- 1National Institute of Animal Biotechnology, Hyderabad, Telangana, India
- 2Regional Centre for Biotechnology, Faridabad, Haryana, India
- 3Department of Avian Sciences, West Bengal University of Animal and Fishery Sciences, Kolkata, West Bengal, India
- 4Department of Animal Genetics and Breeding, West Bengal University of Animal and Fishery Sciences, Kolkata, West Bengal, India
Tuberculosis (TB) in the bovine is one of the most predominant chronic debilitating infectious diseases primarily caused by Mycobacterium bovis. Besides, the incidence of TB in humans due to M. bovis, and that in bovines (bovine TB, bTB) due to M. tuberculosis- indicates cattle as a major reservoir of zoonotic TB. While India accounts for the highest global burden of both TB and multidrug-resistant TB in humans, systematic evaluation of bTB prevalence in India is largely lacking. Recent reports emphasized markedly greater bTB prevalence in exotic and crossbred cattle compared to indigenous cattle breeds that represent more than one-third of the total cattle population in India, which is the largest globally. This study aimed at elucidating the immune responses underlying the differential bTB incidence in prominent indigenous (Sahiwal), and crossbred (Sahiwal x Holstein Friesian) cattle reared in India. Employing the standard Single Intradermal Tuberculin Test (SITT), and mycobacterial gene-targeting single as well as multiplex-PCR-based screening revealed higher incidences of bovine tuberculin reactors as well as Mycobacterium tuberculosis Complex specific PCR positivity amongst the crossbred cattle. Further, ex vivo mycobacterial infection in cultures of bovine peripheral blood mononuclear cells (PBMC) from SITT, and myco-PCR negative healthy cattle exhibited significantly higher intracellular growth of M. bovis BCG, and M. tuberculosis H37Ra in the crossbred cattle PBMCs compared to native cattle. In addition, native cattle PBMCs induced higher pro-inflammatory cytokines and signaling pathways, such as interferon-gamma (IFN-γ), interleukin-17 (IL-17), tank binding kinase-1 (TBK-1), and nitric oxide (NO) upon exposure to live mycobacterial infection in comparison to PBMCs from crossbred cattle that exhibited higher expression of IL-1β transcripts. Together, these findings highlight that differences in the innate immune responses of these cattle breeds might be contributing to the differential susceptibility to bTB infection, and the resultant disparity in bTB incidence amongst indigenous, and crossbred cattle.
Introduction
Bovine tuberculosis (bTB) is a globally prevalent chronic debilitating infectious disease of cattle with a considerable impact on the public health and farm economy. Of the 188 countries and territories globally reporting their bovine TB (bTB) situation to the World Organisation of Animal Health (OIE), 82 countries (44%) reported bTB prevalence (1). Notably, while 97.6% of the affected countries reported bTB prevalence in livestock, 35.4% of countries documented bTB presence in both livestock and wildlife animals (1). In addition, the incidence of TB in humans and bovines due to either the human or bovine tubercle bacilli signifies the impact of bTB on livestock farming, and highlights its transmission between cattle and humans (2–4). Since, about 54.6% of the total workforce in India is engaged in agriculture and animal husbandry, and livestock provides livelihood to two third of the rural community, therefore, bTB exerts a hugely adverse effect on public health (3, 5).
Bovine TB has been largely controlled in many high-income countries due to strict implementation of bTB control programs and policies, whereas in lower, and lower-middle-income countries, control of bTB still poses a major challenge (6, 7). This is largely because of unhygienic farm management practices, lack of regular surveillance, and lack of strict prevention, and control policies. While a meta-analysis of published literature on bTB reported prevalence rates of 2-50% in cattle in India, the true incidence of bTB in India remains ambiguous in the absence of routine national bTB surveillance (8). Seminal findings in the past showed lower incidences of bTB in the indigenous Indian zebu cattle (Bos indicus) compared to exotic European cattle (Bos taurus) (9–14). Susceptibility to bTB has also been estimated to be influenced by various factors such as herd size, nutritional requirement, age, sex, and dairy farm management practices (9, 15, 16). A recent study reported significantly higher bTB prevalence in exotic and crossbred cattle than in indigenous cattle breeds (17). A plethora of studies in the mouse model as well as in humans has indicated that the genetic makeup of a host substantially influences the intracellular survival of mycobacteria by inducing differential immune responses (18–23). However, a systematic study to compare the underlying immune responses amongst indigenous Indian cattle, and crossbred cattle has not been reported. We hypothesized that higher incidences of bTB in the crossbred cattle might be arising due to inadequate immune response to bTB infection compared to the native cattle breeds. To compare the innate cellular responses, first, we segregated the healthy, single intradermal tuberculin test (SITT) negative, and myco-PCR [PCR targeting M. tuberculosis, M. bovis, M. orygis, Mycobacterium tuberculosis complex (MTC)] and Non-Tuberculous Mycobacteria (NTM) negative cattle from two prominent breeds- indigenous breed Sahiwal, and crossbred- Sahiwal x Holstein Friesian (SHF). Subsequently, we performed a comparative mycobacterial growth assay in the PBMCs isolated from these healthy mycobacterium-naive cattle. Concurrently, we compared the innate immune cytokine responses induced by the PBMCs upon mycobacterial infection and antigenic stimulation. We identified considerable differences in key pro-inflammatory cytokine responses between these breeds that potentially contribute to the differential susceptibility to mycobacterial infection and varied incidence of bTB in these breeds of cattle in India.
Results
Higher incidence of tuberculin reactors and myco-PCR positivity in crossbred cattle
To identify, and segregate bTB-negative animals we performed standard SITT, and myco-PCR-based screening of both indigenous Sahiwal breed, and SHF crossbred cattle from a dairy herd (Figure 1). Comparison of SITT response to bovine tuberculin was performed on 24 Sahiwal, and 26 SHF cattle. A total of 9 animals were found to be bovine tuberculin reactors equating to SITT positivity of 18% (9/50) (Figure 1A). Estimation of the breed-specific tuberculin reactors revealed 8.33% (2/24) positivity among Sahiwal cattle, whereas 26.92% (7/26) positivity in the case of crossbred SHF cattle. Concurrently, our myco-PCR methodology that involves a combination of singlet PCRs using previously published primers that detect M. bovis, M. tuberculosis, M. orygis, MTC, and pan non-tuberculous mycobacterial (NTM) DNA including the Mycobacterium avium complex (MAC) (Supplementary Figure S1), and an in-house assembled multiplex-PCR that simultaneously detects and differentiate M. bovis, M. bovis BCG, M. tuberculosis and pan NTM DNA in the cattle milk and urine samples (Figure 1B) revealed presences of 4% of M. bovis positivity (2/50, RD1+, RD4+), 6% M. tuberculosis positivity (3/50, RD1+, RD4-), 14% MTC positivity (7/50), and 32% NTM positivity (16/50) (Table 1) (24–27). Altogether, a considerably higher number of crossbred cattle was found to be myco-PCR positive (8/26) compared to the native Sahiwal breed (1/24). Supplementary Table S1 depicts the detailed distribution of SITT and myco-PCR assay results among all the animals. The primers targeting different mycobacteria are listed in Supplementary Table S2. We have excluded animals showing SITT positivity, or PCR positivity to any of the mycobacterial species screened in this study for the subsequent evaluation of mycobacterial growth, host cellular responses to mycobacterial infection, and antigenic stimulation (Table 1 and Supplementary Table S1). These SITT-negative and Mycobacterial-PCR-negative cattle were considered mycobacteria-naïve animals that are expected to show primary immune responses when exposed to mycobacterial infection of antigenic stimulation.
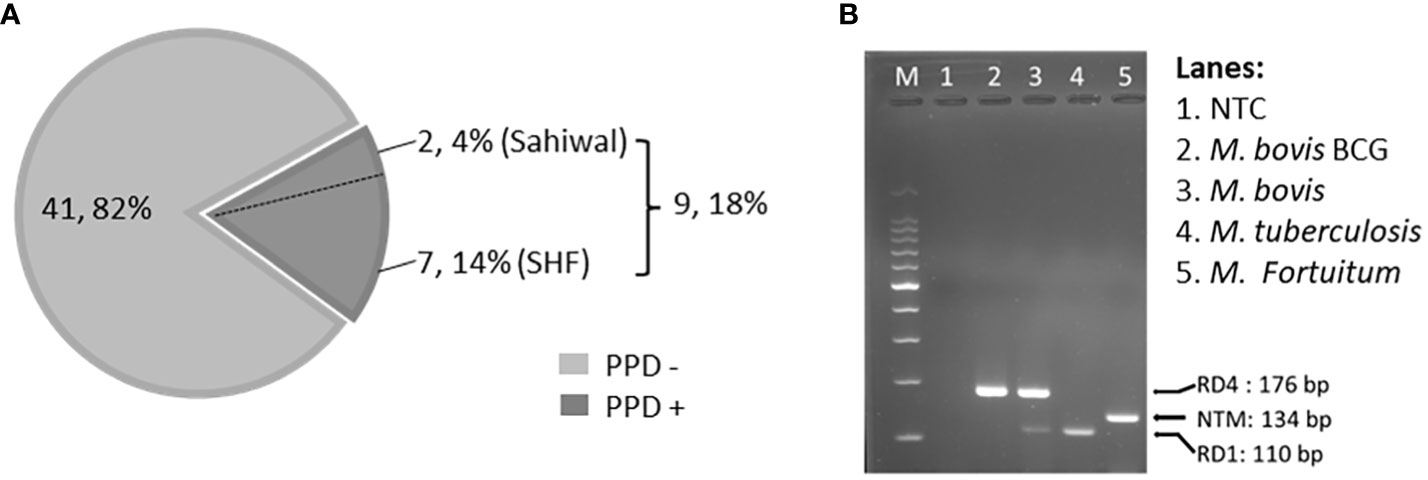
Figure 1 Single Intradermal Tuberculin Test, and PCR-based detection of mycobacterial DNA. (A) The pie chart depicts the incidence of SITT positivity in cattle. A total of 50 cattle were analyzed by SITT. Out of 50 cattle, 9 (18%) were tuberculin reactors. Out of 9 SITT+ cattle, 7 cattle (14%) belonged to Cross Breed and the remaining 2 (4%) were Sahiwal cattle. (B) Agarose gel electrophoresis image of the multiplex PCR using a combination of, - a primer pair targeting specific DNA sequence of the Region of Difference-1 (RD-1) that detects both M. bovis, and M. tuberculosis but not BCG, or NTMs, - a primer pair targeting the upstream and downstream sequences of the RD-4 region specifically detecting BCG, and M. bovis DNA but not M. tuberculosis or NTMs, and – a primer pair that detects the presence of pan NTMs DNA. NTC, no treatment control.
