- Biosafety Level 3 Laboratory, Wuhan Institute of Biological Products Co., Ltd., Wuhan, China
Since May 2022, mutant strains of mpox (formerly monkeypox) virus (MPXV) have been rapidly spreading among individuals who have not traveled to endemic areas in multiple locations, including Europe and the United States. Both intracellular and extracellular forms of mpox virus have multiple outer membrane proteins that can stimulate immune response. Here, we investigated the immunogenicity of MPXV structural proteins such as A29L, M1R, A35R, and B6R as a combination vaccine, and the protective effect against the 2022 mpox mutant strain was also evaluated in BALB/c mice. After mixed 15 μg QS-21 adjuvant, all four virus structural proteins were administered subcutaneously to mice. Antibody titers in mouse sera rose sharply after the initial boost, along with an increased capacity of immune cells to produce IFN-γ alongside an elevated level of cellular immunity mediated by Th1 cells. The vaccine-induced neutralizing antibodies significantly inhibited the replication of MPXV in mice and reduced the pathological damage of organs. This study demonstrates the feasibility of a multiple recombinant vaccine for MPXV variant strains.
1 Introduction
Mpox virus (MPXV) belongs to the Orthopoxvirus genus in the family of Poxviridae and consists of the following two clades: the West and Central African clades (1, 2). The strain prevalent in the 2022 mpox epidemic originated from the West African branch, which was less lethal relative to the Central African branch, although produced higher mutation rate than predicted. The 2022 MPXV diverged from the related 2018–2019 viruses by a mean of 50 single-nucleotide polymorphisms (SNPs), which is far more than expected considering previous estimates of the substitution rate for Orthopoxviruses (3). Due to the possible negative impact once mpox outbroke on human immunity, there has been a renewed interest in the availability of a safe and effective mpox vaccine, particularly against this new 2022 mutant strain. Although smallpox vaccines can offer some protection, widespread skin rashes and atopic dermatitis often occur as a side effect of the vaccination. In more severe cases, this can even lead to life-threatening side effects such as myocarditis and pericarditis (4, 5). Furthermore, attenuated vaccines may revert to pathogenic forms (6). Meanwhile, fears of smallpox virus being used as a biochemical weapon have given further impetus to the development of a safer mpox vaccine (7). Recombinant protein vaccines are prepared by purifying the expressed target protein into a vaccine that does not replicate in the body; this high level of safety is the basis for an alternative to the attenuated vaccines.
The rational design of a recombinant vaccine depends on the classical structure of the Orthopoxvirus and the immunogenicity of the selected protein. Similar to other poxviruses, MPXV also has two forms, namely, extracellular enveloped virus (EEV) and intracellular mature virus (IMV). The most abundant particles are the IMVs, which accumulate in infected cells and can be released as cells die. Meanwhile, EEVs have undergone exocytosis from cells at the plasma membrane to enhance spread within the host and are essentially the IMVs with additional membrane. However, they are highly susceptible to rupture, whereas the EEV membrane bear several important neutralizing epitopes (8, 9). The surface proteins of IMV and EEV are highly differentiated, and studies on smallpox virus have shown that a single protein does not induce as much immunogenicity as multiple proteins in combination (6, 10). Therefore, a mixture of four proteins was selected as a component of the recombinant vaccine in this study. A29L is a surface membrane fusion protein located on the IMV and is responsible for binding to cell surface heparin (11). Additionally, A35R is an envelope glycoprotein in EEV that plays a key role in the formation of effective EEVs, while contributing to the effective intercellular spread of viral particles and being required for the formation of actin-containing microvilli and intercellular spreading (12). Meanwhile, M1R is a highly conserved myristate surface membrane protein in IMV involved in viral particle assembling, and entry (13). Furthermore, B6R is a palmitoylated glycoprotein in EEV which is required for effective cell spreading, while also being involved in complement control (14, 15). All of these proteins or their homologues in smallpox virus and cowpox virus have been shown to be important neutralizing antibody targets (2, 16).
QS-21 is a water-soluble triterpene glycoside extracted from the bark of Quillaja saponaria, which has become the first choice adjuvant in many vaccine clinical trials (17). QS-21 functions as an immunostimulatory adjuvant, stimulating antibody-based and cell-mediated immune responses by acting on antigen presenting cells (APCs) and T cells to produce high titers of antibodies, which is significantly superior to other adjuvants, such as aluminum adjuvant and MF59, in terms of enhancing antibody and T-cell responses to target antigens (18, 19). In addition, it can stimulate the production of antigen-specific cytotoxic T lymphocytes, while inducing the production of Th1 cytokines, interleukin-2, and interferon-gamma (IL-2 and IFN-γ) (20–23). In terms of animal model selection, Ground squirrels, black-tailed prairie dogs, and African dormice are highly susceptible to MPXV (24–27). However, these animals are currently not being bred as experimental animals. In addition, the lack of specific immunological reagents targeting these animals can make the experiment complex and limited. BALB/c and C57BL/6 mice were found to be resistant to MPXV, however, some studies have found that a large number of viruses can still be detected in the ovary, lung, and spleen of BALB/c between 96-120 h after being challenged by the MPXV virus (28). After 240 h, the virus could not be detected, indicating that these animals had cleared the infection (29). Therefore, 96-120 h can be selected as the euthanasia time point for non-lethal models for vaccine evaluation.
In this study, BALB/c mice were immunized with recombinant A29L, M1R, A35R, and B6R proteins combined with QS-21 adjuvant. Sera were collected for neutralizing and binding antibodies test. Then mice were challenged with MPXV, and the viral loads and histopathological changes in organs were examined. Experimental results show that this vaccine induced strong antigen-specific humoral and cellular immune responses in mice. More importantly, it showed excellent protection in MPXV challenge. This information can contribute for further research on mpox vaccines.
2 Materials and methods
2.1 Viruses and cells
Vero E6 cells were maintained at 37 °C with 5% CO2 in Dulbecco’s modified Eagle’s Medium (DMEM, Gibco, New York, NY, USA) supplemented with 10% heat-inactivated fetal bovine serum (FBS, Every Green, Zhejiang, China) alongside 50 units/mL penicillin–streptomycin (Gibco, New York, NY, USA). Meanwhile, the MPXV strain (WIBP-MPXV-001) was cultured in Vero E6 cells. The confluent monolayers Vero E6 cells were infected at a multiplicity of infection (MOI) of 2 for 72 h at 37 °C, followed by three repeated freeze-thaws. Cell debris were then precipitated and removed by centrifugation at 4,000 × g for 5 min. Subsequently, the supernatant was harvested and stored at -80°C as the virulent strain for the MPXV attack challenge for this experiment. All virus culture and challenge were performed in a biosafety level 3 facility (Wuhan Institute of Biological Products Co., Ltd., Wuhan City, China).
2.2 Recombinant proteins and QS-21
Soluble forms of the MPXV recombinant proteins of A29L, M1R, A35R, and B6R (Vazyme, Nanjing, China) were marked with a His-tag respectively. QS-21 (MedChem Express, Monmouth Junction, NJ, USA) were purchased from MedChem Express and was used as vaccine adjuvant (30).
2.3 Western blotting
ACE2-293T cells transformed with pcDNA 3.1-A29L-His tag, pcDNA 3.1-B6R-His tag, pcDNA 3.1-A35R-His tag, and pcDNA 3.1-M1R-His tag were propagated and harvested, respectively. Cell from cultures were resuspended in Laemelli SDS sample buffer (Thermo Fisher Scientific, Waltham, MA, USA) and heated at 95°C for 10 min. These samples were separated on 12% SDS-PAGE, transferred to PVDF membrane (Sangon Biotech, Shanghai, China) and blocked with 5% skimmed milk-Tris buffered saline amended with 0.1% Tween 20 (TBS-T, Sangon Biotech, Shanghai, China) overnight at 4°C. Then wash three times, membrane was incubated for 1 h with anti-A29L, anti-B6R, anti-A35R and anti-M1R antibodies (Vazyme, Nanjing, China) at RT with continuous slow shaking, respectively. Membrane was incubated with 1:3000 anti-rabbit IgG conjugated to horseradish peroxidase (Sigma-Aldrich, St. Louis, MO, USA) for 30 min at RT. Signals were detected using SuperSignal™ West Femto Maximum Sensitivity Substrate (Thermo Fisher Scientific, Waltham, MA, USA).
