- 1State Key Laboratory for Diagnosis and Treatment of Infectious Diseases, National Clinical Research Center for Infectious Diseases, National Medical Center for Infectious Diseases, Collaborative Innovation Center for Diagnosis and Treatment of Infectious Diseases, The First Affiliated Hospital, Zhejiang University School of Medicine, Hangzhou, China
- 2Zhejiang University School of Medicine, Hangzhou, Zhejiang, China
RNA modification plays an important role in epigenetics at the posttranscriptional level, and 5-methylcytosine (m5C) has attracted increasing attention in recent years due to the improvement in RNA m5C site detection methods. By influencing transcription, transportation and translation, m5C modification of mRNA, tRNA, rRNA, lncRNA and other RNAs has been proven to affect gene expression and metabolism and is associated with a wide range of diseases, including malignant cancers. RNA m5C modifications also substantially impact the tumor microenvironment (TME) by targeting different groups of immune cells, including B cells, T cells, macrophages, granulocytes, NK cells, dendritic cells and mast cells. Alterations in immune cell expression, infiltration and activation are highly linked to tumor malignancy and patient prognosis. This review provides a novel and holistic examination of m5C-mediated cancer development by examining the exact mechanisms underlying the oncogenicity of m5C RNA modification and summarizing the biological effects of m5C RNA modification on tumor cells as well as immune cells. Understanding methylation-related tumorigenesis can provide useful insights for the diagnosis as well as the treatment of cancer.
Introduction
Modifications of biological macromolecules, such as DNA, RNA and proteins, are essential for life. RNA modification at the posttranscriptional level, which does not alter the genome, plays a large role in epigenetics. The first RNA modification site, pseudouridine (Ψ), was discovered in the 1950s (1), and a total of 334 types of RNA modifications have been identified since then (2). Commonly recognized RNA modification sites include N6-methyladenosine (m6A), 5-methylcytosine (m5C), 7-methylguanosine (m7G), N1-methyladenosine (m1A), N4-acetylcytidine (ac4C), N6-acetyladenosine (ac6A), pseudouridine (Ψ), uridylation, and phosphorylation (3–5). The deposition, removal and recognition of RNA modification sites are realized through three groups of responsible proteins. “Writers” and “erasers” refer to proteins capable of catalyzing the deposition and removal of a specific RNA modification site, respectively, while “readers”, sometimes also called “binders”, mainly recognize and bind to these modification sites (6, 7). Previous studies have demonstrated that RNA modification occurs not only in messenger RNA (mRNA) but also in noncoding RNAs, such as transfer RNA (tRNA), ribosomal RNA (rRNA), long noncoding RNA (lncRNA), microRNA (miRNA) and small nuclear RNA (snRNA) (8–11). For example, m6A and m5C modifications of mRNA are crucial in embryo development and stem cell fate determination (12), m7G modification of tRNA influences pathogenic infectivity of thermophilic bacteria (13), and m6A modification of lncRNA is likely to participate in the process of cell senescence (14).
Among the types of RNA modifications that have been discovered, m6A modification is the most widely and comprehensively investigated because of its abundance in eukaryotic cells (15, 16). m5C modification, comparatively, is less understood than m6A modification, as it is only moderately abundant (2, 17). A study in 2015 discovered that m5C consists of approximately 1%, 0.01% and 1% of cytosine residues in the samples extracted from mouse brain, E. coli and HEK293T (human embryonic kidney 293 T) cells (18). However, m5C has attracted increasing attention from researchers in recent years as detection methods for m5C have progressed (17, 19), showing the presence of m5C in mRNA, tRNA, rRNA and viral RNA infecting mammalian cells (20, 21). RNA sequencing methods are commonly used in the detection of m5C RNA modification, including RNA bisulphite sequencing, immunoprecipitation-based RNA sequencing and third generation sequencing (21–23). Immunoprecipitation-based RNA sequencing can be further divided into several categories, such as methylated RNA immunoprecipitation sequencing (MeRIP-Seq), 5-azacytidine-mediated RNA immunoprecipitation sequencing (5-azaIP-Seq) and methylation-individual nucleotide resolution crosslinking immunoprecipitation sequencing (miCLIP-Seq) (21, 23). Other detection methods include mass spectrometry, total base composition analysis, nearest neighbor analysis, etc. (24).
Up to now, an increasing amount of evidence has unveiled the importance of m5C in the modulation of gene expression, metabolism and diseases (25–27). Specifically, in the field of oncology, posttranscriptional RNA modification has been discovered to play important roles in the development and pathological process of various types of cancers since more than half a century ago (10, 28, 29), which introduced a promising new area of mechanistic exploration and therapeutic innovation. Alterations in m5C modifications of both coding RNAs and noncoding RNAs are also highly linked to cell proliferation (30, 31), metabolism (32) and tumor metastasis (33, 34) and appear in various kinds of cancer types, such as hepatocellular carcinoma (35), breast cancer (36) and bladder cancer (32). Moreover, m5C has vital impacts on different kinds of immune cells, including B cells, T cells, NK cells, granulocytes and macrophages (37, 38). Obviously, the m5C-associated biological changes in immune cells are not to be neglected in the process of cancer development, but there is no comprehensive summary regarding the relationship between m5C-associated tumorigenesis and alterations in immune cells.
In this review, we provide a novel and holistic review of m5C-mediated cancer development by examining the exact mechanisms underlying the oncogenicity of m5C RNA modification and summarize the biological effects of m5C RNA modification on tumor cells as well as immune cells.
The mechanism and basic biological functions of m5C RNA modification
There are three main groups of molecular effectors in the process of m5C RNA modification, namely, “writers”, “erasers” and “readers” (Table 1). “Writers” refer to proteins that facilitate the formation of methylation sites, such as DNMT2 (DNA methyltransferase homolog 2) and the NSUN (NOL1/NOP2/SUN domain) family proteins. “Readers” are related recognition proteins that bind and identify methylation sites, such as ALYREF (Aly/REF export factor) and YBX1(Y-box binding protein 1). Although they do not directly take part in catalysis, the abnormality of “readers” is often associated with metabolic disorders and diseases. “Erasers”, in contrast, facilitate the deletion of methylation sites, such as TET (ten-eleven translocation) family genes and ALKBH1 (AlkB homolog 1), creating a dynamic balance between the two antagonizing biological processes (Figures 1–3).
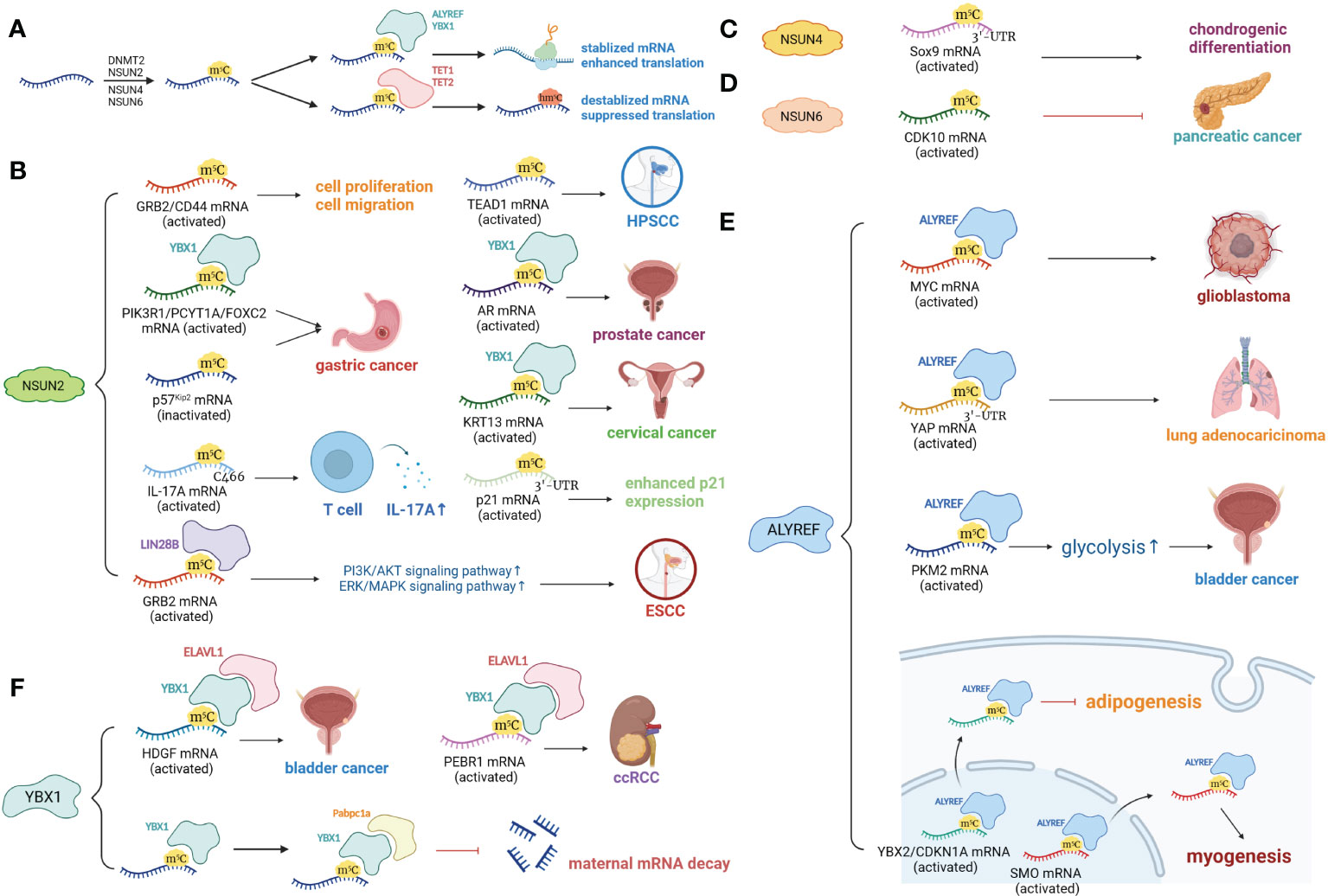
Figure 1 m5C modifications of mRNA. (A) mRNA is methylated at m5C sites by DNMT2, NSUN2, NSUN4 and NSUN6. ALYREF and YBX1 bind and stabilize m5C-modulated mRNAs. TET1, TET2 and ALKBH1 remove m5C sites by turning them into hm5C. (B) Target mRNAs of NSUN2 include GRB2, CD44, PIK3R1, PCYTIA, FOXC2, p57Kip2, TEAD1, AR, KRT13, IL-17A, and p21. Most target mRNAs are stabilized due to m5C modification, with the exception of p57Kip2, and are associated with enhanced cell proliferation and migration. (C) NSUN4-mediated m5C at the 3’-UTR of Sox9 mRNA promotes chondrogenic differentiation. (D) NSUN6-mediated m5C modification suppresses pancreatic cancer by promoting tumor-suppressive CDK10. (E) ALYREF binds with MYC, YAP, PKM2 and NEAT1 lncRNA, promoting tumor development and drug resistance. ALYREF facilitates nuclear export of YBX2, CDKN1A and SMO mRNAs, inhibiting adipogenesis and enhancing myogenesis. (F) YBX1 binds to m5C sites on the FOXC2, HDGF, PEBRQ, AR and KRT13 mRNAs, leading to enhanced mRNA translation and promoting tumorigenesis. YBX1 recognizes and stabilizes m5C-modified mRNAs by recruiting Pabpc1a, facilitating maternal-to-zygotic transition.
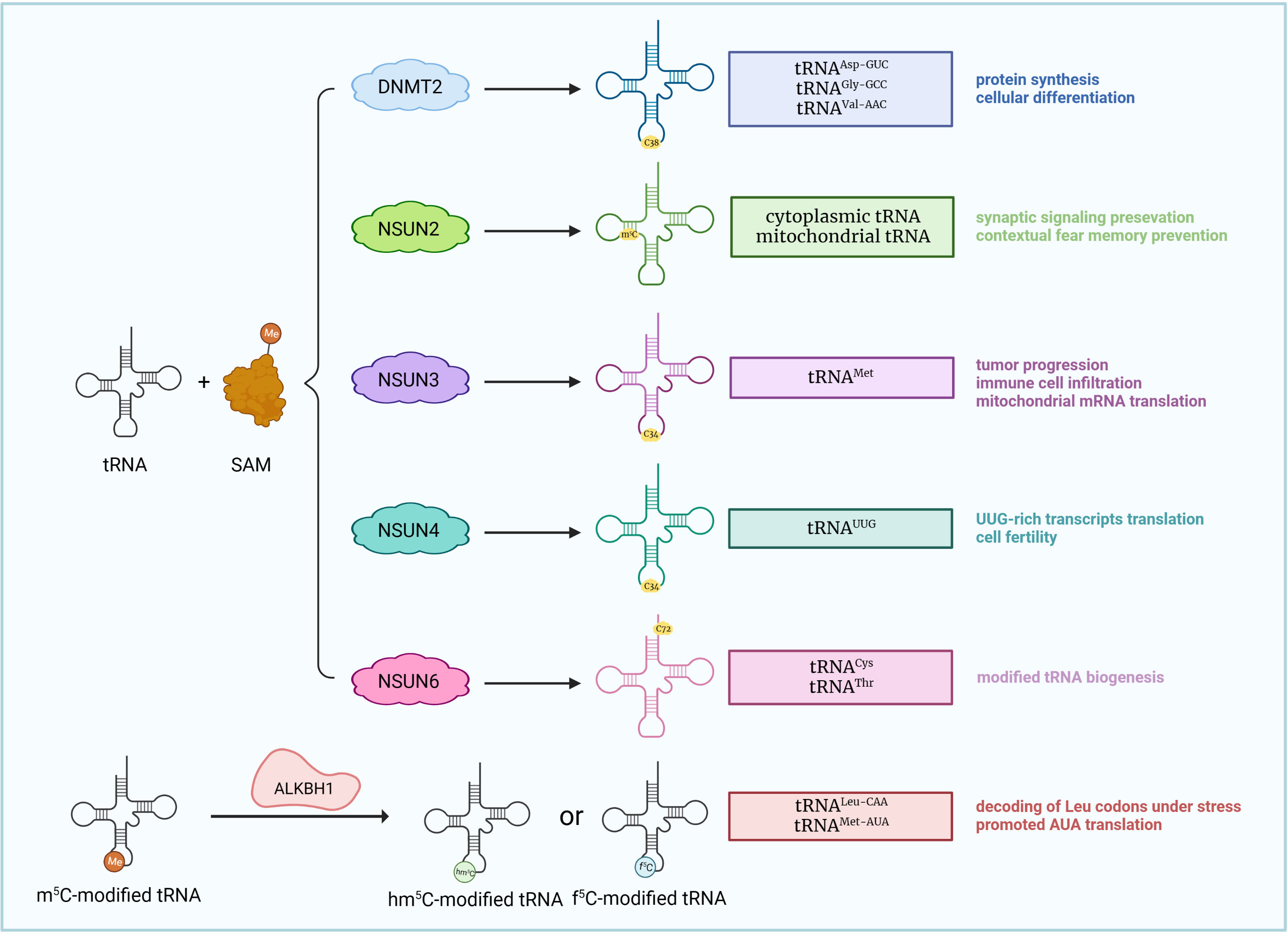
Figure 2 m5C modifications of tRNA. SAM induces tRNA m5C modification. DNMT2, NSUN2-4, and NSUN6 catalyze m5C modification of various tRNAs at different sites, causing distinct biological effects. ALKBH1 removes m5C sites from tRNALeu-CAA and converts them into f5C (5-formylcytidine) sites, promoting the decoding of Leu codons under stress.