Crossbred cattle PBMCs are conducive to mycobacterial replication
For a comparative evaluation of the permissiveness of the two breeds of cattle to mycobacterial infection, a bovine PBMC-mycobacteria in vitro infection assay was established. First, we generated reporter strains of M. bovis BCG, and M. tuberculosis H37Ra expressing mCherry and tdTomato via episomal plasmids pMSP12::mCherry and pTEC27-Hyg, respectively (Supplementary Table S3) (28). The correlation of the fluorescence of the reporter mycobacterial strains to the CFU was evaluated both in the 7H9 broth culture (Supplementary Figures S2A, B), as well as in the bovine macrophage cells (BOMAC) (Supplementary Figures S2C, D) (29, 30). The association of the reporter mycobacterial number to total fluorescence was found to be in strong agreement, and a bacterial number-dependent increase in the total fluorescence was observed over five days in both broth culture and BOMAC cell culture (Supplementary Figure S2). A pre-calibrated MOI of 1:10 (Cell: Bacteria) was used for a 5 days-long bovine PBMC culture along with fluorescence measurement at an interval of 24 hours following infection to evaluate the comparative mycobacterial growth in two breeds of cattle (Figure 2). The mean fluorescence of M. bovis BCG in the SHF derived PBMC exhibited increasing trend compared to the PBMCs derived from Sahiwal breed of cattle, and at day-5 post-infection the bacterial fluorescence was found to be significantly higher in the former group compared to the later (Figure 2A). Further, PBMCs from Sahiwal breed showed a considerably lower fluorescence for M. tuberculosis H37Ra over the course of infection which is significantly lower at day-4 and day-5 post-infection highlighting restricted growth of the bacteria in comparison to the PBMCs from the crossbred SHF cattle (Figure 2B). These observations clearly indicate that indigenous Sahiwal breed of cattle possess significantly greater control over the growth of M. bovis, and M. tuberculosis strains in comparison to the crossbred SHF cattle.
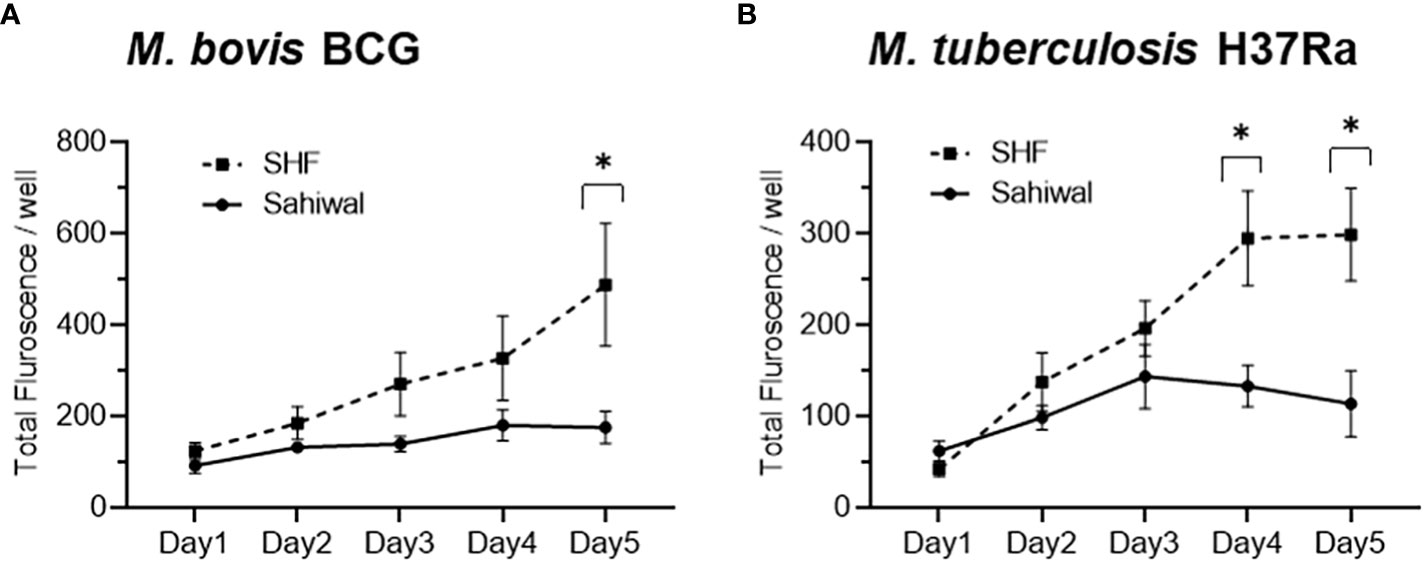
Figure 2 Growth of mycobacteria in bovine PBMC. PBMC from Sahiwal and SHF cattle were infected with fluorescence reporter (A) M. bovis BCG-mCherry; λex:587nm and λem:610nm, and (B) M. tuberculosis H37Ra-tdTomato; λex:554nm and λem:583nm at an MOI of 1:10 (cell: bacteria). The fluorescence intensity of reporter mycobacteria strains was monitored every 24 hours for 5 days post-infection, and data is depicted as line plots by adjoining the mean ± SEM fluorescence/well measured every day for each mycobacterial strain. n = 6, *,p<0.05 (t-test). The data is representative of two experiments.
Higher IFN-γ production by PBMCs from indigenous cattle breed upon mycobacterial infection, and antigenic stimulation
IFN-γ is an important cytokine that regulates innate as well as acquired cell-mediated and humoral immunity to infection by eliciting a number of biological responses in several cell types (31, 32). IFN-γ plays a pivotal role in exerting the host’s protective immunity against Mycobacterial infection (33, 34). Evaluation of the protein level of IFN-γ by ELISA in the bPBMC culture media at 24-hour post-infection with M. bovis BCG, and M. tuberculosis H37Ra revealed a significant difference between Sahiwal and SHF cattle (Figure 3). In the first set of experiments, PBMCs from Sahiwal cattle showed a higher production of IFN-γ than SHF cattle when exposed to M. bovis BCG, and M. tuberculosis H37Ra infection (Figure 3A), while LPS stimulation resulted in a comparable level of IFN-γ production by PBMCs from both the sources. This observation was reconfirmed by subsequent experiments wherein in addition to M. bovis BCG, M. tuberculosis H37Ra infection, bPBMC were also stimulated with bovine PPD (PPD-B), avium PPD (PPD-A), M. tuberculosis- whole cell lysate (WCL), cell wall (CW), and lipoarabinomannan (LAM). We observed that the IFN-γ levels at 24-hour post-infection were significantly higher in PBMCs from Sahiwal cattle than SHF cattle in the case of M. bovis BCG, and M. tuberculosis H37Ra infection, and PPD-B stimulation (Figure 3B). For the rest of the stimulant groups no considerable difference was observed. These observations indicate higher induction of IFN-γ by PBMC from indigenous Sahiwal cattle during infection might contribute to the restriction of mycobacterial growth and the resultant lower incidence of bTB in this breed of cattle.
