- 1NextCure Inc., Beltsville, MD, United States
- 2Nordic Bioscience A/S, Herlev, Denmark
It has been known for decades that the tumor extracellular matrix (ECM) is dysfunctional leading to loss of tissue architecture and promotion of tumor growth. The altered ECM and tumor fibrogenesis leads to tissue stiffness that act as a physical barrier to immune cell infiltration into the tumor microenvironment (TME). It is becoming increasingly clear that the ECM plays important roles in tumor immune responses. A growing body of data now indicates that ECM components also play a more active role in immune regulation when dysregulated ECM components act as ligands to interact with receptors on immune cells to inhibit immune cell subpopulations in the TME. In addition, immunotherapies such as checkpoint inhibitors that are approved to treat cancer are often hindered by ECM changes. In this review we highlight the ways by which ECM alterations affect and regulate immunity in cancer. More specifically, how collagens and major ECM components, suppress immunity in the complex TME. Finally, we will review how our increased understanding of immune and immunotherapy regulation by the ECM is leading towards novel disruptive strategies to overcome immune suppression.
1 Introduction
Tumors consists of cancer cells and their immediate environment, the tumor microenvironment (TME) (1). The TME is a heterogeneous amalgamation of non-malignant stromal cells, immune cells, secreted factors, and the tumor extracellular matrix (ECM). An overview of the TME is shown in Figure 1. It is now established that for anti-cancer treatment to be successful, therapeutics needs to not only eradicate the cancer cells, but it is equally important also target the TME, for example by modulating stromal cell activity, immune cell activity and phenotype, and by interfering with ECM-cell receptor interactions (2–7). Reprogramming of the immune response from pro-tumorigenic to anti-tumorigenic is an essential component for therapeutic success and understanding the drivers of an immunosuppressive environment will help advance the field (8). It is now evident that the ECM is a major player in facilitating both tumor progression and resistance to various treatments, including immunotherapy (9–13).
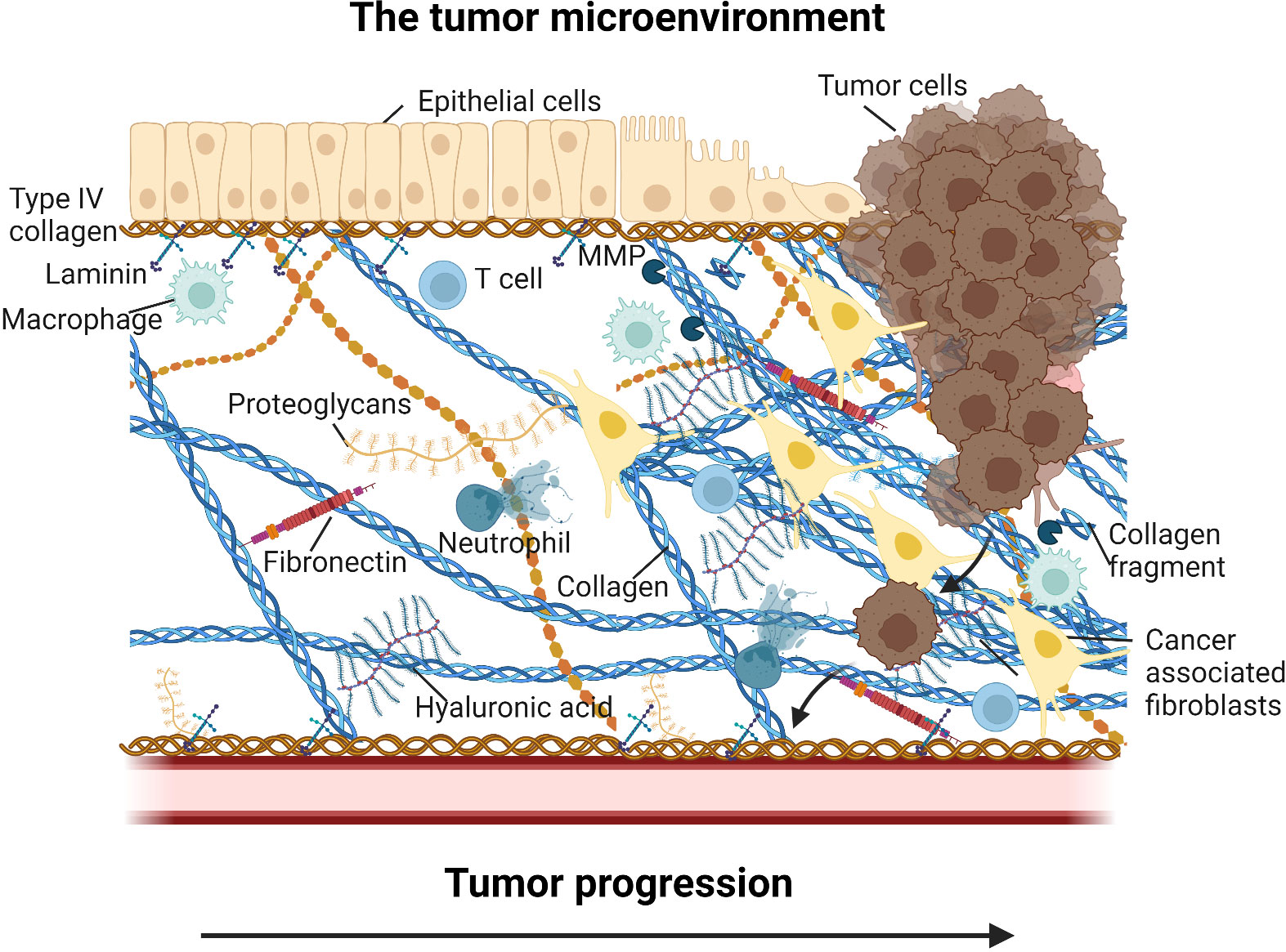
Figure 1 Overview of the tumor microenvironment (TME). In addition to the tumor cells, the TME consist of non-malignant stromal cells such as cancer associated fibroblasts, immune cells such as tumor associated macrophages, tumor associated neutrophils, T cells, secreted factors such proteases, cytokines and growth factors, and the tumor extracellular matrix (ECM). The ECM include core components such as collagens, fibronectin, hyaluronan, and laminins, and a wide array of proteoglycans and associated molecules. Tumor ECM changes may alter ECM-cell interactions and thereby prevent T cell recruitment to the tumor cells (immune exclusion) and drive immune reprogramming and modulation of immune cell activity (immune suppression) supporting tumor progression and lead to poor efficacy of intervention. Figure created with BioRender.com.
Research on the regulation of tumor immunity by the tumor ECM is rapidly expanding. Historically, the tumor ECM has largely been associated with a physical barrier that excludes immune cell access to the tumor. An expanding concept is that ligands from the ECM and its constituent components have direct, active effects on immune cells by binding to receptors that either stimulate or inhibit signaling pathways, and thus play a more active role in cancer immunosurveillance.
Under physiological conditions the ECM forms a proteinaceous network between cells in the tissue and thereby contributes to the arrangement and polarity of cells that support their survival and differentiation and maintain tissue organization (14). The ECM consists of a complex network of macromolecules including collagens, elastin, fibronectin, laminins, proteoglycans, and non-collagenous glycoproteins. Collagens are the major components of the ECM. Twenty-eight different types of collagen have been described, each with a unique role in maintaining tissues structure and function (15).
ECM biology has been a major area of focus in cancer research for well over forty years and aberrant production of ECM constituents is a classic hallmark of cancer progression (10, 16, 17). A wealth of knowledge has been developed that continues to build on our understanding of just how dramatically different cancer ECM is from normal EC. This includes the mechanisms and biophysics that lead to dramatic alterations seen in cancer (18, 19). What has been termed the Matrisome in cancer describes ECM proteins, particularly collagens, that are not only overexpressed, but structurally and biochemically aberrant from normal, healthy tissues (12, 20–22).
Most ECM biology studies in cancer have focused on understanding how the ECM modifies tumor cell transformation, growth, movement and metastasis, with less attention paid to its role in immune surveillance (23). The tools for understanding tumor immunology and developing novel immunotherapies has exploded over the past thirty years, largely due to the now well-established theory of tumor immune surveillance (24). A key aspect of tumor immune surveillance is that costimulatory or coinhibitory ligands, such as B7-1 or PD-L1, that interact with cognate costimulatory or coinhibitory receptors, such as CD28/CTLA-4 or PD-1, on T cells to elicit or suppress tumor-antigen-specific immune responses, respectively (25). These types of ligand-receptor interactions have a direct, active effect on immune cells mediated by downstream signaling. These studies have been fruitful regarding the development of immune checkpoint inhibitors targeting PD-1/PD-L1, CTLA-4, LAG-3 and other emerging therapeutics targeting molecules expressed on immune subpopulations (26). There is abundant literature that not only T cells, but other immune subpopulations such as NK cells, B cells, macrophages, dendritic cells (DCs) and neutrophils are critical to immune surveillance (reference 23 and new reference Shi et al). Tumors subvert the function of multiple cell types to cause immune dysfunction and immune suppression that ultimately leads to immune suppression in the complex TME. Therapeutics that target these immune subpopulations are emerging as potential next-generation immune therapies beyond checkpoint inhibitors (27).
Until recently, much of the work in tumor immune surveillance has focused on identifying and characterizing inter-cellular immune ligand-receptor interactions, with little attention to the ECM as a source of ligands and epitopes with either immune-activating and/or immune-suppressive signaling capacity, depending on the architecture and quality of the ECM. However, the fact that the ECM may be a storage of ligands and epitopes with signaling capacity has been known for decades. There are several hundred molecules that make up the core matrisome and associated ECM constituents (20). The overall composition and quality of core components and associated constituents are vastly altered in cancer (12). Continuing research and new technologies continue to define and elucidate the wealth of ligands and epitopes that may play a role in cancer immune surveillance and could be potentially used as cancer biomarkers and be targeted for cancer immunotherapy.