2.4 Mouse immunization and challenge protocol
Here, 5-to-6-week-old female BALB/c mice were supplied by the animal reproduction facilities of the Wuhan Institute of Biological Products (WIBP) and subsequently kept in the animal laboratory of WIBP. All mice were randomly divided into three groups of eight mice each. A mixture of 15 μg each of A29L, M1R, A35R, and B6R was made and then this mixture was mixed with 15 μg of QS-21 in phosphate-buffered saline (PBS) to compose a recombinant protein vaccine. Mice in the vaccine group were injected subcutaneously with this recombinant protein vaccine on days 0, 21, and 42. Meanwhile, the mice in the QS-21 group were injected subcutaneously with 15 μg QS-21, whereas the mice in PBS group were subcutaneously injected with PBS.
Two weeks after the third immunization, three mice from each group were randomly selected to measured T cell responses, and the remain mice (n = 5 mice/group) were anesthetized with isopentane and inoculated intranasally and intraperitoneally using 20 μL and 480 µL of the MPXV, respectively. MPXV titer was 2.24 × 10 8 PFU/mL. Additionally, the weight and clinical symptoms were monitored during the experiment.
2.5 Sample collection
Eight mice from each group had venous blood collected through the infraorbital plexus on days 0, 21, 42, and 56. Three mice from each group were randomly selected to be sacrificed and collected spleens on day 56. Five days after viral challenge, the remaining five mice from each group were euthanized and blood samples, spleens, lungs and ovaries were all collected. Blood was then incubated at 37°C for 1 h, before being maintained overnight at 4°C to shrink the clot, and subsequently centrifuged at a relative centrifugal force of 2,000 × g for 10 min. Sera were then collected and stored at -80°C until later use.
2.6 Immunofluorescence staining
Vero E6 cells were inoculated in 24-well plates the day before transfection, with transfection starting when cell density reached 80%. System A was 500 μL OPTI-MEM mixed with 10 μg plasmid pcDNA3.1-His-C (Fenghui Biotechnology Co., Ltd, Hunan, China) and then left at room temperature (RT) for 5 min. Meanwhile, System B was 500 μL OPTI-MEM mixed with 20 μL Lipofectamine 2000 (invitrogen, Carlsbad, CA, USA), and then left at RT for 5 min. Systems A and B were then mixed and allowed to stand for 15 min, before being inoculated into 24-well plates with 100 μL per well. The plates were incubated in the carbon-dioxide incubator at 37°C for 6 h, and the medium was changed to DMEM supplemented with 10% FBS and 50 units/mL penicillin–streptomycin and subsequently incubated for 60 h. Cells were washed with PBS and then incubated in PBS containing 5% BSA (YHSM, Beijing, China) and 1% Triton X-100 (Sinopharm Chemical Reagent Co., Ltd, Shanghai, China) at RT for 1 h, followed by incubation overnight at 4°C with primary antibodies. The primary antibody for the positive control was 6 × His Tag Monoclonal Antibody (invitrogen, Carlsbad, CA, USA), and for the rest of the group was mouse serum. After washing to remove primary antibody, cells were incubated with FITC-conjugated AffiniPure Goat Anti-mouse IgG(H+L) (Boster, Wuhan, China) at RT for 1 h, and then washed thoroughly. Cell nuclei were stained with DAPI (Solarbio, Beijing, China) for 10 min, followed by washing thoroughly again and being photographed under a fluorescent microscope to collect images.
2.7 Enzyme-linked immunosorbent assay
Polystyrene 96-well round bottom plates (Corning, New York, NY, USA) were coated with recombinant proteins A29L, M1R, A35R, and B6R in 0.05 M carbonate buffer (15 mM Na2CO3 and 3.5 mM NaHCO3) at 4°C overnight. Plates were then washed with PBS-0.05% Tween 20 and blocked with PBS-10% FBS at 37°C for 1 h. The serum was heat-inactivated at 56°C for 30 min prior to analysis. Two-fold serial dilutions of sera in PBS-10% FBS were then added to the blocked plates and incubated at 37°C for 1 h. After washing, horseradish peroxidase (HRP)-conjugated goat anti-mouse IgG antibody (diluted 1:20,000; BOSTER, Wuhan, China) in PBS-10% FBS was added to the wells and then incubated at 37°C for 1 h. After washing, ELISA was performed by adding a 1:1 mixture of tetramethylbenzidine and hydrogen peroxide. (Sangon Biotech, Shanghai, China) Color development was subsequently stopped with 2 N sulfuric acid, and the absorbance was measured at 450 nm on an Epoch Microplate Reader (Agilent Technologies, Santa Clara, CA, USA) with the reference filter set at 630 nm. There were 8 samples from each group, and three replicates were set up for each sample.
2.8 MPXV plaque reduction neutralization tests
Vero E6 cells were seeded 1 day prior to the experiment in 12-well plates (Corning, New York, NY, USA) at a density of 2 × 105 cells per well. Serum samples were heated at 56 °C for 30 min to inactivate complement, then 1:20 diluted, followed by a 4-fold serial dilution in DMEM supplemented with 2.5% FBS, 50 units/mL penicillin–streptomycin, with each serum sample then being incubated with 150 plaque-forming units of virus (PFU) at 37°C for 1 h. The virus-serum mixtures were added onto pre-formed Vero E6 cell monolayers and incubated at 37 °C for 1 h in a 5% CO2 incubator. Following this, the supernatant was removed, and the cell monolayers were covered with methylcellulose overlay (final concentration: DMEM with 0.9% methylcellulose, 2% FBS and 50 U/mL penicillin–streptomycin). After 96 h, the plates were fixed with an equal volume of 8% (Servicebio, Wuhan, China) paraformaldehyde for at least 1 h, with the cell monolayers being stained with 1% crystal violet, before the plaques being counted and photographed. Neutralizing antibody titers were defined as the highest serum dilution that resulted in 50% (PRNT50) in the number of virus plaques reduction. The PRNT50 was given a value of 10 when no neutralization was observed. There were 8 serum samples at post-prime, post-initial boost and post-second boost these 3 time points while there were only 5 serum samples after challenge.
2.9 IFN-γ ELISpot assay
Mice spleens were collected separately and ground on a 70 μm cell sieve rinsed with mouse lymphocyte separation medium (DAKEWE, Shenzhen, China). Single cell suspensions were subsequently collected in 15 mL centrifuge tubes, with 1 mL RPMI 1640 medium (Gibco, New York, NY, USA) containing 10% FBS (R10) being added. The samples were then centrifuged at 800 × g at RT for 30 min. Next, the lymphocyte layer was aspirated, before adding 10 mL R10 and washing upside down. This was then centrifuged at 250 × g at RT for 10 min before collecting the cells. Subsequently, cells were resuspended with R10 and then counted. ELISpot assays were performed using the Mouse IFN-γ ELISpotPLUS kit (MABTECH, Nacka, Sweden) in accordance with the manufacturer’s instructions. Subsequently, 400,000 splenocytes were plated into each well and stimulated for 30 h with A29L, M1R, A35R, and B6R, at 20 μg/mL each. R10 and concanavalin A (MedChem Express, Monmouth Junction, NJ, USA) were used for negative and positive controls respectively. Spots were scanned and quantified by ImmunoSpot CTL reader (Cellular Technology Limited, Cleveland, OH, USA), and spot-forming unit (SFU) per million cells was calculated by subtracting the negative control wells. There were 3 samples from each group, and three replicates were set up for each sample.
2.10 Quantitative real time PCR for the detection of MPXV
Whenever commercial kits were used, the manufacturer’s instructions were followed without modification. Each tissue sample was weighed to 50 mg and then homogenized in 1 mL PBS (pH 7.4). The homogenized samples were subsequently centrifuged at 5,000 × g at 4°C for 10 min. Thereafter, 200 μL of the supernatant from the centrifugation was used to extract viral DNA using the DAAN Nucleic Acid Extraction or Purification Kit (DAAN Gene, Guangzhou, China). Furthermore, qPCR was performed using QuantStudio 5 real-time PCR system (Thermo Fisher Scientific, Waltham, MA, USA) and MPXV nucleic acid detection kit targeting the F3L fragment (DAAN gene, Guangzhou, China). There were 5 samples from each group, and three replicates were set up for each sample.
