- 1Pediatric Inherited Diseases Research Center, Isfahan University of Medical Sciences, Isfahan, Iran
- 2Department of Genetics and Molecular Biology, School of Medicine, Isfahan University of Medical Sciences, Isfahan, Iran
- 3The Persian Gulf Tropical Medicine Research Center, The Persian Gulf Biomedical Sciences Research Institute, Bushehr University of Medical Sciences, Bushehr, Iran
- 4Department of Tissue Engineering and Biomaterials, School of Advanced Medical Sciences and Technologies, Hamadan University of Medical Sciences, Hamadan, Iran
Angiogenesis is a hallmark of cancer biology, and neoadjuvant therapies targeting either tumor vasculature or VEGF signaling have been developed to treat solid malignant tumors. However, these therapies induce complete vascular depletion leading to hypoxic niche, drug resistance, and tumor recurrence rate or leading to impaired delivery of chemo drugs and immune cell infiltration at the tumor site. Achieving a balance between oxygenation and tumor growth inhibition requires determining vascular normalization after treatment with a low dose of antiangiogenic agents. However, monotherapy within the approved antiangiogenic agents’ benefits only some tumors and their efficacy improvement could be achieved using immunotherapy and emerging nanocarriers as a clinical tool to optimize subsequent therapeutic regimens and reduce the need for a high dosage of chemo agents. More importantly, combined immunotherapies and nano-based delivery systems can prolong the normalization window while providing the advantages to address the current treatment challenges within antiangiogenic agents. This review summarizes the approved therapies targeting tumor angiogenesis, highlights the challenges and limitations of current therapies, and discusses how vascular normalization, immunotherapies, and nanomedicine could introduce the theranostic potentials to improve tumor management in future clinical settings.
1 Introduction
Rapid growth and progression of solid tumors results in hypoxia, which drives tumor angiogenesis to remove waste products and provide nutrients and oxygen to support tumor growth and survival (1). Under hypoxic conditions, activated hypoxia-inducible factor-1 (HIF-1) upregulates vascular endothelial growth factors (VEGFs), which bind to their receptors (VEGFRs), expressed on endothelial cells (ECs) or tip cells in established vessels, followed by remodeling of the surrounding extracellular matrix (ECM) and formation of new blood vessels (1). Tumor-associated blood vessels are characterized by abnormal structure and morphology, immature basement membrane, excessive branching, discontinuous EC junctions resulting in high permeability, and resistance to senescence (2). Cancer cells, regulatory immune cells [e.g., immature dendritic cells (DCs), regulatory T cells (Tregs), myeloid-derived suppressor cells (MDSCs), and tumor-associated macrophages (TAM)], and stromal cells also secrete the various cytokines and proangiogenic factors that support tumor angiogenesis (3). In summary, circulating tumor cells promote metastasis, and angiogenesis facilitates cell proliferation, tumor progression, and migration of invasive types into the bloodstream. Therefore, modulation of tumor vasculature may be a promising alternative therapeutic approach in combination with other standard therapies such as immunotherapy and radio/chemotherapy.
We have focused on immunotherapy, and monoclonal antibodies (mAbs) targeting VEGF/VEGFRs have been developed, leading to FDA approval and improvement in overall survival (OS) in some patients treated with mAbs (4). However, drug resistance and hypoxia induction minimize the clinical benefit of anti-angiogenic therapy in some cancers (5) and, more importantly, disruption of the vasculature itself compromises the therapeutic efficacy of chemotherapy or radiotherapy, which are often used in combination with immunotherapy. Although the tumor microenvironment (TME) and multiple cellular and molecular mechanisms provide immunosuppressive processes and impair the host’s anti-tumor immune surveillance (6), complete disruption of the tumor vasculature also interferes with the clinical benefit of immunotherapeutic approaches such as adoptive cell therapy (ACT), which require infiltration of blood vessels into the tumor (7). In addition, many drugs and immune cells require normal oxygen levels to activate and kill cancer cells (8). Considering these challenges, a promising alternative therapy is the remodeling of the tumor vasculature or vascular normalization (9), which overcomes the hypoxic TME (10) and provides the condition for the administration of immunotherapy (8), chemotherapy (11), and radiotherapy (12). For example, vascular normalization improve the efficacy of administered immune checkpoint blockade (ICB) therapy (anti-PD1/PDL1 mAbs), which causes serious immune-related adverse events (8).
Herein, we briefly review existing monotherapies targeting tumor angiogenesis, discuss the limitations and challenges within these therapies, and then provide a perspective on approaches including TME reprogramming approaches, combination therapy, and nanoimmunotherapy that may improve the therapeutic efficacy of vascular-based immunotherapies.
2 Targeted therapy for tumor angiogenesis
Since the discovery of angiogenesis as a major driver of tumor growth and progression, various anti-angiogenic agents have been developed in the form of monotherapy immunotherapies and chemotherapies or their combinations, and some of them such as bevacizumab, ramucirumab, trastuzumab and pertuzumab have been approved by the FDA for human cancer therapy (Table 1). These therapies include biotherapeutic mAbs that target the ligand-receptor interaction and small molecule tyrosine kinase inhibitors (TKIs) that target the kinase activity of receptor TKs to inhibit downstream signaling cascade activation (34). TKIs and mAb are commonly developed against VEGF/VEGFR, epidermal growth factor receptor (EGFR), and fibroblast growth factor-2 (FGF-2) and their related signaling pathways. FGF-2 has proangiogenic behaviors in paracrine effect and by induction of EC proliferation and migration and promotes angiogenesis by inducing secretion of MMPs to remodel ECM (35). Platelet-derived growth factor (PDGF)-BB (36) and angiopoietins (ANGPTs) (37) are other growth factor molecules that promote vascular maturation and stabilization of newly formed vessels and have been developed for targeted tumor angiogenesis. However, antiangiogenic monotherapy has provided more benefit in highly angiogenesis-dependent tumor types such as advanced renal cell carcinoma (RCC), hepatocellular carcinoma (HCC), and colorectal cancer (CRC) (13) (15, 16). Therefore, it is necessary to combine these therapies (i.e., mAbs and small-molecule drugs targeting VEGF, EGFR, and PDGFR; Table 1) or to combine them with radiotherapy to improve survival in patients with other tumors or combined with radiotherapy to improve OS in patients with other tumors (29, 30) (31). In fact, monotherapy with antiangiogenic agents sometimes resulted in vascular depletion but not normalization, followed by induction of hypoxia, nutrient-deprived effector immune cells, drug resistance, and increased tumor invasiveness (5) (8). Thus, the introduction of the “window of vessel normalization” would lead to better outcomes in the clinic. However, depending on the tumor type and the dose of antiangiogenic agents, this window time would be different and could be optimized and extended by various strategies to improve therapies targeting tumor angiogenesis (Section 4).
3 Challenges in modulating tumor angiogenesis
In addition to hypoxia and niche modulation of effector immune cells, targeting angiogenesis resulted in drug resistance derived from TME components such as stromal cells, progenitor cells, and regulatory immune cells (Tregs, MDSCs, and TAMs) that support tumor progression and immune escape (6, 38, 39) (Figure 1A). Blockade of vascularization leads to ECM remodeling, the release of proinflammatory/proangiogenic factors (42), induction of signaling pathways that mediate EC proliferation (43), and stimulation of proangiogenic myeloid cells (44), followed by recruitment of endothelial progenitor cells (EPCs), angiogenesis, and resistance to anti-VEGF therapy. Cancer-associated fibroblasts (CAFs) are the most common type of stromal cells in the TME that reprogram ECs and produce MMPs to degrade ECM and promote angiogenesis (45). Importantly, high-dose anti-VEGF therapy plus hypoxic nicotine results in a population of cancer stem cells (CSCs) that are responsible for drug resistance, tumor relapse, and metastasis (6, 46). In addition, the heterogeneity of blood vessels in different organs or tissues has complicated anti-VEGF therapies in solid tumors (47).