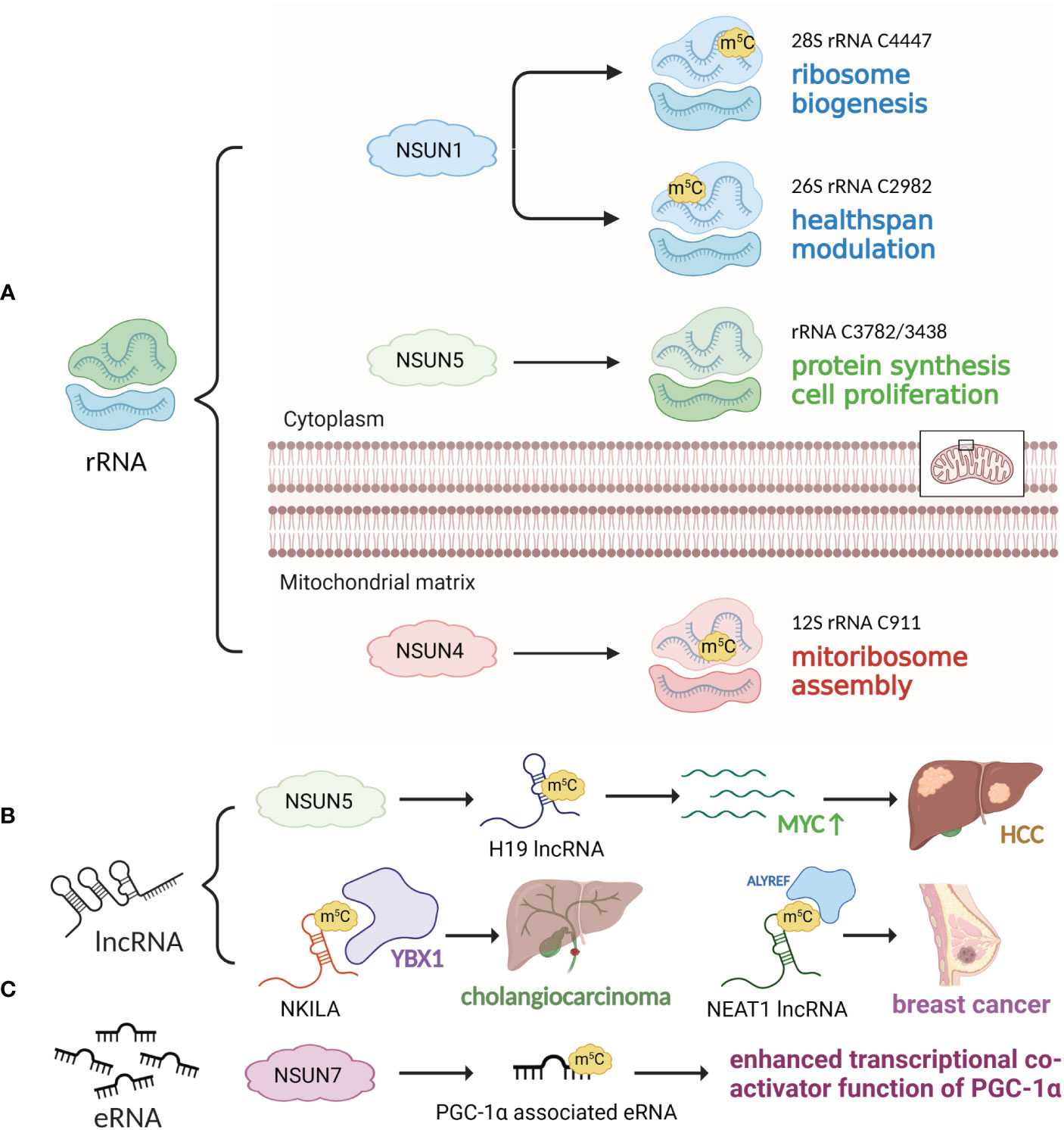
Figure 3 m5C modifications of rRNA, lncRNA and eRNA. (A) rRNA m5C modification is mediated by NSUN1, NSUN4 and NSUN5, facilitating ribosome biogenesis, healthspan modulation, mitoribosomal assembly, protein synthesis and cell proliferation. (B) NSUN2 catalyzes m5C modification of H19 lncRNA, which stimulates MYC expression and HCC development. YBX1 recognizes and stabilizes m5C-modified NKILA, promoting cholangiocarcinoma development. (C) NSUN7 m5C-methylates eRNA associated with PGC-1α, promoting its transcriptional coactivator function.
Writers
To date, the m5C methylation of RNA, including mRNA, rRNA and tRNA, is believed to be mainly mediated by two groups of RNA methyltransferases, DNMT2 and the NSUN protein family.
DNMT2, also known as TRDMT1 (tRNA methyltransferase 1), generally influences tRNA methylation (25). The m5C site is located on cytosine 38 in the anticodon loop of tRNAAsp-GUC, tRNAGly-GCC, tRNAVal-AAC (39, 40), and receives a methyl group from the cofactor S-adenosyl-methionine (SAM) (115). Studies showed that simultaneous knockout of DNMT2 and NSUN2 led to deficient tRNA methylation, protein synthesis and cellular differentiation, causing the death of experimental mice, although deficiency of either DNMT2 or NSUN2 alone did not show detectable effects. The results suggested that DNMT2 plays a role in tRNA methylation and cell survival (41). In addition, the role of DNMT2 in mRNA methylation and expression modulation was also reported. DNMT2 deficiency is associated with alterations in mRNA expression and methylation profiles and the inhibition of cell proliferation and migration (42).
The NSUN family also utilizes SAM as a methyl donor (116). The NSUN family consists of seven members, NSUN1-7, and each target different types of RNAs. The RNA targeting specificity of the NSUN family was reviewed in 2019 by Katherine E. Bohnsack et al., with NSUN1, 4, and 5 responsible for rRNA methylation, NSUN2, 3, and 6 responsible for tRNA methylation (NSUN2 also promotes mRNA methylation), and NUSN7 responsible for enhancer RNA (eRNA) methylation (25). However, within the last three years, studies have provided new insights in this regard. Among the seven RNA methyltransferases, NSUN2 was the first discovered and most widely studied. The role of NSUN2 in promoting tRNA methylation is wellknown, and NSUN2 deficiency directly causes a decrease in tRNA m5C levels (47). Notably, NSUN2 is predominantly distributed in the nucleus and catalyzes methylation of cytoplasmic tRNA; it is also capable of introducing m5C to mitochondrial tRNA (48, 49). However, NSUN2 silencing did not significantly affect mitochondrial tRNA stability (49), which suggested that NSUN2 may not be necessary for tRNA methylation within the mitochondria. NSUN2 is also responsible for biological processes, including cell proliferation (50) and carcinogenesis. Enhanced levels of NSUN2 and NSUN2-mediated m5C are observed in patients with gastric cancer (GC) (30, 34, 51), esophageal squamous cell carcinoma (ESCC) (52, 53), hepatocellular carcinoma (HCC) (35, 54), hypopharyngeal squamous cell carcinoma (HPSCC) (55), prostate cancer (56), cervical cancer (57), nasopharyngeal carcinoma (58) and uveal melanoma (59). NSUN2 also affects immune cells, as hyperexpression of NSUN2 in T cells promotes IL-17A secretion by methylating IL-17A mRNA at cytosine C466 both in vitro and in vivo, which stimulates its translation (60). In addition, under conditions of oxidative stress-induced cellular senescence, NSUN2-mediated m5C, together with METTL3/METTL14-mediated m6A, synergistically upregulates the expression of p21 (61). Note that NSUN2 is also involved in the functioning of m5C readers, including ALYREF, whose nuclear-cytoplasm transportation is partly modulated by NSUN2 (62). Other members of the NSUN family are also active in catalyzing m5C RNA modifications. NSUN1, or NOP2 (nucleolar protein 2), catalyzes rRNA m5C modifications, thus affecting biological processes including ribosome biogenesis (43), cell proliferation (44), healthspan modulation (45) and HIV-1 viral latency (46). NSUN3, a putative tRNA methyltransferase, plays a role in tumor progression (27, 63, 64), immune cell infiltration (64, 66) and multisystem mitochondrial diseases (67). Mechanistically, m5C modification of tRNA occurs at C34 in the anticodon loop (65, 117). Current studies have revealed that NSUN3 expression is upregulated in patients with low-grade glioma (63) and head and neck squamous cell carcinoma (HNSCC) (64), and NSUN3-mediated m5C modification of tRNA enhances metastasis by stimulating the translation of mitochondrial mRNA (27). NSUN3-associated immune cell infiltration mainly includes CD8+ T cells (66) and M2 macrophages (64). Deficiency of NSUN3 also leads to severe dysfunction within the mitochondria, such as combined oxidative phosphorylation deficiency, which may lead to early-onset encephalomyopathy and seizures (67). NSUN4 facilitates mitoribosomal assembly by methylating C911 in 12S rRNA and interacting with MTERF4 (mitochondrial transcription termination factor 4) (68). In addition to rRNA methylating activity, NSUN4 also acts as a tRNA (69) and mRNA (70) methyltransferase, and NSUN4-mediated m5C modification in the 3’-UTR (3’-untranslated region) of SOX9 (SRY-box transcription factor 9) mRNA is necessary for adaptation to higher temperatures (69) and chondrogenic differentiation regulated by SOX9 (70). NSUN4 also promotes HCC generation (71, 72) and neutrophil infiltration (66). NSUN5 participates in rRNA methylation, introducing m5C3782 into human and m5C3438 into mouse 28S rRNA (73, 74). Overexpression of NSUN5 is associated with tumorigenesis in HCC (75) and colorectal cancer (CRC) patients (76), while NSUN5 deficiency causes a reduction in total protein synthesis, thus impairing cell proliferation (73). In patients with tetralogy of Fallot (TOF) (118) and William’s-Beuren syndrome (WBS) (119), NSUN5 is drastically downregulated. Previous studies regarded NSUN6 as at RNA methyltransferase, which identifies C72 at the 3′ end of the tRNA acceptor stem and targets tRNACys and tRNAThr (77, 78), but recent investigations have discovered that NSUN6 exhibits mRNA methylating bioactivity (79, 82, 120, 121). mRNA methylated by NSUN6, which primarily targets the 3’-UTR at the consensus sequence motif CTCCA, increased in transcript and protein levels (79). The role of NSUN6 in cancer development remains unclear, but studies have shown that NSUN6 acts as a protective factor against triple-negative breast cancer (TNBC) (36), pancreatic cancer (31), testis cancer (79), thyroid cancer (79) and ovary cancer (79) but is a risk factor for CRC (80, 81). One possible explanation of the controversial role of NSUN6 in different types of cancers is that NSUN6 expression level in different immune cells within the TME differs based on the tumor context. For instance, NSUN6 is mainly expressed in Tregs in TNBC (36), but in exhausted CD8+ T cells, proliferating T cells and myofibroblasts in CRC (80). In addition, NSUN6 is also related to the promotion of the cell cycle (82), infiltration of B cells, CD4+ T cells and CD8+ T cells (36, 80), and formation of antibody-secreting plasma cells (83). NSUN7 methylates eRNA, a noncoding RNA associated with transcription modulation, and enhances the expression of mRNAs coding for Pfkl, Sirt5, Idh3b and Hmox2 in a peroxisome proliferator-activated receptor-gamma coactivator 1 alpha (PGC-1α)-dependent manner. These effects are likely to facilitate adaptive metabolic alterations under starvation (84).
Readers
Readers, or binding proteins of m5C sites, include ALYREF and YBX1. ALYREF is reported to be an important oncogenic factor and is associated with poor prognosis in patients with various types of cancer, including HCC (72, 85, 86), glioblastoma (87, 88), glioma (63), neuroblastoma (89), lung adenocarcinoma (90, 91), HNSCC (92, 93), bladder cancer (32) and breast cancer (94, 95). For example, elevated levels of ALYREF in HCC patients were found to be responsible for upregulated eIF4A3 expression (86) and abnormal cell cycle and mitosis (72). In lung adenocarcinoma patients, ALYREF, together with NSUN2, promotes m5C modification of YAP (Yes-Associated Protein) mRNA in the 3’-UTR, thus increasing the stability of YAP mRNA and causing enhanced exosome secretion, tumor malignancy and drug resistance (91). In contrast, ALYREF is considered a protective factor against colon adenocarcinoma (81), but the mechanism remains to be elucidated. In addition, ALYREF also participates in the regulation of adipogenesis (96), myogenesis (96, 97) and retrovirus replication (98) and may have biological activity in the context of abdominal aortic aneurysm (AAA) (99).
