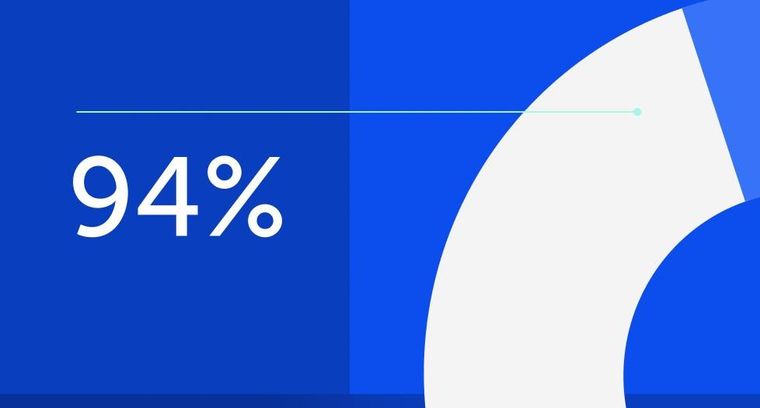
94% of researchers rate our articles as excellent or good
Learn more about the work of our research integrity team to safeguard the quality of each article we publish.
Find out more
REVIEW article
Front. Immunol., 09 October 2023
Sec. Cancer Immunity and Immunotherapy
Volume 14 - 2023 | https://doi.org/10.3389/fimmu.2023.1221097
This article is part of the Research TopicImmunotherapy and Brain Metastasis: Bridging Translational Research to the ClinicView all 7 articles
Brain metastases (BMs) are the most common form of intracranial malignant neoplasms in adults, with a profound impact on quality of life and traditionally associated with a dismal prognosis. Lung cancer accounts for approximately 40%–50% of BM across different tumors. The process leading to BMs is complex and includes local invasion, intravasation, tumor cells circulation into the bloodstream, disruption of the blood–brain barrier, extravasation of tumor cells into the brain parenchyma, and interaction with cells of the brain microenvironment, among others. Once the tumor cells have seeded in the brain parenchyma, they encounter different glial cells of the brain, as well as immune cells. The interaction between these cells and tumor cells is complex and is associated with both antitumoral and protumoral effects. To overcome the lethal prognosis associated with BMs, different treatment strategies have been developed, such as immunotherapy with immune checkpoint inhibitors, particularly inhibitors of the PD-1/PD-L1 axis, which have demonstrated to be an effective treatment in both non-small cell lung cancer and small cell lung cancer. These antibodies have shown to be effective in the treatment of BM, alone or in combination with chemotherapy or radiotherapy. However, many unsolved questions remain to be answered, such as the sequencing of immunotherapy and radiotherapy, the optimal management in symptomatic BMs, the role of the addition of anti–CTLA-4 antibodies, and so forth. The complexity in the management of BMs in the era of immunotherapy requires a multidisciplinary approach to adequately treat this devastating event. The aim of this review is to summarize evidence regarding epidemiology of BM, its pathophysiology, current approach to treatment strategies, as well as future perspectives.
Brain metastases (BMs) are the most common form of intracranial malignant neoplasms in adults and are associated with a dismal prognosis (1–3). Lung cancer is the most frequent primary tumor to develop BM (4) and globally accounts for approximately 40%–50% of all BMs (1, 5). Different risk factors have been associated to lung cancer, namely, cigarette smoking history, radon exposure, and occupational exposure to agents such as arsenic, asbestos, beryllium, cadmium, chromium, coal smoke, diesel fumes, nickel, silica, soot, and uranium. Other risk factors include history of prior malignancies such as lymphoma or head and neck cancers, history of chronic obstructive pulmonary disease, or pulmonary fibrosis (6). Among the different subtypes of lung cancer, it is estimated that up to 22%–57% of patients with non-small cell lung cancer (NSCLC) will develop BMs at some point of the history of their disease (7–10). Overall, 20% of these patients present BM at diagnosis and up to 57% develop BM over the period of the disease (7, 11). The prevalence of BMs in a subset of patients with NSCLC and oncogenic drivers can be higher in some cases and increase throughout the course of their disease. These patients experience different rates of BM at baseline and through the history of their disease, as follows for the most frequent oncogenic drivers: EGFR mutations are 24.4% and 52.9% (12), ALK rearrangements are 23.8% and 72% (12, 13), and ROS1 rearrangements are 19.4% and 34% (13). Regarding the less common genomic alterations, the percentages for baseline BM and throughout the history of the disease are as follows: BRAF V600E mutations are 31% (14) and not reported (possibly due to the low prevalence of BRAF mutations in NSCLC), HER2 mutations are 14% and 28% (15, 16), RET rearrangements are 25% and 46% (17), and KRAS mutations are (G12C and non-G12C) 24% and 35.1% (15, 18). Some patients with oncogenic drivers and BMs benefit from targeted therapies with tyrosine kinase inhibitors, which can surpass the blood–brain barrier (BBB), as evidenced by significant intracranial response rates in different clinical trials (19–22). For patients with small cell lung cancer (SCLC), it is estimated that 10%–20% of patients will present with BM at diagnosis, and up to 50%–80% of patients will develop BMs at some point of the history of their disease (23–26).
Detecting BM at baseline has been associated to a worse prognosis, and developing BM during ongoing therapies reflects tumor progression and resistance to treatment, associated to the disruption of the physiologic BBB.
Current guidelines recommend the use of brain magnetic resonance imaging (MRI) with contrast or, when not possible, brain computed tomography with contrast for patients with NSCLC and SCLC stages II–IV to detect BM (27, 28). Although BMs have a negative impact in survival, in recent years, patients’ outcomes have improved significantly (29, 30). Some of the treatments that have contributed to improving survival of these patients include surgery, stereotactic radiosurgery, whole-brain radiation therapy, prophylactic cranial irradiation, and the addition of better systemic therapies (10, 31–33). To develop effective treatment strategies, it is necessary to understand the genomic and molecular mechanisms leading to BM. The purpose of this review is to provide an integrated synthesis of the epidemiology of BM in lung cancer patients. To describe its pathophysiology and molecular mechanisms, as well as its genomic landscape. Furthermore, we intend to provide a cohesive summary of the clinical evidence for the different treatment strategies, focusing on immunotherapy, and immunotherapy based combinations. Finally, we present future perspectives for the management of BMs.
The mechanisms leading to BMs are complex and different mechanisms are required; among them, local invasion, intravasation, survival in circulation, extravasation, and tissue colonization represent the most remarkable (Figure 1A) (34, 35). The most difficult steps in this scenario are extravasation and colonization, since the BBB must be bypassed (35). Under physiological conditions, the BBB regulates the homeostasis of the central nervous system (CNS); it provides the brain with nutrients, limits the transportation of ionic substances and other molecules, and functions as a critical obstacle for drug transportation to the brain (36–38). Key components of the BBB include endothelial cells, pericytes, astrocytes, and the extracellular matrix. Endothelial cells form tight junctions that control transportation and entry of different molecules, allowing a restricted permeability of the BBB (37, 39, 40). These cells secrete growth molecules such as PDGF-β, TGF-β, and Vascular Endothelial Growth Factor (VEGF), which modulate pericytes (40). Pericytes wrap blood vessels through their end feet processes and regulate the vascular function. They are involved in angiogenesis, neovascularization, and antigen cell presentation (under certain conditions), as well as communicating with astrocytes within the neurovascular unit (41–43). Astrocytes are necessary for different metabolic processes in the brain, including metabolization of different substances and production of antioxidants, as well as regulation of signaling pathways. They contribute to the function of the BBB and secrete growth factors targeted to the endothelial cells (40). Another important component of the BBB is the extracellular matrix, composed of the basal lamina that surrounds the BBB (43). Disruption of the extracellular matrix has been associated with an increased permeability of the BBB and as a consequence development of BM (40). In addition to the BBB, other components of the CNS environment include oligodendrocytes and microglia; the latter are the innate macrophage cells of the brain and can exert disrupting effects in the BBB, facilitating the formation of BM (43, 44).
Figure 1 Establishment of brain metastases. (A) Mechanisms of tumor cell invasion, intravasation, survival in circulation, extravasation, and tissue colonization. (B) Mechanisms associated to the pre-metastatic niche including factors such as VEGF, PLGF, and MMP (matrix metalloproteinases). (B) Tumor-secreted exosomes containing nucleic acids such as miRNAs and lncRNAs, as well as proteins and lipids. (C) Contributing factors that increase permeability of the BBB including alterations in channel transporters, loss of tight junctions, and collagen produced by pericytes. (D) The BBB breakdown and the establishment of the BTB reflected by tumor cell extravasation into the brain parenchyma.
Prior to the development of BM, an adequate microenvironment must be secured for the establishment of the metastatic cells; this pretumoral microenvironment is referred to as the premetastatic niche (Figures 1A, B) (45). Different molecules contribute to the formation of the premetastatic niche, as well as to the disruption of the BBB, including VEGF, PLGF, and matrix metalloproteinases, which elicit changes in the tight junctions leading to a disruption of BBB (46–48). Other contributing mechanisms accounting for the conditioning of the premetastatic niche are tumor-secreted exosomes, which are vesicles surrounded by a bilayer of lipids. Exosomes contain nucleic acids, lipids, and proteins, which can regulate integrins and modulate glucose uptake in astrocytes (49, 50). MicroRNAs (miRNAs) are a type of nucleic acids contained in exosomes; these are characterized by being small non-coding RNAs that can alter gene expression. Different miRNAs have been associated to the development of BM, such as miR-378, which is relevant to the promotion of cell migration, invasion, and angiogenesis via upregulation of PRKCA (51–53). A different type of nucleic acids involved in BMs are long non-coding RNAs (lncRNAs), which are RNA sequences longer than 200 nucleotides, which are not translated into functioning proteins (54). The lncRNA MALTA1 has been associated with promoting BMs by inducing epithelial to mesenchymal transition (EMT), whereas the lncRNA HOTAIR contributed to BMs by increasing cell migration and anchorage (55, 56). In addition, contributing factors to the disruption of the BBB can be attributed to endothelial cells, which express different adhesion molecules prompted by tumor cells, facilitating invasion conditions for these tumor cells (57).
Once the BBB has been disrupted, the blood–tumor barrier (BTB) is established (Figures 1C, D). The BTB is characterized by alterations in the transportation of molecules, changes in protein expression profiles involving transportation channels, and a heterogeneous permeability to different molecules (44). However, this increased permeability does not necessarily translate into a more favorable anti-cancer drug delivery (44). As a former BBB, the BTB presents similar components, such as endothelial cells, which in the BTB scenario upregulate TNF receptors 1 and 2 and develop alterations in cell adhesion proteins leading to a loss of tight junctions. The loss of endothelial tight junctions increases paracellular permeability and downregulates specific transporters related to influx and efflux of molecules (58). Other cells that contribute to the disruption of the BBB are pericytes, which locate around blood vessels, forming double layers of cells and producing collagen, which leads to a thickening of blood vessels (59). An upregulation in the expression of VEGF in the BTB has been described; however, it does not always translate into an effective formation of blood vessels (60, 61). Other components associated with the establishment of the BTB include the production of inflammatory molecules, membrane proteins, and growth factors, such as claudin-5 (62, 63). Following the disruption of the BBB and the formation of the BTB, metastatic cells can then settle in the brain. Once they are established, tumor cells interact with the innate microenvironment of the brain. The tumor microenvironment is a complex ecosystem composed of tumor cells, as well as non-malignant cell types such as endothelial cells, astrocytes, pericytes, and immune cells (64).