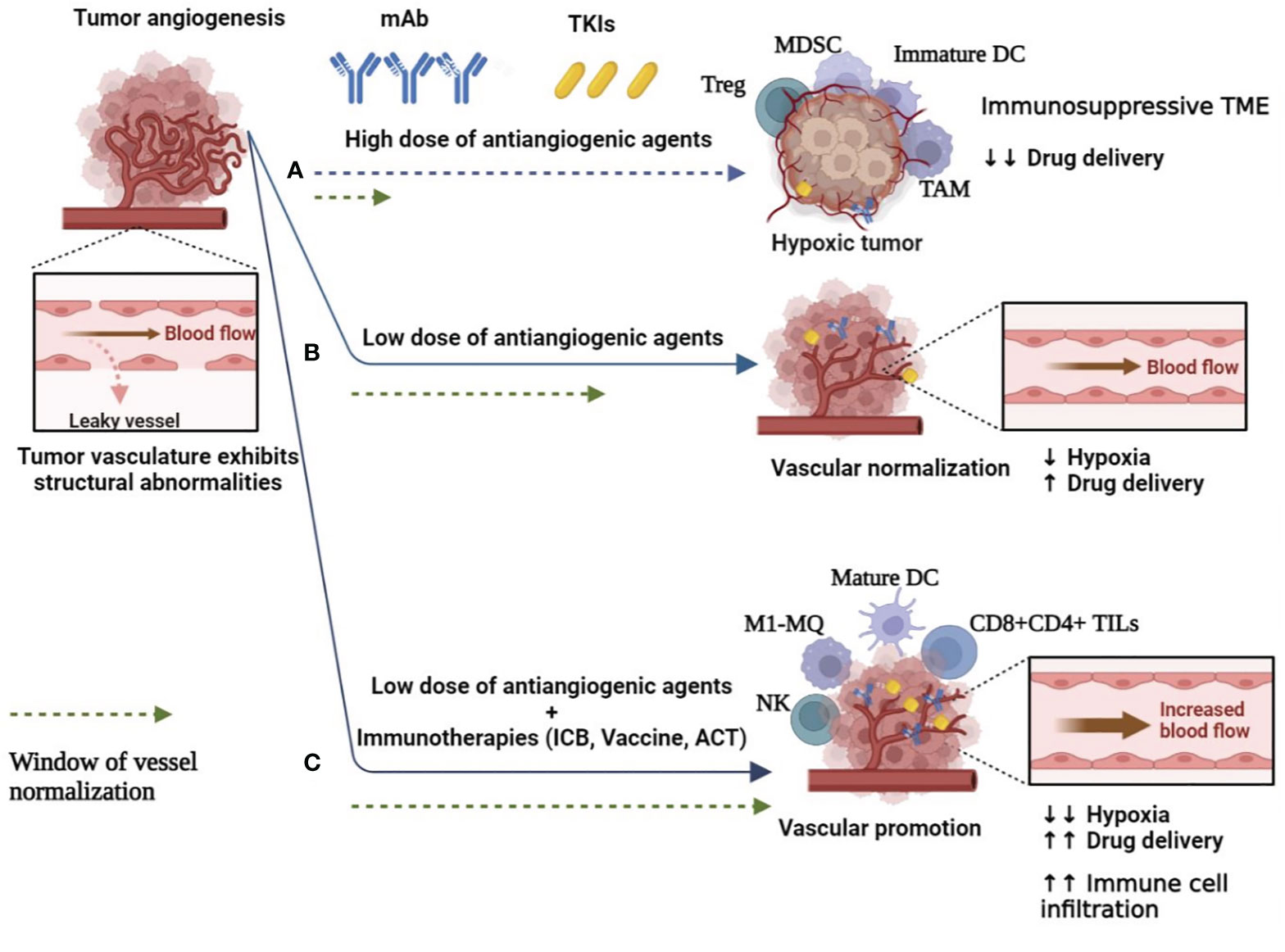
Figure 1 Switching the abnormal and leaky tumor blood vessels to normal function to improve angiogenesis-based cancer therapies. (A) High dose of antiangiogenic agents (mAb and TKIs) resulted in complete vascular disruption and hypoxia, and immunosuppressive TME, which support cancer cell survival, limit drug delivery and induce drug resistance. Furthermore, the abnormal TME is associated with increased infiltration of immunosuppressive Tregs, TAMs, MDCSs, and immature DCs. Hypoxic conditions also impair the therapeutic efficacy of radiotherapy and chemotherapy (6, 38, 39). (B) Low doses of antiangiogenic agents lead to transient restoration of blood flow and perfusion. This reduces hypoxic TME and increases drug delivery to tumor sites (40). (C) Anti-angiogenic agents combined with immunotherapies could expand the normalization window, enhance drug delivery and immune effector cell infiltration, and thus reprogram the immunosuppressive tumor microenvironment to an immunosupportive one (41). DC, dendritic cell; MQ, macrophage; mAb, monoclonal antibodies; MDSC, myeloid-derived suppressor cells; TAMs, tumor-associated macrophages; TKI, tyrosine kinase inhibitor; TIL, tumor infiltration lymphocyte; TME, tumor microenvironment; Tregs, regulatory T cells.
Disruption of the blood supply acts as a double-edged sword, impairing effector T-cell infiltration, limiting drug delivery to tumor sites (Figure 1A), and depriving radiotherapy of the oxygen needed to generate cytotoxic radical ions, all of which reduce antitumor efficacy (5, 8). Cancer cells can potentially differentiate into endothelial-like phenotypes that support neoangiogenesis in an EC-independent manner and are responsible for patients’ resistance to blockade of the VEGF pathway (48). In addition, VEGF blockers induce the production of proangiogenic factors (e.g., VEGF, bFGF, and PDGF), which drive compensatory mechanisms of promoting angiogenesis and disease progression (49), emphasizing the development of a new generation of drugs to simultaneously target the VEGF signaling pathway and alternative angiogenic derivers.
In terms of function and structure of the approved biotherapeutic agent, after intravitreal drug injection, the full size of mAb limited their kidney excreted through urine and the Fc domain of mAb bind to neonatal Fc receptor (FcRn), expressed by ECs, resulting in prolonged systemic exposure as well as possible adverse events (50). Unlike mAbs that bind specific targets, multi-targeted TKIs have broader bioactivity and exhibit greater antitumor activity but are more toxic (51). In addition, productive anti-VEGF agents need to be administered frequently, which asides from drug resistance and commercially present a high cost of treatment that has limited their widespread use. Therefore, validated and sensitive biomarkers and gene expression signatures can monitor response to therapies and predict side effects and tolerability of routine clinical use.
According to the type of anatomical tumor types and in vivo biological parameters, mAb have different pharmacokinetics, which affected their performance and selection for clinical application (52). Considering the safety profile of mAb, advanced biotechnological tools have developed single-chain antibody fragment (scFv)–based anti-VEGF antibodies or Fab-based agents such as Aflibercept and Conbercept that combine the Fc portion of a complete mAb to two highest affinity domains of VEGFR1 and VEGFR within high affinity for VEGF isoforms (52). Moreover, nanotechnological tools encapsulating of BioDrugs are being developed to extend their half-life in vivo or combine them with other therapeutic agents with different mechanisms of action to reduce the frequency and dose of intravitreal injections and minimize undesired systemic exposure, preserved mAb bioactivity, and controlled drug release in tumor sites (53).
4 Normalizing tumor vasculature improves cancer immunotherapy
The limitation of complete vascular occlusion suggests that restoring some degree of vascular perfusion capacity could increase the penetration of therapeutic cells into the tumor and improve the delivery of chemotherapeutics or provide sufficient oxygen to enhance the efficacy of radioactive agents. Considering the short and revisable normalization window and the dose-dependent effect, the low dose of antiangiogenic agents can provide efficient and durable vascular normalization (Figure 1B). When anti-VEGF treatment is used at low doses, blood vessel function is improved by reorganizing the pericyte lining to reduce vascular permeability and hypoxia (40). Prolonging the normalization window also improves the antitumor immune responses by increasing effector T-cell infiltration (54), ECM remodeling (55), and reprogramming of immunosuppressive TME to enhance the therapeutic efficacy of cancer immunotherapies (39, 54), including immune checkpoint blockers (ICBs) by blocking immune checkpoints, programmed death-ligand 1 (PD-L1), and PD-L2 in tumor cells and cytotoxic T-lymphocyte–associated protein 4 (CTLA-4) and programmed cell death protein 1 (PD-1) expressed on T cells (56). Interestingly, depletion of ICBs and Tregs promotes vascular normalization by reducing tumor vascular density and reactivating IFNγ producers (i.e., CD8+/CD4+ effector T cells), which IFNγ has the potential to limit tumor angiogenesis (54). In addition to immune suppression, Tregs expressing VEGFRs, such as neurophilin-1 mediated angiogenesis and anti-VEGF mAb, can lead to Tregs depletion and enhance the immunologically beneficial effects in the TME. VEGF also suppresses DC maturation and upregulates the expression of PD-L1 on DC and PD-1 and CTLA-4 on T cells, leading to T-cell exhaustion and suppression of their function, resulting in immunosuppression (57) (Figure 1A). Therefore, targeting VEGF/VEGFR-enhanced anti-tumor immunity and tumor cell elimination.