While it is not the purpose of this review to address all components of the ECM, collagens are particularly well described beyond their function as passive, structural molecules in the TME, and are now being recognized for their active contribution to several biological effects in the TME. Of the 28 types of collagen, collagens type IV, XIII, XV, and XVIII have received the most attention in the cancer field, consequent to the anti-angiogenic and pro-tumorigenic effects of the cryptic sites and signaling fragments found in the NC1 domains (28). The best characterized basement membrane collagen signals are derived from type IV collagen (Arresten, Canstatin, Tumstatin, Tetrastatin, Pentastatin, Hexastatin), type VIII collagen (Vastatin), type XV collagen (Restin), and type XVIII collagen (Endostatin) (29). Endothrophin, a signaling fragment derived from type VI collagen produced by fibroblasts is receiving increased attention in cancer and other fields where fibroblasts are central players (29, 30). The biological role of endothrophin in cancer is related to epithelial-to-mesenchymal transition and tumor fibrosis. Endothrophin is highly expressed in CAFs and is prognostic in a range of fibrotic diseases, including liver, lung, kidney, and skin fibrosis (31–36). Understanding how collagens and collagen fragments actively bind and regulate immune receptors, in addition to functions described here, will be critical to link cancer matrix biology and immune oncology.
Strides are being made in understanding how ECM components actively regulate immune cellular exclusion, activation, and suppression in cancer. Consequently, targeting the tumor promoting effects of the dysfunctional ECM is a novel approach to cancer immunotherapy. Building on recent understanding of the ECM-immune cell interplay will help overcome current limitations in cancer immunotherapy. Recent studies and concepts have started to combine these fields to identify novel links and biological understanding of these interactive pathways that will help lead the next wave of cancer immunotherapies.
2 Tumor ECM acts as a physical barrier to immune cell infiltration
The altered production and assembly of ECM proteins and collagens generally forms a fibrous connective tissue by interacting with other ECM components and is the major pathological signature of tumor fibrogenesis (also known as desmoplasia). Cross-linking of collagens by lysyl oxidase (LOX) enzymes increases the stiffness of this ECM (37). Transforming growth factor beta (TGF-β) and other pro-fibrotic cytokines signal to fibroblast and activate them into cancer associated fibroblasts (CAFs) with associated increased collagen synthesis (38–41). During cancer, an accumulation of activated CAFs is observed (42). Enhanced CAF activity results in increased deposition of a cross-linked collagen matrix in the TME (43, 44). CAF subtypes is the center of a lot of attention currently and a detailed description is beyond the scope of this review. However, evidence shows that some CAF subsets promote tumor progression and immunosuppression, while others prevent it (45–48), but the overall consensus is that the fibroblasts drive fibrosis and tumor progression (29). In fact, several recent studies suggest that alterations in ECM proteins, fibrillar collagens, CAFs, and increased expression of TGF-β all contribute to fibrosis and play key roles in resistance to immunotherapy by creating a physical barrier inhibiting T cell infiltration (immune exclusion) that is crucial for anti-tumor immunity and concomitant clinical responses to current checkpoint inhibitors (49–61).
3 Tumor ECM ligands interact with immune cell receptors
Beyond the dense and fibrotic tumor ECM barrier associated with immune exclusion, tumor ECM components also play a more active role in immune regulation when dysregulated ECM components act as ligands to interact with receptors on immune cells to inhibit or activate immune cell subpopulations in the TME. Thus, an expanding paradigm is that ECM-derived molecules are capable of interacting with and regulating immune cells not only in the context of well-described adhesive binding interactions and barrier function, but also through active interactions with immune cell inhibitory or stimulatory receptors to modulate T cells, myeloid cells and other immune cell types in the TME (62).
A primary mechanism of T cell suppression is through interaction of T cell surface inhibitory receptors with inhibitory ligands expressed on the cell surface of tumor cells, tumor associated macrophages (TAMs) and DCs (TADCs), myeloid-derived suppressor cells (MDSCs) or other suppressive cell types in the TME (63). Under normal conditions, inhibitory cell-cell interactions between receptors and ligands serve as a means of communication to maintain T cell tolerance to self, and to avoid dangerous autoreactive immune responses leading to autoimmunity. In the TME, aberrant expression of these receptors and ligands interact to circumvent anti-tumor T cell immunity. Blockade of cell-cell interaction-mediated immune inhibition is the basis of immune checkpoint inhibitor (ICI) immunotherapies that promote T cell anti-tumor immunity mediated by tumor-specific T cells. In addition to cell-cell molecular interactions, what is becoming more apparent is that ECM proteins can also function as inhibitory and stimulatory ligands to disrupt T cell and myeloid responses in the TME. T cells expressing inhibitory receptors, often defined as exhausted T cells, and myeloid cells - TAMs, TADCs and MDSCs – that have a suppressive phenotype, and are generally associated with poor prognosis in most cancers. There may be many reasons why tumor associated T cells and myeloid cells develop suppressive activity, but studies now suggest T cell and myeloid cell interaction with the ECM, including collagens, and mechanical properties such as collagen density and stiffness, contribute to direct suppression of immune function, or polarization of cells towards a suppressive phenotype and facilitate recruitment of suppressive cells into the TME (64, 65).
The immune regulatory function of the tumor ECM seems to be confined to specific ECM receptors, of which integrins and growth factor receptors are well known ECM binding receptors (reviewed in (66–69)). More recently, greater attention has been focused on an emerging set of regulatory receptors specifically expressed on immune cells that interact with ECM proteins to directly regulate immune function. The broad dysregulation of collagen and other ECM proteins that occurs in the TME can interact with aberrant expression of these inhibitory and stimulatory immune receptors to drive immune dysfunction in cancer. Emerging receptors in this context include LAIR-1, OSCAR and DDR1/DDR2, that are expressed on immune cells and interact with collagens to regulate immune function. Another receptors emerging in this context is LILRB4, a protein that interacts with fibronectin, a non-collagen component of the ECM CD44 and Toll-Like Receptors (TLRs), which binds more promiscuously ECM and non-ECM ligands.
3.1 ECM receptors that regulate tumor immunity
An overview of the ECM receptors that regulate tumor immunity is shown in Figure 2 and summarized below.
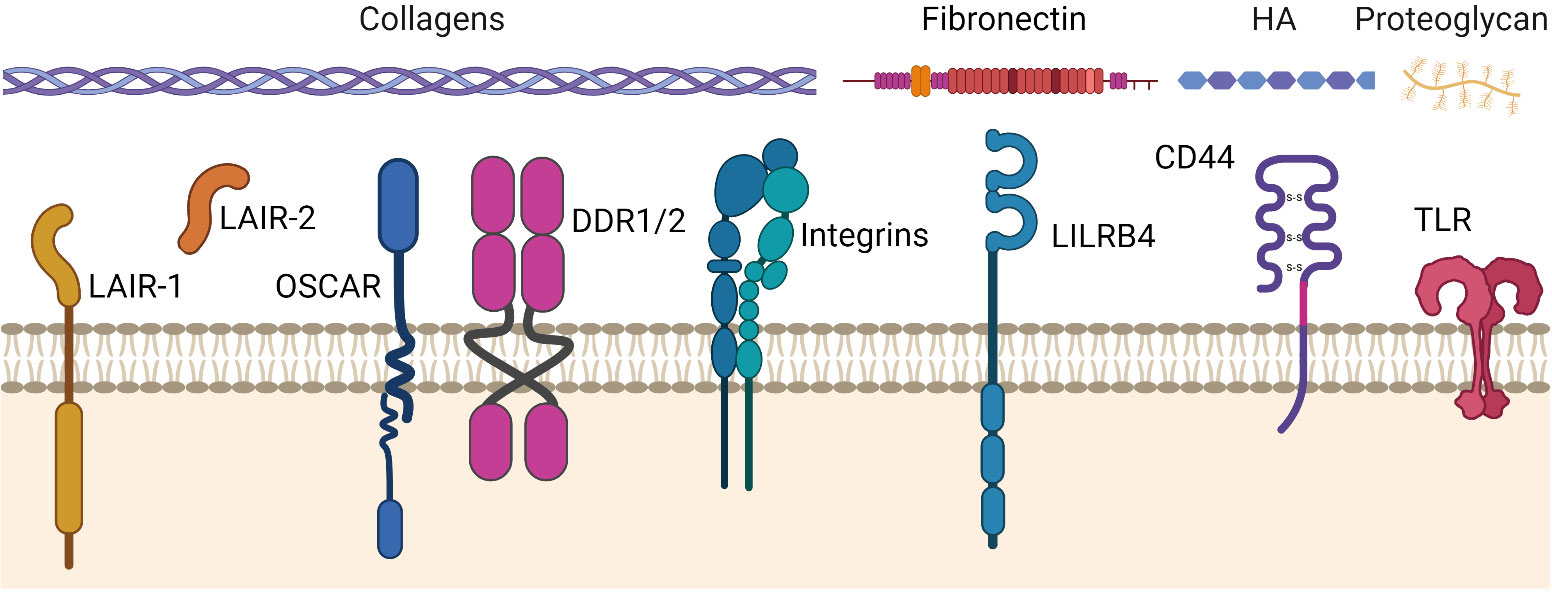
Figure 2 ECM receptors that may regulate tumor immunity in cancers. ECM receptors and their primary ligands (collagens, fibronectin, hyaluronic acid (HA), proteoglycans). The ECM stimulates or inhibits cellular activity through these receptors in response to, and dependent on, an injured, remodeled, or dysregulated ECM composition. LAIR-1, Leukocyte-Associated Immunoglobulin-like Receptor-1. LAIR-2, Leukocyte-Associated Immunoglobulin-like Receptor-2. OSCAR, Osteoclast-associated receptor. DDR-1/2, Discoidin Domain Receptors 1 and 2. LILRB4, Leukocyte immunoglobulin-like receptor subfamily B member 4 (a.k.a. ILT3). CD44, Cluster of differentiation 44. TLR, Toll-Like Receptor. Figure created with BioRender.com.
3.1.1 Integrins
Integrins are so-called heterodimeric receptors that are composed of α and β subunits (there are eight β and 18 α subunits in the integrin family that combine to form at least 24 distinct integrins). Integrins are cell adhesion receptors that play important roles during pathological processes and development (66). Integrins are transmembrane proteins composed of a short cytoplasmic region mediating the downstream signaling from the receptor, a transmembrane helix, and a large extracellular domain. The 24 distinct integrins are divided into four classes (RGD receptors, leukocyte-specific receptors, laminin receptors, and collagens receptors). The integrins that function primarily as collagen receptors are α1β1, α2β1, α3β1, α10β1 and α11β1 (67). The interplay between integrins and immune cells for cancer immunity and the role of integrin receptor interactions with the TME-ECM and targeting for cancer therapy is beyond the scope of this review and has been reviewed elsewhere (68, 69).