Once, in the brain parenchyma, the tumor cells trigger an inflammatory response led by astrocytes, microglia, and other immune cells (65). Astrocytes are able to exert both pro-tumoral and antitumoral functions (66). Initially astrocytes exert antitumoral effects through the production of nitric oxide and plasminogen activator, as well as creating an astrocytic wall, which separates the metastasis from the brain parenchyma (67–69). Plasminogen activator triggers the production of plasmin, which activates the FasL pathway and deactivates L1CAM, ultimately suppressing BM. This results in a death signal for cancer cells and inhibition of metastatic growth. In response to the deleterious effects exerted by plasmin to the tumor cells, the latter secrete serpins which elicit inhibitory effects against the plasminogen activator and therefore counteract the antitumoral functions of astrocytes (68, 70). A worth noting pro-tumoral effect of astrocytes is the ability to establish gap junctions with tumor cells, facilitating the transfer of cGAMP to astrocytes. Consequently, the STING pathway is activated, leading to the production of cytokines such as IFN-α and TNF, promoting tumor cell growth and chemoresistance. Additionally, these gap junctions hinder the uptake of calcium by tumors, further contributing to tumor growth and chemoresistance (71–73). In addition, reactive astrocytes (RAs) are astrocytes that undergo changes in their molecular, morphological, and functional states as response to pathologic situations in the surrounding environment (74). Tumor cells promote the activation of STAT3 in RA, contributing to the modulation of the immune system, and promote the establishment of a pro-metastatic environment through a decrease in the activation of CD8+ T cells (75). RA may also contribute to tumor proliferation through the secretion of IL-6, TNF- α, and IL-1β (69).
The microglia are the resident macrophages of the CNS and not derived from the bone marrow. However, bone marrow–derived macrophages can reach the CNS when the BBB is disrupted, as a response to disturbances in the CNS. Both of them share lineage and activation markers, and in the context of malignant neoplasms, these cells are referred to as tumor-associated macrophages (TAMs) (76, 77). These macrophages can be subsequently divided according to their functional characteristics; M1-like macrophages are usually described to be proinflammatory and are stimulated by Toll-like receptor ligands, as well as IFN-γ and TNF-α. They are thought to exert tumor-suppressive functions, through the production of IL-1, IL-12, and nitric oxide, among other factors (78, 79). On the other hand, M2-like macrophages are usually considered anti-inflammatory; are activated by IL-4 and IL-13; and produce molecules such as TGF-β, arginase, IL-10, and profibrotic factors. M2-like macrophages are associated with tumor promoting functions through the inhibition of CD8+ T-cell proliferation (78, 79) (Figure 2).
Figure 2 The tumor microenvironment of brain metastases. The figure different types of cells present in the tumor microenvironment and its anti- and protumoral effects.
Tumor-infiltrating lymphocytes (TILs) in BMs are usually composed of different subsets of CD8+ T cells and CD4+ T cells, including T-regulatory cells (Tregs) (80). TILs in BM have been associated to peritumoral edema in the brain. Some of the main TILs present in BMs include CD3+, CD8+ cytotoxic TILs, and CD45RO+. A high density of these subpopulations has been associated with a prolonged overall survival (OS) (81). For patients with metastatic SCLC, resection of BM is not a standard of practice; thus, characterization of BM in these patients is not often achieved (82). However, a study in 32 patients with SCLC and resected BM studied the immune microenvironment of these metastases. CD3+ TILs, CD8+ TILs, CD45RO+ TILs, and FOXP3 + TILs were present in over 45% of the studied specimens. PD-L1 expression was positive in 75% of the studied BM, whereas for TILs and TAMs, the percentage of expression was 25% and 28.1%, correspondingly. The expression of PD-L1 on TILs was associated with improved survival (83). In a different study including 49 patients with NSCLC evaluated peripheral blood immune cells, including immunosuppressive monocytes, myeloid-derived suppressor cells, and Tregs. The study found that patients with BM had an increased number of PD-L1+ monocytes, myeloid-derived suppressor cells, and Tregs in peripheral blood, compared to patients who did not have BM and healthy controls. The study also found that patients with elevated numbers of peripheral myeloid PD-L1–expressing cells were associated with a worse progression-free survival and OS (84).
Different studies have evaluated primary NSCLC tumors and paired BM to assess the tumor microenvironment, as well as the expression and mutational profiles in both samples. A study in 39 patients with NSCLC and resected BM performed DNA sequencing of 158 hotspot mutations, TCR-β sequencing analysis, as well as immunohistochemistry staining to assess differences and similarities between the studied specimens. The variant allelic frequency of mutated genes was higher in BM compared to primary tumors, with a median difference of 21.6%. BM showed an inhibition of dendritic cell maturation, Th1 immune response, as well as leukocyte extravasation signaling, and a reduced expression of the adhesion molecule VCAM1. Furthermore, a bioinformatic evaluation of the immune subpopulations based on gene expression detected that the abundance of Th1 or CD8 T genes was lower in BM compared to primary tumors, which was associated with reduced levels of CD8+ T, dendritic cells, and macrophages in BM. The T-cell richness and T-cell densities were significantly lower in BM; however, levels of bone marrow–derived M2-like macrophages were higher in BM. The results from this study suggest that the immune tumor microenvironment of BM presents increased immunosuppressive features compared to the one in primary tumors (85). Another study in 23 patients with matched primary NSCLC and BM identified that PD-L1 expression in tumor cells was higher and CD8+ TILS was lower in BM compared to primary tumors. However, patients categorized as CD8 high stromal TILs in BM had a trend toward a better OS, compared to those with CD8 low stromal TILs (86). In addition, a study involving 43 NSCLC patients evaluated samples of matched primary tumor and BM, identifying higher numbers of neutrophils, CD4+ T cells and dendritic cells, and lower fractions of M1-like macrophages and Tregs in BM. The study also detected a downregulation in the expression of CTLA-4 in BM, as well as lower expression of PD-L1 and CD8A between primary tumors and BM. Interestingly, an analysis between patients with metachronic tumors who received adjuvant treatment and later had a brain relapse, compared to those who were not exposed to prior treatments and later had a brain relapse, showed no differences except for the cell fraction of natural killer cells, which were higher in the group that received adjuvant treatment. Furthermore, this study identified that the immune microenvironment differed between primary tumors and BM in the metachronic group compared to the synchronic group. The results of this study concur with the previous ones in detecting an immunosuppressed tumor microenvironment in BM, compared to the primary tumor (87). A study in 34 patients with NSCLC and BM, who underwent surgical resection or autopsy, compared primary tumors with BM. This study found that only CD204+ cells, corresponding with macrophages, were higher in tumor areas of BM compared to the primary tumor, whereas CD4+ T cells, CD8+ T cells, and CD+ FOXP3 T cells were higher in the primary tumor. The study found that higher densities of CD4+ T cells and CD8+ T cells in both BM and the stromal areas of BM were associated with a higher OS (88).
A study compared the expression of PD-L1 in primary and metastatic lesions; 747 BM samples from patients with NSCLC were included. The study compared the expression of PD-L1 between primary tumors and BM and found a discordance in the expression of PD-L1. BM had a low or negative expression of the biomarker, highlighting the heterogeneity of PD-L1 according to the tumor sample site (89).
Other studies have focused on genomic profiling of NSCLC primary tumors and BM. As such, a study found that non-synonymous tumor mutational burden (TMB) was higher in BM compared to primary tumors; however, the neoantigen load was similar in BM and primary tumors. The study also found a reduced T-cell heterogeneity in BM associated to a immunosuppressive tumor microenvironment (90). A different study profiled BM and primary tumor samples from patients with NSCLC and identified a discrepancy in the mutational landscape between the samples, with a median of 8.3% of shared genetic mutations and higher numbers of somatic mutations in BM. Different pathways were enriched in BM compared to primary tumors, including invasion/metastasis and metabolic associated pathways. The study also found a phylogenetic divergence between BM and primary tumors, indicating parallel progression models for each of the lesions (91). Another study in 11 patients with metastatic lung adenocarcinoma, assessed the mutational landscape of primary tumors and BM. The study found that more unique mutations were present in BM, and identified mutations in FAM129C and ADAMTS present specifically in BM. Alterations in the APOBEC signature were also higher in BM compared to primary tumors (92). A different study performed in samples from 2,309 patients with lung adenocarcinoma, including 238 samples of CNS metastases identified genes associated to the development of BMs. Alterations in the Hippo pathway were associated to a shorter time to developing CNS metastases, and STK11 alterations were more prevalent in CNS metastases (93). Another study evaluated 73 samples of BM from lung adenocarcinoma and compared them against a control population of 503 primary lung adenocarcinoma tumors. The study found different genes that were considered as candidates for the development of BM through the identification of regions with higher amplification frequencies or deletions. These genes included MYC, YAP1, MMP13, and CDKN2A/B (94). A study in 51 patients with lung adenocarcinoma, as well as squamous cell carcinoma, identified that BM had a higher number of somatic copy number alterations. Gene alterations associated with BM driving mechanisms include CDK12, DDR2, ERBB2, and NTRK1 (95). A different study in 54 paired samples from BM and NSCLC patients identified that most driver alterations were present both in primary tumor and BM. Private alterations to one or the other sites were present in 22%–26% of the cases. KRAS mutations were more frequent in primary tumors that developed BM compared to data from The Cancer Genome Atlas (CTGA) (96). The preceding studies highlight that BM are composed of an immunosuppressive tumor environment, which has been associated to a deleterious prognosis. Furthermore, different mutational patterns in primary tumors versus BMs represent the tumor heterogeneity between the two tumor sites and could in part explain the development of BM and the different response to therapy. The most relevant results from these studies are presented in Table 1.
Table 1 Comparison of differentially expressed and regulated genes, molecules, signatures, and cell in primary tumor versus brain metastases.
Different strategies have been used to approach treatment of BM; however, there is not a definitive consensus regarding the sequence of treatment. Neurosurgery should be considered in patients with large tumors that exert a mass effect as well as in patients with minimal intracranial disease. Patients with multiple BM or a single BM could be considered for whole brain radiotherapy (WBRT) or stereotactic radio surgery (SRS) or stereotactic radiotherapy (SRT). However, radiotherapy is not recommended for patients with a Karnofsky Performance Status (KPS) ≤50 or <70 and no systematic therapy options. The use of WBRT, SRS, or SRT can also be considered for patients with a KPS <70 or with comorbidities impeding neurosurgery. Combination of SRS plus WBRT (with our radioprotective strategies) is associated with higher rates of cognitive impairment. Thus, the combination of SRS with WBRT could be used with hippocampal avoidance or administration of memantine to decrease neurological toxicity. In some instances, local treatment can be deferred to avoid potential adverse effects. Situations that might prompt to a deferral for local treatments include asymptomatic BM and a “favorable” location of the BM and systemic treatments with high CNS penetrance and responses (i.e., third-generation EGFR tyrosine kinase inhibitors) (97). Following local treatment strategies, a systemic treatment should be considered, such as immunotherapy, tyrosine kinase inhibitors, chemotherapy, or combination therapies. It is worth noting that administering concurrent radiotherapy to the brain and chemotherapy is not recommended, since it can trigger the development of cognitive impairment, white matter damage, and radionecrosis (98–100).
More recently, in the era of immunotherapy, PD-1 or PD-L1 inhibitors alone or in combination with chemotherapy or anti–CTLA-4 agents have demonstrated activity both NSCLC and SCLC patients, with BM (27, 82, 101–103). The use of Immune checkpoint-inihbitors (ICI) is an attractive strategy to stimulate the immune response of the immunosuppressed tumor microenvironment in BM (104, 105). ICI has demonstrated to be effective in the treatment of BM in other tumors including melanoma, which is coined one of the pioneers of immunotherapy. Clinical trials have demonstrated that patients with asymptomatic BM benefit from the nivolumab–ipilimumab combination, reporting an intracranial benefit of 57%, and an OS at 3 years of 71.9% in this population (106–108). Different clinical trials and retrospective studies have evaluated the effect of ICI alone or in combination with chemotherapy or radiotherapy in BM from lung cancer; these studies are summarized in Table 2. The current use of immunotherapies is based on regulation from medical agencies, as well as clinical guidelines. Current approvals can be summarized as follows: for tumors with a PD-L1 expression 0%–49% treatment recommendations include platinum-based chemotherapy with anti–PD-1/PD-L1 ± ipilimumab ± bevacizumab (141). Tumors with a PD-L1 expression ≥50% benefit from the prior mentioned regimens or anti–PD-1/PD-L1 agents in monotherapy. For patients with SCLC, the current recommendations are the use of chemoimmunotherapy in the first line of treatment (82).
