- 1Department of Immunology and Cell Biology, Faculty of Medicine and Health Sciences, Sherbrooke, QC, Canada
- 2Department of Microbiology and Infectious Diseases, Faculty of Medicine and Health Sciences, Sherbrooke, QC, Canada
- 3Department of Biology, Faculty of Science, University of Sherbrooke, Sherbrooke, QC, Canada
- 4Unité de Recherche Clinique et épidémiologique, Centre de Recherche du CHUS, Sherbrooke, QC, Canada
- 5Department of Microbiology and Immunology, The University of North Carolina at Chapel Hill, Chapel Hill, NC, United States
- 6Mammalian Cell Expression, Human Health Therapeutics Research Centre, National Research Council Canada, Montreal, QC, Canada
- 7Departments of Medicine, Faculty of Medicine and Health Sciences, Sherbrooke, QC, Canada
- 8Laboratoire de Microbiologie, CIUSSS de l’Estrie – CHUS, Sherbrooke, QC, Canada
- 9Faculty of Physical Activity Sciences, University of Sherbrooke, Sherbrooke, QC, Canada
- 10Research Centre on Aging, Affiliated with CIUSSS de l’Estrie-CHUS, Sherbrooke, QC, Canada
Background: Following SARS-CoV-2 infection a significant proportion of convalescent individuals develop the post-COVID condition (PCC) that is characterized by wide spectrum of symptoms encompassing various organs. Even though the underlying pathophysiology of PCC is not known, detection of viral transcripts and antigens in tissues other than lungs raise the possibility that PCC may be a consequence of aberrant immune response to the viral antigens. To test this hypothesis, we evaluated B cell and antibody responses to the SARS-CoV-2 antigens in PCC patients who experienced mild COVID-19 disease during the pre-vaccination period of COVID-19 pandemic.
Methods: The study subjects included unvaccinated male and female subjects who developed PCC or not (No-PCC) after clearing RT-PCR confirmed mild COVID-19 infection. SARS-CoV-2 D614G and omicron RBD specific B cell subsets in peripheral circulation were assessed by flow cytometry. IgG, IgG3 and IgA antibody titers toward RBD, spike and nucleocapsid antigens in the plasma were evaluated by ELISA.
Results: The frequency of the B cells specific to D614G-RBD were comparable in convalescent groups with and without PCC in both males and females. Notably, in females with PCC, the anti-D614G RBD specific double negative (IgD-CD27-) B cells showed significant correlation with the number of symptoms at acute of infection. Anti-spike antibody responses were also higher at 3 months post-infection in females who developed PCC, but not in the male PCC group. On the other hand, the male PCC group also showed consistently high anti-RBD IgG responses compared to all other groups.
Conclusions: The antibody responses to the spike protein, but not the anti-RBD B cell responses diverge between convalescent males and females who develop PCC. Our findings also suggest that sex-related factors may also be involved in the development of PCC via modulating antibody responses to the SARS-CoV-2 antigens.
Highlights
● Post-COVID Condition (PCC) is lingering illness that afflicts a significant proportion of COVID-19 patients from three months after clearing SARS-CoV-2 infection. Therapy for PCC is only palliative and the underlying disease mechanisms are unclear. The wide spectrum of PCC symptoms that can affect different organs and the detection of viral components in tissues distant from lungs raise the possibility that PCC may be associated with aberrant immune response due to presence of viral antigens. Therefore, we studied B cell and antibody responses to the spike and nucleoprotein antigens in PCC patients who cleared mild SARS-CoV-2 infection during the pre-vaccination COVID-19 pandemic period. We observed divergent patterns of immune reactivity to the spike protein in PCC males and females at different times post-infection, suggesting that the immune responses in PCC may also be influenced by sex-related factors.
Introduction
SARS and MERS coronaviruses and a few other viral infections are known to cause lingering illnesses (1–3). Among them SARS-CoV-2 is considered unique because of the high proportion of convalescent COVID-19 patients developing the post-covid condition (PCC) (4). PCC encompasses a spectrum of clinical illnesses affecting multiple organs that persist for more than 3 months after the resolution of the initial infection (5–7). It is estimated that 75-90% of SARS-CoV-2 infected individuals develop a mild COVID-19 disease and 5-50% percent of them develop symptoms of PCC for reasons that still remain unclear (8, 9). Pre-existing co-morbidities, high level of SARS-CoV-2 viremia, presence of antibodies to type-I IFNs, reactivation of EBV or CMV-specific T cells during acute infection and anti-SARS-CoV-2 antibody signatures are some of the factors suggested to predict PCC in individuals with moderate to severe COVID-19 (10–12). In cohorts of predominantly hospitalized patients, autoantibody titers to type-I IFNs and anti-nuclear antibodies correlate negatively with anti-SARS-CoV-2 antibodies (10), pointing towards the possibility of deregulated early innate immune response to the virus and/or abnormal activation of atypical memory B cells involved in autoantibody production (13). In PCC associated with mild COVID-19 infection, alterations in certain T cell subsets were observed (14). Peluso et al. (15) reported a decrease in IFNγ+/CD107a+ SARS-CoV-2 nucleoprotein-specific CD8+ T cells at 9 months post-infection in recovered hospitalized and non-hospitalized COVID-19 patients with PCC, when compared to those without PCC suggesting a possibility of subtle variations in anti-viral immune responses contributing to PCC. These observations also suggest that such altered immune responses towards SARS-CoV-2 antigens in PCC patients may persist long after the resolution of acute COVID-19 disease. To better understand the immune responses towards SARS-CoV-2 antigens in PCC, we focused this study on cryopreserved PBMC and frozen plasma samples collected from unvaccinated individuals who developed PCC following mild, PCR-confirmed COVID-19 disease during the early stages of the pandemic prior to vaccination.
Methodology
Study participants
The Biobanque Québécoise de la COVID-19 (BQC19) is a provincial healthcare initiative undertaken in Quebec, Canada, which collects biological specimens (blood cells and plasma) from individuals with PCR-confirmed SARS-CoV-2 infection and the associated anthropometric and clinical data. Enrolment of adult (>18 years old) participants with different disease severity has been ongoing since 26 March 2020, in several centers throughout the Quebec province. Eligible participants for this study were recruited in the Eastern Townships region of Quebec, between March 2020 and October 2021and were infected before October 2021. Participants were seen at 1, 3, 6, 12, 18 and 24 months after confirmed SARS-CoV-2 infection, and blood samples were obtained during each visit. Acute and persistent symptoms were captured using a 28-symptom questionnaire (Supplemental document-1). The questionnaire included details on demographic data including age, sex, weight, height, body mass index (BMI), details of COVID-19 vaccine received and its timing, smoking status, history of hypertension, chronic cardiovascular disease, asthma, other chronic pulmonary diseases, hepatic disease, kidney disease, chronic neurologic disease, active cancer, HIV status, asplenia and use of immunosuppressive drugs. SARS-CoV-2 infection severity was categorized as asymptomatic, mild, moderate and severe according to the WHO definition (16). Peripheral blood mononuclear cells (PBMC) were isolated and preserved in liquid nitrogen and plasma stored at -80°C. This study was approved by the ethic review board of the Centre de Recherche du Centre Hospitalier Universitaire de Sherbrooke (protocol # 2022-4415).
Detection of RBD specific B cells
To identify RBD-specific B cells, 1-2 × 106 PBMCs were labelled with anti-RBD probes along with a panel of cell surface markers to characterize the different B cell subsets by flow cytometry as previously described (17–19). Fluorescent RBD probes were made by combining biotinylated strain specific (D614G and Omicron strains) Spike protein RBD tetramers (AcroBiosystems) with fluorescent streptavidin conjugates. The different RBD probes were prepared individually in PBS with a 4:1 tetramer-streptavidin molar ratio. SARS-CoV-2-specific B cells were identified by staining PBMCs with 50ng of labeled RBD tetramer at 4°C for 30 minutes. Each PBMC sample was divided in two halves, one for staining with the D614G RBD-APC/FITC cocktail and the second half with the Omicron RBD-APC/FITC cocktail. The cells were then washed with PBS/FBS-2% and stained for cell surface markers for 30 minutes at 4°C. The cells were washed two times and resuspended in PBS. A viability stain (DRAQ7) was added to each sample 5 minutes before data acquisition using Cytoflex-30 flow cytometer (Beckman Coulter, California, USA). The sources and catalogue numbers of antibodies and reagents are indicated in Supplemental Table S1. The data was analyzed using FlowJo software V-10 (BD Biosciences, Mississauga, ON, Canada).
ELISA for anti-RBD, anti-spike and anti-nucleocapsid antibodies
Anti-RBD specific IgG antibodies were detected by ELISA using a protocol adapted from Moura et al. (20). In brief, 96-well high-binding microtiter plates were coated with 50 μL of SMT1-1 spike protein (21), D614G-RBD or omicron RBDantigens at 2 μg/mL in tris-buffered saline (TBS; pH 7.4) (Supplementary Table S2). After overnight incubation at 4°C, the plates were washed three times with 200 μL of TBS containing 0.2% Tween 20 (TBST, wash buffer) and blocked with 100 μL of blocking solution (0.1% BSA in TBS containing 0.05% Tween 20) at 4°C overnight. The plates were washed four times with TBST and incubated with plasma samples (Supplementary Table S3) TBST-0.1% BSA, in duplicate wells. Samples were incubated for 2 h at 37°C, washed four times with TBST and incubated with the biotinylated anti-human IgG (1:5000), anti-human IgG3 (1:1000) or anti-human IgA (1:10 000) diluted in TBST-0.1% BSA for 1 h at 37°C. Plates were washed and incubated with 50 μL of 3 μg/mL streptavidin–peroxidase diluted in TBST-0.1% BSA for 30 min at 37°C. After the final washing step (four times), the wells were incubated with the chromogen tetramethylbenzidine (TMB) for 10 min before the reaction was stopped with 50 μL 2N H2SO4. The optical density at 450 nm (OD450) was measured using SPECTROStar Nano (BMG Labtech, Germany) microplate reader. Plasma samples from 5 convalescent individuals were pooled together to obtain a reference pool. Serial dilutions of the reference pool were used to generate a standard curve with a linear regression equation. The equation was then used to extrapolate the antibody levels in samples taking into account the dilution factor.
Anti-nucleoprotein antibodies were similarly quantified by ELISA using HRP-conjugated secondary antibodies. In brief, 50 μL of NCAP-1 nucleoprotein (21), at 2 μg/mL in TBS was coated in 96-well high-binding microtiter plates overnight at 4°C. The plates were then washed, blocked, incubated with plasma samples, and developed using HRP-conjugated anti-human IgG or anti-human IgA as described above for anti-RBD detection.
Detection of SARS-CoV-2 mRNA in plasma
Plasma samples were analyzed for the presence of SARS-CoV-2 virus by real-time PCR using the cobas® SARS-CoV-2 Duo test kit using the cobas® 6800 instrument (Roche Molecular Systems, Inc., Branchburg, NJ). Selective amplification of SARS-CoV-2 target nucleic acid sequence in the sample was achieved through the use of a dual target virus specific approach from the highly-conserved regions of SARS-CoV-2 located in the ORF1a and ORF1a/b non-structural regions. The viral load was quantified against a non-SARS-CoV-2 armored RNA quantitation standard (RNA-QS) introduced into each specimen during sample preparation. The RNA-QS also functions as an internal control to monitor the entire sample preparation and PCR amplification process. The system used 0.4 mL of plasma and the viral quantification range of the assay is between 1x109 IU/mL and 100 IU/mL. Below this level of viral load, the SARS-CoV-2 virus can still be detected but not quantified.
Statistics
GraphPad Prism version 9.5 software was used for statistical analyses and to generate graphics. Statistical comparisons were carried out using the Mann-Whitney’s test. Correlation matrices were calculated using nonparametric Spearman’s correlation. A linear mixed model was used to assess the evolution of antibody response over time. We considered three fixed effects (time, group, and interaction) and a random intercept for repeated measures. Normality of residuals and homoscedasticity (homogeneity of variances) were validated. Results were presented as modelling coefficient (mean differences) and their 95% confidence intervals. Results and figures were obtained using R v.4.1.3. Since these are exploratory results, no multiple testing adjustments were done to obtain p-values. A cross-sectional approach was used to analyze the B cell and antibody responses at 1, 3, 6 and 12 months and then a longitudinal study was used in a limited number of participants to assess changes in antibody levels overtime.
