- 1Unità Operativa UO Patologia e Immunologia Sperimentale, IRCCS Ospedale Policlinico San Martino, Genova, Italy
- 2Animal Facility, IRCCS Ospedale Policlinico San Martino Genova, Genova, Italy
- 3Laboratory of Immunology and Biotherapy, Department of Human Pathology, University of Messina, Messina, Italy
- 4Dipartimento di Medicina Sperimentale, Università di Genova, Genova, Italy
- 5Department of Surgical Sciences, Bone and Dental Bioengineering Laboratory, C.I.R Dental School, University of Turin, Turin, Italy
- 6Department of Surgical Sciences (DISC), University of Genoa, Genoa, Italy
- 7“Epigenomics and Biomarkers of Solid Tumors”, Fondazione IRCCS Istituto Nazionale dei Tumori, Milan, Italy
Since the first studies, the mouse models have provided crucial support for the most important discoveries on NK cells, on their development, function, and circulation within normal and tumor tissues. Murine tumor models were initially set to study murine NK cells, then, ever more sophisticated human-in-mice models have been developed to investigate the behavior of human NK cells and minimize the interferences from the murine environment. This review presents an overview of the models that have been used along time to study NK cells, focusing on the most popular NOG and NSG models, which work as recipients for the preparation of human-in-mice tumor models, the study of transferred human NK cells, and the evaluation of various enhancers of human NK cell function, including cytokines and chimeric molecules. Finally, an overview of the next generation humanized mice is also provided along with a discussion on how traditional and innovative in-vivo and in-vitro approaches could be integrated to optimize effective pre-clinical studies.
Introduction
Natural Killer (NK) cells are Innate Lymphoid Cells (ILCs) playing a crucial role in the anti-tumor and antiviral immunity (1–4). They carry out their function both by influencing and supporting the activity of different immune cell types (including myeloid cells and T lymphocytes), and by directly killing the target cells (5–8). Through the release of chemoattractant factors (CCL3, CCL4, CCL5, HMGB1) and cytokines (IFN-γ, TNFα, and GM-CSF), they can favor recruitment of immune cells, including NK cells (9, 10), and promote Th1-type inflammatory responses, while through the expression of cell death receptors and, especially, the release of cytotoxic granules they exert a potent lytic activity against tumor and virally infected cells. The activation of the different NK cell functions is orchestrated by the cytokine milieu and, more specifically, it depends on the type of cellular interactions involving NK cells. The cell-to-cell contact with normal cells, certain immune cells, or altered cells can give rise to inhibitory, regulatory, or lytic immune synapses respectively (11), with different patterns of engaged NK receptors, including: inhibitory, activating, or cytokine receptors. In different species, the discrimination between normal cells and altered tumor or virally infected cells is carried out through the action of MHC-I-specific inhibitory receptors, and activating receptors recognizing stress-induced molecules or ectopically expressed antigens. In humans, major inhibitory receptors are represented by certain Killer Ig-like Receptors (KIRs) and the CD94:NKG2A heterodimer, while the most known tumor-recognizing receptors include the Natural Cytotoxicity Receptors (NCRs) (NKp46, NKp30, and NKp44), NKG2D, and DNAM-1 (12, 13). Remarkably, the coordinated engagement of some inhibitory and activating receptors, together with cytokine receptors are involved in complex regulatory cross-talks with immune cells.
This picture of NK cell function and mechanics is the result of the many studies that have been carried out in vitro over the 90s and across the millennium to dissect single molecular pathways and cellular interactions. These results were then impressively expanded with the advent of new global and in-depth cell analytical approaches, such as the “omics” and “single cell RNA-seq”. Application of these techniques led to the identification and fine molecular characterization of new functional NK cell subsets, as naïve or adaptive NK cells, or tumor-associated NK cells (14–16). Despite such important technical advances have significantly extended the power of human in vitro and ex-vivo studies, the still open issues on NK cell differentiation, circulation, homing, tissue residency, and tumor penetration cannot disregard the option of the animal studies.
Along the timeline of the NK cell studies, the utilization of murine models proved crucial for the initial evaluation of the real anti-tumor effects of NK cells and gave the first molecular hints on the role of MHC-I recognition (17–20). Additional studies provided supportive information on how NK cells differentiate, acquire and regulate their cytolytic potential (through the “licensing/arming” process), and, even more strikingly, persist in vivo as memory-like cells (21–24). These new insights are providing important hints for the selection and preparation of optimized NK cell effectors for anti-tumor immuno-therapies, with promising results in the field of hematological malignancies. On the other hand, the successful exploitation of NK cells in the cure of solid tumors is still limited by the active role of the local tumor microenvironment (TME), which hinders infiltration and effector capabilities of NK cells (25, 26). Several in vitro studies have contributed to the fine dissection of the different molecular mechanisms underlying the suppressive/escape properties of the TME. Nevertheless, the murine models are providing the crucial answers on how each of these mechanisms, and its targeting, could impact on the real anti-tumor efficacy of NK cells within the complexity of the TME. Given the importance of this issue, many efforts are being spent to generate ever more effective models to study solid tumors, moving from the full murine to the human-in-mice or humanized models, trying to overcome the not-always obvious problems related to the inter-species differences.
Mouse models for the study of mouse cancer
Murine models are well-characterized systems useful to gain insights into human biology and various pathological conditions, including cancer. Indeed, through the development of specific strains with stable and well-known genetic background, the murine system offers defined and reproducible experimental conditions without losing the complexity of living organisms.
The initial studies to identify and molecularly characterize the anti-tumor functions of NK cells in vivo were performed on mice injected with murine malignant cells. By the use of a C57BL strain and its “beige” variant, characterized by reduced NK cell activity related to the beige-J spontaneous mutation Lystbg-J, it was demonstrated the active role of NK cells in contrasting tumor growth (18, 19). Then, experiments based on the injection of H-2 mismatched or H-2 negative tumor cells in mice bearing given H-2 haplotypes provided important information on the role of the MHC-I-specific NK receptors (27, 28). Finally, the development of induced or spontaneous tumorigenesis mouse models combined with the generation of specific gene silencing (KO mice) were crucial to demonstrate the key role of certain activating NK receptors in vivo. Thus, for instance, in the model of transgenic adenocarcinoma of the mouse prostate (TRAMP) the targeting of the klrk1 gene (TRAMP-NKG2D-KO mice) resulted in higher tumor incidence and the development of larger tumors (29). Similarly, the inactivation of the cd226 gene (DNAM-1-KO mice) favoured the growth of the (3-MethylColanthrene (MCA)-induced fibrosarcoma in BALB/c mice (30). Finally, the inactivation of the Ncr1 gene affected the ability of C57BL/6 mice to control melanoma and lung carcinoma metastases, or lymphoma induced by tumor cell line injection (31, 32).
The “mouse in mouse” studies provided important hints for the characterization of the role of human NK cells in the context of the solid tumors, as many murine NK cell receptors presented human homologues, and homologies between human and mice also emerged studying the development of NK cells, the regulation of their functional properties, and the acquisition of memory-like features. However, although these models have been exceedingly important in advancing our knowledge, there are limits and functional differences that have to be accounted for, especially, considering the translation of the obtained data to the design of new immunotherapy strategies (33). In this context, it has become ever more important the development of models enabling the study of human environments in the mouse.
Mouse models for the study of human cancer
The growth of human tissues and cells in a different species (xenotransplant) requires evasion from the immune system to prevent rejection. Xenotransplants became possible after the discovery, in 1962, of the nu/nu spontaneous mutation (34). Nu/nu Nude mice lacked the thymus, showed T-cell deficiency and impaired B-cell functionality, but retained macrophages and NK cells. Another spontaneous mutation affecting the immune system was discovered in 1983 in C.B-17 mice. Such autosomal recessive mutation, named Prkdcscid, affects the gene encoding for the protein kinase, DNA activated, catalytic polypeptide (PRKDC) and gives rise to severe combined immunodeficiency (SCID) in the mice. SCID mice (i.e. homozygous Prkdscid mutants) lacked both T and B cells and had reduced NK cells (35), however their phenotype was “leaky” and clones of functioning B and T cells could randomly develop in young animals (36). Altered immunity was also observed in nonobese diabetic (NOD) mice (37, 38). These mice were obtained by a combination of inbreeding and selective breeding from a progeny of an outbred Jcl:ICR mouse, and were initially selected as a tool to study autoimmune diabetes, given their genetic predisposition to the development of the disease. However, NOD mice were also characterized by defects in the innate immunity, including the NK cell compartment, and were then considered for the preparation of new immunocompromised models. In particular, by crossing C.B-17 Prkdcscid males with NOD/ShiLtSz females, it was generated the NOD-SCID mouse strain. Transfer of the SCID mutation onto a non-obese diabetic (NOD) background eliminated the leaky phenotype of the SCID model and generated the NOD-SCID mouse strain that rapidly became the best choice for transplant studies using freshly isolated human tumour cells, selected cancer stem cells, or tumour fragments (39, 40). NOD-SCID mice lack T and B lymphocytes and display reduced NK cell function, resulting in increased xenotransplant engraftment. However, they have a limited lifespan (7-8 months), due to the frequent spontaneous development of thymic lymphomas (41). Despite their limits, nu/nu, SCID and NOD-SCID mice have represented the most used strains for xenotransplant of human tumour cell lines in the last 50 years. A great improvement in the rate of human cell engraftment was then obtained with the backcrossing of NOD-SCID mice with either truncated or deleted interleukin-2 receptor common gamma chain giving origin to the NOG (42) or NSG (43, 44) strains, respectively. Specifically, in the early 2000s the NOD.Cg-PrkdcscidIl2rgtm1Sug (NOG) strain was developed at the Central Institute for Experimental Animals, Japan, by backcrossing NOD/ShiJic-Prkdcscid mice with IL2rgtm1Sug/ShiJic mice, carrying a truncation of the intracellular signalling domain of the IL2 receptor gamma chain. The NOD-SCID gamma NOD.Cg-PrkdcscidIl2rgtm1Wjl/Szj (NSG) strain, instead, a brand from the Jackson Laboratories (USA), was developed by backcrossing NOD-SCID mice with B6.129S4-IL2rgtmWjl/J (IL2Rγnull) carrying the knock-out of the IL2 receptor gamma chain. NSG and NOG mice lack mature T and B cells, NK cells, and complement, have defective macrophages and dendritic cells (DCs) and gave a great impulse to the development of humanized mice models. In particular, these mice represent elective recipients for the functional studies on circulating tumor cells.