2.11 Histopathological analysis
Tissues were fixed in 4% paraformaldehyde (Servicebio, Wuhan, China) and then embedded in paraffin, followed by staining with hematoxylin and eosin (Leica, Wetzlar, Germany). Stained slides were subsequently analyzed by veterinary pathologists who were blinded to study groups.
2.12 Statistical analysis
All statistical analyses were performed using GraphPad Prism 8 software (GraphPad Software, San Diego, CA, USA). Unpaired Student’s t-tests were used to compare these data, with significance being set at p < 0.05.
3 Results
3.1 Characterization of multiple recombinant MPXV vaccines from outer membrane proteins constructs and immunization in mice
MPXV proteins A29L, M1R, A35R, and B6R were measured using Western blot analysis, which revealed bands approximate to the predicted protein molecular weights of 8.4 kDa, 20.5 kDa, 14.6 kDa, and 29.6 kDa respectively (Figure 1A). The mice were vaccinated three times and challenged with MPXV on day 56, then animals were euthanasia and samples were collected 5 days later (Figures 1B, C). As a result of immunofluorescence studies, Vero E6 cells transfected with the His-tag can be detected with the mice sera from the vaccine group, whereas neither the QS-21 group, nor the PBS group. (Figure 1D). Spleen sections from mice in the vaccine group after immunization also showed a larger area of germinal centers observed compared to the QS-21 and PBS group (Figure 1E), indicating enhanced immune response.
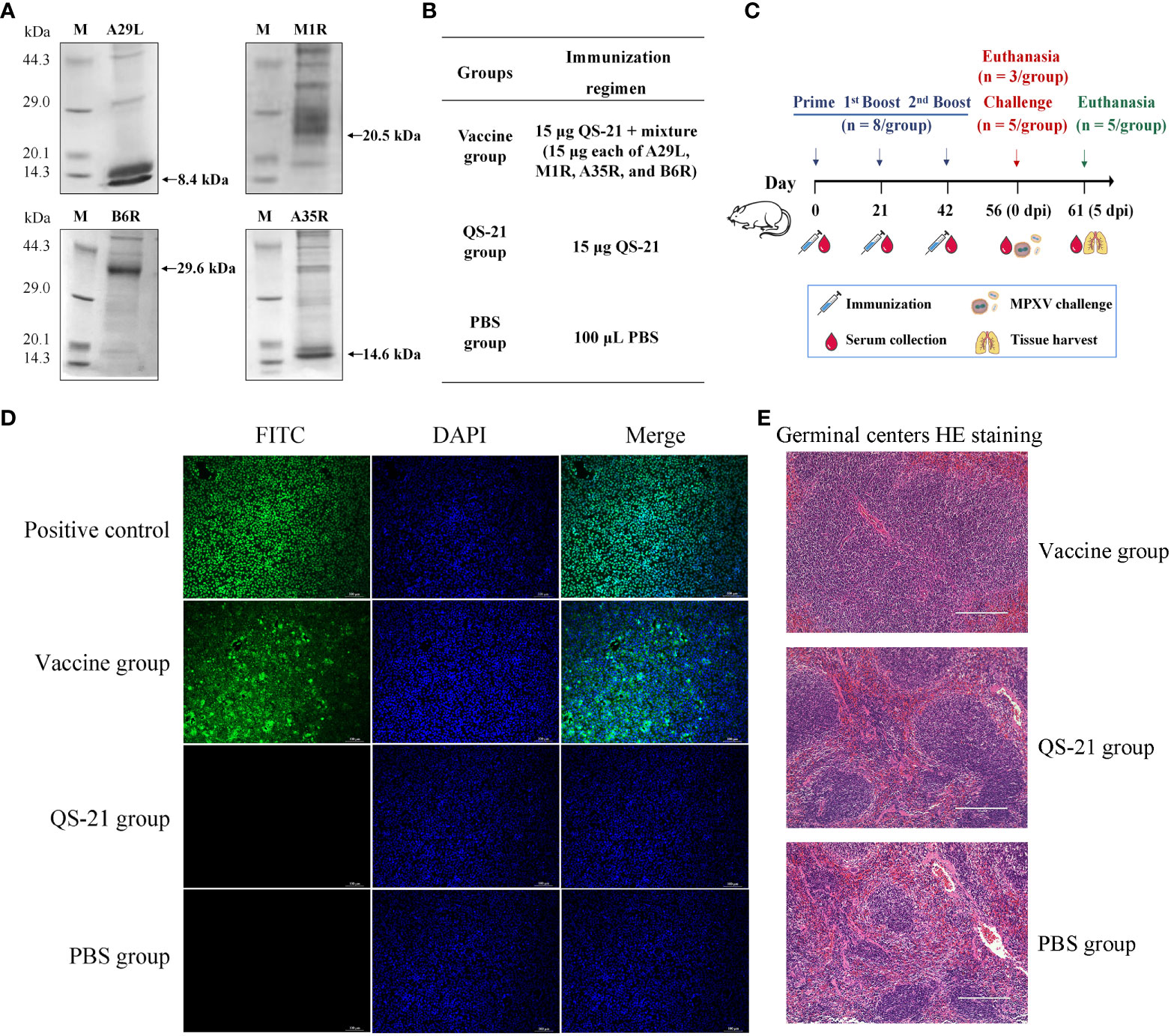
Figure 1 Characterization of multiple recombinant MPXV vaccines from outer membrane proteins constructs and immunization in mice. (A) Detection of MPXV A29L, A35R, M1R, and B6R proteins using western blot. (B) Experimental groups and immunisation regimens. (C) Schematic diagram of immunization regimens. Mice were injected subcutaneously on days 0, 21 and 42, n=8/group. Two weeks after the third immunization, mice were inoculated intranasally and intraperitoneally with MPXV, n=5/group. (D) Immunofluorescence staining with mouse serum to verify the expression of binding antibody. The primary antibody for the positive control was 6x His Tag Monoclonal Antibody, and for the rest of the group, mouse serum was collected after the second boost (D56). (E) HE staining of spleen sections from mice after the second boost (D56) to observe enlargement of the germinal centers induced by the immune response to vaccination.
3.2 Multiple recombinant MPXV vaccines induced high-titer antibody responses
To evaluate the immunogenicity of multiple recombinant MPXV vaccines, mice were inoculated subcutaneously with each protein in QS-21 adjuvant at 3-week intervals. The endpoint ELISA titers of the sera were also determined using plates coated with individual recombinant proteins. After the prime immunization, binding antibodies induced by A29L and B6R were either undetectable or minimal, whereas those induced by A35R and M1R were relatively high, yet all four proteins produced low levels of binding antibodies (Figures 2A, D). After the initial boost, antibodies rose sharply, although the antibodies induced by A35R and M1R were still significantly higher than those from A29L and B6R (Figures 2B, E). After the second boost, A35R and M1R-induced antibody levels did not change nearly, whereas the B6R-induced antibodies continued to increase, reaching the same levels as the A35R and M1R. Meanwhile, the A29L-induced antibody level also continued to rise, although it remained lower than the other three (Figures 2C, F). The immunogenicity of A35R and M1R was also superior to A29L and B6R, considering the higher levels of binding antibodies at each stage of immunization.