Results
Increased prevalence of PCC symptoms and their positive correlation to acute COVID-19 disease in females
We undertook an unmatched case control study of comparing convalescent individuals with (PCC) or without (No-PCC) post-COVID condition at 3 months (Table 1; Figure 1). Individuals who visited the outpatient clinic for suspected SARS-CoV-2 infection or were contacts of infected individuals were recruited. This study also included a small number of uninfected individuals (U, uninfected) who tested negative for SARS-CoV-2 PCR test in nasal swabs. In both PCC and No-PCC groups, viral infection was confirmed by RT-qPCR at diagnosis of COVID-19 and sequential RT-PCR test in nasal swabs was done until a negative test result prior to specimen collection. Samples were collected at 1, 3, 6 and 12 months following confirmed infection. The symptoms included, but were not limited to PCC was defined according to WHO criteria, only when the symptoms associated with the SARS-CoV-2 infection persisted at 3 months after COVID-19 (16). More that 60% of PCC patients reported persistent symptoms at 6 months after infection (75/90). Participants who received SARS-CoV-2 vaccine post-infection prior to sample collection were excluded from analysis. BMI and frequencies of co-morbidities were comparable between the No-PCC and PCC groups (Table 1). Notably, more females were represented in the PCC group than in the No-PCC group in our study cohort (63% versus 38%). In all PCC and No-PCC cases, the acute phase of the disease was mild and none could be categorized as moderate or severe according to WHO criteria. The common symptoms of PCC recorded in the study group were arthralgia, dyspnea, fatigue, headache, myalgia and anosmia (Figure 2). However, the number of the symptoms reported at the acute disease was significantly higher in PCC groups than in No-PCC groups in both males (6 versus 4) and females (7 versus 5) (Mann Whitney test, males- No-PCC versus PCC, p=0.0035; females- No-PCC versus PCC, p<0.0001) (Figure 2), in agreement with previous reports (7, 9, 22, 23). Notably, in female PCC cases the number of comorbidities positively correlated with age, BMI and the number of PCC symptoms reported at 1-, 3- and 6-months post-infection (Supplementary Figure S1; Supplementary Table S4). Moreover, in females the PCC symptoms reported during the acute infection positively correlated with the number of symptoms reported at 1 and 6 months post-COVID, and the symptoms reported at 3 months correlated with those reported at 6 and 12 months in a significant manner. Even though such an impact of acute disease severity with PCC was also observed in males, these correlations were not statistically significant.
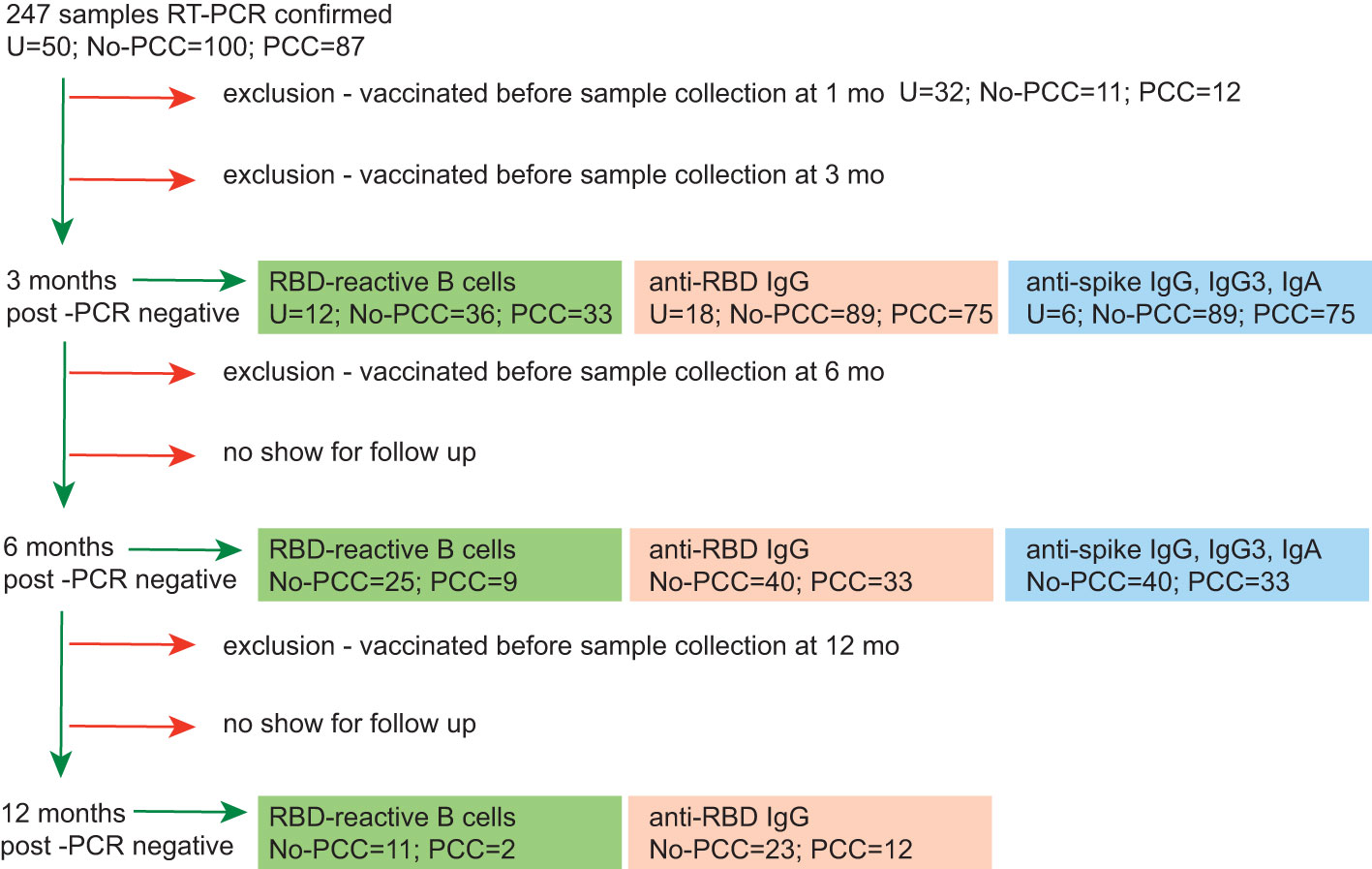
Figure 1 Workflow of sequential immune reactivity analysis of convalescent COVID-19 subjects with or without PCC and sample size distribution. All samples were obtained from the BQC-19 biobank for COVID-19. At recruitment stage, negative COVID-19 status was confirmed by RT-PCR. Sequential RT-PCR tests for SARS-CoV-2 was carried out in COVID-19 positive individuals to confirm virus clearance before specimen collection. PCC, convalescent individuals with persistent symptoms at 3 months post-COVID-19. NO-PCC, no PCC symptoms after acute infection. Plasma was available for experimentation from all PCC or No-PCC individuals at 3 months post-infection. PBMCs were available for experimentation for a subset of individuals. Some individuals had samples available at 1-month post infection. Significant proportion of individuals dropped out at 6- and 12- months during follow up. Individuals who had received SARS-CoV-2 vaccine at any time before sample collection at 1, 3 or 6 months were excluded from the analyses for these time points. Healthy PCR negative individuals with no history of COVID-19 symptoms constituted the uninfected controls (U). Samples from uninfected controls were collected at one time point only.
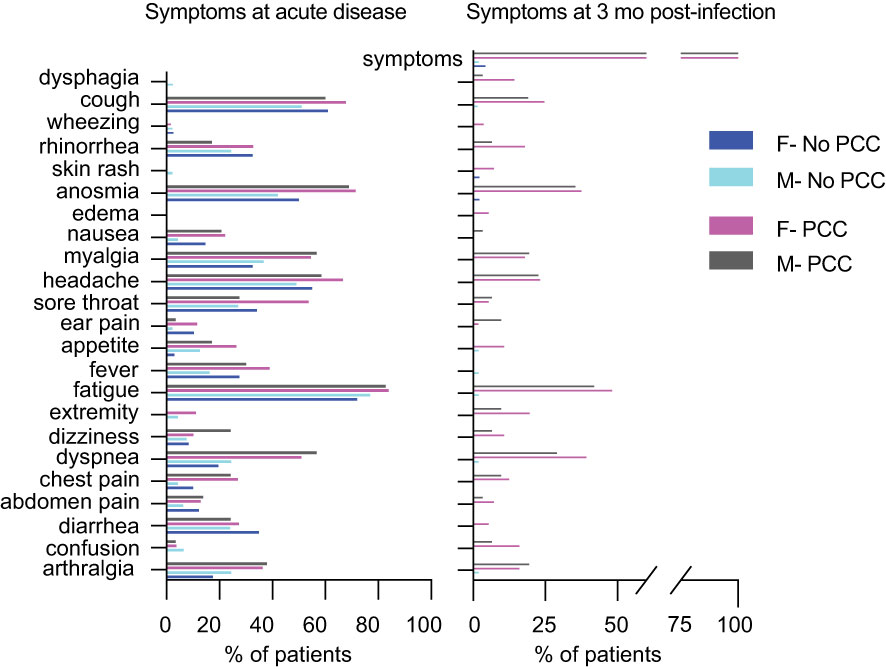
Figure 2 Proportion of individuals exhibiting symptoms at the acute phase of the infection and at 3 mo post-infection. Symptoms at the time of infection and at 3 months post-infection in males and females who were included in this study (data presented in Table 1).
RBD-specific memory B cell frequencies are comparable between PCC and No-PCC groups at three months post-infection
During the early stages of viral infections, antigen-specific cognate B cells may directly differentiate into antibody producing cells in the extra-germinal space (24, 25). As the antiviral immune response progresses with the activation of T cells germinal centers are formed, somatic hypermutation occurs and long-lasting memory B cells are generated (24, 26, 27). The loss of surface IgD and the expression of CD27 are hallmarks of B cells undergoing maturation in germinal centers (28). In addition, IgD-CD27- (double negative, DN) B cells are also reported to be enriched during extrafollicular B cell maturation associated with certain inflammatory conditions and infections including COVID-19 and HIV (24, 26, 27, 29, 30). SARS-CoV-2 infection has been reported to induce viral antigen specific memory B cells within germinal center follicles as well as outside the follicles (27, 29, 31). Therefore, we first assessed whether PCC was associated with altered SARS-CoV-2-specific memory B cells, using RBD region as a surrogate antigen to detect such cells in PBMC.
We assessed the B cell reactivity of convalescent PCC and No-PCC samples as well as SARS-CoV-2 PCR-negative, unvaccinated control samples toward the D614G-RBD epitope, as the samples used in this study were collected between 2020 and 2021 when D614G variant was the most prominent strain before the emergence of delta and omicron strains (https://cov-lineages.org/resources/pangolin.html). Nonetheless, we also assessed B cell reactivity toward the omicron-RBD to assess potential cross reactivity. The gating strategy for identifying RBD specific B cells is shown in Supplementary Figure S2. The staining pattern of CD27 and IgD on CD19+CD20+ B lymphocytes identified naïve (naïve, IgD+CD27-), unswitched memory (USM, IgD+CD27+), switched memory (SM, IgD-CD27+) and double negative (DN, IgD-CD27-) B cells. The reactivity of these B cell subsets to D614G-RBD and omicron-RBD was evaluated using epitope-specific tetramers. The frequency of plasmablasts (CD19+CD20-) in PBMC were very low, hence these cells were not analyzed for RBD binding.
