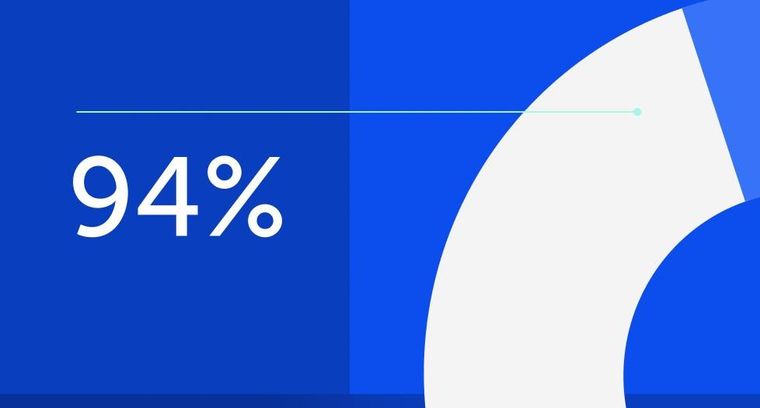
94% of researchers rate our articles as excellent or good
Learn more about the work of our research integrity team to safeguard the quality of each article we publish.
Find out more
MINI REVIEW article
Front. Immunol., 31 October 2023
Sec. Systems Immunology
Volume 14 - 2023 | https://doi.org/10.3389/fimmu.2023.1207746
This article is part of the Research TopicImmunology of CachexiaView all 11 articles
The development of cachexia in the setting of cancer or other chronic diseases is a significant detriment for patients. Cachexia is associated with a decreased ability to tolerate therapies, reduction in ambulation, reduced quality of life, and increased mortality. Cachexia appears intricately linked to the activation of the acute phase response and is a drain on metabolic resources. Work has begun to focus on the important inflammatory factors associated with the acute phase response and their role in the immune activation of cachexia. Furthermore, data supporting the liver, lung, skeletal muscle, and tumor as all playing a role in activation of the acute phase are emerging. Although the acute phase is increasingly being recognized as being involved in cachexia, work in understanding underlying mechanisms of cachexia associated with the acute phase response remains an active area of investigation and still lack a holistic understanding and a clear causal link. Studies to date are largely correlative in nature, nonetheless suggesting the possibility for a role for various acute phase reactants. Herein, we examine the current literature regarding the acute phase response proteins, the evidence these proteins play in the promotion and exacerbation of cachexia, and current evidence of a therapeutic potential for patients.
Cachexia is a devastating process that accompanies chronic inflammatory illnesses such as cancer, sepsis, chronic obstructive pulmonary disease (COPD), chronic heart failure (CHF), rheumatoid arthritis (RA), and chronic kidney disease (CKD.) Clinically, cachexia is diagnosed as a weight loss greater than 5%, weight loss greater than 2% in individuals with BMI < 20 kg/m2, or sarcopenia (1). Cachexia is classically described in cancer and is prevalent in up to 80% of cancer patients, however cachexia also exacerbates chronic illness such as COPD with prevalence as high as 15% of patients (2). Predictive scores have been developed which utilize serum inflammatory markers, nutritional parameters, weight loss, and muscular evaluation to identify those with cachexia and those who are at risk (3–5).
Cachexia is driven by an inflammatory response involving cross-talk between many organs throughout the body. The acute phase response (APR) involves changes in plasma protein concentrations as well as behavioral, psychological, biochemical, and nutritional changes in the organism. A plasma protein whose concentration increases or decreases by at least 25% in response to inflammation is defined as an acute phase protein (6). These proteins are created in response to inflammation as a result of tissue insult and function to restore tissue homeostasis and repair, including regulating cell proliferation, scar formation, and immune defensive functions. Acute phase protein synthesis is initiated by cytokine signaling, mainly through interleukin-6 (IL-6), IL-1, TNF Alpha (TNFa), and Interferon Gamma (IFNg). Previous studies have extensively described the acute phase proteins (APP) produced by the liver. APP it is divided into positive acute phase proteins and negative acute phase proteins. Positive APPs levels increase during the APR whereas negative APPs levels decrease (6–8). Overall, APP concentrations are affected during cancer (Table 1) as well as many other inflammatory states. To date, less is known how these factors regulate metabolism, muscle and fat homeostasis.
Table 1 Acute phase reactant proteins produced by the liver, lung, skeletal muscle, and tumor microenvironment.
The APR has been shown to increase the patient’s resting energy expenditure, a feature of elevated catabolism (159). This supports the assertion APPs have a molecular role in the progression of cancer and chronic illness related cachexia. The liver is not the only organ capable of synthesizing proteins in the APR (160), and understanding the APR relationship to cachexia remains poorly defined. Therefore, we sought to review current understanding of how the APR promotes cachexia. Currently, we could identify no studies demonstrating the in vivo manipulation of APP activation and improvement or other effects on the cachectic response. In our review of the literature, we sought a comprehensive list of acute phase proteins. We used the well-established list of acute phase proteins described by Gabay and Kushner (6) as well as our expert judgement as the basis for our literature search and review. Through our expert consensus we have identified CD-14 and lipocalin as APP of interest and included these in our examination. Herein, we review our current understanding of the positive and negative acute phase response related proteins and their possible role in promoting cachexia. We specifically examine APP production and presence in extrahepatic tissues including lung, skeletal muscle, and tumor microenvironment as contributors towards cachexia.
Inflammation is responsible for protein production during the acute phase response which critically requires IL-6 to produce many APP (161).Cytokines are the primary signaling mechanism by which the up and down regulation of acute phase proteins occurs. Innate immune cells are activated through toll like receptors to produce IL-1 and TNF alpha. These cytokines lead to further release of IL-6 (162, 163). IL-6 stimulation of hepatocytes produces increased positive acute phase reactants such as CRP, SAA, Alpha 1 anti-chymotrypsin, fibrinogen and decreased negative acute phase reactants such as albumin, transferrin, and fibronectin (161). IL-6 also has been shown to directly cause cachexia through a cross-talk mechanism between tumor, muscle, and fat requiring IL-6 signaling (164). Inflammation is the dominant driver of cachexia as the body struggles to maintain the balance of host defense versus the negative impacts of immune defenses on the host itself (165, 166). Measurement of the inflammatory response may be done through surrogate acute phase proteins such as C Reactive Protein (CRP), which is considered the most widely accepted index of systemic inflammation (1). CRP has been predictive of degree of cachexia as well as survival in cancer patients (3, 167). CRP is one of many examples of acute phase reactant that are a byproduct of inflammation and have a relationship to cachexia. There are many examples of regulation of acute phase response signaling in cachexia (168). In the following sections we describe extra-hepatic organ, muscle, and tumor production of such acute phase reactants.
In this section, we document the APP described in the literature as potential mediators of cachexia. Table1 provides a comprehensive, current summary of the literature regarding APP, their tissue of origin, and current evidence for a role in inducing cachexia.
Alpha 1 antitrypsin(A1AT) is synthesized in the liver and serves to inhibit neutrophil elastase in the lung (169). A1AT protein is also produced by the lung and tumor microenvironment of lung cancer where it promotes metastasis, chemotherapy resistance, and decreases survival (16, 17). Although alpha 1 antitrypsin deficiency correlates with pulmonary cachexia (18), a clear role for A1AT has not been established in cancer cachexia.
The complement system is a critical component of innate and adaptive immunity. It consists of about 30 proteins that follow three different pathways to provide many functions such as defense against pathogens, opsonization, chemoattraction, apoptosis, and thrombosis (170). Historically, complement has been described as a product of the liver (171). Complement C4, C9, Factor B, C1 inhibitor, C4b-binding protein, and mannose binding lectin are recognized as acute phase proteins, however only factor B has been shown to participate in the tumor microenvironment (172) and none are synthesized by the lung or skeletal muscle according to the current literature. C3 is unique, in that all three pathways converge on this one common downstream protein, and because it has the most data regarding cachexia, we will discuss it further.
C3 has been shown to be synthesized by the lung, skeletal muscle, and is detected in the tumor microenvironment (33–39). The only study that demonstrated extrahepatic production of complement utilized the colon-26 carcinomas bearing mice (C26 mouse model) which develop cancer cachexia. This study showed increased skeletal muscle production of C3 (40). C3 has been shown in clinical studies to be upregulated in cachexia. Pre-operative pancreatic cancer patients who had cachexia and elevated CRP had an increase in serum C3a compared to non-cachectic pancreatic cancer patients (41). Complement, specifically C3, serves as a candidate for further research in cachexia. Currently, there are no functional studies which evaluate its role in cachexia.
CRP is one of the most measured APP that is secreted by the liver. CRP functions in the immune response in opsonization, phagocytosis, and cytokine signaling (173, 174). CRP mRNA and protein is expressed in the lung, however not in the skeletal muscle or tumor microenvironment (50, 51). When myoblasts are exposed to high levels of CRP, cell proliferation is inhibited (175). One challenge of examining the contribution of CRP to cachexia is that it is not synthesized in murine models of cachexia. Therefore, the relationship observed between CRP and cachexia is predominantly limited to clinical studies. In clinical studies of cachexia, the acute phase response is synonymous with CRP. Pro-inflammatory cytokines induce the APR and in turn CRP (7, 159, 176, 177). Specifically, IL-6 impacts CRP as shown by a reduction in CRP serum levels in weight-losing cancer patients by blocking IL-6 (52). In patients with pancreatic cancer who underwent neoadjuvant chemoradiation therapy, CRP was increased and a value of greater than 10 kU/L was associated with reduced survival (53). Fearon et al. has also noted that CRP may offer prognostic value in cachexia (1). The Glasgow Prognostic Score utilizes CRP as a predictor of cachexia severity (3). The modified Glasgow Prognostic Score has been proposed to identify pre-cachectic patients that may benefit from multimodal therapy to benefit the onset of cachexia (178). CRP has a role in clinical management of patients with cachexia, however it will be difficult to determine an underlying mechanism without murine studies.