Cancer tissue represents a quite complex biological system, with its peculiar microenvironment and heterogeneity of the malignant and non-malignant components (45, 46). Therefore, the strategies to generate human tumor tissues in immunocompromised mice were generally conceived with the aim of reproducing at best such original complexity. A valid approach in this field has been the setting up of Patient Derived Tumour Xenografts (PDX), i.e. the engraftment of small pieces of freshly collected tumour samples in immunocompromised mice. PDXs warrant the conservation of the original tumor components (47–49) and, for that reason, are widely used for maintenance and therapy response experiments. In one study on lung cancer PDX, it has also been shown that lymphocytes present in the transplanted tumor (tumor infiltrating lymphocytes - TIL) could partly reconstitute the immune system in the mouse. Remarkably, in these mice, the combined treatment with IL-15 and PD-1 inhibition could induce tumor regression, which was dependent on human (tumor-derived) NK and T cells (50). Therefore, the model could provide important information on the interaction of the immune system with the tumor in the autologous setting. The use of this model, however, appear to be limited by the possible variability of the TIL component in different engrafted tumor fragments. Moreover, it should be considered that murine stroma can replace human one after two passages in the mice, and a cross-talk between the human tumor component and murine tumor-associated cells can easily take place in these models.
Another approach comes from the observation that certain human tumor cell populations, especially those containing a cancer stem cell component, can generate malignant lesions, partly recapitulating the original tumor complexity, once injected into immunocompromised mice. Following this observation, several human-in-mice tumor models have been reproduced and studied through the injection of selected tumor cells in NOD-SCID or NSG mice. An interesting example is represented by the attempt to reproduce a bone metastasis of human breast cancer or NSCLC in a human bone fragment that has been subcutaneously implanted in SCID mice (51, 52). In the case of NSCLC, it has been possible to identify a subset of human NSCLC cells endowed with metastasis-initiating-cell properties. Those cells, which were marked by the CD133+CXCR4+EpCAM- phenotype, were shown to colonize the bone implant and to generate tumor lesions.
The immunocompromised mice are optimal recipients for the generation and development of human tumours, but their altered, non-human, immune system represents an issue. Indeed, cancer is a dynamic disease, showing plasticity in its cellular and extracellular components, and its dynamics is often influenced by the interaction with the host and, signally, by the selective pressure of the immune system (46). Therefore, the lack of the human immune system in these murine models represents a limit, both for the attainment/maintenance of reliable tumor units, and for the studies focused on the development of immunotherapy strategies.
For this reason, there has been a flourishing interest in the “humanized mice models”: immunocompromised mice transplanted with hematopoietic precursors and/or lymphoid tissues capable of reproducing in the mice several types of human immune cells.
Humanized mice
Engraftment of human lymphoid cells in SCID mice was first reported in 1988 by Mosier and co-workers, who injected intraperitoneally human peripheral blood mononuclear cells (PBMC) from EBV-positive donors and obtained “stable long-term reconstitution of a functional human immune system” (53). However, only human T and B cells could be satisfactorily maintained in these mice, while engraftment of other hematopoietic lineages was not effective. An additional problem was represented by the development in the animals of xenogeneic graft-versus-host-disease (GvHD), which usually took place 4-6 weeks after transfer of huPBMC, thus shortening the available observation time (54, 55). Nevertheless, given its relatively easy preparation, this model has been used over time, and, recently, the generation of NSG mice carrying a deletion of the MHC-I or II genes has been proposed to overcome the problem of xenogeneic GvHD (56).
Encouraging results were also obtained by transferring CD34+ human hematopoietic stem cells (HSC) from cord blood into new-born BALB/c Rag2-/-γc-/- mice by intrahepatic injection. However, the reconstitution level remained low (57). The use of NOD-SCID mice carrying the deletion of the Rag-1 or Rag-2 gene did not change significantly the outcome (58). In these models, T cell function was limited, probably due the absence of human lymphoid organs supporting T cell maturation, selection, and activation. To overcome this problem, in a new study, CD34+ HSC were transferred into NOD-SCID mice that were previously transplanted with human foetal liver and thymic tissue in the renal capsule (hu-bone marrow-liver-thymus - BLT) (59, 60). hu-BLT mouse model exhibits a full reconstitution of human immune cell repertoire, with T cells capable of mounting HLA-II- and HLA-I-restricted specific responses. These models were initially prepared to assess the immune response to viral infections (namely HIV), and were then adapted for studies of onco-immunology (61). Their employment for the study of NK cells in the tumor, however, appeared to be limited by the fact that BLT-mice developed relative low numbers of, poorly functional, NK cells.
The advent of the next generation murine models is now providing specific tools to study restricted human immune cell types in selected human tumors. These models are generated by using “knock in” and “knock out” strategies in immunocompromised mouse background, and can reach considerable complexity (62). Table 1 summarizes the main models that can be used for the study of human NK cells in vivo. An important issue that is generally considered in the setting of humanized mice regards murine cytokines, which may be poorly expressed in immunocompromised mice and, due to the evolutionary divergence, quite different from their human counterparts. Therefore, several models have been developed expressing human cytokines, generally in NOG or NSG background, with the aim to improve development or persistence of given human immune cells, including NK cells (Table 1).
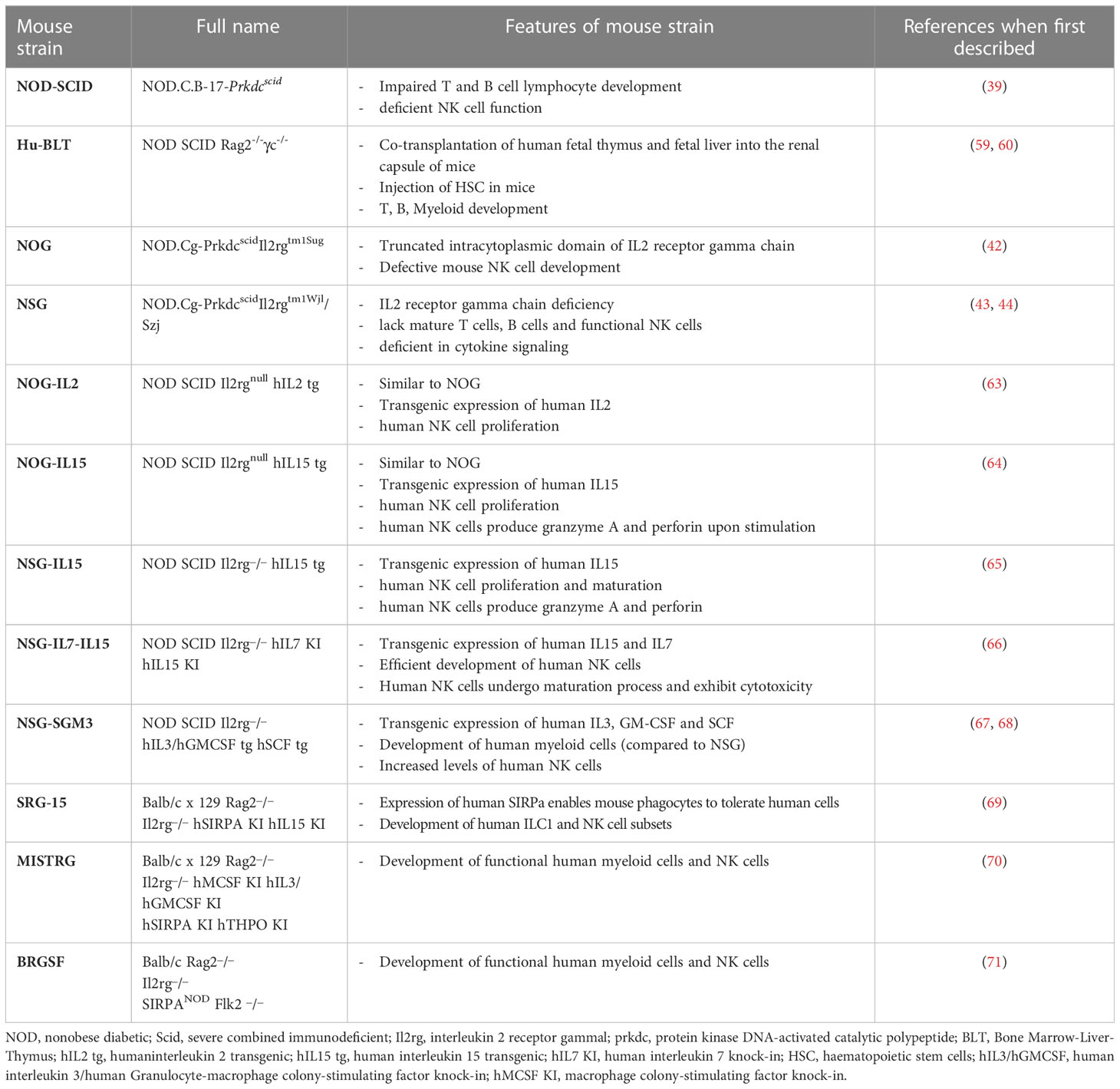
Table 1 Summary of “first” and “next” generation immunodeficient mouse strains to study human NK cells in human solid tumors.
Katano et al. established transgenic NOG mice sub-strains expressing either human IL-2 (NOG-hIL-2 Tg) or human IL-15 (NOG-hIL-15 Tg) (63, 64). In NOG-hIL-2 Tg mice, human cord blood-derived HSCs could mature and give rise to differentiated KIR+ NK cells expressing IL-2–activated phenotype; while in NOG-hIL-15 Tg mice, the high levels of IL-15 in the blood could support survival of NK cells from transferred human PBMC. hIL-15 has also been expressed in NSG, and the strain NSG-IL-15 Tg is now commercially available (65). Recently, to improve the development of human NK cells, Matsuda et al. created hIL-7 and hIL-15 double knock-in (hIL-7xhIL-15 KI) NSG mice (66). Compared to NSG mice, these mice showed increased ability to develop human NK cells after engraftment of human hematopoietic stem cells. A hIL-15 and human signal regulatory protein alpha (hSIRPα) double knock-in mouse on a Rag2-/- il2rg-/- background (SRG-15) was described to support efficient development of innate lymphoid cell subsets and NK cells, including tissue resident cells (69, 72). Even more complex knock in mice have been generated that express different combinations of human factors such as IL-3, IL-15, GM-CSF, M-CSF, SIRPα, and thrombopoietin: NSG-SGM3, MISTRG and BRGSF (see Table 1). On the whole, these latter strains support the development of human myeloid cells, which in turn can produce IL-15 and improve development of functional human NK cells (67, 68, 70, 71). Finally, a potentially interesting humanized model is also represented by the Nonirradiated NOD.B6.SCID Il2rg-/- kitw41/w41 (NBSGW) mouse, which was obtained by the crossing of NSG strain with the C57BL/6.-KitW-41J/J strain (73). This model has the interesting advantage of supporting the human HSC engraftment without the need for pre-transplant conditioning (i.e. γ-irradiation), which could negatively affect the host microenvironment for the HSC development (74). An ovarian cancer PDX has been now developed in NBSGW mice, engrafted with human cord blood-derived HSC, to study the tumor immune environment within the peritoneal cavity (75). To date, such NBSGW model has not been used yet to study human NK cells.
