113968
105. Yoon W, Park SH, Lee JS, Byeon JH, Kim SH, Lim J, et al. Probiotic mixture reduces gut inflammation and microbial dysbiosis in children with atopic dermatitis. Australas J Dermatol (2021) 62(3):e386–e92. doi: 10.1111/ajd.13644
106. Miniello VL, Brunetti L, Tesse R, Natile M, Armenio L, Francavilla R. Lactobacillus reuteri modulates cytokines production in exhaled breath condensate of children with atopic dermatitis. J Pediatr Gastroenterol Nutr (2010) 50(5):573–6. doi: 10.1097/MPG.0b013e3181bb343f
107. Zhou J, Xu G, Li X, Tu H, Li H, Chang H, et al. Limosilactobacillus reuteri FN041 prevents atopic dermatitis in pup mice by remodeling the ileal microbiota and regulating gene expression in Peyer's patches after vertical transmission. Front Nutr (2022) 9:987400. doi: 10.3389/fnut.2022.987400
108. Fang Z, Pan T, Wang H, Zhu J, Zhang H, Zhao J, et al. Limosilactobacillus reuteri Attenuates Atopic Dermatitis via Changes in Gut Bacteria and Indole Derivatives from Tryptophan Metabolism. Int J Mol Sci (2022) 23(14):7735. doi: 10.3390/ijms23147735
109. Forsythe P, Inman MD, Bienenstock J. Oral treatment with live Lactobacillus reuteri inhibits the allergic airway response in mice. Am J Respir Crit Care Med (2007) 175(6):561–9. doi: 10.1164/rccm.200606-821OC
110. Moura JCV, Moura ICG, Gaspar GR, Mendes GMS, Faria BAV, Jentzsch NS, et al. The use of probiotics as a supplementary therapy in the treatment of patients with asthma: a pilot study and implications. Clinics (Sao Paulo). (2019) 74:e950. doi: 10.6061/clinics/2019/e950
111. Satia I, Cusack R, Stevens C, Schlatman A, Wattie J, Mian F, et al. Limosilactobacillus reuteri DSM-17938 for preventing cough in adults with mild allergic asthma: A double-blind randomized placebo-controlled cross-over study. Clin Exp Allergy (2021) 51(9):1133–43. doi: 10.1111/cea.13976
112. Li L, Fang Z, Lee YK, Zhao J, Zhang H, Peng H, et al. Efficacy and safety of lactobacillus reuteri CCFM1040 in allergic rhinitis and asthma: A randomized, placebo-controlled trial. Front Nutr (2022) 9:862934. doi: 10.3389/fnut.2022.862934
113. Oncel MY, Sari FN, Arayici S, Guzoglu N, Erdeve O, Uras N, et al. Lactobacillus Reuteri for the prevention of necrotising enterocolitis in very low birthweight infants: a randomised controlled trial. Arch Dis Child Fetal Neonatal Ed (2014) 99(2):F110–5. doi: 10.1136/archdischild-2013-304745
114. Liu Y, Fatheree NY, Mangalat N, Rhoads JM. Lactobacillus reuteri strains reduce incidence and severity of experimental necrotizing enterocolitis via modulation of TLR4 and NF-kappaB signaling in the intestine. Am J Physiol Gastrointest Liver Physiol (2012) 302(6):G608–17. doi: 10.1152/ajpgi.00266.2011
115. Vadell AKE, Barebring L, Hulander E, Gjertsson I, Lindqvist HM, Winkvist A. Anti-inflammatory Diet In Rheumatoid Arthritis (ADIRA)-a randomized, controlled crossover trial indicating effects on disease activity. Am J Clin Nutr (2020) 111(6):1203–13. doi: 10.1093/ajcn/nqaa019
116. Yeh YL, Lu MC, Tsai BC, Tzang BS, Cheng SM, Zhang X, et al. Heat-killed lactobacillus reuteri GMNL-263 inhibits systemic lupus erythematosus-induced cardiomyopathy in NZB/W F1 mice. Probiotics Antimicrob Proteins. (2021) 13(1):51–9. doi: 10.1007/s12602-020-09668-1
117. Hsu TC, Huang CY, Liu CH, Hsu KC, Chen YH, Tzang BS. Lactobacillus paracasei GMNL-32, Lactobacillus reuteri GMNL-89 and L. reuteri GMNL-263 ameliorate hepatic injuries in lupus-prone mice. Br J Nutr (2017) 117(8):1066–74. doi: 10.1017/S0007114517001039
118. Fan Z, Yang B, Ross RP, Stanton C, Zhao J, Zhang H, et al. The prophylactic effects of different Lactobacilli on collagen-induced arthritis in rats. Food Funct (2020) 11(4):3681–94. doi: 10.1039/C9FO02556A
119. Rothhammer V, Mascanfroni ID, Bunse L, Takenaka MC, Kenison JE, Mayo L, et al. Type I interferons and microbial metabolites of tryptophan modulate astrocyte activity and central nervous system inflammation via the aryl hydrocarbon receptor. Nat Med (2016) 22(6):586–97. doi: 10.1038/nm.4106