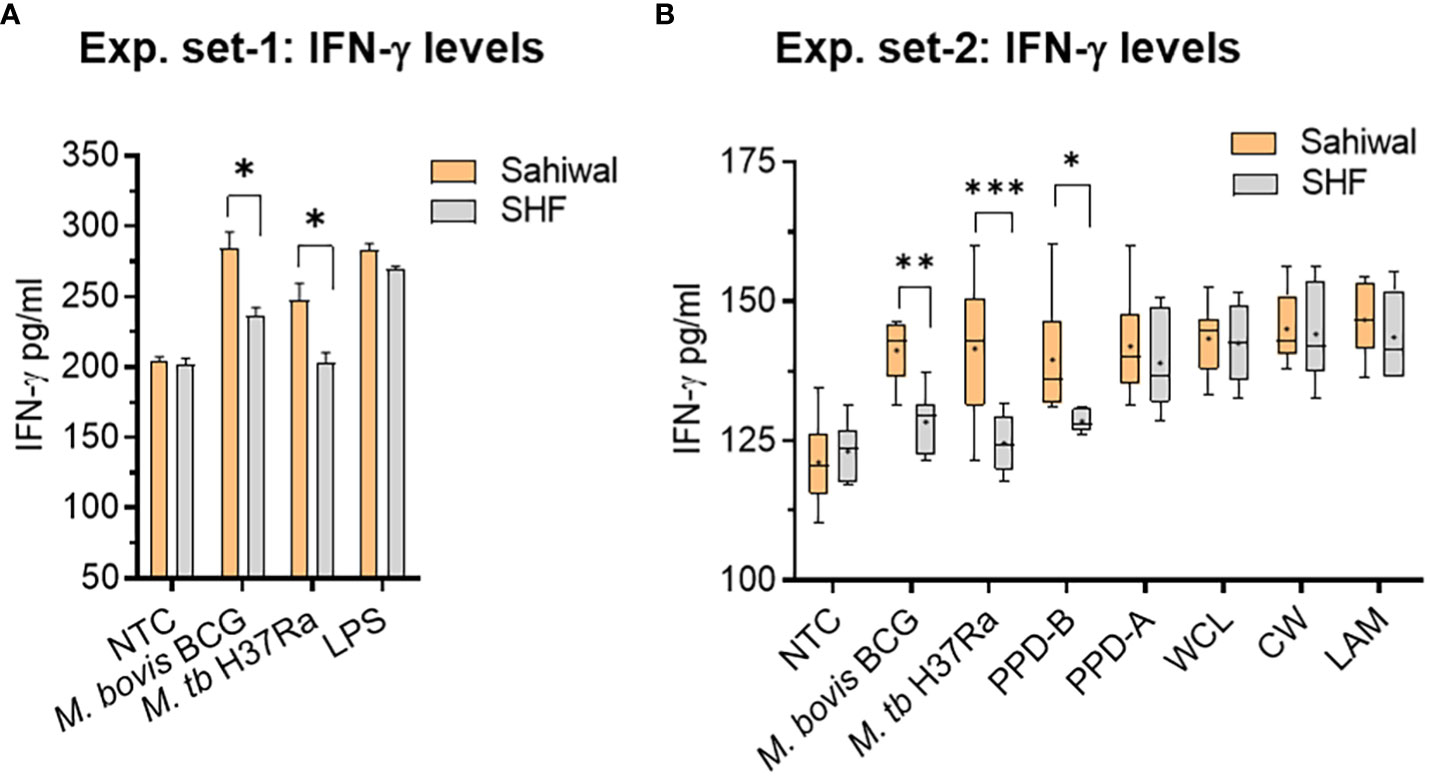
Figure 3 IFN-γ response of bovine PBMC to mycobacterial infection and antigenic stimulation. Two separate sets of experiments were performed at an interval of six months using freshly prepared PBMCs from same cohort of cattle. In the first set of experiment, (A) the PBMCs obtained from SITT and myco-PCR negative Sahiwal and SHF cattle were infected with M. bovis BCG or M. tuberculosis H37Ra at an MOI of 1:10 (cell: bacteria) or stimulated with Lipopolysaccharide (LPS, 5ug/ml). The bar graph represents IFN-γ level in the culture supernatant (pg/ml). Data is mean ± SEM, n=3 animals per group, *,p<0.05. In the second set of experiment, (B) PBMC were either infected with M. bovis BCG or M. tuberculosis H37Ra at an MOI of 1:10 (cell: bacteria) or stimulated with bovine PPD (PPD-B, 300 IU/ml), Avian PPD (PPD-A, 250 IU/ml), M. tuberculosis whole cell lysate (WCL, 5 ug/ml), cell wall (CW, 5 ug/ml) and lipoarabinomannan (LAM, 5ug/ml). IFN-γ level was measured at 24 hours post-infection and graphically represented by a box plot, wherein median values are denoted by the horizontal line, the mean is represented by ‘+’, the interquartile range by boxes, and the maximum and minimum values by whiskers. n=6 animals per group. *, P < 0.05; **, P < 0.01; ***, P < 0.001 (t-test). The data is representative of two experiments. NTC, no treatment control.
Transcriptional induction of pro-inflammatory immuno-signature by indigenous cattle PBMCs
Pathogenesis of pulmonary TB largely depends on the orchestration of the players of the cellular immune system and a synchronized interaction of various pro- and anti-inflammatory cytokines at the site of infection (18, 31, 33). A fine-tuning of multiple cytokines is essential to an effective clearance of the pathogen (35–37). RNA extracted from the PBMCs from the above experiment at 24 hours post-infection were analyzed for several major cytokines, and signaling molecules by real-time RT-PCR using gene-specific primers (Supplementary Table S4). These includes IFN-γ, IL-17, TNF-α, IFN-β, IL-1β, IL-6, IL-10, cGAS, STING, TBK1, IRF3, and IRF7. Figure 4 depicts the relative expression of relevant immune-response genes in bar diagrams. Of these various immunological mediators, significantly higher transcriptional induction of IFN-γ was observed in case of PBMCs from Sahiwal cattle than crossbred cattle when infected with M. bovis BCG and M. tuberculosis H37Ra, (Figure 4A). In addition, a similar pattern of significantly higher induction was observed in case of IL-17 gene expression by PBMCs from Sahiwal cattle compared to the PBMCs from crossbred SHF cattle when exposed to M. bovis BCG and M. tuberculosis H37Ra infection (Figure 4B). The serine/threonine kinase TBK-1, which is known for its involvement in the innate immune response to infection by mediating the cGAS-STING-IFN-β axis of cytosolic surveillance pathway, was also found to be significantly upregulated following M. bovis BCG infection of the PBMCs from Sahiwal cattle in comparison to the PBMCs from crossbred cattle (Figure 4C). In contrast, IL-1β expression was significantly higher in crossbred cattle PBMCs upon infection with both M. bovis BCG and M. tuberculosis H37Ra. (Figure 4D). Rest of the genes analyzed in this study exhibited a comparable expression pattern in case of both the breeds of cattle (Supplementary Figure S3). Stimulation with PPD-B, PPD-A, WCL, CW, and LAM did not exhibit a considerable difference in gene expression between the two breeds of cattle (data not shown). Our findings from quantitative real-time PCR indicates differential transcriptional regulation of important cytokines and signaling pathway such as IFN-γ, IL-17, IL-1β, and TBK-1 upon mycobacterial infection may contribute to the differential permissiveness of the two breeds of cattle to bTB infection.
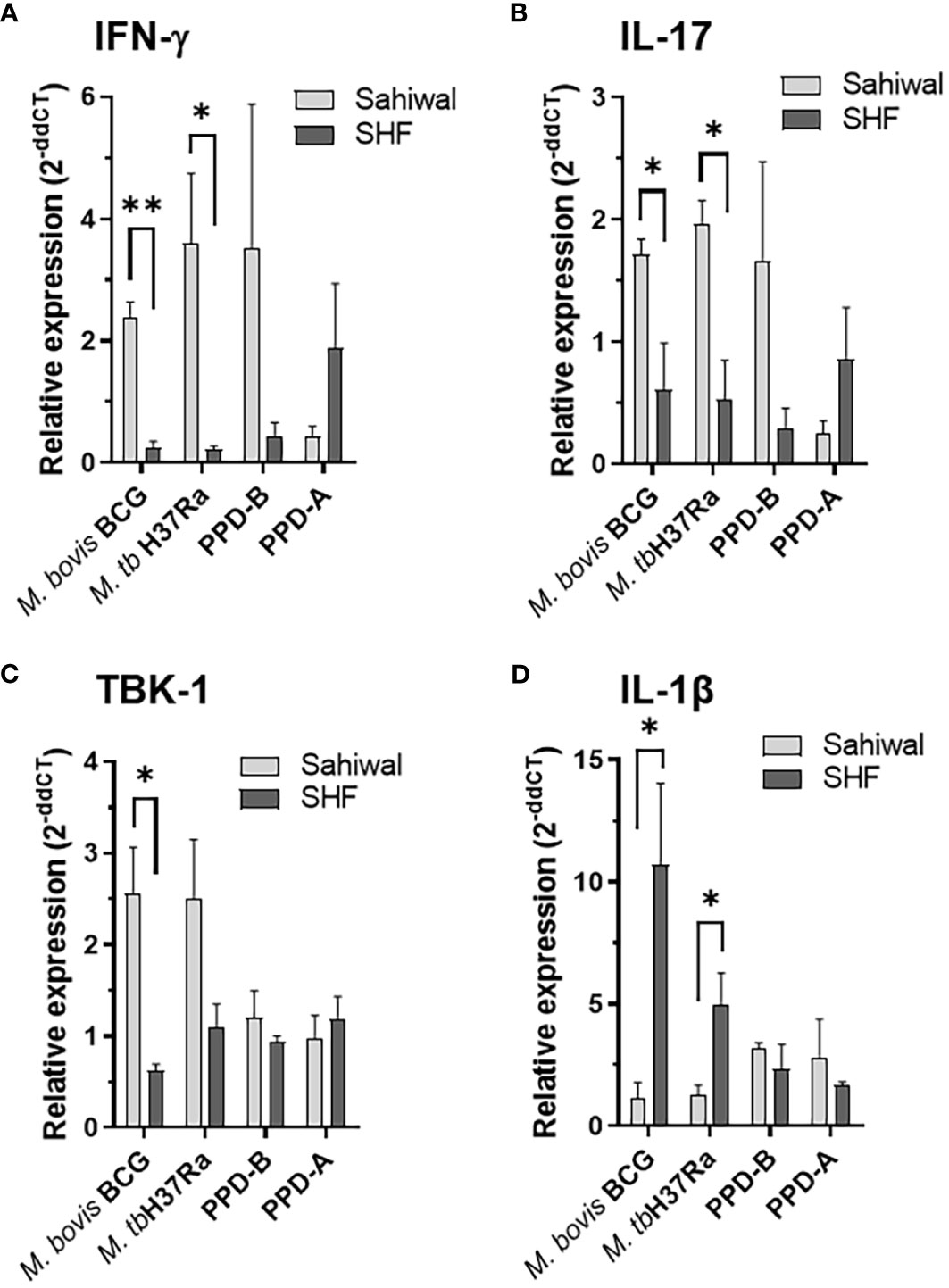
Figure 4 Modulation of host gene expression in bovine PBMC by mycobacterial infection and antigenic stimulation. Expression of various cytokines and immunity-related genes were measured on the RNA extracted from PBMC infected with M. bovis BCG or M. tuberculosis H37Ra at an MOI of 1:10 (cell: bacteria) or stimulated with bovine PPD (PPD-B, 300 IU/ml) and Avium PPD (PPD-A, 250 IU/ml at 24-hour post-infection by semi-quantitative real-time RT-PCR using gene-specific primers. (A) IFN-γ, (B) IL-17, (C) TBK-1 and (D) IL-1β. The data were normalized to RPLP0 expression levels and then normalized to the values of uninfected/unstimulated cells to obtain ddCT values. Data is represented as a bar diagram of mean ± SEM of 2-ddCT values as relative expression, n=3, *, p<0.05 (t-test); **, p<0.01.