The development of different therapies for lung cancer and BM can be represented in a timeline, as depicted in Figure 3 (142, 143).
Figure 3 Temporal evolution of advancements used to treat brain metastases. WBRT, whole brain radiotherapy; SRS, stereotactic radiosurgery.
Among the different clinical trials evaluating ICI monotherapy, the OAK study included 1,225 pretreated NSCLC patients and allowed for the inclusion of patients with treated and asymptomatic CNS BM (n = 123). The study compared atezolizumab versus docetaxel. Patients with BM who were treated with atezolizumab exhibited a trend toward improved OS compared to those who received docetaxel. The OS were 16 and 11.9 months (HR = 0.74, 95% CI: 0.49–1.13; p = 0.1633), for the atezolizumab and docetaxel groups, respectively, in patients with BM. OS estimates at 2 years were 26.6% (95% CI: 15.1–38.1) in the atezolizumab arm and 19.3% (95% CI: 8.2–30.4) in the docetaxel arm in patients with BM (109). The FIR study was a single-arm trial, which evaluated the effect of atezolizumab in 138 previously treated NSCLC patients, with PD-L1–selected tumors (i.e., >5% PD-L1 staining in TC or IC) and allowed the inclusion of patients with treated and asymptomatic BM (n = 13). Patients with BM had an objective response rate (ORR) of 23% (range: 5–54), the median progression-free survival (mPFS) was 2.5 months (range: 1.0–11.3), and the OS was 6.8 months (range: 3.2–19.4). The study found that the activity of atezolizumab regarding ORR and OS was similar between patients with and without BM (111).
A study by Goldberg et al, evaluated the use of pembrolizumab in pretreated patients with NSCLC and untreated BM. In 37 patients with a PD-L1 expression of at least 1% in mononuclear inflammatory cells and tumor cells, the ORR was 18.9% (95% CI: 8.0–35.1), the mPFS was 9 months (95% CI 1.8–3.7), the median OS (mOS) was 9.9 months (95% CI: 7.5–29.8), and the estimated 2-year OS was 34% (range: 21–54) (114, 144).
Wakuda et al. studied the effect of pembrolizumab in treatment naïve NSCLC patients with PD-L1 TPS ≥50, with treated and untreated BM (n = 23) and without BM (n = 64). The study found no significant differences regarding ORR in patients with and without BM (54% and 60%, p = 0.77). However, patients with treated BM had an ORR of 77%, whereas patients with untreated BM had an ORR of 60% (p = 0.21) mPFS were 6.5 months (95% CI: 0.5–not reached), and 5.3 months (95% CI: 0.4–10.8) for patients with and without BM (116).
An Italian study derived from an expanded access program evaluated the use of nivolumab in 1,588 pretreated patients with non-squamous NSCLC, with unselected PD-L1, and allowed the inclusion of patients with BM if they were asymptomatic (n = 409). The ORR for patients with BM was 17%, the disease control rate (DCR) was 39%, the mPFS was 3 months (95% CI: 2.7–3.3), and the mOS was 8.6 months (95% CI: 6.4–10.8) (117).
A study by Watanabe et al. evaluated nivolumab in 48 patients with NSCLC with and without CNS metastases. The study found a systemic ORR for patients with CNS metastases of 11% and 17% for patients without BM; mPFS were 1.8 months and 3.63 months, respectively. Another study by Debieuvre et al, including 2,858 patients with pretreated NSCLC, evaluated the effect of nivolumab; the study included 477 patients with baseline BM. The study did not find differences regarding OS in patients with or without BM (p > 0.05) (120). A different study by Assié et al. evaluated the effect of nivolumab in 10,452 NSCLC patients, including 1,800 with baseline BM. The study found that patients with BMs tended to be younger, more frequently female, with a non-squamous tumor histology and with higher prevalence of malnourishment compared to those without BM. The mOS for patients with BM was 9.9 months (range: 9–10.9), compared to 11.7 months (range: 11.3–12.2) in the overall population (121). An Italian study by Grossi et al, in 1,588 previously treated PD-L1 unselected non-squamous NSCLC patients, including 409 patients with asymptomatic and controlled CNS metastases, evaluated the efficacy of nivolumab. Patients with BM had an ORR and DCR of 17% and 39%, whereas the mPFS and mOS were 3 months (95% CI: 2.7–3.3) and 8.6 months (95% CI: 6.4–10.8), respectively. The study found that patients with BM had higher rates of liver metastases and had received two or more prior lines of therapy, both of which were statistically significantly different from patients without BM (117). A different Italian study by Cortinovis et al. studied the effect of nivolumab in 37 previously treated squamous NSCLC patients with CNS metastases. The study found an ORR of 19%, an mPFS of 4.9 months (95% CI: 2.7–7.1), and a mOS of 5.8 months (95% CI: 1.9–9.8).
As previously described, treatment with ICI monotherapy is an effective therapy for NSCLC patients with BM, however the only prospective evidence comes from the trial by Goldberg et al, in which pembrolizumab demonstrated a benefit in this subset of patients. Regarding both retrospective and prospective studies, PFS and OS ranged from 1.9 to 4.9 months and 5.8 to 21.6 months for patients with BM, respectively. It is worth noting that patients with BM treated locally, who were systemic treatment naïve at the start of the ICI therapy were the ones who had obtained the best survival outcomes. However, these data must be analyzed cautiously, since each study had a different design and the baseline characteristics of the patients were different.
Other treatment strategies involve the use of immunotherapy–immunotherapy combinations; the CheckMate 227 clinical trial evaluated the effect of nivolumab plus ipilimumab compared to chemotherapy in 1,166 chemotherapy-naïve NSCLC patients. Inclusion was based on a TMB of at least 10 mt/Mb, regardless of PD-L1 expression, patients with treated asymptomatic BM were allowed (n = 135). The ORR was 33% and 26%, mPFS 5.4 months (3.1–8.6) and 5.8 months (4.3–8.0) with a HR (95% CI) of 0.79 (0.52–1.19), and mOS 18.8 months (9.2–29.4), and 13.7 months (10.5–16.2) with a HR (95% CI) of 0.57 (0.38–0.85), for nivolumab plus ipilimumab and chemotherapy, respectively (124). A study by Hendriks et al. evaluated the use of ICI monotherapy or ICI combinations in 1,025 patients with NSCLC, of which 255 had BM, including active and symptomatic. Patients with and without BM had an ORR of 20.6% and 22.7% (p = 0.484) and a DCR of 43.9% and 52% (p = 0.024), respectively; the intracranial response of patients with active BM was 27.3%. The mPFS for patients with and without BM were 1.7 months (95% CI: 1.5–2.1) and 2.1 months (95% CI: 1.9–2.5) (p = 0.009), whereas the mOS were 8.6 months (95% CI: 6.8–12), and 11.4 months (95% CI: 8.6–13.8) (p = 0.035), respectively. Patients who previously received cranial radiotherapy did not have a different outcome in terms of OS compared to those who did not (125).
Although a smaller number of patients was included in ICI–ICI combination studies, there seems to be a greater benefit than with ICI monotherapy; however a conclusion should not be drawn since the populations compared were not the same.
Different clinical trials have evaluated the effect of immunotherapy-plus chemotherapy in the treatment of NSCLC patients, including those with BM. The study ATEZO-BRAIN evaluated the effect of atezolizumab, carboplatin, and pemetrexed in 40 previously untreated non-squamous NSCLC patients with untreated BM and unselected PD-L1 expression. The study reported an intracranial response of 40%, a systemic response in 47.5% of the patients; the median systemic PFS was 8.9 months (6.7–13.8), intracranial PFS was 6.9 months (4.7–11.9), and mOS was 13.6 months (9.72–not reached). Patients with a positive PD-L1 tended to present an increased benefit regarding survival (126).
The KEYNOTE-189 clinical trial evaluated the effect of the combination of pembrolizumab, platinum-based chemotherapy, and pemetrexed compared to placebo, platinum-based chemotherapy, and pemetrexed in 616 previously untreated non-squamous NSCLC patients. The study allowed for any level of PD-L1 expression and the inclusion of patients with asymptomatic BM (n = 108). Patients with BM had mPFS of 6.9 months (5.4–11.0) and 4.7 months(2.2-5.5), HR: 0.42 7.5 and the mOS were 19.2 months (15.0–25.9) and 7.5 months (4.6–10.0), HR: 0.41 (0.24–0.67) for the pembrolizumab and the placebo combinations, respectively (127).
A study by Sun et al. evaluated the effects of pembrolizumab alone or in combination with chemotherapy in patients with NSCLC with (n = 126) and without BM (n = 444); the study included patients with treated and untreated BM. Patients with BM had an intracranial ORR of 36.4%; the systemic ORR was 27.8% for patients with BM and 29.7% for patients without BM (p = 0.671). The estimated mPFS for patients with BM was 9.2 months, and for patients without BM was 7.7 months (p = 0.609); the estimated mOS were 18 and 18.7 months (p = 0.966), respectively. The study found that patients with treated BM had a significantly improved mPFS and mOS compared to those with untreated BM. However, the study did not report the differences in terms of response or survival comparing pembrolizumab monotherapy versus pembrolizumab plus chemotherapy (129). A pooled analysis of the KEYNOTE 021, 189, and 407 clinical trials by Powell et al. evaluated the effect of platinum-based chemotherapy with or without pembrolizumab, in 1,298 treatment naïve patients with NSCLC and baseline BM (n = 171) including treated and stable or untreated and asymptomatic BM. Patients with BM who received pembrolizumab based treatment had an ORR of 39% (95% CI: 29.7–49.1), compared to 19.7% (95% CI: 10.9–31.3%) in patients who received the chemotherapy-based treatment, the mPFS were 6.9 months (95% CI: 5.7–8.9) and 4.1 months (95% CI: 2.3–4.6) for each group, respectively, with a HR: 0.44 (95% CI: 0.31–0.62). The mOS were 18.8 months (95% CI: 13.8–25.9) and 7.6 months (95% CI: 5.4–10.9) with a HR for death of 0.48 (95% CI: 0.32–0.70) for the pembrolizumab-plus chemotherapy and chemotherapy treatment groups. Patients without BM had a better ORR, mPFS, and mOS than patients with BM in both treatment groups.
A different strategy was evaluated in the CheckMate 9LA, in which 1,150 treatment naïve NSCLC patients, were randomized to receive nivolumab, plus ipilimumab with two cycles of chemotherapy versus four cycles of chemotherapy alone. The study allowed for any expression of PD-L1 and for patients with treated asymptomatic CNS metastases. The ORRs for the whole population were 38.2% (33.2–43.5) and 24.9% (20.5–29.7); the mOS for patients with CNS metastases was 19.9 months versus 7.9 months, HR: 0.47 (95% CI: 0.31–0.71), for the immunotherapy-based treatment and the chemotherapy arm, respectively (131).
Both prospective and retrospective studies show a clear benefit in the use of chemoimmunotherapy combinations for patients with NSCLC and BM, regardless of the ICI used.
Results from these trials suggest that this combination therapy might be more effective than ICI monotherapy, however, as previously stated comparisons among different trials should not be performed.
Studies evaluating the effect of chemoimmunotherapy in SCLC patients allowing the inclusion of BM include the IMpower 133 and the CASPIAN clinical trial.
The IMpower133 evaluated the use of atezolizumab, carboplatin, and etoposide, compared to carboplatin and etoposide in 403 treatment naïve SCLC patients, allowing the inclusion of patients with treated asymptomatic BM (n = 35). The study reported that patients with BM had no different outcomes regarding PFS and OS regardless of the treatment, with a HR for death (95% CI) of 1.07 (0.47–2.43) (145).