120. He B, Hoang TK, Tian X, Taylor CM, Blanchard E, Luo M, et al. Lactobacillus reuteri reduces the severity of experimental autoimmune encephalomyelitis in mice by modulating gut microbiota. Front Immunol (2019) 10:385. doi: 10.3389/fimmu.2019.00385
121. Li H, Zhang Z, Zhang H, Guo Y, Yao Z. Update on the pathogenesis and therapy of atopic dermatitis. Clin Rev Allergy Immunol (2021) 61(3):324–38. doi: 10.1007/s12016-021-08880-3
122. Meng J, Li Y, Fischer MJM, Steinhoff M, Chen W, Wang J. Th2 modulation of transient receptor potential channels: an unmet therapeutic intervention for atopic dermatitis. Front Immunol (2021) 12:696784. doi: 10.3389/fimmu.2021.696784
123. Protti MP, De Monte L. Thymic stromal lymphopoietin and cancer: th2-dependent and -independent mechanisms. Front Immunol (2020) 11:2088. doi: 10.3389/fimmu.2020.02088
124. He R, Geha RS. Thymic stromal lymphopoietin. Ann N Y Acad Sci (2010) 1183:13–24. doi: 10.1111/j.1749-6632.2009.05128.x
125. Pernomian L, Duarte-Silva M, de Barros Cardoso CR. The aryl hydrocarbon receptor (AHR) as a potential target for the control of intestinal inflammation: insights from an immune and bacteria sensor receptor. Clin Rev Allergy Immunol (2020) 59(3):382–90. doi: 10.1007/s12016-020-08789-3
126. Alexeev EE, Lanis JM, Kao DJ, Campbell EL, Kelly CJ, Battista KD, et al. Microbiota-derived indole metabolites promote human and murine intestinal homeostasis through regulation of Interleukin-10 receptor. Am J Pathol (2018) 188(5):1183–94. doi: 10.1016/j.ajpath.2018.01.011
127. AMalia N, Orchard D, Francis KL, King E. Systematic review and meta-analysis on the use of probiotic supplementation in pregnant mother, breastfeeding mother and infant for the prevention of atopic dermatitis in children. Australas J Dermatol (2020) 61(2):e158–e73. doi: 10.1111/ajd.13186
128. Lunjani N, Hlela C, O'Mahony L. Microbiome and skin biology. Curr Opin Allergy Clin Immunol (2019) 19(4):328–33. doi: 10.1097/ACI.0000000000000542
129. Shi B, Li W, Dong H, Xu M, Hao Y, Gao P. Distribution of inflammatory phenotypes among patients with asthma in Jilin Province, China: a cross-sectional study. BMC Pulm Med (2021) 21(1):364. doi: 10.1186/s12890-021-01722-0
130. Lambrecht BN, Hammad H, Fahy JV. The cytokines of asthma. Immunity (2019) 50(4):975–91. doi: 10.1016/j.immuni.2019.03.018
131. Chen M, Shepard K 2nd, Yang M, Raut P, Pazwash H, Holweg CTJ, et al. Overlap of allergic, eosinophilic and type 2 inflammatory subtypes in moderate-to-severe asthma. Clin Exp Allergy (2021) 51(4):546–55. doi: 10.1111/cea.13790
132. McCauley KE, Flynn K, Calatroni A, DiMassa V, LaMere B, Fadrosh DW, et al. Seasonal airway microbiome and transcriptome interactions promote childhood asthma exacerbations. J Allergy Clin Immunol (2022) 150(1):204–13. doi: 10.1016/j.jaci.2022.01.020
133. Ahluwalia TS, Eliasen AU, Sevelsted A, Pedersen CT, Stokholm J, Chawes B, et al. FUT2-ABO epistasis increases the risk of early childhood asthma and Streptococcus pneumoniae respiratory illnesses. Nat Commun (2020) 11(1):6398. doi: 10.1038/s41467-020-19814-6
134. Diver S, Richardson M, Haldar K, Ghebre MA, Ramsheh MY, Bafadhel M, et al. Sputum microbiomic clustering in asthma and chronic obstructive pulmonary disease reveals a Haemophilus-predominant subgroup. Allergy (2020) 75(4):808–17. doi: 10.1111/all.14058
135. Zhou Y, Jackson D, Bacharier LB, Mauger D, Boushey H, Castro M, et al. The upper-airway microbiota and loss of asthma control among asthmatic children. Nat Commun (2019) 10(1):5714. doi: 10.1038/s41467-019-13698-x
136. Huang C, Ni Y, Du W, Shi G. Effect of inhaled corticosteroids on microbiome and microbial correlations in asthma over a 9-month period. Clin Transl Sci (2022) 15(7):1723–36. doi: 10.1111/cts.13288
137. Budden KF, Gellatly SL, Wood DL, Cooper MA, Morrison M, Hugenholtz P, et al. Emerging pathogenic links between microbiota and the gut-lung axis. Nat Rev Microbiol (2017) 15(1):55–63. doi: 10.1038/nrmicro.2016.142