Higher nitric oxide production by PBMCs from indigenous cattle
Production of NO by macrophages represents an important defense mechanism against M. tuberculosis and contributes to the host’s ability to control and combat the infection (38, 39). We measured nitrite levels in the PBMC culture supernatants, which is an indirect measure of NO production. As shown in Figure 5, PBMCs from the Sahiwal cattle produced relatively higher levels of NO over the crossbred cattle PBMCs upon Mycobacterial infection or antigenic stimulation. Notably, a statistically significant difference was observed in the case of M. tuberculosis H37Ra infection and PPD-A stimulation.
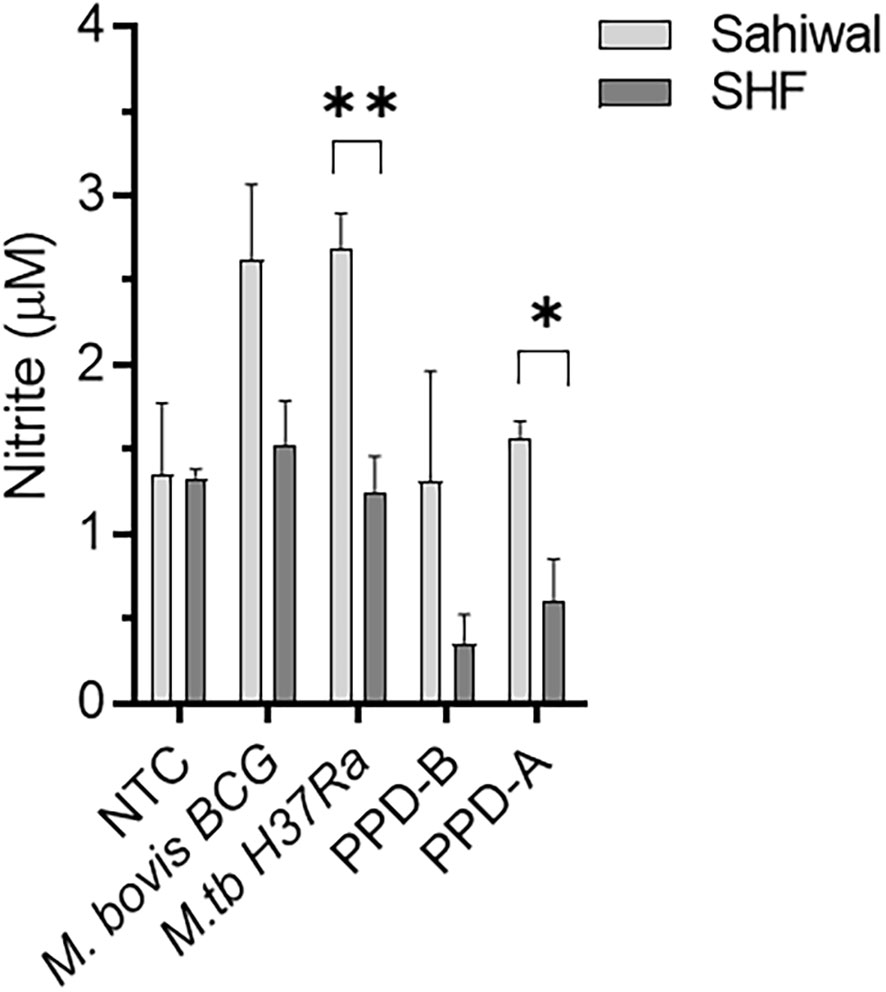
Figure 5 Nitric Oxide production by bovine PBMCs upon Mycobacterial infection and antigenic stimulation. PBMC were either infected with M. bovis BCG or M. tuberculosis H37Ra at an MOI of 1:10 (cell: bacteria) or stimulated with bovine PPD (PPD-B, 300 IU/ml), Avian PPD (PPD-A, 250 IU/ml). Culture supernatant was separated at 24 hours post-infection and subjected to Griess assay for nitrite, a stable metabolite of NO. The bar diagram depicts the Mean ± SEM of Nitrite levels in the culture media. n=3 animals per group. *, P < 0.05, **, P < 0.01, (t-test). NTC, No treatment control. The data are representative of two similar experiments.
Discussion
The susceptibility and/or resistance of a host to TB is influenced by multiple factors which include: the nutritional status of the host, age, sex, underlying diseases, host genetic traits, and interaction between the host and the environment (16). Numerous studies have indicated that genetic diversity among organisms contributes immensely to the differential immune response (21, 22). A number of studies reported that bTB was more prevalent in crossbred cattle compared to the indigenous cattle breeds in India (17, 40). Thakur and colleagues investigated the prevalence of bTB in an organized farm, and a cow shelter in northern India and reported higher bTB positivity in Jersey crossbreds (40). Das and colleagues reported markedly higher incidences of bTB in exotic and crossbred cattle (34.6%) compared to indigenous cattle (10.5%) in India (17). A higher incidence and severity of pathology of bTB in the Holsteins breed compared to Zebu breeds was reported in a study conducted in central Ethiopia (10). As it is apparent that indigenous breeds of cattle have a markedly lower incidence of bTB compared to the exotic and crossbred cattle, a comparison of immune responses to bTB infection in these cattle may discern the clue of protective immune signature to bTB in cattle.
India is home to the largest cattle population in the world with an array of indigenous, and crossbred varieties with enormous genetic variability. Cross-breeding practices remained a preferred approach to enhance the milk yield of indigenous dairy breeds of cattle for more than half a century in India (41). Especially, the use of European donor breeds such as Holstein Friesian, Jersey, Brown Swiss, Red Dane, etc. for improving non-descript Indian cattle as well as pure-breed indigenous cattle, and the impact of cross-breeding on milk production, reproductive performance, and sustainability in Indian agro-climatic conditions were thoroughly studied via a number of cattle development programs. Exotic inheritance of 1/2 and 5/8 was found to be superior in milk production and sustainability parameters in the majority of the studies compared to other genetic grades (41, 42). Lower or higher exotic inheritance than the above-mentioned grades did not result in any economic benefit from such cross-breeding practices but rather resulted in unsustainable breed quality in the Indian agro-climatic conditions. As per the last livestock census, crossbred cattle represent more than one-third of India’s total cattle population and contribute to nearly 48% of total cow milk (43). However, how cross-breeding has influenced the susceptibility and/or resistance trait to different infectious diseases, and the underlying genetic and immunological mechanisms are rarely evaluated systematically.
Here, we studied two of the most prominent dairy breeds of cattle in India, indigenous Sahiwal and crossbred SHF. The crossbred SHF animals included in this study possess 50%-62.5% exotic inheritance. Using myco-PCR alone, and a combination of both standard SITT assay and myco-PCR, we found a significantly (p<.05 and p<.01, respectively) higher incidence of tuberculin reactors-cum-PCR positivity in SHF cattle compared to Sahiwal cattle (Table 2). Our findings are in accordance with the previous studies where a higher percentage of tuberculin reactors was seen in exotic/crossbred cattle than in native cattle (10, 15, 44, 45). Further, the Mycobacterial-PCR assays enabled us to detect the presence of MTC and NTM genomic DNA in the milk and urine samples isolated from cattle. The presence of NTM may interfere not only with the SITT readout but also may affect the cellular immune responses of PBMCs isolated from the cattle. Application of such PCR assays provides a cost-effective method to detect not only the species of the infecting mycobacteria in the clinical settings but also allowed us to identify mycobacterial infection-free cattle to be included in the subsequent ex vivo PBMC-based experiments.
IFN-γ is the key cytokine indispensable in defence against TB. IFN-γ activated macrophages enhance the microbicidal activity of macrophages by allowing the formation of phagolysosomes wherein the mycobacteria are deprived of essential nutrients such as iron, and exposed to anti-microbial peptides, and reactive oxygen or nitrogen intermediates (46–48). Lower production of IFN-γ indicates a reduced activity of macrophages which promotes mycobacterial growth (32, 37). The findings from ELISA and real-time RT-PCR indicated a significantly higher induction of IFN-γ response by PBMCs from Sahiwal cattle compared to crossbreed cattle PBMCs indicating induction of superior anti-TB immune responses in the native Sahiwal cattle (Figures 3, 4). This observation was supported by the lower growth of both M. bovis BCG and M. tuberculosis H37Ra strains in the PBMC cultures of Sahiwal cattle compared to the crossbred SHF cattle (Figure 2). Notably, only live Mycobacterial infection and PPD-B stimulation resulted in differences in IFN-γ levels, whereas neither LPS stimulation nor stimulation with other M. tuberculosis derived antigen complexes such as LAM, CW, and WCL showed any differences between the breeds. This may be due to the upregulation and secretion of immunodominant antigens during live bacilli infection, and the dominant presence of such antigens in PPD-B, resulting in differential immune activation by PBMCs from the two cattle breeds through a mechanism that is yet to be identified. Additionally, comparable immune responses to LPS suggest the presence of similar TLR-4 mediated responses by PBMCs from both cattle breeds.