3.1.2 LAIR-1
The coinhibitory Leukocyte-Associated Immunoglobulin-like Receptor-1 (LAIR-1) is type-I transmembrane receptor expressed on T cells, NK cells, myeloid cells and other immune cell subsets, and binds to collagens and proteins with collagen-like domains (70). LAIR-1 expression is also abundant on TME immune cells and may increase with stage of disease (71–73). LAIR-1 contains motifs in its cytoplasmic region, including immunoreceptor tyrosine-based inhibitory motifs (ITIMs) and (CSK) that induce inhibitory signaling pathways into cells when LAIR-1 binds to collagen domain containing ligands (74, 75). As such, LAIR-1 interaction with collagens plays both a role in cellular adhesion to the ECM, and at the same time delivers signals to instruct immune cells to remain in a sub-optimal state of activation. Interestingly, under normal physiological conditions, LAIR-1 may play a limited role in maintaining immune homeostasis (76). However, when the ECM becomes dysfunctional in TMEs, aberrant collagen expression may both exclude LAIR-1 expressing immune cells from infiltrating the TME, and at the same time prevent tumor antigen-specific T cells from becoming activated and developing into cytotoxic effector T cells through LAIR-1 mediated inhibitory signaling (55, 72, 77, 78) (Figure 3). Expression of LAIR-1 on myeloid cells in cancer has been shown to play a role in immune suppression, also mediated primarily through collagen (79). In contrast, another study suggested an improved response in a pre-clinical model when LAIR-1 was present on myeloid cells (80). However, the observed function was attributed to interaction with a collagen-domain containing protein, COLEC12, rather than structural collagen of the ECM. Additional understanding of collagen-LAIR-1 mediated regulation of T cells and myeloid cells will be an important area of research for therapeutic targeting in both solid tumors and hematologic malignancies.
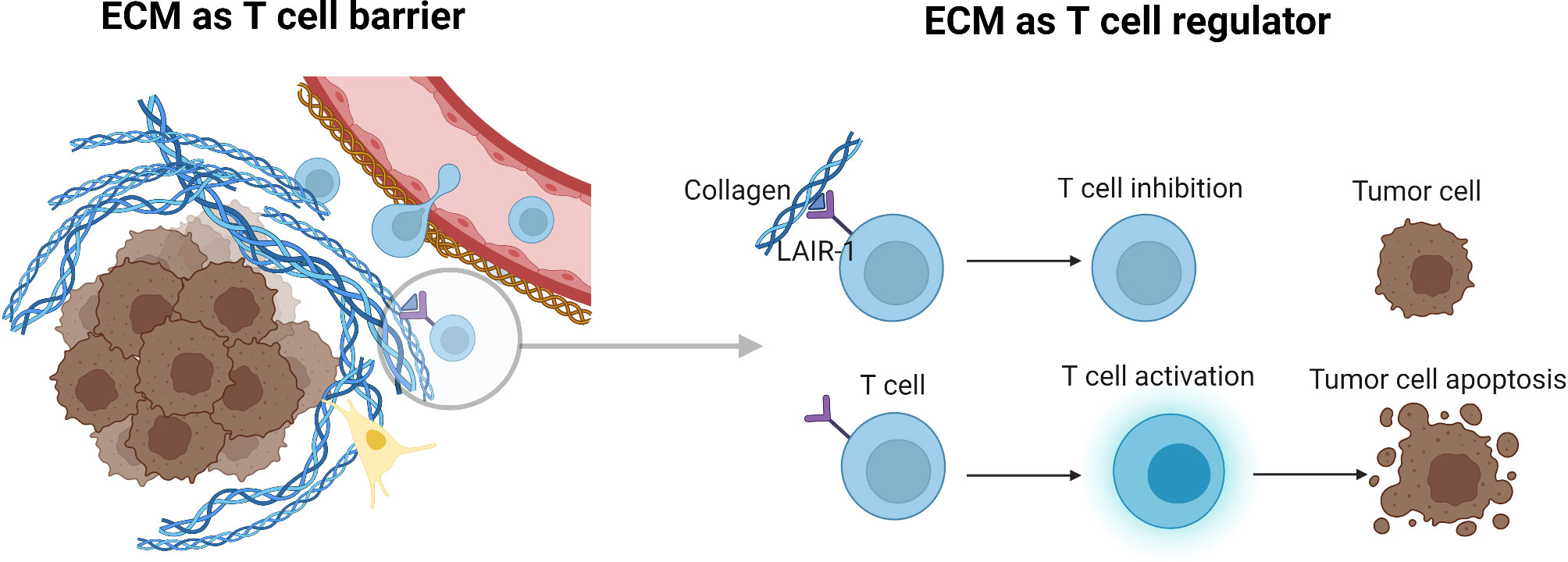
Figure 3 Tumor ECM as a T cell barrier and regulator of T cell activity. Aberrant collagen expression may both exclude immune cells from infiltrating the TME, and at the same time prevent T cells from becoming activated and developing into cytotoxic effector T cells. LAIR-1 as an example plays both a role in cellular adhesion to the ECM, and at the same time delivers signals to instruct immune cells to remain in a sub-optimal state of activation. Figure created with BioRender.com.
3.1.3 DDR1 and DDR2
Discoidin Domain Receptors 1 and 2 (DDR1 and DDR2) are receptor tyrosine kinases (RTKs) that uniquely bind to fibrillar collagens; DDR1 preferentially binds to collagen I–V, VIII (and Periostin), while DDR2 binds to collagen I–III, V, and X (81, 82). Indeed, studies have identified multiple effects of DDR1 and DDR2 on tumor growth and metastasis when expressed on tumor and CAFs (9, 83). DDR2 is also expressed on subpopulations of tumor myeloid cells and drives myeloid inflammatory pathways via RTK signaling (83). The composition and quality of the ECM is important for DDR signaling. For example, protease-cleaved type I collagen and intact type I collagen were shown to have opposing tumorigenic effects through DDR1 engagement in pancreatic cancer (84). Dissection of the role of DDR1/2 in immune regulation will shed light on the role of these important collagen receptors in cancer.
3.1.4 OSCAR
The Osteoclast-associated receptor (OSCAR) is a collagen receptor in the same family as LAIR-1, but with stimulatory signaling capacity through cytoplasmic association with FcRγ, which contains an Immunoreceptor Tyrosine-based Activation Motif (ITAM) (85, 86). OSCAR is expressed not only on osteoclasts, but also on other myeloid cell subsets, and OSCAR RNA is overexpressed in several cancers (87). Interestingly, despite OSCAR’s stimulatory capacity, RNA expression appears to positively associate with M2 macrophage differentiation, T cell exhaustion, cancer progression and metastasis, although much remains to be learned in the context of cancer.
3.1.5 LILRB4
Leukocyte immunoglobulin-like receptor subfamily B member 4 (LILRB4/ILT3) is an inhibitory ITIM containing Ig-superfamily and LILR family receptor that is expressed on DCs and other myeloid cells that binds to fibronectin (85, 88). In a recent study it was shown that LILRB4 interactions with fibronectin are capable of polarizing or maintaining DCs in the TME and draining lymph nodes in an immunosuppressive state, ultimately leading to decreased T cell activation and anti-tumor activity (89). Several additional studies have helped define an immune suppressive role for LILRB4 in cancer [reviewed in (90)].
LILRB4, LAIR-1 and OSCAR are all members of a larger Leukocyte Immunoglobulin-Like Receptor (LILR) family (91), several members of which have been described in cancer (92). It is interesting to speculate on how many additional members of this family of receptors, which are largely restricted to expression on immune subpopulations (93), interact with ECM proteins to stimulate or inhibit immunity in response to injured, remodeled, or dysregulated ECM ligands.
3.1.6 CD44 and toll-like receptors
An example of a receptor capable of binding to multiple ECM constituents is CD44, a receptor expressed on immune and non-immune cells, whose extracellular domain contains binding sites for various ECM proteins including collagen, laminin, and fibronectin, although the primary functional ligand of CD44 is hyaluronic acid (HA) (9). Versican, another ECM constituent that also binds to CD44, can bind to PSGL-1 and Toll-Like Receptors (TLR) on immune cells, demonstrating the potential promiscuity of ECM protein interactions with multiple receptors (94).
TLRs are immune receptors that are critical for linking innate immune pathogen-sensing with adaptive immunity (95). However, reports have shown that TLR2 and TLR4 can interact with ECM-derived hyaluronan fragments as an endogenous danger signal to stimulate TLR signaling (96, 97), as well as the ECM proteoglycan components biglycan and lumican to stimulate cells in cancer (98, 99). TLR4 can also interact with Heparan Sulfate to trigger inflammatory cytokine production (100). Of course, TLRs and other immune receptors may interact with their primary ligands that are embedded in the ECM, or released from the ECM during collagen remodeling, adding to the complexity of how the ECM regulated immunity not only in cancer, but also other diseases (101).
As described above, current studies suggest that both inhibitory and stimulatory collagen receptors are prone to driving immune dysfunction in cancer. This may be attributable to the inherent dysregulation of collagen products in the TME that subsequently drives dysregulation of both stimulatory and inhibitory collagen receptor signaling in ways that synergize to disrupt TME anti-tumor immunity. While the list of ECM proteins that interact in some way with immune and non-immune receptors is extensive, the continued identification of immune receptors that specifically bind to collagen and non-collagen ECM core component ligands, and functionally regulate immune cell responses in cancer, will help build a more comprehensive understanding of the matrix-mediated immune suppression in cancer.