The CASPIAN clinical trial evaluated the use of durvalumab in combination with platinum-based chemotherapy versus platinum-based chemotherapy in 268 treatment naïve SCLC. Patients with untreated asymptomatic or treated and stable BM were allowed (n = 55), only patients in the chemotherapy-based arm were allowed to receive PCI. The mPFS was 4.7 months (range: 4.4–6.4) for the immunotherapy-based arm and 4.5 months (range: 4.5–5.9) for the chemotherapy-based arm, with an unstratified HR of 0.73 (95% CI: 0.42–1.29). The mOS for the durvalumab group was 11.7 months (8.3–16.3), and 8.8 months (5.7–11.9) for the chemotherapy arm, with an unstratified HR of 0.79 (95% CI: 0.44–1.41). The study reported that the durvalumab arm prolonged OS in patients with and without BM (134, 135). A meta-analysis studied the addition of ICI to chemotherapy versus chemotherapy as monotherapy in the first treatment of extensive stage SCLC; the study found that the only OS benefit from the addition of ICI was in patients without BM at diagnosis. These results are possibly associated to the fact that there is few information available for patients with BM (146). Larger real world data studies are expected to report the efficacy of chemoimmunotherapy combinations in SCLC patients with BM.
Combination strategies with radiotherapy and immunotherapy could lead to a synergy through the release of danger associated molecular patterns (DAMPs) and cytokines, thus increasing neoantigen presentation and diversifying the T-cell repertoire. However, combining these therapies could also potentiate the incidence of adverse events. Different retrospective studies have evaluated the effect of these therapy combinations in BM from NSCLC (147). A study by Hubbeling et al. included 163 patients with NSCLC and BM who received either radiotherapy alone (n = 113) or in combination with ICI (n = 50). In patients who received both treatments, radiotherapy was more frequently administered prior to ICI, compared to concurrent administration. The study reported similar rates of radiotherapy related toxicity in both groups, thus, suggesting that ICI administered with radiotherapy may not be related to an increased the risk of toxicity (136). In another study by Schapira et al, 37 patients with NSCLC patients and BM were evaluated to assess the effect of the treatment with anti–PD-1 inhibitors and stereotactic radiosurgery (SRS). The study found that patients treated concurrently with ICI and SRS had an improved OS, compared to those treated with SRS before or after ICI, the 1-year OS were 87.3%, 70.0%, and 0% (p = 0.008) for each treatment, respectively. Distant brain failure (i.e., the emergence of a new BM or tumor progression outside the prior radiation treatment field in the brain) was lower in patients treated with concurrent SRS and ICI, compared to those with sequential treatment. The study found a numerically higher frequency of radiation associated toxicities in patients who received the concurrent treatment (137, 148). A different study by Enright et al. evaluated the use of SRT combined with (n = 33), or without (n = 44) ICI in NSCLC patients with BM. Patients who received both treatments had a decreased distant brain failure, decreased rates of death by neurological causes, as well as a better OS compared to patients who received radiotherapy without ICI; the mOS for the global cohort was 13.9 months (range: 1.1–61.6). Regarding toxicities, symptomatic brain radionecrosis was more frequent in patients receiving radiotherapy alone (11.3%) compared to ICI plus radiotherapy (6%) (138). Another study by Ahmed et al. evaluated the use of SRT with or without anti–PD-1/PD-L1 inhibitors in 17 patients with NSCLC and BM; the study included patients who received SRT prior, concurrent, or after ICI. Administration of radiotherapy concurrent or posterior to ICI was associated with an improved OS (p = 0.006) compared to prior ICI; the mOS for the entire cohort was 17.9 months (139). The study ARIO evaluated the effect of radiotherapy with (n = 100) or without ICI (n = 50) in patients with NSCLC and BM. The study found that patients receiving the treatment combination had a longer intracranial local PFS (p = 0.007); however, no differences were observed in terms of OS. The study found that patients with non-adenocarcinoma histology tumors and a KPS of 70 were associated with a worse OS. Furthermore, patients who received ICI and radiotherapy within ≤7 days of each treatment had an improved OS. The study did not find differences regarding rates of radionecrosis between treatments (140). The evidence derived from these studies highlight the beneficial synergic activity of ICI plus radiotherapy, rendering it a safe and tolerable procedure. Furthermore, it seems that timing in this context is important, since patients receiving radiotherapy (SRS/SRT) either concurrent or posterior to the start of ICI derive the greatest benefit. Additionally, most of the studies reflect that concurrent administration of these treatments leads to an improved OS.
Different mechanisms of resistance to immunotherapy have been identified, not exclusively in the context of BM. These include cancer cell–related mechanisms, such as the loss of neoantigen production, which could be triggered by hypermethylation in the promoter region of genes coding for neoantigens. Another described mechanism is alterations in neoantigen presentation, due to alterations in HLA class 1 genes, loss of function of β2-microglobulin, derangements in proteins related to antigen degradation, as well as disruptions in the IFN-JAK-STAT signaling pathway. Alterations in the latter can be associated with mutations and copy-number variations in the IFN pathway. Other identified mechanisms include those associated with a loss of function of JAK1 and JAK2 (149). Immune cell-associated mechanisms of resistance to ICI can be attributed to tumors that lack TILs, particularly CD8+ T cells. These tumors do not engage in antigen presentation and cannot trigger an inflammatory response. A number of mechanisms have been proposed to explain the absence of TILs in tumors, including tumor signaling through the β-catenin pathway, as well as loss of certain genes such as PTEN, SKT11 (LKB1), and KEAP1, among others. Overall, these alterations lead to an immunosuppressive environment, with impaired inflammatory signaling, which can lead to resistance to ICI. Another mechanism associated with resistance to ICI is the location of the tumor, such as liver metastases, which have traditionally been associated with an immunosuppressive environment. Other immune cells that are associated with an immunosuppressive TME include MDSCs and TAM-M2 cells, among others (149).
A proposed hypothesis is that there is tumor heterogeneity in BM, with different genomic and epigenomic alterations from the primary tumor (as reviewed previously), and among the BMs. In fact, tumors, both primary and metastases, are composed of different subclones with different targets, as well as different mechanisms of resistance (35). As previously stated, there exists a significant heterogeneity regarding the expression of PD-L1 and TMB according to tumor sample sites; thus, tumor heterogeneity of BM can be associated to resistance to immunotherapies.
It has been suggested that metabolic derangements in substrate consumption by tumor cells can lead to ineffective activity of the immune cells in the TME. These events are associated with a metabolic struggle of the tumor infiltrating immune cells. Both T cells and tumor cells heavily depend on glucose for their energy needs. Tumor cells, owing to their rapid growth and energy requirements, predominantly engage in aerobic glycolysis to process glucose. This leads to the generation of high levels of lactic acid in the TME, which reduces the availability of glucose for immune cells, resulting in compromised T cell function as well as a heightened recruitment of Tregs, and polarizes microglial cells and TAMs toward a pro-tumorigenic state. Furthermore, there is a competition for amino acids such as glutamine, glutamate, and tryptophan among T cells, MDSCs, and tumor cells. Within the TME, substances such as kynurenine, produced by the tumor cells, MDSCs, and TAMs, hinder the activation of T cells and promote the generation of immune-suppressive Tregs. The brain microenvironment provides ample glutamine and tryptophan, facilitating tumor cells in adapting and utilizing these amino acids for their growth. Moreover, the accumulation of lactic acid in the TME boosts the expression of PD-1 on T cells and PD-L1 on tumor cells, resulting in the suppression of immune cell function (150). These immune contextures of the brain might require specific therapeutic approaches when treating patients with NSCLC and BMs.
Different biomarkers have been described and are currently being developed to detect and characterize genomic, molecular, or TME alterations in BM, as biomarkers of prognosis or targeted treatment. As such, detection of circulating tumor DNA (ctDNA) in the cerebrospinal fluid (CSF) can be an attractive strategy and less invasive than conventional biopsies. ctDNA allows for the characterization of different genomic alterations within CNS metastases and provides a landscape of tumor heterogeneity (151). High TMB has been associated with response to ICIs, and high TMB has been detected in BM (89). Using TMB as a biomarker of response is an attractive strategy, since it can also be detected in ctDNA from CSF (152). However, there is no clinical evidence that a high TMB in BM correlates to a benefit from ICIs, and the use of ctDNA from CSF in patients with BM is not currently a standard practice.
The most validated biomarker associated with response to ICIs is detection of PD-L1, either in tumor cells, immune cells, or both and to a lesser extent TMB. High expression of both of these biomarkers has been associated with an increased survival in NSCLC patients treated with ICI (153). As previously presented TILs in BM have been associated with an improved survival in patients with NSCLC and SCLC (81, 83) and could potentially serve as biomarkers of benefit. In addition, a different biomarker is a high neutrophil-to-lymphocyte ratio, which has been associated with a worse prognosis in patients being treated with radiosurgery or neurosurgery for BM (154, 155).
Although not particularly associated with BM, an increased representation of different bacteria from the gut microbiota has been detected in the stool of patients who responded to treatment with ICI or ICI-based therapies. Among the most representative bacteria, B longum, E hirae, A muchiniphila, B fragilis, Collinsella aerofaciens, and F prausnitzii stand out as biomarkers associated with response (156–161). Furthermore, clinical trials in melanoma patients have performed fecal microbiota transplantation (FMT) from patients who received ICI and responded to patients with who received the same treatment and did not respond. Patients with primary resistance to ICI who received a FMT from responders had an approximate 20% rate of response following the FMT and continuing treatment with ICI. These results show that manipulation of the gut microbiome through FMT is a strategy useful to overcome resistance to ICI (162, 163).
Regarding future therapeutic strategies, targeting other regulatory checkpoints, such as LAG3, TIGIT, or TIM3, either alone or in combination with anti–PD-1/PD-L1 inhibitors are being explored as means to overcome resistance to current immunotherapies. Many clinical trials with different combinations are currently undergoing. Furthermore, suppression of immune cells of molecules that elicit an immunosuppressive environment could also be a target, such as M2-TAMs, MDSC, and anti-TGFβ treatments. Other strategies are being used in NSCLC patients with BM, such as clinical trials involving vaccines with mRNA tumor antigens pulsed into dendritic cells (NCT02808416) or administration of autologous dendritic cells to the tumor through the intrathecal space (NCT03638765). Results from these studies are expected to provide a new strategy to treat these patients.
BMs represent a devastating event in patients with lung cancer, with alarming numbers regarding its incidence. The development and establishment of BMs is a complex process that needs to be studied from different perspectives, including genetic, epigenetic, immunologic, physiologic, and those associated with the BBB and the brain parenchyma. The cascade of signaling events leading to BMs is complex, and still not completely understood, in part due to a limited access to BMs samples, and the heterogeneity among these tumors. Immunotherapy represents a milestone in the treatment of patients with lung cancer, and it has clearly demonstrated that either alone or in combination with chemotherapy or radiotherapy, patients with BM derive a benefit. However, selecting patients who will benefit from one combination of therapies or another, continues to represent a challenge, and so does the sequence of treatment administration. Although important breakthroughs have been achieved with the arrival of immunotherapy, patients with lung cancer and BMs still present a deleterious diagnosis which needs to be approached by a multidisciplinary team and requires further research.
AR-H performed the literature search and developed the outline as well as the initial draft. EA edited the manuscript, added further content and provided supervision. All authors contributed to the article and approved the submitted version.
AR-H is supported by a grant from the Spanish Society of Medical Oncology (SEOM). EA is supported by grants from Agència de Gestió d’Ajuts Universitaris i de Recerca (2021-SGR-776 and 2021-SGR-628), and Fondo de Investigacion Sanitaria-Instituto de Salud Carlos III co-funded by the European Union (CB16/12/00241 and PI22/00105). Figures created using BioRender.com.
EA reports the following COIs: Consulting or Advisory Role: MSD, Bristol-Myers, Roche, Boehringer Ingelheim, Pfizer, Novartis, AstraZeneca, Lilly, Takeda. Speaking: MSD, Bristol-Myers, Roche, Boehringer Ingelheim, Pfizer, Novartis, AstraZeneca, Lilly, Takeda. Co-founder: Trialing Health S.L.
The remaining author declares that the research was conducted in the absence of any commercial or financial relationships that could be construed as a potential conflict of interest.
All claims expressed in this article are solely those of the authors and do not necessarily represent those of their affiliated organizations, or those of the publisher, the editors and the reviewers. Any product that may be evaluated in this article, or claim that may be made by its manufacturer, is not guaranteed or endorsed by the publisher.