Another strategy to induce vascular normalization is in situ delivery of angiostatic factors such as tumor necrosis factor alpha (TNFα), which at low doses can stabilize the tumor vasculature and improve vascular permeability (58). Moreover, proangiogenic signaling induced by angiopoietin 2 (ANG2)/TIE2 cytokines contributes to vascular VEGF-dependent angiogenesis and increases microvessel density of the TME as well as vascular permeability, and thus, dual targeting of ANG2 and TIE2 or ANG2 and VEGF extends both the window of normalization and reduces metastatic dissemination in patients with glioblastoma compared with VEGF or ANG2 inhibition alone (39, 59). Depending on the cell source, IFNγ produced by T cells limits the tumor angiogenesis (54), while IFNγ-expressing ECs upregulate PD-L1 in tumor cells and limit antitumor immunity, which guided that multi-targeting of ANG2, VEGF, and PD-L1 enhanced antitumor responses in transplanted tumor models (57, 60). Like IFNγ, the anti–VEGF-ANG2 antibody upregulates immune inhibitory molecules such as PD-L1 on both ECs and tumor cells to promote T-cell exhaustion, highlighting the benefit of co-treatment with anti–PD-1 antibody (61). Tumor type also determines the benefits of dual VEGF-ANG2 inhibition that in lymphoid and myeloid cell populations infiltration of CD8+/CD4+ effector T cells and reprogramming of M2-like TAM to M1-like one with an anticancer phenotype, respectively, contribute to the therapeutic efficacy of dual antiangiogenic therapy (8, 39, 59).
It requires accumulation of CD8+ effector T cells in tumor sites before exerting its antitumor activity. In non-inflamed TME and highly angiogenic tumor state, bFGF/VEGF activities influence ECs toward decreased expression of endothelial adhesion molecules (EAMs), including E-selectin, ICAM1, and VCAM1, thus hindering leukocyte homing, extravasation, and infiltration into tumor tissue (62). Thus, the combination of antiangiogenic agents and immunotherapies switch cold TME to hot one, which improves the infiltration and functions of T cells in tumors (such as metastatic RCC and breast cancer) (Figure 1C), especially with a high incidence of high endothelial venules-expressing ICAM1 and VCAM1 to overcome the physical barrier for circulating T cells (41). Preclinical studies have shown that administration of low doses of an anti-VEGFR2 antibody increases CTL infiltration and thus improves the anticancer efficacy of subsequent therapies by tumor vaccination or ACT approaches (56, 63). In terms of cell therapy, nanobody (VHH)-based CAR T cells were engineered to target VEGFR2-expressing tumor cells and were considered as a candidate for ACT-based immunotherapy of solid tumors (64). Like PD-L1, tumor cells and tumor-associated ECs overexpress a protein called galectin 1, which induces apoptosis and exhaustion in resting T cells, limiting their infiltration and function (65). Therefore, the anti-tumor immune activity targeting galectin 1 synergized with anti-PD-L1 antibodies. Combinatorial therapy also reprograms the TME through oxygenation and reduction of hypoxia, tissue perfusion, and interstitial fluid pressure, complementing existing standard therapies. Overall, combinations of antiangiogenic agents and immunotherapy (ICBs, vaccine, and ACT) provide an update on the current clinical immunotherapies and currently being tested with encouraging results or ongoing, and some of them, especially combinations of antiangiogenic agents and ICBs approved in the clinic (Table 2).
Although combinations of anti-angiogenic and cancer vaccines have shown promising results in preclinical models, these combinations have not yet been recapitulated in humans (88). Prior to clinical application, identification of dose-limiting toxicities and maximum tolerated doses of the drug combination are important considerations and highlight the importance of identifying potential biomarkers that can predict effective doses of treatment responses.
5 Nanomaterials improve antiangiogenic cancer immunotherapy
ICBs are known to cause immune-related adverse events, some of which are severe. Although vascular normalization could improve the efficacy of immune checkpoint inhibitors and overcome resistance to cancer immunotherapy (61), this companion yet occurs transiently (Figure 2A) and resulted in acute toxicity grading including hematological and liver function abnormalities (92). Therefore, neoantigen load of tumors in cancer vaccine or delivery of ICBs or antiangiogenic agents within nano platforms or production of nano-sized agents hope to overcome a barrier to effective immunotherapy and elicit the most potent antitumor responses. In addition, passive and active targeted nanomaterials have reduced the frequency of intravitreal injections. It minimizes unwanted systemic exposure by enhancing the binding of NPs to tumor cells, reducing their non-specific uptake, provided passive accumulation of drugs in tumor tissue is called the EPR effect (93) (Figure 2).
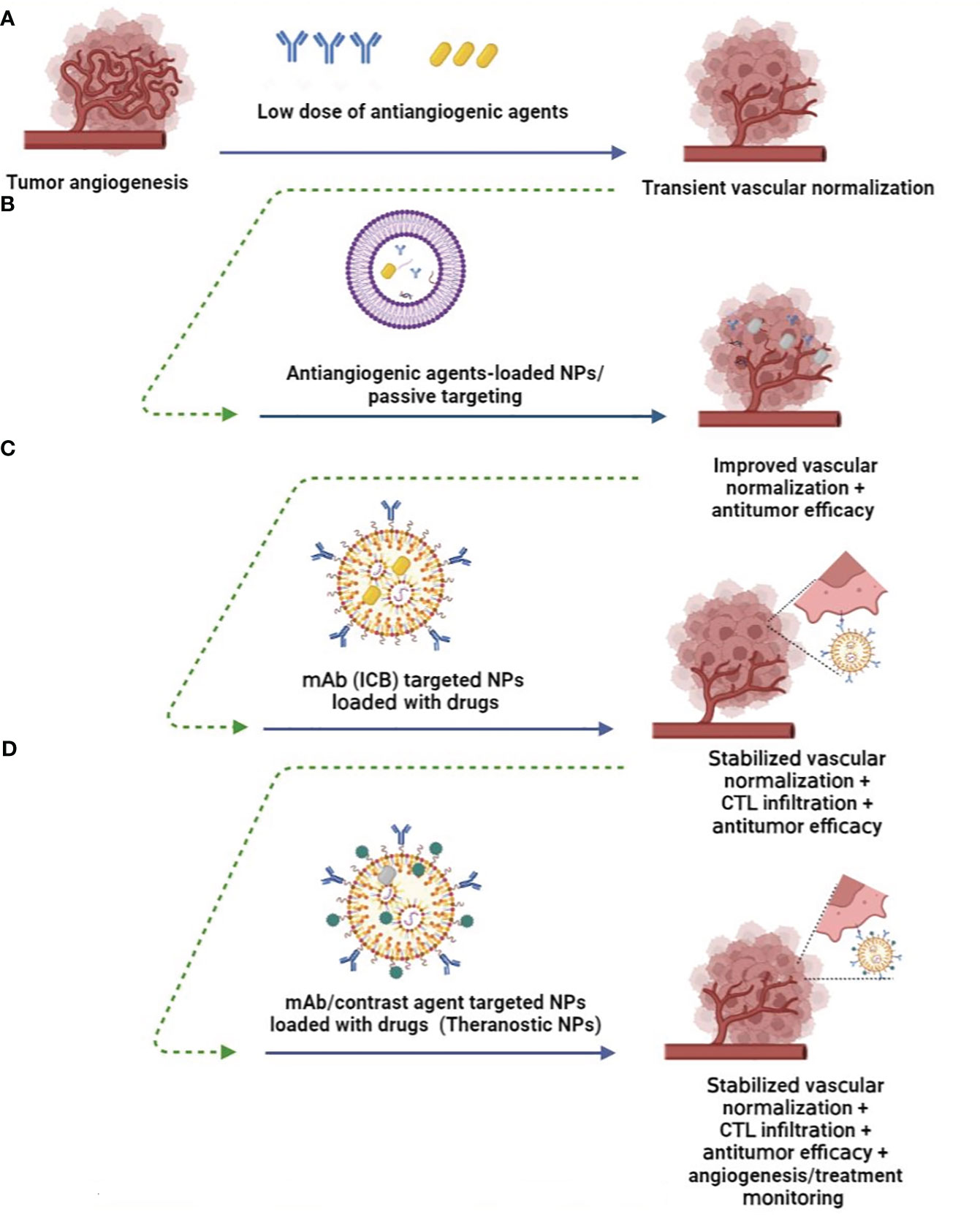
Figure 2 Nanomedicine improves angiogenesis-based cancer therapy. (A) Low doses of antiangiogenic agents induce transient normalization and reduce hypoxic condition (9). (B) Nanoparticles (NPs) loaded with lower doses of anti-angiogenic agents enhance their delivery while protecting them in vivo, provide passive targeting, and promote the EPR effect while promoting vascular normalization (89). (C) Therapeutic ligand-targeted NPs (e.g., mAb) deliver drugs to specific tumor cells, prolong vascular normalization, and induce antitumor immune responses through CTL infiltration or reprogramming of the immunosuppressive TME (90). (D) mAb-targeted theranostic NPs provide antitumor efficacy, while conjugated imaging probes enable angiogenesis visualization, monitoring of therapies, and determining the optimal dosage of therapeutic agents in vivo for clinical setting of vascular normalization and tumor eradication (91).