4 Novel disruptive strategies to overcome tumor collagen ECM-mediated immune suppression
4.1 Tumor ECM restricts the potential of immune checkpoint inhibitors
Immune checkpoint inhibitors (ICIs) have revolutionized how cancer is treated and how we think about cancer. ICIs can actively promote long-term, durable responses that may evolve with and continue to surveil cancer, resulting in curative outcomes, rather than a short-term extension of survival (102). ICIs for treating cancer have demonstrated widespread clinical success over the past decade by targeting PD-1 and CTLA-4 pathways (102). Nevertheless, a majority of patients are not responsive to approved immunotherapies (103). While many factors may be responsible for the lack of response, stromal dependent mechanisms are thought to play an important role (104). These include barrier function and immune exclusion that lead to so-called cold or excluded tumor types (71). While several more recent ICI therapeutics targeting LAG-3, TIM-3, TIGIT and others are currently in various stages of clinical trials or pre-clinical development, it is likely that these therapeutics will face the same limitations posed by stromal elements in a large percentage of patients, and cancer types, similar to the current limitations observed with approved ICIs (25, 26, 102).
The emerging link between cancer immunity and the ECM is based on active regulation of immune receptors by ECM core elements. As such, ECM mechanisms of action become blurred between barrier function in cold or excluded phenotypes, versus immunosuppressed phenotypes from, for example, LAIR-1 signaling, since mechanisms of action can and likely often overlap (71). Understanding the expanding universe of the ECM and how the various components restrict or promote immunity and immunotherapy in cancer needs to be dissected to optimize ICI and other immunotherapies. Studies evaluating collagen-derived peptides (CDPs) such as type III collagen pro-peptides (PRO-C3) in the serum of patients treated with PD-1 or CTLA-4 blockade have suggested an association with poor prognosis (105–108). PRO-C3 has been interpreted as being associated with dense fibrotic TME-ECM, but could also suggest tumor ECM remodeling (109). Emerging therapeutics that target multiple immune-ECM mechanisms of interaction, as well as combination strategies that synergize by targeting separate immune, TME and ECM components, will benefit patients who do not otherwise respond to existing therapies (110).
4.2 Therapeutics that target the intersection of the ECM and immune cell receptors
Several studies show that disrupting LAIR-1 interactions with collagens results in enhanced tumor immunity (55, 72, 77, 79, 111). Across these studies, it was demonstrated that therapeutic targeting of the LAIR-1 pathway in tumor models promoted the activation and function of T cells, NK cells, macrophages, and DCs. LAIR-2 is a soluble homolog of the transmembrane protein LAIR-1 that is present in human and non-human primate genomes, but not most other mammals, including mice (112). LAIR-2 binds to the same ligands as LAIR-1, but with higher affinity. It acts as a natural decoy protein in humans to block LAIR-1 interaction with collagen and downstream signaling, and possibly other collagen binding proteins (72, 112). This natural mechanism was taken advantage of to develop a novel therapeutic that would both target collagenous tumors and prevent LAIR-1 mediated signaling and adhesion. LAIR-2 fusion proteins generated in independent studies demonstrated anti-tumor activity of LAIR-2 Fc in multiple tumor models that was T cell dependent and modified the myeloid compartment (72, 77, 79). In other studies, in vivo overexpression of LAIR-2, or LAIR-1 blocking antibodies, were used to block LAIR-1 with demonstrable anti-tumor effects (55, 111). In studies with the collagen binding LAIR-2 IgG1 fusion protein, NC410, it was suggested that ECM remodeling may be occurring based on detection in changes in CDPs in the serum of NC410 treated mice (72). In support, specific collagen fragments of type IV collagen degraded by granzyme B (C4G) and type VI collagen degraded by MMP (C6M) has been shown in vivo to increase after induction of T cell activation by NC410 (72, 113). Additional studies have indicated that collagen degradative products are suppressive to T cells and therefore blockade of LAIR-1 and potentially other collagen receptor interactions with collagen degradative products may further normalize immune function in cancer (114).
LILRB4 blocking antibodies have demonstrated anti-tumor effects in solid tumor and hematological malignancies (115, 116). Additional modalities have also been developed and tested for targeting LILRB4 in cancer therapy, including antibody drug conjugates for direct cytotoxicity of LILRB4 expressing tumor associated myeloid cells, and LILRB4 CAR-T cells for targeting LILRB4 expressing leukemic cells (117, 118). Dasatinib (BMS-354825, Sprycel) is a small molecule Src inhibitor that non-specifically blocks DDR1/2 and other kinase receptors. It is used to treat chronic myelogenous leukemia and Philadelphia-positive acute lymphoblastic leukemia, but is also being tested in a wide range of solid tumors in a variety of combinations (reviewed in (119)). Recently, blocking DDR1 in vivo was also shown to reverse immune exclusion by disrupting collagen fiber alignment in breast cancer (120). A first-in-human study of this anti-DDR1 alone and in combination with anti-PD1 blockade has recently been initiated (NCT05753722). DDR2 inhibition in combination with PD-1 blockade has also demonstrated reduced tumor growth (121). Based on the accelerating interest in ECM binding immune receptors, the number of clinical trials targeting the LAIR, LILRB4, DDR1, DDR2 and other pathways will continue to expand from the current ongoing trials listed in Table 1.
4.3 Therapeutics that target the ECM for immunotherapy combination strategies
Many attempts have been and continue to be made to target various ECM components in tumors to overcome drug resistance and for stroma normalization (122–124) Unfortunately, targeting these pathways alone has not been effective. Drugs that prevent the excess accumulation of ECM molecules are important for controlling tumor fibrosis, and altering the degradation of the ECM may be equally important for improving tumor immunity and immunotherapy, and eliminating tumor progression (45, 46, 125–127). It is proposed that targeting the ECM in combination with immunotherapies could synergize to activate immune cells and promote immune infiltration into the TME, while simultaneously disrupting other tumor promoting aspects of the ECM. Several studies have now indicated that therapeutic targeting of the collagen:LAIR-1 pathway in combination with PD-1 targeting therapies yields improved and synergistic activity in pre-clinical studies (55, 77). Additionally, a recent study that combines NC410 with Bintrafusp-alfa, a PD-L1 mAb fused with TGF-βRII demonstrated an even better outcome than NC410 with PD-1 blockade, suggesting the combination of checkpoint blockade and TME-ECM remodeling synergize to remodel immune responses in favor of anti-tumor immunity (79). This supports previous studies targeting TGF-β that have demonstrated improvement in anti-tumor immunity in combination with ICIs (128, 129).
LOX inhibitors have been shown to improve the response to PD-1 therapy (130). Along the same lines, modulating collagen expression and deposition in the tumor by targeting intracellular focal adhesion kinase (FAK) renders pancreatic cancers responsive to checkpoint inhibitor immunotherapy in vivo (131, 132). These studies validate the emerging therapeutic strategy of combining ECM targeting with immune checkpoint inhibitors for optimal activity and efficacy.
Importantly, collagen remodeling derived products (CDPs) are emerging as key players for defining the ECM and immune landscape of tumors and response to immunotherapy (133, 134). Such ECM protein biomarkers, ideally serum CDPs, may be identified to select indications and patients that are most likely to benefit from ECM-immune combination strategies (106, 108).
4.4 Directing and localizing therapeutics by targeting tumor specific ECM
Targeting therapeutics to and within tumors by targeting aberrant expression of ECM proteins in tumors is a growing strategy in cancer therapy. Conjugating PD-1 or CTLA-4 antibodies with ECM targeting agents, or fusing cytokines to ECM targeting agents for tumor localization have demonstrated positive pre-clinical results (135, 136). Anchoring of intratumorally administered cytokines to collagen safely potentiates systemic cancer immunotherapy (136). Cytokines have been fused with antibodies, or nanobodies, targeting specific domains of fibronectin (137). Fibronectin, Tenascin-C and other ECM proteins that are abundant in glioblastoma can be targeted for delivery of various payloads including RNA interference (138). These strategies and methodologies will undoubtedly be improved upon with advanced understanding of immune-ECM biology and may likely enter clinical testing soon.
The overall means of strategies described above to overcome tumor ECM/collagen-mediated immune suppression is shown in Figure 4.
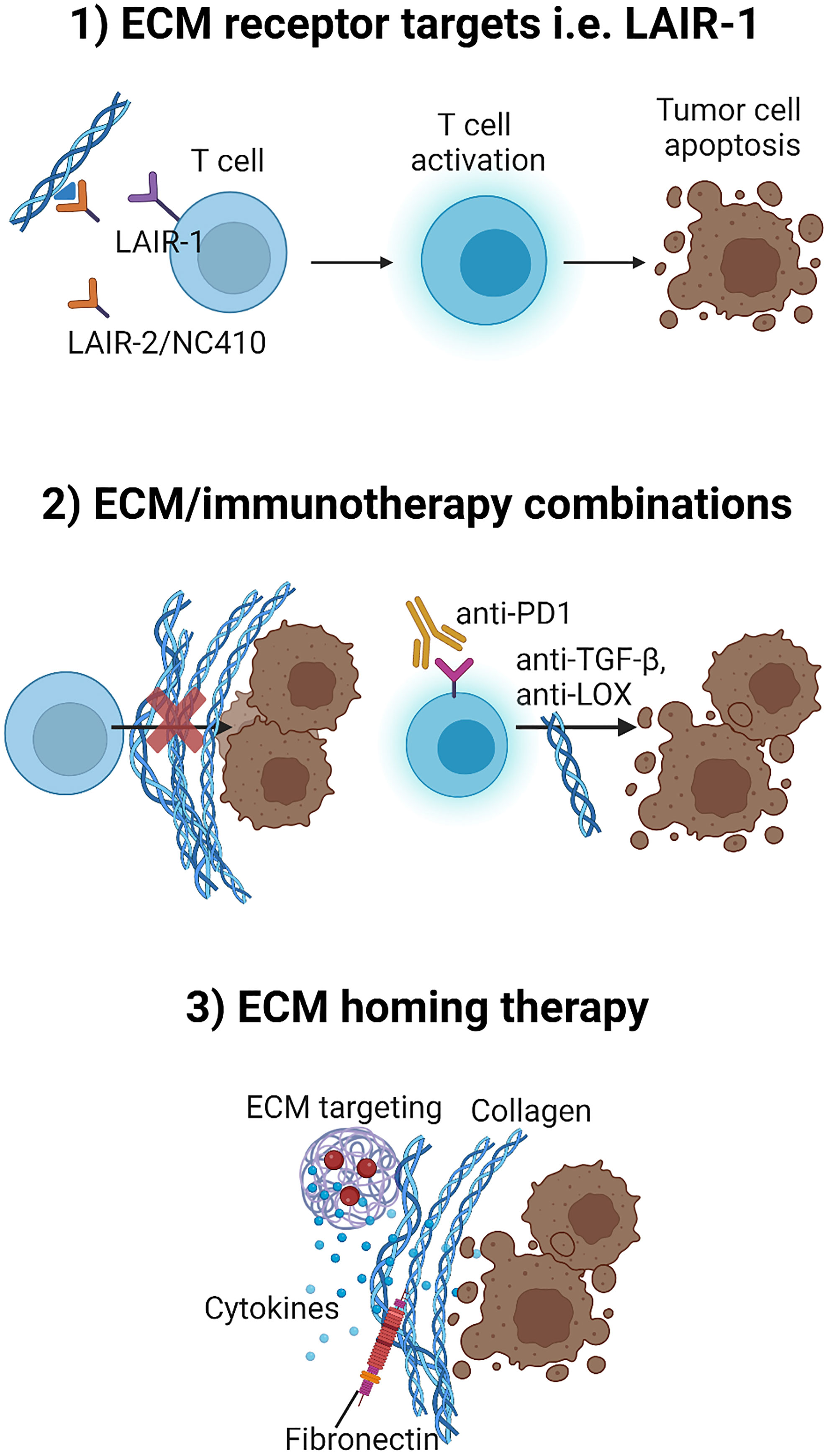
Figure 4 Three potential strategies to overcome tumor ECM/collagen-mediated immune suppression. 1) Developing therapeutics that targets ECM-immune cell receptors such as interfering with the immunosuppressive role of LAIR-1 by treatment with LAIR-2/NC410. 2) Developing combination strategies with therapeutics such as TGF-β inhibitors or LOX inhibitors that may target the tumor ECM for normalization and thereby improve efficacy of immunotherapy (anti-PD-1) combination strategies. 3) Directing and localizing therapeutics such as cytokines to the TME by fusing cytokines to ECM targeting agents for tumor localization. Figure created with BioRender.com.