Some of the above-mentioned next generation humanized mice have been assayed in different xenogeneic solid tumor models to study various immune cell types, especially T cells and myeloid cells, and only rarely NK cells (Table 2). Indeed, as yet, the large majority of the studies on NK cells have been done on “traditional” NOG or NSG mice. Nevertheless, the strategy of transferring human NK cells in such immunocompromised mice proved effective to evaluate which NK cell preparation, or way of cell activation, would be more suitable to overcome the tumor escape mechanisms and to deliver efficient NK cells into the tumor niches.
Human NK cell transfer in human-in-mice tumor models
As mentioned above, the human-in-mice tumor models, combined with human NK cell transfer, represent a suitable mean to assess the real effects of NK cells at the tumor site. Through these studies, it is possible to gain knowledge on spatial localization of NK cells within the tumor, and understand their impact on stroma, tumor cells and other immune cell populations. Likewise, it is possible to characterize phenotype and function of tumor-infiltrating NK cells, and, also, to gain information on their persistence. Therefore, on the whole, these models offer a platform to test possible approaches to optimize survival, function, and safety of adoptively transferred human NK cells. As described below, the animal model receiving human NK cells is generally represented by the NSG mice.
Study of NK cells exposed to cytokines
One of the major limitations to the efficacy of adoptive NK cell therapy is represented by the poor tumor-infiltrating capacity of transferred NK cells (90). The generation of sufficient NK cell numbers for adoptive immunotherapy represents an additional issue. Several strategies, often involving the use of priming cytokines, are employed to expand ex vivo NK cells exhibiting distinct phenotypes, which can be correlated with their anti-tumor potency in vitro and in vivo (91). Cytokines are then used in vivo to sustain persistency and activation of transferred NK cells.
IL-2 has been the most commonly used cytokine to expand NK cells ex vivo and to boost proliferation of adoptively transferred NK cells in vivo (92–95). These IL-2-based therapies, however, generally demonstrated poor efficacy at the bed side, even showing negative side effects. The limits of IL-2 were essentially related to its ability to induce Tregs (with consequent suppressive effects on NK cells) (96–98) and to promote Activation Induced Cell Death (AICD) on NK cells. This latter aspect could be particularly relevant in the context of the solid tumors, as IL-2-primed NK cells undergo AICD following interaction with endothelial cells, with a possible negative impact on extravasation and tumor infiltration (99). Finally, it cannot be disregarded that IL-2 can contribute to the vascular leak syndrome, an important adverse effect that limits its use in therapy (100). In the attempt to improve suitability of IL-2 for immunotherapy, engineered IL-2 molecules (termed superkines) have been developed. Compared to IL-2, IL-2superkines induced superior expansion of cytotoxic T cells, more prolonged and more intense NK cell activation, and improved anti-tumour responses in different syngeneic mouse models (101–103). These studies, however have not yet been conducted using human-in-mice models.
Combining IL-2 with other cytokines to pre-activate NK cells before transfer could improve NK cell efficiency, limiting the IL-2-related negative effects. A candidate for such approach may be IL-21, which has been shown to induce expansion of non-terminally differentiated CD56bright NK cells (104) and functional NK cell maturation (105, 106). The efficacy of human NK cells that were expanded ex vivo using feeder cells + IL-2 + IL-21 was analysed in NSG mice injected with a human melanoma cell line. Adoptive transfer of such pre-activated NK cells resulted in increased in vivo persistence, as compared to NK cells pre-treated with IL-2 alone, and in significant inhibition of melanoma-induced lung metastases (107).
IL-15 has been proposed as valid alternative to IL-2 to stimulate NK cells for therapeutic protocols. IL-15 and IL-2 are structurally similar, share two of the three subunits of their receptors (namely, IL-2Rβ/CD122 and γc/CD132), and are both capable of activating NK cells. However, the presence of distinctive receptor alpha subunits (IL-15Rα/CD125, and IL-2Rα/CD25) accounts for their different behaviour in vitro and in vivo. Thus, for example, IL-15 supports NK cell survival, proliferation and effector functions, but, different from IL-2, it has no stimulatory effect on the CD25+ Tregs (108). In addition, IL-15 can be associated to the IL-15Rα and trans presented to NK and T cells by different immune and non-immune IL-15Rα+ cells (109, 110). In different pre-clinical models of solid tumors, IL-15, alone or in combination with additional cytokines (i.e. IL-2, IL-12, and IL-18), has been assayed to stimulate human NK cells before transfer, and/or to support human NK cell persistency after transfer. For example, in a study aimed at evaluating the anti-tumor properties of human NK cells generated ex vivo from HSPC, recombinant IL-15 was infused together with NK cells in NSG mice bearing ovarian carcinoma, and this infusion was then followed by a further boost of IL-15 (111). The study, showed efficacy of HSPC-derived NK cells in reducing tumor burden, and was then followed by a clinical trial (NCT: 03539406), which is still ongoing. In this study, patients with recurrent ovarian cancer, pre-treated or not with chemotherapy, are infused intra-peritoneally with allogeneic NK cells generated from UCB CD34+ HSPC. Once completed, this trial will give crucial information on feasibility, safety and toxicity of this innovative therapeutic approach. In a xenografted mouse model of colon rectal cancer (CRC), human NK cells were first expanded in vitro in the presence of IL-15 and IL-2, then were transferred into the mice combining infusion of NK cells and IL-2. Further boosts of IL-2 were given to the mice to maintain NK cells in vivo. NK cell transfer induced a delay in tumor growth at an early stage (112).
To improve biological activity and to extend in vivo half-life of IL-15 (≈40 min), a super-agonistic molecular complex has been developed that combines mutated activating IL-15, the trans-presenting IL-15Rα sushi domain and IgG1-Fc (113). Such super-agonist, termed N-803 (also known as ALT-803) has been tested in an ovarian carcinoma model, where it was demonstrated to promote HPC-NK cell expansion and functionality (114, 115). First reported clinical trials (NCT: 01885897) (NCT: 01727076) of ALT-803 in cancer patients revealed that it is well tolerated and stimulates NK cell activation and expansion and CD8+ T cells, but not Tregs (116, 117).
IL-15 is also the main component of the IL-12/15/18 cytokine cocktail known to induce the so-called cytokine-induced memory-like (CIML) NK cells. These cells, which can be easily induced in vitro from PB NK cells, display enhanced anti-tumor effector functions that can be preserved in vitro and even increased in vivo (118–120). CIML-NK cells have been initially considered for the cure of hematologic malignancies, and clinically assessed for the immunotherapy of acute myeloid leukemia (AML) (NCT: 01898793) (118, 120). Interestingly, in this clinical study CIML NK cells were shown to persist in the patients, and further differentiate. With regard to solid tumors, CIML NK cells have not yet been assessed in clinics, however they revealed potent anti-tumor activity after transfer in xenogenic melanoma and ovarian cancer NSG mouse models (121, 122). As CIML NK cells have been shown to express CD25, mice bearing the melanoma xenograft were given serial boosts of IL-2 to support persistency and activation of transferred NK cells.
An additional interesting anti-tumor effector lymphocyte population is represented by Cytokine Induced Killer (CIK) T cells. These effectors, which can be expanded in vitro by exposure to IFN-γ and IL-2, are characterized by the expression of the CD56 NK cell marker and the activating NKG2D receptor. Remarkably, NKG2D supports their TCR-independent anti-tumor activity, which has been demonstrated both in vitro and in xenogeneic tumor models developed into NOD-SCID mice (123–125). Given the promising results obtained in vitro and in vivo, the use of CIK could contribute to innovative clinical approaches for both hematologic and solid malignancies (126, 127).
Study of CAR-NK and NK cell engagers
Innovative tools to enhance and address anti-tumor NK cell function are represented by engineered chimeric activating receptors (CARs), whose expression is induced on NK cells by transduction protocols to generate CAR-NK, or by multivalent soluble molecules, which are developed as NK cell engagers. In both the cases, the effectiveness of the effector molecules or cells has been evaluated in xenogeneic tumor models.
CARs are generally constituted by a single-chain Fragment variable (scFv), recognizing a specific tumor-expressed antigen, combined with an intracytoplasmic tail of activating transducing molecules (such as the CD3ζ chain). Once expressed on transduced T or NK cells, CARs drive recognition of tumor cells and the subsequent triggering of the effector functions. CARs have been initially set to enhance and address T cell anti-tumor activity and several studies have already been done on these engineered effectors. Therapy with CAR-T, however, appears to be still hampered by several issues, including T cell exhaustion, the appearance of suppressive/regulatory responses, the induction of the cytokine release syndrome (CRS), and even GVHD (128). Given their features, NK cells could be advantageously employed to generate CAR-effectors with minimized side effects.
CAR-NK have been developed especially for hematological malignancies (129, 130), while for solid tumors the studies are still limited. An interesting CAR-NK effector targeting solid tumors has been prepared by transducing ex-vivo expanded human NK cells to express the DAP-12-anti-HLA-G CAR. HLA-G molecules are ligands for the inhibitory immune receptors, LILRB1 and LILRB2 (also known as ILT2 and ILT4) (131), and are frequently expressed by solid tumors, therefore, anti-HLA-G CAR-NK can both target tumor cells and relieve immunosuppression. These anti-HLA-G CAR-NK showed tumor cytotoxicity in orthotopic xenograft models of triple negative breast cancer and glioblastoma, developed in NSG mice (132).
Several bivalent or trivalent NK cell engagers have been synthesized and defined with different acronyms depending on the used technical platform and the originating lab. Bi- or tri-specific killer engagers (BiKE or TriKE) consist of a single-chain Fragment variable (scFv) targeting tumor specific antigens, a scFv targeting activating receptors on NK cells (generally CD16), and (in the case of TriKEs) an additional domain generally targeting cytokine receptors to support activation and survival of NK cells (133, 134). The therapeutic efficacy of BiKEs/TRiKEs with different tumor antigen specificities has been demonstrated in various xenograft tumor models, using NSG recipients and evaluating transferred human NK cells (135–138). In a study, the authors analysed the effectiveness of both the engager (which was the bi-specific anti-CD30/CD16 antibody) and of different NK cell effectors, by transferring into the mice either CIML-NK or cord blood-derived NK cells (139).
Recently, a trifunctional NK cell engager (NKCE) triggering simultaneously NKp46 and CD16 on NK cells and targeting a tumor antigen has been developed (140). An upgrade of this engager is then represented by the tetraspecific antibody-based natural killer cell engager therapeutics (ANKETs), which adds the ability to engage IL-R2b to the previous multiple specificity (141). For the preclinical studies, engagers with surrogate anti murine NKp46 were generated and evaluated for their capability to trigger murine NK cells in SCID mice injected with the RAJI or other human lymphoma cells. In some cases, the RAGko huNKp46Tg mice were used to better track NK cells in the tissues, as in these mice NK cells expressed both the human and the murine NKp46, and could be stained by anti-human NKp46 abs without the interference of the anti-murine ANKET.
Several engagers proved promising in the preclinical models, so that phase I/II clinical trials in advanced solid tumors are ongoing (133).