138. Dumas A, Bernard L, Poquet Y, Lugo-Villarino G, Neyrolles O. The role of the lung microbiota and the gut-lung axis in respiratory infectious diseases. Cell Microbiol (2018) 20(12):e12966. doi: 10.1111/cmi.12966
139. Marsland BJ, Trompette A, Gollwitzer ES. The gut-lung axis in respiratory disease. Ann Am Thorac Soc (2015) 12 Suppl 2:S150–6. doi: 10.1513/AnnalsATS.201503-133AW
140. Frati F, Salvatori C, Incorvaia C, Bellucci A, Di Cara G, Marcucci F, et al. The role of the microbiome in asthma: the gut(-)Lung axis. Int J Mol Sci (2018) 20(1):123. doi: 10.3390/ijms20010123
141. Durack J, Kimes NE, Lin DL, Rauch M, McKean M, McCauley K, et al. Delayed gut microbiota development in high-risk for asthma infants is temporarily modifiable by Lactobacillus supplementation. Nat Commun (2018) 9(1):707. doi: 10.1038/s41467-018-03157-4
142. Stokholm J, Blaser MJ, Thorsen J, Rasmussen MA, Waage J, Vinding RK, et al. Maturation of the gut microbiome and risk of asthma in childhood. Nat Commun (2018) 9(1):141. doi: 10.1038/s41467-017-02573-2
143. Krzych-Falta E, Furmanczyk K, Tomaszewska A, Olejniczak D, Samolinski B, Samolinska-Zawisza U. Probiotics: Myths or facts about their role in allergy prevention. Adv Clin Exp Med (2018) 27(1):119–24. doi: 10.17219/acem/65476
144. Liu A, Ma T, Xu N, Jin H, Zhao F, Kwok LY, et al. Adjunctive probiotics alleviates asthmatic symptoms via modulating the gut microbiome and serum metabolome. Microbiol Spectr. (2021) 9(2):e0085921. doi: 10.1128/Spectrum.00859-21
145. Chen JC, Tsai CC, Hsieh CC, Lan A, Huang CC, Leu SF. Multispecies probiotics combination prevents ovalbumin-induced airway hyperreactivity in mice. Allergol Immunopathol (Madr). (2018) 46(4):354–60. doi: 10.1016/j.aller.2018.02.001
146. Cervantes-Garcia D, Jimenez M, Rivas-Santiago CE, Gallegos-Alcala P, Hernandez-Mercado A, Santoyo-Payan LS, et al. Lactococcus lactis NZ9000 Prevents Asthmatic Airway Inflammation and Remodelling in Rats through the Improvement of Intestinal Barrier Function and Systemic TGF-beta Production. Int Arch Allergy Immunol (2021) 182(4):277–91. doi: 10.1159/000511146
147. Jamalkandi SA, Ahmadi A, Ahrari I, Salimian J, Karimi M, Ghanei M. Oral and nasal probiotic administration for the prevention and alleviation of allergic diseases, asthma and chronic obstructive pulmonary disease. Nutr Res Rev (2021) 34(1):1–16. doi: 10.1017/S0954422420000116
148. Bellodas Sanchez J, Kadrofske M. Necrotizing enterocolitis. Neurogastroenterol Motil. (2019) 31(3):e13569. doi: 10.1111/nmo.13569
149. Imren C, Vlug LE, de Koning BAE, Diertens T, Snel HE, Suurland J, et al. Necrotizing enterocolitis in a dutch cohort of very preterm infants: prevalence, mortality, and long-term outcomes. Eur J Pediatr Surg (2022) 32(1):111–9. doi: 10.1055/s-0041-1741544
150. Jia CH, Feng ZS, Lin XJ, Cui QL, Han SS, Jin Y, et al. Short term outcomes of extremely low birth weight infants from a multicenter cohort study in Guangdong of China. Sci Rep (2022) 12(1):11119. doi: 10.1038/s41598-022-14432-2
151. Nino DF, Sodhi CP, Hackam DJ. Necrotizing enterocolitis: new insights into pathogenesis and mechanisms. Nat Rev Gastroenterol Hepatol (2016) 13(10):590–600. doi: 10.1038/nrgastro.2016.119
152. Chen WY, Lo YC, Huang PH, Chen YX, Tsao PC, Lee YS, et al. Increased antibiotic exposure in early life is associated with adverse outcomes in very low birth weight infants. J Chin Med Assoc (2022) 85(9):939–43. doi: 10.1097/JCMA.0000000000000749
153. Pammi M, Cope J, Tarr PI, Warner BB, Morrow AL, Mai V, et al. Intestinal dysbiosis in preterm infants preceding necrotizing enterocolitis: a systematic review and meta-analysis. Microbiome (2017) 5(1):31. doi: 10.1186/s40168-017-0248-8
154. He Y, Du W, Xiao S, Zeng B, She X, Liu D, et al. Colonization of fecal microbiota from patients with neonatal necrotizing enterocolitis exacerbates intestinal injury in germfree mice subjected to necrotizing enterocolitis-induction protocol via alterations in butyrate and regulatory T cells. J Transl Med (2021) 19(1):510. doi: 10.1186/s12967-021-03109-5