Ferritin is an APP involved in the cellular stress response as well as iron regulation and homeostasis by storing iron and releasing it during times of cellular need (179). It is mainly produced in the liver. In chronic inflammatory states such as cancer, in addition to ferritin, cytokines upregulate hepcidin which acts to block iron absorption (180). Cytokines inhibit the proliferation of erythroid progenitor cells in bone marrow and erythropoietin production by the kidney. Ultimately all of these mechanisms lead to anemia of chronic disease, a common condition in cachexia (181) Currently, erythropoiesis-stimulating-agents such as epopoetin alpha, which has similar actions as endogenous erythropoietin, are given to treat this anemia (182). The series of interactions with ferritin, hepcidin, and iron homeostasis serve as potential targets for cachexia therapy that may alleviate the morbidity of anemia of chronic disease. The lung expresses ferritin mRNA and protein when exposed to stressed conditions. The skeletal muscle increases ferritin mRNA after denervation, it increases protein expression when exposed to adiponectin, and the tumor microenvironment displays ferritin protein accumulation (55–61). In-vitro models of cancer cachexia have demonstrated upregulated ferritin mRNA and protein expression in various tumor cell lines (62–64). In cachectic patients with gastric cancer, ferritin protein expression was upregulated in skeletal muscle compared to non-cachectic gastric cancer patients (65). Finally, it has been shown that by providing iron supplement in both mice and human patients with cancer cachexia, muscle function and strength improved (64). Ferritin has demonstrated evidence of involvement in cachexia both preclinically and clinically, and further studies should target the ferritin and hepcidin mechanisms of iron storage in chronic disease to increase bioavailability of iron in cachectic patients.
The majority of fibrinogen is predominantly synthesized in the liver and is involved in coagulation where it is cleaved into fibrin to form a platelet plug. The platelet plug can also trap other cell types including erythrocytes and leukocytes in pathological situations (183). Fibrinogen is produced extrahepatically in the lung during inflammation, skeletal muscle during cachectic catabolism, and in the tumor microenvironment where it is deposited from serum as well as synthesized by tumor cells themselves (66–68, 184, 185). Fibrinogen has been studied extensively in cachexia. Initial work by O’Keefe and colleagues identified protein turnover in cachexia contributes to fibrinogen production (186). Preston and colleagues demonstrated that using labeled phenylalanine, increase in circulating amino acids from skeletal muscle breakdown were ultimately used for fibrinogen synthesis in patients with pancreatic adenocarcinoma (187). Functional studies of cancer cachexia mice treated with C26 tumors had increased serum fibrinogen, however the synthesis was assumed to be hepatic (188). Another study of C26 treated mice performed a secretome analysis on the skeletal muscle and identified fibrinogen as potentially being synthesized and secreted by the skeletal muscle. Bonetto and colleagues have shown that myotubes exposed to IL-6 induced STAT3 signaling which coincided with increased fibrinogen protein expression (68). Fibrinogen production was increased as cachexia severity worsened in the C26 mouse model. Given that fibrinogen is produced by many extrahepatic tissues and is a protein of interest in cancer cachexia, it remains a protein of great interest in future studies on the APR in cachexia.
Fibronectin is mainly synthesized by the liver and has several functions. It is involved in extracellular matrix cell adhesion, it allows for host defense through participation in the immune response, and it stabilizes clot in thrombogenesis (189). Fibronectin mRNA and protein is expressed in the lung during inflammation, the protein is found in normal and regenerating skeletal muscle, and the extracellular matrix of the tumor microenvironment influencing tumor spread and growth (72–74, 76, 190, 191). Fibronectin has been examined in cachexia models. Transforming growth factor-beta (TGF-b) null mice experienced leukocyte infiltration in the heart, lungs, pancreas, stomach, colon, and salivary glands that ultimately lead to cachexia. This immune response was reduced by daily administration of fibronectin, which may have impeded the ability of leukocytes to invade target tissues (77). Fibronectin was found to be deposited in subcutaneous adipose tissue from gastrointestinal cancer patients with cachexia. There was also increased TGF-b found in these samples suggesting that TGF-b signaling may have resulted in fibronectin deposition (78). Further investigation of the inflammatory response, fibronectin, and cachexia may elucidate an underlying mechanism. The current evidence does not offer a clear mechanism at this time.
Haptoglobin is a protein produced by the liver that scavenges and binds to hemoglobin, the oxygen carrying component of red blood cells, after destruction of red blood cells. Haptoglobin may also play a role in host defense during the inflammatory response (192). Haptoglobin is synthesized by the lung during inflammation, skeletal muscle during cachexia, and tumor microenvironment where it causes cellular changes, which promote metastasis (40, 88, 90). Haptoglobin protein levels are increased in a ciliary neurotrophic factor induced cachexia rat model and cachexia associated with congestive heart failure in human patients (91, 92). Bonetto and colleagues have also shown an increased serum haptoglobin in the C26 cancer cachexia mouse model (68), in addition to several other acute phase proteins. Massart and colleagues demonstrated similar findings (40). Analysis of the contribution of lung and tumor microenvironment derived haptoglobin in cachexia serves as an area for future research.
Similar to haptoglobin, hemopexin is mainly synthesized in the liver and binds to heme after hemolysis to prevent toxicity from generation of reactive oxidative species (193). Hemopexin is expressed in the lung during inflammation and infection, skeletal muscle during atrophy, and it is present in the tumor microenvironment where it is associated with metastatic disease (93–96). There are several studies of cancer cachexia models that demonstrate a relationship to hemopexin. Proteomic profiling of C26 cancer cachexia mice gastrocnemius show an increased expression of a hemopexin precursor (97). This is consistent with other C26 data that demonstrates a 17-fold increase in hemopexin gene expression in skeletal muscle as well as almost 9000 times more hemopexin protein found in C26 skeletal muscle compared to controls (40, 68). Although these studies are associative a clear mechanistic link has yet to be demonstrated.
As the name suggests, Interleukin 1 Receptor Antagonist (IL-1Ra) is an acute phase protein produced by the liver, inhibits the IL-1 receptor and thereby acts as an anti-inflammatory mechanism (194, 195). In addition to synthesis by the liver, alveolar macrophages in the lung synthesize IL-1Ra, the protein has been detected in the skeletal muscle of patients with the muscular inflammatory diseases, dermatomyositis and polymyositis. High IL-1Ra levels were detected within the tumor microenvironment after immunotherapy and its anti-inflammatory properties may promote cancer growth (105–109). IL1-Ra has a role in cachexia however it may not directly affect tumor growth. IL1-Ra injected intratumorally in a C26 cancer cachexia model reduced IL-1 signaling within the tumor and increased mouse lean mass and fat mass, at least partially reversing the wasting effects of cachexia (110). IL-1Ra requires further evaluation as an APP in cachexia, but it has shown promise that an underlying mechanism may exist.
Lipocalin 2 is also known as neutrophil gelatinase-associated lipocalin and is a member of the lipocalin superfamily. Lipocalin 2 is mainly produced by neutrophils and is involved in the inflammatory response as well as metabolism (116). Lipocalin 2 also occurs in the lung, which produces lipocalin mRNA. Skeletal muscle produces lipocalin mRNA and protein in ob/ob mice, and the tumor microenvironment has increased lipocalin mRNA and protein (47, 111–115). Lipocalin mRNA has been upregulated in the quadriceps of mouse model of cachexia (68). Lipocalin has been further studied in a mouse model of cachexia and was shown to control cachexia-anorexia by inhibiting appetite and exacerbating the cachexia phenotype. Infusion of an antagonist for the lipocalin receptor reversed cachexia. Interestingly, this study also correlated lipocalin levels in patients with pancreatic cancer and found increased mortality in the setting of lipocalin upregulation (116). This study implicates lipocalin as a regulator of cachexia metabolism, and future studies should attempt to evaluate lipocalin antagonists in human patients.
Plasminogen Activator Inhibitor Type 1 (PAI-1) is a pro-coagulation protein involved in the coagulation cascade. Tissue plasminogen activator(tPA) and urokinase plasminogen activator (u-PA) help transform plasminogen into plasmin, which dissolves fibrin clot. PAI-1 serves as an inhibitor of both tPA and u-PA (196). PAI-1 is synthesized by the liver as well as the lung, skeletal muscle, and tumor microenvironment (120–123, 125, 197). PAI-1 has a relationship to cachexia as well. PAI-1 has been shown to be elevated in the myocardium of Walker-256 carcinoma bearing cachectic mice (126). A study of radiation therapy applied to U87MG cells, a glioblastoma cell line, caused these cells to secrete PAI-1 exosomes. When C2C12 myoblasts were treated with PAI-1 exosomes they experienced muscle wasting (127). This preliminary data regarding PAI-1 in cachexia is unable to demonstrate a clear mechanism, and this remains an area of focus for research of the APR in cachexia.
Serum Amyloid A(SAA) is synthesized by the liver during the APR and has many functions including both pro and anti-inflammatory actions critical for host survival (198). Increased SAA mRNA and protein are found in the lung and skeletal muscle during a state of inflammation, as well as the tumor microenvironment which may lead to worse tumor-free survival in patients (139–143). C26 cancer cachexia mice show elevated levels of both SAA mRNA and protein in the skeletal muscle (40, 68). SAA lacks a mechanistic connection to cachexia and more work should be done to evaluate SAA in cachexia.
Albumin is made primarily by the liver and regulates intravascular oncotic pressure and transports ligands throughout the body (199). Albumin mRNA and protein are expressed in the lung and skeletal muscle (9, 10, 200). Clinically, albumin has been studied as a biomarker to predict cachexia using scores such as the Glasgow Prognostic Score (3, 4, 12). Furthermore, there are several lines of preclinical evidence, as well. Decreased albumin in cancer cachexia is correlated to increased mortality (201). Intramuscular injections of TNFα, induced cachexia and coincided with decreased hepatic albumin synthesis (11). Of note, the decrease in albumin preceded the development of cachexia. Albumin is currently viewed as a marker of cachexia, and there is a lack of data that may explain its role in contributing to the process of cachexia.
Insulin Like Growth Factor 1 (IGF1) is a protein that is widely produced but mainly synthesized in the liver. IGF1 is involved in growth and anabolism as well as cell homeostasis and the bioavailability of sex hormones (202). IGF expression is present in skeletal muscle during growth, development and damage repair. In the tumor microenvironment, IGF protein may lead to tumor progression (98–102). Cisplatin is a commonly used chemotherapy and is sufficient to induce muscle wasting. IGF administered in conjunction with Cisplatin prevented wasting of myotubes and mouse skeletal muscle suggesting a mechanism to prevent chemotherapy induced muscle wasting (103). In congestive heart failure-associated cachexia, skeletal muscle displays reduced IGF and reactivation of IGF signaling pathways prevented skeletal muscle proteolysis (203). IGF requires more functional data in vitro and in vivo as well as human studies specifically evaluating IGF in cancer cachexia.