Other ways to enhance NK cell activity to solid tumors
As mentioned above, the use of irradiated feeder cells combined with cytokines represents a possible strategy to obtain large-scale expansion and activation of NK cells. A recently proposed approach involves, as feeder cells, the NK-92 cell line engineered to express OX40L and to secrete neoleukin-2/15, an artificial peptide that binds with high affinity the human IL-2Rβγ complex. These engineered NK-92 cells were irradiated and used to expand ex vivo-derived human NK cells, which were then transferred in three different xenogeneic tumor mouse models: lung, liver, or ovarian cancers, all developed into NOD-SCID mice. In these experiments, NK-92-induced NK cells showed stronger capability to infiltrate the tumors and a higher antitumor effect compared to NK cells expanded with IL-2 (142).
Chemical approach to increase the antitumor activity of NK cells was reported by Choi et al. who showed how 25kDa branched polyethyleneimine (25KbPEI) could enhance cytotoxicity and migration properties of human NK cells. 25KbPEI-induced NK cells were transferred into xenogeneic breast and ovarian cancer models, which were developed in SCID/nude and NSG mice respectively. In both models 25KbPEI-induced NK cells were demonstrated more effective than IL-2-induced NK cells in infiltrating the tumor and limiting its growth (143).
Study of the strategies to sensitize tumor cells to NK cell activity
More recently, human tumor xenografts in NSG mice have also been used to evaluate strategies directly targeting the tumor to increase its susceptibility to NK cells. Most strategies are focused on the study of genotoxic or non-genotoxic agents acting on the DNA-Damage-Response (DDR) or related pathways, which lead to increased expression of activating NK Receptor ligands (144). These agents are first evaluated in vitro and then validated in vivo. Thus, for example, in a xenogeneic neuroblastoma model, mice were given nutlin3a, a non-toxic p53-activating molecule that was demonstrated to increase expression of NKG2D- and DNAM-1-ligands on tumor cells. These mice, compared to those receiving vehicle alone, showed increased NK cell infiltration in their tumor xenografts and reduced tumor growth following human NK cell transfer (95). Analogously, in a xenogeneic ovarian carcinoma model, it has been demonstrated that the chemotherapeutic drug gemcitabine increased the expression of NKG2D ligands and death receptors on tumor cells, and the adoptive transfer of NK cells in combination with gemcitabine additively decreased ovarian cancer growth (145). Finally, in a recent study, combined high-dose radiotherapy and adoptive human NK cell transfer resulted in improved tumor control over monotherapies in NSG mice engrafted with melanoma and pancreatic tumor cells. Such improvement, however, appeared to be related to the radiotherapy-induced CXCL8 release by tumor cells and subsequent recall of CD56dim cytotoxic NK cells (146).
Final considerations and future development
The increasingly sophisticated analytic tools for the in-vivo and ex-vivo characterization of tumors are improving clinical decision making, and also, provide means for conducting research directly in the patients. These new approaches add support to the preclinical research but do not replace its fundamental phases, which comprise the studies in vitro to dissect molecular and cellular processes, and the validation studies in vivo.
The “traditional” 2D in vitro cultures address important questions on specific biological, genetic and epigenetic features and on the direct cellular effects of drugs or cytokines, while animal models are amply used to evaluate the actual significance of the dissected processes and to test therapeutic efficacy of drugs and dose-limiting toxicity of clinical treatments. On the other hand, the animal studies are generally expensive, time-consuming, and, for years, they have been based on a limited range of models (147, 148). Furthermore, there are ethical issues, which, for the animal welfare, limit the use of animals to what is strictly necessary. The principle of the 3Rs (Replacement, Reduction, and Refinement) is presently applied in all projects involving the use of animals. In particular, the Replacement is supported by the development and use of predictive and robust models and tools, based on the latest in vitro technologies, to address important scientific questions (149).
In this context, the setting of the 3D cultures has been proposed as an important tool for the development of innovative in vitro assays and models of neoplastic cell growth with potentially high clinical relevance. By the setting of 3D models, it is tempting to partly reproduce the complexity of the TME, in terms of extra-cellular matrix, cell types, vasculature, and oxygen, nutrients and catabolites distribution. If fully realized, these 3D tumor models would be a powerful mean to study a number of tumor-related processes, to validate specific findings, and to test the efficacy/toxicity of drug treatments, replacing the employment of animals (150, 151). This goal, however, is presently far to be achieved, as it is not easy to set a reliable balance among all the different fundamental components within a single in vitro-generated structure.
Therefore, the employment of animals remains indispensable for the preclinical studies, especially considering the recent development of the next generation humanized mouse models (65, 76–79, 81–89) (Table 2). Indeed, these models can reproduce ever more accurately the complexity of the immune response within the TME, and also offer the interesting perspective of dissecting in vivo crucial frames of such complexity. In this context, these models also represent suitable tools to study the behaviour of human NK cells. In conclusion, a powerful strategy to efficiently struggle against cancer should consider the wise integration of the different available tools to gain precise data on the single malignancies, to dissect molecular and cellular pathways, and to evaluate new related therapeutic strategies (Figure 1). In this scenario, in which different strategies are connected, the animal models maintain a pivotal role.
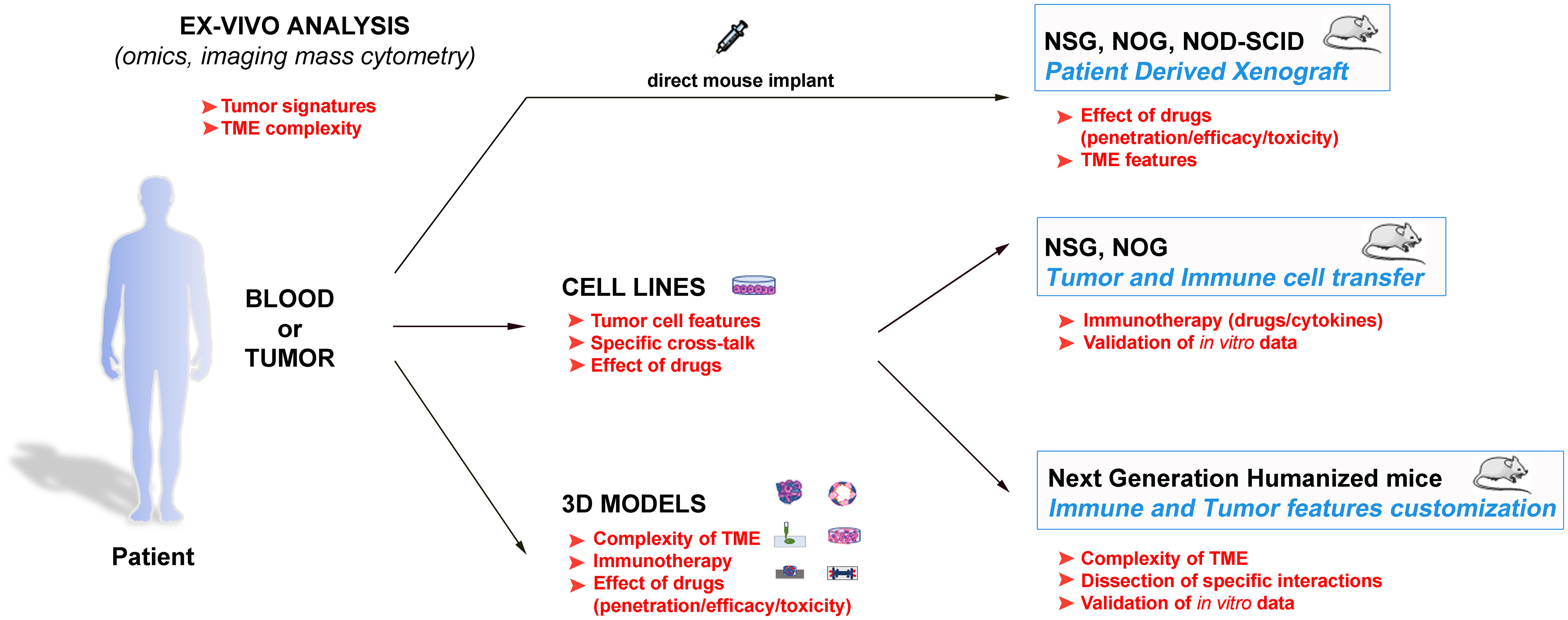
Figure 1 Schematic representation of the different available approaches to get insights in the tumour biology, and to design innovative and personalized therapies. These strategies span from the direct evaluation of the patients’ samples with advanced analytic techniques to the tumour complexity reproduction and the data validation in both in vitro and in vivo models. The main goals of the various approaches are indicated in red, and suggest how they can be inter-connected to obtain optimal results.
Author contributions
MP, SA, MV, and PO contributed to conception and the outline of the review article. All authors contributed to the article and approved the submitted version.
Funding
Fondazione AIRC, grant number IG 2020 id. 25023 (MV); Italian Ministry of Health (project RF-2018-12366714 (MV, GB); 5permille 2022-24 IRCCS Ospedale Policinico San Martino (to GP); Ricerca Corrente 2022-24 IRCCS Ospedale Policlinico San Martino (to GP and SA); Fondazione CRT 2020 (to IR).
Conflict of interest
The authors declare that the research was conducted in the absence of any commercial or financial relationships that could be construed as a potential conflict of interest.
The reviewer VL declared a shared affiliation with the authors RF and IR to the handling editor at the time of review.
Publisher’s note
All claims expressed in this article are solely those of the authors and do not necessarily represent those of their affiliated organizations, or those of the publisher, the editors and the reviewers. Any product that may be evaluated in this article, or claim that may be made by its manufacturer, is not guaranteed or endorsed by the publisher.