5 Conclusion
Our expanded understanding in the fields of matrix biology, cancer biology, and immunobiology have contributed independently to improving upon the efficacy of cancer therapeutics. However, limited interaction and overlap has occurred to bring these fields together for developing novel cancer treatments. Expanding knowledge of the effects of collagen and other ECM components that actively regulate populations of immune cells in cancer will be important in helping to advance this emerging and exciting field of research. Importantly, it will also aid our ability to develop new classes of therapeutics to treat cancer and patients with unmet needs.
Author contributions
All authors contributed equally to writing this manuscript.
Conflict of interest
Authors DF and SL are employed by the company NextCure Inc. Authors MK, CJ and NW are employed by the company Nordic Bioscience.
Publisher’s note
All claims expressed in this article are solely those of the authors and do not necessarily represent those of their affiliated organizations, or those of the publisher, the editors and the reviewers. Any product that may be evaluated in this article, or claim that may be made by its manufacturer, is not guaranteed or endorsed by the publisher.
References
2. Xiao Y, Yu D. Tumor microenvironment as a therapeutic target in cancer. Pharmacol Ther (2021) 221:107753. doi: 10.1016/j.pharmthera.2020.107753
3. Wu T, Dai Y. Tumor microenvironment and therapeutic response. Cancer Lett (2017) 387:61–8. doi: 10.1016/j.canlet.2016.01.043
4. Dzobo K, Senthebane DA, Dandara C. The tumor microenvironment in tumorigenesis and therapy resistance revisited. Cancers (Basel) (2023) 15(2):376. doi: 10.3390/cancers15020376
5. Senthebane DA, Jonker T, Rowe A, Thomford NE, Munro D, Dandara C, et al. The role of tumor microenvironment in chemoresistance: 3D extracellular matrices as accomplices. Int J Mol Sci (2018) 19(10):2861. doi: 10.3390/ijms19102861
6. Senthebane DA, Rowe A, Thomford NE, Shipanga H, Munro D, Mazeedi MAM, et al. The role of tumor microenvironment in chemoresistance: to survive, keep your enemies closer. Int J Mol Sci (2017) 18(7):1586. doi: 10.3390/ijms18071586
7. Jarosz-Biej M, Smolarczyk R, Cichoń T, Kułach N. Tumor microenvironment as A “Game changer” in cancer radiotherapy. Int J Mol Sci (2019) 20(13):3212. doi: 10.3390/ijms20133212
8. Hinshaw DC, Shevde LA. The tumor microenvironment innately modulates cancer progression. Cancer Res (2019) 79(18):4557–66. doi: 10.1158/0008-5472.CAN-18-3962
9. Lorusso G, Rüegg C, Kuonen F. Targeting the extra-cellular matrix — Tumor cell crosstalk for anti-cancer therapy: emerging alternatives to integrin inhibitors. Front Oncol (2020) 10:1–17. doi: 10.3389/fonc.2020.01231
10. Venning FA, Wullkopf L, Erler JT. Targeting ECM disrupts cancer progression. Front Oncol (2015) 5(October):1–15. doi: 10.3389/fonc.2015.00224
11. Mayorca-Guiliani AE, Madsen CD, Cox TR, Horton ER, Venning FA, Erler JT. ISDoT: in situ decellularization of tissues for high-resolution imaging and proteomic analysis of native extracellular matrix. Nat Med (2017) 23:890–8. doi: 10.1038/nm.4352
12. Socovich AM, Naba A. The cancer matrisome: From comprehensive characterization to biomarker discovery. Semin Cell Dev Biol (2018) 89:157–66. doi: 10.1016/j.semcdb.2018.06.005
13. Deb G, Cicala A, Papadas A. Matrix proteoglycans in tumor inflammation and immunity. Am J Physiiol Cell Physiol (2022) 323:678–93. doi: 10.1152/ajpcell.00023.2022
14. Frantz C, Stewart KM, Weaver VM. The extracellular matrix at a glance. J Cell Sci (2010) 123(24):4195–200. doi: 10.1242/jcs.023820
15. Karsdal M. Biochemistry of collagens: structure, function and biomarkers. Cambridge, Massachusetts, United States: Academic Press (2016).
16. Pickup MW, Mouw JK, Weaver VM. The extracellular matrix modulates the hallmarks of cancer. EMBO Rep (2014) 15(12):1243–53. doi: 10.15252/embr.201439246
17. Bonnans C, Chou J, Werb Z. Remodelling the extracellular matrix in development and disease. Nat Rev Mol Cell Biol (2014) 15(12):786–801. doi: 10.1038/nrm3904
18. Winkler J, Abisoye-Ogunniyan A, Metcalf KJ, Werb Z. Concepts of extracellular matrix remodelling in tumour progression and metastasis. Nat Commun (2020) 11(1):1–19. doi: 10.1038/s41467-020-18794-x
19. Emon B, Bauer J, Jain Y, Jung B, Saif T. Biophysics of tumor microenvironment and cancer metastasis - A mini review. Comput Struct Biotechnol J (2018) 16:279–87. doi: 10.1016/j.csbj.2018.07.003
20. Hynes RO, Naba A. Overview of the matrisome — An inventory of extracellular matrix constituents and functions. Cold Spring Harb Perspect Biol (2012) 4:a004903. doi: 10.1101/cshperspect.a004903
21. Chen Y, Yang S, Tavormina J, Tampe D, Zeisberg M, Wang H, et al. Oncogenic collagen I homotrimers from cancer cells bind to α3β1 integrin and impact tumor microbiome and immunity to promote pancreatic cancer. Cancer Cell (2022) 40(8):818–834.e9. doi: 10.1016/j.ccell.2022.06.011
22. Papanicolaou M, Parker AL, Yam M, Filipe EC, Wu SZ, Chitty JL, et al. Temporal profiling of the breast tumour microenvironment reveals collagen XII as a driver of metastasis. Nat Commun (2022) 13(1):4587. doi: 10.1038/s41467-022-32255-7
23. Filipe EC, Chitty JL, Cox TR. Charting the unexplored extracellular matrix in cancer. Int J Exp Pathol (2018) 99(2):58–76. doi: 10.1111/iep.12269
24. Burnet FM. The concept of immunological surveillance. Prog Exp Tumor Res (1970) 13:1–27. doi: 10.1159/000386035
25. Chen DS, Mellman I. Oncology meets immunology: The cancer-immunity cycle. Immunity (2013) 39(1):1–10. doi: 10.1016/j.immuni.2013.07.012
26. Belli C, Antonarelli G, Repetto M, Bielo LB, Crimini E, Curigliano G. Targeting cellular components of the tumor microenvironment in solid Malignancies. Cancers (Basel) (2022) 14(4278):1–22. doi: 10.3390/cancers14174278
27. Shi Y, Tomczak K, Li J, Ochieng JK, Lee Y, Haymaker C. Next-generation immunotherapies to improve anticancer immunity. Front Pharmacol (2020) 11:566401. doi: 10.3389/fphar.2020.566401
28. Sund M, Kalluri R. Endogenous inhibitors of angiogenesis. In: Tumor Angiogenesis: Basic Mechanisms and Cancer Therapy. (Berlin Heidelberg, Germany: Springer-Verlag) (2008).
29. Karsdal MA, Nielsen SH, Leeming DJ, Langholm LL, Nielsen MJ, Manon-Jensen T, et al. The good and the bad collagens of fibrosis – Their role in signaling and organ function. Adv Drug Delivery Rev (2017) 121:43–56. doi: 10.1016/j.addr.2017.07.014
30. Wang J, Pan W. The biological role of the collagen alpha-3 (VI) chain and its cleaved C5 domain fragment endotrophin in cancer. Onco Targets Ther (2020) 13:5779–93. doi: 10.2147/OTT.S256654
31. Fenton A, Jesky MD, Ferro CJ, Sørensen J, Karsdal MA, Cockwell P, et al. Serum endotrophin, a type VI collagen cleavage product, is associated with increased mortality in chronic kidney disease. PloS One (2017) 12(4):1–14. doi: 10.1371/journal.pone.0175200
32. Rasmussen DGK, Fenton A, Jesky M, Ferro C, Boor P, Tepel M, et al. Urinary endotrophin predicts disease progression in patients with chronic kidney disease. Sci Rep (2017) 7(1):17328. doi: 10.1038/s41598-017-17470-3
33. Rønnow SR, Langholm LL, Karsdal MA, Manon-Jensen T, Tal-Singer R, Miller BE, et al. Endotrophin, an extracellular hormone, in combination with neoepitope markers of von Willebrand factor improves prediction of mortality in the ECLIPSE COPD cohort. Respir Res (2020) 21(202):1–6.