Although IFN-γ plays a key role in the defence against TB, this cytokine alone can’t generate the necessary immune response to provide protection against TB. The TB disease progression is controlled by a coordinated network of several different cytokines, chemokines, and signaling molecules. We analyzed the mRNA levels of a number of pro-inflammatory, anti-inflammatory, and immuno-regulatory mediators in addition to IFN-γ such as IL-17, TNF-α, IFN-β, IL-1β, IL-10, cGAS, STING, TBK1, IL-6, IRF3, and IRF7. While IL-17, IL-1β, and TBK1 exhibited considerable differential regulation in the mRNA levels, a comparable level of expression was observed for the rest of the immune mediators (Figure 4). A significantly higher induction of IL-17 was exhibited by PBMCs from Sahiwal cattle compared to crossbreed cattle PBMCs (Figure 4B). IL-17 is another key cytokine involved in exhibiting protective immunity against M. tuberculosis infection. It plays a major role in combating the growth of the tubercle bacilli by promoting a Th1-biased immune response (49). The differentiation of Th17 cells occurs as a result of an increase in the level of pro-inflammatory cytokines such as IL-6, IL-23, IL-1β, and TNF-α (36, 49–51). IL-17 induces the recruitment of neutrophils, macrophages, and Th1 cells to the site of inflammation. IL-17 also restricts the growth of Mycobacteria by inducing the expression of various chemokines, and by recruiting IFN-γ-producing cells (35). In contrast, IL-1β expression was significantly higher in crossbred cattle PBMCs upon mycobacterial infection (Figure 4D). While IFN-γ and IL-17 primarily promote macrophage activation, granuloma formation, and clearance of intracellular tubercle bacilli, IL-1β has been implicated in aggravated inflammation (52). Further, phosphorylation of TBK-1 is involved in a plethora of intracellular signaling events including the cGAS-STING-IFN-β axis of the cytosolic surveillance pathway of the host to respond to invading infectious agents including regulation of cell proliferation, autophagy, and apoptosis (53, 54). While the role of TBK-1 in antiviral response is well documented, divergent views on the anti-bacterial effect especially anti-mycobacterial responses are linked to this cytosolic kinase (55–58). Several studies reported the essentiality of the TBK-1 phosphorylation-mediated activation of the IRF-1 pathway for mycobacterial clearance, while others associated it with higher immunopathology (56, 57). These observations indicate the necessity of tightly regulated TBK-1-mediated signaling for a host-favored immune response.
During M. tuberculosis infection, the association of increased IFN-γ and with increased NO production by macrophages plays a critical role in host defense against the pathogen (38, 39). IFN-γ is a pro-inflammatory cytokine produced by activated T cells and natural killer cells, and it stimulates macrophages to enhance their antimicrobial activities (46). When macrophages encounter M. tuberculosis, the production of IFN-γ is triggered, leading to the activation of macrophages. Activated macrophages then produce increased levels of NO through the inducible nitric oxide synthase pathway, which serves as a potent bactericidal agent against M. tuberculosis. Thus, increased IFN-γ levels coupled with enhanced NO production by PBMCs from Sahiwal cattle represents a crucial immune response that aids in the control of the Mycobacterial growth.
In some, our study elucidated the association of important mediators of immune responses with the differential bTB susceptibility phenotype of the indigenous Sahiwal cattle and the crossbred SHF cattle by employing ex vivo bovine PBMC-mycobacterial infection model using M. bovis BCG vaccine strain, and M. tuberculosis H37Ra strain. Especially, our study highlighted that divergence in the expression of host factors such as IFN-γ, IL-17, IL-1β, and TBK-1 potentially play a major role in determining the degree of susceptibility to mycobacterial infection in cattle. In addition, heightened activation of macrophages as evident from increased IFN-γ, and NO levels in the Sahiwal cattle might be contributing to a greater control of Mycobacterial growth. However, it is important to acknowledge certain limitations to this study that require further investigation. Based on our use of BSL2 grade mycobacteria- M. bovis BCG and M. tuberculosis H37Ra, this study primarily serves as a proof-of-principle that susceptibility to bTB is higher in crossbred SHF cattle compared to native Sahiwal cattle. Further studies with virulent human and bovine tubercle bacilli, a greater number of indigenous and crossbred cattle, and application of genomic, transcriptomic and proteomic approaches would elucidate the association of the global immune response signature to bTB susceptibility and/or resistance in cattle.
Our study also highlighted the importance of the use of PCR-based mycobacterial DNA detection and differentiation of mycobacteria species in studies that allow the identification of mycobacterial infection-naïve cattle to evaluate the innate immune response of PBMCs to mycobacterial stimulation or infection. Further, a highly sensitive multiplex-PCR-based assay would be immensely useful for screening cattle herds as well as human clinical TB cases that would aid in the epidemiological characterization of causative mycobacterial species and devising appropriate treatment strategies.
Finally, this study is an important step forward toward identifying the association of bTB susceptibility to the underlying innate immune responses in indigenous and crossbred cattle in India. This study addresses an extremely important yet untouched aspect of the bTB scenario in Indian cattle which is identifying host factors conferring susceptibility and/or resistance to bTB that remained one of the biggest public health problems for centuries. Our results not only provide proof-of-concept data for the hypothesis that genetic variability of bovine due to breed variation influences bTB susceptibility and resistance but also provide a reason for adopting an appropriate crossbreeding policy that balances production and disease resistance traits for sustainable livestock farming.
Materials and methods
Bacteria, plasmids, and generation of reporter mycobacterial strains
The mycobacterial strains and plasmids used in the study are listed in Supplementary Table S1. M. bovis BCG (Danish 1331 sub-strain), and M. tuberculosis H37Ra strain was kindly provided by Prof. S. Banerjee, University of Hyderabad, India. Non-Tuberculous Mycobacteria (NTM) M. fortuitum was procured form MTCC, CSIR-IMTECH, Chandigarh, India. Mycobacterial strains were grown to mid-log phase in Middle Brook (MB) 7H9 media and glycerol stocks were prepared and stored at -80°C as described earlier (59). For in vitro infection, fresh bacterial cultures were grown to the mid-log phase, and bacterial cells were washed thoroughly with 1XPBS, and finally resuspended in cell-culture media following pre-calibrated dilutions, as described previously (56). For generating Mycobacterial reporter strains first, electrocompetent cells of M. bovis BCG and M. tuberculosis were prepared as described previously (56), and transformed with pMSP12::mCherry (was a gift from Lalita Ramakrishnan, Addgene plasmid # 30169; http://n2t.net/addgene:30169), and pTEC27-Hyg (was a gift from Lalita Ramakrishnan, Addgene plasmid # 30182; http://n2t.net/addgene:30182) (28). Briefly, 100µl of competent Mycobacterial cells were mixed with 100ng of plasmid DNA, and transferred to a Micro Pulser Electroporation cuvette (Biorad # 1652086 with 0.2 cm gap) and pulsed at 2500V, 25uF capacitance, and 1000 Ω resistance using an electroporator (Gene Pulser Xcell Microbial System #1652662, Bio-Rad). The cells were immediately aspirated and inoculated in 2ml of MB-7H9 broth without any selective antibiotics and incubated at 37°C under shaking conditions for 48 hours. Subsequently, bacterial cells are plated onto MB-7H11 agar containing selective antibiotics- 25 µg/ml of Kanamycin, and 150 µg/ml of Hygromycin, respectively, and incubated at 37°C for 4 weeks. Transformed bacterial clones grown on the selective plates were detected by colored colonies, and also confirmed for the presence of plasmid by colony PCR, and subsequently confirmed by fluorescence microscopy. Glycerol stocks of the reporter mycobacterial strains were prepared, and stored for future use. All the mycobacterial strains used in this study were of the BSL-2 category, and these are cultured in a BSL-2 laboratory.
Genomic DNA extraction from mycobacteria
Standard methodology was followed for genomic DNA extraction from various mycobacteria as described previously (60). Briefly, the bacterial culture was incubated with 1% glycine in a 37°C shaker for 24 hours. After incubation, the cells were harvested by centrifuging the bacteria at 8000 rpm for 10 minutes. The cells were resuspended in 5ml of TEG (Tris 25mM pH 8.0, EDTA 10mM pH 8.0, Glucose 50 mM) solution and mixed gently. 500µ of lysozyme (10mg/ml) was added to this suspension and incubated overnight at 37°C a shaker incubator. Later, 1 ml of 10% SDS and 500µl of Proteinase K (20mg/ml) were added to the cell lysate and incubated at 55°C for 40 minutes. Subsequently, a solution comprising 2ml of 5M NaCl and 1.6ml of pre-warmed 10% CTAB was added to the cell lysate which was later incubated at 65°C for 10 minutes. The suspension was then centrifuged at 12000 rpm for 30 minutes at room temperature. The supernatant was subjected to phenol-chloroform extraction twice, and genomic DNA was precipitated by the addition of isopropanol. The DNA pellet was washed twice with 70% ethanol, air dried, and resuspended in autoclaved distilled water and stored at -20°C. The genomic DNA was used as a template for PCR experiments. Genomic DNA from Mycobacterium bovis BCG, M. tuberculosis H37Ra, and M. fortuitum were extracted by the above method. Genomic DNA extracted from Mycobacterium bovis irradiated whole cells (#NR-31210), and purified Genomic DNA of M. tuberculosis H37Rv strain (#NR-13648) procured from BEI resources, USA was used as PCR templates.
Animals
For this study, we selected cattle herds from two neighboring organized dairy farms in the Nadia district of West Bengal, India. Both farms follow similar feeding and management practices, and both raise a mixed population of Sahiwal and SHF crossbred cattle. We selected cattle (cows) that were over two years old, not pregnant during the study period, and had not undergone any tuberculin testing in the previous one year. Based on these criteria, a total of 50 cattle were included in the study, consisting of 24 Sahiwal and 26 SHF cattle.