The APR is highly active in response to inflammatory stimuli and a critical part of the innate immune system including the potentially detrimental cachexia response. APR proteins are traditionally thought to be produced mainly by the liver. However, increasing evidence demonstrates that the lung, skeletal muscle, and tumor microenvironment express large quantities of APPs at the mRNA and protein level. Furthermore, it has been shown that cachexia provides the substrates that fuel synthesis of APPs. As an example, Preston and colleagues have demonstrated amino acids from muscle produced fibrinogen is increased in cachectic patients (187). Various studies described above have associated the APR proteins with cachexia. Albumin and CRP concentration have been predictive of patient outcomes and serve as examples of APP that have clinical applicability to cachexia. Only fibrinogen and lipocalin have had a sufficiently demonstrated role in cachexia. Fibrinogen has both increased mRNA and protein expression that is synthesized by the liver and skeletal muscle through IL-6/STAT3. Fibrinogen concentration increases as severity of cachexia increases and a mechanism of STAT3 increasing fibrinogen synthesis through proteolysis resulting in cachexia has been proposed. Lipocalin offers another plausible APP-induced cachexia mechanism as lipocalin crosses the blood-brain barrier and alters feeding behavior resulting in cachexia. However, there is a clear paucity of functional experimental data surrounding the APR in cachexia and further research is needed to elucidate this relationship. Murine models are critical in cachexia research, especially in the vital role of testing treatment efficacy and safety prior to human exposure (204). These results must be interpreted with caution, as murine models may be limited in their ability to replicate the human phenotypes of cancer and their complex microenvironment. Such models are injected with tumor cell lines as opposed to spontaneous growth, develop cachexia within weeks, and grow tumors that may be proportionately larger then human tumors (205). The acute phase response serves as a candidate for a potential molecular target that may help improve patient prognosis from cancer cachexia. Expression of many APR change and correlate to cachexia, but a mechanistic link has remained elusive. Investigating the molecular mechanisms by which the APPs are involved in cachexia has the potential to identify new targets and open new strategies to help the patients overcome cancer-associated cachexia. Untangling the relationship between the APR and cachexia still requires further investigation as it remains unclear if the APR is a mediator of cachexia.
TR: Primary research, draft manuscript, editing. BC: Additional research and content, editing of manuscript. TH: Additional research and content, editing of manuscript. DG: Additional research and content, editing of manuscript. MO: Additional research and content, editing of manuscript. TZ: Manuscript idea, additional research and content, editing of manuscript. LK: Manuscript idea, additional research and content, editing of manuscript. All authors contributed to the article and approved the submitted version.
This work was supported by by the National Institute of General Medical Sciences grant number 5R01GM137656-02(LK), the National Cancer Institute grant number 5P01CA236778-02(LK, TZ, DG, MO).
We would like to acknowledge the Department of Surgery at Indiana University School of Medicine, the Department of Surgery at Oregon Health Sciences University, and the Hollings Cancer Center at the Medical University of South Carolina for additional support for this work. The graphical abstract was created with biorender.com
The authors declare that the research was conducted in the absence of any commercial or financial relationships that could be construed as a potential conflict of interest.
All claims expressed in this article are solely those of the authors and do not necessarily represent those of their affiliated organizations, or those of the publisher, the editors and the reviewers. Any product that may be evaluated in this article, or claim that may be made by its manufacturer, is not guaranteed or endorsed by the publisher.
1. Fearon K, Strasser F, Anker SD, Bosaeus I, Bruera E, Fainsinger R, et al. Definition and classification of cancer cachexia: an international consensus. Lancet Oncol (2011) 12(5):489–95. doi: 10.1016/S1470-2045(10)70218-7
2. von Haehling S, Anker SD. Cachexia as a major underestimated and unmet medical need: facts and numbers. J Cachexia Sarcopenia Muscle (2010) 1(1):1–5. doi: 10.1007/s13539-010-0002-6
3. Forrest LM, McMillan DC, McArdle CS, Angerson WJ, Dunlop DJ. Evaluation of cumulative prognostic scores based on the systemic inflammatory response in patients with inoperable non-small-cell lung cancer. Br J Cancer (2003) 89(6):1028–30. doi: 10.1038/sj.bjc.6601242
4. Bye A, Wesseltoft-Rao N, Iversen PO, Skjegstad G, Holven KB, Ulven S, et al. Alterations in inflammatory biomarkers and energy intake in cancer cachexia: a prospective study in patients with inoperable pancreatic cancer. Med Oncol (2016) 33(6):54. doi: 10.1007/s12032-016-0768-2
5. Douglas E, McMillan DC. Towards a simple objective framework for the investigation and treatment of cancer cachexia: the Glasgow Prognostic Score. Cancer Treat Rev (2014) 40(6):685–91. doi: 10.1016/j.ctrv.2013.11.007
6. Gabay C, Kushner I. Acute-phase proteins and other systemic responses to inflammation. N Engl J Med (1999) 340(6):448–54. doi: 10.1056/NEJM199902113400607
7. Stephens NA, Skipworth RJ, Fearon KC. Cachexia, survival and the acute phase response. Curr Opin Support Palliat Care (2008) 2(4):267–74. doi: 10.1097/SPC.0b013e3283186be2
8. Tavares P, Gonçalves DM, Santos LL, Ferreira R. Revisiting the clinical usefulness of C-reactive protein in the set of cancer cachexia. Porto BioMed J (2021) 6(1):e123. doi: 10.1097/j.pbj.0000000000000123
9. Nahon JL, Tratner I, Poliard A, Presse F, Poiret M, Gal A, et al. Albumin and alpha-fetoprotein gene expression in various nonhepatic rat tissues. J Biol Chem (1988) 263(23):11436–42. doi: 10.1016/S0021-9258(18)37976-6
10. Lee Y, Hwang YH, Kim KJ, Park AK, Paik MJ, Kim SH, et al. Proteomic and transcriptomic analysis of lung tissue in OVA-challenged mice. Arch Pharm Res (2018) 41(1):87–100. doi: 10.1007/s12272-017-0972-4
11. Brenner DA, Buck M, Feitelberg SP, Chojkier M. Tumor necrosis factor-alpha inhibits albumin gene expression in a murine model of cachexia. J Clin Invest (1990) 85(1):248–55. doi: 10.1172/JCI114419
12. McMillan DC. The systemic inflammation-based Glasgow Prognostic Score: a decade of experience in patients with cancer. Cancer Treat Rev (2013) 39(5):534–40. doi: 10.1016/j.ctrv.2012.08.003
13. Fournier T, Medjoubi NN, Porquet D. Alpha-1-acid glycoprotein. Biochim Biophys Acta (2000) 1482(1-2):157–71. doi: 10.1016/S0167-4838(00)00153-9
14. Twining SS, Brecher AS. Identification of alpha1-acid glycoprotein, alpha2-macroglobulin and antithrombin III as components of normal and Malignant human tissues. Clin Chim Acta (1977) 75(1):143–8. doi: 10.1016/0009-8981(77)90510-1
15. Akaaboune M, Verdière-Sahuqué M, Lachkar S, Festoff BW, Hantaï D. Serine proteinase inhibitors in human skeletal muscle: expression of beta-amyloid protein precursor and alpha 1-antichymotrypsin in vivo and during myogenesis in vitro. J Cell Physiol (1995) 165(3):503–11. doi: 10.1002/jcp.1041650308
16. Strnad P, McElvaney NG, Lomas DA. Alpha(1)-antitrypsin deficiency. N Engl J Med (2020) 382(15):1443–55. doi: 10.1056/NEJMra1910234
17. Wu DM, Liu T, Deng SH, Han R, Zhang T, Li J, et al. Alpha-1 antitrypsin induces epithelial-to-mesenchymal transition, endothelial-to-mesenchymal transition, and drug resistance in lung cancer cells. Onco Targets Ther (2020) 13:3751–63. doi: 10.2147/OTT.S242579
18. Nakanishi T, Forgetta V, Handa T, Hirai T, Mooser V, Lathrop G, et al. The undiagnosed disease burden associated with alpha-1 antitrypsin deficiency genotypes. Eur Respir J (2020) 56(6). doi: 10.1183/13993003.01441-2020
19. Cichy J, Potempa J, Travis J. Biosynthesis of alpha1-proteinase inhibitor by human lung-derived epithelial cells. J Biol Chem (1997) 272(13):8250–5. doi: 10.1074/jbc.272.13.8250
20. Sallenave JM, Tremblay GM, Gauldie J, Richards CD. but not interleukin-6 or leukemia inhibitory factor, stimulates expression of alpha1-proteinase inhibitor in A549 human alveolar epithelial cells. J Interferon Cytokine Res (1997) 17(6):337–46. doi: 10.1089/jir.1997.17.337
21. Anes AB, Nasr HB, Hammann P, Kuhn L, Trimeche M, Hamrita B, et al. Assessment of the clinical significance of antigenic and functional levels of α1-proteinase inhibitor (α1-Pi) in infiltrating ductal breast carcinomas. Clin Biochem (2012) 45(16-17):1421–31. doi: 10.1016/j.clinbiochem.2012.07.099
22. Yoshida K, Suzuki Y, Yamamoto K, Sinohara H. cDNA sequencing of Guinea pig alpha 2-HS glycoprotein, its expression in various tissues and acute phase expression. Biol Chem (1999) 380(1):95–9. doi: 10.1515/BC.1999.013
23. Rho JH, Roehrl MH, Wang JY. Glycoproteomic analysis of human lung adenocarcinomas using glycoarrays and tandem mass spectrometry: differential expression and glycosylation patterns of vimentin and fetuin A isoforms. Protein J (2009) 28(3-4):148–60. doi: 10.1007/s10930-009-9177-0