34. Organ LA, Duggan AMR, Oballa E, Taggart SC, Simpson JK, Kang’Ombe AR, et al. Biomarkers of collagen synthesis predict progression in the PROFILE idiopathic pulmonary fibrosis cohort. Respir Res (2019) 20(148):1–10. doi: 10.1186/s12931-019-1118-7
35. Juhl P, Bay-Jensen AC, Hesselstrand R, Siebuhr AS, Wuttge DM. Type III, IV, and VI collagens turnover in systemic sclerosis – a longitudinal study. Sci Rep (2020) 10:7145–51. doi: 10.1038/s41598-020-64233-8
36. Luo Y, Oseini A, Gagnon R, Charles ED, Sidik K, Vincent R, et al. An evaluation of the collagen fragments related to fibrogenesis and fibrolysis in nonalcoholic steatohepatitis. Sci Rep (2018) 8(12414):1–9. doi: 10.1038/s41598-018-30457-y
37. Payne SL, Hendrix MJ, Kirschmann DA. Paradoxical roles for lysyl oxidases in cancer–a prospect. J Cell Biochem (2007) 101(6):1338–54. doi: 10.1002/jcb.21371
38. Meng X, Nikolic-Paterson DJ, Lan HY. TGF-β: the master regulator of fibrosis. Nat Rev Nephrol (2016) 12(6):325–38. doi: 10.1038/nrneph.2016.48
39. Borthwick LA, Wynn TA, Fisher AJ. Cytokine mediated tissue fibrosis. Biochim Biophys Acta Mol Basis Dis (2013) 1832(7):1049–60. doi: 10.1016/j.bbadis.2012.09.014
40. Ricard-Blum S, Baffet G, Théret N. Molecular and tissue alterations of collagens in fibrosis. Matrix Biol (2018) 68–69:122–49. doi: 10.1016/j.matbio.2018.02.004
41. Nissen NI, Karsdal M, Willumsen N. Collagens and Cancer associated fibroblasts in the reactive stroma and its relation to Cancer biology. J Exp Clin Cancer Res (2019) 38(1):115. doi: 10.1186/s13046-019-1110-6
42. Kalluri R, Zeisberg M. Fibroblasts in cancer. Nat Rev Cancer (2006) 6(5):392–401. doi: 10.1038/nrc1877
43. Pankova D, Chen Y, Terajima M, Schliekelman MJ, Baird BN, Fahrenholtz M, et al. Cancer-associated fibroblasts induce a collagen cross-link switch in tumor stroma. Mol Cancer Res (2016) 14(3):287–95. doi: 10.1158/1541-7786.MCR-15-0307
44. Yamauchi M, Barker TH, Gibbons DL, Kurie JM. The fibrotic tumor stroma. J Clin Invest (2018) 128(1):16–25. doi: 10.1172/JCI93554
45. Özdemir BC, Pentcheva-Hoang T, Carstens JL, Zheng X, Wu CC, Simpson TR, et al. Depletion of carcinoma-associated fibroblasts and fibrosis induces immunosuppression and accelerates pancreas cancer with reduced survival. Cancer Cell (2014) 25(6):719–34. doi: 10.1016/j.ccr.2014.04.005
46. Chen Y, Kim J, Yang S, Wang H, Wu C-J, Sugimoto H, et al. Type I collagen deletion in αSMA+ myofibroblasts augments immune suppression and accelerates progression of pancreatic cancer. Cancer Cell (2021) 39(4):548–65. doi: 10.1016/j.ccell.2021.02.007
47. Öhlund D, Handly-Santana A, Biffi G, Elyada E, Almeida AS, Ponz-Sarvise M, et al. Distinct populations of inflammatory fibroblasts and myofibroblasts in pancreatic cancer. J Exp Med (2017) 214(3):579–96. doi: 10.1084/jem.20162024
48. Bhattacharjee S, Hamberger F, Ravichandra A, Miller M, Nair A, Affo S, et al. Tumor restriction by type I collagen opposes tumor-promoting effects of cancer-associated fibroblasts. J Clin Invest (2021) 131(11):e146987. doi: 10.1172/JCI146987
49. Mariathasan S, Turley SJ, Nickles D, Castiglioni A, Yuen K, Wang Y, et al. TGFβ attenuates tumour response to PD-L1 blockade by contributing to exclusion of T cells. Nature (2018) 554(7693):544–8. doi: 10.1038/nature25501
50. Wang L, Saci A, Szabo PM, Chasalow SD, Castillo-Martin M, Domingo-Domenech J, et al. EMT- and stroma-related gene expression and resistance to PD-1 blockade in urothelial cancer. Nat Commun (2018) 9(1):1–12. doi: 10.1038/s41467-018-05992-x
51. Subrahmanyam PB, Dong Z, Gusenleitner D, Giobbie-Hurder A, Severgnini M, Zhou J, et al. Distinct predictive biomarker candidates for response to anti-CTLA-4 and anti-PD-1 immunotherapy in melanoma patients. J Immunother Cancer (2018) 6(1):18. doi: 10.1186/s40425-018-0328-8
52. Chen PL, Roh W, Reuben A, Cooper ZA, Spencer CN, Prieto PA, et al. Analysis of immune signatures in longitudinal tumor samples yields insight into biomarkers of response and mechanisms of resistance to immune checkpoint blockade. Cancer Discovery (2016) 6(8):827–37. doi: 10.1158/2159-8290.CD-15-1545
53. Hurkmans DP, Basak EA, Schepers N, Oomen-De Hoop E, Van Der Leest CH, El Bouazzaoui S, et al. Granzyme B is correlated with clinical outcome after PD-1 blockade in patients with stage IV non-small-cell lung cancer. J Immunother Cancer (2020) 8(1):e000586. doi: 10.1136/jitc-2020-000586
54. Chakravarthy A, Khan L, Bensler NP, Bose P, De Carvalho DD. TGF-β-associated extracellular matrix genes link cancer-associated fibroblasts to immune evasion and immunotherapy failure. Nat Commun (2018) 9(1):4692. doi: 10.1038/s41467-018-06654-8
55. Peng DH, Rodriguez BL, Diao L, Chen L, Wang J, Byers LA, et al. Collagen promotes anti-PD-1/PD-L1 resistance in cancer through LAIR1-dependent CD8+ T cell exhaustion. Nat Commun (2020) 11(1):4520. doi: 10.1038/s41467-020-18298-8
56. Kuczek DE, Larsen AMH, Thorseth ML, Carretta M, Kalvisa A, Siersbæk MS, et al. Collagen density regulates the activity of tumor-infiltrating T cells. J Immunother Cancer (2019) 7(1):1–15. doi: 10.1186/s40425-019-0556-6
57. Okrah K, Tarighat S, Liu B, Koeppen H, Wagle MC, Cheng G, et al. Transcriptomic analysis of hepatocellular carcinoma reveals molecular features of disease progression and tumor immune biology. NPJ Precis Oncol (2018) 15(2):25. doi: 10.1038/s41698-018-0068-8
58. Tumeh PC, Harview CL, Yearley JH, Shintaku IP, Taylor EJM, Robert L, et al. PD-1 blockade induces responses by inhibiting adaptive immune resistance. Nature (2014) 515(7528):568–71. doi: 10.1038/nature13954
59. Jessurun CAC, Vos JAM, Limpens J, Luiten RM. Biomarkers for response of melanoma patients to immune checkpoint inhibitors: A systematic review. Front Oncol (2017) 7:233. doi: 10.3389/fonc.2017.00233
60. Hamid O, Schmidt H, Nissan A, Ridolfi L, Aamdal S, Hansson J, et al. A prospective phase II trial exploring the association between tumor microenvironment biomarkers and clinical activity of ipilimumab in advanced melanoma. J Transl Med (2011) 9(1):204. doi: 10.1186/1479-5876-9-204
61. Bjoern J, Juul Nitschke N, Zeeberg Iversen T, Schmidt H, Fode K, Svane IM. Immunological correlates of treatment and response in stage IV Malignant melanoma patients treated with Ipilimumab. Oncoimmunology (2016) 5(4):e1100788. doi: 10.1080/2162402X.2015.1100788
62. Mette A, Rømer A, Thorseth M, Madsen DH. Immune modulatory properties of collagen in cancer. Front Immunol (2021) 12:1–15.