Anti-angiogenic nanoagents inhibit neoangiogenesis directly and indirectly by targeting ECs and signaling pathways that support tumor angiogenesis (Figure 2B). Recombinant human endostatin (Endostar/rhES) mediates vascular disruption by targeting VEGF-induced angiogenesis and has been used in the clinical setting to treat non-small-cell lung cancer. To improve in vivo efficacy and reduce dosing frequency, PEGylated gold (Au)NPs loaded with rhES were developed. Results indicate that rhES-AuNPs-PEG reduced vascular permeability and improved vascular normalization. Subsequently, this nanosystem improved the intratumoral delivery and antitumor efficacy of cytotoxic 5-FU in H22 tumor-bearing mice (94). Folic acid-modified Au NPs induced tumor vessel normalization by upregulating EC-VE-cadherin, which mediates tight junctions between pericytes. This was followed by improved vascular perfusion and accumulation of CD3+ CD8+ T cells in the TME (95). Taking into account the specific pH, temperature and enzymes formed by TME, some TME-responsive nanomaterials have been developed. For example, pH-sensitive delivery system selenium (Se) NPs loaded with anti-VEGF siRNA significantly accumulated in the tumor region and reduced tumor growth in vivo mediated by VEGF gene silencing (96).
The surface of NPs could be modified by targeting ligands (e.g., peptides, antibodies, and aptamers) to increase the accumulation of NPs at the tumor site and provide more efficient targeted therapy (Figure 2C). For example, APTEDB(fibronectin extra domain B) peptide-conjugated PEG-PLA (polylactide) NPs loaded with paclitaxel can target fibronectin on both neovascular and glioma cells to exert its antiangiogenic therapeutic effect and inhibition of tube formation by ECs, while inducing antitumor activity by delivered paclitaxel (97).
However, due to the complexity of tumor angiogenesis, targeting only a single factor may lead to drug resistance, which could be addressed by targeting NPs to deliver multiple agents. PEGylated Ag2S quantum dots (QDs)-NPs surface modified by cRGD peptide and loaded with an antiangiogenic agent (TNP-470) and doxorubicin (DOX), T&D@RGD-Ag2S, showed long-lasting blood circulation and highly specific vascular binding, resulting in accumulation at the tumor site and inhibited angiogenesis and tumor growth in a human glioma xenograft model (98). NPs can also realize the combined treatment of antiangiogenic agents with photothermal therapy (PDT). For example, dual-functional liposomes containing near-infrared (NIR) dye (IR780) and sunitinib inhibited angiogenesis-mediated tumor growth in vivo after sunitinib was released from NPs by laser irradiation, and further, this platform induced tumor cell killing upon PDT mediated by IR780-loaded liposomes (99). Biocompatibility that reduces toxicity is a key biological requirement for developed NPs, which could be achieved by biosynthesizing NPs or using natural biomolecules or cells as biomimetic drug delivery systems. For example, RBCs were decorated with RGD peptide and then co-loaded with DOX and NIR dye indocyanine green bound to bovine serum albumin, IB&D@RBC-RGD, which demonstrated the synergistic therapeutic effect of combined PPT and chemotherapy, thus showing significant antitumor activity (100).
With the combination of anti-angiogenic agents and immunotherapy, NPs can also restore tumor vasculature to promote the infiltration of anti-tumor immune cells into the immunosuppressive TME and reduce immune-related side effects caused by ICB (Figure 2B). Micellar NPs encapsulated with sunitinib in the combination of lipid-coated calcium phosphate (LCP) NPs containing tyrosinase-related protein 2 (Trp2) peptide and CpG ODN, LCP-Trp2 vaccine, increased CTL infiltration and decreased the number and percentage of MDSCs and Tregs in the TME of an advanced murine melanoma model (90). Nitric oxide (NO)–based NPs provide sustained NO release in TME and HCC that reprogrammed the immunosuppressive TME, as evidenced by downregulation of PD-L1 expression and inhibition of M1 macrophage polarization to M2 phenotype, all of which improved the therapeutic effects of cancer vaccines (101). NPs decorated with anti-PD-L1 and 4-1BB antibodies simultaneously blocked PD-L1 pathway-mediated CTL apoptosis and reactivated them by inducing the 4-1BB co-stimulatory pathway in CTLs (102). Since tumor ECs express 4-1BB, these immunoswitch NPs can stimulate ECs to express adhesion molecules (i.e., ICAM-1, VCAM-1, and E-selectin) required for CTL infiltration (103). Combination therapy of engineered platelets loaded with anti-PDL1 mAb (P@aPDL1) and vadimezan, as an inducer of local tumor bleeding, led to the development of an off-the-shelf cell therapy and biomimetic carrier that recruits platelets to the hemorrhagic tumor site and locally enhances the accumulation and release of aPDL1 to elicit T-cell–based immunotherapy in a metastatic breast tumor model (104). Recently, a combination of radiotherapy, liposomal irinotecan with radiosensitizing properties, apatinib (TKI targeting VEGFR-2) and PD-1 antibody was evaluated in a phase II clinical trial that enrolled patients with advanced solid tumors and showed promising tolerability and anti-tumor activity (Clinical Trial ID: NCT04569916). Taken together, the application of multifunctional nanocarriers for delivery of anti-angiogenic agents in combination with approved immunotherapies may induce lower interstitial fluid pressure, increased intratumoral oxygenation, reduced drug dosage and resistance, and increased accumulation of BioDrugs or promoted trafficking of immune cells within the tumor site.
Finally, it is noteworthy that nanomaterials carrying diagnostic or imaging agents also allow non-invasive visualization of tumor angiogenesis, monitoring of response to anti-angiogenic therapies and, more importantly, determining the optimal dosage of therapeutic agents (105) (Figure 2D). Targeting VEGFR and ECs has been proposed for targeted imaging of tumor angiogenesis using CT, PET, SPECT, MRI, optical imaging, and ultrasound imaging. However, these imaging techniques present too low resolution for microvascular imaging, which could be improved by nano-sized agents or NPs containing low-dose antiangiogenic drug-mediated tumor vessel, tissue on, and tissue perfusion and oxygenation. In addition, an RGD-functionalized nanocarrier has been used as a contrast agent that allows the detection of ongoing angiogenesis. For example, cyclized asparagine-glycine-arginine peptide (cNGR) peptide surface functionalized of Au NPs targets aminopeptidase-N on the endothelium and provides imaging using computed tomography (CT) in 4T1 xenograft model (91). Considering the role of MMPs in ECM degradation and angiogenesis promotion, Ryu et al. developed MMP-responsive nanoprobes decorated with nanoparticulted human serum albumin that exhibited prolonged circulation half-life and enhanced fluorescence for optical imaging emission during angiogenesis in a mouse hindlimb ischemia model (106). Biomedical NPs such as carbon-derived quantum dots are other promising candidates that have been used for molecular imaging of tumor angiogenesis in vivo (93).
Theranostic NPs (e.g., mesoporous silica NPs and Au NPs) could act as anti-angiogenesis agents and tumor vascular normalizers even in a drug-free manner, which subsequently this tumor vascular normalization improves the delivery efficiency of nanotheranostic agents. Moreover, the fluorescence recovery after the release of DOX (a chemo drug with fluorescent property) from the liposomal formulation can be used as an indicator to monitor the chemotherapeutic progress (107). Nanosized iron oxide NPs with their intrinsic imaging capabilities are exquisitely suitable and even approved (e.g., ferumoxsil) for theranostic applications. pH-degradable bovine serum albumin-functionalized Fe3O4 NPs and loaded with lenvatinib was able to induce vessel normalization and modulate immunosuppressive TME of HCC while Fe3O4 provided in vivo visualization tracking by magnetic resonance imaging (MRI) and also magnetic particle imaging (108). Combined, nanotechnology has and will continue to maximize the clinical benefits of existing therapies targeting tumor angiogenesis. Although NPs are potent, their intrinsic efficacy must be optimized by determining their various parameters, including safety, size, shape, wettability, charge, and effective concentration (53). In summary, therapeutic agent NPs with the function of vascular normalization inducers to achieve specific targeting of solid tumors while exerting efficient antitumor effects in vivo.
6 Conclusion
Here, we summarize the recent developments in integrated therapies of antiangiogenic agents, immunotherapy, and nanotechnology for tumor angiogenesis. An in-depth understanding of the angiogenesis processes, selective biomarkers, and immunoregulatory factors involved in the TME of solid cancer tumorigenesis, especially in metastatic tumors, may allow the development and application of efficient cancer therapies in the future. To date, the vast majority of vascular remodeling drugs or contrast agents used in routine monitoring are administered systemically, causing off-target effects in healthy tissues. The development of surface-modified nanosystems has provided more robust and durable normalization strategies to widen the therapeutic window while allowing monitoring of therapies and changes in the TME vasculature. In addition, nanotherapeutics address the challenges of timing and dosing of vascular remodeling agents or combinatorial therapies. They could become part of the treatment approach for advanced solid tumors.
Author contributions
HD-M: Conceptualization, Investigation, writing – original/revised draft, Writing - review & editing, Visualization, Supervision, and Project administration. RN, MKe, and MA: Investigation, writing – original/revised draft. RS and MKa: Investigation, Writing - review & editing. All authors have read and agreed to the published version of the manuscript.