155. Schonherr-Hellec S, Klein GL, Delannoy J, Ferraris L, Roze JC, Butel MJ, et al. Clostridial strain-specific characteristics associated with necrotizing enterocolitis. Appl Environ Microbiol (2018) 84(7):e02428–17. doi: 10.1128/AEM.02428-17
156. Tarracchini C, Milani C, Longhi G, Fontana F, Mancabelli L, Pintus R, et al. Unraveling the microbiome of necrotizing enterocolitis: insights in novel microbial and metabolomic biomarkers. Microbiol Spectr. (2021) 9(2):e0117621. doi: 10.1128/Spectrum.01176-21
157. Olm MR, Bhattacharya N, Crits-Christoph A, Firek BA, Baker R, Song YS, et al. Necrotizing enterocolitis is preceded by increased gut bacterial replication, Klebsiella, and fimbriae-encoding bacteria. Sci Adv (2019) 5(12):eaax5727. doi: 10.1126/sciadv.aax5727
158. Paveglio S, Ledala N, Rezaul K, Lin Q, Zhou Y, Provatas AA, et al. Cytotoxin-producing Klebsiella oxytoca in the preterm gut and its association with necrotizing enterocolitis. Emerg Microbes Infect (2020) 9(1):1321–9. doi: 10.1080/22221751.2020.1773743
159. Itani T, Ayoub Moubareck C, Melki I, Rousseau C, Mangin I, Butel MJ, et al. Preterm infants with necrotising enterocolitis demonstrate an unbalanced gut microbiota. Acta Paediatr (2018) 107(1):40–7. doi: 10.1111/apa.14078
160. Stafford IA, Rodrigue E, Berra A, Adams W, Heard AJ, Hagan JL, et al. The strong correlation between neonatal early-onset Group B Streptococcal disease and necrotizing enterocolitis. Eur J Obstet Gynecol Reprod Biol (2018) 223:93–7. doi: 10.1016/j.ejogrb.2018.02.024
161. Brunse A, Deng L, Pan X, Hui Y, Castro-Mejia JL, Kot W, et al. Fecal filtrate transplantation protects against necrotizing enterocolitis. ISME J (2022) 16(3):686–94. doi: 10.1038/s41396-021-01107-5
162. Morgan RL, Preidis GA, Kashyap PC, Weizman AV, Sadeghirad B, McMaster Probiotic P, et al. Probiotics reduce mortality and morbidity in preterm, low-birth-weight infants: A systematic review and network meta-analysis of randomized trials. Gastroenterology (2020) 159(2):467–80. doi: 10.1053/j.gastro.2020.05.096
163. Sowden M, van Weissenbruch MM, Bulabula ANH, van Wyk L, Twisk J, van Niekerk E. Effect of a multi-strain probiotic on the incidence and severity of necrotizing enterocolitis and feeding intolerances in preterm neonates. Nutrients (2022) 14(16):3305. doi: 10.3390/nu14163305
164. Delaplain PT, Bell BA, Wang J, Isani M, Zhang E, Gayer CP, et al. Effects of artificially introduced Enterococcus faecalis strains in experimental necrotizing enterocolitis. PloS One (2019) 14(11):e0216762. doi: 10.1371/journal.pone.0216762
165. Hui Y, Smith B, Mortensen MS, Krych L, Sorensen SJ, Greisen G, et al. The effect of early probiotic exposure on the preterm infant gut microbiome development. Gut Microbes (2021) 13(1):1951113. doi: 10.1080/19490976.2021.1951113
166. Yu R, Jiang S, Tao Y, Li P, Yin J, Zhou Q. Inhibition of HMGB1 improves necrotizing enterocolitis by inhibiting NLRP3 via TLR4 and NF-kappaB signaling pathways. J Cell Physiol (2019) 234(8):13431–8. doi: 10.1002/jcp.28022
167. Sun Q, Ji YC, Wang ZL, She X, He Y, Ai Q, et al. Sodium butyrate alleviates intestinal inflammation in mice with necrotizing enterocolitis. Mediators Inflamm (2021) 2021:6259381. doi: 10.1155/2021/6259381
168. Le Mandat Schultz A, Bonnard A, Barreau F, Aigrain Y, Pierre-Louis C, Berrebi D, et al. Expression of TLR-2, TLR-4, NOD2 and pNF-kappaB in a neonatal rat model of necrotizing enterocolitis. PloS One (2007) 2(10):e1102. doi: 10.1371/journal.pone.0001102
169. Hunter C, Dimaguila MA, Gal P, Wimmer JE Jr., Ransom JL, Carlos RQ, et al. Effect of routine probiotic, Lactobacillus reuteri DSM 17938, use on rates of necrotizing enterocolitis in neonates with birthweight < 1000 grams: a sequential analysis. BMC Pediatr (2012) 12:142. doi: 10.1186/1471-2431-12-142