24. Chen YY, Hsu WH, Hu HM, Wu DC, Lin WY. A case of alpha-fetoprotein-producing esophageal adenocarcinoma. Kaohsiung J Med Sci (2013) 29(2):106–10. doi: 10.1016/j.kjms.2012.08.018
25. Nuevo-Gonzalez JA, Cano-Ballesteros JC, Lopez B, Andueza-Lillo JA, Audibert L. Alpha-fetoprotein-producing extrahepatic tumor: clinical and histopathological significance of a case. J Gastrointest Cancer (2012) 43 Suppl 1:S28–31. doi: 10.1007/s12029-011-9310-0
26. Tai CS, Lin YR, Teng TH, Lin PY, Tu SJ, Chou CH, et al. Haptoglobin expression correlates with tumor differentiation and five-year overall survival rate in hepatocellular carcinoma. PloS One (2017) 12(2):e0171269. doi: 10.1371/journal.pone.0171269
27. Campbell DJ, Habener JF. Hybridization in situ studies of angiotensinogen gene expression in rat adrenal and lung. Endocrinology (1989) 124(1):218–22. doi: 10.1210/endo-124-1-218
28. Kitamura N, Ohkubo H, Nakanishi S. Molecular biology of the angiotensinogen and kininogen genes. J Cardiovasc Pharmacol (1987) 10 Suppl 7:S49–53. doi: 10.1097/00005344-198706107-00008
29. Uhal BD, Zhang H, Abdul-Hafez A, Shu R, Li X. Amiodarone induces angiotensinogen gene expression in lung alveolar epithelial cells through activation protein-1. Basic Clin Pharmacol Toxicol (2007) 100(1):59–66. doi: 10.1111/j.1742-7843.2007.00006.x
30. Johnston AP, Baker J, De Lisio M, Parise G. Skeletal muscle myoblasts possess a stretch-responsive local angiotensin signalling system. J Renin Angiotensin Aldosterone Syst (2011) 12(2):75–84. doi: 10.1177/1470320310381795
31. Gorman JL, Liu ST, Slopack D, Shariati K, Hasanee A, Olenich S, et al. Angiotensin II evokes angiogenic signals within skeletal muscle through co-ordinated effects on skeletal myocytes and endothelial cells. PloS One (2014) 9(1):e85537. doi: 10.1371/journal.pone.0085537
32. Bujak-Gizycka B, Madej J, Bystrowska B, Toton-Zuranska J, Kus K, Kolton-Wroz M, et al. Angiotensin 1-7 formation in breast tissue is attenuated in breast cancer - a study on the metabolism of angiotensinogen in breast cancer cell lines. J Physiol Pharmacol (2019) 70(4). doi: 10.26402/jpp.2019.4.02
33. Chaudhary N, Jayaraman A, Reinhardt C, Campbell JD, Bosmann M. A single-cell lung atlas of complement genes identifies the mesothelium and epithelium as prominent sources of extrahepatic complement proteins. Mucosal Immunol (2022) 15(5):927–39. doi: 10.1038/s41385-022-00534-7
34. Varsano S, Kaminsky M, Kaiser M, Rashkovsky L. Generation of complement C3 and expression of cell membrane complement inhibitory proteins by human bronchial epithelium cell line. Thorax (2000) 55(5):364–9. doi: 10.1136/thorax.55.5.364
35. Legoedec J, Gasque P, Jeanne JF, Fontaine M. Expression of the complement alternative pathway by human myoblasts in vitro: biosynthesis of C3, factor B, factor H and factor I. Eur J Immunol (1995) 25(12):3460–6. doi: 10.1002/eji.1830251238
36. Boire A, Zou Y, Shieh J, Macalinao DG, Pentsova E, Massagué J. Complement component 3 adapts the cerebrospinal fluid for leptomeningeal metastasis. Cell (2017) 168(6):1101–1113.e13. doi: 10.1016/j.cell.2017.02.025
37. Davidson S, Efremova M, Riedel A, Mahata B, Pramanik J, Huuhtanen J, et al. Single-cell RNA sequencing reveals a dynamic stromal niche that supports tumor growth. Cell Rep (2020) 31(7):107628. doi: 10.1016/j.celrep.2020.107628
38. Yuan K, Ye J, Liu Z, Ren Y, He W, Xu J, et al. Complement C3 overexpression activates JAK2/STAT3 pathway and correlates with gastric cancer progression. J Exp Clin Cancer Res (2020) 39(1):9. doi: 10.1186/s13046-019-1514-3
39. Gadwa J, Bickett TE, Darragh LB, Knitz MW, Bhatia S, Piper M, et al. Complement C3a and C5a receptor blockade modulates regulatory T cell conversion in head and neck cancer. J Immunother Cancer (2021) 9(3). doi: 10.1136/jitc-2021-002585
40. Massart IS, Paulissen G, Loumaye A, Lause P, Pötgens SA, Thibaut MM, et al. Marked increased production of acute phase reactants by skeletal muscle during cancer cachexia. Cancers (Basel) (2020) 12(11):3221. doi: 10.3390/cancers12113221
41. Deng M, Vaes RDW, van Bijnen AAJHM, Olde Damink SWM, Rensen SS. Activation of the complement system in patients with cancer cachexia. Cancers (Basel) (2021) 13(22). doi: 10.3390/cancers13225767
42. Yamamoto T, Ebe Y, Hasegawa G, Kataoka M, Yamamoto S, Naito M. Expression of scavenger receptor class A and CD14 in lipopolysaccharide-induced lung injury. Pathol Int (1999) 49(11):983–92. doi: 10.1046/j.1440-1827.1999.00978.x
43. Fearns C, Kravchenko VV, Ulevitch RJ, Loskutoff DJ. Murine CD14 gene expression in vivo: extramyeloid synthesis and regulation by lipopolysaccharide. J Exp Med (1995) 181(3):857–66. doi: 10.1084/jem.181.3.857
44. Nishimura M, Naito S. Tissue-specific mRNA expression profiles of human toll-like receptors and related genes. Biol Pharm Bull (2005) 28(5):886–92. doi: 10.1248/bpb.28.886
45. Stigliani S, Croce M, Morandi F, Scaruffi P, Rigo V, Carlini B. Expression of FOXP3, CD14, and ARG1 in neuroblastoma tumor tissue from high-risk patients predicts event-free and overall survival. BioMed Res Int 2015. (2015) p:347867. doi: 10.1155/2015/347867
46. Cheah MT, Chen JY, Sahoo D, Contreras-Trujillo H, Volkmer AK, Scheeren FA, et al. CD14-expressing cancer cells establish the inflammatory and proliferative tumor microenvironment in bladder cancer. Proc Natl Acad Sci U.S.A. (2015) 112(15):4725–30. doi: 10.1073/pnas.1424795112
47. Sun B, Guo W, Hu S, Yao F, Yu K, Xing J, et al. Gprc5a-knockout mouse lung epithelial cells predicts ceruloplasmin, lipocalin 2 and periostin as potential biomarkers at early stages of lung tumorigenesis. Oncotarget (2017) 8(8):13532–44. doi: 10.18632/oncotarget.14589
48. Fleming RE, Whitman IP, Gitlin JD. Induction of ceruloplasmin gene expression in rat lung during inflammation and hyperoxia. Am J Physiol (1991) 260(2 Pt 1):L68–74. doi: 10.1152/ajplung.1991.260.2.L68
49. Matsuoka R, Shiba-Ishii A, Nakano N, Togayachi A, Sakashita S, Sato Y, et al. Heterotopic production of ceruloplasmin by lung adenocarcinoma is significantly correlated with prognosis. Lung Cancer (2018) 118:97–104. doi: 10.1016/j.lungcan.2018.01.012
50. Lopez-Campos JL, Calero C, Rojano B, López-Porras M, Sáenz-Coronilla J, Blanco AI, et al. C-reactive protein and serum amyloid a overexpression in lung tissues of chronic obstructive pulmonary disease patients: a case-control study. Int J Med Sci (2013) 10(8):938–47. doi: 10.7150/ijms.6152
51. Arellano-Orden E, Calero C, López-Ramírez C, Sánchez-López V, López-Villalobos JL, Abad Arranz M, et al. Evaluation of lung parenchyma, blood vessels, and peripheral blood lymphocytes as a potential source of acute phase reactants in patients with COPD. Int J Chron Obstruct Pulmon Dis (2019) 14:1323–32. doi: 10.2147/COPD.S188567
52. O’Riordain MG, Falconer JS, Maingay J, Fearon KC, Ross JA. Peripheral blood cells from weight-losing cancer patients control the hepatic acute phase response by a primarily interleukin-6 dependent mechanism. Int J Oncol (1999) 15(4):823–7. doi: 10.3892/ijo.15.4.823
53. Naumann P, Eberlein J, Farnia B, Liermann J, Hackert T, Debus J, et al. Cachectic body composition and inflammatory markers portend a poor prognosis in patients with locally advanced pancreatic cancer treated with chemoradiation. Cancers (Basel) (2019) 11(11):1655. doi: 10.3390/cancers11111655
54. Jablonska E, Markart P, Zakrzewicz D, Preissner KT, Wygrecka M. Transforming growth factor-beta1 induces expression of human coagulation factor XII via Smad3 and JNK signaling pathways in human lung fibroblasts. J Biol Chem (2010) 285(15):11638–51. doi: 10.1074/jbc.M109.045963
55. Carraway MS, Ghio AJ, Taylor JL, Piantadosi CA. Induction of ferritin and heme oxygenase-1 by endotoxin in the lung. Am J Physiol (1998) 275(3):L583–92. doi: 10.1152/ajplung.1998.275.3.L583
56. Ghio AJ, Carter JD, Samet JM, Reed W, Quay J, Dailey LA, et al. Metal-dependent expression of ferritin and lactoferrin by respiratory epithelial cells. Am J Physiol (1998) 274(5):L728–36. doi: 10.1152/ajplung.1998.274.5.L728
57. Smith KR, Aust AE. Mobilization of iron from urban particulates leads to generation of reactive oxygen species in vitro and induction of ferritin synthesis in human lung epithelial cells. Chem Res Toxicol (1997) 10(7):828–34. doi: 10.1021/tx960164m
58. Kitahara T, Kiryu S, Takeda N, Kubo T, Kiyama H. Up-regulation of ferritin heavy chain mRNA expression in the rat skeletal muscle after denervation: detected by means of differential display. Neurosci Res (1995) 23(4):353–60. doi: 10.1016/0168-0102(95)00963-T
59. Ikegami Y, Inukai K, Imai K, Sakamoto Y, Katagiri H, Kurihara S, et al. Adiponectin upregulates ferritin heavy chain in skeletal muscle cells. Diabetes (2009) 58(1):61–70. doi: 10.2337/db07-0690
60. Kukulj S, Jaganjac M, Boranic M, Krizanac S, Santic Z, Poljak-Blazi M. Altered iron metabolism, inflammation, transferrin receptors, and ferritin expression in non-small-cell lung cancer. Med Oncol (2010) 27(2):268–77. doi: 10.1007/s12032-009-9203-2
61. Petronek MS, Tomanek-Chalkley AM, Monga V, Milhem MM, Miller BJ, Magnotta VA, et al. Detection of ferritin expression in soft tissue sarcomas with MRI: potential implications for iron metabolic therapy. Iowa Orthop J (2022) 42(1):255–62.