Funding
This work was financially supported by Isfahan University of Medical Science, Isfahan, Iran (Scientific Code: 2402102 and Approval ID: IR.ARI.MUI.REC.1402.134).
Acknowledgments
This study was morally supported by Pediatric Inherited Diseases Research Center, Isfahan University of Medical Sciences, Isfahan, Iran.
Conflict of interest
The authors declare that the research was conducted in the absence of any commercial or financial relationships that could be construed as a potential conflict of interest.
Publisher’s note
All claims expressed in this article are solely those of the authors and do not necessarily represent those of their affiliated organizations, or those of the publisher, the editors and the reviewers. Any product that may be evaluated in this article, or claim that may be made by its manufacturer, is not guaranteed or endorsed by the publisher.
References
1. Blouw B, Song H, Tihan T, Bosze J, Ferrara N, Gerber H-P, et al. The hypoxic response of tumors is dependent on their microenvironment. Cancer Cell (2003) 4(2):133–46. doi: 10.1016/S1535-6108(03)00194-6
2. De Bock K, Cauwenberghs S, Carmeliet P. Vessel abnorMalization: another hallmark of cancer?: molecular mechanisms and therapeutic implications. Curr Opin Genet Dev (2011) 21(1):73–9. doi: 10.1016/j.gde.2010.10.008
3. De Palma M, Biziato D, Petrova TV. Microenvironmental regulation of tumour angiogenesis. Nat Rev Cancer (2017) 17(8):457–74. doi: 10.1038/nrc.2017.51
4. Yang J, Yan J, Liu B. Targeting VEGF/VEGFR to modulate antitumor immunity. Front Immunol (2018) 9:978. doi: 10.3389/fimmu.2018.00978
5. Raphael J, Chan K, Karim S, Kerbel R, Lam H, delos Santos K, et al. Antiangiogenic therapy in advanced non–small-cell lung cancer: a meta-analysis of phase III randomized trials. Clin Lung cancer (2017) 18(4):345–53.e5. doi: 10.1016/j.cllc.2017.01.004
6. Dianat-Moghadam H, Mahari A, Salahlou R, Khalili M, Azizi M, Sadeghzadeh H. Immune evader cancer stem cells direct the perspective approaches to cancer immunotherapy. Stem Cell Res Ther (2022) 13(1):1–12. doi: 10.1186/s13287-022-02829-9
7. Marofi F, Achmad H, Bokov D, Abdelbasset WK, Alsadoon Z, Chupradit S, et al. Hurdles to breakthrough in CAR T cell therapy of solid tumors. Stem Cell Res Ther (2022) 13(1):1–19. doi: 10.1186/s13287-022-02819-x
8. Fukumura D, Kloepper J, Amoozgar Z, Duda DG, Jain RK. Enhancing cancer immunotherapy using antiangiogenics: opportunities and challenges. Nat Rev Clin Oncol (2018) 15(5):325–40. doi: 10.1038/nrclinonc.2018.29
9. Jain RK. NorMalizing tumor microenvironment to treat cancer: bench to bedside to biomarkers. J Clin Oncol (2013) 31(17):2205. doi: 10.1200/JCO.2012.46.3653
10. Mahase S, Rattenni RN, Wesseling P, Leenders W, Baldotto C, Jain R, et al. Hypoxia-mediated mechanisms associated with antiangiogenic treatment resistance in glioblastomas. Am J pathol (2017) 187(5):940–53. doi: 10.1016/j.ajpath.2017.01.010
11. Kerbel RS. Antiangiogenic therapy: a universal chemosensitization strategy for cancer? Science (2006) 312(5777):1171–5. doi: 10.1126/science.1125950
12. Lee C-G, Heijn M, di Tomaso E, Griffon-Etienne G, Ancukiewicz M, Koike C, et al. Anti-vascular endothelial growth factor treatment augments tumor radiation response under normoxic or hypoxic conditions. Cancer Res (2000) 60(19):5565–70.
13. Garcia J, Hurwitz HI, Sandler AB, Miles D, Coleman RL, Deurloo R, et al. Bevacizumab (Avastin®) in cancer treatment: A review of 15 years of clinical experience and future outlook. Cancer Treat Rev (2020) 86:102017. doi: 10.1016/j.ctrv.2020.102017
14. Tiwari P. Ramucirumab: boon or bane. J Egyptian Natl Cancer Institute (2016) 28(3):133–40. doi: 10.1016/j.jnci.2016.03.001
15. Heinemann V, von Weikersthal LF, Decker T, Kiani A, Vehling-Kaiser U, Al-Batran S-E, et al. FOLFIRI plus cetuximab versus FOLFIRI plus bevacizumab as first-line treatment for patients with metastatic colorectal cancer (FIRE-3): a randomised, open-label, phase 3 trial. Lancet Oncol (2014) 15(10):1065–75. doi: 10.1016/S1470-2045(14)70330-4
16. Price TJ, Peeters M, Kim TW, Li J, Cascinu S, Ruff P, et al. Panitumumab versus cetuximab in patients with chemotherapy-refractory wild-type KRAS exon 2 metastatic colorectal cancer (ASPECCT): a randomised, multicentre, open-label, non-inferiority phase 3 study. Lancet Oncol (2014) 15(6):569–79. doi: 10.1016/S1470-2045(14)70118-4
17. Mahmoudi R, Dianat-Moghadam H, Poorebrahim M, Siapoush S, Poortahmasebi V, Salahlou R, et al. Recombinant immunotoxins development for HER2-based targeted cancer therapies. Cancer Cell Int (2021) 21:1–17. doi: 10.1186/s12935-021-02182-6
18. Breitkreutz I, Anderson KC. Thalidomide in multiple myeloma–clinical trials and aspects of drug metabolism and toxicity. Expert Opin Drug Metab Toxicol (2008) 4(7):973–85. doi: 10.1517/17425255.4.7.973
19. Pulte ED, Dmytrijuk A, Nie L, Goldberg KB, McKee AE, Farrell AT, et al. FDA approval summary: lenalidomide as maintenance therapy after autologous stem cell transplant in newly diagnosed multiple myeloma. Oncologist (2018) 23(6):734–9. doi: 10.1634/theoncologist.2017-0440
20. Thatcher N, Hirsch FR, Luft AV, Szczesna A, Ciuleanu TE, Dediu M, et al. Necitumumab plus gemcitabine and cisplatin versus gemcitabine and cisplatin alone as first-line therapy in patients with stage IV squamous non-small-cell lung cancer (SQUIRE): an open-label, randomised, controlled phase 3 trial. Lancet Oncol (2015) 16(7):763–74. doi: 10.1016/S1470-2045(15)00021-2
21. Chang E, Weinstock C, Zhang L, Fiero MH, Zhao M, Zahalka E, et al. FDA approval summary: tivozanib for relapsed or refractory renal cell carcinoma. Clin Cancer Res (2022) 28(3):441–5. doi: 10.1158/1078-0432.CCR-21-2334
22. Goodman VL, Rock EP, Dagher R, Ramchandani RP, Abraham S, Gobburu JV, et al. Approval summary: sunitinib for the treatment of imatinib refractory or intolerant gastrointestinal stromal tumors and advanced renal cell carcinoma. Clin Cancer Res (2007) 13(5):1367–73. doi: 10.1158/1078-0432.CCR-06-2328
23. Carlisle BG, Zheng T, Kimmelman J. Imatinib and the long tail of targeted drug development. Nat Rev Clin Oncol (2020) 17(1):1–3. doi: 10.1038/s41571-019-0287-0
24. Bukowski RM, Yasothan U, Kirkpatrick P. Pazopanib. Nat Rev Drug Discov (2010) 9(1):17–8. doi: 10.1038/nrd3073
25. Kim ES, Hirsh V, Mok T, Socinski MA, Gervais R, Wu Y-L, et al. Gefitinib versus docetaxel in previously treated non-small-cell lung cancer (INTEREST): a randomised phase III trial. Lancet (2008) 372(9652):1809–18. doi: 10.1016/S0140-6736(08)61758-4