170. Silverman GJ, Deng J, Azzouz DF. Sex-dependent Lupus Blautia (Ruminococcus) gnavus strain induction of zonulin-mediated intestinal permeability and autoimmunity. Front Immunol (2022) 13:897971. doi: 10.3389/fimmu.2022.897971
171. Ma Y, Guo R, Sun Y, Li X, He L, Li Z, et al. Lupus gut microbiota transplants cause autoimmunity and inflammation. Clin Immunol (2021) 233:108892. doi: 10.1016/j.clim.2021.108892
172. Xiang K, Wang P, Xu Z, Hu YQ, He YS, Chen Y, et al. Causal effects of gut microbiome on systemic lupus erythematosus: A two-sample mendelian randomization study. Front Immunol (2021) 12:667097. doi: 10.3389/fimmu.2021.667097
173. Liu F, Ren T, Li X, Zhai Q, Xu X, Zhang N, et al. Distinct microbiomes of gut and saliva in patients with systemic lupus erythematous and clinical associations. Front Immunol (2021) 12:626217. doi: 10.3389/fimmu.2021.626217
174. He J, Chan T, Hong X, Zheng F, Zhu C, Yin L, et al. Microbiome and metabolome analyses reveal the disruption of lipid metabolism in systemic lupus erythematosus. Front Immunol (2020) 11:1703. doi: 10.3389/fimmu.2020.01703
175. Tomofuji Y, Maeda Y, Oguro-Igashira E, Kishikawa T, Yamamoto K, Sonehara K, et al. Metagenome-wide association study revealed disease-specific landscape of the gut microbiome of systemic lupus erythematosus in Japanese. Ann Rheum Dis (2021) 80(12):1575–83. doi: 10.1136/annrheumdis-2021-220687
176. Chen BD, Jia XM, Xu JY, Zhao LD, Ji JY, Wu BX, et al. An autoimmunogenic and proinflammatory profile defined by the gut microbiota of patients with untreated systemic lupus erythematosus. Arthritis Rheumatol (2021) 73(2):232–43. doi: 10.1002/art.41511
177. Shirakashi M, Maruya M, Hirota K, Tsuruyama T, Matsuo T, Watanabe R, et al. Effect of impaired T cell receptor signaling on the gut microbiota in a mouse model of systemic autoimmunity. Arthritis Rheumatol (2022) 74(4):641–53. doi: 10.1002/art.42016
178. Azzouz D, Omarbekova A, Heguy A, Schwudke D, Gisch N, Rovin BH, et al. Lupus nephritis is linked to disease-activity associated expansions and immunity to a gut commensal. Ann Rheum Dis (2019) 78(7):947–56. doi: 10.1136/annrheumdis-2018-214856
179. Li Y, Wang HF, Li X, Li HX, Zhang Q, Zhou HW, et al. Disordered intestinal microbes are associated with the activity of Systemic Lupus Erythematosus. Clin Sci (Lond). (2019) 133(7):821–38. doi: 10.1042/CS20180841
180. Ma Y, Xu X, Li M, Cai J, Wei Q, Niu H. Gut microbiota promote the inflammatory response in the pathogenesis of systemic lupus erythematosus. Mol Med (2019) 25(1):35. doi: 10.1186/s10020-019-0102-5
181. Tampe D, Hakroush S, Tampe B. Dissecting signalling pathways associated with intrarenal synthesis of complement components in lupus nephritis. RMD Open (2022) 8(2):e00251. doi: 10.1136/rmdopen-2022-002517
182. Pucci G, Vaudo G. Cardiac and vascular damage in systemic erythematosus lupus. Is Dis activity mediator? Eur J Intern Med (2020) 73:23–4. doi: 10.1016/j.ejim.2020.01.013
183. Mauro D, Nerviani A. Endothelial dysfunction in systemic lupus erythematosus: pathogenesis, assessment and therapeutic opportunities. Rev Recent Clin Trials. (2018) 13(3):192–8. doi: 10.2174/1574887113666180314091831
184. de la Visitacion N, Robles-Vera I, Toral M, O'Valle F, Moleon J, Gomez-Guzman M, et al. Lactobacillus fermentum CECT5716 prevents renal damage in the NZBWF1 mouse model of systemic lupus erythematosus. Food Funct (2020) 11(6):5266–74. doi: 10.1039/D0FO00578A
185. Hu WS, Rajendran P, Tzang BS, Yeh YL, Shen CY, Chen RJ, et al. Lactobacillus paracasei GMNL-32 exerts a therapeutic effect on cardiac abnorMalities in NZB/W F1 mice. PloS One (2017) 12(9):e0185098. doi: 10.1371/journal.pone.0185098
186. de la Visitacion N, Robles-Vera I, Moleon-Moya J, Sanchez M, Jimenez R, Gomez-Guzman M, et al. Probiotics prevent hypertension in a murine model of systemic lupus erythematosus induced by toll-like receptor 7 activation. Nutrients (2021) 13(8):2669. doi: 10.3390/nu13082669