References
1. Vivier E, Artis D, Colonna M, Diefenbach A, Di Santo JP, Eberl G, et al. Innate lymphoid cells: 10 years on. Cell (2018) 174(5):1054–66. doi: 10.1016/j.cell.2018.07.017
2. Cantoni C, Grauwet K, Pietra G, Parodi M, Mingari MC, De Maria A, et al. Role of NK cells in immunotherapy and virotherapy of solid tumors. Immunotherapy (2015) 7(8):861–82. doi: 10.2217/imt.15.53
3. Sivori S, Meazza R, Quintarelli C, Carlomagno S, Della Chiesa M, Falco M, et al. NK cell-based immunotherapy for hematological malignancies. J Clin Med (2019) 8(10). doi: 10.3390/jcm8101702
4. Bjorkstrom NK, Strunz B, Ljunggren HG. Natural killer cells in antiviral immunity. Nat Rev Immunol (2022) 22(2):112–23. doi: 10.1038/s41577-021-00558-3
5. Crinier A, Narni-Mancinelli E, Ugolini S, Vivier E. SnapShot: natural killer cells. Cell (2020) 180(6):1280– e1. doi: 10.1016/j.cell.2020.02.029
6. Moretta A, Marcenaro E, Sivori S, Della Chiesa M, Vitale M, Moretta L. Early liaisons between cells of the innate immune system in inflamed peripheral tissues. Trends Immunol (2005) 26(12):668–75. doi: 10.1016/j.it.2005.09.008
7. Bellora F, Castriconi R, Dondero A, Reggiardo G, Moretta L, Mantovani A, et al. The interaction of human natural killer cells with either unpolarized or polarized macrophages results in different functional outcomes. Proc Natl Acad Sci U S A. (2010) 107(50):21659–64. doi: 10.1073/pnas.1007654108
8. Scordamaglia F, Balsamo M, Scordamaglia A, Moretta A, Mingari MC, Canonica GW, et al. Perturbations of natural killer cell regulatory functions in respiratory allergic diseases. J Allergy Clin Immunol (2008) 121(2):479–85. doi: 10.1016/j.jaci.2007.09.047
9. Fauriat C, Long EO, Ljunggren HG, Bryceson YT. Regulation of human NK-cell cytokine and chemokine production by target cell recognition. Blood (2010) 115(11):2167–76. doi: 10.1182/blood-2009-08-238469
10. Parodi M, Pedrazzi M, Cantoni C, Averna M, Patrone M, Cavaletto M, et al. Natural killer (NK)/melanoma cell interaction induces NK-mediated release of chemotactic high mobility group box-1 (HMGB1) capable of amplifying NK cell recruitment. Oncoimmunology (2015) 4(12):e1052353. doi: 10.1080/2162402X.2015.1052353
11. Mace EM, Orange JS. Multiple distinct NK-cell synapses. Blood (2011) 118(25):6475–6. doi: 10.1182/blood-2011-10-381392
12. Vitale M, Cantoni C, Della Chiesa M, Ferlazzo G, Carlomagno S, Pende D, et al. An historical overview: the discovery of how NK cells can kill enemies, recruit defense troops, and more. Front Immunol (2019) 10:1415. doi: 10.3389/fimmu.2019.01415
13. Pende D, Falco M, Vitale M, Cantoni C, Vitale C, Munari E, et al. Killer ig-like receptors (KIRs): their role in NK cell modulation and developments leading to their clinical exploitation. Front Immunol (2019) 10:1179. doi: 10.3389/fimmu.2019.01179
14. Schlums H, Cichocki F, Tesi B, Theorell J, Beziat V, Holmes TD, et al. Cytomegalovirus infection drives adaptive epigenetic diversification of NK cells with altered signaling and effector function. Immunity (2015) 42(3):443–56. doi: 10.1016/j.immuni.2015.02.008
15. Freud AG, Mundy-Bosse BL, Yu J, Caligiuri MA. The broad spectrum of human natural killer cell diversity. Immunity (2017) 47(5):820–33. doi: 10.1016/j.immuni.2017.10.008
16. Brownlie D, Scharenberg M, Mold JE, Hard J, Kekalainen E, Buggert M, et al. Expansions of adaptive-like NK cells with a tissue-resident phenotype in human lung and blood. Proc Natl Acad Sci U.S.A. (2021) 118(11). doi: 10.1073/pnas.2016580118
17. Kiessling R, Klein E, Pross H, Wigzell H. "Natural" killer cells in the mouse. II. cytotoxic cells with specificity for mouse moloney leukemia cells. characteristics of the killer cell. Eur J Immunol (1975) 5(2):117–21. doi: 10.1002/eji.1830050209
18. Karre K, Klein GO, Kiessling R, Klein G, Roder JC. In vitro NK-activity and in vivo resistance to leukemia: studies of beige, beige//nude and wild-type hosts on C57BL background. Int J Cancer. (1980) 26(6):789–97. doi: 10.1002/ijc.2910260613
19. Aboud M, Kingsmore S, Segal S. Role of natural killer cells in controlling local tumor formation and metastatic manifestation of different 3LL Lewis lung carcinoma cell clones. Nat Immun (1993) 12(1):17–24.
20. Bennett M. Biology and genetics of hybrid resistance. Adv Immunol (1987) 41:333–445. doi: 10.1016/S0065-2776(08)60034-6
21. Sun JC, Beilke JN, Lanier LL. Adaptive immune features of natural killer cells. Nature (2009) 457(7229):557–61. doi: 10.1038/nature07665
22. Sun JC. Re-educating natural killer cells. J Exp Med (2010) 207(10):2049–52. doi: 10.1084/jem.20101748
23. Kim S, Poursine-Laurent J, Truscott SM, Lybarger L, Song YJ, Yang L, et al. Licensing of natural killer cells by host major histocompatibility complex class I molecules. Nature (2005) 436(7051):709–13. doi: 10.1038/nature03847
24. Sojka DK, Tian Z, Yokoyama WM. Tissue-resident natural killer cells and their potential diversity. Semin Immunol (2014) 26(2):127–31. doi: 10.1016/j.smim.2014.01.010
25. Vitale M, Cantoni C, Pietra G, Mingari MC, Moretta L. Effect of tumor cells and tumor microenvironment on NK-cell function. Eur J Immunol (2014) 44(6):1582–92. doi: 10.1002/eji.201344272
26. Stojanovic A, Cerwenka A. Natural killer cells and solid tumors. J Innate Immun (2011) 3(4):355–64. doi: 10.1159/000325465
27. Hoglund P, Ljunggren HG, Ohlen C, Ahrlund-Richter L, Scangos G, Bieberich C, et al. Natural resistance against lymphoma grafts conveyed by h-2Dd transgene to C57BL mice. J Exp Med (1988) 168(4):1469–74. doi: 10.1084/jem.168.4.1469
28. Glas R, Sturmhofel K, Hammerling GJ, Karre K, Ljunggren HG. Restoration of a tumorigenic phenotype by beta 2-microglobulin transfection to EL-4 mutant cells. J Exp Med (1992) 175(3):843–6. doi: 10.1084/jem.175.3.843
29. Guerra N, Tan YX, Joncker NT, Choy A, Gallardo F, Xiong N, et al. NKG2D-deficient mice are defective in tumor surveillance in models of spontaneous malignancy. Immunity (2008) 28(4):571–80. doi: 10.1016/j.immuni.2008.02.016
30. Iguchi-Manaka A, Kai H, Yamashita Y, Shibata K, Tahara-Hanaoka S, Honda S, et al. Accelerated tumor growth in mice deficient in DNAM-1 receptor. J Exp Med (2008) 205(13):2959–64. doi: 10.1084/jem.20081611
31. Halfteck GG, Elboim M, Gur C, Achdout H, Ghadially H, Mandelboim O. Enhanced in vivo growth of lymphoma tumors in the absence of the NK-activating receptor NKp46/NCR1. J Immunol (2009) 182(4):2221–30. doi: 10.4049/jimmunol.0801878
32. Glasner A, Ghadially H, Gur C, Stanietsky N, Tsukerman P, Enk J, et al. Recognition and prevention of tumor metastasis by the NK receptor NKp46/NCR1. J Immunol (2012) 188(6):2509–15. doi: 10.4049/jimmunol.1102461
33. Rangarajan A, Weinberg RA. Opinion: comparative biology of mouse versus human cells: modelling human cancer in mice. Nat Rev Cancer. (2003) 3(12):952–9. doi: 10.1038/nrc1235
34. Flanagan SP. 'Nude', a new hairless gene with pleiotropic effects in the mouse. Genet Res (1966) 8(3):295–309. doi: 10.1017/s0016672300010168
35. Bosma GC, Custer RP, Bosma MJ. A severe combined immunodeficiency mutation in the mouse. Nature (1983) 301(5900):527–30. doi: 10.1038/301527a0
36. Bosma MJ, Carroll AM. The SCID mouse mutant: definition, characterization, and potential uses. Annu Rev Immunol (1991) 9:323–50. doi: 10.1146/annurev.iy.09.040191.001543
37. Leiter EH. The NOD mouse: a model for analyzing the interplay between heredity and environment in development of autoimmune disease. ILAR J (1993) 35(1):4–14. doi: 10.1093/ilar.35.1.4
38. Makino S, Kunimoto K, Muraoka Y, Mizushima Y, Katagiri K, Tochino Y. Breeding of a non-obese, diabetic strain of mice. Jikken Dobutsu. (1980) 29(1):1–13. doi: 10.1093/ilar.35.1.4
39. Shultz LD, Schweitzer PA, Christianson SW, Gott B, Schweitzer IB, Tennent B, et al. Multiple defects in innate and adaptive immunologic function in NOD/LtSz-scid mice. J Immunol (1995) 154(1):180–91. doi: 10.4049/jimmunol.154.1.180
40. Hesselton RM, Greiner DL, Mordes JP, Rajan TV, Sullivan JL, Shultz LD. High levels of human peripheral blood mononuclear cell engraftment and enhanced susceptibility to human immunodeficiency virus type 1 infection in NOD/LtSz-scid/scid mice. J Infect Dis (1995) 172(4):974–82. doi: 10.1093/infdis/172.4.974
41. Hudson WA, Li Q, Le C, Kersey JH. Xenotransplantation of human lymphoid malignancies is optimized in mice with multiple immunologic defects. Leukemia (1998) 12(12):2029–33. doi: 10.1038/sj.leu.2401236
42. Ito M, Hiramatsu H, Kobayashi K, Suzue K, Kawahata M, Hioki K, et al. NOD/SCID/gamma(c)(null) mouse: an excellent recipient mouse model for engraftment of human cells. Blood (2002) 100(9):3175–82. doi: 10.1182/blood-2001-12-0207
43. Ishikawa F, Yasukawa M, Lyons B, Yoshida S, Miyamoto T, Yoshimoto G, et al. Development of functional human blood and immune systems in NOD/SCID/IL2 receptor gamma chain(null) mice. Blood (2005) 106(5):1565–73. doi: 10.1182/blood-2005-02-0516
44. Shultz LD, Lyons BL, Burzenski LM, Gott B, Chen X, Chaleff S, et al. Human lymphoid and myeloid cell development in NOD/LtSz-scid IL2R gamma null mice engrafted with mobilized human hemopoietic stem cells. J Immunol (2005) 174(10):6477–89. doi: 10.4049/jimmunol.174.10.6477