YBX1 is another m5C reader that has multiple functions in cancer development and embryo development. Oncogenic effects of YBX1 are found in GC (51), bladder cancer (100), glioblastoma (101), CRC (76, 102), cholangiocarcinoma (103), clear cell renal cell carcinoma (ccRCC) (104), prostate cancer (56), epithelial ovarian cancer (105) and cervical cancer (57), mainly owing to YBX1-RNA interactions that have stabilizing effects on target RNAs. For example, in GC patients, YBX1 binds with FOXC2 (Forkhead box protein C2) mRNA, which is m5C-modulated by NSUN2, to enhance its tumor-promoting ability (51). In bladder cancer patients, YBX1 stabilizes oncogenic HDGF (heparin binding growth factor) mRNA by targeting the m5C-modified site on its 3′-UTR (100). Interestingly, in addition to its oncogenic effects, YBX1 is also important in normal cell proliferation and embryo development. For instance, animal experiments revealed that YBX1 is essential for embryonic brain development in mice (101), and YBX1 deficiency causes early gastrulation defects in zebrafish embryos (106).
Erasers
Currently discovered m5C demethyltransferases are the TET family and ALKBH1. The TET family is a group of Fe(II) and alpha-ketoglutarate-dependent m5C dioxygenases that convert 5-methylcytosine (m5C) to 5-hydroxymethylcytosine (hm5C) (122). These enzymes were originally discovered in the translocation breakpoint of t(10;11) in patients with infant acute myeloid leukemia (AML), hence the name (123). Overall, the scarcity of research focusing on m5C erasers limits the comprehensive understanding of these proteins. TET1-mediated mRNA m5C demethylation is essential for completion of DNA repair and survival of cells in the context of DNA damage (107). TET2 mainly facilitates the conversion of m5C into hm5C, thus leading to the elimination of m5C modification in RNA (108, 109), but the effects are not as strong as ALKBH1 (109). TET2 expression was measured in patients with various types of cancers, and it was upregulated in low-grade glioma patients (63) but downregulated in ccRCC (110, 111), ovarian cancer (112) and prostate adenocarcinoma (113) patients. Note that the potential tumor-suppressive effect of TET2 in prostate adenocarcinoma is likely to be linked with enhanced immune cell infiltration (113). TET3-mediated m5C elimination has not been clarified to date. However, some researchers have reported that upregulated TET3 expression in prostate cancer patients might be associated with poor prognosis (38).
ALKBH1 has been identified as a demethyltransferase for both RNA and DNA (124), but most studies focused on ALKBH1-demethylated DNA modifications, with fewer researchers concentrating on the RNA part. So far, studies have revealed that ALKBH1 takes part in the transformation of m5C RNA modifications to either hm5C or f5C (5-formylcytidine) RNA modifications (109, 114). More specifically, in cytoplasmic tRNA, ALKBH1 targets the wobble position (position 34) of tRNALeu-CAA and converts m5C RNA modifications to hm5C or f5C, promoting the decoding of Leu codons under stress (109, 114). At the same position (position 34) of mitochondrial tRNAMet, only the alteration from m5C to f5C was found, which was proved indispensable for the translation of AUA, a non-universal codon in mammalian mitochondria, indicating that ALKBH1-mediated m5C RNA modification removal is significant for mammalian mitochondrial functions (114). Interestingly, an in vitro experiment showed that ALKBH1 first hydroxylates m5C to form hm5C, and then oxidizes hm5C to form f5C, meaning ALKBH1-mediated biogenesis of hm5C and f5C is actually two relevant and coherent processes (114).
m5C RNA modification in cancer cells
As discussed above, RNA m5C modification has been discovered to be an important biological process in many types of diseases, including cancer. Dysfunction or alterations in the expression levels of m5C writers, readers and erasers influence tumor development, malignancy and metastasis by changing both mRNA and noncoding RNAs at the expressional and transcriptional levels (Figure 4). Here, we present a detailed overview of m5C-mediated alterations within tumor cells that have been clarified to date (Table 2). Understanding the molecular mechanisms of m5C-mediated tumorigenesis is vastly important for increasing the therapeutic efficiency of antitumor treatments.
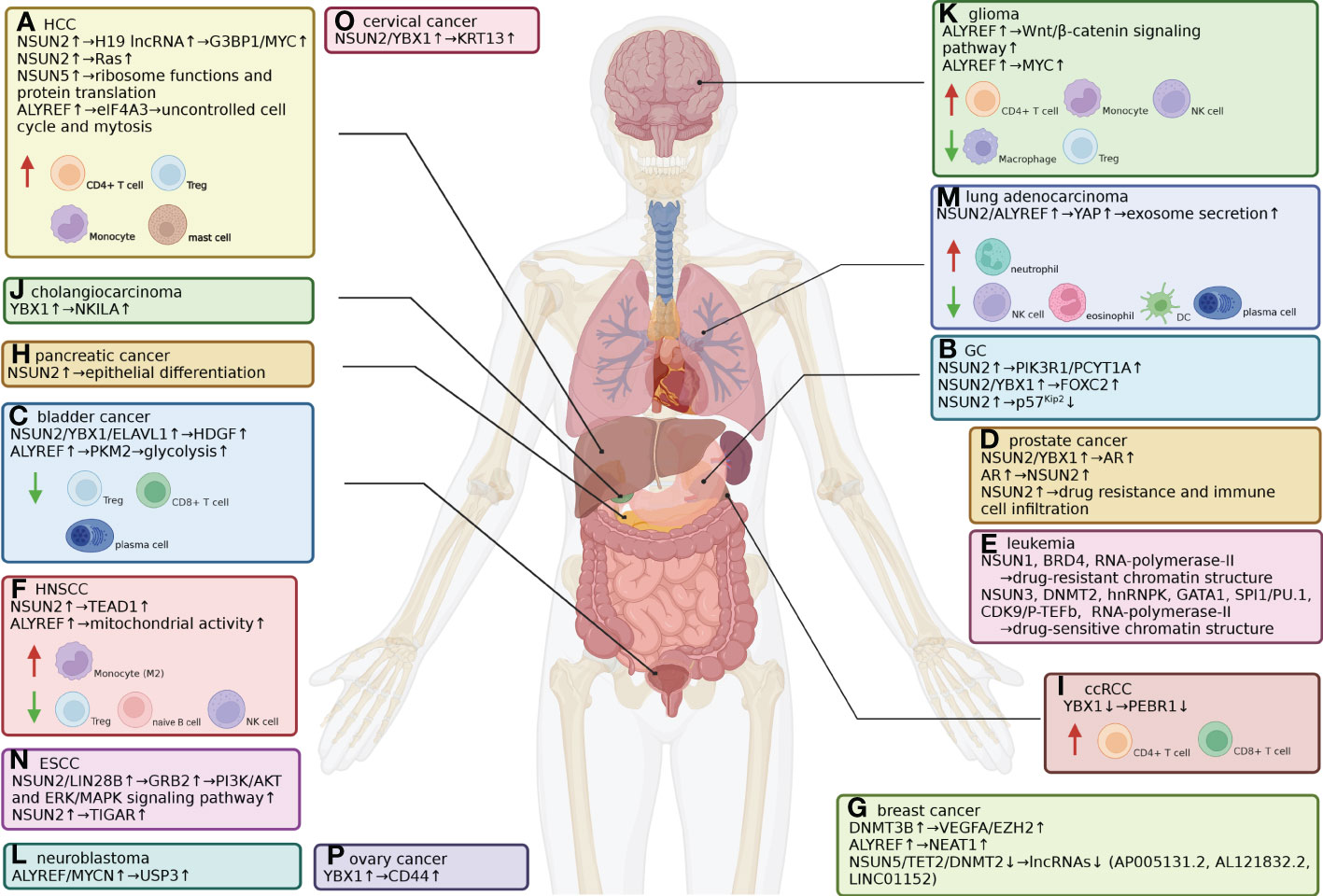
Figure 4 Expression of m5C-related genes and immune cell infiltration in different cancer types. (A) HCC. NSUN2-mediated m5C modulation of H19 lncRNA increases its stability, leading to enhanced recruitment of G3BP1 and MYC. NSUN2 also promotes HCC progression by modulating the Ras signaling pathway, the cell cycle and drug resistance. NSUN5 facilitates ribosomal functions and protein translation. ALYREF upregulates eIF4A3 expression, which leads to uncontrolled mitosis. The abundance of CD4+ T cells (including Tregs), M0, M1 and M2 macrophages and resting mast cells is higher in HCC tissues from patients with poor prognoses. (B) GC. NSUN2 methylates PIK3R1 and PCYT1A mRNA, stabilizing them and activating downstream cancerous signaling pathways. NSUN2 methylates FOXC2 mRNA, enhancing its interaction with the m5C reader YBX1. NSUN2 destabilizes tumor-suppressive p57Kip2 mRNA by m5C-methylation in its 3’-UTR. (C) Bladder cancer. ALYREF binds to the 3’-UTR of PKM2 mRNA, stabilizing it and enhancing PKM2-mediated glycolysis. NSUN2 mediates m5C modification in the 3’-UTR of oncogenic HDGF mRNA; YBX1 recruits ELAVL1 to form a m5C-binding complex to stabilize HDGF mRNA. Lower Treg, CD8+ T-cell and plasma cell infiltration rates indicate poor prognosis. (D) Prostate cancer. NSUN2 catalyzes and YBX1 recognizes m5C modification sites on androgen receptor (AR) mRNA, and AR positively regulates NSUN2 transcription in return. NSUN2 expression also leads to drug resistance and immune cell infiltration. (E) Leukemia. NSUN1 forms an active drug-resistant chromatin structure with BRD4 and RNA polymerase-II, while SUN3 and DNMT2 form a drug-sensitive structure with hnRNPK, GATA1, SPI1/PU.1, and CDK9/P-TEFb to recruit RNA polymerase-II. (F) HNSCC. NSUN2 promotes HNSCC by suppressing immune infiltration and methylates and stabilizes TEAD1 mRNA. ALYREF increases mitochondrial activity to ensure tumor cells are supplied with energy. Lower Treg, naïve B-cell and NK cell infiltration indicates poor prognosis, while higher M2 macrophage infiltration indicates poor prognosis. (G) Breast cancer. ALYREF promotes breast cancer by enhancing the transcription of NEAT1 lncRNA. DNMT3B targets VEGFA and EZH2 as tumor promoters. NSUN5, TET2 and DNMT2 exert inhibitory effects on breast cancer by modifying three lncRNAs. (H) Pancreatic cancer. NSUN2 promotes pancreatic cancer and epithelial differentiation. (I) ccRCC. YBX1 negatively modulates ccRCC by binding and stabilizing PEBR1 mRNA. The abundance of CD4+ T cells and CD8+ T cells was higher in ccRCC tissues. (J) Cholangiocarcinoma. YBX1 promotes tumor development by stabilizing m5C-methylated NKILA. (K) Glioma. ALYREF activates the Wnt/β-catenin signaling pathway and stabilizes MYC mRNA, promoting the development of glioblastoma, a malignant type of glioma. The infiltration of CD4+ T cells, monocytes and NK cells decreases in glioma tissues, while macrophage and Treg infiltration increases. (L) Neuroblastoma. ALYREF forms a nuclear coactivator complex with MYCN to stimulate USP3 transcription, which promotes tumorigenesis. (M) Lung adenocarcinoma. SUN2 and ALYREF increase YAP mRNA stability, thus enhancing exosome secretion, tumor malignancy and drug resistance. Lower plasma cell, eosinophil, NK cell and DC infiltration rates and higher neutrophil infiltration rates indicate poor prognosis. (N) ESCC. NSUN2 methylates GRB2 in a LIN28B-dependent manner, thus activating the PI3K/AKT and ERK/MAPK signaling pathways. NSUN2 also promotes TIGAR to enhance tumor growth. (O) Cervical cancer. NSUN2 and YBX1 promote cervical cancer by increasing the expression levels of KRT13 mRNA. (P) Ovarian cancer. YBX1 modulates CD44 expression to enhance chemoresistance.
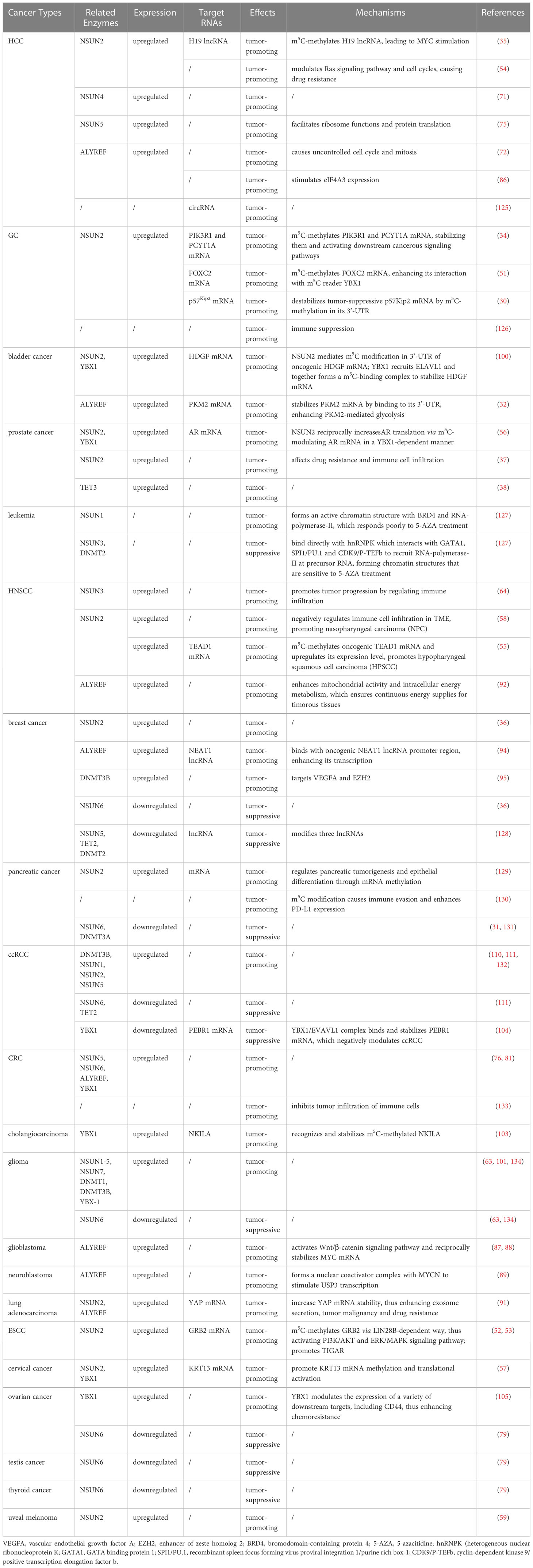
Table 2 Expression of m5C-related genes and tumor-promoting/suppressing mechanisms in different types of tumors.