187. Zhang Y, Murugesan P, Huang K, Cai H. NADPH oxidases and oxidase crosstalk in cardiovascular diseases: novel therapeutic targets. Nat Rev Cardiol (2020) 17(3):170–94. doi: 10.1038/s41569-019-0260-8
188. Tschudi MR, Mesaros S, Luscher TF, Malinski T. Direct in situ measurement of nitric oxide in mesenteric resistance arteries. Increased decomposition by superoxide in hypertension. Hypertension (1996) 27(1):32–5. doi: 10.1161/01.HYP.27.1.32
189. Mardani F, Mahmoudi M, Esmaeili SA, Khorasani S, Tabasi N, Rastin M. In vivo study: Th1-Th17 reduction in pristane-induced systemic lupus erythematosus mice after treatment with tolerogenic Lactobacillus probiotics. J Cell Physiol (2018) 234(1):642–9. doi: 10.1002/jcp.26819
190. Wang X, Xia Y. Anti-double stranded DNA antibodies: origin, pathogenicity, and targeted therapies. Front Immunol (2019) 10:1667. doi: 10.3389/fimmu.2019.01667
191. Wang Y, Xiao S, Xia Y, Wang H. The therapeutic strategies for SLE by targeting anti-dsDNA antibodies. Clin Rev Allergy Immunol (2022) 63(2):152–65. doi: 10.1007/s12016-021-08898-7
192. Pisetsky DS, Lipsky PE. New insights into the role of antinuclear antibodies in systemic lupus erythematosus. Nat Rev Rheumatol (2020) 16(10):565–79. doi: 10.1038/s41584-020-0480-7
193. Li PH, Wong WH, Lee TL, Lau CS, Chan TM, Leung AM, et al. Relationship between autoantibody clustering and clinical subsets in SLE: cluster and association analyses in Hong Kong Chinese. Rheumatol (Oxford). (2013) 52(2):337–45. doi: 10.1093/rheumatology/kes261
194. Talaat RM, Mohamed SF, Bassyouni IH, Raouf AA. Th1/Th2/Th17/Treg cytokine imbalance in systemic lupus erythematosus (SLE) patients: Correlation with disease activity. Cytokine (2015) 72(2):146–53. doi: 10.1016/j.cyto.2014.12.027
195. Alvarez-Rodriguez L, Martinez-Taboada V, Calvo-Alen J, Beares I, Villa I, Lopez-Hoyos M. Altered Th17/treg ratio in peripheral blood of systemic lupus erythematosus but not primary antiphospholipid syndrome. Front Immunol (2019) 10:391. doi: 10.3389/fimmu.2019.00391
196. Yuliasih Y, Rahmawati LD, Putri RM. Th17/treg ratio and disease activity in systemic lupus erythematosus. Caspian J Intern Med (2019) 10(1):65–72. doi: 10.22088/cjim.10.1.65
197. Li D, Pan Y, Xia X, Liang J, Liu F, Dou H, et al. Bacteroides fragilis alleviates the symptoms of lupus nephritis via regulating CD1d and CD86 expressions in B cells. Eur J Pharmacol (2020) 884:173421. doi: 10.1016/j.ejphar.2020.173421
198. Kim DS, Park Y, Choi JW, Park SH, Cho ML, Kwok SK. Lactobacillus acidophilus Supplementation Exerts a Synergistic Effect on Tacrolimus Efficacy by Modulating Th17/Treg Balance in Lupus-Prone Mice via the SIGNR3 Pathway. Front Immunol (2021) 12:696074. doi: 10.3389/fimmu.2021.696074
199. Zhou T, Lin S, Yang S, Lin W. Efficacy and safety of tacrolimus in induction therapy of patients with lupus nephritis. Drug Des Devel Ther (2019) 13:857–69. doi: 10.2147/DDDT.S189156
200. Suzuki K, Kameda H, Amano K, Nagasawa H, Takei H, Nishi E, et al. Single center prospective study of tacrolimus efficacy and safety in the treatment of various manifestations in systemic lupus erythematosus. Rheumatol Int (2011) 31(6):757–63. doi: 10.1007/s00296-010-1366-9
201. Tani C, Elefante E, Martin-Cascon M, Belhocine M, Lavilla Olleros C, Vagelli R, et al. Tacrolimus in non-Asian patients with SLE: a real-life experience from three European centres. Lupus Sci Med (2018) 5(1):e000274. doi: 10.1136/lupus-2018-000274
202. Park JS, Kim SM, Hwang SH, Choi SY, Kwon JY, Kwok SK, et al. Combinatory treatment using tacrolimus and a STAT3 inhibitor regulate Treg cells and plasma cells. Int J Immunopathol Pharmacol (2018) 32:2058738418778724. doi: 10.1177/2058738418778724
203. Mueller AL, Payandeh Z, Mohammadkhani N, Mubarak SMH, Zakeri A, Alagheband Bahrami A, et al. Recent advances in understanding the pathogenesis of rheumatoid arthritis: new treatment strategies. Cells (2021) 10(11):3017. doi: 10.3390/cells10113017