26. Martin M, Holmes FA, Ejlertsen B, Delaloge S, Moy B, Iwata H, et al. Neratinib after trastuzumab-based adjuvant therapy in HER2-positive breast cancer (ExteNET): 5-year analysis of a randomised, double-blind, placebo-controlled, phase 3 trial. Lancet Oncol (2017) 18(12):1688–700. doi: 10.1016/S1470-2045(17)30717-9
27. Baselga J, Bradbury I, Eidtmann H, Di Cosimo S, De Azambuja E, Aura C, et al. Lapatinib with trastuzumab for HER2-positive early breast cancer (NeoALTTO): a randomised, open-label, multicentre, phase 3 trial. Lancet (2012) 379(9816):633–40. doi: 10.1016/S0140-6736(11)61847-3
28. Sequist LV, Yang JC-H, Yamamoto N, O'Byrne K, Hirsh V, Mok T, et al. Phase III study of afatinib or cisplatin plus pemetrexed in patients with metastatic lung adenocarcinoma with EGFR mutations. J Clin Oncol (2013) 31(27):3327–34. doi: 10.1200/JCO.2012.44.2806
29. Moore MJ, Goldstein D, Hamm J, Figer A, Hecht JR, Gallinger S, et al. Erlotinib plus gemcitabine compared with gemcitabine alone in patients with advanced pancreatic cancer: a phase III trial of the National Cancer Institute of Canada Clinical Trials Group. J Clin Oncol (2007) 25(15):1960–6. doi: 10.1200/JCO.2006.07.9525
30. de Gramont A, Van Cutsem E, Schmoll H-J, Tabernero J, Clarke S, Moore MJ, et al. Bevacizumab plus oxaliplatin-based chemotherapy as adjuvant treatment for colon cancer (AVANT): a phase 3 randomised controlled trial. Lancet Oncol (2012) 13(12):1225–33. doi: 10.1016/S1470-2045(12)70509-0
31. Bonner JA, Harari PM, Giralt J, Azarnia N, Shin DM, Cohen RB, et al. Radiotherapy plus cetuximab for squamous-cell carcinoma of the head and neck. New Engl J Med (2006) 354(6):567–78. doi: 10.1056/NEJMoa053422
32. Hurwitz H, Fehrenbacher L, Novotny W, Cartwright T, Hainsworth J, Heim W, et al. Bevacizumab plus irinotecan, fluorouracil, and leucovorin for metastatic colorectal cancer. New Engl J Med (2004) 350(23):2335–42. doi: 10.1056/NEJMoa032691
33. Stintzing S, Miller-Phillips L, Modest D, von Weikersthal LF, Decker T, Kiani A, et al. Impact of BRAF and RAS mutations on first-line efficacy of FOLFIRI plus cetuximab versus FOLFIRI plus bevacizumab: analysis of the FIRE-3 (AIO KRK-0306) study. Eur J Cancer (2017) 79:50–60. doi: 10.1016/j.ejca.2017.03.023
34. Dudley AC, Griffioen AW. Pathological angiogenesis: mechanisms and therapeutic strategies. Angiogenesis (2023) 26(3):1–35. doi: 10.1007/s10456-023-09876-7
35. Dianat-Moghadam H, Teimoori-Toolabi L. Implications of fibroblast growth factors (FGFs) in cancer: from prognostic to therapeutic applications. Curr Drug targets (2019) 20(8):852–70. doi: 10.2174/1389450120666190112145409
36. Guo P, Hu B, Gu W, Xu L, Wang D, Huang H-JS, et al. Platelet-derived growth factor-B enhances glioma angiogenesis by stimulating vascular endothelial growth factor expression in tumor endothelia and by promoting pericyte recruitment. Am J pathol (2003) 162(4):1083–93. doi: 10.1016/S0002-9440(10)63905-3
37. Kiss EA, Saharinen P. Anti-angiogenic targets: angiopoietin and angiopoietin receptors. Tumor angiogenesis: key target Cancer Ther (2019) 227–50. doi: 10.1007/978-3-319-33673-2_4
38. Amoozgar Z, Kloepper J, Ren J, Tay RE, Kazer SW, Kiner E, et al. Targeting Treg cells with GITR activation alleviates resistance to immunotherapy in murine glioblastomas. Nat Commun (2021) 12(1):2582. doi: 10.1038/s41467-021-22885-8
39. Kloepper J, Riedemann L, Amoozgar Z, Seano G, Susek K, Yu V, et al. Ang-2/VEGF bispecific antibody reprograms macrophages and resident microglia to anti-tumor phenotype and prolongs glioblastoma survival. Proc Natl Acad Sci (2016) 113(16):4476–81. doi: 10.1073/pnas.1525360113
40. Sitohy B, Nagy JA, Jaminet S-CS, Dvorak HF. Tumor-surrogate blood vessel subtypes exhibit differential susceptibility to anti-VEGF therapyAflibercept (VEGF trap)-resistant tumor-surrogate blood vessels. Cancer Res (2011) 71(22):7021–8. doi: 10.1158/0008-5472.CAN-11-1693
41. Zhang Q, Wu S. Tertiary lymphoid structures are critical for cancer prognosis and therapeutic response. Front Immunol (2022) 13. doi: 10.3389/fimmu.2022.1063711
42. Moccia F, Zuccolo E, Poletto V, Cinelli M, Bonetti E, Guerra G, et al. Endothelial progenitor cells support tumour growth and metastatisation: implications for the resistance to anti-angiogenic therapy. Tumor Biol (2015) 36:6603–14. doi: 10.1007/s13277-015-3823-2
43. Fraineau S, Palii CG, McNeill B, Ritso M, Shelley WC, Prasain N, et al. Epigenetic activation of pro-angiogenic signaling pathways in human endothelial progenitors increases vasculogenesis. Stem Cell Rep (2017) 9(5):1573–87. doi: 10.1016/j.stemcr.2017.09.009
44. Rivera LB, Meyronet D, Hervieu V, Frederick MJ, Bergsland E, Bergers G. Intratumoral myeloid cells regulate responsiveness and resistance to antiangiogenic therapy. Cell Rep (2015) 11(4):577–91. doi: 10.1016/j.celrep.2015.03.055
45. Wang FT, Sun W, Zhang JT, Fan YZ. Cancer−associated fibroblast regulation of tumor neo−angiogenesis as a therapeutic target in cancer. Oncol letters (2019) 17(3):3055–65. doi: 10.3892/ol.2019.9973
46. Conley SJ, Gheordunescu E, Kakarala P, Newman B, Korkaya H, Heath AN, et al. Antiangiogenic agents increase breast cancer stem cells via the generation of tumor hypoxia. Proc Natl Acad Sci (2012) 109(8):2784–9. doi: 10.1073/pnas.1018866109
47. Easwaran H, Tsai H-C, Baylin SB. Cancer epigenetics: tumor heterogeneity, plasticity of stem-like states, and drug resistance. Mol Cell (2014) 54(5):716–27. doi: 10.1016/j.molcel.2014.05.015
48. Angara K, Borin TF, Arbab AS. Vascular mimicry: a novel neovascularization mechanism driving anti-angiogenic therapy (AAT) resistance in glioblastoma. Trans Oncol (2017) 10(4):650–60. doi: 10.1016/j.tranon.2017.04.007
49. Loizzi V, Del Vecchio V, Gargano G, De Liso M, Kardashi A, Naglieri E, et al. Biological pathways involved in tumor angiogenesis and bevacizumab based anti-angiogenic therapy with special references to ovarian cancer. Int J Mol Sci (2017) 18(9):1967. doi: 10.3390/ijms18091967
50. Fogli S, Del Re M, Rofi E, Posarelli C, Figus M, Danesi R. Clinical pharmacology of intravitreal anti-VEGF drugs. Eye (2018) 32(6):1010–20. doi: 10.1038/s41433-018-0021-7
51. Zhao S, Wang P, Yin F, Wu J, Wang Y, Li P, et al. Cardiovascular toxicity associated with the multitargeted tyrosine kinase inhibitor anlotinib. Tumori J (2023) 109(2):186–96. doi: 10.1177/03008916221084362
52. Formica ML, Awde Alfonso HG, Palma SD. Biological drug therapy for ocular angiogenesis: Anti-VEGF agents and novel strategies based on nanotechnology. Pharmacol Res Perspectives (2021) 9(2):e00723. doi: 10.1002/prp2.723