Hepatocellular carcinoma (HCC)
Previous studies have demonstrated a clear relationship between high m5C levels and HCC development, migration and malignancy. Recent studies have mainly focused on lncRNAs. For example, during HCC, the expression levels of m5C-associated genes, including NSUN2 (35, 54), NSUN4 (71), NSUN5 (75) and ALYREF (86), increase. NSUN2-mediated m5C modulation of H19 lncRNA increases its stability, leading to enhanced recruitment of the G3BP1 (Ras-GTPase-activating protein-binding protein 1) oncoprotein, a potential enhancer of MYC accumulation (35). NSUN2 also modulates the Ras signaling pathway as well as the cell cycle, thus allowing for tumor escape from chemotherapy (54). A bioinformatics analysis discovered that NSUN5 overexpression was positively associated with enhanced ribosome functions and protein translation within HCC cells (75). ALYREF dysfunction is responsible for aberrant cell cycle regulation and mitosis of HCC cells (72) and promotes HCC possibly via stimulation of eIF4A3 expression (86). Thus, suppressors for ALYREF and eIF4A3, such as miR-4666a-5p and miR-6124, are promising therapeutic agents (86). Moreover, m5C modulation of circRNA is also important in HCC development (125). In addition, alterations in the tumor microenvironment (TME) and immune cell infiltration also contribute to m5C-mediated HCC development (135, 136). Currently, researchers are investigating new methods for prognosis prediction in HCC patients and constructed speculating models based on m5C-related modulators, such as the NSUN family, TET1, and YBX1 (72, 137, 138). These findings may have profound clinical implications.
Gastric cancer (GC)
The role of m5C RNA modification in GC is generally oncogenic, with high levels of m5C indicating poor prognosis and a low overall survival (OS) rate (126). Modifications of both mRNA (30, 34, 51) and lncRNA (126, 139) have been observed during GC progression, and risk models based on m5C levels were developed for prognosis prediction (139). NSUN2 is the main oncogenic m5C-methyltransferase in GC, targeting the mRNAs of PIK3R1 (phosphoinositide-3-kinase regulatory subunit 1) (34), PCYT1A (phosphate cytidylyltransferase 1 choline-alpha) (34), FOXC2 (Forkhead box protein C2) (51) and p57Kip2 (a type of cyclin-dependent kinase (CDK) inhibitor) (30). After m5C modulation and binding to m5C readers, such as YBX1, the transcriptional activity of PIK3R1, PCYT1A and FOXC2 mRNA was increased, while tumor-suppressive p57Kip2 mRNA was destabilized as a result of m5C modulation in the 3’-UTR. Consequently, the elevated NSUN2 levels in GC patients lead to enhanced proliferation, migration, and invasion of cancerous cells. NSUN2 activators, such as small ubiquitin-like modifier (SUMO)-2/3, which directly interact with NSUN2 to stabilize and mediate its nuclear transport, promote the development of GC (34). The oncogenic interaction between NSUN2 and FOXC2 mRNA can be facilitated by lncRNA FOXC2-AS1 (FOXC2 antisense RNA 1) (51). The oncogenic role of m5C modulation may also be linked to immune suppression, as patients with lower levels of m5C modulation were found to have higher levels of immune activation and longer progression-free survival (PFS) and OS (126).
Bladder cancer
m5C-mediated cell proliferation is considered one of causes of bladder cell malignancy. Overexpressed ALYREF in bladder cancer cells interacts with the 3’-UTR of PKM2 (pyruvate kinase M2) mRNA, causing its stabilization and enhanced PKM2-associated glycolysis (32). ALYREF stimulators, such as hypoxia‐inducible factor‐1alpha (HIF‐1α), significantly increase the expression levels of ALYREF and PKM2 and are correlated with poor prognosis (32). Another m5C reader, YBX1,maintains the stability of its target mRNA, oncogenic HDGF mRNA methylated by NSUN2, by forming a 3’-UTR-binding complex with ELAVL1 (ELAV like RNA binding protein 1) (100). Moreover, high expression of immune cells, including regulatory T cells (Tregs), CD8+ T cells, plasma cells and activated dendritic cells, is related to a good prognosis, while high expression of resting CD4+ memory T cells, M0 macrophages, M1 macrophages, M2 macrophages and neutrophils show the opposite trend (140).
Prostate cancer
Several prognostic models based on m5C modulators (38) or m5C-related lncRNAs (141) have been developed for prostate cancer patients. Specifically, experimental results showed that increased NSUN2 (56), YBX1 (56) and TET3 (38) levels correlate with a poor prognosis. Posttranscriptional m5C modification of androgen receptor (AR) mRNA by NSUN2 is recognized by YBX1, increasing AR mRNA stability and translation (56). Interestingly, AR positively regulates NSUN2 at the transcriptional level (56), forming a reciprocal activation loop. High NSUN2 expression is also associated with low chemotherapeutic sensitivity and immune cell infiltration (37). In addition to NSUN2, immune cell infiltration characteristics are associated with many other m5C regulators, such as NSUN6 and TET1-3 (38, 113).
Leukemia
RNA m5C modifications affect tumor malignancy and drug resistance not only in solid tumors but also in nonparenchymal tumors, including leukemia. In 2018, David G. Courtney et al. determined that NSUN1 is partly responsible for the formation of the 5-AZA (5-azacitidine)-insensitive chromatin structure during leukemia, which causes drug resistance (127). Mechanistically, NSUN1 forms an active chromatin structure with BRD4 (bromodomain-containing protein 4) and RNA-polymerase-II, which responds poorly to 5-AZA but well to the BRD4 inhibitor JQ1 or miRNA targeting NSUN1. In contrast, another two m5C regulators, NSUN3 and DNMT2, bind directly with heterogeneous nuclear ribonucleoprotein K (hnRNPK), a conserved RNA-binding protein that interacts with the lineage-determining transcription factors GATA binding protein 1 (GATA1), recombinant spleen focus forming virus proviral integration 1/purine rich box-1 (SPI1/PU.1) and cyclin-dependent kinase 9 (CDK9)/positive transcription elongation factor b (P-TEFb) to recruit RNA-polymerase-II to RNA precursors, forming chromatin structures that are sensitive to 5-AZA (127).
Head and neck squamous cell carcinoma (HNSCC)
HNSCC refers to a group of epithelium-derived cancers that occur in the mucosal surfaces of the head and neck, including the oral and nasal cavity, oropharynx, nasopharynx, larynx and hypopharynx. To date, statistics have revealed that almost all m5C regulators show elevated expression levels during HNSCC, with the exception of NSUN7 and TET2 (93, 142, 143), suggesting that they play different roles in HNSCC tumorigenesis. Mechanistically, NSUN3 promotes tumor progression by regulating immune infiltration (64), and ALYREF enhances mitochondrial activity and intracellular energy metabolism, which ensures continuous energy supplies for timorous tissues (92). In nasopharyngeal carcinoma (NPC) specifically, NSUN2 negatively regulates immune cell infiltration in the TME (58). In addition, NSUN2 promotes hypopharyngeal squamous cell carcinoma (HPSCC) by m5C-methylating oncogenic TEAD1 (TEA domain transcription factor 1) mRNA, which upregulates its expression level (55).
Breast cancer
m5C RNA modification has dual effects on breast cancer development. Recent studies suggest NSUN2 (36), ALYREF (94, 95) and DNMT3B (95) as risk factors, while NSUN5 (128), NSUN6 (36), TET2 (128), and DNMT2 (128)are protective factors. Mechanistically, ALYREF, which was found amplified both at the mRNA and protein levels, binds with the oncogenic NEAT1 lncRNA promoter region, enhancing its transcription (94). Additionally, enrichment analysis revealed that vascular endothelial growth factor A (VEGFA) and enhancer of zeste homolog 2 (EZH2) were potential targets of DNMT3B (95). In addition, NSUN5, TET2, and DNMT2 modified three lncRNAs, namely, AP005131.2, AL121832.2, and LINC01152, to be protective factors against breast cancer (128).
Pancreatic cancer
As in many other types of cancers, NSUN2 regulates pancreatic tumorigenesis and epithelial differentiation through mRNA methylation (129). In contrast, NSUN6 (31) and DNMT3A (131) have inhibitory effects on pancreatic cancer and suppress the proliferation of cancerous cells, but the mechanisms remain to be elucidated. m5C modification profoundly influences the tumor immune microenvironment (130, 144), interfering with the infiltration of CD8+ T cells and upregulating PD-L1 expression (130). Risk models based on m5C-related lncRNAs have also been constructed to provide prognostic information (145).
Clear cell renal cell carcinoma (ccRCC)
m5C modification has dual effects on ccRCC development. YBX1 negatively modulates ccRCC by binding and stabilizing PEBR1 mRNA, a tumor suppressor gene (104). Other m5C-related genes, such as DNMT3B, NSUN1, NSUN2 and NSUN5, are highly expressed in ccRCC patients and correlate with worse prognosis (110, 111, 132), while NSUN6 and TET2 mainly function as protective factors (111). Notably, the role of NSUN4 in ccRCC remains controversial, as studies have obtained opposing results (110, 111).
Other cancer types
In CRC patients, increased levels of m5C-related regulators, such as NSUN5, NSUN6, ALYREF and YBX1, were found (76, 81). The m5C levels of peripheral blood immune cells showed higher CRC diagnostic value than that of common blood tumor biomarkers (76), which is correlated with the discovery that m5C modification inhibits tumor infiltration of immune cells (133). In cholangiocarcinoma patients, the m5C-modified functional lncRNA NKILA (NF-kappa B interacting lncRNA), which is recognized and stabilized by YBX1, is associated with advanced TNM stage and poor prognosis (103). In glioma patients, m5C-associated genes, including NSUN1-5, NSUN7, DNMT1, DNMT3B and YBX-1, are upregulated, with the exception of NSUN6 (63, 101, 134). In patients with glioblastoma, the most aggressive diffuse glioma, upregulated ALYREF plays an oncogenic role by activating the Wnt/β-catenin signaling pathway and stabilizing MYC mRNA (87, 88). Interestingly, MYC also exerts positive impacts on ALYREF, forming a positive feedback loop (87). In neuroblastoma patients, the m5C reader ALYREF forms a nuclear coactivator complex with MYCN to stimulate USP3 transcription, which promotes the tumorigenesis of neuroblastoma (89). In lung adenocarcinoma patients, NSUN2 and ALYREF were found to be oncogenic through interacting with YAP mRNA. The m5C modification in the 328-331 3’-UTR of YAP mRNA increases its stability, enhances exosome secretion, and stimulates the transcription of seven downstream exosome-promoting genes. Together, m5C-mediated YAP stimulation leads to increased tumor malignancy and drug resistance (91). In addition, risk models based on m5C regulators or m5C-related lncRNAs were also developed for prognosis prediction (11, 90, 146). In patients with ESCC, NSUN2 promotes ESCC progression and chemoresistance by promoting TIGAR (TP53 induced glycolysis regulatory phosphatase) (53) and GRB2 (growth factor receptor bound protein 2) (52). The positive influence on GRB2 is achieved by NSUN2-mediated LIN28B-dependent m5C modification of GRB2 mRNA, which indirectly activates the PI3K/AKT and ERK/MAPK signaling pathways (52). In cervical cancer patients, NSUN2 and YBX1, which catalyze and recognize methylation sites, respectively, induce KRT13 mRNA methylation and translational activation (57). In ovarian cancer patients, YBX1 modulates the expression of a variety of downstream targets, including CD44, thus enhancing chemoresistance (105). In contrast, RNA m5C modification mediated by the methyltransferase NSUN6 suppresses testis, thyroid and ovary cancers (79). Finally, NSUN2-mediated RNA m5C modification modulates uveal melanoma cell proliferation and migration, although the exact mechanisms remain unknown (59).
m5C RNA modification in immune cells
Current studies have revealed that most immune cells, including T cells from different subgroups (e.g., CD4+ T cells, CD8+ T cells, Tregs), B cells and plasma cells, NK cells, NKT cells, macrophages, granulocytes and mast cells, manifest alterations in cell expression, infiltration and recruitment rate, which is concluded by several prognostic models constructed based on m5C-related lncRNAs (147), m5C-regulated genes (38, 148) or m5C-related differentially expressed genes (DEGs) (149), especially in different types of cancers, and leads to a varied immune microenvironment (Figure 4), although most scattered studies did not provide systematized and convincing results (Table 3).
T cells
Subgroups of T lymphocytes (mainly CD4+ and CD8+ T cells) are distinguished in T cells by surface markers using flow cytometry (154). Upon leaving the thymus, naïve CD4+ T cells further differentiate into T cell subsets according to different stimulating signals, such as T helper (Th) cells (e.g., Th1, Th2, Th9, Th17, Th22), T follicular helper (Tfh) cells and regulatory T cells (Tregs) (155).
Recent studies have mainly focused on the m5C RNA modification of T cells in the context of cancer. For instance, the abundance of CD4+ T cells is higher in patients with soft tissue sarcoma (STS) (149), ccRCC (110) and HCC (148) but the opposite is found in glioma patients (156). Tregs appear to be slightly different from common CD4+ T cells, as they correlate with poor prognosis in STS (149) and HCC (138) patients and positive outcomes in HNSCC (147) and bladder cancer (140) patients. The role of CD8+ T cells in cancer is also controversial since they are considered protective factors in bladder cancer (140) and lung adenocarcinoma (157) patients but risk factors in ccRCC (110) patients.