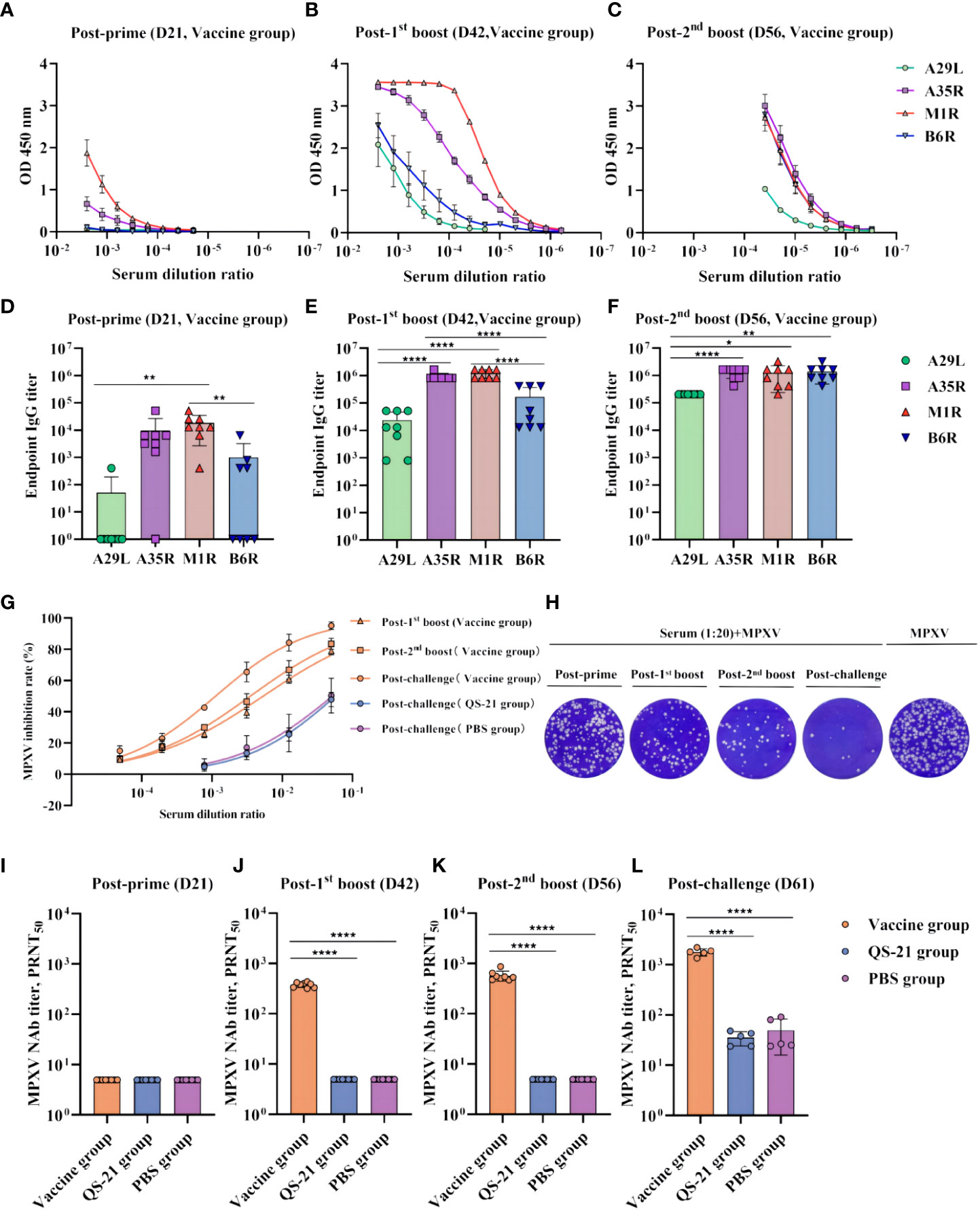
Figure 2 Humoral immune responses in multiple recombinant vaccine immunization in mice. (A–C) Measurement of serum on the day of post-prime, post-initial boost, post-second boost, and post-challenge (D21, D42, D56, and D61) binding antibodies against MPXV A29L, A35R, M1R, and B6R proteins by ELISA. Data shown represent mean OD 450 nm values (mean + SD) for each group of eight mice, n = 8/group. (D–F) Endpoint IgG titers by ELISA, n = 8/group. (G) Inhibition rate of MPXV virus by neutralizing antibodies in serum as measured by PRNT, n = 8/group at post-prime, post-initial boost, post-second boost, n = 5/group at post-challenge. (H) Plaque reduction assay for MPXV by serum at a dilution of 1:20. (I–L) PRNT of neutralizing antibodies against MPXV live viruses on the day of post-prime, post-initial boost, post-second boost, and post-challenge (D21, D42, D56, and D61). Data shown are geometric mean titers and are mean plus SD. Data were analyzed by one-way ANOVA with a multiple comparison test, n = 8/group at post-prime, post-initial boost, post-second boost, n = 5/group at post-challenge. P < 0.05 was considered statistically significant. *P < 0.05, **P < 0.01, ***P < 0.001, ****P < 0.0001.
The level of neutralizing antibodies was assessed by PRNT assay with live MPXV in the serum at each stage after vaccination, which represented the protective effect of the vaccine against MPXV virus in mice (Figures 2G, H). The results showed that neutralizing antibody levels were so low as to be undetectable after prime immunization (Figure 2I). However, after the first boost, neutralizing antibody titers of all eight vaccinated mice reached 300 (Figure 2J). Furthermore, after the second boost, the geometric mean of neutralizing antibody titer in the eight mice was 574, with the highest being 865 (Figure 2K). Notably, after virus challenge, there was a significant neutralizing antibody increase in the vaccine group, with all five mice exceeding 1300 and the highest being 2188. Neutralizing antibodies were also detected in the QS-21 and PBS groups, representing a normal functioning immune system in all mice, the virus stimulated mice to produce neutralizing antibodies, but the antibody titer did not exceed 100 (Figure 2L).
3.3 Immunization of mice with multiple recombinant MPXV vaccines induced strong cellular immune responses
T cell responses against MPXV proteins A29L, M1R, A35R, and B6R were detected by IFN-γ ELISpot. Three mice from each group were euthanized 2 weeks after receiving the second boost, and the splenocytes were harvested. A single-cell suspension was stimulated for 30 h with recombinant proteins of A29L, M1R, A35R, and B6R. As expected, the vaccine induced significantly higher levels of IFN-γ + T cells compared to the QS-21 and PBS groups on day 56 (Figures 3A, B). Average 690 spots in the vaccine group, 101 spots in the QS-21 group, and 17 spots in the PBS group.