63. Chen L, Flies DB. Molecular mechanisms of T cell co-stimulation and co-inhibition. Nat Rev Immunol (2013) 13(4):227–42. doi: 10.1038/nri3405
64. DeNardo DG, Ruffell B. Macrophages as regulators of tumour immunity and immunotherapy. Nat Rev Immunol (2019) 19(6):369–82. doi: 10.1038/s41577-019-0127-6
65. Larsen AMH, Kuczek DE, Kalvisa A, Siersbæk MS, Thorseth M-L, Johansen AZ, et al. Collagen density modulates the immunosuppressive functions of macrophages. J Immunol (2020) 205(5):1461–72. doi: 10.4049/jimmunol.1900789
66. Hynes RO. Integrins: bidirectional, allosteric signaling machines. Cell (2002) 110(6):673–87. doi: 10.1016/S0092-8674(02)00971-6
67. Barczyk M, Carracedo S, Gullberg D. Integrins. Cell Tissue Res (2010) 339(1):269–80. doi: 10.1007/s00441-009-0834-6
68. Zhang Q, Zhang S, Chen J, Xie Z. The interplay between integrins and immune cells as a regulator in cancer immunology. Int J Mol Sci (2023) 24(7):6170. doi: 10.3390/ijms24076170
69. Bergonzini C, Kroese K, Zweemer AJM, Danen EHJ. Targeting integrins for cancer therapy - disappointments and opportunities. Front Cell Dev Biol (2022) 10:1–13. doi: 10.3389/fcell.2022.863850
70. Meyaard L. The inhibitory collagen receptor LAIR-1 (CD305). J Leukoc Biol (2008) 83(4):799–803. doi: 10.1189/jlb.0907609
71. Galon J, Bruni D. Approaches to treat immune hot, altered and cold tumours with combination immunotherapies. Nat Rev Drug Discovery (2019) 18(3):197–218. doi: 10.1038/s41573-018-0007-y
72. Ramos MIP, Tian L, de Ruiter EJ, Song C, Paucarmayta A, Singh A, et al. Cancer immunotherapy by NC410, a LAIR-2 Fc protein blocking human LAIR-collagen interaction. Elife (2021) 10:e62927. doi: 10.7554/eLife.62927
73. Flies DB, Higuchi T, Harris JC, Jha V, Gimotty PA, Adams SF. Immune checkpoint blockade reveals the stimulatory capacity of tumor-associated CD103+ dendritic cells in late-stage ovarian cancer. Oncoimmunology (2016) 5(8):e1185583. doi: 10.1080/2162402X.2016.1185583
74. Lebbink RJ, de Ruiter T, Adelmeijer J, Brenkman AB, van Helvoort JM, Koch M, et al. Collagens are functional, high affinity ligands for the inhibitory immune receptor LAIR-1. J Exp Med (2006) 203(6):1419–25. doi: 10.1084/jem.20052554
75. Rygiel TP, Stolte EH, de Ruiter T, van de Weijer ML, Meyaard L. Tumor-expressed collagens can modulate immune cell function through the inhibitory collagen receptor LAIR-1. Mol Immunol (2011) 49(1–2):402–6. doi: 10.1016/j.molimm.2011.09.006
76. Tang X, Tian L, Esteso G, Choi S, Barrow AD, Colonna M, et al. Leukocyte-associated ig-like receptor-1 – deficient mice have an altered immune cell phenotype. J Immunol (2012) 188(2):548–58. doi: 10.4049/jimmunol.1102044
77. Xu L, Wang S, Li J, Li J, Li B. Cancer immunotherapy based on blocking immune suppression mediated by an immune modulator LAIR-1. Oncoimmunology (2020) 9(1):e1740477. doi: 10.1080/2162402X.2020.1740477
78. Xie J, Gui X, Deng M, Chen H, Chen Y, Liu X, et al. Blocking LAIR1 signaling in immune cells inhibits tumor development. Front Immunol (2022) 13:996026. doi: 10.3389/fimmu.2022.996026
79. Horn LA, Chariou PL, Gameiro SR, Qin H, Iida M, Fousek K, et al. Remodeling the tumor microenvironment via blockade of LAIR-1 and TGF-β signaling enables PD-L1–mediated tumor eradication. J Clin Invest (2022) 132(8):e155148. doi: 10.1172/JCI155148
80. Keerthivasan S, Şenbabaoğlu Y, Martinez-Martin N, Husain B, Verschueren E, Wong A, et al. Homeostatic functions of monocytes and interstitial lung macrophages are regulated via collagen domain-binding receptor LAIR1. Immunity (2021) 54:1511–26. doi: 10.1016/j.immuni.2021.06.012
81. Fu H, Valiathan RR, Arkwright R, Sohail A, Mihai C, Kumarasiri M. Discoidin domain receptors: unique receptor tyrosine kinases in collagen-mediated. J Biol Chem (2013) 288(11):7430–7. doi: 10.1074/jbc.R112.444158
82. Han T, Mignatti P, Abramson SB, Id MA. Periostin interaction with discoidin domain degeneration. PLoS One (2020) 1:1–13.
83. Majo S, Auguste P. The yin and yang of discoidin domain receptors (DDRs): implications in tumor growth and metastasis development. Cancers (Basel) (2021) 13(1725):1–27. doi: 10.3390/cancers13071725
84. Su H, Yang F, Fu R, Trinh B, Sun N, Liu J, et al. Collagenolysis-dependent DDR1 signalling dictates pancreatic cancer outcome. Nature (2022) 610(7931):366–72. doi: 10.1038/s41586-022-05169-z
85. Barrow AD, Choi Y, Trowsdale J, Barrow AD, Raynal N, Andersen TL, et al. OSCAR is a collagen receptor that costimulates osteoclastogenesis in DAP12-deficient humans and mice. JCI (2011) 121(9):3505–16. doi: 10.1172/JCI45913
86. Nedeva IR, Vitale M, Elson A, Hoyland JA, Bella J. Role of OSCAR signaling in osteoclastogenesis and bone disease. Front Cell Dev Biol (2021) 9:1–19. doi: 10.3389/fcell.2021.641162
87. Liao X, Bu Y, Zhang Y, Xu B, Liang J, Jia Q, et al. OSCAR facilitates Malignancy with enhanced metastasis correlating to inhibitory immune microenvironment in multiple cancer types. J Cancer (2021) 12:3769–80. doi: 10.7150/jca.51964
88. Manavalan JS, Rossi PC, Vlad G, Piazza F, Yarilina A, Cortesini R, et al. High expression of ILT3 and ILT4 is a general feature of tolerogenic dendritic cells. Transpl Immunol (2003) 11(3–4):245–58. doi: 10.1016/S0966-3274(03)00058-3
89. Paavola KJ, Roda JM, Lin VY, Chen P, O’Hollaren KP, Ventura R, et al. The fibronectin–ILT3 interaction functions as a stromal checkpoint that suppresses myeloid cells. Cancer Immunol Res (2021) 9(11):1283–97. doi: 10.1158/2326-6066.CIR-21-0240
90. Yang T, Qian Y, Liang X, Wu J, Zou M, Deng M. LILRB4, an immune checkpoint on myeloid cells. Blood Sci (Baltimore Md) (2022) 4(2):49–56. doi: 10.1097/BS9.0000000000000109
91. Storm L, Bruijnesteijn J, De Groot NG, Bontrop RE. The genomic organization of the LILR region remained largely conserved throughout primate evolution: implications for health and disease. Front Immunol (2021) 12:1–19. doi: 10.3389/fimmu.2021.716289
92. Zhang F, Zheng J, Kang X, Deng M, Lu Z, Kim J, et al. Inhibitory leukocyte immunoglobulin-like receptors in cancer development. Sci China Life Sci (2015) 58:1216–25. doi: 10.1007/s11427-015-4925-1
93. Zhang J, Mai S, Chen H, Kang K, Li XC, Chen S, et al. Leukocyte immunoglobulin-like receptors in human diseases: an overview of their distribution , function , and potential application for immunotherapies. JLB (2017) 102:351–60. doi: 10.1189/jlb.5MR1216-534R
94. Wight TN, Kang I, Evanko SP, Harten IA, Chang MY, Pearce OMT, et al. Versican — A critical extracellular matrix regulator of immunity and inflammation. Front Immunol (2020) 11:1–12. doi: 10.3389/fimmu.2020.00512
95. Iwasaki A, Medzhitov R. Regulation of adaptive immunity by the innate immune system. Science (2010) 327(5963):291–5. doi: 10.1126/science.1183021
96. Scheibner KA, Lutz MA, Boodoo S, Fenton MJ, Powell JD, Horton MR. Hyaluronan fragments act as an endogenous danger signal by engaging TLR2. J Immunol (2006) 177(2):1272–81. doi: 10.4049/jimmunol.177.2.1272
97. Taylor KR, Trowbridge JM, Rudisill JA, Termeer CC, Simon JC, Gallo RL. Hyaluronan fragments stimulate endothelial recognition of injury through TLR4. J Biol Chem (2004) 279(17):17079–84. doi: 10.1074/jbc.M310859200
98. Hu L, Zang M, Wang H-X, Li J-F, Su L-P, Yan M, et al. Biglycan stimulates VEGF expression in endothelial cells by activating the TLR signaling pathway. Mol Oncol (2016) 10(9):1473–84. doi: 10.1016/j.molonc.2016.08.002
99. Lohr K, Sardana H, Lee S, Wu F, Huso DL, Hamad AR, et al. Extracellular matrix protein lumican regulates inflammation in a mouse model of colitis. Inflammation Bowel Dis (2012) 18(1):143–51. doi: 10.1002/ibd.21713
100. Goodall KJ, Poon IKH, Phipps S, Hulett MD. Soluble heparan sulfate fragments generated by heparanase trigger the release of pro-inflammatory cytokines through TLR-4. PloS One (2014) 9(10):e109596. doi: 10.1371/journal.pone.0109596
101. Mcquitty CE, Williams R, Chokshi S, Urbani L. Immunomodulatory role of the extracellular matrix within the liver disease microenvironment. Front Immunol (2020) 11:574276. doi: 10.3389/fimmu.2020.574276
102. Ma W, Xue R, Zhu Z, Farrukh H, Song W, Li T. Increasing cure rates of solid tumors by immune checkpoint inhibitors. Exp Hematol Oncol (2023) 12(10):1–19. doi: 10.1186/s40164-023-00372-8
103. Xin Yu J, Hubbard-Lucey VM, Tang J. Immuno-oncology drug development goes global. Nat Rev Drug Discovery (2019) 18(12):899–900.