For CD4+ T cells, associated m5C-related regulators include NSUN1 (46), NSUN2 (37, 60, 151, 152), DNMT3A (80), TET1 (150) and TET2 (150). Downregulated NSUN2 expression, along with decreased mRNA m5C levels of CD4+ T cells, was observed in systemic lupus erythematosus (SLE) patients, while the number of m5C-containing RNAs increased. In addition, m5C sites were mainly distributed in mRNA translation initiation sites, and hypermethylated m5C and/or upregulated genes in SLE were enriched immune-related and inflammatory pathways, including immune system signaling pathway, cytokine signaling pathway, and interferon signaling pathway (151). In HIV-1-infected CD4+ T cells, NSUN2, as the primary HIV-1 m5C methyltransferase, facilitates HIV-1 transcript methylation as well as viral replication (152). Note that NSUN2 inactivation did not reduce HIV-1 mRNA expression levels but did downregulate protein expression, suggesting the role of m5C in HIV-1 translation. Additionally, m5C loss dysregulates the alternative splicing of viral RNAs (152). In contrast, NSUN1 deficiency caused latently infected HIV-1 proviruses to reactivate, revealing the viral suppressive effects of NSUN1 (46). Moreover, NSUN2 enhances IL-17A secretion by T cells by methylating IL-17A mRNA at C466, stimulating its translation (60). Finally, the m5C erasers TET1 and TET2 regulate pre-mRNA splicing in a CCCTC‐binding factor (CTCF)-dependent manner.
Moreover, m5C-related regulators affecting CD8+ T cells include NSUN3, NSUN6, TET1 and TET3 (38, 66, 80), but no further studies were found.
B cells and plasma cells
B cells are derived from hematopoietic stem cells (HSCs) in the bone marrow. Naïve B cells, once properly activated, mature into plasma cells, the antibody-secreting form of B cells, following an intrinsic developmental process (158). Recent studies on B cells mainly concentrated on alterations in m5C RNA modifications during tumor pathology. In HNSCC patients, a higher number of naïve B cells is negatively correlated with the risk score for poor prognosis (147). Activated plasma cells exert similar effects in bladder cancer (140) and lung adenocarcinoma (157) patients. Also, a prognostic model for pancreatic cancer based on m6A/m5C/m1A-associated lncRNAs showed that the low-risk group has a significantly higher concentration of naïve B cells and plasma cells within the TME (159), suggesting the protective role of B cells and plasma cells. Genes associated with m5C RNA modifications in B cells include NSUN2, NSUN6, DNMT3A, TET1 and TET3 (37, 38, 80). Specifically, although NSUN6 is dispensable for germinal center (GC) B-cell formation, it plays vital roles in the formation of antibody-secreting plasma cells (83).
Macrophages
Monocytes and macrophages stem from hematological precursors in the bone marrow and are important in the innate immune system due to their phagocytic and antigen-presenting activity (160). Monocytes accumulate in peripheral blood, while macrophages are tissue-resident mature monocytes (161). Classically activated macrophages, or M1 macrophages, are proinflammatory, while alternatively activated macrophages, or M2 macrophages, are anti-inflammatory (162). Similar to T cells and B cells, most studies on m5C RNA modifications in macrophages are in the context of cancer development, especially macrophage infiltration in the TME. The risk scores based on m5C-related genes of patients with four types of cancer (HCC, HNSCC, glioma and pancreatic cancer) were positively correlated with infiltration of resting macrophages (M0), M1 or M2 macrophages (138, 147, 148, 156, 159). Analysis of the TME in prostate cancer patients showed differentially expressed NSUN6, TET1 and TET3 in M1 and M2 macrophages (38). NSUN3 promotes infiltration of M2 macrophages but suppresses M1 macrophage infiltration in HNSCC patients (64). Moreover, in AAA patients, ALYREF-interacting lncRNAs are involved in immune system processes and macrophage infiltration (99).
Granulocytes
Granulocytes refer to a group of leukocytes with specific cytoplasmic granules distinguished by Romanowsky staining into three main subsets, namely, neutrophils, eosinophils and basophils (163). Currently, the scarcity of research focusing on m5C RNA modification of eosinophils and basophils makes it difficult to conclude m5C-related alterations in these two types of granulocytes. Only in lung adenocarcinoma patients is eosinophil infiltration correlated with a favorable prognostic pattern (157). Neutrophil abundance is generally associated with poor prognosis (138, 147), and a study on lung squamous cell carcinoma patients discovered that NSUN4 exerts a regulatory effect on neutrophil m5C RNA modification (66). Studies have also reported that TET2 and TET3 influence neutrophil granulation, phagocytosis and cytokine signaling by demethylating and destabilizing socs3b (suppressors of cytokine signaling 3b) mRNA, a member of the suppression of cytokine signaling gene family (153). TET2/3-defective embryos showed aberrant granule formation, defective phagocytosis and dysregulation of cytokine signaling in neutrophils due to accumulation of socs3b mRNA, which binds the Jak receptor to prevent Stat phosphorylation and downstream signaling via the Jak/Stat pathway (153).
Others
Natural killer (NK) cells constitute a first line of innate immunity against tumors due to their capabilities of killing aberrant cells (164). Regarding m5C RNA modifications in NK cells, both resting and activated NK cells are correlated with positive outcomes in HNSCC (147), glioma (156) and lung adenocarcinoma (157) patients, with NSUN2 being the most closely associated m5C gene (37).
Dendritic cells (DCs) are generally regarded as the most potent antigen-presenting cells, thus modulating both immunity and tolerance (165). In lung adenocarcinoma patients, more DC infiltration was discovered in the low-risk group, suggesting the protective role of m5C in DCs (157). In contrast, a prognostic model for pancreatic cancer based on m6A/m5C/m1A-associated lncRNAs showed that the high-risk group has a significantly higher concentration of activated DCs within the TME (159), showing the tumor-promoting effects of activated DCs. The conflicting results might result from the differences in cancer types, DC subtypes, activation extent and patient characteristics. Consequently, rigorous future studies are needed in this regard. In CRC patients, the m5C writer DNMT3A was found to be involved in regulating DCs (80).
Mast cells are tissue-resident cells that function in inflammatory responses and tissue homeostasis (166). These cells are usually recognized clinically for their roles in IgE-mediated degranulation and allergic inflammation (167). A risk model based on m6A/m1A/m5C-regulated genes in HCC patients indicated the links between poor prognosis and high infiltration of resting mast cells (148).
In summary, the roles of m5C RNA modification of immune cells in the context of tumorigenesis largely remain to be further clarified. A better understanding of the mechanisms by which alterations in cell expression, infiltration and activation are regulated by methylation can be extremely helpful for the development of novel methods for tumor diagnosis as well as treatment.
Summary and perspectives
In this review, we provide a detailed review concerning the roles of m5C RNA modifications in cancer by discussing m5C RNA-related genes and alterations in gene expression and immune cell infiltration. The modifications involve mainly mRNAs but also other noncoding RNAs such as tRNA, rRNA, and lncRNA, and are regulated by RNA m5C writers, readers and erasers, leading to changes in RNA processes, including transcription, transportation, translation and metabolism. Risk models made for prognosis prediction are based on m5C regulators as well as genes with m5C modification, which indicates the relationship between prognosis and alterations in immune cell infiltration in the TME.
However, numerous questions regarding oncogenic m5C RNA modifications remain to be elucidated. For example, the mechanisms by which m5C writers, readers and erasers function have only been investigated in a limited manner. What are the target genes of RNA m5C modifications? How are these genes linked to cell signal transduction and tumor malignancy? Additionally, we now know that RNA m5C modifications are related to immune cell infiltration within the TME, but scarce and controversial study results offer no comprehensive and fully convincing conclusions. What alterations do RNA m5C modifications cause indifferent groups of immune cells? How are these alterations linked to the progression of cancer? Is immune cell infiltration protective or destructive for patients with malignant tumors? These questions might provide deeper insights into the diagnosis and treatment of different cancer types.
Nevertheless, studies on RNA m5C modifications in cancer patients have massively progressed within the last five years, providing new analytical results from clinical samples. It should be pointed out that most of the studies based on clinical samples were only limited at the laboratory level, and no m5C-related clinical trials against cancer has been performed so far. However, as detection methods for RNA m5C sites continue to improve, cancerous RNA m5C modifications will most likely remain a popular scientific topic in the years to come and, hopefully, instill new hope for millions of patients fighting cancer.
Author contributions
XG and XM have equal contributions to this study. XG and HZ designed the whole study. XG and XM drafted the manuscript. CC, JG, JW, and SW made the relevant edits to the manuscript. XG and XM revised the manuscript. All authors contributed to the article and approved the submitted version.
Funding
This study was supported by grants awarded by the National Science and Technology Major Project of China (NO 2018ZX10302206), and the Science and Technology Major Projects of Zhejiang Province (NO 2018C04016).
Conflict of interest
The authors declare that the research was conducted in the absence of any commercial or financial relationships that could be construed as a potential conflict of interest.
Publisher’s note
All claims expressed in this article are solely those of the authors and do not necessarily represent those of their affiliated organizations, or those of the publisher, the editors and the reviewers. Any product that may be evaluated in this article, or claim that may be made by its manufacturer, is not guaranteed or endorsed by the publisher.
References
1. Cohn WE. Pseudouridine, a carbon-carbon linked ribonucleoside in ribonucleic acids: isolation, structure, and chemical characteristics. J Biol Chem (1960) 235:1488–98. doi: 10.1016/S0021-9258(18)69432-3
2. Boccaletto P, Stefaniak F, Ray A, Cappannini A, Mukherjee S, Purta E, et al. MODOMICS: a database of RNA modification pathways. 2021 update. Nucleic Acids Res (2022) 50:D231–5.
3. Jonkhout N, Tran J, Smith MA, Schonrock N, Mattick JS, Novoa EM. The RNA modification landscape in human disease. RNA N Y N (2017) 23:1754–69. doi: 10.1261/rna.063503.117
4. Roundtree IA, Evans ME, Pan T, He C. Dynamic RNA modifications in gene expression regulation. Cell (2017) 169:1187–200. doi: 10.1016/j.cell.2017.05.045
5. Barbieri I, Kouzarides T. Role of RNA modifications in cancer. Nat Rev Cancer (2020) 20:303–22. doi: 10.1038/s41568-020-0253-2
6. Shi H, Wei J, He C. Where, when, and how: context-dependent functions of RNA methylation writers, readers, and erasers. Mol Cell (2019) 74:640–50. doi: 10.1016/j.molcel.2019.04.025
7. Zaccara S, Ries RJ, Jaffrey SR. Reading, writing and erasing mRNA methylation. Nat Rev Mol Cell Biol (2019) 20:608–24. doi: 10.1038/s41580-019-0168-5
8. Xue C, Zhao Y, Li L. Advances in RNA cytosine-5 methylation: detection, regulatory mechanisms, biological functions and links to cancer. biomark Res (2020) 8:43. doi: 10.1186/s40364-020-00225-0
9. Trixl L, Lusser A. The dynamic RNA modification 5-methylcytosine and its emerging role as an epitranscriptomic mark. Wiley Interdiscip Rev RNA. (2019) 10:e1510. doi: 10.1002/wrna.1510
10. Haruehanroengra P, Zheng YY, Zhou Y, Huang Y, Sheng J. RNA Modifications and cancer. RNA Biol (2020) 17:1560–75. doi: 10.1080/15476286.2020.1722449
11. Bai M, Sun C. M5C-related lncRNA predicts lung adenocarcinoma and tumor microenvironment remodeling: computational biology and basic science. Front Cell Dev Biol (2022) 10:885568. doi: 10.3389/fcell.2022.885568
12. Liang Z, Riaz A, Chachar S, Ding Y, Du H, Gu X. Epigenetic modifications of mRNA and DNA in plants. Mol Plant (2020) 13:14–30. doi: 10.1016/j.molp.2019.12.007
13. Tomikawa C. 7-methylguanosine modifications in transfer RNA (tRNA). Int J Mol Sci (2018) 19:4080. doi: 10.3390/ijms19124080
14. Li G, Ma L, He S, Luo R, Wang B, Zhang W, et al. WTAP-mediated m6A modification of lncRNA NORAD promotes intervertebral disc degeneration. Nat Commun (2022) 13:1469. doi: 10.1038/s41467-022-28990-6
15. Sun T, Wu R, Ming L. The role of m6A RNA methylation in cancer. BioMed Pharmacother Biomede Pharmacother (2019) 112:108613. doi: 10.1016/j.biopha.2019.108613
16. Deng X, Su R, Weng H, Huang H, Li Z, Chen J. RNA N6-methyladenosine modification in cancers: current status and perspectives. Cell Res (2018) 28:507–17. doi: 10.1038/s41422-018-0034-6
17. Wiener D, Schwartz S. The epitranscriptome beyond m6A. Nat Rev Genet (2021) 22:119–31. doi: 10.1038/s41576-020-00295-8
18. Huber SM, van Delft P, Mendil L, Bachman M, Smollett K, Werner F, et al. Formation and abundance of 5-hydroxymethylcytosine in RNA. Chembiochem Eur J Chem Biol (2015) 16:752–5. doi: 10.1002/cbic.201500013
19. Guo G, Pan K, Fang S, Ye L, Tong X, Wang Z, et al. Advances in mRNA 5-methylcytosine modifications: detection, effectors, biological functions, and clinical relevance. Mol Ther Nucleic Acids (2021) 26:575–93. doi: 10.1016/j.omtn.2021.08.020
20. Squires JE, Patel HR, Nousch M, Sibbritt T, Humphreys DT, Parker BJ, et al. Widespread occurrence of 5-methylcytosine in human coding and non-coding RNA. Nucleic Acids Res (2012) 40:5023–33. doi: 10.1093/nar/gks144
21. Gao Y, Fang J. RNA 5-methylcytosine modification and its emerging role as an epitranscriptomic mark. RNA Biol (2021) 18:117–27. doi: 10.1080/15476286.2021.1950993
22. Saplaoura E, Perrera V, Colot V, Kragler F. Methylated RNA immunoprecipitation assay to study m5C modification in arabidopsis. J Vis Exp JoVE (2020). doi: 10.3791/61231
23. García-Vílchez R, Sevilla A, Blanco S. Post-transcriptional regulation by cytosine-5 methylation of RNA. Biochim Biophys Acta Gene Regul Mech (2019) 1862:240–52. doi: 10.1016/j.bbagrm.2018.12.003
24. Squires JE, Preiss T. Function and detection of 5-methylcytosine in eukaryotic RNA. Epigenomics (2010) 2:709–15. doi: 10.2217/epi.10.47
25. Bohnsack KE, Höbartner C, Bohnsack MT. Eukaryotic 5-methylcytosine (m5C) RNA methyltransferases: mechanisms, cellular functions, and links to disease. Genes (2019) 10:102. doi: 10.3390/genes10020102
26. Teng P-C, Liang Y, Yarmishyn AA, Hsiao Y-J, Lin T-Y, Lin T-W, et al. RNA Modifications and epigenetics in modulation of lung cancer and pulmonary diseases. Int J Mol Sci (2021) 22:10592. doi: 10.3390/ijms221910592
27. Delaunay S, Pascual G, Feng B, Klann K, Behm M, Hotz-Wagenblatt A, et al. Mitochondrial RNA modifications shape metabolic plasticity in metastasis. Nature (2022) 607:593–603. doi: 10.1038/s41586-022-04898-5
28. Agarwal MK, Weinstein IB. Modifications of ribonucleic acid by chemical carcinogens. II. In vivo reaction of n-2-acetylaminofluorene with rat liver ribonucleic acid. Biochemistry (1970) 9:503–8. doi: 10.1021/bi00805a008
29. Zamecnik PC. Summary of symposium on transfer RNA and transfer RNA modification in differentiation and neoplasia. Cancer Res (1971) 31:716–21.