62. Monitto CL, Berkowitz D, Lee KM, Pin S, Li D, Breslow M. Differential gene expression in a murine model of cancer cachexia. Am J Physiol Endocrinol Metab (2001) 281(2):E289–97. doi: 10.1152/ajpendo.2001.281.2.E289
63. Modjtahedi N, Frebourg T, Fossar N, Lavialle C, Cremisi C, Brison O. Increased expression of cytokeratin and ferritin-H genes in tumorigenic clones of the SW 613-S human colon carcinoma cell line. Exp Cell Res (1992) 201(1):74–82. doi: 10.1016/0014-4827(92)90349-D
64. Wyart E, Hsu MY, Sartori R, Mina E, Rausch V, Pierobon ES. Iron supplementation is sufficient to rescue skeletal muscle mass and function in cancer cachexia. EMBO Rep (2022) 23(4):e53746. doi: 10.15252/embr.202153746
65. Zhou D, Zhang Y, Mamtawla G, Wan S, Gao X, Zhang L, et al. Iron overload is related to muscle wasting in patients with cachexia of gastric cancer: using quantitative proteome analysis. Med Oncol (2020) 37(12):113. doi: 10.1007/s12032-020-01439-w
66. Simpson-Haidaris PJ, Courtney MA, Wright TW, Goss R, Harmsen A, Gigliotti F. Induction of fibrinogen expression in the lung epithelium during Pneumocystis carinii pneumonia. Infect Immun (1998) 66(9):4431–9. doi: 10.1128/IAI.66.9.4431-4439.1998
67. Haidaris PJ. Induction of fibrinogen biosynthesis and secretion from cultured pulmonary epithelial cells. Blood (1997) 89(3):873–82. doi: 10.1182/blood.V89.3.873
68. Bonetto A, Aydogdu T, Kunzevitzky N, Guttridge DC, Khuri S, Koniaris LG, et al. STAT3 activation in skeletal muscle links muscle wasting and the acute phase response in cancer cachexia. PloS One (2011) 6(7):e22538. doi: 10.1371/journal.pone.0022538
69. Dzikowski L, Mirzaei R, Sarkar S, Kumar M, Bose P, Bellail A, et al. Fibrinogen in the glioblastoma microenvironment contributes to the invasiveness of brain tumor-initiating cells. Brain Pathol (2021) 31(5):e12947. doi: 10.1111/bpa.12947
70. Rybarczyk BJ, Simpson-Haidaris PJ. Fibrinogen assembly, secretion, and deposition into extracellular matrix by MCF-7 human breast carcinoma cells. Cancer Res (2000) 60(7):2033–9.
71. Adams GN, Rosenfeldt L, Frederick M, Miller W, Waltz D, Kombrinck K, et al. Colon cancer growth and dissemination relies upon thrombin, stromal PAR-1, and fibrinogen. Cancer Res (2015) 75(19):4235–43. doi: 10.1158/0008-5472.CAN-15-0964
72. Muñoz-Esquerre M, Huertas D, Escobar I, López-Sánchez M, Penín R, Peinado V, et al. Gene and protein expression of fibronectin and tenascin-C in lung samples from COPD patients. Lung (2015) 193(3):335–43. doi: 10.1007/s00408-015-9717-7
73. Adachi Y, Mio T, Striz I, Carnevali S, Romberger DJ, Spurzem JR, et al. Lipopolysaccharide increases fibronectin production and release from cultured lung fibroblasts partially through proteolytic activity. J Lab Clin Med (1996) 127(5):448–55. doi: 10.1016/S0022-2143(96)90062-1
74. Gulati AK, Reddi AH, Zalewski AA. Distribution of fibronectin in normal and regenerating skeletal muscle. Anat Rec (1982) 204(3):175–83. doi: 10.1002/ar.1092040302
75. Zanotti S, Gibertini S, Blasevich F, Bragato C, Ruggieri A, Saredi S, et al. Exosomes and exosomal miRNAs from muscle-derived fibroblasts promote skeletal muscle fibrosis. Matrix Biol (2018) 74:77–100. doi: 10.1016/j.matbio.2018.07.003
76. Castellani P, Borsi L, Carnemolla B, Birò A, Dorcaratto A, Viale GL, et al. Differentiation between high- and low-grade astrocytoma using a human recombinant antibody to the extra domain-B of fibronectin. Am J Pathol (2002) 161(5):1695–700. doi: 10.1016/S0002-9440(10)64446-X
77. Hines KL, Kulkarni AB, McCarthy JB, Tian H, Ward JM, Christ M, et al. Synthetic fibronectin peptides interrupt inflammatory cell infiltration in transforming growth factor beta 1 knockout mice. Proc Natl Acad Sci U.S.A. (1994) 91(11):5187–91. doi: 10.1073/pnas.91.11.5187
78. Alves MJ, Figuerêdo RG, Azevedo FF, Cavallaro DA, Neto NIP, Lima JDC, et al. Adipose tissue fibrosis in human cancer cachexia: the role of TGFbeta pathway. BMC Cancer (2017) 17(1):190. doi: 10.1186/s12885-017-3178-8
79. Ramachandran R, Morice AH, Compton SJ. Proteinase-activated receptor2 agonists upregulate granulocyte colony-stimulating factor, IL-8, and VCAM-1 expression in human bronchial fibroblasts. Am J Respir Cell Mol Biol (2006) 35(1):133–41. doi: 10.1165/rcmb.2005-0362OC
80. Wiedermann FJ, Mayr AJ, Kaneider NC, Fuchs D, Mutz NJ, Schobersberger W. Alveolar granulocyte colony-stimulating factor and alpha-chemokines in relation to serum levels, pulmonary neutrophilia, and severity of lung injury in ARDS. Chest (2004) 125(1):212–9. doi: 10.1378/chest.125.1.212
81. Ohashi M, Okubo K, Mizuno S, Yoda M, Shirasawa H, Chiba K, et al. Granulocyte-colony stimulating factor enhances load-induced muscle hypertrophy in mice. Biochem Biophys Res Commun (2018) 506(4):944–9. doi: 10.1016/j.bbrc.2018.10.196
82. Hara M, Yuasa S, Shimoji K, Onizuka T, Hayashiji N, Ohno Y, et al. G-CSF influences mouse skeletal muscle development and regeneration by stimulating myoblast proliferation. J Exp Med (2011) 208(4):715–27. doi: 10.1084/jem.20101059
83. Saunders AS, Bender DE, Ray AL, Wu X, Morris KT. Colony-stimulating factor 3 signaling in colon and rectal cancers: Immune response and CMS classification in TCGA data. PloS One (2021) 16(2):e0247233. doi: 10.1371/journal.pone.0247233
84. Mueller MM, Peter W, Mappes M, Huelsen A, Steinbauer H, Boukamp P, et al. Tumor progression of skin carcinoma cells in vivo promoted by clonal selection, mutagenesis, and autocrine growth regulation by granulocyte colony-stimulating factor and granulocyte-macrophage colony-stimulating factor. Am J Pathol (2001) 159(4):1567–79. doi: 10.1016/S0002-9440(10)62541-2
85. Tamura K, Ishigaki K, Iizuka K, Nagumo T, Yoshida O, Asano K, et al. Neutrophilic leucocytosis induced by granulocyte colony-stimulating factor and interleukin-6 in canine primary lung adenocarcinoma. Vet Med Sci (2022) 8(2):483–91. doi: 10.1002/vms3.694
86. Miyazaki K, Shiba A, Ikeda T, Higashi Y, Aga M, Hamakawa Y, et al. Programmed cell death ligand 1 measurement study in granulocyte colony-stimulating factor-producing lung cancer: an observational study. BMC Cancer (2022) 22(1):977. doi: 10.1186/s12885-022-10065-w
87. Yoshinaga Y, Kiyozaki H, Okada S, Konishi F, Yamada S. Granulocyte-colony-stimulating factor-producing gastric metastasis from large cell type lung cancer. Clin J Gastroenterol (2011) 4(1):10–4. doi: 10.1007/s12328-010-0196-3
88. Abdullah M, Schultz H, Kähler D, Branscheid D, Dalhoff K, Zabel P, et al. Expression of the acute phase protein haptoglobin in human lung cancer and tumor-free lung tissues. Pathol Res Pract (2009) 205(9):639–47. doi: 10.1016/j.prp.2009.04.007
89. Kruger K, Dischereit G, Seimetz M, Wilhelm J, Weissmann N, Mooren FC. Time course of cigarette smoke-induced changes of systemic inflammation and muscle structure. Am J Physiol Lung Cell Mol Physiol (2015) 309(2):L119–28. doi: 10.1152/ajplung.00074.2015
90. Garibay-Cerdenares OL, Hernández-Ramírez VI, Osorio-Trujillo JC, Gallardo-Rincón D, Talamás-Rohana P, et al. Haptoglobin and CCR2 receptor expression in ovarian cancer cells that were exposed to ascitic fluid: exploring a new role of haptoglobin in the tumoral microenvironment. Cell Adh Migr (2015) 9(5):394–405. doi: 10.1080/19336918.2015.1035504
91. Henderson JT, Seniuk NA, Richardson PM, Gauldie J, Roder JC. Systemic administration of ciliary neurotrophic factor induces cachexia in rodents. J Clin Invest (1994) 93(6):2632–8. doi: 10.1172/JCI117276
92. Karim A, Muhammad T, Shah I, Khan J, Qaisar R. Relationship of haptoglobin phenotypes with sarcopaenia in patients with congestive heart failure. Heart Lung Circ (2022) 31(6):822–31. doi: 10.1016/j.hlc.2022.01.003
93. Yang N, Zhang L, Tian D, Wang P, Men K, Ge Y, et al. Tanshinone increases Hemopexin expression in lung cells and macrophages to protect against cigarette smoke-induced COPD and enhance antiviral responses. Cell Cycle (2022) 22(6):1–21. doi: 10.1080/15384101.2022.2129933
94. Wang XF, Zhang XY, Gao X, Liu XX, Wang YH. Proteomic profiling of a respiratory syncytial virus-infected rat pneumonia model. Jpn J Infect Dis (2016) 69(4):285–92. doi: 10.7883/yoken.JJID.2015.244
95. Nagase T, Tohda C. Skeletal muscle atrophy-induced hemopexin accelerates onset of cognitive impairment in Alzheimer’s disease. J Cachexia Sarcopenia Muscle (2021) 12(6):2199–210. doi: 10.1002/jcsm.12830
96. Suzuki Y, Takadate T, Mizuma M, Shima H, Suzuki T, Tachibana T, et al. Stromal expression of hemopexin is associated with lymph-node metastasis in pancreatic ductal adenocarcinoma. PloS One (2020) 15(7):e0235904. doi: 10.1371/journal.pone.0235904
97. Shum AMY, Poljak A, Bentley NL, Turner N, Tan TC, Polly P. Proteomic profiling of skeletal and cardiac muscle in cancer cachexia: alterations in sarcomeric and mitochondrial protein expression. Oncotarget (2018) 9(31):22001–22. doi: 10.18632/oncotarget.25146
98. Tollefsen SE, Lajara R, McCusker RH, Clemmons DR, Rotwein P. Insulin-like growth factors (IGF) in muscle development. Expression IGF-I IGF-I receptor an IGF binding Protein during myoblast differentiation. J Biol Chem (1989) 264(23):13810–7. doi: 10.1016/S0021-9258(18)80073-4
99. Philippou A, Papageorgiou E, Bogdanis G, Halapas A, Sourla A, Maridaki M, et al. Expression of IGF-1 isoforms after exercise-induced muscle damage in humans: characterization of the MGF E peptide actions in vitro. In Vivo (2009) 23(4):567–75.