104. Jenkins RW, Barbie DA, Flaherty KT. Mechanisms of resistance to immune checkpoint inhibitors. Br J Cancer (2018) 118(1):9–16. doi: 10.1038/bjc.2017.434
105. Jensen C, Madsen DH, Hansen M, Schmidt H, Svane IM, Karsdal MA, et al. Non-invasive biomarkers derived from the extracellular matrix associate with response to immune checkpoint blockade ( anti- CTLA-4 ) in metastatic melanoma patients. J Immunother Cancer (2018) 6(152):1–10. doi: 10.1186/s40425-018-0474-z
106. Willumsen N, Thomsen LB, Bager CL, Jensen C, Karsdal MA. Quantification of altered tissue turnover in a liquid biopsy: a proposed precision medicine tool to assess chronic inflammation and desmoplasia associated with a pro-cancerous niche and response to immuno-therapeutic anti-tumor modalities. Cancer Immunol Immunother (2018) 67(1):1–12. doi: 10.1007/s00262-017-2074-z
107. Hurkmans DP, Jensen C, Koolen SLW, Aerts J, Karsdal MA, Mathijssen RHJ, et al. Blood-based extracellular matrix biomarkers are correlated with clinical outcome after PD-1 inhibition in patients with metastatic melanoma. J Immunother Cancer (2020) 8(2):e001193. doi: 10.1136/jitc-2020-001193
108. Jensen C, Nissen NI, Von Arenstorff CS, Karsdal MA, Willumsen N. Serological assessment of collagen fragments and tumor fibrosis may guide immune checkpoint inhibitor therapy. J Exp Clin Cancer Res (2021) 40(1):1–11. doi: 10.1186/s13046-021-02133-z
109. Willumsen N, Jensen C, Green G, Nissen NI, Neely J, Nelson DM, et al. Fibrotic activity quantified in serum by measurements of type III collagen pro-peptides can be used for prognosis across different solid tumor types. Cell Mol Life Sci (2022) 79(4):1–11. doi: 10.1007/s00018-022-04226-0
110. Pitt JM, Marabelle A, Eggermont A, Soria J-C, Kroemer G, Zitvogel L. Targeting the tumor microenvironment: removing obstruction to anticancer immune responses and immunotherapy. Ann Oncol Off J Eur Soc Med Oncol (2016) 27(8):1482–92. doi: 10.1093/annonc/mdw168
111. Xie J, Gui X, Deng M, Chen H, Chen Y, Liu X, et al. Blocking LAIR1 signaling in immune cells inhibits tumor development. Front Endocrinol (Lausanne) (2022) 13:1–14. doi: 10.3389/fimmu.2022.996026
112. Lebbink RJ, van den Berg MCW, de Ruiter T, Raynal N, van Roon JAG, Lenting PJ, et al. The soluble Leukocyte-Associated Ig-Like Receptor (LAIR)-2 antagonizes the collagen/LAIR-1 inhibitory immune interaction. J Immunol (2008) 1:2–9. doi: 10.4049/jimmunol.180.3.1662
113. Jensen C, Sinkeviciute D, Madsen DH, Önnerfjord P, Hansen M, Schmidt H, et al. Granzyme B degraded type IV collagen products in serum identify melanoma patients responding to immune checkpoint blockade. Cancers (Basel) (2020) 12(10):2786. doi: 10.3390/cancers12102786
114. Vijver SV, Singh A, Mommers-Elshof ETAM, Meeldijk J, Copeland R, Boon L, et al. Collagen fragments produced in cancer mediate T cell suppression through leukocyte-associated immunoglobulin-like receptor 1. Front Immunol (2021) 0:3725. doi: 10.3389/fimmu.2021.733561
115. Deng M, Gui X, Kim J, Xie L, Chen W, Li Z, et al. LILRB4 signalling in leukaemia cells mediates T cell suppression and tumour infiltration. Nature (2018) 562(7728):605–9. doi: 10.1038/s41586-018-0615-z
116. Sharma N, Atolagbe OT, Ge Z, Allison JP. LILRB4 suppresses immunity in solid tumors and is a potential target for immunotherapy. J Exp Med (2021) 218(7):e20201811. doi: 10.1084/jem.20201811
117. John S, Chen H, Deng M, Gui X, Wu G, Chen W, et al. A novel anti-LILRB4 CAR-T cell for the treatment of monocytic AML. Mol Ther (2018) 26(10):2487–95. doi: 10.1016/j.ymthe.2018.08.001
118. Anami Y, Deng M, Gui X, Yamaguchi A, Yamazaki CM, et al. LILRB4-targeting antibody–drug conjugates for the treatment of acute myeloid leukemia. Mol Cancer Ther (2020) 19(11):2330–9. doi: 10.1158/1535-7163.MCT-20-0407
119. Martellucci S, Clementi L, Sabetta S, Mattei V, Botta L, Angelucci A. Src family kinases as therapeutic targets in advanced solid tumors: what we have learned so far. Cancers (Basel) (2020) 12(1448):1–28. doi: 10.3390/cancers12061448
120. Liu J, Chiang H-C, Xiong W, Laurent V, Griffiths SC, Dülfer J, Deng H, et al. A highly selective humanized DDR1 mAb reverses immune exclusion by disrupting collagen fiber alignment in breast cancer. J Immunother Cancer (2023) 11(6):e006720. doi: 10.1136/jitc-2023-006720
121. Tu MM, Lee FYF, Jones RT, Kimball AK, Saravia E, Graziano RF, et al. Targeting DDR2 enhances tumor response to anti-PD-1 immunotherapy. Sci Adv (2019) 5:eaav2437. doi: 10.1126/sciadv.aav2437
122. Venning FA, Wullkopf L, Erler JT. Targeting ECM disrupts cancer progression. Front Oncol (2015) 5:1–15. doi: 10.3389/fonc.2015.00224
123. Henke E, Nandigama R, Ergün S. Extracellular matrix in the tumor microenvironment and its impact on cancer therapy. Front Mol Biosci (2020) 6:1–24. doi: 10.3389/fmolb.2019.00160
124. Dzobo K, Dandara C. The extracellular matrix: its composition, function, remodeling, and role in tumorigenesis. Biomimetics (2023) 8(2):146. doi: 10.3390/biomimetics8020146
125. Froeling FEM, Kocher HM. Homeostatic restoration of desmoplastic stroma rather than its ablation slows pancreatic cancer progression. Gastroenterology (2015) 148(4):849–50. doi: 10.1053/j.gastro.2015.02.043
126. Hauge A, Rofstad EK. Antifibrotic therapy to norMalize the tumor microenvironment. J Transl Med (2020) 18(207):1–11. doi: 10.1186/s12967-020-02376-y
127. Jiang H, Torphy RJ, Steiger K, Hongo H, Ritchie AJ, Kriegsmann M, et al. Pancreatic ductal adenocarcinoma progression is restrained by stromal matrix. J Clin Invest (2020) 130(9):4704–9. doi: 10.1172/JCI136760
128. Liu J, Liao S, Diop-Frimpong B, Chen W, Goel S, Naxerova K, et al. TGF-β blockade improves the distribution and efficacy of therapeutics in breast carcinoma by norMalizing the tumor stroma. Proc Natl Acad Sci U.S.A. (2012) 109(41):16618–23. doi: 10.1073/pnas.1117610109
129. Ludwig N, Wieteska Ł, Hinck CS, Yerneni SS, Azambuja JH, Bauer RJ, et al. Novel TGFb inhibitors ameliorate oral squamous cell carcinoma progression and improve the antitumor immune response of anti-PD-L1 immunotherapy. Mol Cancer Ther (2021) 20(6):1102–11. doi: 10.1158/1535-7163.MCT-20-0944
130. Nicolas-Boluda A, Vaquero J, Vimeux L, Guilbert T, Barrin S, Kantari-Mimoun C, et al. Tumor stiffening reversion through collagen crosslinking inhibition improves t cell migration and anti-pd-1 treatment. Elife (2021) 10:e58688. doi: 10.7554/eLife.58688.sa2
131. Jiang H, Hegde S, Knolhoff BL, Zhu Y, Herndon JM, Meyer MA, et al. Targeting focal adhesion kinase renders pancreatic cancers responsive to checkpoint immunotherapy. Nat Med (2016) 22(8):851–60. doi: 10.1038/nm.4123
132. Lander VE, Belle JI, Kingston NL, Herndon JM, Hogg GD, Liu X, et al. Stromal reprogramming by FAK inhibition overcomes radiation resistance to allow for immune priming and response to checkpoint blockade. Cancer Discovery (2022) 12(12):2774–99. doi: 10.1158/2159-8290.CD-22-0192
133. Jiang H, Hegde S, DeNardo DG. Tumor-associated fibrosis as a regulator of tumor immunity and response to immunotherapy. Cancer Immunol Immunother (2017) 66(8):1037–48. doi: 10.1007/s00262-017-2003-1
134. Mushtaq MU, Papadas A, Pagenkopf A, Flietner E, Morrow Z. Tumor matrix remodeling and novel immunotherapies: the promise of matrix- derived immune biomarkers. JITC (2018) 6(1):1–14. doi: 10.1186/s40425-018-0376-0
135. Ishihara J, Ishihara A, Sasaki K, Lee SSY, Williford JM, Yasui M, et al. Targeted antibody and cytokine cancer immunotherapies through collagen affinity. Sci Transl Med (2019) 11(487):eaau3259. doi: 10.1126/scitranslmed.aau3259
136. Momin N, Mehta NK, Bennett NR, Ma L, Palmeri JR, Chinn MM, et al. Anchoring of intratumorally administered cytokines to collagen safely potentiates systemic cancer immunotherapy. Sci Transl Med (2019) 11(498):1–14. doi: 10.1126/scitranslmed.aaw2614
137. Lutz EA, Hynes RO. Intratumoral nanobody – IL-2 fusions that bind the tumor extracellular matrix suppress solid tumor growth in mice. PNAS Nexus (2022) 1:1–12. doi: 10.1093/pnasnexus/pgac244
Keywords: cancer biology, collagen, cancer immunotherapy, ECM - extracellular matrix, LAIR-1, tumor microenvironment (TME)
Citation: Flies DB, Langermann S, Jensen C, Karsdal MA and Willumsen N (2023) Regulation of tumor immunity and immunotherapy by the tumor collagen extracellular matrix. Front. Immunol. 14:1199513. doi: 10.3389/fimmu.2023.1199513
Received: 03 April 2023; Accepted: 28 July 2023;
Published: 17 August 2023.
Edited by:
Zoi Piperigkou, University of Patras, GreeceReviewed by:
Sohel M. Julovi, Westmead Institute for Medical Research, AustraliaKevin Dzobo, University of Cape Town, South Africa
Copyright © 2023 Flies, Langermann, Jensen, Karsdal and Willumsen. This is an open-access article distributed under the terms of the Creative Commons Attribution License (CC BY). The use, distribution or reproduction in other forums is permitted, provided the original author(s) and the copyright owner(s) are credited and that the original publication in this journal is cited, in accordance with accepted academic practice. No use, distribution or reproduction is permitted which does not comply with these terms.
*Correspondence: Nicholas Willumsen, bndpQG5vcmRpY2Jpby5jb20=