204. Qiu J, Wu B, Goodman SB, Berry GJ, Goronzy JJ, Weyand CM. Metabolic control of autoimmunity and tissue inflammation in rheumatoid arthritis. Front Immunol (2021) 12:652771. doi: 10.3389/fimmu.2021.652771
205. Zhou N, Zou F, Cheng X, Huang Y, Zou H, Niu Q, et al. Porphyromonas gingivalis induces periodontitis, causes immune imbalance, and promotes rheumatoid arthritis. J Leukoc Biol (2021) 110(3):461–73. doi: 10.1002/JLB.3MA0121-045R
206. Cheng Z, Do T, Mankia K, Meade J, Hunt L, Clerehugh V, et al. Dysbiosis in the oral microbiomes of anti-CCP positive individuals at risk of developing rheumatoid arthritis. Ann Rheum Dis (2021) 80(2):162–8. doi: 10.1136/annrheumdis-2020-216972
207. Kroese JM, Brandt BW, Buijs MJ, Crielaard W, Lobbezoo F, Loos BG, et al. Differences in the oral microbiome in patients with early rheumatoid arthritis and individuals at risk of rheumatoid arthritis compared to healthy individuals. Arthritis Rheumatol (2021) 73(11):1986–93. doi: 10.1002/art.41780
208. Kishikawa T, Maeda Y, Nii T, Motooka D, Matsumoto Y, Matsushita M, et al. Metagenome-wide association study of gut microbiome revealed novel aetiology of rheumatoid arthritis in the Japanese population. Ann Rheum Dis (2020) 79(1):103–11. doi: 10.1136/annrheumdis-2019-215743
209. Jubair WK, Hendrickson JD, Severs EL, Schulz HM, Adhikari S, Ir D, et al. Modulation of inflammatory arthritis in mice by gut microbiota through mucosal inflammation and autoantibody generation. Arthritis Rheumatol (2018) 70(8):1220–33. doi: 10.1002/art.40490
210. Kitamura K, Shionoya H, Suzuki S, Fukai R, Uda S, Abe C, et al. Oral and intestinal bacterial substances associated with disease activities in patients with rheumatoid arthritis: A cross-sectional clinical study. J Immunol Res (2022) 2022:6839356. doi: 10.1155/2022/6839356
211. Cannarella LAT, Mari NL, Alcantara CC, Iryioda TMV, Costa NT, Oliveira SR, et al. Mixture of probiotics reduces inflammatory biomarkers and improves the oxidative/nitrosative profile in people with rheumatoid arthritis. Nutrition (2021) 89:111282. doi: 10.1016/j.nut.2021.111282
212. Dourado E, Ferro M, Sousa Guerreiro C, Fonseca JE. Diet as a modulator of intestinal microbiota in rheumatoid arthritis. Nutrients (2020) 12(11):3504. doi: 10.3390/nu12113504
213. Gioia C, Lucchino B, Tarsitano MG, Iannuccelli C, Di Franco M. Dietary habits and nutrition in rheumatoid arthritis: can diet influence disease development and clinical manifestations? Nutrients (2020) 12(5):1456. doi: 10.3390/nu12051456
214. Hulander E, Barebring L, Turesson Wadell A, Gjertsson I, Calder PC, Winkvist A, et al. Diet intervention improves cardiovascular profile in patients with rheumatoid arthritis: results from the randomized controlled cross-over trial ADIRA. Nutr J (2021) 20(1):9. doi: 10.1186/s12937-021-00663-y
215. Wang Y, Zhu D, Ortiz-Velez LC, Perry JL, Pennington MW, Hyser JM, et al. A bioengineered probiotic for the oral delivery of a peptide Kv1.3 channel blocker to treat rheumatoid arthritis. Proc Natl Acad Sci U.S.A. (2023) 120(2):e2211977120. doi: 10.1073/pnas.2211977120
216. Hemmer B, Kerschensteiner M, Korn T. Role of the innate and adaptive immune responses in the course of multiple sclerosis. Lancet Neurol (2015) 14(4):406–19. doi: 10.1016/S1474-4422(14)70305-9
217. Rodriguez Murua S, Farez MF, Quintana FJ. The immune response in multiple sclerosis. Annu Rev Pathol (2022) 17:121–39. doi: 10.1146/annurev-pathol-052920-040318
218. Elsayed NS, Aston P, Bayanagari VR, Shukla SK. The gut microbiome molecular mimicry piece in the multiple sclerosis puzzle. Front Immunol (2022) 13:972160. doi: 10.3389/fimmu.2022.972160
219. Bianchimano P, Britton GJ, Wallach DS, Smith EM, Cox LM, Liu S, et al. Mining the microbiota to identify gut commensals modulating neuroinflammation in a mouse model of multiple sclerosis. Microbiome (2022) 10(1):174. doi: 10.1186/s40168-022-01364-2
220. Cox LM, Maghzi AH, Liu S, Tankou SK, Dhang FH, Willocq V, et al. Gut microbiome in progressive multiple sclerosis. Ann Neurol (2021) 89(6):1195–211. doi: 10.1002/ana.26084