1. Achrol AS, Rennert RC, Anders C, Soffietti R, Ahluwalia MS, Nayak L, et al. Brain metastases. Nat Rev Dis Prim (2019) 5:5. doi: 10.1038/s41572-018-0055-y
2. Nussbaum ES, Djalilian HR, Cho KH, Hall WA. Brain metastases. Histology, multiplicity, surgery, and survival. Cancer (1996) 78:1781–8. doi: 10.1002/9781444317008.Ch138
3. Gavrilovic IT, Posner JB. Brain metastases: epidemiology and pathophysiology. J Neurooncol (2005) 75:5–14. doi: 10.1007/s11060-004-8093-6
4. Le Rhun E, Guckenberger M, Smits M, Dummer R, Bachelot T, Sahm F, et al. EANO–ESMO Clinical Practice Guidelines for diagnosis, treatment and follow-up of patients with brain metastasis from solid tumours. Ann Oncol (2021) 32:1332–47. doi: 10.1016/j.annonc.2021.07.016
5. Amin S, Baine MJ, Meza JL, Lin C. Association of immunotherapy with survival among patients with brain metastases whose cancer was managed with definitive surgery of the primary tumor. JAMA Netw Open (2020) 3:e2015444. doi: 10.1001/jamanetworkopen.2020.15444
6. Wood DE, Kazerooni EA, Aberle DR, Brown LM, Eapen GA, Ettinger DS, et al. NCCN clinical practice guidelines in oncology (NCCN guidelines®). Lung cancer screening. Version 1.2024.
7. Barnholtz-Sloan JS, Sloan AE, Davis FG, Vigneau FD, Lai P, Sawaya RE. Incidence proportions of brain metastases in patients diagnosed (1973 to 2001) in the Metropolitan Detroit Cancer Surveillance System. J Clin Oncol (2004) 22:2865–72. doi: 10.1200/JCO.2004.12.149
8. Shi AA, Digumarthy SR, Temel JS, Halpern EF, Kuester LB, Aquino SL. Does initial staging or tumor histology better identify asymptomatic brain metastases in patients with non–small cell lung cancer? J Thorac Oncol (2006) 1:205–10. doi: 10.1016/S1556-0864(15)31569-0
9. Komaki R, Cox JD, Stark R. Frequency of brain metastasis in adenocarcinoma and large cell carcinoma of the lung: Correlation with survival. Int J Radiat Oncol (1983) 9:1467–70. doi: 10.1016/0360-3016(83)90319-X
10. Ernani V, Stinchcombe TE. Management of brain metastases in non–small-cell lung cancer. J Oncol Pract (2019) 15:563–70. doi: 10.1200/JOP.19.00357
11. Sorensen JB, Hansen HH, Hansen M, Dombernowsky P. Brain metastases in adenocarcinoma of the lung: Frequency, risk groups, and prognosis. J Clin Oncol (1988) 6:1474–80. doi: 10.1200/JCO.1988.6.9.1474
12. Rangachari D, Yamaguchi N, VanderLaan PA, Folch E, Mahadevan A, Floyd SR, et al. Brain metastases in patients with EGFR-mutated or ALK-rearranged non-small-cell lung cancers. Lung Cancer (2015) 88:108–11. doi: 10.1016/j.lungcan.2015.01.020
13. Gainor JF, Tseng D, Yoda S, Dagogo-Jack I, Friboulet L, Lin JJ, et al. Patterns of metastatic spread and mechanisms of resistance to crizotinib in ROS1 -positive non–small-cell lung cancer. JCO Precis Oncol (2017) 1:1–13. doi: 10.1200/PO.17.00063
14. Dagogo-Jack I, Martinez P, Yeap BY, Ambrogio C, Ferris LA, Lydon C, et al. Impact of BRAF mutation class on disease characteristics and clinical outcomes in BRAF-mutant lung cancer. Clin Cancer Res (2019) 25:158–65. doi: 10.1158/1078-0432.CCR-18-2062
15. Offin M, Feldman D, Ni A, Myers ML, Lai WV, Pentsova E, et al. Frequency and outcomes of brain metastases in patients with HER2 -mutant lung cancers. Cancer (2019) 125:4380–7. doi: 10.1002/cncr.32461
16. Yang S, Wang Y, Zhao C, Li X, Liu Q, Mao S, et al. Exon 20 YVMA insertion is associated with high incidence of brain metastasis and inferior outcome of chemotherapy in advanced non-small cell lung cancer patients with HER2 kinase domain mutations. Transl Lung Cancer Res (2021) 10:753–65. doi: 10.21037/tlcr-20-559
17. Drilon A, Lin JJ, Filleron T, Ni A, Milia J, Bergagnini I, et al. Frequency of brain metastases and multikinase inhibitor outcomes in patients with RET–rearranged lung cancers. J Thorac Oncol (2018) 13:1595–601. doi: 10.1016/j.jtho.2018.07.004
18. Lamberti G, Aizer A, Ricciuti B, Alessi JV, Pecci F, Tseng S-C, et al. Incidence of brain metastases and preliminary evidence of intracranial activity with sotorasib in patients with KRAS G12C -mutant non–small-cell lung cancer. JCO Precis Oncol (2023) 7:1–8. doi: 10.1200/po.22.00621
19. Reungwetwattana T, Nakagawa K, Cho BC, Cobo M, Cho EK, Bertolini A, et al. CNS response to osimertinib versus standard epidermal growth factor receptor tyrosine kinase inhibitors in patients with untreated EGFR -mutated advanced non–small-cell lung cancer. J Clin Oncol (2018) 36:3290–7. doi: 10.1200/JCO.2018.78.3118
20. Le X, Paz-Ares LG, Van Meerbeeck J, Viteri S, Cabrera Galvez C, Vicente Baz D, et al. Tepotinib in patients (pts) with advanced non-small cell lung cancer (NSCLC) with MET amplification ( MET amp). J Clin Oncol (2021) 39:9021–1. doi: 10.1200/JCO.2021.39.15_suppl.9021
21. Gainor JF, Curigliano G, Kim D-W, Lee DH, Besse B, Baik CS, et al. Pralsetinib for RET fusion-positive non-small-cell lung cancer (ARROW): a multi-cohort, open-label, phase 1/2 study. Lancet Oncol (2021) 22:959–69. doi: 10.1016/S1470-2045(21)00247-3
22. Jänne PA, Riely GJ, Gadgeel SM, Heist RS, Ou S-HI, Pacheco JM, et al. Adagrasib in non–small-cell lung cancer harboring a KRAS G12C mutation. N Engl J Med (2022) 387:1–12. doi: 10.1056/nejmoa2204619
23. Schouten LJ, Rutten J, Huveneers HAM, Twijnstra A. Incidence of brain metastases in a cohort of patients with carcinoma of the breast, colon, kidney, and lung and melanoma. Cancer (2002) 94:2698–705. doi: 10.1002/cncr.10541
24. Nugent JL, Bunn PA, Matthews MJ, Ihde DC, Cohen MH, Gazdar A, et al. CNS metastases in small cell bronchogenic carcinoma.Increasing frequency and changing pattern with lengthening survival. Cancer (1979) 44:1885–93. doi: 10.1002/1097-0142(197911)44:5<1885::AID-CNCR2820440550>3.0.CO;2-F
25. Li N, Chu Y, Song Q. Brain metastasis in patients with small cell lung cancer. Int J Gen Med (2021) 14:10131–9. doi: 10.2147/IJGM.S342009
26. Hou R, Li H, Cao J, Song X, Zhang X, Wang W. Validation of a novel prognostic index: BMS-Score for patients with brain metastases of small cell lung cancer. Ann Palliat Med (2021) 10:29–36. doi: 10.21037/apm-20-1819
27. Ettinger DS, Wood DE, Aisner DL, Akerley W, Bauman JR, Bharat A, et al. NCCN clinical practice guidelines in oncology (NCCN guidelines®). Non-small cell lung cancer. (2023).
28. Ganti AKP, Loo BW, Bassetti M, Chiang A, D’Amico TA, D’Avella CA, et al. NCCN clinical practice guidelines in oncology (NCCN guidelines®). Small Cell Lung Cancer (2023).
29. Sperduto PW, Yang TJ, Beal K, Pan H, Brown PD, Bangdiwala A, et al. spwlcbm. JAMA Oncol (2017) 3:827. doi: 10.1001/jamaoncol.2016.3834
30. Seute T, Leffers P, Ten Velde GPM, Twijnstra A. Neurologic disorders in 432 consecutive patients with small cell lung carcinoma. Cancer (2004) 100:801–6. doi: 10.1002/cncr.20043
31. Slotman B, Faivre-Finn C, Kramer G, Rankin E, Snee M, Hatton M, et al. Prophylactic cranial irradiation in extensive small-cell lung cancer. N Engl J Med (2007) 357:664–72. doi: 10.1056/NEJMoa071780
32. Edelman MJ. Prophylactic cranial irradiation for small-cell lung cancer: time for a reassessment. Am Soc Clin Oncol Educ B (2020) 40:24–8. doi: 10.1200/EDBK_281041
33. Edelman MJ. Prophylactic cranial irradiation for small-cell lung cancer: time for a reassessment. Am Soc Clin Oncol Educ B (2020) 40:24–8. doi: 10.1200/edbk_281041
34. Chiang AC, Massagué J. Molecular basis of metastasis. N Engl J Med (2008) 359:2814–23. doi: 10.1056/NEJMra0805239
35. Seoane J, De Mattos-Arruda L. Brain metastasis: New opportunities to tackle therapeutic resistance. Mol Oncol (2014) 8:1120–31. doi: 10.1016/j.molonc.2014.05.009
36. Abbott NJ, Rönnbäck L, Hansson E. Astrocyte-endothelial interactions at the blood-brain barrier. Nat Rev Neurosci (2006) 7:41–53. doi: 10.1038/nrn1824
37. Daneman R. The blood-brain barrier in health and disease. Ann Neurol (2012) 72:648–72. doi: 10.1002/ana.23648
38. Wong AD, Ye M, Levy AF, Rothstein JD, Bergles DE, Searson PC. The blood-brain barrier: An engineering perspective. Front Neuroeng (2013) 6:7. doi: 10.3389/fneng.2013.00007
39. Nag S. Morphology and Properties of Brain Endothelial cells. In: Methods in Molecular Biology (2011) New Jersey, USA: Springer Protocols, Humana Press. p. 3–47. doi: 10.1007/978-1-60761-938-3_1
40. Cabezas R, Ávila M, Gonzalez J, El-Bachá RS, Báez E, García-Segura LM, et al. Astrocytic modulation of blood brain barrier: Perspectives on Parkinson’s disease. Front Cell Neurosci (2014) 8:211. doi: 10.3389/fncel.2014.00211
41. Lange S, Trost A, Tempfer H, Bauer HC, Bauer H, Rohde E, et al. Brain pericyte plasticity as a potential drug target in CNS repair. Drug Discovery Today (2013) 18:456–63. doi: 10.1016/j.drudis.2012.12.007
42. Hurtado-Alvarado G, Cabañas-Morales AM, Gómez-Gónzalez B. Pericytes: brain-immune interface modulators. Front Integr Neurosci (2014) 7:80. doi: 10.3389/fnint.2013.00080