DNA extraction from cow milk and urine
Genomic DNA was isolated from cow milk and urine samples as described previously (2). Briefly, milk samples were centrifuged at 12,000 x g for 20 minutes at room temperature. A sterile cotton swab was used to remove the fat layer, and the supernatant was discarded. The pellet was vortexed and subsequently resuspended in 500µl of IRS [Inhibitory Removing Solution with pH (7.4) containing 25M guanidium isothiocyanate, 0.025M EDTA, 0.05M Tris, 0.5% Sarkosyl and 0.186M β-mercaptoethanol]. Later the samples were incubated at 37°C for 60 minutes. After incubation, the samples were again centrifuged at 12,000 x g for 10 minutes and the supernatant was discarded. The pellet was washed with water once and resuspended in 100µl of lysis buffer (10% Chelex 100, 0.3% tween 20, 0.03% Triton X100). The samples were then incubated at 90°C for 40 minutes and subjected to another round of centrifugation at 10,000 x g for 10 minutes at room temperature. The supernatant was collected and used as template DNA for PCR reactions, or stored at -20°C for future use.
Single and multiplex- PCR assay for mycobacterial DNA detection
PCR assays were carried out targeting the genomic DNA of single mycobacterial species as well as multiple species in a single reaction. We observed higher sensitivity of single species/gene-specific PCR compared to the multiplex-PCR assay. Comparative genomics has revealed that based on the presence or absence of regions of difference (RD) mycobacterial species or strains can be differentiated. In this study, a combination of previously published primers targeting specific sequences of RD1, RD4, RD12, and rpoB gene was used to develop the single and multiplex- PCR to detect and differentiate M. tuberculosis, M. bovis, M. bovis BCG, M. orygis, pan-MTC and pan-NTM (including MACs) in the cattle samples (Supplementary Figure S1). Supplementary Table S1 shows the list of mycobacterial genomic region-specific primers used in this study. The PCR protocol was executed using the Sapphire PCR master mix (TAKARA) following manufacturer protocol. Subsequently, PCR amplicons were analyzed by agarose gel electrophoresis and visualized using a UV trans-illuminator. The expected size of amplicons with RD1, RD4, RD12-M. orygis, MTC, and NTM primers are 110bp, 176bp, 264bp, 235bp, and 134bp, respectively (Supplementary Figure S1). While the multiplex-PCR could detect mycobacteria in 0.1ng of template DNA, the single species PCR could detect mycobacteria in 0.01ng of total genomic DNA extracted from cattle milk or urine.
Single intradermal tuberculin test
Fifty cows from two adjacent herds were subjected to the single intradermal tuberculin test. The neck region of the animals was shaved and the thickness of the skin was measured with the use of a caliper before injecting bPPD. One hundred microliters (0.1ml) of bovine PPD (2,000 U/animal of bPPD) (obtained from Indian Veterinary Research Institute, Izatnagar, India; 1mg protein/ml) was injected into the skin of the cervical region. Seventy-two-hour post bPPD injection skin induration was evaluated by measuring the skin thickness with a caliper. The result was graded as bPPD positive reactor when differences in the skin thickness at the injection sites are at least 5 mm or greater.
In vitro bovine macrophage cell culture and mycobacterial growth assay
Bovine macrophage cell line- BOMAC was used for calibrating the infection dose (MOI), and evaluating the association of fluorescence measurements of the reporter mycobacterial strains with the number of bacteria over the period of infection (29, 30). BOMAC cells were maintained in DMEM supplemented with 10% heat-inactivated Fetal Bovine Serum (FBS). BOMAC cells were infected with the reporter strains of M. tuberculosis H37Ra-tdTomato, and M. bovis BCG-mCherry at different MOIs. The cells were infected for 3 hours, subsequently, the cells were washed thoroughly, and incubated in a TC incubator in fresh media. The fluorescence intensity was measured at λex/λem 554/581nm (M. tuberculosis H37Ra-pTEC27), and 587/610nm (M. bovis BCG-mCherry) respectively at an interval of 24 hours daily for 5 days using a fluorescence multimode plate reader (Biorad). An MOI of 1: 10 was considered for ex vivo mycobacterial growth in the bPBMC.
Ex vivo bovine PBMC culture and mycobacterial growth assay
Blood samples were collected from clinically healthy, SITT-negative, and myco-PCR-negative cattle. Five ml of blood was collected from the jugular vein of each animal, and bPBMC was isolated using Histopaque®-1077 (Sigma) following manufacturer recommendations. Briefly, blood was diluted with DPBS at a ratio of 1:1. The sample was layered slowly on top of the Ficoll density gradient buffer (5 ml diluted Blood on 3 ml Ficoll) and centrifuged at 400 x g for 30 minutes. The monocyte layer was carefully separated and washed twice with DPBS at 200 x g for 10 minutes. Contaminating RBCs were removed from the cell suspension by adding RBC lysis buffer (Sigma-Aldrich) and following manufacturer protocol. Purified bPBMC were then finally suspended in 5ml of DMEM complete media containing antibiotics, and added to an ultra-low attachment 6-well plate. The cells were incubated in a TC incubator for 24 hours. For mycobacterial growth assay, bPBMC were seeded on to 96-well TC plate at a density of 5X104 cells/well. The cells were infected with mid-log phase cultures of reporter mycobacteria (M. tuberculosis H37Ra-pTEC27 and M. bovis BCG-mCherry) at a pre-calibrated MOI of 1:10 and were incubated in a TC incubator. The fluorescence intensity was measured at λex/λem 554/581nm (M. tuberculosis H37Ra-pTEC27), and 587/610nm (M. bovis BCG-mCherry) respectively at an interval of 24 hours daily for 5 days using a fluorescence multimode plate reader.
Infection and antigenic stimulation of bovine PBMC for evaluation of innate immune responses
For the evaluation of innate immune responses, bPBMC were seeded onto 24-well TC plates at a density of 2X105 cells/well, and infected with either of the mycobacteria (M. tuberculosis H37Ra, or M. bovis BCG) at a pre-calibrated MOI of 1:10, or cells were stimulated with LPS (1µg/ml), PPD-B (300 IU/ml), PPD-A (250 IU/ml), M. tuberculosis H37Rv Whole Cell Lysate (WCL, 5 µg/ml), cell wall (CW, 5 µg/ml) and purified Lipoarabinomannan (LAM, 5µg/ml). Twenty-four hours post-infection cell culture supernatants were separated for measurement of IFN-γ protein levels, and total RNA was extracted from bPBMC for measurement of the mRNA transcripts levels of major cytokines, chemokines, and innate immune-signaling mediators.
RNA extraction, and real-time RT-PCR
Total RNA was extracted from bPBMC using RNeasy Plus Kit (Qiagen Inc, CA, USA) following manufacturer protocol. Contaminating genomic DNA was removed by additional treatment with RNase-free DNase (Qiagen Inc, CA, USA). The quality and quantity of RNA were analyzed using a NanoDrop Spectrophotometer (Thermo Scientific). cDNA was synthesized from RNA using the Prime script 1st-strand cDNA synthesis kit (Takara) as per the manufacturer’s instructions and using a mixture of random hexamer and oligo dT primers. Primers were designed for bovine gene targets (IFN-γ, IL-17, TNF-α, IFN-β, IL-1β, IL-6, IL-10, cGAS, STING, TBK1, IRF3, and IRF7) (Supplementary Table S4) using Primer-BLAST (NCBI) and real-time PCR was performed using a CFX96 Touch System (Biorad). Real-time PCR protocol started with an initial denaturation and enzyme activation at 95°C for 2 minutes followed by 40 cycles of denaturation at 95°C for 15 seconds, annealing and extension were carried out for 1 minute at a temperature ranging from 55°C to 65°C (based on the target gene). Melt curve analysis was performed by heating the samples from 65°C to 95°C with an increment of 0.5 and fluorescence was recorded. Relative gene expression of the target genes was calculated using the 2–ΔΔCT method with RPLP0 as an internal control.
Bovine cytokine enzyme-linked immunosorbent assay
Twenty-four hours post-infection cell culture supernatants were separated, filtered through 0.2 µ membrane plate filters, and subjected to ELISA for the measurement of IFN-γ protein levels using bovine IFN-γ specific sandwich ELISA kit as per the manufacturer protocol (K04-0002, Krishgen Biosystems). The absolute concentrations were estimated by referring to a standard curve and expressed as picogram per millilitre.
Nitric oxide measurement by Griess assay
The NO levels were determined using Griess reagent (# 35657, Sisco Research Laboratories Pvt. Ltd.) according to the method described previously (61). Briefly, the assay was performed in 96-well microtiter plate format using cell culture supernatants collected 24 hours post-infection and filtered through 0.2 µ membrane plate filters. 100μl of the culture supernatants were mixed with 100μl of Griess Reagent (0.1% naphthylethylene diamine dihydrochloric acid and 1% sulphanilamide in 5% phosphoric acid) and added to each well in technical duplicates. The samples were incubated at room temperature (25-30°C) for 10 minutes, and optical density was measured with a spectrophotometer at 546nm and the nitrite levels (as an indirect measure of NO) in the samples were quantified according to the standard graph for sodium nitrite.
Statistical analysis
GraphPad Prism 9 was used to perform the statistical analysis and preparation of the graphs. For comparison of group means Student’s t-tests were performed, and differences were considered significant when p<0.05. All the results are shown as the mean ± SEM unless otherwise described in the figure legends. For comparison of SITT, Myco-PCR, and combined tests data, SPSS vr.25 software was used for performing statistical significance tests.
Data availability statement
The original contributions presented in the study are included in the article/Supplementary Material. Further inquiries can be directed to the corresponding author.
Ethics statement
The animal study was approved by Animal Ethics Committee of the West Bengal University of Animal and Fishery Sciences, Kolkata, India (Approval No. IAEC/22 (B), CPCSEA Reg. No.763/GO/Re/SL/03/CPCSEA, Committee for the Purpose of Control and Supervision on Experiments on Animals, India). The study was conducted in accordance with the local legislation and institutional requirements.