PCC and No-PCC groups displayed a significantly elevated proportion of D614G-RBD reactive B cells within total CD19+ and CD19+CD20+ (excludes plasmablasts) B cell pools when compared to uninfected controls (Figures 3A, B; left panels). The increased frequency of RBD specific B cells mainly resulted from class-switched (IgD-) B cells (Figure 3C; left panel), whereas IgD+ B cells (constitutes unswitched and naïve) showed very low reactivity (Figure 3D; left panel). Comparison of the frequencies of D614G-RBD specific B cell subsets between males and females showed no significant differences between the two convalescent groups (Supplementary Figure S3). Hence, further analyses on RBD reactivities were carried out on pooled data without segregating them by sex. Naïve B cells lose surface IgD expression following antigen mediated activation and class switching. IgD-CD27+ SM B cells that undergo T cell dependent class switching in germinal centers, showed significantly increased frequencies of D614G-RBD reactive cells in the two convalescent groups compared to uninfected controls (Figure 3E; left panel). IgD-CD27- DN B cells, which predominantly originate from extrafollicular class switching, also harbored significantly more D614G-RBD specific cells in both PCC and No-PCC convalescent groups compared to uninfected controls (Figure 3F; left panel).
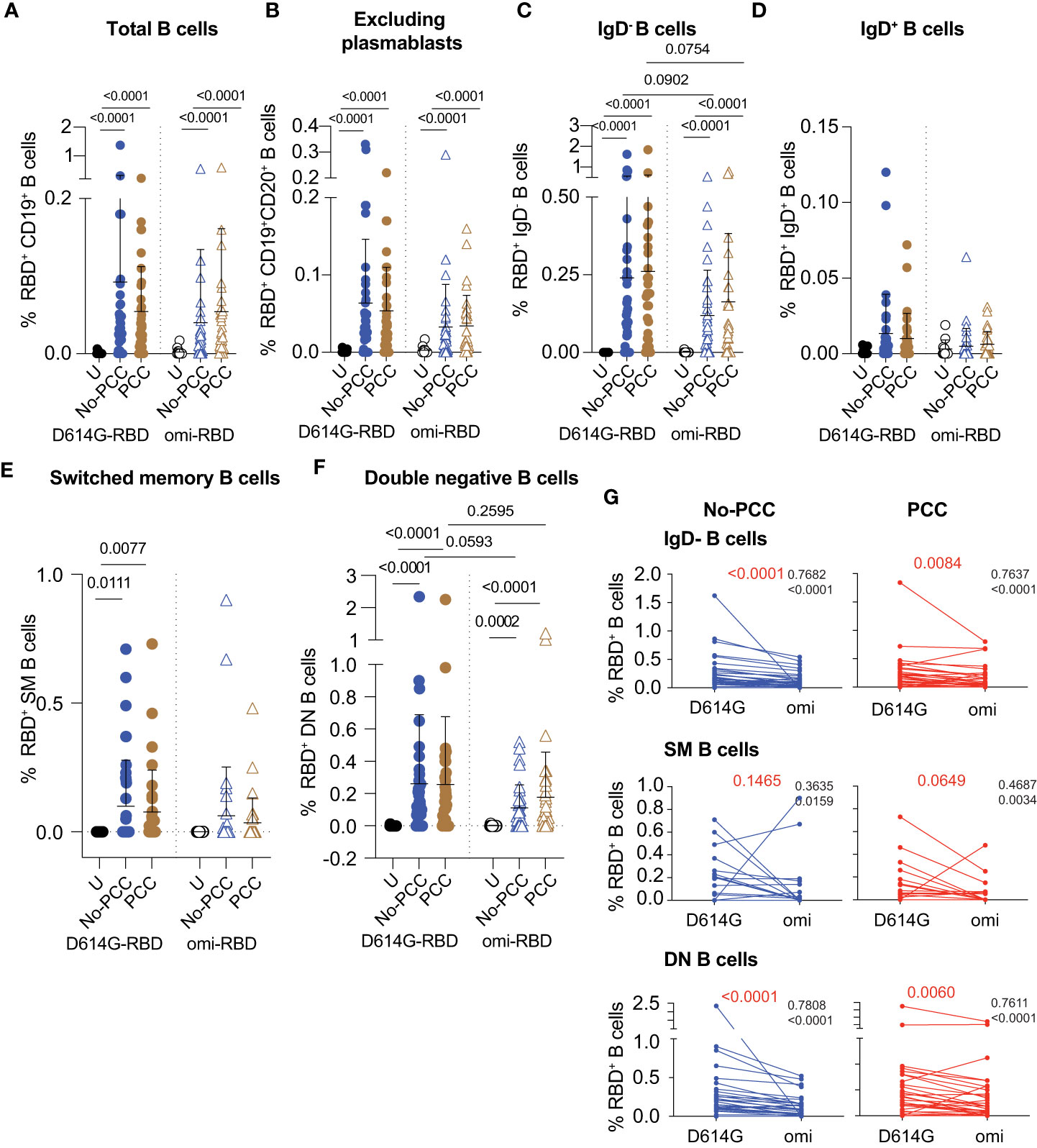
Figure 3 Frequencies of RBD specific B cell subsets at 3 months after mild SARS-CoV-2 infection. PBMCs were labelled with D614G-RBD or omicron-RBD and specific B cell markers, and B cell subpopulations were gated as shown in Supplementary Figure S2. (A-F) Frequencies of D614G-RBD and omicron-RBD specific B cells in total CD19+ B cells (A). CD19+CD20+ (plasmablasts excluded) B cells (B). CD19+CD20+IgD- B cells (C). CD19+CD20+IgD- B cells (D). CD19+CD20+CD27+IgD- switched memory (SM) B cells (E). CD19+CD20+CD27-IgD- double negative (DN) B cells (F). The groups were compared by Mann Whitney’s test and significance values are indicated. Sample numbers: Uninfected (U); convalescent individuals without PCC (No-PCC); convalescent individuals with PCC (PCC). (G) Correlation between anti-D614G-RBD and omicron-RBD specific B cell frequencies in No-PCC and PCC groups. The values in black are ρ value for Spearman coefficient and the corresponding p value. The values in red represent the p values for comparisons between D614G and omicron-RBD specificities by Wilcoxon matched-pairs signed rank test.
Evaluation of B cell reactivity to omicron-RBD also showed significantly increased frequencies within total CD19+ and CD19+CD20+ B cell pools and in the IgD- subset in No-PCC and PCC groups compared to uninfected controls (Figures 3A–C; right panels). Reflecting the specificity of SM B cells to the eliciting antigen-D614G-RBD, the omicron specificity in SM B cells in the convalescent groups was not significantly different from the uninfected group (Figure 3E; right panel). However, omicron-RBD specific B cell frequencies were significantly higher in the DN subset of both PCC and No-PCC convalescent groups than in uninfected controls (Figure 3F; right panel). The frequencies of omicron specific B cells were markedly lower than that of D614G-RBD specific B cells in IgD- and DN B cell subsets, but were not statistically significant (Figures 3C, F). However, the anti-D614G and anti-omicron-RBD responses showed significant correlation in these B cells subsets in both No-PCC and PCC groups (Figure 3G), suggesting potential cross-reactivity between D614G and omicron-RBD that may confer partial protection to the omicron variant.
Distinct anti-RBD and anti-spike antibody responses in PCC and No-PCC groups at three months post-infection
Parallel analysis of antibody titers to SARS-CoV-2 antigens revealed significantly elevated anti-D614G-RBD IgG antibody titers in the plasma samples obtained at three months post-infection in both PCC and No-PCC groups compared to uninfected controls (Figure 4A). Notably, the PCC group showed significantly higher levels of anti-D614G-RBD IgG response than the No-PCC group (Figure 4A), even though their D614G-RBD specific B cell frequencies were comparable within IgD-, DN and SM B cell subsets (Figure 3). On the other hand, IgG response to omicron-RBD was significantly increased in No-PCC and PCC groups when compared to uninfected controls but was not different between the two convalescent groups, suggesting no differences in the response to omicron epitopes in PCC (Supplementary Figure S4). Segregation of the serology data by sex showed that the significantly elevated anti-D614G-RBD IgG responses of PCC group compared to No-PCC group was recapitulated only in males with PCC but not in females (Figure 4B). Moreover, within the PCC group males displayed significantly higher anti-D614G-RBD IgG levels than females (Figure 4B). However, analysis of the antibody response against the whole spike protein showed a different pattern in males and females. Anti-spike IgG, IgG3 and IgA levels were significantly higher in females with PCC when compared to No-PCC females, whereas these responses were comparable between PCC and No-PCC males (Figures 4C–E). Within the No-PCC group, anti-spike IgG and IgA levels were markedly higher in males than in females, but this was not seen within the PCC group (Figures 4C–E). In contrast to anti-spike antibody responses, anti-nucleocapsid IgG responses were similarly elevated in both PCC and No-PCC groups when compared to uninfected controls (Figure 4F). However, there were no differences in anti-nucleocapsid IgG and IgA responses between males and females in both groups (Figure 4G). Overall, the anti-D614G-RBD IgG levels were significantly higher in the PCC group than in No-PCC groups among males, whereas anti-spike IgG, IgG3 and IgA levels were elevated in PCC than in No-PCC group among females at three months post-infection.
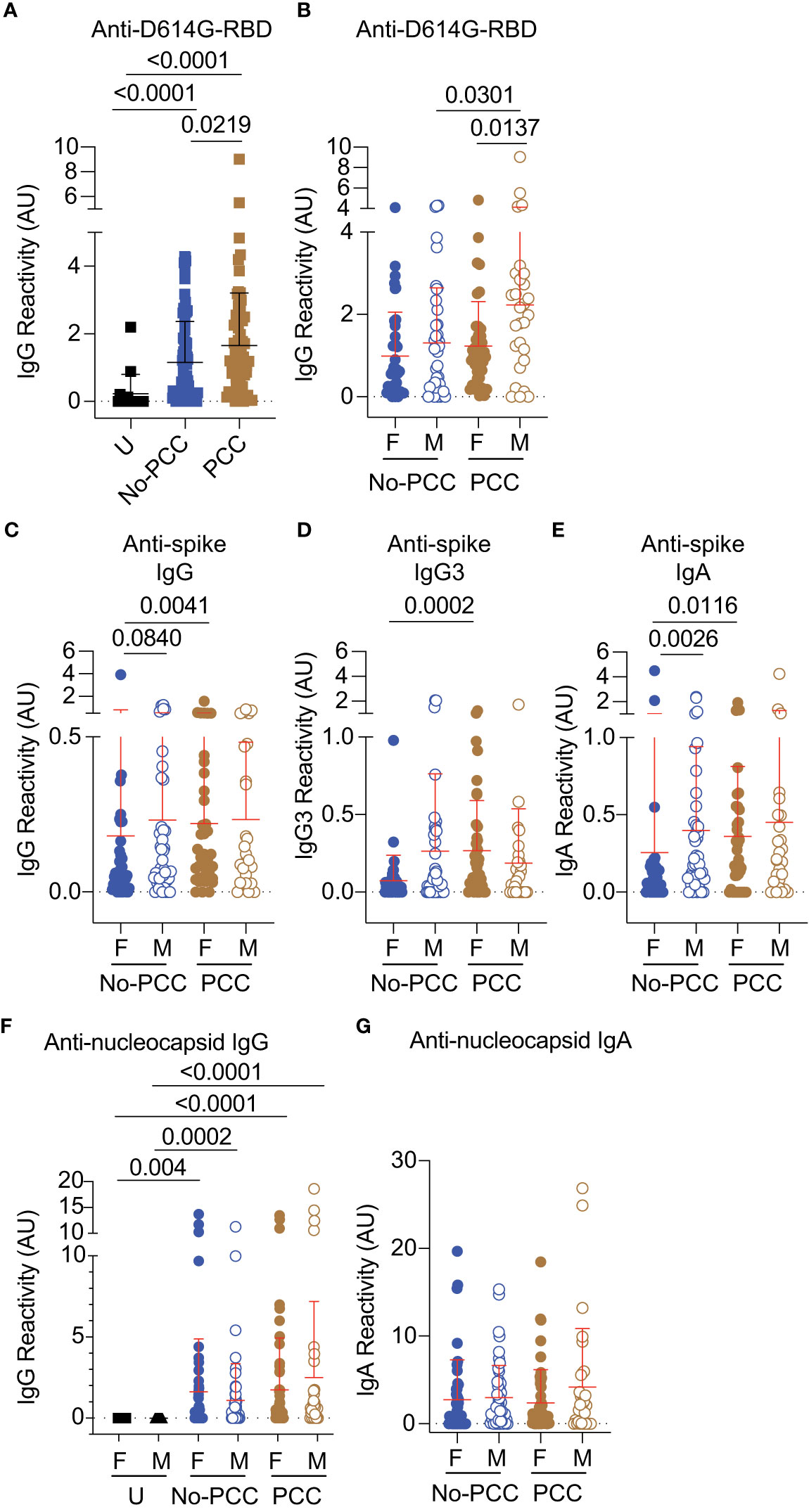
Figure 4 Anti- RBD, spike and nucleocapsid antibody responses in No-PCC and PCC groups at 3 months post-infection. Plasma samples were collected during routine clinical visit at 3 months post PCR-positive diagnosis. Total IgG, IgG3 and IgA responses in uninfected (U) controls and convalescent PCC and No-PCC groups were determined by ELISA. (A) anti-D614G responses in pooled (males and females) samples. (B) anti-D614G-RBD IgG responses in males and females within PCC and No-PCC groups. (C-E) Anti-spike IgG, IgG3 and IgA responses in males and females with or without PCC. (F, G) Anti-nucleocapsid IgG and IgA responses in males and females within U, PCC and No-PCC groups. The groups were compared by Mann Whitney’s test.