43. Arvanitis CD, Ferraro GB, Jain RK. The blood–brain barrier and blood–tumour barrier in brain tumours and metastases. Nat Rev Cancer (2020) 20:26–41. doi: 10.1038/s41568-019-0205-x
44. Steeg PS. The blood–tumour barrier in cancer biology and therapy. Nat Rev Clin Oncol (2021) 18:696–714. doi: 10.1038/s41571-021-00529-6
45. Liu Y, Cao X. Characteristics and significance of the pre-metastatic niche. Cancer Cell (2016) 30:668–81. doi: 10.1016/j.ccell.2016.09.011
46. Feng S, Cen J, Huang Y, Shen H, Yao L, Wang Y, et al. Matrix metalloproteinase-2 and -9 secreted by leukemic cells increase the permeability of blood-brain barrier by disrupting tight junction proteins. PloS One (2011) 6:e20599. doi: 10.1371/journal.pone.0020599
47. Feng S, Huang Y, Chen Z. Does VEGF secreted by leukemic cells increase the permeability of blood-brain barrier by disrupting tight-junction proteins in central nervous system leukemia? Med Hypotheses (2011) 76:618–21. doi: 10.1016/j.mehy.2010.12.001
48. Li B, Wang C, Zhang Y, Zhao XY, Huang B, Wu PF, et al. Elevated PLGF contributes to small-cell lung cancer brain metastasis. Oncogene (2013) 32:2952–62. doi: 10.1038/onc.2012.313
49. Théry C, Zitvogel L, Amigorena S. Exosomes: composition, biogenesis and function. Nat Rev Immunol (2002) 2:569–79. doi: 10.1038/nri855
50. Hoshino A, Costa-Silva B, Shen T-L, Rodrigues G, Hashimoto A, Tesic Mark M, et al. Tumour exosome integrins determine organotropic metastasis. Nature (2015) 527:329–35. doi: 10.1038/nature15756
51. Peng Y, Croce CM. The role of MicroRNAs in human cancer. Signal Transduct Target Ther (2016) 1:15004. doi: 10.1038/sigtrans.2015.4
52. Chen L, Xu S, Xu H, Zhang J, Ning J, Wang S. MicroRNA-378 is associated with non-small cell lung cancer brain metastasis by promoting cell migration, invasion and tumor angiogenesis. Med Oncol (2012) 29:1673–80. doi: 10.1007/s12032-011-0083-x
53. Arora S, Ranade AR, Tran NL, Nasser S, Sridhar S, Korn RL, et al. MicroRNA-328 is associated with (non-small) cell lung cancer (NSCLC) brain metastasis and mediates NSCLC migration. Int J Cancer (2011) 129:2621–31. doi: 10.1002/ijc.25939
54. Statello L, Guo C-J, Chen L-L, Huarte M. Gene regulation by long non-coding RNAs and its biological functions. Nat Rev Mol Cell Biol (2021) 22:96–118. doi: 10.1038/s41580-020-00315-9
55. Shen L, Chen L, Wang Y, Jiang X, Xia H, Zhuang Z. Long noncoding RNA MALAT1 promotes brain metastasis by inducing epithelial-mesenchymal transition in lung cancer. J Neurooncol (2015) 121:101–8. doi: 10.1007/s11060-014-1613-0
56. Nakagawa T, Endo H, Yokoyama M, Abe J, Tamai K, Tanaka N, et al. Large noncoding RNA HOTAIR enhances aggressive biological behavior and is associated with short disease-free survival in human non-small cell lung cancer. Biochem Biophys Res Commun (2013) 436:319–24. doi: 10.1016/j.bbrc.2013.05.101
57. Soto MS, Serres S, Anthony DC, Sibson NR. Functional role of endothelial adhesion molecules in the early stages of brain metastasis. Neuro Oncol (2014) 16:540–51. doi: 10.1093/neuonc/not222
58. Bao X, Wu J, Jiang J, Tien AC, Sanai N, Li J. Quantitative protein expression of blood-brain barrier transporters in the vasculature of brain metastases of patients with lung and breast cancer. Clin Transl Sci (2021) 14:1265–71. doi: 10.1111/cts.12978
59. Teglasi V, Csury DT, Dezso K, Bugyik E, Szabo V, Szallasi Z, et al. Origin and distribution of connective tissue and pericytes impacting vascularization in brain metastases with different growth patterns. J Neuropathol Exp Neurol (2019) 78:326–39. doi: 10.1093/jnen/nlz007
60. Lyle LT, Lockman PR, Adkins CE, Mohammad AS, Sechrest E, Hua E, et al. Alterations in pericyte subpopulations are associated with elevated blood-tumor barrier permeability in experimental brain metastasis of breast cancer. Clin Cancer Res (2016) 22:5287–99. doi: 10.1158/1078-0432.CCR-15-1836
61. Amer NA. Resistance to Targeted Therapies Against Adult Brain Cancers. Tivnan A, editor. Cham, Switzerland: Springer International Publishing (2016). p. 1179. doi: 10.1007/978-3-319-46505-0
62. Connell JJ, Chatain G, Cornelissen B, Vallis KA, Hamilton A, Seymour L, et al. Selective permeabilization of the blood-brain barrier at sites of metastasis. J Natl Cancer Inst (2013) 105:1634–43. doi: 10.1093/jnci/djt276
63. Ma SC, Li Q, Peng JY, Zhouwen JL, Diao JF, Niu JX, et al. Claudin-5 regulates blood-brain barrier permeability by modifying brain microvascular endothelial cell proliferation, migration, and adhesion to prevent lung cancer metastasis. CNS Neurosci Ther (2017) 23:947–60. doi: 10.1111/cns.12764
64. de Visser KE, Joyce JA. The evolving tumor microenvironment: From cancer initiation to metastatic outgrowth. Cancer Cell (2023) 41:374–403. doi: 10.1016/j.ccell.2023.02.016
65. Lorger M, Felding-Habermann B. Capturing changes in the brain microenvironment during initial steps of breast cancer brain metastasis. Am J Pathol (2010) 176:2958–71. doi: 10.2353/ajpath.2010.090838
66. Placone AL, Quiñones-Hinojosa A, Searson PC. The role of astrocytes in the progression of brain cancer: complicating the picture of the tumor microenvironment. Tumor Biol (2016) 37:61–9. doi: 10.1007/s13277-015-4242-0
67. Samdani AF, Kuchner EB, Rhines L, Adamson DC, Lawson C, Tyler B, et al. Astroglia induce cytotoxic effects on brain tumors via a nitric oxide-dependent pathway both in vitro and in vivo. Neurosurgery (2004) 54:1231–8. doi: 10.1227/01.NEU.0000119576.76193.B8
68. Valiente M, Obenauf AC, Jin X, Chen Q, Zhang XHF, Lee DJ, et al. Serpins promote cancer cell survival and vascular Co-option in brain metastasis. Cell (2014) 156:1002–16. doi: 10.1016/j.cell.2014.01.040
69. Seike T, Fujita K, Yamakawa Y, Kido MA, Takiguchi S, Teramoto N, et al. Interaction between lung cancer cells and astrocytes via specific inflammatory cytokines in the microenvironment of brain metastasis. Clin Exp Metastasis (2011) 28:13–25. doi: 10.1007/s10585-010-9354-8
70. Strickland MR, Alvarez-Breckenridge C, Gainor JF, Brastianos PK. Tumor immune microenvironment of brain metastases: toward unlocking antitumor immunity. Cancer Discovery (2022) 12:1199–216. doi: 10.1158/2159-8290.CD-21-0976
71. Chen Q, Boire A, Jin X, Valiente M, Er EE, Lopez-Soto A, et al. Carcinoma-astrocyte gap junctions promote brain metastasis by cGAMP transfer. Nature (2016) 533:493–8. doi: 10.1038/nature18268
72. Zhang L, Zhang S, Yao J, Lowery FJ, Zhang Q, Huang WC, et al. Microenvironment-induced PTEN loss by exosomal microRNA primes brain metastasis outgrowth. Nature (2015) 527:100–4. doi: 10.1038/nature15376
73. Eichler AF, Chung E, Kodack DP, Loeffler JS, Fukumura D, Jain RK. The biology of brain metastases-translation to new therapies. Nat Rev Clin Oncol (2011) 8:344–56. doi: 10.1038/nrclinonc.2011.58
74. Escartin C, Galea E, Lakatos A, O’Callaghan JP, Petzold GC, Serrano-Pozo A, et al. Reactive astrocyte nomenclature, definitions, and future directions. Nat Neurosci (2021) 24:312–25. doi: 10.1038/s41593-020-00783-4
75. Priego N, Zhu L, Monteiro C, Mulders M, Wasilewski D, Bindeman W, et al. STAT3 labels a subpopulation of reactive astrocytes required for brain metastasis article. Nat Med (2018) 24:1024–35. doi: 10.1038/s41591-018-0044-4
76. Du W, Bos PD. Tracing Bone Marrow-Derived Microglia in Brain Metastatic Tumors. 1st ed. Cambridge, Massachusetts, United States: Elsevier Inc (2020) p. 95–110. doi: 10.1016/bs.mie.2019.08.017
77. Bowman RL, Klemm F, Akkari L, Pyonteck SM, Sevenich L, Quail DF, et al. Macrophage ontogeny underlies differences in tumor-specific education in brain Malignancies. Cell Rep (2016) 17:2445–59. doi: 10.1016/j.celrep.2016.10.052
78. Ma RY, Black A, Qian BZ. Macrophage diversity in cancer revisited in the era of single-cell omics. Trends Immunol (2022) 43:546–63. doi: 10.1016/j.it.2022.04.008
79. Wang Y, Chen R, Wa Y, Ding S, Yang Y, Liao J, et al. Tumor immune microenvironment and immunotherapy in brain metastasis from non-small cell lung cancer. Front Immunol (2022) 13:829451. doi: 10.3389/fimmu.2022.829451
80. Paijens ST, Vledder A, de Bruyn M, Nijman HW. Tumor-infiltrating lymphocytes in the immunotherapy era. Cell Mol Immunol (2021) 18:842–59. doi: 10.1038/s41423-020-00565-9
81. Berghoff AS, Fuchs E, Ricken G, Mlecnik B, Bindea G, Spanberger T, et al. Density of tumor-infiltrating lymphocytes correlates with extent of brain edema and overall survival time in patients with brain metastases. Oncoimmunology (2016) 5:e1057388. doi: 10.1080/2162402X.2015.1057388
82. Ganti AKP, Loo BW, Bassetti M,AC, D’Amico T, D’Avella C, Dowlati A, et al. Small cell lung cancer. NCCN Clin Pract guidelines Oncol (2023).