30. Mei L, Shen C, Miao R, Wang J-Z, Cao M-D, Zhang Y-S, et al. RNA Methyltransferase NSUN2 promotes gastric cancer cell proliferation by repressing p57Kip2 by an m5C-dependent manner. Cell Death Dis (2020) 11:270. doi: 10.1038/s41419-020-2487-z
31. Yang R, Liang X, Wang H, Guo M, Shen H, Shi Y, et al. The RNA methyltransferase NSUN6 suppresses pancreatic cancer development by regulating cell proliferation. EBioMedicine (2021) 63:103195. doi: 10.1016/j.ebiom.2020.103195
32. Wang J-Z, Zhu W, Han J, Yang X, Zhou R, Lu H-C, et al. The role of the HIF-1α/ALYREF/PKM2 axis in glycolysis and tumorigenesis of bladder cancer. Cancer Commun Lond Engl (2021) 41:560–75. doi: 10.1002/cac2.12158
33. Zhang Q, Liu F, Chen W, Miao H, Liang H, Liao Z, et al. The role of RNA m5C modification in cancer metastasis. Int J Biol Sci (2021) 17:3369–80. doi: 10.7150/ijbs.61439
34. Hu Y, Chen C, Tong X, Chen S, Hu X, Pan B, et al. NSUN2 modified by SUMO-2/3 promotes gastric cancer progression and regulates mRNA m5C methylation. Cell Death Dis (2021) 12:842. doi: 10.1038/s41419-021-04127-3
35. Sun Z, Xue S, Zhang M, Xu H, Hu X, Chen S, et al. Aberrant NSUN2-mediated m5C modification of H19 lncRNA is associated with poor differentiation of hepatocellular carcinoma. Oncogene (2020) 39:6906–19. doi: 10.1038/s41388-020-01475-w
36. Huang Z, Pan J, Wang H, Du X, Xu Y, Wang Z, et al. Prognostic significance and tumor immune microenvironment heterogenicity of m5C RNA methylation regulators in triple-negative breast cancer. Front Cell Dev Biol (2021) 9:657547. doi: 10.3389/fcell.2021.657547
37. Sun G, Ma S, Zheng Z, Wang X, Chen S, Chang T, et al. Multi-omics analysis of expression and prognostic value of NSUN members in prostate cancer. Front Oncol (2022) 12:965571. doi: 10.3389/fonc.2022.965571
38. Yu G, Bao J, Zhan M, Wang J, Li X, Gu X, et al. Comprehensive analysis of m5C methylation regulatory genes and tumor microenvironment in prostate cancer. Front Immunol (2022) 13:914577. doi: 10.3389/fimmu.2022.914577
39. Goll MG, Kirpekar F, Maggert KA, Yoder JA, Hsieh C-L, Zhang X, et al. Methylation of tRNAAsp by the DNA methyltransferase homolog Dnmt2. Science (2006) 311:395–8. doi: 10.1126/science.1120976
40. Schaefer M, Pollex T, Hanna K, Tuorto F, Meusburger M, Helm M, et al. RNA Methylation by Dnmt2 protects transfer RNAs against stress-induced cleavage. Genes Dev (2010) 24:1590–5. doi: 10.1101/gad.586710
41. Tuorto F, Liebers R, Musch T, Schaefer M, Hofmann S, Kellner S, et al. RNA Cytosine methylation by Dnmt2 and NSun2 promotes tRNA stability and protein synthesis. Nat Struct Mol Biol (2012) 19:900–5. doi: 10.1038/nsmb.2357
42. Xue S, Xu H, Sun Z, Shen H, Chen S, Ouyang J, et al. Depletion of TRDMT1 affects 5-methylcytosine modification of mRNA and inhibits HEK293 cell proliferation and migration. Biochem Biophys Res Commun (2019) 520:60–6. doi: 10.1016/j.bbrc.2019.09.098
43. Liao H, Gaur A, McConie H, Shekar A, Wang K, Chang JT, et al. Human NOP2/NSUN1 regulates ribosome biogenesis through non-catalytic complex formation with box C/D snoRNPs. Nucleic Acids Res (2022) 50:10695–716. doi: 10.1093/nar/gkac817
44. Kosi N, Alić I, Kolačević M, Vrsaljko N, Jovanov Milošević N, Sobol M, et al. Nop2 is expressed during proliferation of neural stem cells and in adult mouse and human brain. Brain Res (2015) 1597:65–76. doi: 10.1016/j.brainres.2014.11.040
45. Heissenberger C, Rollins JA, Krammer TL, Nagelreiter F, Stocker I, Wacheul L, et al. The ribosomal RNA m5C methyltransferase NSUN-1 modulates healthspan and oogenesis in caenorhabditis elegans. eLife (2020) 9:e56205. doi: 10.7554/eLife.56205
46. Kong W, Biswas A, Zhou D, Fiches G, Fujinaga K, Santoso N, et al. Nucleolar protein NOP2/NSUN1 suppresses HIV-1 transcription and promotes viral latency by competing with tat for TAR binding and methylation. PloS Pathog (2020) 16:e1008430. doi: 10.1371/journal.ppat.1008430
47. Blaze J, Navickas A, Phillips HL, Heissel S, Plaza-Jennings A, Miglani S, et al. Neuronal Nsun2 deficiency produces tRNA epitranscriptomic alterations and proteomic shifts impacting synaptic signaling and behavior. Nat Commun (2021) 12:4913. doi: 10.1038/s41467-021-24969-x
48. Shinoda S, Kitagawa S, Nakagawa S, Wei F-Y, Tomizawa K, Araki K, et al. Mammalian NSUN2 introduces 5-methylcytidines into mitochondrial tRNAs. Nucleic Acids Res (2019) 47:8734–45. doi: 10.1093/nar/gkz575
49. Van Haute L, Lee S-Y, McCann BJ, Powell CA, Bansal D, Vasiliauskaitė L, et al. NSUN2 introduces 5-methylcytosines in mammalian mitochondrial tRNAs. Nucleic Acids Res (2019) 47:8720–33. doi: 10.1093/nar/gkz559
50. Sun Z, Xue S, Xu H, Hu X, Chen S, Yang Z, et al. Effects of NSUN2 deficiency on the mRNA 5-methylcytosine modification and gene expression profile in HEK293 cells. Epigenomics (2019) 11:439–53. doi: 10.2217/epi-2018-0169
51. Yan J, Liu J, Huang Z, Huang W, Lv J. FOXC2-AS1 stabilizes FOXC2 mRNA via association with NSUN2 in gastric cancer cells. Hum Cell (2021) 34:1755–64. doi: 10.1007/s13577-021-00583-3
52. Su J, Wu G, Ye Y, Zhang J, Zeng L, Huang X, et al. NSUN2-mediated RNA 5-methylcytosine promotes esophageal squamous cell carcinoma progression via LIN28B-dependent GRB2 mRNA stabilization. Oncogene (2021) 40:5814–28. doi: 10.1038/s41388-021-01978-0
53. Niu X, Peng L, Liu W, Miao C, Chen X, Chu J, et al. A cis-eQTL in NSUN2 promotes esophageal squamous-cell carcinoma progression and radiochemotherapy resistance by mRNA-m5C methylation. Signal Transduct Target Ther (2022) 7:267. doi: 10.1038/s41392-022-01063-2
54. Song D, An K, Zhai W, Feng L, Xu Y, Sun R, et al. NSUN2-mediated mRNA m5C modification regulates the progression of hepatocellular carcinoma. Genomics Proteomics Bioinf (2022) S1672-0229(22):00123–1. doi: 10.1016/j.gpb.2022.09.007
55. Chen L, Ding J, Wang B, Chen X, Ying X, Yu Z, et al. RNA Methyltransferase NSUN2 promotes hypopharyngeal squamous cell carcinoma proliferation and migration by enhancing TEAD1 expression in an m5C-dependent manner. Exp Cell Res (2021) 404:112664. doi: 10.1016/j.yexcr.2021.112664
56. Zhu W, Wan F, Xu W, Liu Z, Wang J, Zhang H, et al. Positive epigenetic regulation loop between AR and NSUN2 promotes prostate cancer progression. Clin Transl Med (2022) 12:e1028. doi: 10.1002/ctm2.1028
57. Wang L, Zhang J, Su Y, Maimaitiyiming Y, Yang S, Shen Z, et al. Distinct roles of m5C RNA methyltransferase NSUN2 in major gynecologic cancers. Front Oncol (2022) 12:786266. doi: 10.3389/fonc.2022.786266
58. Tong X, Xiang Y, Hu Y, Hu Y, Li H, Wang H, et al. NSUN2 promotes tumor progression and regulates immune infiltration in nasopharyngeal carcinoma. Front Oncol (2022) 12:788801. doi: 10.3389/fonc.2022.788801
59. Luo G, Xu W, Chen X, Wang S, Wang J, Dong F, et al. NSUN2-mediated RNA m5C modification modulates uveal melanoma cell proliferation and migration. Epigenetics (2022) 17:922–33. doi: 10.1080/15592294.2022.2088047
60. Wang N, Tang H, Wang X, Wang W, Feng J. Homocysteine upregulates interleukin-17A expression via NSun2-mediated RNA methylation in T lymphocytes. Biochem Biophys Res Commun (2017) 493:94–9. doi: 10.1016/j.bbrc.2017.09.069
61. Li Q, Li X, Tang H, Jiang B, Dou Y, Gorospe M, et al. NSUN2-mediated m5C methylation and METTL3/METTL14-mediated m6A methylation cooperatively enhance p21 translation. J Cell Biochem (2017) 118:2587–98. doi: 10.1002/jcb.25957
62. Yang X, Yang Y, Sun B-F, Chen Y-S, Xu J-W, Lai W-Y, et al. 5-methylcytosine promotes mRNA export - NSUN2 as the methyltransferase and ALYREF as an m5C reader. Cell Res (2017) 27:606–25. doi: 10.1038/cr.2017.55
63. Li X, Meng Y. Expression and prognostic characteristics of m5 c regulators in low-grade glioma. J Cell Mol Med (2021) 25:1383–93. doi: 10.1111/jcmm.16221
64. Jin S, Li J, Shen Y, Wu Y, Zhang Z, Ma H. RNA 5-methylcytosine regulator NSUN3 promotes tumor progression through regulating immune infiltration in head and neck squamous cell carcinoma. Oral Dis (2022). doi: 10.1111/odi.14357
65. Van Haute L, Dietmann S, Kremer L, Hussain S, Pearce SF, Powell CA, et al. Deficient methylation and formylation of mt-tRNA(Met) wobble cytosine in a patient carrying mutations in NSUN3. Nat Commun (2016) 7:12039. doi: 10.1038/ncomms12039
66. Pan J, Huang Z, Xu Y. m5C RNA methylation regulators predict prognosis and regulate the immune microenvironment in lung squamous cell carcinoma. Front Oncol (2021) 11:657466. doi: 10.3389/fonc.2021.657466
67. Paramasivam A, Meena AK, Venkatapathi C, Pitceathly RDS, Thangaraj K. Novel biallelic NSUN3 variants cause early-onset mitochondrial encephalomyopathy and seizures. J Mol Neurosci MN (2020) 70:1962–5. doi: 10.1007/s12031-020-01595-8
68. Metodiev MD, Spåhr H, Loguercio Polosa P, Meharg C, Becker C, Altmueller J, et al. NSUN4 is a dual function mitochondrial protein required for both methylation of 12S rRNA and coordination of mitoribosomal assembly. PLoS Genet (2014) 10:e1004110. doi: 10.1371/journal.pgen.1004110
69. Navarro IC, Tuorto F, Jordan D, Legrand C, Price J, Braukmann F, et al. Translational adaptation to heat stress is mediated by RNA 5-methylcytosine in caenorhabditis elegans. EMBO J (2021) 40. doi: 10.15252/embj.2020105496
70. Yang L, Ren Z, Yan S, Zhao L, Liu J, Zhao L, et al. Nsun4 and Mettl3 mediated translational reprogramming of Sox9 promotes BMSC chondrogenic differentiation. Commun Biol (2022) 5:495. doi: 10.1038/s42003-022-03420-x
71. Cui M, Qu F, Wang L, Liu X, Yu J, Tang Z, et al. m5C RNA methyltransferase-related gene NSUN4 stimulates malignant progression of hepatocellular carcinoma and can be a prognostic marker. Cancer biomark Sect Dis Markers (2022) 33:389–400. doi: 10.3233/CBM-210154
72. He Y, Yu X, Li J, Zhang Q, Zheng Q, Guo W. Role of m5C-related regulatory genes in the diagnosis and prognosis of hepatocellular carcinoma. Am J Transl Res (2020) 12:912–22.