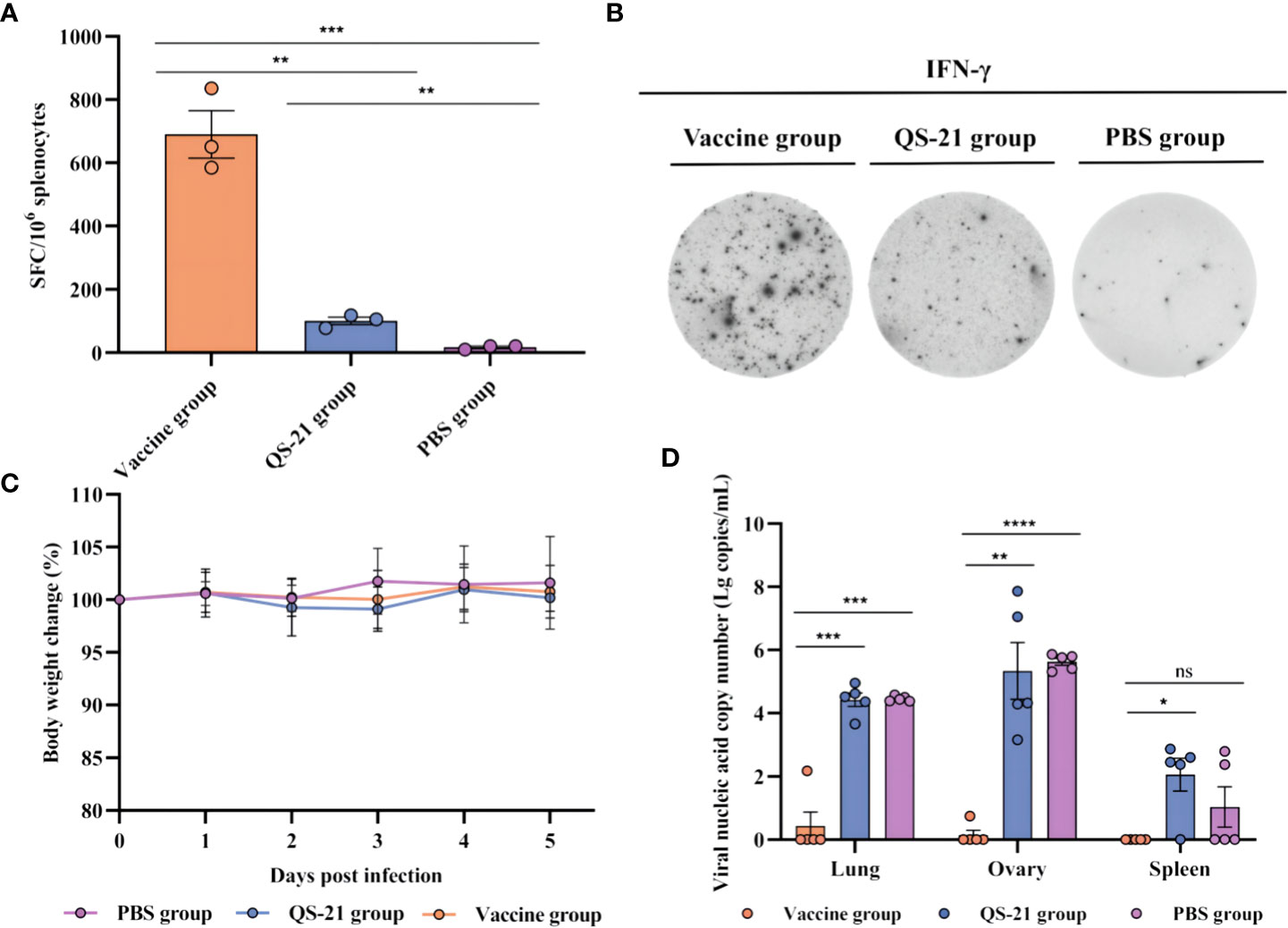
Figure 3 Cellular immune responses and protection against challenges of MPXV in multiple recombinant vaccine immunization in mice. (A, B) T cell responses against MPXV proteins A29L, M1R, A35R, and B6R as measured by IFN-γ ELISpot. Three mice from each group were euthanized 2 weeks after the second boost, before splenocytes were harvested, and a single-cell suspension was stimulated for 30 h with recombinant proteins of A29L, M1R, A35R, and B6R, n = 3/group, each sample contained three replicates. (C) Body weight changes following virus challenges, n = 5/group. (D) qPCR detection of viral loads in the lungs, ovaries, and spleen. Data shown are mean plus SD. Data were analyzed by one-way ANOVA with a multiple comparison test. P < 0.05 was considered statistically significant, n = 5/group, each sample contained three replicates. * P < 0.05, ** P < 0.01, *** P < 0.001, **** P < 0.0001.
3.4 Multiple recombinant MPXV vaccines significantly inhibited virus proliferation
Some literature states that MPXV multiplies in BALB/c mice and peaks on days 4–7, and the highest viral loads were observed in the ovaries and lungs, followed by progressive clearance, and viral challenge had no major effect on body weight or the mental status of the mice (28, 29, 31). We therefore chose to euthanize mice on day 5 of virus infection. Body weight data from our experiments showed the same trend, with no significant differences between any of the three groups of mice (Figure 3C). Additionally, qPCR results showed significant differences in viral load within the lungs, ovaries, and spleens of the vaccine group mice compared to the QS-21 group, as well as in the lungs and ovaries of the vaccine group mice compared to the PBS group. The highest amount of virus detected in the tissues of mice in the vaccine group was 102.2 copies/mL, while the highest amount of virus detected in the tissues of mice in the QS-21 group reached 107.9 copies/mL for the ovaries. The highest viral load in the PBS group was also in the ovaries, reached 105.9 copies/mL (Figure 3D). The results of the attack-protection assay sufficiently demonstrate the ability of our vaccine to inhibit the multiplication of the virus in mice.
3.5 Histopathological changes in mice infected with MPXV
All mice showed histopathological changes in the lungs on day 5 after the challenge, including five mice in the vaccine group, thus indicating that virus infection had occurred. However, the extent of the lesions in the vaccine group was not as severe as observed in the control group. Mice in the PBS and QS-21 groups showed extensive alveolar cell necrosis with significant congestion in the necrotic alveoli, alongside a large infiltration of lymphocytes and neutrophils. Additionally, some of the alveolar walls were thickened, congested, and edematous. Additionally, the alveolar septum was significantly widened, and the blood vessels were dilated and congested and interstitial was infiltrated by a large number of inflammatory cells. In contrast, the histopathological changes in the lungs of the mice in the vaccine group were generally dilated and congested lung vessels, with a small amount of inflammatory cell infiltration in the interstitial and slight thickening of the alveolar walls (Figure 4).
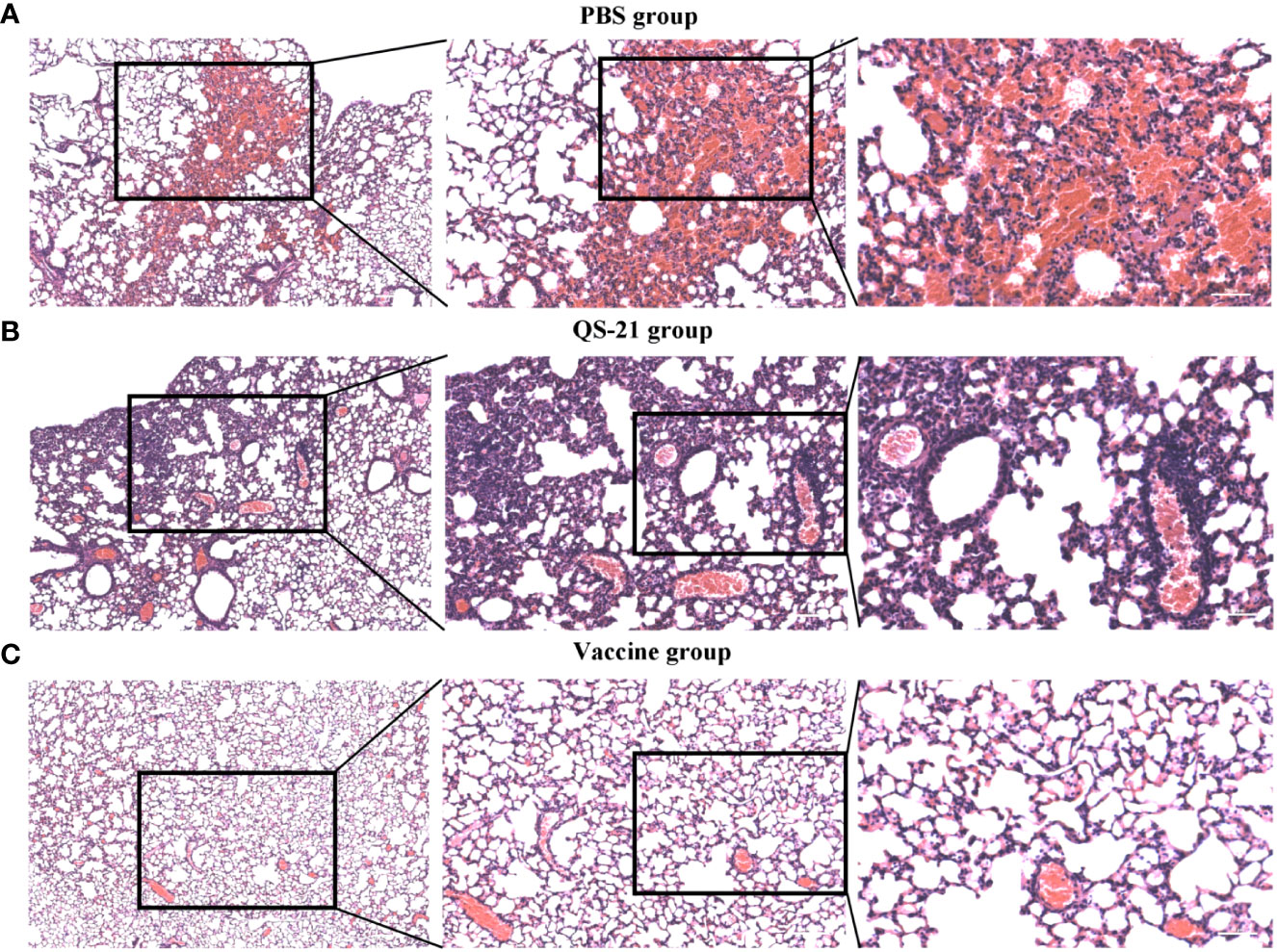
Figure 4 HE staining of lung sections from mice after virus challenge to visualize lung lesions. (A) Lung sections of mice in PBS group. (B) Lung sections of mice in QS-21 group. (C) Lung sections of mice in vaccine group. Scale bars from left to right are 200 μm, 100 μm, 50 μm.