221. Reynders T, Devolder L, Valles-Colomer M, Van Remoortel A, Joossens M, De Keyser J, et al. Gut microbiome variation is associated to Multiple Sclerosis phenotypic subtypes. Ann Clin Transl Neurol (2020) 7(4):406–19. doi: 10.1002/acn3.51004
222. Gandy KAO, Zhang J, Nagarkatti P, Nagarkatti M. The role of gut microbiota in shaping the relapse-remitting and chronic-progressive forms of multiple sclerosis in mouse models. Sci Rep (2019) 9(1):6923. doi: 10.1038/s41598-019-43356-7
223. Horton MK, McCauley K, Fadrosh D, Fujimura K, Graves J, Ness J, et al. Gut microbiome is associated with multiple sclerosis activity in children. Ann Clin Transl Neurol (2021) 8(9):1867–83. doi: 10.1002/acn3.51441
224. Levi I, Gurevich M, Perlman G, Magalashvili D, Menascu S, Bar N, et al. Potential role of indolelactate and butyrate in multiple sclerosis revealed by integrated microbiome-metabolome analysis. Cell Rep Med (2021) 2(4):100246. doi: 10.1016/j.xcrm.2021.100246
225. Roager HM, Licht TR. Microbial tryptophan catabolites in health and disease. Nat Commun (2018) 9(1):3294. doi: 10.1038/s41467-018-05470-4
226. Chyan YJ, Poeggeler B, Omar RA, Chain DG, Frangione B, Ghiso J, et al. Potent neuroprotective properties against the Alzheimer beta-amyloid by an endogenous melatonin-related indole structure, indole-3-propionic acid. J Biol Chem (1999) 274(31):21937–42. doi: 10.1074/jbc.274.31.21937
227. Tankou SK, Regev K, Healy BC, Cox LM, Tjon E, Kivisakk P, et al. Investigation of probiotics in multiple sclerosis. Multiple sclerosis (Houndmills Basingstoke England). (2018) 24(1):58–63. doi: 10.1177/1352458517737390
228. Rahimlou M, Hosseini SA, Majdinasab N, Haghighizadeh MH, Husain D. Effects of long-term administration of Multi-Strain Probiotic on circulating levels of BDNF, NGF, IL-6 and mental health in patients with multiple sclerosis: a randomized, double-blind, placebo-controlled trial. Nutr Neurosci (2022) 25(2):411–22. doi: 10.1080/1028415X.2020.1758887
229. Tanis KQ, Newton SS, Duman RS. Targeting neurotrophic/growth factor expression and signaling for antidepressant drug development. CNS neurological Disord Drug targets. (2007) 6(2):151–60. doi: 10.2174/187152707780363276
Keywords: Limosilactobacillus reuteri, immune system disease, atopic dermatitis (AD), asthma, systemic lupus erythematosus (SLE), rheumatoid arthritis, multiple sclerosis, necrotizing enterocolitis (NEC)
Citation: Luo Z, Chen A, Xie A, Liu X, Jiang S and Yu R (2023) Limosilactobacillus reuteri in immunomodulation: molecular mechanisms and potential applications. Front. Immunol. 14:1228754. doi: 10.3389/fimmu.2023.1228754
Received: 25 May 2023; Accepted: 18 July 2023;
Published: 09 August 2023.
Edited by:
Jian Shen, Shanghai Jiao Tong University, ChinaReviewed by:
Jinhui Li, Saint Louis University, United StatesCopyright © 2023 Luo, Chen, Xie, Liu, Jiang and Yu. This is an open-access article distributed under the terms of the Creative Commons Attribution License (CC BY). The use, distribution or reproduction in other forums is permitted, provided the original author(s) and the copyright owner(s) are credited and that the original publication in this journal is cited, in accordance with accepted academic practice. No use, distribution or reproduction is permitted which does not comply with these terms.
*Correspondence: Renqiang Yu, eXVyZW5xaWFuZzU1M0AxNjMuY29t
†These authors have contributed equally to this work
Disclaimer: All claims expressed in this article are solely those of the authors and do not necessarily represent those of their affiliated organizations, or those of the publisher, the editors and the reviewers. Any product that may be evaluated in this article or claim that may be made by its manufacturer is not guaranteed or endorsed by the publisher.
Research integrity at Frontiers
Learn more about the work of our research integrity team to safeguard the quality of each article we publish.