Pattern of immune responses at three months distinguish male and female convalescent groups with and without PCC
Next, we determined how the symptoms at acute infection correlated with the B cell reactivities and antibody responses at three months post infection in convalescent PCC and No-PCC groups in males and females using correlation matrices. The Spearman correlation and the corresponding significance values depicted in Supplementary Figure S5 (Supplementary Table S5) showed a significant positive correlation between anti-spike IgG and anti-D614G-RBD IgG antibodies in all groups, as expected (Supplementary Figure S5, orange asterisks). Similarly, anti-D614G-RBD specific DN B cells showed a significant positive correlation with D614G-RBD specific CD19+CD20+ B cells and the class-switched IgD- subset in all the groups (Supplementary Figure S5, yellow asterisks), indicating that our observations on the D614G-RBD specific DN B cells reflect the B cell responses to the RBD domain in these individuals as expected. Curiously, the IgG, IgG3 and IgA response to spike protein showed significant correlation to D614G-RBD specific CD19+, CD19+CD20+ and IgD- B cell responses only in the convalescent males who do not develop PCC at 3 months post infection expected (Supplementary Figure S5B, blue asterisks). In all the other groups (males and females with PCC and females without PCC), the antibody responses showed no specific correlation to D614G-RBD specific B cell responses, despite the presence of detectable levels of circulating antibodies to spike and D614G-RBD (Supplementary Figures S5A, C, D; Supplementary Table S5; Figure 4). Notably, the number of symptoms at the acute infection did not correlate with any B cell or Ab parameters in male or female PCC cohorts except with anti-D614G-RBD specific IgD- B cells in females with PCC (Supplementary Figure 5C, red asterisk). Female PCC cases also showed a strong positive correlation between CD19+ and SM B cells that was not observed in other groups (Supplementary Figure 5C, green asterisks). Collectively, these observations indicate that the convalescent males show a coordinated immune response that appear to be deregulated by PCC in males.
To determine how the B cell reactivities and antibody responses towards SARS-CoV-2 antigens during the early convalescent period correlated with progression towards PCC, we evaluated these immune parameters at 1-month post-infection. The proportion of D614G-RBD specific B cells and IgG were comparable between the two convalescent groups in females and males at 1-month post-infection as at three months (Figures 3A, 5A, B; Supplementary Figure S3). Antibody responses to nucleocapsid antigens were comparable between the PCC and No-PCC group among males and females at 1-month post-infection as at 3-months (Figures 4F, G, 5D). Our study cohort included a few individuals who got infected after vaccination among males and females within the No-PCC group and females within the PCC group. These vaccinated individuals exhibited higher anti-D614G RBD and anti-spike IgG responses but reduced antibody responses to nucleocapsid at 1-month post-infection (Figures 5A-D), however, these sample numbers are too low to permit meaningful comparisons.
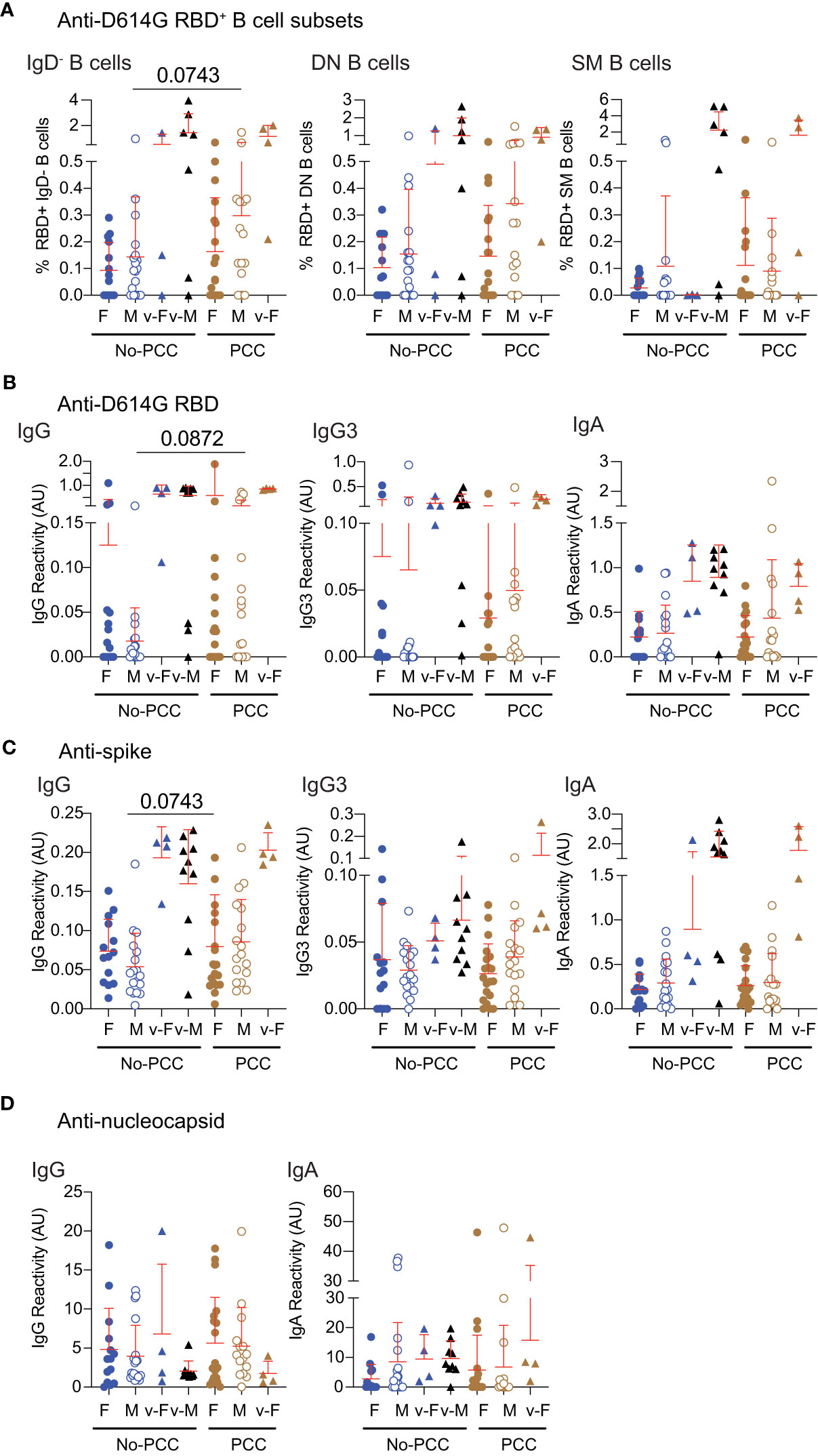
Figure 5 Anti-RBD, spike and nucleocapsid specific responses in samples obtained at 1-month post-infection. Plasma samples were collected during routine clinical visit at 1 month after PCR-positive diagnosis. (A) D614GRBD reactive B cell subsets. (B) anti-D614G-RBD (C) anti-spike and (D) anti-nucleocapsid antibody responses were determined in females and males with or without PCC. v-M and v-F denote vaccinated individuals within the indicated groups. The groups were compared by Mann Whitney’s test.
PCA analyses of the data obtained at one- and three-months post-infection indicated clear differences between the two timepoints, but did not distinguish between convalescent individuals with and without PCC (Supplementary Figure S6). Nonetheless, we examined the concordance between the different antibody responses in the four study cohorts at 1-month post-infection. The anti-D614G-RBD and anti-spike antibody responses were comparably coordinated in No-PCC groups among males and females (Supplementary Figures S7A, B; Supplementary Table S6). However, in the females who developed PCC, anti-D614G-RBD and anti-spike antibody responses showed a high degree of correlation with anti-nucleocapsid responses (Supplementary Figure S7C, yellow asterisks), whereas these correlations were mostly non-significant or weak in the male PCC group. In all the four study groups, the anti-D614G-RBD levels did reveal any meaningful correlation with the D614G-RBD-reactive B cell subsets (Supplementary Figure S8). In both males and females with PCC, the symptoms at the acute phase significantly correlated with anti-D614G-RBD IgG responses at 1 month (Supplementary Figures S7C, D), although they did not correlate at 3 months post-infection (Supplementary Figures 5C, D). These observations suggest that the IgG response to D614G-RBD at 1-month post-infection appears to be a notable hallmark to predict PCC in males and females.
D614G-RBD specific IgG levels persists at 6 months post infection in convalescent males with PCC group
As the strength of adaptive immune response to SARS-CoV-2, SARS and MERS is proportional to severity of infection (18, 32–42), attrition of SARS-CoV-2 specific antibodies and B cells is observed in mildly symptomatic infections over time. Sequential plasma and PBMC samples obtained from the same individuals at one-, three-, six- and twelve- months post-infection and were analyzed for anti-D614G-RBD responses. Only individuals who had not been vaccinated at the time of sample collection at 6 months post-COVID-19 diagnosis were included in the analyses. As reported by others in asymptomatic or mild infections (39, 43–51), anti-D614G-RBD antibody response was barely detectable in male and female convalescent groups without PCC, whereas males in the PCC group showed persistently high levels of anti-D614G-RBD IgG levels at 6 months post-infection (Figure 6A). Evaluation of the longevity of these antibody responses at 1, 3 and 6 months showed a rise in anti-D614G-RBD IgG levels at 3 months posit-infection that returned to baseline levels in No-PCC males and females (Figures 6B, C). Interestingly, anti-spike IgG, IgG3 and IgA titers did not show appreciable decline at 6 months in both No-PCC and PCC groups among males and females (Supplementary Figure S9). Comparison of the pattern of antibody responses to spike and RBD at 1-, 3- and 6-months post infection, also revealed a better coordinated immune response in males without PCC over time (Supplementary Figure S10; Supplementary Table S7). On the other hand, in males and females with PCC, these obvious patterns were not evident. Taken together, these observations point towards the possibility that the pattern of immune responses generated at early time points (1 to 3 months) after the infection may be associated with PCC.