53. Shen R, Peng L, Zhou W, Wang D, Jiang Q, Ji J, et al. Anti-angiogenic nano-delivery system promotes tumor vascular norMalizing and micro-environment reprogramming in solid tumor. J Controlled Release (2022) 349:550–64. doi: 10.1016/j.jconrel.2022.07.015
54. Khan KA, Kerbel RS. Improving immunotherapy outcomes with anti-angiogenic treatments and vice versa. Nat Rev Clin Oncol (2018) 15(5):310–24. doi: 10.1038/nrclinonc.2018.9
55. Rahbari NN, Kedrin D, Incio J, Liu H, Ho WW, Nia HT, et al. Anti-VEGF therapy induces ECM remodeling and mechanical barriers to therapy in colorectal cancer liver metastases. Sci Trans Med (2016) 8(360):360ra135–360ra135. doi: 10.1126/scitranslmed.aaf5219
56. Huang Y, Yuan J, Righi E, Kamoun WS, Ancukiewicz M, Nezivar J, et al. Vascular norMalizing doses of antiangiogenic treatment reprogram the immunosuppressive tumor microenvironment and enhance immunotherapy. Proc Natl Acad Sci (2012) 109(43):17561–6. doi: 10.1073/pnas.1215397109
57. Amoozgar Z, Ren J, Wang N, Andersson P, Ferraro G, Rajan S, et al. Combined blockade of VEGF, Angiopoietin-2, and PD1 reprograms glioblastoma endothelial cells into quasi-antigen-presenting cells. bioRxiv (2022) 2022:09. doi: 10.1101/2022.09.03.506476
58. Johansson A, Hamzah J, Payne CJ, Ganss R. Tumor-targeted TNFα stabilizes tumor vessels and enhances active immunotherapy. Proc Natl Acad Sci (2012) 109(20):7841–6. doi: 10.1073/pnas.1118296109
59. Peterson TE, Kirkpatrick ND, Huang Y, Farrar CT, Marijt KA, Kloepper J, et al. Dual inhibition of Ang-2 and VEGF receptors norMalizes tumor vasculature and prolongs survival in glioblastoma by altering macrophages. Proc Natl Acad Sci (2016) 113(16):4470–5. doi: 10.1073/pnas.1525349113
60. Schmittnaegel M, Rigamonti N, Kadioglu E, Cassará A, Wyser Rmili C, Kiialainen A, et al. Dual angiopoietin-2 and VEGFA inhibition elicits antitumor immunity that is enhanced by PD-1 checkpoint blockade. Sci Trans Med (2017) 9(385):eaak9670. doi: 10.1126/scitranslmed.aak9670
61. Allen E, Jabouille A, Rivera LB, Lodewijckx I, Missiaen R, Steri V, et al. Combined antiangiogenic and anti–PD-L1 therapy stimulates tumor immunity through HEV formation. Sci Trans Med (2017) 9(385):eaak9679. doi: 10.1126/scitranslmed.aak9679
62. Majewska A, Wilkus K, Brodaczewska K, Kieda C. Endothelial cells as tools to model tissue microenvironment in hypoxia-dependent pathologies. Int J Mol Sci (2021) 22(2):520. doi: 10.3390/ijms22020520
63. Shigeta K, Matsui A, Kikuchi H, Klein S, Mamessier E, Chen IX, et al. Regorafenib combined with PD1 blockade increases CD8 T-cell infiltration by inducing CXCL10 expression in hepatocellular carcinoma. J Immunother Cancer (2020) 8(2):e001435. doi: 10.1136/jitc-2020-001435
64. Hajari Taheri F, Hassani M, Sharifzadeh Z, Behdani M, Arashkia A, Abolhassani M. T cell engineered with a novel nanobody-based chimeric antigen receptor against VEGFR2 as a candidate for tumor immunotherapy. IUBMB Life (2019) 71(9):1259–67. doi: 10.1002/iub.2019
65. Cagnoni AJ, Giribaldi ML, Blidner AG, Cutine AM, Gatto SG, Morales RM, et al. Galectin-1 fosters an immunosuppressive microenvironment in colorectal cancer by reprogramming CD8+ regulatory T cells. Proc Natl Acad Sci (2021) 118(21):e2102950118. doi: 10.1073/pnas.2102950118
66. Socinski MA, Jotte RM, Cappuzzo F, Orlandi F, Stroyakovskiy D, Nogami N, et al. Atezolizumab for first-line treatment of metastatic nonsquamous NSCLC. New Engl J Med (2018) 378(24):2288–301. doi: 10.1056/NEJMoa1716948
67. Rini BI, Plimack ER, Stus V, Gafanov R, Hawkins R, Nosov D, et al. Pembrolizumab plus axitinib versus sunitinib for advanced renal-cell carcinoma. New Engl J Med (2019) 380(12):1116–27. doi: 10.1056/NEJMoa1816714
68. Motzer RJ, Penkov K, Haanen J, Rini B, Albiges L, Campbell MT, et al. Avelumab plus axitinib versus sunitinib for advanced renal-cell carcinoma. New Engl J Med (2019) 380(12):1103–15. doi: 10.1056/NEJMoa1816047
69. Loriot Y, Balar AV, De Wit R, Garcia JA, Grivas P, Matsubara N, et al. Phase III study of first-line pembrolizumab (pembro) plus lenvatinib (len) in patients (pts) with advanced urothelial carcinoma (UC) ineligible for platinum-based chemotherapy: LEAP-011. Am Soc Clin Oncology (2020) TPS597. doi: 10.1200/JCO.2020.38.6_suppl.TPS597
70. Ducreux M, Zhu AX, Cheng A-L, Galle PR, Ikeda M, Nicholas A, et al. IMbrave150: Exploratory analysis to examine the association between treatment response and overall survival (OS) in patients (pts) with unresectable hepatocellular carcinoma (HCC) treated with atezolizumab (atezo)+ bevacizumab (bev) versus sorafenib (sor). Wolters Kluwer Health (2021) 4071. doi: 10.1200/JCO.2021.39.15_suppl.4071
71. Advani S, Kopetz S. Ongoing and future directions in the management of metastatic colorectal cancer: Update on clinical trials. J Surg Oncol (2019) 119(5):642–52. doi: 10.1002/jso.25441
72. Sostelly A, Smart K, Jaminion F, Boetsch C, Mercier F. Leveraging tumor size and time to death from bevacizumab (BEV) historical data to predict overall survival in ovarian cancer patients treated with vanucizumab (VAN). Cancer Res (2018) 78(13_Supplement):1643. doi: 10.1158/1538-7445.AM2018-1643
73. Wu X, Giobbie-Hurder A, Liao X, Lawrence D, McDermott D, Zhou J, et al. VEGF neutralization plus CTLA-4 blockade alters soluble and cellular factors associated with enhancing lymphocyte infiltration and humoral recognition in melanomaIpilimumab plus anti-VEGF augments tumor immune recognition. Cancer Immunol Res (2016) 4(10):858–68. doi: 10.1158/2326-6066.CIR-16-0084
74. Lindskog M, Laurell A, Kjellman A, Melichar B, Niezabitowski J, Maroto P, et al. A randomized phase II study with ilixadencel, a cell-based immune primer, plus sunitinib versus sunitinib alone in synchronous metastatic renal cell carcinoma. Am Soc Clin Oncology (2020), 11. doi: 10.1200/JCO.2020.38.5_suppl.11
75. Amin A, Dudek AZ, Logan TF, Lance RS, Holzbeierlein JM, Knox JJ, et al. Survival with AGS-003, an autologous dendritic cell–based immunotherapy, in combination with sunitinib in unfavorable risk patients with advanced renal cell carcinoma (RCC): phase 2 study results. J Immunother Cancer (2015) 3:1–13. doi: 10.1186/s40425-015-0055-3
76. ShriMali RK, Yu Z, Theoret MR, Chinnasamy D, Restifo NP, Rosenberg SA. Antiangiogenic agents can increase lymphocyte infiltration into tumor and enhance the effectiveness of adoptive immunotherapy of cancerAdoptive cell therapy and antiangiogenesis. Cancer Res (2010) 70(15):6171–80. doi: 10.1158/0008-5472.CAN-10-0153
77. Gabrilovich DI, Ishida T, Nadaf S, Ohm JE, Carbone DP. Antibodies to vascular endothelial growth factor enhance the efficacy of cancer immunotherapy by improving endogenous dendritic cell function. Clin Cancer Res (1999) 5(10):2963–70.