100. Reinmuth N, Kloos S, Warth A, Risch A, Muley T, Hoffmann H, et al. Insulin-like growth factor 1 pathway mutations and protein expression in resected non-small cell lung cancer. Hum Pathol (2014) 45(6):1162–8. doi: 10.1016/j.humpath.2014.01.010
101. Fu S, Tang H, Liao Y, Xu Q, Liu C, Deng Y, et al. Expression and clinical significance of insulin-like growth factor 1 in lung cancer tissues and perioperative circulation from patients with non-small-cell lung cancer. Curr Oncol (2016) 23(1):12–9. doi: 10.3747/co.23.2669
102. Tang H, Liao Y, Xu L, Zhang C, Liu Z, Deng Y, et al. Estrogen and insulin-like growth factor 1 synergistically promote the development of lung adenocarcinoma in mice. Int J Cancer (2013) 133(10):2473–82. doi: 10.1002/ijc.28262
103. Sakai H, Asami M, Naito H, Kitora S, Suzuki Y, Miyauchi Y, et al. Exogenous insulin-like growth factor 1 attenuates cisplatin-induced muscle atrophy in mice. J Cachexia Sarcopenia Muscle (2021) 12(6):1570–81. doi: 10.1002/jcsm.12760
104. Martin J, Midgley A, Meran S, Woods E, Bowen T, Phillips A, et al. Tumor necrosis factor-stimulated gene 6 (TSG-6)-mediated interactions with the inter-alpha-inhibitor heavy chain 5 facilitate tumor growth factor beta1 (TGFbeta1)-dependent fibroblast to myofibroblast differentiation. J Biol Chem (2016) 291(26):13789–801. doi: 10.1074/jbc.M115.670521
105. Moore SA, Strieter RM, Rolfe MW, Standiford TJ, Burdick MD, Kunkel SL. Expression and regulation of human alveolar macrophage-derived interleukin-1 receptor antagonist. Am J Respir Cell Mol Biol (1992) 6(6):569–75. doi: 10.1165/ajrcmb/6.6.569
106. Yanagawa H, Sone S, Haku T, Mizuno K, Yano S, Ohmoto Y. Contrasting effect of interleukin-13 on interleukin-1 receptor antagonist and proinflammatory cytokine production by human alveolar macrophages. Am J Respir Cell Mol Biol (1995) 12(1):71–6. doi: 10.1165/ajrcmb.12.1.7811472
107. Grundtman C, Salomonsson S, Dorph C, Bruton J, Andersson U, Lundberg IE. Immunolocalization of interleukin-1 receptors in the sarcolemma and nuclei of skeletal muscle in patients with idiopathic inflammatory myopathies. Arthritis Rheum (2007) 56(2):674–87. doi: 10.1002/art.22388
108. Fan YC, Lee KD, Tsai YC. Roles of interleukin-1 receptor antagonist in prostate cancer progression. Biomedicines (2020) 8(12):602. doi: 10.3390/biomedicines8120602
109. Lu S, Dong Z. Overexpression of secretory phospholipase A2-IIa supports cancer stem cell phenotype via HER/ERBB-elicited signaling in lung and prostate cancer cells. Int J Oncol (2017) 50(6):2113–22. doi: 10.3892/ijo.2017.3964
110. Strassmann G, Masui Y, Chizzonite R, Fong M. Mechanisms of experimental cancer cachexia. Local involvement IL-1 colon-26 tumor. J Immunol (1993) 150(6):2341–5.
111. Cowland JB, Borregaard N. Molecular characterization and pattern of tissue expression of the gene for neutrophil gelatinase-associated lipocalin from humans. Genomics (1997) 45(1):17–23. doi: 10.1006/geno.1997.4896
112. Choi EB, Jeong JH, Jang HM, Ahn YJ, Kim KH, An HS, et al. Skeletal lipocalin-2 is associated with iron-related oxidative stress in ob/ob mice with sarcopenia. Antioxidants (Basel) (2021) 10(5):758. doi: 10.3390/antiox10050758
113. Zhang PX, Chang JX, Xie JJ, Yuan HM, Du ZP, Zhang FR, et al. Regulation of neutrophil gelatinase-associated lipocalin expression by C/EBPbeta in lung carcinoma cells. Oncol Lett (2012) 4(5):919–24. doi: 10.3892/ol.2012.859
114. Shiiba M, Saito K, Fushimi K, Ishigami T, Shinozuka K, Nakashima D, et al. Lipocalin-2 is associated with radioresistance in oral cancer and lung cancer cells. Int J Oncol (2013) 42(4):1197–204. doi: 10.3892/ijo.2013.1815
115. Gomez-Chou SB, Swidnicka-Siergiejko AK, Badi N, Chavez-Tomar M, Lesinski GB, Bekaii-Saab T, et al. Lipocalin-2 promotes pancreatic ductal adenocarcinoma by regulating inflammation in the tumor microenvironment. Cancer Res (2017) 77(10):2647–60. doi: 10.1158/0008-5472.CAN-16-1986
116. Olson B, Zhu X, Norgard MA, Levasseur PR, Butler JT, Buenafe A, et al. Lipocalin 2 mediates appetite suppression during pancreatic cancer cachexia. Nat Commun (2021) 12(1):2057. doi: 10.1038/s41467-021-22361-3
117. Heymann CJF, Bobin-Dubigeon C, Muñoz-Garcia J, Cochonneau D, Ollivier E, Heymann MF, et al. Lipopolysaccharide-binding protein expression is associated to the metastatic status of osteosarcoma patients. J Bone Oncol (2022) 36:100451. doi: 10.1016/j.jbo.2022.100451
118. Qiao B, Chen Z, Huang J, Lam AK, Mei Z, Li Y, et al. Lipopolysaccharide-binding protein as a biomarker in oral and maxillofacial tumors. Oral Dis (2021) 29(3):892–901. doi: 10.1111/odi.14042
119. Shibata T, Ogawa M, Takata N, Matsuda K, Niinobu T, Uda K, et al. Distribution of pancreatic secretory trypsin inhibitor in various human tissues and its inactivation in the gastric mucosa. Res Commun Chem Pathol Pharmacol (1987) 55(2):243–8.