Author contributions
Conceived and designed the experiments: BD. Performed the experiments: RK, SG, AR, HM, US, and BD. Analyzed the data: RK, SG, US, and BD. Contributed reagents/materials/analysis tools/facility: BD and US. Wrote the paper: RK, SG, US, and BD. Provided overall supervision throughout the study: BD. All authors contributed to the article and approved the submitted version.
Funding
Financial support from the NIAB intramural grant, and the Department of Biotechnology (DBT), Govt. of India (Grant No. BT/PR31378/AAQ/1/745/2019) are thankfully acknowledged. Support by DBT for providing Junior Research Fellowship to RK and AR; Department of Science and Technology (DST), Govt. of India for providing the Inspire fellowship (JRF) to SG.
Acknowledgments
Prof. Sharmistha Banerjee, University of Hyderabad, India is thankfully acknowledged for providing the M. tuberculosis H37Ra strain.
Conflict of interest
The authors declare that the research was conducted in the absence of any commercial or financial relationships that could be construed as a potential conflict of interest.
Publisher’s note
All claims expressed in this article are solely those of the authors and do not necessarily represent those of their affiliated organizations, or those of the publisher, the editors and the reviewers. Any product that may be evaluated in this article, or claim that may be made by its manufacturer, is not guaranteed or endorsed by the publisher.
Supplementary material
The Supplementary Material for this article can be found online at: https://www.frontiersin.org/articles/10.3389/fimmu.2023.1199092/full#supplementary-material
References
1. Murai K, Tizzani P, Awada L, Wall L, Mapitse NJ, Cceres P. Panorama 2019-1: Bovine tuberculosis: global distribution and implementation of prevention and control measures according to WAHIS data. In: Panorama 2019-1: Controlling bovine tuberculosis: a One Health challenge. (2019) OIE Bulletin. p1:3. doi: 10.20506/bull.2019.1.2912
2. Mishra A, Singhal A, Chauhan DS, Katoch VM, Srivastava K, Thakral SS, et al. Direct detection and identification of Mycobacterium tuberculosis and Mycobacterium bovis in bovine samples by a novel nested PCR assay: correlation with conventional techniques. J Clin Microbiol (2005) 43(11):5670–8. doi: 10.1128/JCM.43.11.5670-5678.2005
3. Prasad HK, Singhal A, Mishra A, Shah NP, Katoch VM, Thakral SS, et al. Bovine tuberculosis in India: potential basis for zoonosis. Tuberculosis (Edinb) (2005) 85(5-6):421–8. doi: 10.1016/j.tube.2005.08.005
4. Muller B, Durr S, Alonso S, Hattendorf J, Laisse CJ, Parsons SD, et al. Zoonotic Mycobacterium bovis-induced tuberculosis in humans. Emerg Infect Dis (2013) 19(6):899–908. doi: 10.3201/eid1906.120543
5. Annual Report: 2020-2021, Department of Agriculture, Cooperation & Farmers’ Welfare, Ministry of Agriculture & Farmers Welfare, Government of INDIA. New Delhi: Government of India (2021). p. 295.
6. Ramanujam H, Palaniyandi K. Bovine tuberculosis in India: The need for One Health approach and the way forward. One Health (2023) 16:100495. doi: 10.1016/j.onehlt.2023.100495
7. Refaya AK, Bhargavi G, Mathew NC, Rajendran A, Krishnamoorthy R, Swaminathan S, et al. A review on bovine tuberculosis in India. Tuberculosis (Edinb) (2020) 122:101923. doi: 10.1016/j.tube.2020.101923
8. Srinivasan S, Easterling L, Rimal B, Niu XM, Conlan AJK, Dudas P, et al. Prevalence of Bovine Tuberculosis in India: A systematic review and meta-analysis. Transbound Emerg Dis (2018) 65(6):1627–40. doi: 10.1111/tbed.12915
9. Allen AR, Minozzi G, Glass EJ, Skuce RA, McDowell SW, Woolliams JA, et al. Bovine tuberculosis: the genetic basis of host susceptibility. Proc Biol Sci (2010) 277(1695):2737–45. doi: 10.1098/rspb.2010.0830
10. Ameni G, Aseffa A, Engers H, Young D, Gordon S, Hewinson G, et al. High prevalence and increased severity of pathology of bovine tuberculosis in Holsteins compared to zebu breeds under field cattle husbandry in central Ethiopia. Clin Vaccine Immunol (2007) 14(10):1356–61. doi: 10.1128/CVI.00205-07
11. Firdessa R, Tschopp R, Wubete A, Sombo M, Hailu E, Erenso G, et al. High prevalence of bovine tuberculosis in dairy cattle in central Ethiopia: implications for the dairy industry and public health. PloS One (2012) 7(12):e52851. doi: 10.1371/journal.pone.0052851
12. Vordermeier M, Ameni G, Berg S, Bishop R, Robertson BD, Aseffa A, et al. The influence of cattle breed on susceptibility to bovine tuberculosis in Ethiopia. Comp Immunol Microbiol Infect Dis (2012) 35(3):227–32. doi: 10.1016/j.cimid.2012.01.003
13. Driscoll EE, Hoffman JI, Green LE, Medley GF, Amos W. A preliminary study of genetic factors that influence susceptibility to bovine tuberculosis in the British cattle herd. PloS One (2011) 6(4):e18806. doi: 10.1371/journal.pone.0018806
14. Brotherstone S, White IM, Coffey M, Downs SH, Mitchell AP, Clifton-Hadley RS, et al. Evidence of genetic resistance of cattle to infection with Mycobacterium bovis. J Dairy Sci (2010) 93(3):1234–42. doi: 10.3168/jds.2009-2609
15. Islam SKS, Rumi TB, Kabir SML, van der Zanden AGM, Kapur V, Rahman A, et al. Bovine tuberculosis prevalence and risk factors in selected districts of Bangladesh. PloS One (2020) 15(11):e0241717. doi: 10.1371/journal.pone.0241717
16. Humblet MF, Boschiroli ML, Saegerman C. Classification of worldwide bovine tuberculosis risk factors in cattle: a stratified approach. Vet Res (2009) 40(5):50. doi: 10.1051/vetres/2009033
17. Das RD, Dandapat P, Chakrabarty A, Nanda PK, Bandyopadhyay S, Bandyopadhyay S. A cross-sectional study on prevalence of bovine tuberculosis in Indian and crossbred cattle in Gangetic delta region of West Bengal, India. Int J One Health (2018) 4:7. doi: 10.14202/IJOH.2018.1-7
18. Dey B, Bishai WR. Crosstalk between Mycobacterium tuberculosis and the host cell. Semin Immunol (2014) 26(6):486–96. doi: 10.1016/j.smim.2014.09.002
19. Kramnik I, Dietrich WF, Demant P, Bloom BR. Genetic control of resistance to experimental infection with virulent Mycobacterium tuberculosis. Proc Natl Acad Sci USA (2000) 97(15):8560–5. doi: 10.1073/pnas.150227197
20. North RJ, LaCourse R, Ryan L, Gros P. Consequence of Nramp1 deletion to Mycobacterium tuberculosis infection in mice. Infect Immun (1999) 67(11):5811–4. doi: 10.1128/IAI.67.11.5811-5814.1999
21. Aravindan PP. Host genetics and tuberculosis: Theory of genetic polymorphism and tuberculosis. Lung India (2019) 36(3):244–52. doi: 10.4103/lungindia.lungindia_146_15
22. Moller M, Kinnear CJ, Orlova M, Kroon EE, van Helden PD, Schurr E, et al. Genetic resistance to Mycobacterium tuberculosis infection and disease. Front Immunol (2018) 9:2219. doi: 10.3389/fimmu.2018.02219
23. Naranbhai V. The role of host genetics (and genomics) in tuberculosis. Microbiol Spectr (2016) 4(5):p1–36. doi: 10.1128/microbiolspec.TBTB2-0011-2016
24. Kim BJ, Hong SK, Lee KH, Yun YJ, Kim EC, Park YG, et al. Differential identification of Mycobacterium tuberculosis complex and nontuberculous mycobacteria by duplex PCR assay using the RNA polymerase gene (rpoB). J Clin Microbiol (2004) 42(3):1308–12. doi: 10.1128/JCM.42.3.1308-1312.2004
25. Duffy SC, Srinivasan S, Schilling MA, Stuber T, Danchuk SN, Michael JS, et al. Reconsidering Mycobacterium bovis as a proxy for zoonotic tuberculosis: a molecular epidemiological surveillance study. Lancet Microbe (2020) 1(2):e66–73. doi: 10.1016/S2666-5247(20)30038-0
26. Taylor GM, Worth DR, Palmer S, Jahans K, Hewinson RG. Rapid detection of Mycobacterium bovis DNA in cattle lymph nodes with visible lesions using PCR. BMC Vet Res (2007) 3:12. doi: 10.1186/1746-6148-3-12
27. Halse TA, Escuyer VE, Musser KA. Evaluation of a single-tube multiplex real-time PCR for differentiation of members of the Mycobacterium tuberculosis complex in clinical specimens. J Clin Microbiol (2011) 49(7):2562–7. doi: 10.1128/JCM.00467-11
28. Takaki K, Davis JM, Winglee K, Ramakrishnan L. Evaluation of the pathogenesis and treatment of Mycobacterium marinum infection in zebrafish. Nat Protoc (2013) 8(6):1114–24. doi: 10.1038/nprot.2013.068