45. Fidler IJ, Kripke ML. Metastasis results from preexisting variant cells within a malignant tumor. Science (1977) 197(4306):893–5. doi: 10.1126/science.887927
46. Saha S, Pradhan N BN, Mahadevappa R, Minocha S, Kumar S. Cancer plasticity: investigating the causes for this agility. Semin Cancer Biol (2023) 88:138–56. doi: 10.1016/j.semcancer.2022.12.005
47. Siolas D, Hannon GJ. Patient-derived tumor xenografts: transforming clinical samples into mouse models. Cancer Res (2013) 73(17):5315–9. doi: 10.1158/0008-5472.CAN-13-1069
48. Rygaard J, Povlsen CO. Heterotransplantation of a human malignant tumour to "Nude" mice. Acta Pathol Microbiol Scand (1969) 77(4):758–60. doi: 10.1111/j.1699-0463.1969.tb04520.x
49. Gao H, Korn JM, Ferretti S, Monahan JE, Wang Y, Singh M, et al. High-throughput screening using patient-derived tumor xenografts to predict clinical trial drug response. Nat Med (2015) 21(11):1318–25. doi: 10.1038/nm.3954
50. Le DT, Huynh TR, Burt B, Van Buren G, Abeynaike SA, Zalfa C, et al. Natural killer cells and cytotoxic T lymphocytes are required to clear solid tumor in a patient-derived xenograft. JCI Insight (2021) 6(13). doi: 10.1172/jci.insight.140116
51. Kuperwasser C, Dessain S, Bierbaum BE, Garnet D, Sperandio K, Gauvin GP, et al. A mouse model of human breast cancer metastasis to human bone. Cancer Res (2005) 65(14):6130–8. doi: 10.1158/0008-5472.CAN-04-1408
52. Bertolini G, D'Amico L, Moro M, Landoni E, Perego P, Miceli R, et al. Microenvironment-modulated metastatic CD133+/CXCR4+/EpCAM- lung cancer-initiating cells sustain tumor dissemination and correlate with poor prognosis. Cancer Res (2015) 75(17):3636–49. doi: 10.1158/0008-5472.CAN-14-3781
53. Mosier DE, Gulizia RJ, Baird SM, Wilson DB. Transfer of a functional human immune system to mice with severe combined immunodeficiency. Nature (1988) 335(6187):256–9. doi: 10.1038/335256a0
54. King MA, Covassin L, Brehm MA, Racki W, Pearson T, Leif J, et al. Human peripheral blood leucocyte non-obese diabetic-severe combined immunodeficiency interleukin-2 receptor gamma chain gene mouse model of xenogeneic graft-versus-host-like disease and the role of host major histocompatibility complex. Clin Exp Immunol (2009) 157(1):104–18. doi: 10.1111/j.1365-2249.2009.03933.x
55. Yue X, Petersen F, Shu Y, Kasper B, Magatsin JDT, Ahmadi M, et al. Transfer of PBMC from SSc patients induces autoantibodies and systemic inflammation in Rag2-/-/IL2rg-/- mice. Front Immunol (2021) 12:677970. doi: 10.3389/fimmu.2021.677970
56. Brehm MA, Kenney LL, Wiles MV, Low BE, Tisch RM, Burzenski L, et al. Lack of acute xenogeneic graft- versus-host disease, but retention of T-cell function following engraftment of human peripheral blood mononuclear cells in NSG mice deficient in MHC class I and II expression. FASEB J (2019) 33(3):3137–51. doi: 10.1096/fj.201800636R
57. Traggiai E, Chicha L, Mazzucchelli L, Bronz L, Piffaretti JC, Lanzavecchia A, et al. Development of a human adaptive immune system in cord blood cell-transplanted mice. Science (2004) 304(5667):104–7. doi: 10.1126/science.1093933
58. Goldman JP, Blundell MP, Lopes L, Kinnon C, Di Santo JP, Thrasher AJ. Enhanced human cell engraftment in mice deficient in RAG2 and the common cytokine receptor gamma chain. Br J Haematol (1998) 103(2):335–42. doi: 10.1046/j.1365-2141.1998.00980.x
59. Melkus MW, Estes JD, Padgett-Thomas A, Gatlin J, Denton PW, Othieno FA, et al. Humanized mice mount specific adaptive and innate immune responses to EBV and TSST-1. Nat Med (2006) 12(11):1316–22. doi: 10.1038/nm1431
60. Brainard DM, Seung E, Frahm N, Cariappa A, Bailey CC, Hart WK, et al. Induction of robust cellular and humoral virus-specific adaptive immune responses in human immunodeficiency virus-infected humanized BLT mice. J Virol (2009) 83(14):7305–21. doi: 10.1128/JVI.02207-08
61. Vatakis DN, Bristol GC, Kim SG, Levin B, Liu W, Radu CG, et al. Using the BLT humanized mouse as a stem cell based gene therapy tumor model. J Vis Exp (2012) 70):e4181. doi: 10.3791/4181
62. Chuprin J, Buettner H, Seedhom MO, Greiner DL, Keck JG, Ishikawa F, et al. Humanized mouse models for immuno-oncology research. Nat Rev Clin Oncol (2023) 20(3):192–206. doi: 10.1038/s41571-022-00721-2
63. Katano I, Takahashi T, Ito R, Kamisako T, Mizusawa T, Ka Y, et al. Predominant development of mature and functional human NK cells in a novel human IL-2-producing transgenic NOG mouse. J Immunol (2015) 194(7):3513–25. doi: 10.4049/jimmunol.1401323
64. Katano I, Nishime C, Ito R, Kamisako T, Mizusawa T, Ka Y, et al. Long-term maintenance of peripheral blood derived human NK cells in a novel human IL-15- transgenic NOG mouse. Sci Rep (2017) 7(1):17230. doi: 10.1038/s41598-017-17442-7
65. Aryee KE, Burzenski LM, Yao LC, Keck JG, Greiner DL, Shultz LD, et al. Enhanced development of functional human NK cells in NOD-scid-IL2rg(null) mice expressing human IL15. FASEB J (2022) 36(9):e22476. doi: 10.1096/fj.202200045R
66. Matsuda M, Ono R, Iyoda T, Endo T, Iwasaki M, Tomizawa-Murasawa M, et al. Human NK cell development in hIL-7 and hIL-15 knockin NOD/SCID/IL2rgKO mice. Life Sci Alliance (2019) 2(2). doi: 10.26508/lsa.201800195
67. Sippel TR, Radtke S, Olsen TM, Kiem HP, Rongvaux A. Human hematopoietic stem cell maintenance and myeloid cell development in next-generation humanized mouse models. Blood Adv (2019) 3(3):268–74. doi: 10.1182/bloodadvances.2018023887
68. Wunderlich M, Chou FS, Sexton C, Presicce P, Chougnet CA, Aliberti J, et al. Improved multilineage human hematopoietic reconstitution and function in NSGS mice. PloS One (2018) 13(12):e0209034. doi: 10.1371/journal.pone.0209034
69. Herndler-Brandstetter D, Shan L, Yao Y, Stecher C, Plajer V, Lietzenmayer M, et al. Humanized mouse model supports development, function, and tissue residency of human natural killer cells. Proc Natl Acad Sci U S A. (2017) 114(45):E9626–E34. doi: 10.1073/pnas.1705301114
70. Rongvaux A, Willinger T, Martinek J, Strowig T, Gearty SV, Teichmann LL, et al. Development and function of human innate immune cells in a humanized mouse model. Nat Biotechnol (2014) 32(4):364–72. doi: 10.1038/nbt.2858
71. Lopez-Lastra S, Masse-Ranson G, Fiquet O, Darche S, Serafini N, Li Y, et al. A functional DC cross talk promotes human ILC homeostasis in humanized mice. Blood Adv (2017) 1(10):601–14. doi: 10.1182/bloodadvances.2017004358
72. Shan L, Flavell RA, Herndler-Brandstetter D. Development of humanized mouse models for studying human NK cells in health and disease. Methods Mol Biol (2022) 2463:53–66. doi: 10.1007/978-1-0716-2160-8_5
73. McIntosh BE, Brown ME, Duffin BM, Maufort JP, Vereide DT, Slukvin II, et al. Nonirradiated NOD,B6.SCID Il2rgamma-/- Kit(W41/W41) (NBSGW) mice support multilineage engraftment of human hematopoietic cells. Stem Cell Rep (2015) 4(2):171–80. doi: 10.1016/j.stemcr.2014.12.005
74. Hess NJ, Lindner PN, Vazquez J, Grindel S, Hudson AW, Stanic AK, et al. Different human immune lineage compositions are generated in non-conditioned NBSGW mice depending on HSPC source. Front Immunol (2020) 11:573406. doi: 10.3389/fimmu.2020.573406
75. Steinkamp MP, Lagutina I, Brayer KJ, Schultz F, Burke D, Pankratz VS, et al. Humanized patient-derived xenograft models of disseminated ovarian cancer recapitulate key aspects of the tumor immune environment within the peritoneal cavity. Cancer Res Commun (2023) 3(2):309–24. doi: 10.1158/2767-9764.CRC-22-0300
76. Ny L, Rizzo LY, Belgrano V, Karlsson J, Jespersen H, Carstam L, et al. Supporting clinical decision making in advanced melanoma by preclinical testing in personalized immune-humanized xenograft mouse models. Ann Oncol (2020) 31(2):266–73. doi: 10.1016/j.annonc.2019.11.002
77. Forsberg EMV, Lindberg MF, Jespersen H, Alsen S, Bagge RO, Donia M, et al. HER2 CAR-T cells eradicate uveal melanoma and T-cell therapy-resistant human melanoma in IL2 transgenic NOD/SCID IL2 receptor knockout mice. Cancer Res (2019) 79(5):899–904. doi: 10.1158/0008-5472.CAN-18-3158
78. Jespersen H, Lindberg MF, Donia M, Soderberg EMV, Andersen R, Keller U, et al. Clinical responses to adoptive T-cell transfer can be modeled in an autologous immune-humanized mouse model. Nat Commun (2017) 8(1):707. doi: 10.1038/s41467-017-00786-z
79. Rademacher MJ, Cruz A, Faber M, Oldham RAA, Wang D, Medin JA, et al. Sarcoma IL-12 overexpression facilitates NK cell immunomodulation. Sci Rep (2021) 11(1):8321. doi: 10.1038/s41598-021-87700-2
80. Flieswasser T, Van den Eynde A, Freire Boullosa L, Melis J, Hermans C, Merlin C, et al. Targeting CD70 in combination with chemotherapy to enhance the anti-tumor immune effects in non-small cell lung cancer. Oncoimmunology (2023) 12(1):2192100. doi: 10.1080/2162402X.2023.2192100
81. Zenga J, Awan MJ, Frei A, Petrie E, Sharma GP, Shreenivas A, et al. Chronic stress promotes an immunologic inflammatory state and head and neck cancer growth in a humanized murine model. Head Neck. (2022) 44(6):1324–34. doi: 10.1002/hed.27028
82. Wang Y, Buck A, Grimaud M, Culhane AC, Kodangattil S, Razimbaud C, et al. Anti-CAIX BBzeta CAR4/8 T cells exhibit superior efficacy in a ccRCC mouse model. Mol Ther Oncolytics. (2022) 24:385–99. doi: 10.1016/j.omto.2021.12.019