83. Berghoff AS, Ricken G, Wilhelm D, Rajky O, Widhalm G, Dieckmann K, et al. Tumor infiltrating lymphocytes and PD-L1 expression in brain metastases of small cell lung cancer (SCLC). J Neurooncol (2016) 130:19–29. doi: 10.1007/s11060-016-2216-8
84. Li YD, Lamano JB, Lamano JB, Quaggin-Smith J, Veliceasa D, Kaur G, et al. Tumor-induced peripheral immunosuppression promotes brain metastasis in patients with non-small cell lung cancer. Cancer Immunol Immunother (2019) 68:1501–13. doi: 10.1007/s00262-019-02384-y
85. Kudo Y, Haymaker C, Zhang J, Reuben A, Duose DY, Fujimoto J, et al. Suppressed immune microenvironment and repertoire in brain metastases from patients with resected non-small-cell lung cancer. Ann Oncol (2019) 30:1521–30. doi: 10.1093/annonc/mdz207
86. Zhou J, Gong Z, Jia Q, Wu Y, Yang ZZ, Zhu B. Programmed death ligand 1 expression and CD8+ tumor-infiltrating lymphocyte density differences between paired primary and brain metastatic lesions in non-small cell lung cancer. Biochem Biophys Res Commun (2018) 498:751–7. doi: 10.1016/j.bbrc.2018.03.053
87. Li M, Hou X, Sai K, Wu L, Chen J, Zhang B, et al. Immune suppressive microenvironment in brain metastatic non-small cell lung cancer: comprehensive immune microenvironment profiling of brain metastases versus paired primary lung tumors (GASTO 1060). Oncoimmunology (2022) 11:1–13. doi: 10.1080/2162402X.2022.2059874
88. Ikarashi D, Okimoto T, Shukuya T, Onagi H, Hayashi T, Sinicropi-Yao SL, et al. Comparison of tumor microenvironments between primary tumors and brain metastases in patients with NSCLC. JTO Clin Res Rep (2021) 2:100230. doi: 10.1016/j.jtocrr.2021.100230
89. Moutafi MK, Tao W, Huang R, Haberberger J, Alexander B, Ramkissoon S, et al. Comparison of programmed death-ligand 1 protein expression between primary and metastatic lesions in patients with lung cancer. J Immunother Cancer (2021) 9:1–5. doi: 10.1136/jitc-2020-002230
90. Mansfield AS, Ren H, Sutor S, Sarangi V, Nair A, Davila J, et al. Contraction of T cell richness in lung cancer brain metastases. Sci Rep (2018) 8:1–9. doi: 10.1038/s41598-018-20622-8
91. Jiang T, Yan Y, Zhou K, Su C, Ren S, Li N, et al. Characterization of evolution trajectory and immune profiling of brain metastasis in lung adenocarcinoma. NPJ Precis Oncol (2021) 5:6. doi: 10.1038/s41698-021-00151-w
92. Li L, Liu Z, Han R, Li L, Wang M, Huang D, et al. Genetic heterogeneity between paired primary and brain metastases in lung adenocarcinoma. Clin Med Insights Oncol (2020) 14:179554920947335333. doi: 10.1177/1179554920947335
93. Lengel HB, Mastrogiacomo B, Connolly JG, Tan KS, Liu Y, Fick CN, et al. Genomic mapping of metastatic organotropism in lung adenocarcinoma. Cancer Cell (2023) 41:970–985.e3. doi: 10.1016/j.ccell.2023.03.018
94. Shih DJH, Nayyar N, Bihun I, Dagogo-Jack I, Gill CM, Aquilanti E, et al. Genomic characterization of human brain metastases identifies drivers of metastatic lung adenocarcinoma. Nat Genet (2020) 52:371–7. doi: 10.1038/s41588-020-0592-7
95. Nicoś M, Harbers L, Patrucco E, Kramer-Drauberg M, Zhang X, Voena C, et al. Genomic profiling identifies putative pathogenic alterations in NSCLC brain metastases. JTO Clin Res Rep (2022) 3:100435. doi: 10.1016/j.jtocrr.2022.100435
96. Vassella E, Kashani E, Zens P, Kündig A, Fung C, Scherz A, et al. Mutational profiles of primary pulmonary adenocarcinoma and paired brain metastases disclose the importance of KRAS mutations. Eur J Cancer (2021) 159:227–36. doi: 10.1016/j.ejca.2021.10.006
97. Vogelbaum MA, Brown PD, Messersmith H, Brastianos PK, Burri S, Cahill D, et al. Treatment for brain metastases: ASCO-SNO-ASTRO guideline. J Clin Oncol (2022) 40:492–516. doi: 10.1200/JCO.21.02314
98. Smart DD. Radiation toxicity in the central nervous system: mechanisms and strategies for injury reduction. Semin Radiat Oncol (2017) 27:332–9. doi: 10.1016/j.semradonc.2017.04.006
99. Kosmin M, Rees J. Radiation and the nervous system. Pract Neurol (2022) 22:450–60. doi: 10.1136/pn-2022-003343
100. Wilke C, Grosshans D, Duman J, Brown P, Li J. Radiation-induced cognitive toxicity: Pathophysiology and interventions to reduce toxicity in adults. Neuro Oncol (2018) 20:597–607. doi: 10.1093/neuonc/nox195
101. Hu H, Xu ZY, Zhu Q, Liu X, Jiang SC, Zheng JH. Brain metastases status and immunotherapy efficacy in advanced lung cancer: A systematic review and meta-analysis. Front Immunol (2021) 12:669398. doi: 10.3389/fimmu.2021.669398
102. Barlesi F, Tomasini P. Non-small-cell lung cancer brain metastases and PD-(L)1 immune checkpoint inhibitors. Lancet Oncol (2020) 21:607–8. doi: 10.1016/S1470-2045(20)30207-2
103. Berghoff AS, Venur VA, Preusser M, Ahluwalia MS. Immune checkpoint inhibitors in brain metastases: from biology to treatment. Am Soc Clin Oncol Educ B (2016) 36:e116–22. doi: 10.14694/edbk_100005
104. Srinivasan ES, Deshpande K, Neman J, Winkler F, Khasraw M. The microenvironment of brain metastases from solid tumors. Neuro-Oncology Adv (2021) 3:v121–32. doi: 10.1093/noajnl/vdab121
105. Boussiotis VA. Molecular and biochemical aspects of the PD-1 checkpoint pathway. N Engl J Med (2016) 375:1767–78. doi: 10.1056/NEJMra1514296
106. Tawbi HA, Forsyth PA, Algazi A, Hamid O, Hodi FS, Moschos SJ, et al. Combined nivolumab and ipilimumab in melanoma metastatic to the brain. N Engl J Med (2018) 379:722–30. doi: 10.1056/NEJMoa1805453
107. Long GV, Atkinson V, Lo S, Sandhu S, Guminski AD, Brown MP, et al. Combination nivolumab and ipilimumab or nivolumab alone in melanoma brain metastases: a multicentre randomised phase 2 study. Lancet Oncol (2018) 19:672–81. doi: 10.1016/S1470-2045(18)30139-6
108. Tawbi HA, Forsyth PA, Hodi FS, Algazi AP, Hamid O, Lao CD, et al. Long-term outcomes of patients with active melanoma brain metastases treated with combination nivolumab plus ipilimumab (CheckMate 204): final results of an open-label, multicentre, phase 2 study. Lancet Oncol (2021) 22:1692–704. doi: 10.1016/S1470-2045(21)00545-3
109. Gadgeel SM, Lukas RV, Goldschmidt J, Conkling P, Park K, Cortinovis D, et al. Atezolizumab in patients with advanced non-small cell lung cancer and history of asymptomatic, treated brain metastases: Exploratory analyses of the phase III OAK study. Lung Cancer (2019) 128:105–12. doi: 10.1016/j.lungcan.2018.12.017
110. Fehrenbacher L, von Pawel J, Park K, Rittmeyer A, Gandara DR, Ponce Aix S, et al. Updated efficacy analysis including secondary population results for OAK: A randomized phase III study of atezolizumab versus docetaxel in patients with previously treated advanced non–small cell lung cancer. J Thorac Oncol (2018) 13:1156–70. doi: 10.1016/j.jtho.2018.04.039
111. Spigel DR, Chaft JE, Gettinger S, Chao BH, Dirix L, Schmid P, et al. FIR: efficacy, safety, and biomarker analysis of a phase II open-label study of atezolizumab in PD-L1–selected patients with NSCLC. J Thorac Oncol (2018) 13:1733–42. doi: 10.1016/j.jtho.2018.05.004
112. Barlesi F, Vansteenkiste J, Spigel D, Ishii H, Garassino M, de Marinis F, et al. Avelumab versus docetaxel in patients with platinum-treated advanced non-small-cell lung cancer (JAVELIN Lung 200): an open-label, randomised, phase 3 study. Lancet Oncol (2018) 19:1468–79. doi: 10.1016/S1470-2045(18)30673-9
113. Park K, Özgüroğlu M, Vansteenkiste J, Spigel D, Yang JCH, Ishii H, et al. Avelumab versus docetaxel in patients with platinum-treated advanced NSCLC: 2-year follow-up from the JAVELIN lung 200 phase 3 trial. J Thorac Oncol (2021) 16:1369–78. doi: 10.1016/j.jtho.2021.03.009
114. Goldberg SB, Schalper KA, Gettinger SN, Mahajan A, Herbst RS, Chiang AC, et al. Pembrolizumab for management of patients with NSCLC and brain metastases: long-term results and biomarker analysis from a non-randomised, open-label, phase 2 trial. Lancet Oncol (2020) 21:655–63. doi: 10.1016/S1470-2045(20)30111-X
115. Goldberg SB, Gettinger SN, Mahajan A, Herbst RS, Chiang AC, Lilenbaum R, et al. Durability of brain metastasis response and overall survival in patients with non-small cell lung cancer (NSCLC) treated with pembrolizumab. J Clin Oncol (2018) 36:2009–9. doi: 10.1200/JCO.2018.36.15_suppl.2009
116. Wakuda K, Yabe M, Kodama H, Nishioka N, Miyawaki T, Miyawaki E, et al. Efficacy of pembrolizumab in patients with brain metastasis caused by previously untreated non-small cell lung cancer with high tumor PD-L1 expression. Lung Cancer (2021) 151:60–8. doi: 10.1016/j.lungcan.2020.11.009
117. Crinò L, Bronte G, Bidoli P, Cravero P, Minenza E, Cortesi E, et al. Nivolumab and brain metastases in patients with advanced non-squamous non-small cell lung cancer. Lung Cancer (2019) 129:35–40. doi: 10.1016/j.lungcan.2018.12.025
118. Dudnik E, Yust-Katz S, Nechushtan H, Goldstein DA, Zer A, Flex D, et al. Intracranial response to nivolumab in NSCLC patients with untreated or progressing CNS metastases. Lung Cancer (2016) 98:114–7. doi: 10.1016/j.lungcan.2016.05.031
119. Watanabe H, Kubo T, Ninomiya T, Ohashi K, Ichihara E, Sato A, et al. The effect of nivolumab treatment for central nervous system metastases in non-small cell lung cancer. J Clin Oncol (2017) 35:e20601–1. doi: 10.1200/jco.2017.35.15_suppl.e20601
120. Debieuvre D, Juergens RA, Asselain B, Audigier-Valette C, Auliac JB, Barlesi F, et al. Two-year survival with nivolumab in previously treated advanced non–small-cell lung cancer: A real-world pooled analysis of patients from France, Germany, and Canada. Lung Cancer (2021) 157:40–7. doi: 10.1016/j.lungcan.2021.04.022
121. Assié JB, Corre R, Levra MG, Calvet CY, Gaudin AF, Grumberg V, et al. Nivolumab treatment in advanced non-small cell lung cancer: real-world long-term outcomes within overall and special populations (the UNIVOC study). Ther Adv Med Oncol (2020) 12:1–11. doi: 10.1177/1758835920967237
122. Grossi F, Genova C, Crinò L, Delmonte A, Turci D, Signorelli D, et al. Real-life results from the overall population and key subgroups within the Italian cohort of nivolumab expanded access program in non-squamous non–small cell lung cancer. Eur J Cancer (2019) 123:72–80. doi: 10.1016/j.ejca.2019.09.011
123. Cortinovis D, Chiari R, Catino A, Grossi F, DE Marinis F, Sperandi F, et al. Italian cohort of the nivolumab EAP in squamous NSCLC: efficacy and safety in patients with CNS metastases. Anticancer Res (2019) 39:4265–71. doi: 10.21873/anticanres.13590
124. Borghaei H, Pluzanski A, Caro RB, Provencio M, Burgers S, Carcereny E, et al. Abstract CT221: Nivolumab (NIVO) + ipilimumab (IPI) as first-line (1L) treatment for patients with advanced non-small cell lung cancer (NSCLC) with brain metastases: Results from CheckMate 227. Cancer Res (2020) 80:CT221–1. doi: 10.1158/1538-7445.AM2020-CT221
125. Hendriks LEL, Henon C, Auclin E, Mezquita L, Ferrara R, Audigier-Valette C, et al. Outcome of patients with non–small cell lung cancer and brain metastases treated with checkpoint inhibitors. J Thorac Oncol (2019) 14:1244–54. doi: 10.1016/j.jtho.2019.02.009