4 Discussion
Following the occurrence of the 2022 mpox epidemic, attention quickly shifted to the development of a mpox vaccine based on the lessons learned from the COVID-19 outbreak. Although previous studies have shown that smallpox vaccines offer some protection against both MPXV and current mutant strains, this also raised similar concerns around the safety of an attenuated smallpox virus vaccine. In contrast, the WHO has declared the smallpox virus to be extinct and it is less feasible to reproduce an attenuated smallpox vaccine. According to Madeley CR, inactivated vaccinia virus vaccine does not produce satisfactory immunity (32). Inactivated mpox vaccines are unable to stimulate sufficient immunity in the body, since there are many important neutralizing epitopes on the EEV particles of MPXV, and inactivation of the virus would lose these important antigens. Protein subunit vaccines have an excellent safety profile and can provide good protection when combined with adjuvants for immunization. Another important point is that the 2022 mpox epidemic was concentrated within the homosexual community, which is also vulnerable to a higher prevalence of AIDS (33–38). The immunodeficiency caused by AIDS can prevent them from being immunized with live attenuated vaccines, which provides a powerful shock to their immune system (39–42), and therefore a recombinant protein vaccine may be a better option for them. In this study, we demonstrate that multiple recombinant vaccines can trigger antibodies against both IMV and EEV forms of MPXV. Three immunizations of mice with a recombinant protein-linked QS-21 adjuvant activated cellular and humoral immunities and induced high titers of binding antibodies, however, against each protein at different titers. More importantly, the neutralizing antibodies induced by this vaccine sufficiently inhibited the multiplication of the virus, while protecting the mice from persistent infection by MPXV. In addition, the level of antibodies correlates with the number of immunizations and this result may further guide the designation of immunization strategies in the future.
IFN-γ plays an important role in the induction of antiviral immunity as a cytokine secreted by immunoreactive cells. Once Th1 cells have matured, they secrete IFN-γ and promote more Th1 cell differentiation. IFN-γ secreted by Th1 cells activates macrophages, while also stimulating B cells to produce receptors that enhance viral attachment to macrophages (43). IFN is one of the primary innate mediators after viral infection, and susceptibility to and severity of the infection are both increased when IFN is insufficient (44). The ability of mouse splenocytes to secrete IFN-γ was significantly stronger within the vaccine group than in the control group, as measured by ELISpot, thus indicating that the vaccine elicited a strong T-cell immune response, as indicated by the increased size and number of spleen germinal centers in mice. Although protein vaccines generally require multiple immunizations, the high titer of binding antibodies produced by three immunizations of A29L, M1R, A35R, and B6R mixed with QS-21 demonstrated good immunogenicity of this protein vaccine. After the second boost, the geometric mean of neutralizing antibody titer in the eight mice was 574, with the highest being 865. An article published in November 2022 on the MPXV mRNA vaccine also chose BALB/c mice as experimental animals and the encoded proteins chosen were A35R and M1R, which were immunized twice on days 0 and 14, and serum was collected on day 35, and both vaccine groups (VGPox 1 and VGPox 2) showed over 80% inhibition at a dilution of 1:500 (45). Another MPXV DNA vaccine based on vaccinia virus genes (L1R, A27L, A33R, and B5R) produced a mean titer of 9600 conjugated antibodies and a mean titer of 373 neutralizing antibodies in an monkey model (46). And there are just as many reviews of attenuated vaccines. Hatch GJ (47) compared ACAM-2000, a second-generation smallpox vaccine manufactured by Dryvax/NYCBH, with Imvamune, a third-generation smallpox vaccine manufactured by Bavarian Nordic, on a crab-eating monkey model. After vaccination with ACAM-2000, the maximum median titer at 6 days before challenge was 132 U/ml animals receiving two Imvamune boosters reached 69 U/ml at 6 days before challenge, which was significantly higher than the titer in the single-dose Imvamune group (P < 0.05). In the MPXV challenge, all monkeys immunized with ACAM-2000 and twice with Imvamune survived, all control monkeys died, while two monkeys died in the group immunized with only one dose of Imvamune. Another article directly compares the efficacies of MVA alone and in combination with classical VV-based vaccines in a cynomolgus macaque mpox model. Four vaccine strains, MVA-BN, IMVAMUNE, Elstree-RIVM and Elstree-BN, were included. However, the neutralization titers at 9 weeks after last vaccination were low, with MVA-BN, IMVAMUNE and Elstree-BN not exceeding 100 (48). Although attenuated vaccines do not show a significant advantage in stimulating the production of neutralizing antibodies in the organism, this may be due to large differences in animal models and evaluation methods between studies that do not facilitate comparison. One aspect of note is that Several studies have shown that antibodies persist in the host for several years after attenuated smallpox vaccination (49–51) and this will be an important part of future evaluations of subunit vaccines such as recombinant protein and nucleic acid vaccines. Furthermore, it is interesting to note that according to our results, the titer of binding antibodies against A29L was consistently the lowest; According to Hooper JW, vaccination with A27L (homologous to mpox virus A29L) alone failed to protect mice from vaccinia virus (52). Future experiments should be designed aiming to verify whether A29L plays a key role in this vaccine.
We believe that the antibody targeting strategy for the MPXV contributed significantly to the protection of protein-immune mice from infection. Antibodies produced by immunized mice prevented virus recognition, entry into host cells, and the spread of mature viruses between cells. Protein immunization alone does not completely protect mice from a viral infection, since the antibodies they produce may only act on one stage of viral infection. In vitro testing of immune sera to neutralize IMV showed that a combination of A29L-, M1R-, A35R-, and B6R-specific antibodies was very effective in inactivating the MPXV. This was also supported by the results of the mpox virus attack challenge. Furthermore, our results are consistent with those published by Osorio JE (29), whereby MPXV was replicated heavily in the ovaries, lungs, and spleens of mice, and high viral loads were detected in the tissues of control mice and were almost undetectable in those of vaccinated mice. We believe that neutralizing antibodies in vaccine group mice protect them from MPXV challenge. Notably, the serum levels of neutralizing antibodies in the vaccine group of mice that received the virus challenge were substantially elevated, with an average antibody titer of 1785 in the five mice. The neutralizing antibody titer after the second boost was 574, with an average threefold increase observed within 5 days of the virus challenge, again indicating that the three prior immunizations had established a complete immune system response in the mice against MPXV. Therefore, antibody titers increased 3-fold upon exposure to a large number of viral attacks, and this increase occurred even after several neutralizing antibodies had bound to the antigen and been cleared together. Although this vaccine does not prevent the virus from causing histopathological changes in the lung, it induces antibodies that neutralize the virus and moderate tissue damage from the virus by reducing the virus titer and area of infection. This reinforced the fact that the vaccine provided complete protection in mice from MPXV persistent infection.
A combination of these four proteins and QS-21 provided complete protection, although which specific protein played the key role in immune protection was not investigated in this study, thus more research is required to explore the specificity of the antibody response. However, depending on the ELISA results, the immunization dose of each protein could be optimized to achieve optimal immunization. In terms of the cellular immune response, we were unable to schedule ELISpot assay for the four proteins stimulated individually to further evaluate the individual effect of each protein in the ability of immune cells to produce IFN-γ, considering the insufficient total number of spleen cells. All of the above await our further adjustment and evaluation of this vaccine in the future. In conclusion, this study demonstrated the feasibility of a multiple recombinant MPXV vaccination with A29L, M1R, A35R, and B6R.
Data availability statement
The original contributions presented in the study are included in the article/Supplementary Material. Further inquiries can be directed to the corresponding authors.
Ethics statement
The animal study was reviewed and approved by the animal ethics committee of WIBP (Ethics No. WIBP-A II 442022006). Wuhan Institute of Biological Products Co., Ltd., Wuhan City, China.