83. Scherer SD, Riggio AI, Haroun F, DeRose YS, Ekiz HA, Fujita M, et al. An immune-humanized patient-derived xenograft model of estrogen-independent, hormone receptor positive metastatic breast cancer. Breast Cancer Res (2021) 23(1):100. doi: 10.1186/s13058-021-01476-x
84. Yu CI, Martinek J, Wu TC, Kim KI, George J, Ahmadzadeh E, et al. Human KIT+ myeloid cells facilitate visceral metastasis by melanoma. J Exp Med (2021) 218(6). doi: 10.1084/jem.20182163
85. Lee YS, O'Brien LJ, Walpole CM, Pearson FE, Leal-Rojas IM, Masterman KA, et al. Human CD141(+) dendritic cells (cDC1) are impaired in patients with advanced melanoma but can be targeted to enhance anti-PD-1 in a humanized mouse model. J Immunother Cancer (2021) 9(3). doi: 10.1136/jitc-2020-001963
86. Garcia-Moure M, Gonzalez-Huarriz M, Labiano S, Guruceaga E, Bandres E, Zalacain M, et al. Delta-24-RGD, an oncolytic adenovirus, increases survival and promotes proinflammatory immune landscape remodeling in models of AT/RT and CNS-PNET. Clin Cancer Res (2021) 27(6):1807–20. doi: 10.1158/1078-0432.CCR-20-3313
87. Zhai L, Ladomersky E, Lauing KL, Wu M, Genet M, Gritsina G, et al. Infiltrating T cells increase IDO1 expression in glioblastoma and contribute to decreased patient survival. Clin Cancer Res (2017) 23(21):6650–60. doi: 10.1158/1078-0432.CCR-17-0120
88. Klichinsky M, Ruella M, Shestova O, Lu XM, Best A, Zeeman M, et al. Human chimeric antigen receptor macrophages for cancer immunotherapy. Nat Biotechnol (2020) 38(8):947–53. doi: 10.1038/s41587-020-0462-y
89. Odunsi A, McGray AJR, Miliotto A, Zhang Y, Wang J, Abiola A, et al. Fidelity of human ovarian cancer patient-derived xenografts in a partially humanized mouse model for preclinical testing of immunotherapies. J Immunother Cancer (2020) 8(2). doi: 10.1136/jitc-2020-001237
90. Chiossone L, Dumas PY, Vienne M, Vivier E. Natural killer cells and other innate lymphoid cells in cancer. Nat Rev Immunol (2018) 18(11):671–88. doi: 10.1038/s41577-018-0061-z
91. Nayyar G, Chu Y, Cairo MS. Overcoming resistance to natural killer cell based immunotherapies for solid tumors. Front Oncol (2019) 9:51. doi: 10.3389/fonc.2019.00051
92. Miller JS, Soignier Y, Panoskaltsis-Mortari A, McNearney SA, Yun GH, Fautsch SK, et al. Successful adoptive transfer and in vivo expansion of human haploidentical NK cells in patients with cancer. Blood (2005) 105(8):3051–7. doi: 10.1182/blood-2004-07-2974
93. Curti A, Ruggeri L, D'Addio A, Bontadini A, Dan E, Motta MR, et al. Successful transfer of alloreactive haploidentical KIR ligand-mismatched natural killer cells after infusion in elderly high risk acute myeloid leukemia patients. Blood (2011) 118(12):3273–9. doi: 10.1182/blood-2011-01-329508
94. Chen M, Li Y, Wu Y, Xie S, Ma J, Yue J, et al. Anti-tumor activity of expanded PBMC-derived NK cells by feeder-free protocol in ovarian cancer. Cancers (Basel) (2021) 13(22). doi: 10.3390/cancers13225866
95. Veneziani I, Infante P, Ferretti E, Melaiu O, Battistelli C, Lucarini V, et al. Nutlin-3a enhances natural killer cell-mediated killing of neuroblastoma by restoring p53-dependent expression of ligands for NKG2D and DNAM-1 receptors. Cancer Immunol Res (2021) 9(2):170–83. doi: 10.1158/2326-6066.CIR-20-0313
96. Mitra S, Leonard WJ. Biology of IL-2 and its therapeutic modulation: mechanisms and strategies. J Leukoc Biol (2018) 103(4):643–55. doi: 10.1002/JLB.2RI0717-278R
97. Ahmadzadeh M, Rosenberg SA. IL-2 administration increases CD4+ CD25(hi) Foxp3+ regulatory T cells in cancer patients. Blood (2006) 107(6):2409–14. doi: 10.1182/blood-2005-06-2399
98. Wei S, Kryczek I, Edwards RP, Zou L, Szeliga W, Banerjee M, et al. Interleukin-2 administration alters the CD4+FOXP3+ T-cell pool and tumor trafficking in patients with ovarian carcinoma. Cancer Res (2007) 67(15):7487–94. doi: 10.1158/0008-5472.CAN-07-0565
99. Rodella L, Zamai L, Rezzani R, Artico M, Peri G, Falconi M, et al. Interleukin 2 and interleukin 15 differentially predispose natural killer cells to apoptosis mediated by endothelial and tumour cells. Br J Haematol (2001) 115(2):442–50. doi: 10.1046/j.1365-2141.2001.03055.x
100. Mortara L, Balza E, Bruno A, Poggi A, Orecchia P, Carnemolla B. Anti-cancer therapies employing IL-2 cytokine tumor targeting: contribution of innate, adaptive and immunosuppressive cells in the anti-tumor efficacy. Front Immunol (2018) 9:2905. doi: 10.3389/fimmu.2018.02905
101. Levin AM, Bates DL, Ring AM, Krieg C, Lin JT, Su L, et al. Exploiting a natural conformational switch to engineer an interleukin-2 'superkine'. Nature (2012) 484(7395):529–33. doi: 10.1038/nature10975
102. Merchant R, Galligan C, Munegowda MA, Pearce LB, Lloyd P, Smith P, et al. Fine-tuned long-acting interleukin-2 superkine potentiates durable immune responses in mice and non-human primate. J Immunother Cancer (2022) 10(1). doi: 10.1136/jitc-2021-003155
103. Wolf NK, Blaj C, Picton LK, Snyder G, Zhang L, Nicolai CJ, et al. Synergy of a STING agonist and an IL-2 superkine in cancer immunotherapy against MHC I-deficient and MHC i(+) tumors. Proc Natl Acad Sci U S A. (2022) 119(22):e2200568119. doi: 10.1073/pnas.2200568119
104. Wendt K, Wilk E, Buyny S, Schmidt RE, Jacobs R. Interleukin-21 differentially affects human natural killer cell subsets. Immunology (2007) 122(4):486–95. doi: 10.1111/j.1365-2567.2007.02675.x
105. Kasaian MT, Whitters MJ, Carter LL, Lowe LD, Jussif JM, Deng B, et al. IL-21 limits NK cell responses and promotes antigen-specific T cell activation: a mediator of the transition from innate to adaptive immunity. Immunity (2002) 16(4):559–69. doi: 10.1016/S1074-7613(02)00295-9
106. Brady J, Hayakawa Y, Smyth MJ, Nutt SL. IL-21 induces the functional maturation of murine NK cells. J Immunol (2004) 172(4):2048–58. doi: 10.4049/jimmunol.172.4.2048
107. Granzin M, Stojanovic A, Miller M, Childs R, Huppert V, Cerwenka A. Highly efficient IL-21 and feeder cell-driven ex vivo expansion of human NK cells with therapeutic activity in a xenograft mouse model of melanoma. Oncoimmunology (2016) 5(9):e1219007. doi: 10.1080/2162402X.2016.1219007
108. Zhou Y, Husman T, Cen X, Tsao T, Brown J, Bajpai A, et al. Interleukin 15 in cell-based cancer immunotherapy. Int J Mol Sci (2022) 23(13). doi: 10.3390/ijms23137311
109. Anton OM, Peterson ME, Hollander MJ, Dorward DW, Arora G, Traba J, et al. Trans-endocytosis of intact IL-15Ralpha-IL-15 complex from presenting cells into NK cells favors signaling for proliferation. Proc Natl Acad Sci U S A. (2020) 117(1):522–31. doi: 10.1073/pnas.1911678117
110. Ferlazzo G, Pack M, Thomas D, Paludan C, Schmid D, Strowig T, et al. Distinct roles of IL-12 and IL-15 in human natural killer cell activation by dendritic cells from secondary lymphoid organs. Proc Natl Acad Sci U S A. (2004) 101(47):16606–11. doi: 10.1073/pnas.0407522101
111. Hoogstad-van Evert JS, Cany J, van den Brand D, Oudenampsen M, Brock R, Torensma R, et al. Umbilical cord blood CD34(+) progenitor-derived NK cells efficiently kill ovarian cancer spheroids and intraperitoneal tumors in NOD/SCID/IL2Rg(null) mice. Oncoimmunology (2017) 6(8):e1320630. doi: 10.1080/2162402X.2017.1320630
112. Lanuza PM, Alonso MH, Hidalgo S, Uranga-Murillo I, Garcia-Mulero S, Arnau R, et al. Adoptive NK cell transfer as a treatment in colorectal cancer patients: analyses of tumour cell determinants correlating with efficacy In vitro and in vivo. Front Immunol (2022) 13:890836. doi: 10.3389/fimmu.2022.890836
113. Han KP, Zhu X, Liu B, Jeng E, Kong L, Yovandich JL, et al. IL-15:IL-15 receptor alpha superagonist complex: high-level co-expression in recombinant mammalian cells, purification and characterization. Cytokine (2011) 56(3):804–10. doi: 10.1016/j.cyto.2011.09.028
114. Van der Meer JMR, Maas RJA, Guldevall K, Klarenaar K, de Jonge P, Evert JSH, et al. IL-15 superagonist n-803 improves IFNgamma production and killing of leukemia and ovarian cancer cells by CD34(+) progenitor-derived NK cells. Cancer Immunol Immunother. (2021) 70(5):1305–21. doi: 10.1007/s00262-020-02749-8
115. Felices M, Chu S, Kodal B, Bendzick L, Ryan C, Lenvik AJ, et al. IL-15 super-agonist (ALT-803) enhances natural killer (NK) cell function against ovarian cancer. Gynecol Oncol (2017) 145(3):453–61. doi: 10.1016/j.ygyno.2017.02.028
116. Romee R, Cooley S, Berrien-Elliott MM, Westervelt P, Verneris MR, Wagner JE, et al. First-in-human phase 1 clinical study of the IL-15 superagonist complex ALT-803 to treat relapse after transplantation. Blood (2018) 131(23):2515–27. doi: 10.1182/blood-2017-12-823757
117. Margolin K, Morishima C, Velcheti V, Miller JS, Lee SM, Silk AW, et al. Phase I trial of ALT-803, a novel recombinant IL15 complex, in patients with advanced solid tumors. Clin Cancer Res (2018) 24(22):5552–61. doi: 10.1158/1078-0432.CCR-18-0945
118. Romee R, Rosario M, Berrien-Elliott MM, Wagner JA, Jewell BA, Schappe T, et al. Cytokine-induced memory-like natural killer cells exhibit enhanced responses against myeloid leukemia. Sci Transl Med (2016) 8(357):357ra123. doi: 10.1126/scitranslmed.aaf2341