73. Heissenberger C, Liendl L, Nagelreiter F, Gonskikh Y, Yang G, Stelzer EM, et al. Loss of the ribosomal RNA methyltransferase NSUN5 impairs global protein synthesis and normal growth. Nucleic Acids Res (2019) 47:11807–25. doi: 10.1093/nar/gkz1043
74. Burgess AL, David R, Searle IR. Conservation of tRNA and rRNA 5-methylcytosine in the kingdom plantae. BMC Plant Biol (2015) 15:199. doi: 10.1186/s12870-015-0580-8
75. Zhang X-W, Wu L-Y, Liu H-R, Huang Y, Qi Q, Zhong R, et al. NSUN5 promotes progression and predicts poor prognosis in hepatocellular carcinoma. Oncol Lett (2022) 24. doi: 10.3892/ol.2022.13559
76. Yin H, Huang Z, Niu S, Ming L, Jiang H, Gu L, et al. 5-methylcytosine (m5C) modification in peripheral blood immune cells is a novel non-invasive biomarker for colorectal cancer diagnosis. Front Immunol (2022) 13:967921. doi: 10.3389/fimmu.2022.967921
77. Haag S, Warda AS, Kretschmer J, Günnigmann MA, Höbartner C, Bohnsack MT. NSUN6 is a human RNA methyltransferase that catalyzes formation of m5C72 in specific tRNAs. RNA N Y N (2015) 21:1532–43. doi: 10.1261/rna.051524.115
78. Long T, Li J, Li H, Zhou M, Zhou X-L, Liu R-J, et al. Sequence-specific and shape-selective RNA recognition by the human RNA 5-methylcytosine methyltransferase NSun6. J Biol Chem (2016) 291:24293–303. doi: 10.1074/jbc.M116.742569
79. Selmi T, Hussain S, Dietmann S, Heiß M, Borland K, Flad S, et al. Sequence- and structure-specific cytosine-5 mRNA methylation by NSUN6. Nucleic Acids Res (2021) 49:1006–22. doi: 10.1093/nar/gkaa1193
80. Fang X, Miao C, Zeng T, Chu W, Zheng Y, Sun X, et al. Role of m5 c RNA methylation regulators in colorectal cancer prognosis and immune microenvironment. J Clin Lab Anal (2022) 36:e24303.
81. Huang Y, Huang C, Jiang X, Yan Y, Zhuang K, Liu F, et al. Exploration of potential roles of m5C-related regulators in colon adenocarcinoma prognosis. Front Genet (2022) 13:816173. doi: 10.3389/fgene.2022.816173
82. Liu J, Huang T, Chen W, Ding C, Zhao T, Zhao X, et al. Developmental mRNA m5C landscape and regulatory innovations of massive m5C modification of maternal mRNAs in animals. Nat Commun (2022) 13:2484. doi: 10.1038/s41467-022-30210-0
83. Wang W, Huang H, Jiang H, Tian C, Tang Y, Gan D, et al. A cross-tissue investigation of molecular targets and physiological functions of Nsun6 using knockout mice. Int J Mol Sci (2022) 23:6584. doi: 10.3390/ijms23126584
84. Aguilo F, Li S, Balasubramaniyan N, Sancho A, Benko S, Zhang F, et al. Deposition of 5-methylcytosine on enhancer RNAs enables the coactivator function of PGC-1α. Cell Rep (2016) 14:479–92. doi: 10.1016/j.celrep.2015.12.043
85. Li X-Y, Yang X-T. Correlation between the RNA methylation genes and immune infiltration and prognosis of patients with hepatocellular carcinoma: a pan-cancer analysis. J Inflammation Res (2022) 15:3941–56. doi: 10.2147/JIR.S373776
86. Xue C, Zhao Y, Li G, Li L. Multi-omic analyses of the m5C regulator ALYREF reveal its essential roles in hepatocellular carcinoma. Front Oncol (2021) 11:633415. doi: 10.3389/fonc.2021.633415
87. Wang J, Li Y, Xu B, Dong J, Zhao H, Zhao D, et al. ALYREF drives cancer cell proliferation through an ALYREF-MYC positive feedback loop in glioblastoma. OncoTargets Ther (2021) 14:145–55. doi: 10.2147/OTT.S286408
88. Zottel A, Jovčevska I, Šamec N, Mlakar J, Šribar J, Križaj I, et al. Anti-vimentin, anti-TUFM, anti-NAP1L1 and anti-DPYSL2 nanobodies display cytotoxic effect and reduce glioblastoma cell migration. Ther Adv Med Oncol (2020) 12:1758835920915302. doi: 10.1177/1758835920915302
89. Nagy Z, Seneviratne JA, Kanikevich M, Chang W, Mayoh C, Venkat P, et al. An ALYREF-MYCN coactivator complex drives neuroblastoma tumorigenesis through effects on USP3 and MYCN stability. Nat Commun (2021) 12:1881. doi: 10.1038/s41467-021-22143-x
90. Liu T, Hu X, Lin C, Shi X, He Y, Zhang J, et al. 5-methylcytosine RNA methylation regulators affect prognosis and tumor microenvironment in lung adenocarcinoma. Ann Transl Med (2022) 10:259. doi: 10.21037/atm-22-500
91. Yu W, Zhang C, Wang Y, Tian X, Miao Y, Meng F, et al. YAP 5-methylcytosine modification increases its mRNA stability and promotes the transcription of exosome secretion-related genes in lung adenocarcinoma. Cancer Gene Ther (2023) 30:149–62. doi: 10.1038/s41417-022-00533-7
92. Xue M, Shi Q, Zheng L, Li Q, Yang L, Zhang Y. Gene signatures of m5C regulators may predict prognoses of patients with head and neck squamous cell carcinoma. Am J Transl Res (2020) 12:6841–52.
93. Suárez E, González L, Pérez-Mitchell C, Ortiz AP, Ramírez-Sola M, Acosta J, et al. Pathway analysis using gene-expression profiles of HPV-positive and HPV-negative oropharyngeal cancer patients in a Hispanic population: methodological procedures. P R Health Sci J (2016) 35:3–8.
94. Klec C, Knutsen E, Schwarzenbacher D, Jonas K, Pasculli B, Heitzer E, et al. ALYREF, a novel factor involved in breast carcinogenesis, acts through transcriptional and post-transcriptional mechanisms selectively regulating the short NEAT1 isoform. Cell Mol Life Sci CMLS (2022) 79:391. doi: 10.1007/s00018-022-04402-2
95. Liu J, Xiao S, Chen J, Lou W, Chen X. A comprehensive analysis for expression, diagnosis, and prognosis of m5C regulator in breast cancer and its ncRNA-mRNA regulatory mechanism. Front Genet (2022) 13:822721. doi: 10.3389/fgene.2022.822721
96. Liu Y, Zhao Y, Wu R, Chen Y, Chen W, Liu Y, et al. mRNA m5C controls adipogenesis by promoting CDKN1A mRNA export and translation. RNA Biol (2021) 18:711–21. doi: 10.1080/15476286.2021.1980694
97. Liu Y, Yang Y, Wu R, Gao C-C, Liao X, Han X, et al. mRNA m5C inhibits adipogenesis and promotes myogenesis by respectively facilitating YBX2 and SMO mRNA export in ALYREF-m5C manner. Cell Mol Life Sci CMLS (2022) 79:481. doi: 10.1007/s00018-022-04474-0
98. Eckwahl M, Xu R, Michalkiewicz J, Zhang W, Patel P, Cai Z, et al. 5-methylcytosine RNA modifications promote retrovirus replication in an ALYREF reader protein-dependent manner. J Virol (2020) 94:e00544–20. doi: 10.1128/JVI.00544-20
99. He Y, Zhang H, Yin F, Guo P, Wang S, Wu Y, et al. Novel insights into the role of 5-methylcytosine RNA methylation in human abdominal aortic aneurysm. Front Biosci Landmark Ed (2021) 26:1147–65. doi: 10.52586/5016
100. Chen X, Li A, Sun B-F, Yang Y, Han Y-N, Yuan X, et al. 5-methylcytosine promotes pathogenesis of bladder cancer through stabilizing mRNAs. Nat Cell Biol (2019) 21:978–90. doi: 10.1038/s41556-019-0361-y
101. Fotovati A, Abu-Ali S, Wang P-S, Deleyrolle LP, Lee C, Triscott J, et al. YB-1 bridges neural stem cells and brain tumor-initiating cells via its roles in differentiation and cell growth. Cancer Res (2011) 71:5569–78. doi: 10.1158/0008-5472.CAN-10-2805
102. Chen S, Cao X, Ben S, Zhu L, Gu D, Wu Y, et al. Genetic variants in RNA m5 c modification genes associated with survival and chemotherapy efficacy of colorectal cancer. Cancer Med (2023) 12:1376–88. doi: 10.1002/cam4.5018
103. Zheng H, Zhu M, Li W, Zhou Z, Wan X. m5 c and m6 a modification of long noncoding NKILA accelerates cholangiocarcinoma progression via the miR-582-3p-YAP1 axis. Liver Int Off J Int Assoc Study Liver (2022) 42:1144–57.
104. Yang L, Yin H, Chen Y, Pan C, Hang H, Lu Y, et al. Low expression of PEBP1P2 promotes metastasis of clear cell renal cell carcinoma by post-transcriptional regulation of PEBP1 and KLF13 mRNA. Exp Hematol Oncol (2022) 11:87. doi: 10.1186/s40164-022-00346-2
105. Kang Y, Hu W, Ivan C, Dalton HJ, Miyake T, Pecot CV, et al. Role of focal adhesion kinase in regulating YB-1-mediated paclitaxel resistance in ovarian cancer. J Natl Cancer InstJ Natl Cancer Inst (2013) 105. doi: 10.1093/jnci/djt210
106. Yang Y, Wang L, Han X, Yang W-L, Zhang M, Ma H-L, et al. RNA 5-methylcytosine facilitates the maternal-to-Zygotic transition by preventing maternal mRNA decay. Mol Cell (2019) 75:1188–202.e11. doi: 10.1016/j.molcel.2019.06.033
107. Yang H, Wang Y, Xiang Y, Yadav T, Ouyang J, Phoon L, et al. FMRP promotes transcription-coupled homologous recombination via facilitating TET1-mediated m5C RNA modification demethylation. Proc Natl Acad Sci U S A (2022) 119:e2116251119. doi: 10.1073/pnas.2116251119
108. Shen H, Ontiveros RJ, Owens MC, Liu MY, Ghanty U, Kohli RM, et al. TET-mediated 5-methylcytosine oxidation in tRNA promotes translation. J Biol Chem (2021) 296:100087. doi: 10.1074/jbc.RA120.014226
109. Arguello AE, Li A, Sun X, Eggert TW, Mairhofer E, Kleiner RE. Reactivity-dependent profiling of RNA 5-methylcytidine dioxygenases. Nat Commun (2022) 13:4176. doi: 10.1038/s41467-022-31876-2
110. Wu J, Hou C, Wang Y, Wang Z, Li P, Wang Z. Comprehensive analysis of m5C RNA methylation regulator genes in clear cell renal cell carcinoma. Int J Genomics (2021) 2021:3803724. doi: 10.1155/2021/3803724
111. Li H, Jiang H, Huang Z, Chen Z, Chen N. Prognostic value of an m5C RNA methylation regulator-related signature for clear cell renal cell carcinoma. Cancer Manag Res (2021) 13:6673–87. doi: 10.2147/CMAR.S323072
112. Xu J, Liu X, Chen Y, Wang Y, Liu T, Yi P. RNA 5-methylcytosine regulators contribute to metabolism heterogeneity and predict prognosis in ovarian cancer. Front Cell Dev Biol (2022) 10:807786. doi: 10.3389/fcell.2022.807786
113. Xu Z, Chen S, Zhang Y, Liu R, Chen M. Roles of m5C RNA modification patterns in biochemical recurrence and tumor microenvironment characterization of prostate adenocarcinoma. Front Immunol (2022) 13:869759. doi: 10.3389/fimmu.2022.869759
114. Kawarada L, Suzuki T, Ohira T, Hirata S, Miyauchi K, Suzuki T. ALKBH1 is an RNA dioxygenase responsible for cytoplasmic and mitochondrial tRNA modifications. Nucleic Acids Res (2017) 45:7401–15. doi: 10.1093/nar/gkx354
115. Jeltsch A, Ehrenhofer-Murray A, Jurkowski TP, Lyko F, Reuter G, Ankri S, et al. Mechanism and biological role of Dnmt2 in nucleic acid methylation. RNA Biol (2017) 14:1108–23. doi: 10.1080/15476286.2016.1191737
116. Chellamuthu A, Gray SG. The RNA methyltransferase NSUN2 and its potential roles in cancer. Cells (2020) 9:1758. doi: 10.3390/cells9081758
117. Nakano S, Suzuki T, Kawarada L, Iwata H, Asano K, Suzuki T. NSUN3 methylase initiates 5-formylcytidine biogenesis in human mitochondrial tRNA(Met). Nat Chem Biol (2016) 12:546–51. doi: 10.1038/nchembio.2099
118. Wang Y, Jiang T, Xu J, Gu Y, Zhou Y, Lin Y, et al. Mutations in RNA methyltransferase gene NSUN5 confer high risk of outflow tract malformation. Front Cell Dev Biol (2021) 9:623394. doi: 10.3389/fcell.2021.623394
119. Sharma S, Yang J, Watzinger P, Kötter P, Entian K-D. Yeast Nop2 and Rcm1 methylate C2870 and C2278 of the 25S rRNA, respectively. Nucleic Acids Res (2013) 41:9062–76. doi: 10.1093/nar/gkt679
120. Liu J, Huang T, Zhang Y, Zhao T, Zhao X, Chen W, et al. Sequence- and structure-selective mRNA m5C methylation by NSUN6 in animals. Natl Sci Rev (2021) 8:nwaa273. doi: 10.1093/nsr/nwaa273
121. Fang L, Wang W, Li G, Zhang L, Li J, Gan D, et al. CIGAR-seq, a CRISPR/Cas-based method for unbiased screening of novel mRNA modification regulators. Mol Syst Biol (2020) 16:e10025. doi: 10.15252/msb.202010025
122. Seethy A, Pethusamy K, Chattopadhyay I, Sah R, Chopra A, Dhar R, et al. TETology: epigenetic mastermind in action. Appl Biochem Biotechnol (2021) 193:1701–26. doi: 10.1007/s12010-021-03537-5
123. Bowman RL, Levine RL. TET2 in normal and malignant hematopoiesis. Cold Spring Harb Perspect Med (2017) 7:a026518. doi: 10.1101/cshperspect.a026518
124. Chen Y-S, Yang W-L, Zhao Y-L, Yang Y-G. Dynamic transcriptomic m5 c and its regulatory role in RNA processing. Wiley Interdiscip Rev RNA. (2021) 12:e1639.