Author contributions
XGL, ZW, and JL designed and supervised the study. DT, XKL, HF, XX, KS, RW, CL, DD, and HD performed the experiments. DT, XKL and JL performed the statistical analysis. DT and XKL wrote the manuscript. XGL, ZW, and JL revised the manuscript. All authors contributed to the article and approved the submitted version.
Funding
This work was supported by The National Key Research and Development Program of China(2023YFC0871900).
Acknowledgments
The authors would like to thank Nanjing Vazyme Biotechnology Co. for helping us with the purified protein and QZ of the Huazhong Agricultural University for help with the production of spleen germinal center sections.
Conflict of interest
All authors were employed by the company Wuhan Institute of Biological Products Co., Ltd.
Publisher’s note
All claims expressed in this article are solely those of the authors and do not necessarily represent those of their affiliated organizations, or those of the publisher, the editors and the reviewers. Any product that may be evaluated in this article, or claim that may be made by its manufacturer, is not guaranteed or endorsed by the publisher.
Supplementary material
The Supplementary Material for this article can be found online at: https://www.frontiersin.org/articles/10.3389/fimmu.2023.1203410/full#supplementary-material
References
1. Petersen E, Kantele A, Koopmans M, Asogun D, Yinka-Ogunleye A, Ihekweazu C, et al. Human monkeypox: epidemiologic and clinical characteristics, diagnosis, and prevention. Infect Dis Clin North Am (2019) 33(4):1027–43. doi: 10.1016/j.idc.2019.03.001
2. Papukashvili D, Rcheulishvili N, Liu C, Wang X, He Y, Wang PG. Strategy of developing nucleic acid-based universal monkeypox vaccine candidates. Front Immunol (2022) 13:1050309. doi: 10.3389/fimmu.2022.1050309
3. Isidro J, Borges V, Pinto M, Sobral D, Santos JD, Nunes A, et al. Phylogenomic characterization and signs of microevolution in the 2022 multi-country outbreak of monkeypox virus. Nat Med (2022) 28(8):1569–72. doi: 10.1038/s41591-022-01907-y
4. Fulginiti VA, Papier A, Lane JM, Neff JM, Henderson DA. Smallpox vaccination: a review, part ii. adverse events. Clin Infect Dis (2003) 37(2):251–71. doi: 10.1086/375825
5. Rizk JG, Lippi G, Henry BM, Forthal DN, Rizk Y. Prevention and treatment of monkeypox. Drugs (2022) 82(9):957–63. doi: 10.1007/s40265-022-01742-y
6. Cid R, Bolivar J. Platforms for production of protein-based vaccines: from classical to next-generation strategies. Biomolecules (2021) 11(8). doi: 10.3390/biom11081072
7. Henderson DA. The looming threat of bioterrorism. Science (1999) 283(5406):1279–82. doi: 10.1126/science.283.5406.1279
8. Putz MM, Midgley CM, Law M, Smith GL. Quantification of antibody responses against multiple antigens of the two infectious forms of vaccinia virus provides a benchmark for smallpox vaccination. Nat Med (2006) 12(11):1310–5. doi: 10.1038/nm1457
9. Smith GL, Law M. The exit of vaccinia virus from infected cells. Virus Res (2004) 106(2):189–97. doi: 10.1016/j.virusres.2004.08.015
10. Gilchuk I, Gilchuk P, Sapparapu G, Lampley R, Singh V, Kose N, et al. Cross-neutralizing and protective human antibody specificities to poxvirus infections. Cell (2016) 167(3):684–94.e9. doi: 10.1016/j.cell.2016.09.049
11. Hirao LA, Draghia-Akli R, Prigge JT, Yang M, Satishchandran A, Wu L, et al. Multivalent smallpox DNA vaccine delivered by intradermal electroporation drives protective immunity in nonhuman primates against lethal monkeypox challenge. J Infect Dis (2011) 203(1):95–102. doi: 10.1093/infdis/jiq017
12. Perdiguero B, Blasco R. Interaction between vaccinia virus extracellular virus envelope A33 and B5 glycoproteins. J Virol (2006) 80(17):8763–77. doi: 10.1128/JVI.00598-06
13. Fang M, Cheng H, Dai Z, Bu Z, Sigal LJ. Immunization with a single extracellular enveloped virus protein produced in bacteria provides partial protection from a lethal orthopoxvirus infection in a natural host. Virology (2006) 345(1):231–43. doi: 10.1016/j.virol.2005.09.056
14. Law M, Smith GL. Antibody neutralization of the extracellular enveloped form of vaccinia virus. Virology (2001) 280(1):132–42. doi: 10.1006/viro.2000.0750
15. Bell E, Shamim M, Whitbeck JC, Sfyroera G, Lambris JD, Isaacs SN. Antibodies against the extracellular enveloped virus B5r protein are mainly responsible for the eev neutralizing capacity of vaccinia immune globulin. Virology (2004) 325(2):425–31. doi: 10.1016/j.virol.2004.05.004
16. Shchelkunov SN, Totmenin AV, Safronov PF, Mikheev MV, Gutorov VV, Ryazankina OI, et al. Analysis of the monkeypox virus genome. Virology (2002) 297(2):172–94. doi: 10.1006/viro.2002.1446
17. Kensil CR, Kammer R. Qs-21: a water-soluble triterpene glycoside adjuvant. Expert Opin Investig Drugs (1998) 7(9):1475–82. doi: 10.1517/13543784.7.9.1475
18. Lacaille-Dubois MA. Updated insights into the mechanism of action and clinical profile of the immunoadjuvant qs-21: a review. Phytomedicine (2019) 60:152905. doi: 10.1016/j.phymed.2019.152905
19. Kensil CR, Patel U, Lennick M, Marciani D. Separation and characterization of saponins with adjuvant activity from quillaja saponaria Molina cortex. J Immunol (1991) 146(2):431–7. doi: 10.4049/jimmunol.146.2.431
20. Ragupathi G, Gardner JR, Livingston PO, Gin DY. Natural and synthetic saponin adjuvant qs-21 for vaccines against cancer. Expert Rev Vaccines (2011) 10(4):463–70. doi: 10.1586/erv.11.18
21. Sun HX, Xie Y, Ye YP. Advances in saponin-based adjuvants. Vaccine (2009) 27(12):1787–96. doi: 10.1016/j.vaccine.2009.01.091
22. Garcon N, Chomez P, Van Mechelen M. Glaxosmithkline adjuvant systems in vaccines: concepts, achievements and perspectives. Expert Rev Vaccines (2007) 6(5):723–39. doi: 10.1586/14760584.6.5.723
23. Kashala O, Amador R, Valero MV, Moreno A, Barbosa A, Nickel B, et al. Safety, tolerability and immunogenicity of new formulations of the plasmodium falciparum malaria peptide vaccine Spf66 combined with the immunological adjuvant qs-21. Vaccine (2002) 20(17-18):2263–77. doi: 10.1016/s0264-410x(02)00115-9
24. Tesh RB, Watts DM, Sbrana E, Siirin M, Popov VL, Xiao SY. Experimental infection of ground squirrels (Spermophilus tridecemlineatus) with monkeypox virus. Emerg Infect Dis (2004) 10(9):1563–7. doi: 10.3201/eid1009.040310
25. Langohr IM, Stevenson GW, Thacker HL, Regnery RL. Extensive lesions of monkeypox in a prairie dog (Cynomys sp). Vet Pathol (2004) 41(6):702–7. doi: 10.1354/vp.41-6-702
26. Xiao SY, Sbrana E, Watts DM, Siirin M, da Rosa AP, Tesh RB. Experimental infection of prairie dogs with monkeypox virus. Emerg Infect Dis (2005) 11(4):539–45. doi: 10.3201/eid1104.040907
27. Schultz DA, Sagartz JE, Huso DL, Buller RM. Experimental infection of an African dormouse (Graphiurus kelleni) with monkeypox virus. Virology (2009) 383(1):86–92. doi: 10.1016/j.virol.2008.09.025