120. Liu F, Lagares D, Choi KM, Stopfer L, Marinković A, Vrbanac V, et al. Mechanosignaling through YAP and TAZ drives fibroblast activation and fibrosis. Am J Physiol Lung Cell Mol Physiol (2015) 308(4):L344–57. doi: 10.1152/ajplung.00300.2014
121. Lardot C, Heusterpreute M, Mertens P, Philippe M, Lison D. Expression of plasminogen activator inhibitors type-1 and type-2 in the mouse lung after administration of crystalline silica. Eur Respir J (1998) 11(4):912–21. doi: 10.1183/09031936.98.11040912
122. Huebner BR, Moore EE, Moore HB, Gonzalez E, Kelher MR, Sauaia A, et al. Thrombin stimulates increased plasminogen activator inhibitor-1 release from liver compared to lung endothelium. J Surg Res (2018) 225:1–5. doi: 10.1016/j.jss.2017.12.017
123. Fibbi G, Barletta E, Dini G, Del Rosso A, Pucci M, Cerletti M, et al. Cell invasion is affected by differential expression of the urokinase plasminogen activator/urokinase plasminogen activator receptor system in muscle satellite cells from normal and dystrophic patients. Lab Invest (2001) 81(1):27–39. doi: 10.1038/labinvest.3780209
124. Naderi J, Bernreuther C, Grabinski N, Putman CT, Henkel B, Bell G, et al. Plasminogen activator inhibitor type 1 up-regulation is associated with skeletal muscle atrophy and associated fibrosis. Am J Pathol (2009) 175(2):763–71. doi: 10.2353/ajpath.2009.081009
125. Kubala MH, Punj V, Placencio-Hickok VR, Fang H, Fernandez GE, Sposto R, et al. Plasminogen activator inhibitor-1 promotes the recruitment and polarization of macrophages in cancer. Cell Rep (2018) 25(8):2177–2191.e7. doi: 10.1016/j.celrep.2018.10.082
126. Toneto AT, Ferreira Ramos LA, Salomão EM, Tomasin R, Aereas MA, Gomes-Marcondes MC, et al. Nutritional leucine supplementation attenuates cardiac failure in tumour-bearing cachectic animals. J Cachexia Sarcopenia Muscle (2016) 7(5):577–86. doi: 10.1002/jcsm.12100
127. Shin E, Kang H, Lee H, Lee S, Jeon J, Seong K, et al. Exosomal plasminogen activator inhibitor-1 induces ionizing radiation-adaptive glioblastoma cachexia. Cells (2022) 11(19):3102. doi: 10.3390/cells11193102
128. Kramer PA, Duan J, Gaffrey MJ, Shukla AK, Wang L, Bammler TK, et al. Fatiguing contractions increase protein S-glutathionylation occupancy in mouse skeletal muscle. Redox Biol (2018) 17:367–76. doi: 10.1016/j.redox.2018.05.011
129. Al Kafri N, Hafizi S. Tumour-secreted protein S (ProS1) activates a tyro3-erk signalling axis and protects cancer cells from apoptosis. Cancers (Basel) (2019) 11(12):1843. doi: 10.3390/cancers11121843
130. Soprano DR, Herbert J, Soprano KJ, Schon EA, Goodman DS. Demonstration of transthyretin mRNA in the brain and other extrahepatic tissues in the rat. J Biol Chem (1985) 260(21):11793–8. doi: 10.1016/S0021-9258(17)39100-7
131. Deng X, Ren J, Bi Z, Fu Z. Positive expression of retinol-binding protein 4 is related to the Malignant clinical features leading to poor prognosis of glioblastoma. Genet Res (Camb) 2022. (2022) p:5435523. doi: 10.1155/2022/5435523
132. Li M, Wang Z, Zhu L, Shui Y, Zhang S, Guo W. Down-regulation of RBP4 indicates a poor prognosis and correlates with immune cell infiltration in hepatocellular carcinoma. Biosci Rep (2021) 41(4). doi: 10.1042/BSR20210328
133. Papiernik D, Urbaniak A, Kłopotowska D, Nasulewicz-Goldeman A, Ekiert M, Nowak M, et al. Retinol-binding protein 4 accelerates metastatic spread and increases impairment of blood flow in mouse mammary gland tumors. Cancers (Basel) (2020) 12(3):623. doi: 10.3390/cancers12030623
134. Wu T, Levine SJ, Lawrence MG, Logun C, Angus CW, Shelhamer JH. Interferon-gamma induces the synthesis and activation of cytosolic phospholipase A2. J Clin Invest (1994) 93(2):571–7. doi: 10.1172/JCI117009
135. Yang CM, Lee IT, Chi PL, Cheng SE, Hsiao LD, Hsu CK. TNF-alpha induces cytosolic phospholipase A2 expression via Jak2/PDGFR-dependent Elk-1/p300 activation in human lung epithelial cells. Am J Physiol Lung Cell Mol Physiol (2014) 306(6):L543–51. doi: 10.1152/ajplung.00320.2013
136. Valentin E, Ghomashchi F, Gelb MH, Lazdunski M, Lambeau G. Novel human secreted phospholipase A(2) with homology to the group III bee venom enzyme. J Biol Chem (2000) 275(11):7492–6. doi: 10.1074/jbc.275.11.7492
137. Gelb MH, Valentin E, Ghomashchi F, Lazdunski M, Lambeau G. Cloning and recombinant expression of a structurally novel human secreted phospholipase A2. J Biol Chem (2000) 275(51):39823–6. doi: 10.1074/jbc.C000671200
138. Hiyoshi M, Kitayama J, Kazama S, Taketomi Y, Murakami M, Tsuno NH, et al. The expression of phospholipase A2 group X is inversely associated with metastasis in colorectal cancer. Oncol Lett (2013) 5(2):533–8. doi: 10.3892/ol.2012.1067
139. Calero C, Arellano E, Lopez-Villalobos JL, Sánchez-López V, Moreno-Mata N, López-Campos JL. Differential expression of C-reactive protein and serum amyloid A in different cell types in the lung tissue of chronic obstructive pulmonary disease patients. BMC Pulm Med (2014) 14:95. doi: 10.1186/1471-2466-14-95
140. Ray A, Ray BK. Persistent expression of serum amyloid A during experimentally induced chronic inflammatory condition in rabbit involves differential activation of SAF, NF-kappa B, and C/EBP transcription factors. J Immunol (1999) 163(4):2143–50. doi: 10.4049/jimmunol.163.4.2143
141. Langhans C, Weber-Carstens S, Schmidt F, Hamati J, Kny M, Zhu X, et al. Inflammation-induced acute phase response in skeletal muscle and critical illness myopathy. PloS One (2014) 9(3):e92048. doi: 10.1371/journal.pone.0092048
142. Yang M, Liu F, Higuchi K, Sawashita J, Fu X, Zhang L, et al. Serum amyloid A expression in the breast cancer tissue is associated with poor prognosis. Oncotarget (2016) 7(24):35843–52. doi: 10.18632/oncotarget.8561
143. Ignacio RMC, Gibbs CR, Kim S, Lee ES, Adunyah SE, Son DS. Serum amyloid A predisposes inflammatory tumor microenvironment in triple negative breast cancer. Oncotarget (2019) 10(4):511–26. doi: 10.18632/oncotarget.26566
144. Gershengorn MC, Glinoer D, Fox SH, Robbins J. Thyroxine-binding globulin synthesis by hepatocarcinoma cells in continuous culture: effect of physiological concentrations of thyroxine. Biochem Biophys Res Commun (1976) 71(1):76–82. doi: 10.1016/0006-291X(76)90251-5
145. Yang F, Friedrichs WE, Coalson JJ. Regulation of transferrin gene expression during lung development and injury. Am J Physiol (1997) 273(2 Pt 1):L417–26. doi: 10.1152/ajplung.1997.273.2.L417
146. Skinner SJ, Somervell CE, Buch S, Post M. Transferrin gene expression and transferrin immunolocalization in developing foetal rat lung. J Cell Sci (1991) 99(Pt 3):651–6. doi: 10.1242/jcs.99.3.651
147. Li Y, Cheng JX, Yang HH, Chen LP, Liu FJ, Wu Y, et al. Transferrin receptor 1 plays an important role in muscle development and denervation-induced muscular atrophy. Neural Regener Res (2021) 16(7):1308–16. doi: 10.4103/1673-5374.301024
148. O’Connell K, Gannon J, Doran P, Ohlendieck K. Proteomic profiling reveals a severely perturbed protein expression pattern in aged skeletal muscle. Int J Mol Med (2007) 20(2):145–53.
149. Lee EJ, Bhat AR, Kamli MR, Pokharel S, Chun T, Lee YH, et al. Transthyretin is a key regulator of myoblast differentiation. PloS One (2013) 8(5):e63627. doi: 10.1371/journal.pone.0063627
150. Lee EJ, Shaikh S, Choi D, Ahmad K, Baig MH, Lim JH, et al. Transthyretin maintains muscle homeostasis through the novel shuttle pathway of thyroid hormones during myoblast differentiation. Cells (2019) 8(12):1565. doi: 10.3390/cells8121565
151. Hao S, Sun S, Xiao X, He D, Liu L. Selective expression of transthyretin in subtypes of lung cancer. J Mol Histol (2016) 47(3):239–47. doi: 10.1007/s10735-016-9666-3
152. Lee CC, Ding X, Zhao T, Wu L, Perkins S, Du H, et al. Transthyretin stimulates tumor growth through regulation of tumor, immune, and endothelial cells. J Immunol (2019) 202(3):991–1002. doi: 10.4049/jimmunol.1800736
153. Wells JM, Strickland S. Regulated localization confers multiple functions on the protease urokinase plasminogen activator. J Cell Physiol (1997) 171(2):217–25. doi: 10.1002/(SICI)1097-4652(199705)171:2<217::AID-JCP13>3.0.CO;2-A
154. Bolon I, Devouassoux M, Robert C, Moro D, Brambilla C, Brambilla E.. Expression of urokinase-type plasminogen activator, stromelysin 1, stromelysin 3, and matrilysin genes in lung carcinomas. Am J Pathol (1997) 150(5):1619–29.
155. Zhu C, Jiang L, Xu J, Ren A, Ju F, Shu Y. The urokinase-type plasminogen activator and inhibitors in resectable lung adenocarcinoma. Pathol Res Pract (2020) 216(4):152885. doi: 10.1016/j.prp.2020.152885
156. Seiffert D, Crain K, Wagner NV, Loskutoff DJ. Vitronectin gene expression in vivo. Evidence for extrahepatic synthesis and acute phase regulation. J Biol Chem (1994) 269(31):19836–42.
157. Tomasini-Johansson BR, Sundberg C, Lindmark G, Gailit JO, Rubin K. Vitronectin in colorectal adenocarcinoma–synthesis by stromal cells in culture. Exp Cell Res (1994) 214(1):303–12. doi: 10.1006/excr.1994.1262
158. Löhr M, Trautmann B, Göttler M, Peters S, Zauner I, Maillet B, et al. Human ductal adenocarcinomas of the pancreas express extracellular matrix proteins. Br J Cancer (1994) 69(1):144–51. doi: 10.1038/bjc.1994.24
159. Falconer JS, Fearon KC, Plester CE, Ross JA, Carter DC. Cytokines, the acute-phase response, and resting energy expenditure in cachectic patients with pancreatic cancer. Ann Surg (1994) 219(4):325–31. doi: 10.1097/00000658-199404000-00001
160. Lin Y, Rajala MW, Berger JP, Moller DE, Barzilai N, Scherer PE. Hyperglycemia-induced production of acute phase reactants in adipose tissue. J Biol Chem (2001) 276(45):42077–83. doi: 10.1074/jbc.M107101200
161. Castell JV, Gómez-Lechón MJ, David M, Andus T, Geiger T, Trullenque R, et al. Interleukin-6 is the major regulator of acute phase protein synthesis in adult human hepatocytes. FEBS Lett (1989) 242(2):237–9. doi: 10.1016/0014-5793(89)80476-4