126. Nadal E, Rodriguez-Abreu D, Massuti B, Juan-Vidal O, Huidobro Vence G, Lopez R, et al. Updated analysis from the ATEZO-BRAIN trial: Atezolizumab plus carboplatin and pemetrexed in patients with advanced nonsquamous non–small cell lung cancer with untreated brain metastases. J Clin Oncol (2022) 40:9010–0. doi: 10.1200/JCO.2022.40.16_suppl.9010
127. Garassino MC, Gadgeel S, Esteban E, Felip E, Speranza G, De Angelis F, et al. PD2.02 pembrolizumab plus pemetrexed-platinum for patients with metastatic nonsquamous NSCLC and liver or brain metastases: results from KEYNOTE-189. J Thorac Oncol (2019) 14:S1170–1. doi: 10.1016/j.jtho.2019.09.129
128. Gandhi L, Rodríguez-Abreu D, Gadgeel S, Esteban E, Felip E, De Angelis F, et al. Pembrolizumab plus chemotherapy in metastatic non–small-cell lung cancer. N Engl J Med (2018) 378:2078–92. doi: 10.1056/nejmoa1801005
129. Sun L, Davis CW, Hwang WT, Jeffries S, Sulyok LF, Marmarelis ME, et al. Outcomes in patients with non–small-cell lung cancer with brain metastases treated with pembrolizumab-based therapy. Clin Lung Cancer (2021) 22:58–66.e3. doi: 10.1016/j.cllc.2020.10.017
130. Powell SF, Rodríguez-Abreu D, Langer CJ, Tafreshi A, Paz-Ares L, Kopp H-G, et al. Outcomes with pembrolizumab plus platinum-based chemotherapy for patients with NSCLC and stable brain metastases: pooled analysis of KEYNOTE-021, -189, and -407. J Thorac Oncol (2021) 16:1883–92. doi: 10.1016/j.jtho.2021.06.020
131. Reck M, Ciuleanu T-E, Cobo M, Schenker M, Zurawski B, Menezes J, et al. First-line nivolumab plus ipilimumab with two cycles of chemotherapy versus chemotherapy alone (four cycles) in advanced non-small-cell lung cancer: CheckMate 9LA 2-year update. ESMO Open (2021) 6:100273. doi: 10.1016/j.esmoop.2021.100273
132. Paz-Ares L, Ciuleanu T-E, Cobo M, Schenker M, Zurawski B, Menezes J, et al. First-line nivolumab plus ipilimumab combined with two cycles of chemotherapy in patients with non-small-cell lung cancer (CheckMate 9LA): an international, randomised, open-label, phase 3 trial. Lancet Oncol (2021) 22:198–211. doi: 10.1016/S1470-2045(20)30641-0
133. Horn L, Mansfield AS, Szczęsna A, Havel L, Krzakowski M, Hochmair MJ, et al. First-line atezolizumab plus chemotherapy in extensive-stage small-cell lung cancer. N Engl J Med (2018) 379:2220–9. doi: 10.1056/NEJMoa1809064
134. Chen Y, Paz-Ares L, Reinmuth N, Garassino MC, Statsenko G, Hochmair MJ, et al. Impact of brain metastases on treatment patterns and outcomes with first-line durvalumab plus platinum-etoposide in extensive-stage SCLC (CASPIAN): A brief report. JTO Clin Res Rep (2022) 3:100330. doi: 10.1016/j.jtocrr.2022.100330
135. Paz-Ares L, Dvorkin M, Chen Y, Reinmuth N, Hotta K, Trukhin D, et al. Durvalumab plus platinum–etoposide versus platinum–etoposide in first-line treatment of extensive-stage small-cell lung cancer (CASPIAN): a randomised, controlled, open-label, phase 3 trial. Lancet (2019) 394:1929–39. doi: 10.1016/S0140-6736(19)32222-6
136. Hubbeling HG, Schapira EF, Horick NK, Goodwin KEH, Lin JJ, Oh KS, et al. Safety of combined PD-1 pathway inhibition and intracranial radiation therapy in non–small cell lung cancer. J Thorac Oncol (2018) 13:550–8. doi: 10.1016/j.jtho.2018.01.012
137. Schapira E, Hubbeling H, Yeap BY, Mehan WA, Shaw AT, Oh K, et al. Improved overall survival and locoregional disease control with concurrent PD-1 pathway inhibitors and stereotactic radiosurgery for lung cancer patients with brain metastases. Int J Radiat Oncol Biol Phys (2018) 101:624–9. doi: 10.1016/j.ijrobp.2018.02.175
138. Enright TL, Witt JS, Burr AR, Yadav P, Leal T, Baschnagel AM. Combined immunotherapy and stereotactic radiotherapy improves neurologic outcomes in patients with non–small-cell lung cancer brain metastases. Clin Lung Cancer (2021) 22:110–9. doi: 10.1016/j.cllc.2020.10.014
139. Ahmed KA, Kim S, Arrington J, Naghavi AO, Dilling TJ, Creelan BC, et al. Outcomes targeting the PD-1/PD-L1 axis in conjunction with stereotactic radiation for patients with non-small cell lung cancer brain metastases. J Neurooncol (2017) 133:331–8. doi: 10.1007/s11060-017-2437-5
140. Scoccianti S, Olmetto E, Pinzi V, Osti MF, Di Franco R, Caini S, et al. Immunotherapy in association with stereotactic radiotherapy for non-small cell lung cancer brain metastases: results from a multicentric retrospective study on behalf of AIRO. Neuro Oncol (2021) 23:1750–64. doi: 10.1093/neuonc/noab129
141. Ettinger DS, Wood DE, Aisner DL, Akerley W, Bauman JR, Bahrat A. NCCN Clinical Practice Guidelines in Oncology (NCCN Guidelines®). (2023) United States: Non-Small Cell Lung Cancer. Available at: https://www.nccn.org/professionals/physician_gls/pdf/nscl.pdf.
142. Goetz P, Ebinu JO, Roberge D, Zadeh G. Current standards in the management of cerebral metastases. Int J Surg Oncol (2012) 2012:1–9. doi: 10.1155/2012/493426
143. Myall NJ, Yu H, Soltys SG, Wakelee HA, Pollom E. Management of brain metastases in lung cancer: evolving roles for radiation and systemic treatment in the era of targeted and immune therapies. Neuro-Oncology Adv (2021) 3:v52–62. doi: 10.1093/noajnl/vdab106
144. Dolled-Filhart M, Locke D, Murphy T, Lynch F, Yearley JH, Frisman D, et al. Development of a prototype immunohistochemistry assay to measure programmed death ligand-1 expression in tumor tissue. Arch Pathol Lab Med (2016) 140:1259–66. doi: 10.5858/arpa.2015-0544-OA
145. Horn L, Mansfield AS, Szczęsna A, Havel L, Krzakowski M, Hochmair MJ, et al. First-line atezolizumab plus chemotherapy in extensive-stage small-cell lung cancer. N Engl J Med (2018) 379:2220–9. doi: 10.1056/nejmoa1809064
146. Arriola E, González-Cao M, Domine M, De Castro J, Cobo M, Bernabé R, et al. Addition of immune checkpoint inhibitors to chemotherapy vs chemotherapy alone as first-line treatment in extensive-stage small-cell lung carcinoma: A systematic review and meta-analysis. Oncol Ther (2022) 10:167–84. doi: 10.1007/s40487-021-00182-0
147. Hwang WL, Pike LRG, Royce TJ, Mahal BA, Loeffler JS. Safety of combining radiotherapy with immune-checkpoint inhibition. Nat Rev Clin Oncol (2018) 15:477–94. doi: 10.1038/s41571-018-0046-7
148. He Q, Zhang C, Tang S, Li J, Ren Q. Intracranial radiotherapy with or without immune checkpoint inhibition for brain metastases: a systematic review and meta-analysis. Transl Cancer Res (2020) 9:5909–24. doi: 10.21037/tcr-20-902
149. Aldea M, Andre F, Marabelle A, Dogan S, Barlesi F, Soria J-C. Overcoming resistance to tumor-targeted and immune-targeted therapies. Cancer Discovery (2021) 11:874–99. doi: 10.1158/2159-8290.CD-20-1638
150. Ahmad A, Khan P, Rehman AU, Batra SK, Nasser MW. Immunotherapy: an emerging modality to checkmate brain metastasis. Mol Cancer (2023) 22:111. doi: 10.1186/s12943-023-01818-7
151. Escudero L, Martínez-Ricarte F, Seoane J. ctDNA-based liquid biopsy of cerebrospinal fluid in brain cancer. Cancers (Basel) (2021) 13:1989. doi: 10.3390/cancers13091989
152. Hickman RA, Miller AM, Arcila ME. Cerebrospinal fluid: A unique source of circulating tumor DNA with broad clinical applications. Transl Oncol (2023) 33:101688. doi: 10.1016/j.tranon.2023.101688
153. Ricciuti B, Wang X, Alessi JV, Rizvi H, Mahadevan NR, Li YY, et al. Association of high tumor mutation burden in non–small cell lung cancers with increased immune infiltration and improved clinical outcomes of PD-L1 blockade across PD-L1 expression levels. JAMA Oncol (2022) 8:1–9. doi: 10.1001/jamaoncol.2022.1981
154. Mitsuya K, Nakasu Y, Kurakane T, Hayashi N, Harada H, Nozaki K. Elevated preoperative neutrophil-to-lymphocyte ratio as a predictor of worse survival after resection in patients with brain metastasis. J Neurosurg (2017) 127:433–7. doi: 10.3171/2016.8.JNS16899
155. Cho A, Kranawetter B, Untersteiner H, Khalaveh F, Dorfer C, Rössler K, et al. Neutrophil-to-lymphocyte ratio is superior to other leukocyte-based ratios as a prognostic predictor in non–small cell lung cancer patients with radiosurgically treated brain metastases under immunotherapy or targeted therapy. World Neurosurg (2021) 151:e324–31. doi: 10.1016/j.wneu.2021.04.033
156. Derosa L, Routy B, Thomas AM, Iebba V, Zalcman G, Friard S, et al. Intestinal Akkermansia muciniphila predicts clinical response to PD-1 blockade in patients with advanced non-small-cell lung cancer. Nat Med (2022) 28:315–24. doi: 10.1038/s41591-021-01655-5
157. Gopalakrishnan V, Spencer CN, Nezi L, Reuben A, Andrews MC, Karpinets TV, et al. Gut microbiome modulates response to anti-PD-1 immunotherapy in melanoma patients. Sci (80- ) (2018) 359:97–103. doi: 10.1126/science.aan4236
158. Routy B, Le Chatelier E, Derosa L, Duong CPM, Alou MT, Daillère R, et al. Gut microbiome influences efficacy of PD-1–based immunotherapy against epithelial tumors. Sci (80- ) (2018) 359:91–7. doi: 10.1126/science.aan3706
159. Gopalakrishnan V, Spencer CN, Nezi L, Reuben A, Andrews MC, Karpinets TV, et al. Gut microbiome modulates response to anti–PD-1 immunotherapy in melanoma patients. Sci (80- ) (2018) 359:97–103. doi: 10.1126/science.aan4236
160. Matson V, Fessler J, Bao R, Chongsuwat T, Zha Y, Alegre M-L, et al. The commensal microbiome is associated with anti–PD-1 efficacy in metastatic melanoma patients. (2018) 359(6371):104–108.
161. Sivan A, Corrales L, Hubert N, Williams JB, Aquino-Michaels K, Earley ZM, et al. Commensal Bifidobacterium promotes antitumor immunity and facilitates anti–PD-L1 efficacy. Sci (80- ) (2015) 350:1084–9. doi: 10.1126/science.aac4255
162. Baruch EN, Youngster I, Ben-Betzalel G, Ortenberg R, Lahat A, Katz L, et al. Fecal microbiota transplant promotes response in immunotherapy-refractory melanoma patients. Sci (80- ) (2021) 371:602–9. doi: 10.1126/science.abb5920
Keywords: brain metastases, NSCLC, SCLC, immunotherapy, immune microenvironment
Citation: Rios-Hoyo A and Arriola E (2023) Immunotherapy and brain metastasis in lung cancer: connecting bench side science to the clinic. Front. Immunol. 14:1221097. doi: 10.3389/fimmu.2023.1221097
Received: 11 May 2023; Accepted: 15 September 2023;
Published: 09 October 2023.
Edited by:
Xabier Mielgo-Rubio, Hospital Universitario Fundación Alcorcón, SpainCopyright © 2023 Rios-Hoyo and Arriola. This is an open-access article distributed under the terms of the Creative Commons Attribution License (CC BY). The use, distribution or reproduction in other forums is permitted, provided the original author(s) and the copyright owner(s) are credited and that the original publication in this journal is cited, in accordance with accepted academic practice. No use, distribution or reproduction is permitted which does not comply with these terms.
*Correspondence: Edurne Arriola, ZWFycmlvbGFAcHNtYXIuY2F0
Disclaimer: All claims expressed in this article are solely those of the authors and do not necessarily represent those of their affiliated organizations, or those of the publisher, the editors and the reviewers. Any product that may be evaluated in this article or claim that may be made by its manufacturer is not guaranteed or endorsed by the publisher.
Research integrity at Frontiers
Learn more about the work of our research integrity team to safeguard the quality of each article we publish.