29. Donofrio G, van Santen VL. A bovine macrophage cell line supports bovine herpesvirus-4 persistent infection. J Gen Virol (2001) 82(Pt 5):1181–5. doi: 10.1099/0022-1317-82-5-1181
30. Stabel JR, Stabel TJ. Immortalization and characterization of bovine peritoneal macrophages transfected with SV40 plasmid DNA. Vet Immunol Immunopathol (1995) 45(3-4):211–20. doi: 10.1016/0165-2427(94)05348-V
31. Cooper AM, Khader SA. The role of cytokines in the initiation, expansion, and control of cellular immunity to tuberculosis. Immunol Rev (2008) 226:191–204. doi: 10.1111/j.1600-065X.2008.00702.x
32. Khan TA, Mazhar H, Saleha S, Tipu HN, Muhammad N, Abbas MN. Interferon-Gamma Improves Macrophages Function against M. tuberculosis in Multidrug-Resistant Tuberculosis Patients. Chemother Res Pract (2016) 2016:7295390. doi: 10.1155/2016/7295390
33. Lalvani A, Millington KA. T cells and tuberculosis: beyond interferon-gamma. J Infect Dis (2008) 197(7):941–3. doi: 10.1086/529049
34. Flynn JL, Chan J, Triebold KJ, Dalton DK, Stewart TA, Bloom BR. An essential role for interferon gamma in resistance to Mycobacterium tuberculosis infection. J Exp Med (1993) 178(6):2249–54. doi: 10.1084/jem.178.6.2249
35. Khader SA, Bell GK, Pearl JE, Fountain JJ, Rangel-Moreno J, Cilley GE, et al. IL-23 and IL-17 in the establishment of protective pulmonary CD4+ T cell responses after vaccination and during Mycobacterium tuberculosis challenge. Nat Immunol (2007) 8(4):369–77. doi: 10.1038/ni1449
36. Happel KI, Dubin PJ, Zheng M, Ghilardi N, Lockhart C, Quinton LJ, et al. Divergent roles of IL-23 and IL-12 in host defense against Klebsiella pneumoniae. J Exp Med (2005) 202(6):761–9. doi: 10.1084/jem.20050193
37. Cavalcanti YV, Brelaz MC, Neves JK, Ferraz JC, Pereira VR. Role of TNF-alpha, IFN-gamma, and IL-10 in the development of pulmonary tuberculosis. Pulm Med (2012) 2012:745483. doi: 10.1155/2012/745483
38. MacMicking JD, North RJ, LaCourse R, Mudgett JS, Shah SK, Nathan CF. Identification of nitric oxide synthase as a protective locus against tuberculosis. Proc Natl Acad Sci USA (1997) 94(10):5243–8. doi: 10.1073/pnas.94.10.5243
39. Nathan C, Shiloh MU. Reactive oxygen and nitrogen intermediates in the relationship between mammalian hosts and microbial pathogens. Proc Natl Acad Sci USA (2000) 97(16):8841–8. doi: 10.1073/pnas.97.16.8841
40. Thakur AS, Mandeep S, Katoch V, Dhar P, Katoch RC. A study on the prevalence of Bovine Tuberculosis in farmed dairy cattle in Himachal Pradesh. Vet World (2010) 3(9):6. doi: 10.5455/vetworld.2010.408-413
41. Singh CV. Cross-breeding in cattle for milk production: achievements, challenges and opportunities in India-A review. Adv Dairy Res (2016) 4(3):14. doi: 10.4172/2329-888X.1000158
42. Dhillon JS, Jain AK. Comparison of Sahiwal and different grades of Holstein Friesian × Sahiwal crossbreds for efficiency of milk production. Indian J Dairy Sci (India) (1977) 30(3):4.
43. Ministry of Fisheries, A.H.D., Govt. of India, 20th Livestock Census. All Q25 INDIA Report. Ministry of Fisheries, Animal Husbandry & Dairying, Govt. of India, New Delhi. p. 1–55.
44. Almaw G, Conlan AJK, Ameni G, Gumi B, Alemu A, Guta S, et al. The variable prevalence of bovine tuberculosis among dairy herds in Central Ethiopia provides opportunities for targeted intervention. PloS One (2021) 16(7):e0254091. doi: 10.1371/journal.pone.0254091
45. Ambaw M, Gelalcha BD, Bayissa B, Worku A, Yohannis A, Zewude A, et al. Pathology of bovine tuberculosis in three breeds of dairy cattle and spoligotyping of the causative mycobacteria in Ethiopia. Front Vet Sci (2021) 8:715598. doi: 10.3389/fvets.2021.715598
46. Koul A, Herget T, Klebl B, Ullrich A. Interplay between mycobacteria and host signalling pathways. Nat Rev Microbiol (2004) 2(3):189–202. doi: 10.1038/nrmicro840
47. Purdy GE, Russell DG. Lysosomal ubiquitin and the demise of Mycobacterium tuberculosis. Cell Microbiol (2007) 9(12):2768–74. doi: 10.1111/j.1462-5822.2007.01039.x
48. Gutierrez MG, Master SS, Singh SB, Taylor GA, Colombo MI, Deretic V. Autophagy is a defense mechanism inhibiting BCG and Mycobacterium tuberculosis survival in infected macrophages. Cell (2004) 119(6):753–66. doi: 10.1016/j.cell.2004.11.038
49. Aujla SJ, Dubin PJ, Kolls JK. Th17 cells and mucosal host defense. Semin Immunol (2007) 19(6):377–82. doi: 10.1016/j.smim.2007.10.009
50. van Beelen AJ, Zelinkova Z, Taanman-Kueter EW, Muller FJ, Hommes DW, Zaat SA, et al. Stimulation of the intracellular bacterial sensor NOD2 programs dendritic cells to promote interleukin-17 production in human memory T cells. Immunity (2007) 27(4):660–9. doi: 10.1016/j.immuni.2007.08.013
51. Gerosa F, Baldani-Guerra B, Lyakh LA, Batoni G, Esin S, Winkler-Pickett RT, et al. Differential regulation of interleukin 12 and interleukin 23 production in human dendritic cells. J Exp Med (2008) 205(6):1447–61. doi: 10.1084/jem.20071450
52. Ling WL, Wang LJ, Pong JC, Lau AS, Li JC. A role for interleukin-17A in modulating intracellular survival of Mycobacterium bovis bacillus Calmette-Guerin in murine macrophages. Immunology (2013) 140(3):323–34. doi: 10.1111/imm.12140
53. Pilli M, Arko-Mensah J, Ponpuak M, Roberts E, Master S, Mandell MA. TBK-1 promotes autophagy-mediated antimicrobial defense by controlling autophagosome maturation. Immunity (2012) 37(2):223–34. doi: 10.1016/j.immuni.2012.04.015
54. Decout A, Katz JD, Venkatraman S, Ablasser A. The cGAS-STING pathway as a therapeutic target in inflammatory diseases. Nat Rev Immunol (2021) 21(9):548–69. doi: 10.1038/s41577-021-00524-z
55. Guo B, Cheng G. Modulation of the interferon antiviral response by the TBK1/IKKi adaptor protein TANK. J Biol Chem (2007) 282(16):11817–26. doi: 10.1074/jbc.M700017200
56. Dey B, Dey RJ, Cheung LS, Pokkali S, Guo H, Lee JH, et al. A bacterial cyclic dinucleotide activates the cytosolic surveillance pathway and mediates innate resistance to tuberculosis. Nat Med (2015) 21(4):401–6. doi: 10.1038/nm.3813
57. Wassermann R, Gulen MF, Sala C, Perin SG, Lou Y, Rybniker J, et al. Mycobacterium tuberculosis Differentially Activates cGAS- and Inflammasome-Dependent Intracellular Immune Responses through ESX-1. Cell Host Microbe (2015) 17(6):799–810. doi: 10.1016/j.chom.2015.05.003
58. Watson RO, Bell SL, MacDuff DA, Kimmey JM, Diner EJ, Olivas J, et al. The Cytosolic Sensor cGAS Detects Mycobacterium tuberculosis DNA to Induce Type I Interferons and Activate Autophagy. Cell Host Microbe (2015) 17(6):811–9. doi: 10.1016/j.chom.2015.05.004
59. Jain R, Dey B, Dhar N, Rao V, Singh R, Gupta UD, et al. Enhanced and enduring protection against tuberculosis by recombinant BCG-Ag85C and its association with modulation of cytokine profile in lung. PloS One (2008) 3(12):e3869. doi: 10.1371/journal.pone.0003869
60. De Almeida IN, Da Silva Carvalho W, Rossetti ML, Costa ER, De Miranda SS. Evaluation of six different DNA extraction methods for detection of Mycobacterium tuberculosis by means of PCR-IS6110: preliminary study. BMC Res Notes (2013) 6:561. doi: 10.1186/1756-0500-6-561
Keywords: tuberculosis, bovine tuberculosis, Mycobacterium tuberculosis, Mycobacterium bovis, BCG
Citation: Kumar R, Gandham S, Rana A, Maity HK, Sarkar U and Dey B (2023) Divergent proinflammatory immune responses associated with the differential susceptibility of cattle breeds to tuberculosis. Front. Immunol. 14:1199092. doi: 10.3389/fimmu.2023.1199092
Received: 02 April 2023; Accepted: 29 August 2023;
Published: 19 September 2023.
Edited by:
Zhidong Hu, Fudan University, ChinaReviewed by:
Suraj P. Parihar, University of Cape Town, South AfricaAmandine Hauer, University of Gdansk, Poland
Copyright © 2023 Kumar, Gandham, Rana, Maity, Sarkar and Dey. This is an open-access article distributed under the terms of the Creative Commons Attribution License (CC BY). The use, distribution or reproduction in other forums is permitted, provided the original author(s) and the copyright owner(s) are credited and that the original publication in this journal is cited, in accordance with accepted academic practice. No use, distribution or reproduction is permitted which does not comply with these terms.
*Correspondence: Bappaditya Dey, YmRleUBuaWFiLm9yZy5pbg==