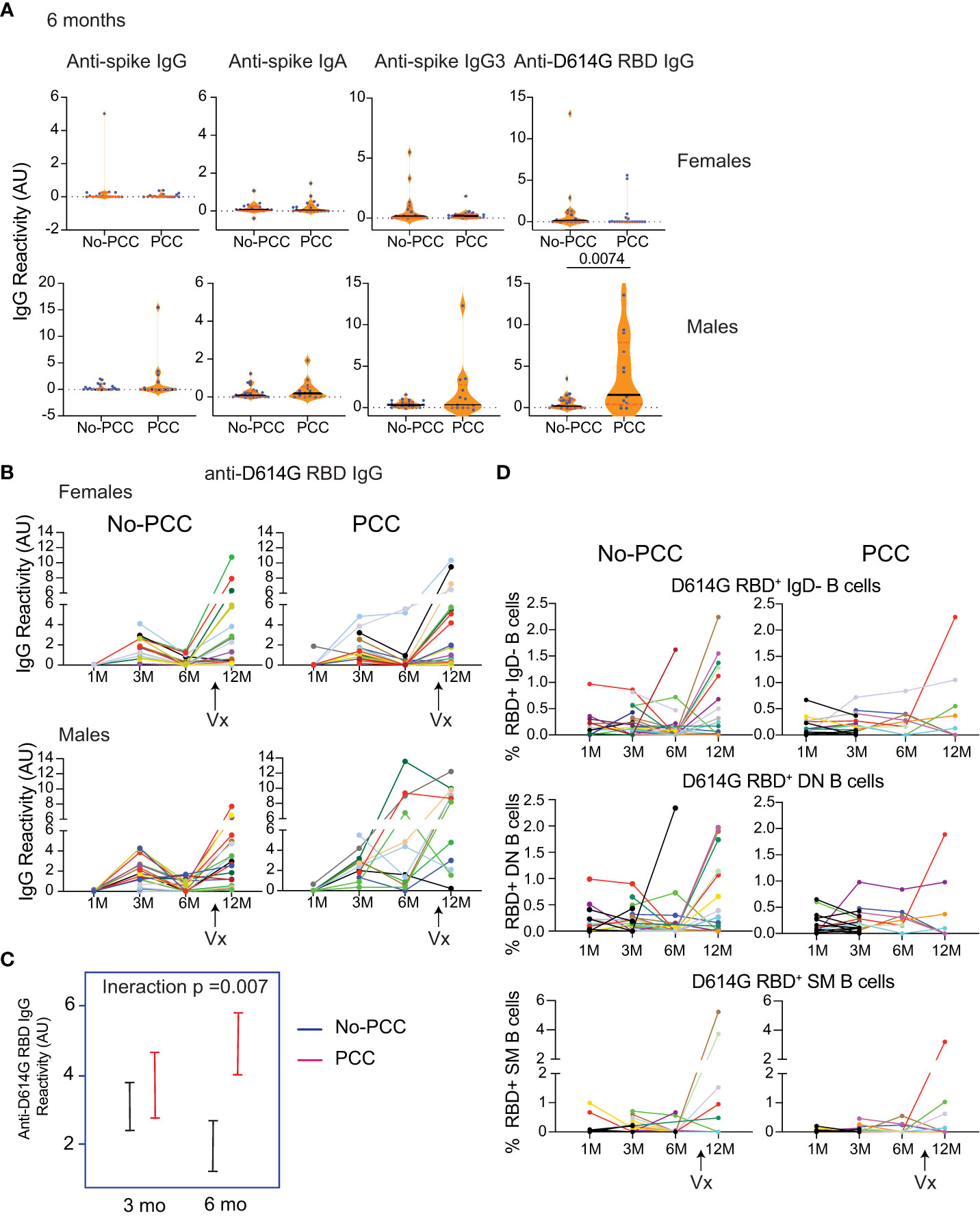
Figure 6 Persistent D614G-RBD specific IgG antibodies at 6 months in the male PCC group. Plasma samples acquired at 1-, 3-, 6- and 12-months post-SARS-CoV-2 PCR positivity were analyzed for anti-D614G-RBD specific IgG antibodies as described in Figure 3. (A) Anti-spike IgG, IgG3 and IgA antibodies and anti-D614G-RBD specific IgG antibodies at 6 months post-infection in non-vaccinated No-PCC and PCC groups among females and males. (B) evolution of anti-RBD IgG antibody from 1-12 months in females and males without or with PCC. (C) Comparison of the changes in the anti-RBD IgG between convalescent males without (blue) or with PCC (red) from 3 to 6 months. (D) Changes in RBD specific B cells. Statistical analysis was carried out by Mann-Whitney’s test (A) and by linear mixed model (C).
At 12 months, most individuals included in this study had received at least 1 dose of vaccine (Pfizer, Moderna or Comirty). Vaccination in general induced a strong anti-D614G-RBD IgG response in all groups to a comparable level, although significant numbers of non-responders were evident in all groups (Figure 6B). This increase in IgG response was associated with a trend towards an increase in the proportion of D614G-RBD reactive IgD-, DN and SM B cells that was more apparent in No-PCC cases than PCC cases, which were limited by small sample size for statistical comparisons (Supplementary Figures S7C, D, S11).
Discussion
To our knowledge this is the first follow-up study on convalescent COVID-19 subjects who recovered from the disease but developed PCC that analyzes immune responses towards SARS-CoV-2 antigens at different time points post-infection. The results presented here point towards sex-based differences in anti-SARS-CoV-2 immune responses. As the study included only the subjects with mild COVID-19 disease, the overall antibody titers were low in both PCC and control No-PCC convalescent groups in agreement with other reports (32–37). Anti-RBD B cell responses were comparable between convalescent males and females and between those with and without PCC. However, differences were observed at the level of circulating antibodies to spike antigen. Given the differences in the severity of the symptoms at the acute disease and the differential susceptibility of males and females to PCC, we analyzed the immune responses separately in the two sexes.
Differences in the antibody responses between convalescent males and females
The immune responses to the spike antigen seem to differ between males and females after mild COVID-19. At 3 months post-infection, the magnitude of anti-spike antibody responses is lower in the No-PCC convalescent females when compared to No-PCC convalescent males (Figure 4). The anti-RBD specific B cells, used as a surrogate for B cell responses to SARS-CoV-2 antigens, were comparable between the sexes irrespective of their PCC status at 1, 3 and 6 months post-infection (Figures 3, 5, 6), supporting the observations that the sex-dependent influences are manifested following the generation of the antigen-specific B cells (52, 53). Anti-viral immune responses are reported to be reduced in amplitude in females when compared to males during the early stages of COVID-19 pandemic and for other infections at the mucosal surfaces (54–58). These observations may explain why males are more susceptible to SARS, MERS and SARS-CoV-2 infections and that the viremia tends to be higher in males than in females (59). In contrast to SARS infections, females were more susceptible during 1957 and 2009 influenza pandemics (60–62). However, innate immune responses to viral infections have been reported to be higher in females (63, 64), which can explain the increased responsiveness of females to vaccines (65). COVID-19 vaccines elicited higher levels of IgG antibodies in females than in males, probably reflecting the heightened innate immune responses in females (66, 67). The increased responsiveness of female biological sex when compared to male biological sex has been observed across various vaccination protocols even in younger age groups (68–70). Similarly, females are more prone to develop autoimmunity (reviewed in (71)). In the light of the above reports, our results showed that the anti-spike responses are lower in convalescent females and suggest that the sex-based differences in susceptibility to infections, immune response to vaccines and the propensity to develop immune-related pathologies (PCC here and autoimmunity in discussion) are likely modulated in context dependent manner such as on the nature of antigen exposure (vaccine versus infection), the nature of the antigen encountered and the pathogen in question. Various factors such as the dosage effect of immune genes on X chromosome and regulation of innate and adaptive immune response by estrogen can contribute to the differences in the immune responses between the two sexes (65).
Another notable observation of our study is the positive correlation between anti-spike B cell and antibody responses at 1 month in both females and males irrespective of whether they progress toward PCC or not. In mild COVID-19 disease, coordinated innate, T and B cell responses at 3 weeks post infection has been observed (72). However, by 3 months post-infection, this correlation becomes stronger in the males who do not develop PCC whereas in other groups studied such correlation is restricted to the D614G-RBD specific B cell subsets. Omics based systems biology approach indicated that males who recovered from COVID-19 showed a better coordinated innate, B cell and antibody responses to subsequent influenza vaccination (73). While the history of infections and influenza vaccinations are not known in the participants involved in this study, nevertheless it suggests that immune responses are continually being shaped by past infections. It is possible that SARS infections shape the immune responses in males and females in a distinct manner such that immune responses to a heterologous antigen, such as influenza vaccine studied by Sparks et al., are altered (73). Immunodominance hierarchies have been observed to be have a sex/gender bias in T cell responses (74, 75). Androgens have been shown to influence the T cell responses, thereby accentuating the sex-based T cell responses (76, 77). Thus, it is not unlikely that each infection displays a distinct sex bias that influences the outcome of subsequent infections/vaccinations. As data comparing the immune responses of post-puberty males and females to other infections are unavailable to our knowledge, it is difficult to determine whether our observations on convalescent COVID-19 males and females are unique to SARS-CoV-2 infection or can be generalized to other infections. While trained innate immune response following SARS-CoV-2 infection in males appear to correlate with the outcome of influenza vaccination (73), it raises the possibility that immune responses in males are continuously shaped by the previous infections while they may be subject to modulation by sex-intrinsic factors in females.
B cell responses
In most of the convalescent individuals with or without PCC, we observed that the RBD specific B cells were predominant in circulation, although there was no significant correlation between the RBD specific B cell subsets and anti-RBD specific antibody levels, as reported in SARS-CoV-2 vaccinated individuals (78). While IgD− memory B cells are mostly generated through the germinal center reaction, DN B cells are generated in the interfollicular space where they interact and get activated by T cells, bypassing the germinal center events to undergo affinity maturation and somatic hypermutation (79). Such B cells are dominant in several autoimmune conditions such as lupus and in neuro-inflammatory diseases (13, 24). They are also increased early following vaccinations (24) and are thought to be important for the initial antiviral response that generates short-lived plasmablasts and gradually replaced by germinal center-derived B cell as the infection progresses and the immune system undergoes full activation (27, 79, 80). Persistence of DN B cells could reflect the inability to develop efficient germinal centers as previously reported by us in a more severe form of COVID-19 infection where Bcl-6-expressing T follicular helper cells and germinal centers are not able to form (29). Alternatively, sustained activation of spike protein-specific B cells in the peripheral reservoir due to viral persistence or antigen spreading could sustain DN B cell development and deregulate the immune response and lead to PCC symptoms. However, our flow cytometry analyses confined to circulating lymphocytes did not permit exhaustive characterization of DN B cell subsets. As the DN subsets are heterogeneous, their detailed characterization and BCR repertoire mapping could provide information on the trajectory of the RBD specific B cell subsets (13, 26). Alterations in DN B cell subsets have been observed to be associated with autoantibodies in COVID-19 (81). Even though our analyses of RBD specific DN B cell frequencies did not show significant difference between PCC and No-PCC subjects in both sexes, a possible difference in DN subsets cannot be excluded.
Coordinated antibody responses
Our observation that the anti-spike and anti-RBD IgG, IgG3 and IgA responses appear to be less coordinated in individuals with PCC points towards the possibility that the propensity to develop PCC may be associated with inherent differences in the evolution of anti-viral immune responses. However, a previous study did not observe differences in the total anti-RBD Ig titers between PCC and No-PCC (82). As the differences between the convalescent groups with and without PCC observed in our study are subtle and nuanced, it is possible that total Ig levels may not truly reflect the differences as each antibody isotype has different functions. For example, Cervia et al. (11) observed that SARS-CoV-2 infected patients with mild or severe disease who went on to develop PCC had higher titers of anti-spike S1 IgG and IgA at the time of infection. Similarly, T cell responses have been observed to be altered in PCC, but the implication of these changes are not clear (83–85). Increased antibody responses to the spike antigen following vaccination in the individuals with PCC suggests that differences in immune responses persist over long time after the initial infection is resolved (83). These observations suggest that the immune responses to SARS-CoV-2 antigens are qualitatively different in PCC and clearly additional parameters need to be included to predict the immune trajectory toward PCC. In this context, it is noteworthy we observed a strong positive correlation between the number of symptoms at the acute and anti-RBD D614G IgG responses at 1-month post-infection in PCC in males and females.