28. Americo JL, Moss B, Earl PL. Identification of wild-derived inbred mouse strains highly susceptible to monkeypox virus infection for use as small animal models. J Virol (2010) 84(16):8172–80. doi: 10.1128/JVI.00621-10
29. Osorio JE, Iams KP, Meteyer CU, Rocke TE. Comparison of monkeypox viruses pathogenesis in mice by in vivo imaging. PloS One (2009) 4(8):e6592. doi: 10.1371/journal.pone.0006592
30. Wenbin Tuo DZ. Qs-21: a potent vaccine adjuvant. Natural Products Chem Res (2015) 03(04). doi: 10.4172/2329-6836.1000e113
31. Hutson CL, Abel JA, Carroll DS, Olson VA, Braden ZH, Hughes CM, et al. Comparison of West African and Congo basin monkeypox viruses in Balb/C and C57bl/6 mice. PloS One (2010) 5(1):e8912. doi: 10.1371/journal.pone.0008912
32. Madeley CR. The immunogenicity of heat-inactivated vaccinia virus in rabbits. J Hyg (Lond) (1968) 66(1):89–107. doi: 10.1017/s0022172400040973
33. Khaity A, Hasan H, Albakri K, Elsayed H, HA HA, Islam F, et al. Monkeypox from Congo 1970 to Europe 2022; is there a difference? Int J Surg (2022) 104:106827. doi: 10.1016/j.ijsu.2022.106827
34. Bragazzi NL, Kong JD, Mahroum N, Tsigalou C, Khamisy-Farah R, Converti M, et al. Epidemiological trends and clinical features of the ongoing monkeypox epidemic: a preliminary pooled data analysis and literature review. J Med Virol (2023) 95(1):e27931. doi: 10.1002/jmv.27931
35. Inigo Martinez J, Gil Montalban E, Jimenez Bueno S, Martin Martinez F, Nieto Julia A, Sanchez Diaz J, et al. Monkeypox outbreak predominantly affecting men who have sex with men, Madrid, Spain, 26 April to 16 June 2022. Euro Surveill (2022) 27(27). doi: 10.2807/1560-7917.ES.2022.27.27.2200471
36. Patel A, Bilinska J, Tam JCH, Da Silva Fontoura D, Mason CY, Daunt A, et al. Clinical features and novel presentations of human monkeypox in a central London centre during the 2022 outbreak: descriptive case series. BMJ (2022) 378:e072410. doi: 10.1136/bmj-2022-072410
37. Ogoina D, Iroezindu M, James HI, Oladokun R, Yinka-Ogunleye A, Wakama P, et al. Clinical course and outcome of human monkeypox in Nigeria. Clin Infect Dis (2020) 71(8):e210–e4. doi: 10.1093/cid/ciaa143
38. Boesecke C, Monin MB, van Bremen K, Schlabe S, Hoffmann C. Severe monkeypox-virus infection in undiagnosed advanced hiv infection. Infection (2022) 50(6):1633–4. doi: 10.1007/s15010-022-01901-z
39. Jordan R, Leeds JM, Tyavanagimatt S, Hruby DE. Development of St-246(R) for treatment of poxvirus infections. Viruses (2010) 2(11):2409–35. doi: 10.3390/v2112409
40. Centers for Disease Control and Prevention. Progressive vaccinia in a military smallpox vaccinee - united states, 2009 (2009). Available at: https://www.cdc.gov/mmwr/preview/mmwrhtml/mm58e0519a1.htm (Accessed March 18, 2023).
41. Centers for Disease Control and Prevention. Clinical guidance for smallpox vaccine use in a postevent vaccination program . (2015). Available at: https://www.cdc.gov/mmwr/preview/mmwrhtml/rr6402a1.htm (Accessed March 18, 2023).
42. Greenberg RN, Overton ET, Haas DW, Frank I, Goldman M, von Krempelhuber A, et al. Safety, immunogenicity, and surrogate markers of clinical efficacy for modified vaccinia Ankara as a smallpox vaccine in hiv-infected subjects. J Infect Dis (2013) 207(5):749–58. doi: 10.1093/infdis/jis753
43. Estep RD, Messaoudi I, O'Connor MA, Li H, Sprague J, Barron A, et al. Deletion of the monkeypox virus inhibitor of complement enzymes locus impacts the adaptive immune response to monkeypox virus in a nonhuman primate model of infection. J Virol (2011) 85(18):9527–42. doi: 10.1128/JVI.00199-11
44. Earl PL, Americo JL, Moss B. Lethal monkeypox virus infection of Cast/Eij mice is associated with a deficient gamma interferon response. J Virol (2012) 86(17):9105–12. doi: 10.1128/JVI.00162-12
45. Fujun Hou YZ, Liu X, Murad Y, Xu J, Yu Z, Hua X, et al. Novel mrna vaccines encoding monkeypox virus M1r and A35r protect mice from a lethal virus challenge. bioRxiv (2022). doi: 10.1101/2022.11.19.517190
46. Hooper JW, Thompson E, Wilhelmsen C, Zimmerman M, Ichou MA, Steffen SE, et al. Smallpox DNA vaccine protects nonhuman primates against lethal monkeypox. J Virol (2004) 78(9):4433–43. doi: 10.1128/jvi.78.9.4433-4443.2004
47. Hatch GJ, Graham VA, Bewley KR, Tree JA, Dennis M, Taylor I, et al. Assessment of the protective effect of imvamune and Acam2000 vaccines against aerosolized monkeypox virus in cynomolgus macaques. J Virol (2013) 87(14):7805–15. doi: 10.1128/JVI.03481-12
48. Stittelaar KJ, van Amerongen G, Kondova I, Kuiken T, van Lavieren RF, Pistoor FH, et al. Modified vaccinia virus Ankara protects macaques against respiratory challenge with monkeypox virus. J Virol (2005) 79(12):7845–51. doi: 10.1128/JVI.79.12.7845-7851.2005
49. Zeng Y, Liu X, Li Y, Lu J, Wu Q, Dan D, et al. The assessment on cross immunity with smallpox virus and antiviral drug sensitivity of the isolated mpox virus strain wibp-Mpxv-001 in China. Emerg Microbes Infect (2023) 12(1):2208682. doi: 10.1080/22221751.2023.2208682
50. Hammarlund E, Lewis MW, Carter SV, Amanna I, Hansen SG, Strelow LI, et al. Multiple diagnostic techniques identify previously vaccinated individuals with protective immunity against monkeypox. Nat Med (2005) 11(9):1005–11. doi: 10.1038/nm1273
51. Taub DD, Ershler WB, Janowski M, Artz A, Key ML, McKelvey J, et al. Immunity from smallpox vaccine persists for decades: a longitudinal study. Am J Med (2008) 121(12):1058–64. doi: 10.1016/j.amjmed.2008.08.019
Keywords: mpox virus, recombinant protein, vaccination, antibody response, virus challenge
Citation: Tang D, Liu X, Lu J, Fan H, Xu X, Sun K, Wang R, Li C, Dan D, Du H, Wang Z, Li X and Yang X (2023) Recombinant proteins A29L, M1R, A35R, and B6R vaccination protects mice from mpox virus challenge. Front. Immunol. 14:1203410. doi: 10.3389/fimmu.2023.1203410
Received: 10 April 2023; Accepted: 13 June 2023;
Published: 26 June 2023.
Edited by:
Rama Rao Amara, Emory University, United StatesReviewed by:
Dmitriy Shcherbakov, Altai State University, RussiaNanda Kishore Routhu, Emory University, United States
Copyright © 2023 Tang, Liu, Lu, Fan, Xu, Sun, Wang, Li, Dan, Du, Wang, Li and Yang. This is an open-access article distributed under the terms of the Creative Commons Attribution License (CC BY). The use, distribution or reproduction in other forums is permitted, provided the original author(s) and the copyright owner(s) are credited and that the original publication in this journal is cited, in accordance with accepted academic practice. No use, distribution or reproduction is permitted which does not comply with these terms.
*Correspondence: Zejun Wang, d2FuZ3planVuQHNpbm9waGFybS5jb20=; Xinguo Li, TGl4aW5ndW9Ac2lub3BoYXJtLmNvbQ==; Xiaoming Yang, eWFuZ3hpYW9taW5nQHNpbm9waGFybS5jb20=
†These authors have contributed equally to this work and share first authorship