125. He Y, Zhang Q, Zheng Q, Yu X, Guo W. Distinct 5-methylcytosine profiles of circular RNA in human hepatocellular carcinoma. Am J Transl Res (2020) 12:5719–29.
126. Zhang Q, Sun X, Sun J, Lu J, Gao X, Shen K, et al. RNA m5C regulator-mediated modification patterns and the cross-talk between tumor microenvironment infiltration in gastric cancer. Front Immunol (2022) 13:905057. doi: 10.3389/fimmu.2022.905057
127. Cheng JX, Chen L, Li Y, Cloe A, Yue M, Wei J, et al. RNA Cytosine methylation and methyltransferases mediate chromatin organization and 5-azacytidine response and resistance in leukaemia. Nat Commun (2018) 9:1163. doi: 10.1038/s41467-018-03513-4
128. Huang Z, Li J, Chen J, Chen D. Construction of prognostic risk model of 5-Methylcytosine-Related long non-coding RNAs and evaluation of the characteristics of tumor-infiltrating immune cells in breast cancer. Front Genet (2021) 12:748279. doi: 10.3389/fgene.2021.748279
129. Chen S-Y, Chen K-L, Ding L-Y, Yu C-H, Wu H-Y, Chou Y-Y, et al. RNA Bisulfite sequencing reveals NSUN2-mediated suppression of epithelial differentiation in pancreatic cancer. Oncogene (2022) 41:3162–76. doi: 10.1038/s41388-022-02325-7
130. Yun D, Yang Z, Zhang S, Yang H, Liu D, Grützmann R, et al. An m5C methylation regulator-associated signature predicts prognosis and therapy response in pancreatic cancer. Front Cell Dev Biol (2022) 10:975684. doi: 10.3389/fcell.2022.975684
131. Yu X, Zhang Q, Gao F, Zhang M, Zheng Q, He Y, et al. Predictive value of m5C regulatory gene expression in pancreatic adenocarcinoma. Sci Rep (2021) 11:17529. doi: 10.1038/s41598-021-96470-w
132. Li L, Tao Z, Zhao Y, Li M, Zheng J, Li Z, et al. Prognostic characteristics and immune effects of N6-methyladenosine and 5-Methylcytosine-Related regulatory factors in clear cell renal cell carcinoma. Front Genet (2022) 13:864383. doi: 10.3389/fgene.2022.864383
133. Geng Q, Wei Q, Shen Z, Zheng Y, Wang L, Xue W, et al. Comprehensive analysis of the prognostic value and immune infiltrates of the three-m5C signature in colon carcinoma. Cancer Manag Res (2021) 13:7989–8002. doi: 10.2147/CMAR.S331549
134. Wang P, Wu M, Tu Z, Tao C, Hu Q, Li K, et al. Identification of RNA: 5-methylcytosine methyltransferases-related signature for predicting prognosis in glioma. Front Oncol (2020) 10. doi: 10.3389/fonc.2020.01119
135. Liu Y, Zheng S, Wang T, Fang Z, Kong J, Liu J. Identification of the expression patterns and potential prognostic role of 5-methylcytosine regulators in hepatocellular carcinoma. Front Cell Dev Biol (2022) 10:842220. doi: 10.3389/fcell.2022.842220
136. Gu X, Zhou H, Chu Q, Zheng Q, Wang J, Zhu H. Uncovering the association between m5C regulator-mediated methylation modification patterns and tumour microenvironment infiltration characteristics in hepatocellular carcinoma. Front Cell Dev Biol (2021) 9:727935. doi: 10.3389/fcell.2021.727935
137. Yang X, Yang F, Lan L, Wen N, Li H, Sun X. Diagnostic and prognostic value of m5C regulatory genes in hepatocellular carcinoma. Front Genet (2022) 13:972043. doi: 10.3389/fgene.2022.972043
138. Lu Q, Liu L, Wang S, Zhang Q, Li L. Comprehensive analysis of m5C-related lncRNAs in the prognosis and immune landscape of hepatocellular carcinoma. Front Genet (2022) 13:990594. doi: 10.3389/fgene.2022.990594
139. He C, Zhu X, Kong F, Zhang X, Chai X, Zou C, et al. The value of m5C-related lncRNAs in the prognostic assessment and immunotherapy of stomach adenocarcinoma. BioMed Res Int (2022) 2022:2747799. doi: 10.1155/2022/2747799
140. Li Z, Wang S, Chen Y, Huang Y, Li T. 5-Methylcytosine-Related long noncoding RNAs are potential biomarkers to predict overall survival and regulate tumor-immune environment in patients with bladder cancer. Dis Markers (2022) 2022:3117359. doi: 10.1155/2022/3117359
141. Wang K, Zhong W, Long Z, Guo Y, Zhong C, Yang T, et al. 5-methylcytosine RNA methyltransferases-related long non-coding RNA to develop and validate biochemical recurrence signature in prostate cancer. Front Mol Biosci (2021) 8:775304. doi: 10.3389/fmolb.2021.775304
142. Han Z, Yang B, Wang Y, Zeng X, Tian Z. Identification of expression patterns and potential prognostic significance of m5C-related regulators in head and neck squamous cell carcinoma. Front Oncol (2021) 11:592107. doi: 10.3389/fonc.2021.592107
143. Gao L, Chen R, Sugimoto M, Mizuta M, Zhou L, Kishimoto Y, et al. The RNA methylation modification 5-methylcytosine impacts immunity characteristics, prognosis and progression of oral squamous cell carcinoma by bioinformatics analysis. Front Bioeng Biotechnol (2021) 9:760724. doi: 10.3389/fbioe.2021.760724
144. Wang R, Guo Y, Ma P, Song Y, Min J, Zhao T, et al. Comprehensive analysis of 5-methylcytosine (m5C) regulators and the immune microenvironment in pancreatic adenocarcinoma to aid immunotherapy. Front Oncol (2022) 12:851766. doi: 10.3389/fonc.2022.851766
145. Yuan H, Liu J, Zhao L, Wu P, Chen G, Chen Q, et al. Prognostic risk model and tumor immune environment modulation of m5C-related LncRNAs in pancreatic ductal adenocarcinoma. Front Immunol (2021) 12:800268. doi: 10.3389/fimmu.2021.800268
146. Pan J, Huang Z, Xu Y. m5C-related lncRNAs predict overall survival of patients and regulate the tumor immune microenvironment in lung adenocarcinoma. Front Cell Dev Biol (2021) 9:671821. doi: 10.3389/fcell.2021.671821
147. Wang E, Li Y, Ming R, Wei J, Du P, Zhou P, et al. The prognostic value and immune landscapes of a m6A/m5C/m1A-related LncRNAs signature in head and neck squamous cell carcinoma. Front Cell Dev Biol (2021) 9:718974. doi: 10.3389/fcell.2021.718974
148. Li D, Li K, Zhang W, Yang K-W, Mu D-A, Jiang G-J, et al. The m6A/m5C/m1A regulated gene signature predicts the prognosis and correlates with the immune status of hepatocellular carcinoma. Front Immunol (2022) 13:918140. doi: 10.3389/fimmu.2022.918140
149. Qi L, Zhang W, Ren X, Xu R, Yang Z, Chen R, et al. Cross-talk of multiple types of RNA modification regulators uncovers the tumor microenvironment and immune infiltrates in soft tissue sarcoma. Front Immunol (2022) 13:921223. doi: 10.3389/fimmu.2022.921223
150. Marina RJ, Sturgill D, Bailly MA, Thenoz M, Varma G, Prigge MF, et al. TET-catalyzed oxidation of intragenic 5-methylcytosine regulates CTCF-dependent alternative splicing. EMBO J (2016) 35:335–55. doi: 10.15252/embj.201593235
151. Guo G, Wang H, Shi X, Ye L, Yan K, Chen Z, et al. Disease activity-associated alteration of mRNA m5 c methylation in CD4+ T cells of systemic lupus erythematosus. Front Cell Dev Biol (2020) 8:430. doi: 10.3389/fcell.2020.00430
152. Courtney DG, Tsai K, Bogerd HP, Kennedy EM, Law BA, Emery A, et al. Epitranscriptomic addition of m5C to HIV-1 transcripts regulates viral gene expression. Cell Host Microbe (2019) 26:217–27.e6. doi: 10.1016/j.chom.2019.07.005
153. Banks KM, Lan Y, Evans T. Tet proteins regulate neutrophil granulation in zebrafish through demethylation of socs3b mRNA. Cell Rep (2021) 34:108632. doi: 10.1016/j.celrep.2020.108632
154. Mousset CM, Hobo W, Woestenenk R, Preijers F, Dolstra H, van der Waart AB. Comprehensive phenotyping of T cells using flow cytometry. Cytom Part J Int Soc Anal Cytol (2019) 95:647–54. doi: 10.1002/cyto.a.23724
155. Wang W, Sung N, Gilman-Sachs A, Kwak-Kim J. T Helper (Th) cell profiles in pregnancy and recurrent pregnancy losses: Th1/Th2/Th9/Th17/Th22/Tfh cells. Front Immunol (2020) 11:2025. doi: 10.3389/fimmu.2020.02025
156. Wang LJ, Lv P, Lou Y, Ye J. Gene expression-based predication of RNA pseudouridine modification in tumor microenvironment and prognosis of glioma patients. Front Cell Dev BiolFront Cell Dev Biol (2022) 9. doi: 10.3389/fcell.2021.727595
157. Mao S, Chen Z, Wu Y, Xiong H, Yuan X. Crosstalk of eight types of RNA modification regulators defines tumor microenvironments, cancer hallmarks, and prognosis of lung adenocarcinoma. J Oncol (2022) 2022. doi: 10.1155/2022/1285632
158. Eibel H, Kraus H, Sic H, Kienzler A-K, Rizzi M. B cell biology: an overview. Curr Allergy Asthma Rep (2014) 14:434. doi: 10.1007/s11882-014-0434-8
159. Huang Y, Zhang W, Li Q, Wang Z, Yang X. Identification of m6A/m5C/m1A-associated LncRNAs for prognostic assessment and immunotherapy in pancreatic cancer. Sci Rep (2023) 13:3661. doi: 10.1038/s41598-023-30865-9
160. Jakubzick CV, Randolph GJ, Henson PM. Monocyte differentiation and antigen-presenting functions. Nat Rev Immunol (2017) 17:349–62. doi: 10.1038/nri.2017.28
161. Guilliams M, Mildner A, Yona S. Developmental and functional heterogeneity of monocytes. Immunity (2018) 49:595–613. doi: 10.1016/j.immuni.2018.10.005
162. Shapouri-Moghaddam A, Mohammadian S, Vazini H, Taghadosi M, Esmaeili S-A, Mardani F, et al. Macrophage plasticity, polarization, and function in health and disease. J Cell Physiol (2018) 233:6425–40. doi: 10.1002/jcp.26429
163. Siemińska I, Poljańska E, Baran J. Granulocytes and cells of granulocyte origin-the relevant players in colorectal cancer. Int J Mol Sci (2021) 22:3801. doi: 10.3390/ijms22073801
164. Guillerey C. NK cells in the tumor microenvironment. Adv Exp Med Biol (2020) 1273:69–90. doi: 10.1007/978-3-030-49270-0_4
165. Waisman A, Lukas D, Clausen BE, Yogev N. Dendritic cells as gatekeepers of tolerance. Semin Immunopathol (2017) 39:153–63. doi: 10.1007/s00281-016-0583-z
166. Aponte-López A, Muñoz-Cruz S. Mast cells in the tumor microenvironment. Adv Exp Med Biol (2020) 1273:159–73. doi: 10.1007/978-3-030-49270-0_9
Keywords: RNA modification, m5C, cancer, immune cells, cancer immunity
Citation: Gu X, Ma X, Chen C, Guan J, Wang J, Wu S and Zhu H (2023) Vital roles of m5C RNA modification in cancer and immune cell biology. Front. Immunol. 14:1207371. doi: 10.3389/fimmu.2023.1207371
Received: 17 April 2023; Accepted: 22 May 2023;
Published: 31 May 2023.
Edited by:
Lin Qi, Central South University, ChinaReviewed by:
Yafeng He, National Institutes of Health (NIH), United StatesZhenhua Chen, Beckman Research Institute, City of Hope, United States
Copyright © 2023 Gu, Ma, Chen, Guan, Wang, Wu and Zhu. This is an open-access article distributed under the terms of the Creative Commons Attribution License (CC BY). The use, distribution or reproduction in other forums is permitted, provided the original author(s) and the copyright owner(s) are credited and that the original publication in this journal is cited, in accordance with accepted academic practice. No use, distribution or reproduction is permitted which does not comply with these terms.
*Correspondence: Haihong Zhu, emh1aGg3MkB6anUuZWR1LmNu
†These authors have contributed equally to this work