The human common cold coronaviruses and the three pandemic-associated coronaviruses are not known to cause latent infections (86). Infective SARS-CoV-2 viral particles are not known to persist in immunocompetent individuals beyond 9 days after the onset of COVID-19 symptoms even though viral RNA can be detected in respiratory tract, serum and stools for up to 20 days (87, 88). Nonetheless, both the spike protein and viral RNA has been detected in the circulation of PCC patients (89, 90). While we did not detect viral transcripts in the nasal swabs or plasma samples (except for two samples where the amount of virus was below the limit of quantification >100 UI/ml, Ct values of 40.56 and 40.77) that were analyzed at 3 months post-infection for viral transcripts, we cannot rule out possible sequestration of viral particles elsewhere as observed in other internal organs (91). Fc-gamma receptor-mediated infection of monocytes leads to abortive replication of SARS-CoV-2 and induction of inflammation (92), suggesting the generation of non-infectious viral particles. ACE2 expression, which mediates viral entry in lung alveolar epithelial cells, has been detected in a wide range of tissues including intestinal enterocytes, vascular endothelium, smooth muscle cells, a subset of dorsal root ganglion neurons, trigeminal sensory ganglia and specialized neuroepithelial cells (93). Despite low levels of viral copy numbers, SARS-CoV-2 has been detected in the brain, kidney, and other organs (91, 94). Hence, it is possible that viral particles persist in some individuals for a longer duration, possibly due to the infection of various cell types, resulting in prolonged antibody generation contributing to PCC development. Even though coronavirus infections in upper respiratory tract are cleared by humoral and cell mediated immune responses (95, 96), neuroimaging of PCC patients 10 months after the acute infection points towards certain alterations in functional connectivity and reduction in grey matter in the associated regions (97). Using (18)F-FDG-PET scan to evaluate glucose uptake by different organs, hypometabolism was observed in the brains of patients with PCC (98–100). However, it is not known whether PCC is associated with some form of abortive infection of distal organs and tissues in addition to upper respiratory tract. Such a scenario can explain some of the PCC associated symptoms such as the neuro/muscular problems, chronic fatigue and brain fog. It is possible that the heterogeneity in PCC subtypes may be associated with not only the lingering viral particles in the associated organs and tissues but also the variations in immune responses.
Limitations
One important limitation of our study is the reduced availability of unvaccinated samples after 3 months post infection. This can be explained by the increasing number of people consenting to take the vaccine as recommended by the WHO and national guidelines. Also, samples from vaccinated individuals with or without PCC was not included in this study. Comparable analyses of vaccinated convalescent individuals with and without PCC, taking into consideration breakthrough infections and vaccination details can provide information on whether repeated vaccination alters the pattern of immune responses in individuals with PCC. However, consensus from various studies suggest that, while vaccination before the first SARS-CoV-2 infection can reduce the incidence of PCC, vaccination post-infection in individuals with PCC appears to have minimal influence on their symptoms (23, 101–103). The second limitation is the use of custom-made ELISA and the absence of neutralization assays limit the conclusions that can be arrived at from this study. Nonetheless, the conclusions of the study are valid and will permit additional in-depth analyses on larger cohorts. The third limitation is the lack of detailed analyses of the various RBD specific B cell subsets including the DN B cells and plasmablasts caused by the access to a very limited quantity of peripheral blood samples. Nonetheless, the results presented in this study support the notion that PCC could be associated with dysregulated immune responses to the viral antigens, at least in males. As we did not analyze germinal center responses, it is not clear whether the observed differences in antibody responses can be considered a proxy for germinal center reactions. Furthermore, whether these altered immune responses are a consequence or a disease promoter awaits further studies in larger and diverse cohorts using additional clinical and immune parameters.
Data availability statement
The datasets presented in this article are not readily available because Permission from the sample biobank will be required. Requests to access the datasets should be directed to c2hlZWxhLnJhbWFuYXRoYW5AdXNoZXJicm9va2UuY2E=.
Ethics statement
The studies involving humans were approved by Institutional human ethics committee, CIUSSS de l’Estrie – CHUS, Université de Sherbrooke (approval number 2022-4415). The studies were conducted in accordance with the local legislation and institutional requirements. The participants provided their written informed consent to participate in this study.
Author contributions
Study design and funding: SR, AP, ID, HA-C, SI, AC and AM. Identification of clinical samples: CR-P. Sample preparations for flow cytometry, data acquisition and analysis: M-AL and HA-C. ELISA: AQ, M-AL, FR, SA, MoN, MaN and MC and SR. LP and YD provided reagents. Viral detection in plasma samples: AP, SL and CR-P. Data compilation and analyses: M-AL and SR. PCA analysis: J-FL. Statistical analyses: SL-P. manuscript writing: M-AL, HA-C, AM, SI, AP and SR. Manuscript editing, reviewing: M-AL, AQ, SR, AP, ID, HA-C, SI, AC and AM. All authors contributed to the article and approved the submitted version.
Funding
This work was supported by the Canadian Institutes of Health Research pandemic response (GA4-177773 to SR and AP). HA-C is a Junior 1 Clinical Research Scholar from the Fonds de recherche du Queíbec-Santeí and holds the Andreí-Lussier research chair of the Université de Sherbrooke. MC is the recipient of PhD scholarship from the Faculty of Medicine, Université de Sherbrooke. ID holds the Research Chair on Healthy Aging from Foundation JL Gravel et B Breton.
Acknowledgments
We want to acknowledge Roche Diagnostics Canada for providing the cobas® SARS-CoV-2 Duo kits. The graphic abstract was generated using Biorender.
Conflict of interest
The authors declare that the research was conducted in the absence of any commercial or financial relationships that could be construed as a potential conflict of interest.
Publisher’s note
All claims expressed in this article are solely those of the authors and do not necessarily represent those of their affiliated organizations, or those of the publisher, the editors and the reviewers. Any product that may be evaluated in this article, or claim that may be made by its manufacturer, is not guaranteed or endorsed by the publisher.
Supplementary material
The Supplementary Material for this article can be found online at: https://www.frontiersin.org/articles/10.3389/fimmu.2023.1223936/full#supplementary-material
References
1. Hickie I, Davenport T, Wakefield D, Vollmer-Conna U, Cameron B, Vernon SD, et al. Post-infective and chronic fatigue syndromes precipitated by viral and non-viral pathogens: prospective cohort study. BMJ (2006) 333:575. doi: 10.1136/bmj.38933.585764.AE
2. Clark DV, Kibuuka H, Millard M, Wakabi S, Lukwago L, Taylor A, et al. Long-term sequelae after Ebola virus disease in Bundibugyo, Uganda: a retrospective cohort study. Lancet Infect Dis (2015) 15:905–12. doi: 10.1016/S1473-3099(15)70152-0
3. Ong KC, Ng AW, Lee LS, Kaw G, Kwek SK, Leow MK, et al. Pulmonary function and exercise capacity in survivors of severe acute respiratory syndrome. Eur Respir J (2004) 24:436–42. doi: 10.1183/09031936.04.00007104
4. Carfi A, Bernabei R, Landi F, Gemelli Against COVID-19 Post-Acute Care Study Group. Persistent symptoms in patients after acute COVID-19. JAMA (2020) 324:603–5. doi: 10.1001/jama.2020.12603
5. Nalbandian A, Sehgal K, Gupta A, Madhavan MV, McGroder C, Stevens JS, et al. Post-acute COVID-19 syndrome. Nat Med (2021) 27:601–15. doi: 10.1038/s41591-021-01283-z
6. Sykes DL, Holdsworth L, Jawad N, Gunasekera P, Morice AH, Crooks MG. Post-COVID-19 symptom burden: what is long-COVID and how should we manage it? Lung (2021) 199:113–9. doi: 10.1007/s00408-021-00423-z
7. Sudre CH, Murray B, Varsavsky T, Graham MS, Penfold RS, Bowyer RC, et al. Attributes and predictors of long COVID. Nat Med (2021) 27:626–31. doi: 10.1038/s41591-021-01292-y
8. C.C. Global Burden of Disease Long, Wulf Hanson S, Abbafati C, Aerts JG, Al-Aly Z, Ashbaugh C, et al. Estimated global proportions of individuals with persistent fatigue, cognitive, and respiratory symptom clusters following symptomatic COVID-19 in 2020 and 2021. JAMA (2022) 328:1604–15. doi: 10.1001/jama.2022.18931
9. Gallant M, Rioux-Perreault C, Lemaire-Paquette S, Piché A. SARS-CoV-2 infection outcomes associated with the Delta variant: a prospective cohort study. J Assoc Med Microbiol Infect Dis Canada (2022) e20220022. doi: 10.3138/jammi-2022-0022
10. Su Y, Yuan D, Chen DG, Ng RH, Wang K, Choi J, et al. Multiple early factors anticipate post-acute COVID-19 sequelae. Cell (2022) 185:881–895.e20. doi: 10.1016/j.cell.2022.01.014
11. Cervia C, Zurbuchen Y, Taeschler P, Ballouz T, Menges D, Hasler S, et al. Immunoglobulin signature predicts risk of post-acute COVID-19 syndrome. Nat Commun (2022) 13:446. doi: 10.1038/s41467-021-27797-1
12. Peluso MJ, Deeks SG. Early clues regarding the pathogenesis of long-COVID. Trends Immunol (2022) 43:268–70. doi: 10.1016/j.it.2022.02.008
13. Jenks SA, Cashman KS, Zumaquero E, Marigorta UM, Patel AV, Wang X, et al. Distinct effector B cells induced by unregulated toll-like receptor 7 contribute to pathogenic responses in systemic lupus erythematosus. Immunity (2018) 49:725–739.e6. doi: 10.1016/j.immuni.2018.08.015
14. Glynne P, Tahmasebi N, Gant V, Gupta R. Long COVID following mild SARS-CoV-2 infection: characteristic T cell alterations and response to antihistamines. J Investig Med (2022) 70:61–7. doi: 10.1136/jim-2021-002051
15. Peluso MJ, Deitchman AN, Torres L, Iyer NS, Munter SE, Nixon CC, et al. Long-term SARS-CoV-2-specific immune and inflammatory responses in individuals recovering from COVID-19 with and without post-acute symptoms. Cell Rep (2021) 36:109518. doi: 10.1016/j.celrep.2021.109518
16. WHO (2021). Available at: https://www.who.int/publications/i/item/WHO-2019-nCoV-Post_COVID-19_condition-Clinical_case_definition-2021.1.
17. Cohen KW, Linderman SL, Moodie Z, Czartoski J, Lai L, Mantus G, et al. Longitudinal analysis shows durable and broad immune memory after SARS-CoV-2 infection with persisting antibody responses and memory B and T cells. Cell Rep Med (2021) 2:100354. doi: 10.1016/j.xcrm.2021.100354
18. Robbiani DF, Gaebler C, Muecksch F, Lorenzi JCC, Wang Z, Cho A, et al. Convergent antibody responses to SARS-CoV-2 in convalescent individuals. Nature (2020) 584:437–42. doi: 10.1038/s41586-020-2456-9
19. Limoges MA, Lortie A, Demontier E, Quenum AJI, Lessard F, Drouin Z, et al. SARS-cov-2 mRNA vaccine-induced immune responses in rheumatoid arthritis. J Leukoc Biol (2023). doi: 10.1093/jleuko/qiad086
20. Moura AD, da Costa HHM, Correa VA, de SLAK, Lindoso JAL, De Gaspari E, et al. Assessment of avidity related to IgG subclasses in SARS-CoV-2 Brazilian infected patients. Sci Rep (2021) 11:17642. doi: 10.1038/s41598-021-95045-z
21. Colwill K, Galipeau Y, Stuible M, Gervais C, Arnold C, Rathod B, et al. A scalable serology solution for profiling humoral immune responses to SARS-CoV-2 infection and vaccination. Clin Transl Immunol (2022) 11:e1380. doi: 10.1002/cti2.1380
22. Gallant M, Mercier K, Rioux-Perreault C, Lemaire-Paquette S, Piché A. Prevalence of persistent symptoms at least 1 month after SARS-CoV-2 Omicron infection in adults. J Assoc Med Microbiol Infect Dis Canada (2022) 8:57–63.