78. Shi S, Wang R, Chen Y, Song H, Chen L, Huang G. Combining antiangiogenic therapy with adoptive cell immunotherapy exerts better antitumor effects in non-small cell lung cancer models. PloS One (2013) 8(6):e65757. doi: 10.1371/journal.pone.0065757
79. Bocca P, Di Carlo E, Caruana I, Emionite L, Cilli M, De Angelis B, et al. Bevacizumab-mediated tumor vasculature remodelling improves tumor infiltration and antitumor efficacy of GD2-CAR T cells in a human neuroblastoma preclinical model. Oncoimmunology (2018) 7(1):e1378843. doi: 10.1080/2162402X.2017.1378843
80. Yin W-m, Li Y-w, Gu Y-q, Luo M. Nanoengineered targeting strategy for cancer immunotherapy. Acta Pharmacologica Sinica (2020) 41(7):902–10. doi: 10.1038/s41401-020-0417-3
81. Bloch O, Shi Q, Anderson SK, Knopp M, Raizer J, Clarke J, et al. ATIM-14. Alliance A071101: A phase II randomized trial comparing the efficacy of heat shock protein peptide complex-96 (HSPPC-96) vaccine given with bevacizumab versus bevacizumab alone in the treatment of surgically resectable recurrent glioblastoma. Neuro-Oncology (2017) 19(suppl_6):vi29–vi. doi: 10.1093/neuonc/nox168.110
82. Autio KA, Higano CS, Nordquist L, Appleman LJ, Zhang T, Zhu X-H, et al. First-in-human, phase 1 study of PF-06753512, a vaccine-based immunotherapy regimen (VBIR), in non-metastatic hormone-sensitive biochemical recurrence and metastatic castration-resistant prostate cancer (mCRPC). J Immunother Cancer (2023) 11(3):e005702. doi: 10.1136/jitc-2022-005702
83. Rini BI, Stenzl A, Zdrojowy R, Kogan M, Shkolnik M, Oudard S, et al. IMA901, a multipeptide cancer vaccine, plus sunitinib versus sunitinib alone, as first-line therapy for advanced or metastatic renal cell carcinoma (IMPRINT): a multicentre, open-label, randomised, controlled, phase 3 trial. Lancet Oncol (2016) 17(11):1599–611. doi: 10.1016/S1470-2045(16)30408-9
84. Bose A, Taylor JL, Alber S, Watkins SC, Garcia JA, Rini BI, et al. Sunitinib facilitates the activation and recruitment of therapeutic anti-tumor immunity in concert with specific vaccination. Int J Cancer (2011) 129(9):2158–70. doi: 10.1002/ijc.25863
85. Renner DN, Malo CS, Jin F, Parney IF, Pavelko KD, Johnson AJ. Improved treatment efficacy of antiangiogenic therapy when combined with picornavirus vaccination in the GL261 glioma model. Neurotherapeutics (2016) 13:226–36. doi: 10.1007/s13311-015-0407-1
86. Li B, Lalani AS, Harding TC, Luan B, Koprivnikar K, Huan Tu G, et al. Vascular endothelial growth factor blockade reduces intratumoral regulatory T cells and enhances the efficacy of a GM-CSF–secreting cancer immunotherapy. Clin Cancer Res (2006) 12(22):6808–16. doi: 10.1158/1078-0432.CCR-06-1558
87. Farsaci B, Higgins JP, Hodge JW. Consequence of dose scheduling of sunitinib on host immune response elements and vaccine combination therapy. Int J Cancer (2012) 130(8):1948–59. doi: 10.1002/ijc.26219
88. Mougel A, Terme M, Tanchot C. Therapeutic cancer vaccine and combinations with antiangiogenic therapies and immune checkpoint blockade. Front Immunol (2019) 10:467. doi: 10.3389/fimmu.2019.00467
89. Liang P, Ballou B, Lv X, Si W, Bruchez MP, Huang W, et al. Monotherapy and combination therapy using anti-angiogenic nanoagents to fight cancer. Advanced Mater (2021) 33(15):2005155. doi: 10.1002/adma.202005155
90. Huo M, Zhao Y, Satterlee AB, Wang Y, Xu Y, Huang L. Tumor-targeted delivery of sunitinib base enhances vaccine therapy for advanced melanoma by remodeling the tumor microenvironment. J Controlled Release (2017) 245:81–94. doi: 10.1016/j.jconrel.2016.11.013
91. Wu M, Zhang Y, Zhang Y, Wu M, Wu M, Wu H, et al. Tumor angiogenesis targeting and imaging using gold nanoparticle probe with directly conjugated cyclic NGR. RSC Advances (2018) 8(3):1706–16. doi: 10.1039/C7RA10155D
92. Matsuda N, Wang X, Lim B, Krishnamurthy S, Alvarez RH, Willey JS, et al. Safety and efficacy of panitumumab plus neoadjuvant chemotherapy in patients with primary HER2-negative inflammatory breast cancer. JAMA Oncol (2018) 4(9):1207–13. doi: 10.1001/jamaoncol.2018.1436
93. Azizi M, Dianat-Moghadam H, Salehi R, Farshbaf M, Iyengar D, Sau S, et al. Interactions between tumor biology and targeted nanoplatforms for imaging applications. Advanced Funct Mater (2020) 30(19):1910402. doi: 10.1002/adfm.201910402
94. Li W, Zhao X, Du B, Li X, Liu S, Yang X-Y, et al. Gold nanoparticle–mediated targeted delivery of recombinant human endostatin norMalizes tumour vasculature and improves cancer therapy. Sci Rep (2016) 6(1):30619. doi: 10.1038/srep30619
95. Huang N, Liu Y, Fang Y, Zheng S, Wu J, Wang M, et al. Gold nanoparticles induce tumor vessel norMalization and impair metastasis by inhibiting endothelial Smad2/3 signaling. ACS Nano (2020) 14(7):7940–58. doi: 10.1021/acsnano.9b08460
96. Yu Q, Liu Y, Cao C, Le F, Qin X, Sun D, et al. The use of pH-sensitive functional selenium nanoparticles shows enhanced in vivo VEGF-siRNA silencing and fluorescence imaging. Nanoscale (2014) 6(15):9279–92. doi: 10.1039/C4NR02423K
97. Gu G, Hu Q, Feng X, Gao X, Menglin J, Kang T, et al. PEG-PLA nanoparticles modified with APTEDB peptide for enhanced anti-angiogenic and anti-glioma therapy. Biomaterials (2014) 35(28):8215–26. doi: 10.1016/j.biomaterials.2014.06.022
98. Song C, Zhang Y, Li C, Chen G, Kang X, Wang Q. Enhanced nanodrug delivery to solid tumors based on a tumor vasculature-targeted strategy. Advanced Funct Mater (2016) 26(23):4192–200. doi: 10.1002/adfm.201600417
99. Yang X, Li H, Qian C, Guo Y, Li C, Gao F, et al. Near-infrared light-activated IR780-loaded liposomes for anti-tumor angiogenesis and Photothermal therapy. Nanomed: Nanotechnol Biol Med (2018) 14(7):2283–94. doi: 10.1016/j.nano.2018.06.011
100. Sun X, Wang C, Gao M, Hu A, Liu Z. Remotely controlled red blood cell carriers for cancer targeting and near-infrared light-triggered drug release in combined photothermal–chemotherapy. Advanced Funct Mater (2015) 25(16):2386–94. doi: 10.1002/adfm.201500061
101. Sung Y-C, Jin P-R, Chu L-A, Hsu F-F, Wang M-R, Chang C-C, et al. Delivery of nitric oxide with a nanocarrier promotes tumour vessel norMalization and potentiates anti-cancer therapies. Nat Nanotechnol (2019) 14(12):1160–9. doi: 10.1038/s41565-019-0570-3
102. Kosmides AK, Sidhom J-W, Fraser A, Bessell CA, Schneck JP. Dual targeting nanoparticle stimulates the immune system to inhibit tumor growth. ACS Nano (2017) 11(6):5417–29. doi: 10.1021/acsnano.6b08152
103. Palazón A, Teijeira A, Martínez-Forero I, Hervás-Stubbs S, Roncal C, Peñuelas I, et al. Agonist anti-CD137 mAb act on tumor endothelial cells to enhance recruitment of activated T lymphocytes. Cancer Res (2011) 71(3):801–11. doi: 10.1158/0008-5472.CAN-10-1733
104. Li H, Wang Z, Chen Z, Ci T, Chen G, Wen D, et al. Disrupting tumour vasculature and recruitment of aPDL1-loaded platelets control tumour metastasis. Nat Commun (2021) 12(1):2773. doi: 10.1038/s41467-021-22674-3
105. Azizi M, Valizadeh H, Shahgolzari M, Talebi M, Baybordi E, Dadpour MR, et al. Synthesis of self-targeted carbon dot with ultrahigh quantum yield for detection and therapy of cancer. ACS Omega (2020) 5(38):24628–38. doi: 10.1021/acsomega.0c03215
106. Ryu JH, Shin J-Y, Kim SA, Kang S-W, Kim H, Kang S, et al. Non-invasive optical imaging of matrix metalloproteinase activity with albumin-based fluorogenic nanoprobes during angiogenesis in a mouse hindlimb ischemia model. Biomaterials (2013) 34(28):6871–81. doi: 10.1016/j.biomaterials.2013.05.074
107. Lu N, Huang P, Fan W, Wang Z, Liu Y, Wang S, et al. Tri-stimuli-responsive biodegradable theranostics for mild hyperthermia enhanced chemotherapy. Biomaterials (2017) 126:39–48. doi: 10.1016/j.biomaterials.2017.02.025
Keywords: angiogenesis, biomarker, hypoxia, immunotherapy, tumor vasculature, nanoparticles
Citation: Dianat-Moghadam H, Nedaeinia R, Keshavarz M, Azizi M, Kazemi M and Salehi R (2023) Immunotherapies targeting tumor vasculature: challenges and opportunities. Front. Immunol. 14:1226360. doi: 10.3389/fimmu.2023.1226360
Received: 21 May 2023; Accepted: 31 July 2023;
Published: 01 September 2023.
Edited by:
Zohreh Amoozgar, Massachusetts General Hospital and Harvard Medical School, United StatesReviewed by:
Demin Li, University of Oxford, United KingdomIgor Lucas Gomes-Santos, Massachusetts General Hospital and Harvard Medical School, United States
Copyright © 2023 Dianat-Moghadam, Nedaeinia, Keshavarz, Azizi, Kazemi and Salehi. This is an open-access article distributed under the terms of the Creative Commons Attribution License (CC BY). The use, distribution or reproduction in other forums is permitted, provided the original author(s) and the copyright owner(s) are credited and that the original publication in this journal is cited, in accordance with accepted academic practice. No use, distribution or reproduction is permitted which does not comply with these terms.
*Correspondence: Hassan Dianat-Moghadam, RGlhbmF0LmhAbWVkLm11aS5hYy5pcg==