119. Ni J, Holsken O, Miller M, Hammer Q, Luetke-Eversloh M, Romagnani C, et al. Adoptively transferred natural killer cells maintain long-term antitumor activity by epigenetic imprinting and CD4(+) T cell help. Oncoimmunology (2016) 5(9):e1219009. doi: 10.1080/2162402X.2016.1219009
120. Berrien-Elliott MM, Cashen AF, Cubitt CC, Neal CC, Wong P, Wagner JA, et al. Multidimensional analyses of donor memory-like NK cells reveal new associations with response after adoptive immunotherapy for leukemia. Cancer Discovery (2020) 10(12):1854–71. doi: 10.1158/2159-8290.CD-20-0312
121. Uppendahl LD, Felices M, Bendzick L, Ryan C, Kodal B, Hinderlie P, et al. Cytokine-induced memory-like natural killer cells have enhanced function, proliferation, and in vivo expansion against ovarian cancer cells. Gynecol Oncol (2019) 153(1):149–57. doi: 10.1016/j.ygyno.2019.01.006
122. Marin ND, Krasnick BA, Becker-Hapak M, Conant L, Goedegebuure SP, Berrien-Elliott MM, et al. Memory-like differentiation enhances NK cell responses to melanoma. Clin Cancer Res (2021) 27(17):4859–69. doi: 10.1158/1078-0432.CCR-21-0851
123. Sangiolo D, Mesiano G, Gammaitoni L, Leuci V, Todorovic M, Giraudo L, et al. Cytokine-induced killer cells eradicate bone and soft-tissue sarcomas. Cancer Res (2014) 74(1):119–29. doi: 10.1158/0008-5472.CAN-13-1559
124. Gammaitoni L, Giraudo L, Leuci V, Todorovic M, Mesiano G, Picciotto F, et al. Effective activity of cytokine-induced killer cells against autologous metastatic melanoma including cells with stemness features. Clin Cancer Res (2013) 19(16):4347–58. doi: 10.1158/1078-0432.CCR-13-0061
125. Wendel P, Reindl LM, Bexte T, Kunnemeyer L, Sarchen V, Albinger N, et al. Arming immune cells for battle: a brief journey through the advancements of T and NK cell immunotherapy. Cancers (Basel) (2021) 13(6). doi: 10.3390/cancers13061481
126. Vaseq R, Sharma A, Li Y, Schmidt-Wolf IGH. Revising the landscape of cytokine-induced killer cell therapy in lung cancer: focus on immune checkpoint inhibitors. Int J Mol Sci (2023) 24(6). doi: 10.3390/ijms24065626
127. Introna M, Correnti F. Innovative clinical perspectives for CIK cells in cancer patients. Int J Mol Sci (2018) 19(2). doi: 10.3390/ijms19020358
128. Mhaidly R, Verhoeyen E. Humanized mice are precious tools for preclinical evaluation of CAR T and CAR NK cell therapies. Cancers (Basel). (2020) 12(7). doi: 10.3390/cancers12071915
129. Caruso S, De Angelis B, Carlomagno S, Del Bufalo F, Sivori S, Locatelli F, et al. NK cells as adoptive cellular therapy for hematological malignancies: advantages and hurdles. Semin Hematol (2020) 57(4):175–84. doi: 10.1053/j.seminhematol.2020.10.004
130. Caruso S, De Angelis B, Del Bufalo F, Ciccone R, Donsante S, Volpe G, et al. Safe and effective off-the-shelf immunotherapy based on CAR.CD123-NK cells for the treatment of acute myeloid leukaemia. J Hematol Oncol (2022) 15(1):163. doi: 10.1186/s13045-022-01376-3
131. Carosella ED, Rouas-Freiss N, Tronik-Le Roux D, Moreau P, LeMaoult J. HLA-G: an immune checkpoint molecule. Adv Immunol (2015) 127:33–144. doi: 10.1016/bs.ai.2015.04.001
132. Jan CI, Huang SW, Canoll P, Bruce JN, Lin YC, Pan CM, et al. Targeting human leukocyte antigen G with chimeric antigen receptors of natural killer cells convert immunosuppression to ablate solid tumors. J Immunother Cancer (2021) 9(10). doi: 10.1136/jitc-2021-003050
133. Lian G, Mak TS, Yu X, Lan HY. Challenges and recent advances in NK cell-targeted immunotherapies in solid tumors. Int J Mol Sci (2021) 23(1). doi: 10.3390/ijms23010164
134. Myers JA, Miller JS. Exploring the NK cell platform for cancer immunotherapy. Nat Rev Clin Oncol (2021) 18(2):85–100. doi: 10.1038/s41571-020-0426-7
135. Vallera DA, Oh F, Kodal B, Hinderlie P, Geller MA, Miller JS, et al. A HER2 tri-specific NK cell engager mediates efficient targeting of human ovarian cancer. Cancers (Basel) (2021) 13(16). doi: 10.3390/cancers13163994
136. Vallera DA, Ferrone S, Kodal B, Hinderlie P, Bendzick L, Ettestad B, et al. NK-Cell-Mediated targeting of various solid tumors using a B7-H3 tri-specific killer engager in vitro and In vivo. Cancers (Basel) (2020) 12(9). doi: 10.3390/cancers12092659
137. Kaminski MF, Bendzick L, Hopps R, Kauffman M, Kodal B, Soignier Y, et al. TEM8 tri-specific killer engager binds both tumor and tumor stroma to specifically engage natural killer cell anti-tumor activity. J Immunother Cancer (2022) 10(9). doi: 10.1136/jitc-2022-004725
138. Kennedy PR, Vallera DA, Ettestad B, Hallstrom C, Kodal B, Todhunter DA, et al. A tri-specific killer engager against mesothelin targets NK cells towards lung cancer. Front Immunol (2023) 14:1060905. doi: 10.3389/fimmu.2023.1060905
139. Kerbauy LN, Marin ND, Kaplan M, Banerjee PP, Berrien-Elliott MM, Becker-Hapak M, et al. Combining AFM13, a bispecific CD30/CD16 antibody, with cytokine-activated blood and cord blood-derived NK cells facilitates CAR-like responses against CD30(+) malignancies. Clin Cancer Res (2021) 27(13):3744–56. doi: 10.1158/1078-0432.CCR-21-0164
140. Gauthier L, Morel A, Anceriz N, Rossi B, Blanchard-Alvarez A, Grondin G, et al. Multifunctional natural killer cell engagers targeting NKp46 trigger protective tumor immunity. Cell (2019) 177(7):1701–13 e16. doi: 10.1016/j.cell.2019.04.041
141. Demaria O, Gauthier L, Vetizou M, Blanchard Alvarez A, Vagne C, Habif G, et al. Antitumor immunity induced by antibody-based natural killer cell engager therapeutics armed with not-alpha IL-2 variant. Cell Rep Med (2022) 3(10):100783. doi: 10.1016/j.xcrm.2022.100783
142. Guo M, Sun C, Qian Y, Zhu L, Ta N, Wang G, et al. Proliferation of highly cytotoxic human natural killer cells by OX40L armed NK-92 with secretory neoleukin-2/15 for cancer immunotherapy. Front Oncol (2021) 11:632540. doi: 10.3389/fonc.2021.632540
143. Choi SH, Kim HJ, Park JD, Ko ES, Lee M, Lee DK, et al. Chemical priming of natural killer cells with branched polyethylenimine for cancer immunotherapy. J Immunother Cancer (2022) 10(8). doi: 10.1136/jitc-2022-004964
144. Zingoni A, Ardolino M, Santoni A, Cerboni C. NKG2D and DNAM-1 activating receptors and their ligands in NK-T cell interactions: role in the NK cell-mediated negative regulation of T cell responses. Front Immunol (2012) 3:408. doi: 10.3389/fimmu.2012.00408
145. Van der Meer JMR, de Jonge P, van der Waart AB, Geerlings AC, Moonen JP, Brummelman J, et al. CD34(+) progenitor-derived NK cell and gemcitabine combination therapy increases killing of ovarian cancer cells in NOD/SCID/IL2Rg(null) mice. Oncoimmunology (2021) 10(1):1981049. doi: 10.1080/2162402X.2021.1981049
146. Walle T, Kraske JA, Liao B, Lenoir B, Timke C, von Bohlen Und Halbach E, et al. Radiotherapy orchestrates natural killer cell dependent antitumor immune responses through CXCL8. Sci Adv (2022) 8(12):eabh4050. doi: 10.1126/sciadv.abh4050
147. Huh D, Matthews BD, Mammoto A, Montoya-Zavala M, Hsin HY, Ingber DE. Reconstituting organ-level lung functions on a chip. Science (2010) 328(5986):1662–8. doi: 10.1126/science.1188302
148. Lv D, Hu Z, Lu L, Lu H, Xu X. Three-dimensional cell culture: a powerful tool in tumor research and drug discovery. Oncol Lett (2017) 14(6):6999–7010. doi: 10.3892/ol.2017.7134
149. Diaz L, Zambrano E, Flores ME, Contreras M, Crispin JC, Aleman G, et al. Ethical considerations in animal research: the principle of 3R's. Rev Invest Clin (2020) 73(4):199–209. doi: 10.24875/RIC.20000380
150. Bussard KM, Mutkus L, Stumpf K, Gomez-Manzano C, Marini FC. Tumor-associated stromal cells as key contributors to the tumor microenvironment. Breast Cancer Res (2016) 18(1):84. doi: 10.1186/s13058-016-0740-2
Keywords: natural killer cells, humanized mice, solid tumor, human-in-mouse model, tumor immunity
Citation: Parodi M, Astigiano S, Carrega P, Pietra G, Vitale C, Damele L, Grottoli M, Guevara Lopez MdlL, Ferracini R, Bertolini G, Roato I, Vitale M and Orecchia P (2023) Murine models to study human NK cells in human solid tumors. Front. Immunol. 14:1209237. doi: 10.3389/fimmu.2023.1209237
Received: 20 April 2023; Accepted: 02 June 2023;
Published: 14 June 2023.
Edited by:
Ombretta Melaiu, University of Rome Tor Vergata, ItalyReviewed by:
Valeria Leuci, University of Turin, ItalyValeria Lucarini, Sapienza University of Rome, Italy
Jaya Lakshmi Thangaraj, University of California, San Diego, United States
Adeleh Taghi Khani, Beckman Research Institute, United States
Copyright © 2023 Parodi, Astigiano, Carrega, Pietra, Vitale, Damele, Grottoli, Guevara Lopez, Ferracini, Bertolini, Roato, Vitale and Orecchia. This is an open-access article distributed under the terms of the Creative Commons Attribution License (CC BY). The use, distribution or reproduction in other forums is permitted, provided the original author(s) and the copyright owner(s) are credited and that the original publication in this journal is cited, in accordance with accepted academic practice. No use, distribution or reproduction is permitted which does not comply with these terms.
*Correspondence: Massimo Vitale, bWFzc2ltby52aXRhbGVAaHNhbm1hcnRpbm8uaXQ=; Paola Orecchia, cGFvbGEub3JlY2NoaWFAaHNhbm1hcnRpbm8uaXQ=
†These authors have contributed equally to this work
‡These authors share senior authorship