23. Perlis RH, Santillana M, Ognyanova K, Safarpour A, Lunz Trujillo K, Simonson MD, et al. Prevalence and correlates of long COVID symptoms among US adults. JAMA Netw Open (2022) 5:e2238804. doi: 10.1001/jamanetworkopen.2022.38804
24. Ruschil C, Gabernet G, Lepennetier G, Heumos S, Kaminski M, Hracsko Z, et al. Specific induction of double negative B cells during protective and pathogenic immune responses. Front Immunol (2020) 11:606338. doi: 10.3389/fimmu.2020.606338
25. Beckers L, Somers V, Fraussen J. IgD(-)CD27(-) double negative (DN) B cells: origins and functions in health and disease. Immunol Lett (2023) 255:67–76. doi: 10.1016/j.imlet.2023.03.003
26. Sanz I, Wei C, Jenks SA, Cashman KS, Tipton C, Woodruff MC, et al. Challenges and opportunities for consistent classification of human B cell and plasma cell populations. Front Immunol (2019) 10:2458. doi: 10.3389/fimmu.2019.02458
27. Sokal A, Chappert P, Barba-Spaeth G, Roeser A, Fourati S, Azzaoui I, et al. Maturation and persistence of the anti-SARS-CoV-2 memory B cell response. Cell (2021) 184:1201–1213.e14. doi: 10.1016/j.cell.2021.01.050
28. Klein U, Rajewsky K, Kuppers R. Human immunoglobulin (Ig)M+IgD+ peripheral blood B cells expressing the CD27 cell surface antigen carry somatically mutated variable region genes: CD27 as a general marker for somatically mutated (memory) B cells. J Exp Med (1998) 188:1679–89. doi: 10.1084/jem.188.9.1679
29. Kaneko N, Kuo HH, Boucau J, Farmer JR, Allard-Chamard H, Mahajan VS, et al. Massachusetts consortium on pathogen readiness specimen working, loss of bcl-6-expressing T follicular helper cells and germinal centers in COVID-19. Cell (2020) 183:143–57.e13. doi: 10.1016/j.cell.2020.08.025
30. Krause R, Snyman J, Shi-Hsia H, Muema D, Karim F, Ganga Y, et al. HIV skews the SARS-CoV-2 B cell response towards an extrafollicular maturation pathway. Elife (2022) 11:e79924. doi: 10.7554/eLife.79924
31. Woodruff M, Ramonell R, Cashman K, Nguyen D, Ley A, Kyu S, et al. Critically ill SARS-CoV-2 patients display lupus-like hallmarks of extrafollicular B cell activation. medRxiv (2020). doi: 10.1101/2020.04.29.20083717
32. Wheatley AK, Juno JA, Wang JJ, Selva KJ, Reynaldi A, Tan HX, et al. Evolution of immune responses to SARS-CoV-2 in mild-moderate COVID-19. Nat Commun (2021) 12:1162. doi: 10.1038/s41467-021-21444-5
33. Crawford KHD, Dingens AS, Eguia R, Wolf CR, Wilcox N, Logue JK, et al. Dynamics of neutralizing antibody titers in the months after severe acute respiratory syndrome coronavirus 2 infection. J Infect Dis (2021) 223:197–205. doi: 10.1093/infdis/jiaa618
34. Beaudoin-Bussieres G, Laumaea A, Anand SP, Prevost J, Gasser R, Goyette G, et al. Decline of humoral responses against SARS-CoV-2 spike in convalescent individuals. mBio (2020) 11:e02590-20. doi: 10.1128/mBio.02590-20
35. Rodda LB, Netland J, Shehata L, Pruner KB, Morawski PA, Thouvenel CD, et al. Functional SARS-CoV-2-specific immune memory persists after mild COVID-19. Cell (2021) 184:169–83.e17. doi: 10.1016/j.cell.2020.11.029
36. Dan JM, Mateus J, Kato Y, Hastie KM, Yu ED, Faliti CE, et al. Immunological memory to SARS-CoV-2 assessed for up to 8 months after infection. Science (2021) 371:eabf4063. doi: 10.1126/science.abf4063
37. Gaebler C, Wang Z, Lorenzi JCC, Muecksch F, Finkin S, Tokuyama M, et al. Evolution of antibody immunity to SARS-CoV-2. Nature (2021) 591:639–44. doi: 10.1038/s41586-021-03207-w
38. Figueiredo-Campos P, Blankenhaus B, Mota C, Gomes A, Serrano M, Ariotti S, et al. Seroprevalence of anti-SARS-CoV-2 antibodies in COVID-19 patients and healthy volunteers up to 6 months post disease onset. Eur J Immunol (2020) 50:2025–40. doi: 10.1002/eji.202048970
39. Yan X, Chen G, Jin Z, Zhang Z, Zhang B, He J, et al. Anti-SARS-CoV-2 IgG levels in relation to disease severity of COVID-19. J Med Virol (2022) 94:380–3. doi: 10.1002/jmv.27274
40. Luo H, Jia T, Chen J, Zeng S, Qiu Z, Wu S, et al. The characterization of disease severity associated igG subclasses response in COVID-19 patients. Front Immunol (2021) 12:632814. doi: 10.3389/fimmu.2021.632814
41. Ho MS, Chen WJ, Chen HY, Lin SF, Wang MC, Di J, et al. Neutralizing antibody response and SARS severity. Emerg Infect Dis (2005) 11:1730–7. doi: 10.3201/eid1111.040659
42. Alshukairi AN, Khalid I, Ahmed WA, Dada AM, Bayumi DT, Malic LS, et al. Antibody response and disease severity in healthcare worker MERS survivors. Emerg Infect Dis 22 (2016). doi: 10.3201/eid2206.160010
43. Takeshita M, Nishina N, Moriyama S, Takahashi Y, Uwamino Y, Nagata M, et al. Incomplete humoral response including neutralizing antibodies in asymptomatic to mild COVID-19 patients in Japan. Virology (2021) 555:35–43. doi: 10.1016/j.virol.2020.12.020
44. Lazor-Blanchet C, Zygoura P, Dafni U, Lamoth F, Tsourti Z, Lobritz MA, et al. Low neutralizing antibody titers after asymptomatic or non-severe SARS-CoV-2 infection over a 6-month assessment period. J Infect (2022) 84:722–46. doi: 10.1016/j.jinf.2022.02.001
45. Lau EHY, Tsang OTY, Hui DSC, Kwan MYW, Chan WH, Chiu SS, et al. Neutralizing antibody titres in SARS-CoV-2 infections. Nat Commun (2021) 12:63. doi: 10.1038/s41467-020-20247-4
46. Breuer A, Raphael A, Stern H, Odeh M, Fiszlinski J, Algur N, et al. SARS-CoV-2 antibodies started to decline just four months after COVID-19 infection in a paediatric population. Acta Paediatr (2021) 110:3054–62. doi: 10.1111/apa.16031
47. Long QX, Liu BZ, Deng HJ, Wu GC, Deng K, Chen YK, et al. Antibody responses to SARS-CoV-2 in patients with COVID-19. Nat Med (2020) 71:2027–34. doi: 10.1093/cid/ciaa344
48. Patel MM, Thornburg NJ, Stubblefield WB, Talbot HK, Coughlin MM, Feldstein LR, et al. Change in antibodies to SARS-CoV-2 over 60 days among health care personnel in nashville, tennessee. JAMA (2020) 324:1781–2. doi: 10.1001/jama.2020.18796
49. Ibarrondo FJ, Fulcher JA, Goodman-Meza D, Elliott J, Hofmann C, Hausner MA, et al. Rapid decay of anti-SARS-CoV-2 antibodies in persons with mild covid-19. N Engl J Med (2020) 383:1085–7. doi: 10.1056/NEJMc2025179
50. Horton DB, Barrett ES, Roy J, Gennaro ML, Andrews T, Greenberg P, et al. Determinants and dynamics of SARS-CoV-2 infection in a diverse population: 6-month evaluation of a prospective cohort study. J Infect Dis (2021) 224:1345–56. doi: 10.1093/infdis/jiab411
51. Fjelltveit EB, Blomberg B, Kuwelker K, Zhou F, Onyango TB, Brokstad KA, et al. Symptom burden and immune dynamics 6 to 18 months following mild severe acute respiratory syndrome coronavirus 2 infection (SARS-CoV-2): a case-control study. Clin Infect Dis (2023) 76:e60–70. doi: 10.1093/cid/ciac655
52. Paavonen T, Andersson LC, Adlercreutz H. Sex hormone regulation of in vitro immune response. Estradiol enhances human B cell maturation via inhibition of suppressor T cells in pokeweed mitogen-stimulated cultures. J Exp Med (1981) 154:1935–45. doi: 10.1084/jem.154.6.1935
53. Sthoeger ZM, Chiorazzi N, Lahita RG. Regulation of the immune response by sex hormones. I. In vitro effects of estradiol and testosterone on pokeweed mitogen-induced human B cell differentiation. J Immunol (1988) 141:91–8. doi: 10.4049/jimmunol.141.1.91
54. Grzelak L, Velay A, Madec Y, Gallais F, Staropoli I, Schmidt-Mutter C, et al. Sex differences in the evolution of neutralizing antibodies to severe acute respiratory syndrome coronavirus 2. J Infect Dis (2021) 224:983–8. doi: 10.1093/infdis/jiab127
55. Falagas ME, Mourtzoukou EG, Vardakas KZ. Sex differences in the incidence and severity of respiratory tract infections. Respir Med (2007) 101:1845–63. doi: 10.1016/j.rmed.2007.04.011
56. Mitchell LA, Zhang T, Tingle AJ. Differential antibody responses to rubella virus infection in males and females. J Infect Dis (1992) 166:1258–65. doi: 10.1093/infdis/166.6.1258
57. Ozgocer T, Dagli SN, Ceylan MR, Disli F, Ucar C, Yildiz S. Analysis of long-term antibody response in COVID-19 patients by symptoms grade, gender, age, BMI, and medication. J Med Virol (2022) 94:1412–8. doi: 10.1002/jmv.27452
58. Zhai B, Clarke K, Bauer DL, Moehling Geffel KK, Kupul S, Schratz LJ, et al. SARS-coV-2 antibody response is associated with age and body mass index in convalescent outpatients. J Immunol (2022) 208:1711–8. doi: 10.4049/jimmunol.2101156
59. Peckham H, de Gruijter NM, Raine C, Radziszewska A, Ciurtin C, Wedderburn LR, et al. Male sex identified by global COVID-19 meta-analysis as a risk factor for death and ITU admission. Nat Commun (2020) 11:6317. doi: 10.1038/s41467-020-19741-6
60. Serfling RE, Sherman IL, Houseworth WJ. Excess pneumonia-influenza mortality by age and sex in three major influenza A2 epidemics, United States, 1957-58, 1960 and 1963. Am J Epidemiol (1967) 86:433–41. doi: 10.1093/oxfordjournals.aje.a120753
61. Kilbourne ED. Influenza pandemics of the 20th century. Emerg Infect Dis (2006) 12:9–14. doi: 10.3201/eid1201.051254
62. Morgan R, Klein SL. The intersection of sex and gender in the treatment of influenza. Curr Opin Virol (2019) 35:35–41. doi: 10.1016/j.coviro.2019.02.009
63. Regis E, Fontanella S, Lin L, Howard R, Haider S, Curtin JA, et al. Sex differences in innate anti-viral immune responses to respiratory viruses and in their clinical outcomes in a birth cohort study. Sci Rep (2021) 11:23741. doi: 10.1038/s41598-021-03044-x
64. Kovats S. Estrogen receptors regulate innate immune cells and signaling pathways. Cell Immunol (2015) 294:63–9. doi: 10.1016/j.cellimm.2015.01.018
65. Fischinger S, Boudreau CM, Butler AL, Streeck H, Alter G. Sex differences in vaccine-induced humoral immunity. Semin Immunopathol (2019) 41:239–49. doi: 10.1007/s00281-018-0726-5
66. Demonbreun AR, Sancilio A, Velez ME, Ryan DT, Pesce L, Saber R, et al. COVID-19 mRNA vaccination generates greater immunoglobulin G levels in women compared to men. J Infect Dis (2021) 224:793–7. doi: 10.1093/infdis/jiab314
67. Ebinger JE, Joung S, Liu Y, Wu M, Weber B, Claggett B, et al. Demographic and clinical characteristics associated with variations in antibody response to BNT162b2 COVID-19 vaccination among healthcare workers at an academic medical centre: a longitudinal cohort analysis. BMJ Open (2022) 12:e059994. doi: 10.1136/bmjopen-2021-059994