162. Baumann H, Gauldie J. The acute phase response. Immunol Today (1994) 15(2):74–80. doi: 10.1016/0167-5699(94)90137-6
163. Gulhar R, Ashraf MA, Jialal I. Physiology, acute phase reactants. Treasure Island, FL: StatPearls. (2023).
164. Rupert JE, Narasimhan A, Jengelley DHA, Jiang Y, Liu J, Au E, et al. Tumor-derived IL-6 and trans-signaling among tumor, fat, and muscle mediate pancreatic cancer cachexia. J Exp Med (2021) 218(6). doi: 10.1084/jem.20190450
165. Maccio A, Sanna E, Neri M, Oppi S, Madeddu C. Cachexia as evidence of the mechanisms of resistance and tolerance during the evolution of cancer disease. Int J Mol Sci (2021) 22(6):2890. doi: 10.3390/ijms22062890
166. McGovern J, Dolan RD, Skipworth RJ, Laird BJ, McMillan DC. Cancer cachexia: a nutritional or a systemic inflammatory syndrome? Br J Cancer (2022) 127(3):379–82. doi: 10.1038/s41416-022-01826-2
167. Silva GAD, Wiegert EVM, Calixto-Lima L, Oliveira LC. Clinical utility of the modified Glasgow Prognostic Score to classify cachexia in patients with advanced cancer in palliative care. Clin Nutr (2020) 39(5):1587–92. doi: 10.1016/j.clnu.2019.07.002
168. Narasimhan A, Zhong X, Au EP, Ceppa EP, Nakeeb A, House MG. Profiling of adipose and skeletal muscle in human pancreatic cancer cachexia reveals distinct gene profiles with convergent pathways. Cancers (Basel) (2021) 13(8):1975. doi: 10.3390/cancers13081975
169. Brantly M, Nukiwa T, Crystal RG. Molecular basis of alpha-1-antitrypsin deficiency. Am J Med (1988) 84(6A):13–31. doi: 10.1016/S0002-9343(88)80066-4
170. Sarma JV, Ward PA. The complement system. Cell Tissue Res (2011) 343(1):227–35. doi: 10.1007/s00441-010-1034-0
171. Nesargikar PN, Spiller B, Chavez R. The complement system: history, pathways, cascade and inhibitors. Eur J Microbiol Immunol (Bp) (2012) 2(2):103–11. doi: 10.1556/EuJMI.2.2012.2.2
172. Shimazaki R, Takano S, Satoh M, Takada M, Miyahara Y, Sasaki K. Complement factor B regulates cellular senescence and is associated with poor prognosis in pancreatic cancer. Cell Oncol (Dordr) (2021) 44(4):937–50. doi: 10.1007/s13402-021-00614-z
173. Du Clos TW. Function of C-reactive protein. Ann Med (2000) 32(4):274–8. doi: 10.3109/07853890009011772
174. Sproston NR, Ashworth JJ. Role of C-reactive protein at sites of inflammation and infection. Front Immunol (2018) 9:754. doi: 10.3389/fimmu.2018.00754
175. Wahlin-Larsson B, Carnac G, Kadi F. The influence of systemic inflammation on skeletal muscle in physically active elderly women. Age (Dordr) (2014) 36(5):9718. doi: 10.1007/s11357-014-9718-0
176. Krzystek-Korpacka M, Matusiewicz M, Diakowska D, Grabowski K, Blachut K, Kustrzeba-Wojcicka I. Acute-phase response proteins are related to cachexia and accelerated angiogenesis in gastroesophageal cancers. Clin Chem Lab Med (2008) 46(3):359–64. doi: 10.1515/CCLM.2008.089
177. Kemik O, Sumer A, Kemik AS, Hasirci I, Purisa S, Dulger AC, et al. The relationship among acute-phase response proteins, cytokines and hormones in cachectic patients with colon cancer. World J Surg Oncol (2010) 8:85. doi: 10.1186/1477-7819-8-85
178. MacDonald N. Terminology in cancer cachexia: importance and status. Curr Opin Clin Nutr Metab Care (2012) 15(3):220–5. doi: 10.1097/MCO.0b013e328352a895
179. Kotla NK, Dutta P, Parimi S, Das NK. The role of ferritin in health and disease: recent advances and understandings. Metabolites (2022) 12(7):609. doi: 10.3390/metabo12070609
180. Weiss G, Ganz T, Goodnough LT. Anemia of inflammation. Blood (2019) 133(1):40–50. doi: 10.1182/blood-2018-06-856500
181. Tuomisto AE, Mäkinen MJ, Väyrynen JP. Systemic inflammation in colorectal cancer: Underlying factors, effects, and prognostic significance. World J Gastroenterol (2019) 25(31):4383–404. doi: 10.3748/wjg.v25.i31.4383
182. Patel S, Patel JB. Epoetin alfa. In: StatPearls. Treasure Island, FL: StatPearls Publishing (2023).
183. Budzynski AZ. Fibrinogen and fibrin: biochemistry and pathophysiology. Crit Rev Oncol Hematol (1986) 6(2):97–146. doi: 10.1016/S1040-8428(86)80019-1
184. Nguyen MD, Simpson-Haidaris PJ. Cell type-specific regulation of fibrinogen expression in lung epithelial cells by dexamethasone and interleukin-1beta. Am J Respir Cell Mol Biol (2000) 22(2):209–17. doi: 10.1165/ajrcmb.22.2.3746
185. Simpson-Haidaris PJ, Rybarczyk B. Tumors and fibrinogen. The role of fibrinogen as an extracellular matrix protein. Ann N Y Acad Sci (2001) 936:406–25. doi: 10.1111/j.1749-6632.2001.tb03525.x
186. O’Keefe SJ, Ogden SJ, Ramjee J, Rund GJ. Contribution of elevated protein turnover and anorexia to cachexia in patients with hepatocellular carcinoma. Cancer Res (1990) 50(4):1226–30.
187. Preston T, Slater C, McMillan DC, Falconer JS, Shenkin A, Fearon KC. Fibrinogen synthesis is elevated in fasting cancer patients with an acute phase response. J Nutr (1998) 128(8):1355–60. doi: 10.1093/jn/128.8.1355
188. Reddel CJ, Allen JD, Ehteda A, Taylor R, Chen VM, Curnow JL, et al. Increased thrombin generation in a mouse model of cancer cachexia is partially interleukin-6 dependent. J Thromb Haemost (2017) 15(3):477–86. doi: 10.1111/jth.13612
189. Proctor RA. Fibronectin: a brief overview of its structure, function, and physiology. Rev Infect Dis (1987) 9 Suppl 4:S317–21. doi: 10.1093/clinids/9.Supplement_4.S317
190. Lin TC, Yang CH, Cheng LH, Chang WT, Lin YR, Cheng HC. Fibronectin in cancer: friend or foe. Cells (2019) 9(1):27. doi: 10.3390/cells9010027
191. Mohiuddin E, Wakimoto H. Extracellular matrix in glioblastoma: opportunities for emerging therapeutic approaches. Am J Cancer Res (2021) 11(8):3742–54.
192. di Masi A, De Simone G, Ciaccio C, D'Orso S, Coletta M, Ascenzi P. Haptoglobin: From hemoglobin scavenging to human health. Mol Aspects Med (2020) 73:100851. doi: 10.1016/j.mam.2020.100851
193. Smith A, McCulloh RJ. Hemopexin and haptoglobin: allies against heme toxicity from hemoglobin not contenders. Front Physiol (2015) 6:187. doi: 10.3389/fphys.2015.00187
194. Arend WP, Malyak M, Guthridge CJ, Gabay C. Interleukin-1 receptor antagonist: role in biology. Annu Rev Immunol (1998) 16:27–55. doi: 10.1146/annurev.immunol.16.1.27
195. Steinkasserer A, Estaller C, Weiss EH, Sim RB. Human interleukin-1 receptor antagonist is expressed in liver. FEBS Lett (1992) 310(1):60–2. doi: 10.1016/0014-5793(92)81146-D
196. Cesari M, Pahor M, Incalzi RA. Plasminogen activator inhibitor-1 (PAI-1): a key factor linking fibrinolysis and age-related subclinical and clinical conditions. Cardiovasc Ther (2010) 28(5):e72–91. doi: 10.1111/j.1755-5922.2010.00171.x
197. Kucharewicz I, Kowal K, Buczko W, Bodzenta-Łukaszyk A. The plasmin system in airway remodeling. Thromb Res (2003) 112(1-2):1–7. doi: 10.1016/j.thromres.2003.10.011
199. Ward ES, Gelinas D, Dreesen E, Van Santbergen J, Andersen JT, Silvestri NJ, et al. Clinical significance of serum albumin and implications of fcRn inhibitor treatment in igG-mediated autoimmune disorders. Front Immunol (2022) 13:892534. doi: 10.3389/fimmu.2022.892534
200. Wagatsuma A, Fujimoto K, Yamada S. Alteration in albumin level during modified muscular activity. Scand J Med Sci Sports (2002) 12(3):143–9. doi: 10.1034/j.1600-0838.2002.120304.x
201. Liu XY, Zhang X, Ruan GT, Zhang KP, Tang M, Zhang Q, et al. One-year mortality in patients with cancer cachexia: association with albumin and total protein. Cancer Manag Res (2021) 13:6775–83. doi: 10.2147/CMAR.S318728
202. Kaaks R, Lukanova A. Energy balance and cancer: the role of insulin and insulin-like growth factor-I. Proc Nutr Soc (2001) 60(1):91–106. doi: 10.1079/PNS200070
203. Schulze PC, Spate U. Insulin-like growth factor-1 and muscle wasting in chronic heart failure. Int J Biochem Cell Biol (2005) 37(10):2023–35. doi: 10.1016/j.biocel.2005.04.017
204. Deboer MD. Animal models of anorexia and cachexia. Expert Opin Drug Discovery (2009) 4(11):1145–55. doi: 10.1517/17460440903300842
Keywords: cachexia, cancer, acute phase response, acute phase reactant, positive acute phase reactant, negative acute phase reactant, chronic disease
Citation: Robinson TP, Hamidi T, Counts B, Guttridge DC, Ostrowski MC, Zimmers TA and Koniaris LG (2023) The impact of inflammation and acute phase activation in cancer cachexia. Front. Immunol. 14:1207746. doi: 10.3389/fimmu.2023.1207746
Received: 18 April 2023; Accepted: 13 October 2023;
Published: 31 October 2023.
Edited by:
Clelia Madeddu, University of Cagliari, ItalyReviewed by:
Giamila Fantuzzi, University of Illinois Chicago, United StatesCopyright © 2023 Robinson, Hamidi, Counts, Guttridge, Ostrowski, Zimmers and Koniaris. This is an open-access article distributed under the terms of the Creative Commons Attribution License (CC BY). The use, distribution or reproduction in other forums is permitted, provided the original author(s) and the copyright owner(s) are credited and that the original publication in this journal is cited, in accordance with accepted academic practice. No use, distribution or reproduction is permitted which does not comply with these terms.
*Correspondence: Leonidas G. Koniaris, S29uaWFyaXNAT0hTVS5lZHU=
Disclaimer: All claims expressed in this article are solely those of the authors and do not necessarily represent those of their affiliated organizations, or those of the publisher, the editors and the reviewers. Any product that may be evaluated in this article or claim that may be made by its manufacturer is not guaranteed or endorsed by the publisher.
Research integrity at Frontiers
Learn more about the work of our research integrity team to safeguard the quality of each article we publish.