68. Voysey M, Barker CI, Snape MD, Kelly DF, Truck J, Pollard AJ. Sex-dependent immune responses to infant vaccination: an individual participant data meta-analysis of antibody and memory B cells. Vaccine (2016) 34:1657–64. doi: 10.1016/j.vaccine.2016.02.036
69. Webster SE, Tsuji NL, Clemente MJ, Holodick NE. Age-related changes in antigen-specific natural antibodies are influenced by sex. Front Immunol (2022) 13:1047297. doi: 10.3389/fimmu.2022.1047297
70. Ohm M, Boef AGC, Stoof SP, van Ravenhorst MB, van der Klis FRM, Berbers GAM, et al. Sex-related differences in the immune response to meningococcal vaccinations during adolescence. Front Public Health (2022) 10:871670. doi: 10.3389/fpubh.2022.871670
71. Klein SL, Flanagan KL. Sex differences in immune responses. Nat Rev Immunol (2016) 16:626–38. doi: 10.1038/nri.2016.90
72. Capelle CM, Cire S, Domingues O, Ernens I, Hedin F, Fischer A, et al. Combinatorial analysis reveals highly coordinated early-stage immune reactions that predict later antiviral immunity in mild COVID-19 patients. Cell Rep Med (2022) 3:100600. doi: 10.1016/j.xcrm.2022.100600
73. Sparks R, Lau WW, Liu C, Han KL, Vrindten KL, Sun G, et al. Influenza vaccination reveals sex dimorphic imprints of prior mild COVID-19. Nature (2023) 614:752–61. doi: 10.1038/s41586-022-05670-5
74. Mifsud NA, Purcell AW, Chen W, Holdsworth R, Tait BD, McCluskey J. Immunodominance hierarchies and gender bias in direct T(CD8)-cell alloreactivity. Am J Transplant (2008) 8:121–32. doi: 10.1111/j.1600-6143.2007.02044.x
75. Zhang X, Castelli FA, Zhu X, Wu M, Maillere B, BenMohamed L. Gender-dependent HLA-DR-restricted epitopes identified from herpes simplex virus type 1 glycoprotein D. Clin Vaccine Immunol (2008) 15:1436–49. doi: 10.1128/CVI.00123-08
76. Xu C, Cheng S, Chen K, Song Q, Liu C, Fan C, et al. Sex differences in genomic features of hepatitis B-associated hepatocellular carcinoma with distinct antitumor immunity. Cell Mol Gastroenterol Hepatol (2023) 15:327–54. doi: 10.1016/j.jcmgh.2022.10.009
77. Yang C, Jin J, Yang Y, Sun H, Wu L, Shen M, et al. Androgen receptor-mediated CD8(+) T cell stemness programs drive sex differences in antitumor immunity. Immunity (2022) 55:1268–1283 e9. doi: 10.1016/j.immuni.2022.05.012
78. Nayrac M, Dube M, Sannier G, Nicolas A, Marchitto L, Tastet O, et al. Temporal associations of B and T cell immunity with robust vaccine responsiveness in a 16-week interval BNT162b2 regimen. Cell Rep (2022) 39:111013. doi: 10.1016/j.celrep.2022.111013
79. Elsner RA, Shlomchik MJ. Germinal center and extrafollicular B cell responses in vaccination, immunity, and autoimmunity. Immunity (2020) 53:1136–50. doi: 10.1016/j.immuni.2020.11.006
80. Vijay R, Guthmiller JJ, Sturtz AJ, Surette FA, Rogers KJ, Sompallae RR, et al. Infection-induced plasmablasts are a nutrient sink that impairs humoral immunity to malaria. Nat Immunol (2020) 21:790–801. doi: 10.1038/s41590-020-0678-5
81. Castleman MJ, Stumpf MM, Therrien NR, Smith MJ, Lesteberg KE, Palmer BE, et al. Autoantibodies elicited with SARS-CoV-2 infection are linked to alterations in double negative B cells. Front Immunol (2022) 13:988125. doi: 10.3389/fimmu.2022.988125
82. Pereira C, Harris BHL, Di Giovannantonio M, Rosadas C, Short CE, Quinlan R, et al. Antibody response to SARS-CoV-2 infection is not associated with Post-COVID-19 Syndrome in healthcare workers. J Infect Dis (2021) 223:1671–6. doi: 10.1093/infdis/jiab120
83. Joung S, Weber B, Wu M, Liu Y, Tang AB, Driver M, et al. Serological response to vaccination in post-acute sequelae of COVID. BMC Infect Dis (2023) 23:97. doi: 10.1186/s12879-023-08060-y
84. Santa Cruz A, Mendes-Frias A, Azarias-da-Silva M, Andre S, Oliveira AI, Pires O, et al. Post-acute sequelae of COVID-19 is characterized by diminished peripheral CD8(+)beta7 integrin(+) T cells and anti-SARS-CoV-2 IgA response. Nat Commun (2023) 14:1772. doi: 10.1038/s41467-023-37368-1
85. Peluso MJ, Lu S, Tang AF, Durstenfeld MS, Ho HE, Goldberg SA, et al. Markers of immune activation and inflammation in individuals with postacute sequelae of severe acute respiratory syndrome coronavirus 2 infection. J Infect Dis (2021) 224:1839–48. doi: 10.1093/infdis/jiab490
86. Cui J, Li F, Shi ZL. Origin and evolution of pathogenic coronaviruses. Nat Rev Microbiol (2019) 17:181–92. doi: 10.1038/s41579-018-0118-9
87. Cevik M, Tate M, Lloyd O, Maraolo AE, Schafers J, Ho A. SARS-CoV-2, SARS-CoV, and MERS-CoV viral load dynamics, duration of viral shedding, and infectiousness: a systematic review and meta-analysis. Lancet Microbe (2021) 2:e13–22. doi: 10.1016/S2666-5247(20)30172-5
88. Pan D, Sze S, Abraham S, Williams CM, Tang JW, Barer MR, et al. Rapid tests for quantification of infectiousness are urgently required in patients with COVID-19. Lancet Microbe (2021) 2:e286–7. doi: 10.1016/S2666-5247(21)00089-6
89. Swank Z, Senussi Y, Manickas-Hill Z, Yu XG, Li JZ, Alter G, et al. Persistent circulating SARS-CoV-2 spike is associated with post-acute COVID-19 sequelae. Clin Infect Dis (2022) 76:e487–90. doi: 10.1101/2022.06.14.22276401
90. Tejerina F, Catalan P, Rodriguez-Grande C, Adan J, Rodriguez-Gonzalez C, Munoz P, et al. Post-COVID-19 syndrome. SARS-CoV-2 RNA detection in plasma, stool, and urine in patients with persistent symptoms after COVID-19. BMC Infect Dis (2022) 22:211. doi: 10.1186/s12879-022-07153-4
91. Stein SR, Ramelli SC, Grazioli A, Chung JY, Singh M, Yinda CK, et al. SARS-CoV-2 infection and persistence in the human body and brain at autopsy. Nature (2022) 612:758–63. doi: 10.1038/s41586-022-05542-y
92. Junqueira C, Crespo A, Ranjbar S, de Lacerda LB, Lewandrowski M, Ingber J, et al. FcgammaR-mediated SARS-CoV-2 infection of monocytes activates inflammation. Nature (2022) 606:576–84. doi: 10.1038/s41586-022-04702-4
93. Hamming I, Timens W, Bulthuis ML, Lely AT, Navis G, van Goor H. Tissue distribution of ACE2 protein, the functional receptor for SARS coronavirus. A first step in understanding SARS pathogenesis. J Pathol (2004) 203:631–7. doi: 10.1002/path.1570
94. Puelles VG, Lutgehetmann M, Lindenmeyer MT, Sperhake JP, Wong MN, Allweiss L, et al. Multiorgan and renal tropism of SARS-cov-2. N Engl J Med (2020) 383:590–2. doi: 10.1056/NEJMc2011400
95. Callow KA, Parry HF, Sergeant M, Tyrrell DA. The time course of the immune response to experimental coronavirus infection of man. Epidemiol Infect (1990) 105:435–46. doi: 10.1017/S0950268800048019
96. Woldemeskel BA, Kwaa AK, Garliss CC, Laeyendecker O, Ray SC, Blankson JN. Healthy donor T cell responses to common cold coronaviruses and SARS-CoV-2. J Clin Invest (2020) 130:6631–8. doi: 10.1172/JCI143120
97. Diez-Cirarda M, Yus M, Gomez-Ruiz N, Polidura C, Gil-Martinez L, Delgado-Alonso C, et al. Multimodal neuroimaging in post-COVID syndrome and correlation with cognition. Brain Awac (2022) 384. doi: 10.1093/brain/awac384
98. Guedj E, Campion JY, Dudouet P, Kaphan E, Bregeon F, Tissot-Dupont H, et al. (18)F-FDG brain PET hypometabolism in patients with long COVID. Eur J Nucl Med Mol Imaging (2021) 48:2823–33. doi: 10.1007/s00259-021-05215-4
99. Sollini M, Morbelli S, Ciccarelli M, Cecconi M, Aghemo A, Morelli P, et al. Long COVID hallmarks on [18F]FDG-PET/CT: a case-control study. Eur J Nucl Med Mol Imaging (2021) 48:3187–97. doi: 10.1007/s00259-021-05294-3
100. Guedj E, Million M, Dudouet P, Tissot-Dupont H, Bregeon F, Cammilleri S, et al. (18)F-FDG brain PET hypometabolism in post-SARS-CoV-2 infection: substrate for persistent/delayed disorders? Eur J Nucl Med Mol Imag (2021) 48:592–5. doi: 10.1007/s00259-020-04973-x
101. Ceban F, Kulzhabayeva D, Rodrigues NB, Di Vincenzo JD, Gill H, Subramaniapillai M, et al. COVID-19 vaccination for the prevention and treatment of long COVID: A systematic review and meta-analysis. Brain Behav Immun (2023) 111:211–29. doi: 10.1016/j.bbi.2023.03.022
102. Watanabe A, Iwagami M, Yasuhara J, Takagi H, Kuno T. Protective effect of COVID-19 vaccination against long COVID syndrome: a systematic review and meta-analysis. Vaccine (2023) 41:1783–90. doi: 10.1016/j.vaccine.2023.02.008
Keywords: post covid condition (PCC), long covid, anti-RBD antibody titer, RBD specific B cells, SARS-CoV-2 infection
Citation: Limoges M-A, Quenum AJI, Chowdhury MMH, Rexhepi F, Namvarpour M, Akbari SA, Rioux-Perreault C, Nandi M, Lucier J-F, Lemaire-Paquette S, Premkumar L, Durocher Y, Cantin A, Lévesque S, Dionne IJ, Menendez A, Ilangumaran S, Allard-Chamard H, Piché A and Ramanathan S (2023) SARS-CoV-2 spike antigen-specific B cell and antibody responses in pre-vaccination period COVID-19 convalescent males and females with or without post-covid condition. Front. Immunol. 14:1223936. doi: 10.3389/fimmu.2023.1223936
Received: 16 May 2023; Accepted: 25 August 2023;
Published: 21 September 2023.
Edited by:
Maike Hofmann, University of Freiburg Medical Center, GermanyReviewed by:
Suresh Pallikkuth, University of Miami, United StatesMin Kyung Jung, Institute for Basic Science (IBS), Republic of Korea
Copyright © 2023 Limoges, Quenum, Chowdhury, Rexhepi, Namvarpour, Akbari, Rioux-Perreault, Nandi, Lucier, Lemaire-Paquette, Premkumar, Durocher, Cantin, Lévesque, Dionne, Menendez, Ilangumaran, Allard-Chamard, Piché and Ramanathan. This is an open-access article distributed under the terms of the Creative Commons Attribution License (CC BY). The use, distribution or reproduction in other forums is permitted, provided the original author(s) and the copyright owner(s) are credited and that the original publication in this journal is cited, in accordance with accepted academic practice. No use, distribution or reproduction is permitted which does not comply with these terms.
*Correspondence: Sheela Ramanathan, c2hlZWxhLnJhbWFuYXRoYW5AdXNoZXJicm9va2UuY2E=
†These authors have contributed equally to this work and share first authorship
‡These authors share senior authorship