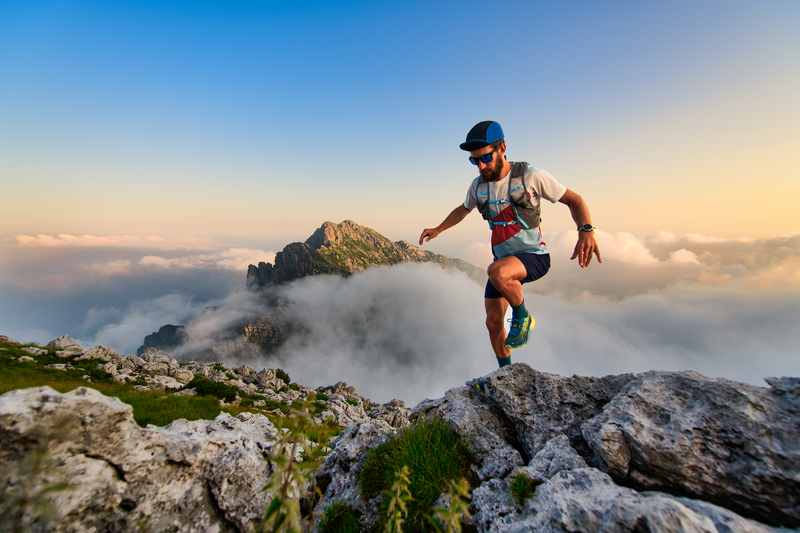
94% of researchers rate our articles as excellent or good
Learn more about the work of our research integrity team to safeguard the quality of each article we publish.
Find out more
REVIEW article
Front. Immunol. , 13 July 2023
Sec. Cancer Immunity and Immunotherapy
Volume 14 - 2023 | https://doi.org/10.3389/fimmu.2023.1196970
This article is part of the Research Topic Monoclonal Antibodies and Immune Checkpoint Inhibitors in the treatment of Cancer View all 28 articles
The PD-1/PD-L1 signaling pathway plays a crucial role in cancer immune evasion, and the use of anti-PD-1/PD-L1 antibodies represents a significant milestone in cancer immunotherapy. However, the low response rate observed in unselected patients and the development of therapeutic resistance remain major obstacles to their clinical application. Accumulating studies showed that overexpressed TGF-β is another immunosuppressive factor apart from traditional immune checkpoints. Actually, the effects of PD-1 and TGF-β pathways are independent and interactive, which work together contributing to the immune evasion of cancer cell. It has been verified that blocking TGF-β and PD-L1 simultaneously could enhance the efficacy of PD-L1 monoclonal antibody and overcome its treatment resistance. Based on the bispecific antibody or fusion protein technology, multiple bispecific and bifunctional antibodies have been developed. In the preclinical and clinical studies, these updated antibodies exhibited potent anti-tumor activity, superior to anti-PD-1/PD-L1 monotherapies. In the review, we summarized the advances of bispecific antibodies targeting TGF-β and PD-L1 in cancer immunotherapy. We believe these next-generation immune checkpoint inhibitors would substantially alter the cancer treatment paradigm, especially in anti-PD-1/PD-L1-resistant patients.
Programmed cell death 1 (PD-1) is a crucial signaling pathway that inhibits the immune response and helps maintain immune homeostasis (1). However, when overactivated in the tumor microenvironment, this pathway hinders host immune surveillance and clearance of tumor cells (2). Monoclonal antibodies targeting PD-1 or its ligand PD-L1 can restore the activity of exhausted immune cells and enhance the killing effect on tumor cells by blocking this immunosuppressive signaling (3–5). While anti-PD-1/PD-L1 monoclonal antibodies have been clinically approved for treating multiple malignancies and have exhibited promising anti-tumor effects in some patients, the low objective response rate of patients remains a challenge (6). In fact, the cancer-immunity cycle model suggests that in addition to the highly activated PD-1/PD-L1 pathway, multiple factors may become rate-limiting steps that restrict the anti-tumor immune response. Several studies have demonstrated that the activity of the TGF-β pathway in immunotherapy-resistant tumor is significantly increased (7, 8). The highly expressed TGF-β in the tumor microenvironment is also involved in cancer immune escape (9). The immunosuppressive mechanisms of TGF-β and PD-1 pathways are independent and complementary to each other, jointly promoting tumors to escape from host immune surveillance (10).
Highly expressed TGF-β in tumor tissues is primarily secreted by tumor cells and stromal cells. Highly expressed TGF-β not only promotes the epithelial-mesenchymal transition of tumor cells, but also regulates multiple tumor-infiltrating immune cells, leading to the formation of the immunosuppressive tumor microenvironment (11–14). On the one hand, TGF-β suppresses the functions of CD8+ T cells and natural killer cells (NK), and on the other hand, upregulates the numbers of regulatory T cells (Treg), M2-like macrophage, and myeloid-derived suppressor cells (MDSCs) (15–18). In addition, it has been confirmed that the high TGF-β tumor microenvironment can improve the activity of tumor-associated fibroblasts (CAFs) and promote the generation of collagen fibers in tumor stroma. The thickened collagen fibers around the tumor tissue are not conducive to immune cell infiltration, eventually forming the immune-excluded tumor type (7). Commonly, this type of tumor does not respond to anti-PD-1/PD-L1 monoclonal antibodies, while blocking TGF-β signaling can significantly reverse the therapeutic resistance of PD-1/PD-L1 blockade therapy and enhance anti-tumor immunotherapy effects (19–22). Theoretically, agents simultaneously blocking TGF-β and PD-1/PD-L1 pathways might have superior anti-tumor activity, relative to anti-PD-1/PD-L1 monoclonal antibodies.
Currently, Merck reported a bifunctional antibody called M7824 that simultaneously blocks PD-L1 and TGF-β (23). M7824 combines PD-L1 antibody with a trap structure targeting TGF-β, acting as a neutralizing receptor for TGF-β. Phase I clinical data indicate that the side effects of M7824 treatment are manageable and therapeutic effects have been observed in multiple types of cancers (24). Later, more bispecific antibodies (BsAbs) such as YM101 and BiTP are developed, which also exhibit potent anti-tumor activities in preclinical and clinical studies (25, 26). BsAbs targeting both PD-1/PD-L1 and TGF-β represent a significant breakthrough and an upgrade to current PD-1/PD-L1 monoclonal antibodies. By synergistically blocking both PD-1/PD-L1 and TGF-β inhibitory signals, these antibodies can effectively promote the transformation from immune-excluded tumors into immune-inflamed tumors. This can improve the efficacy of current PD-1/PD-L1 monoclonal antibodies and broaden their anti-tumor effects spectrum. In this review, we provide a summary of the recent advances in anti-TGF-β/PD-L1 BsAb development. Additionally, we discuss both the advantages and disadvantages of this next-generation immune checkpoint inhibitor.
PD-1 is a pivotal immune regulation signal molecule distributed in a wide breadth of immune cells, including DC, T cells, B cells and natural killer cells (NK), and activated monocytes or macrophages (27). PD-1, together with its ligands, PD-L1 and PD-L2, was found to take immunosuppressive effects in the antiviral inflammation and the tumor microenvironment (28, 29). Contemporarily, monoclonal antibodies targeting PD-1/PD-L1 have been widely utilized in clinical settings and exhibited remarkable therapeutic efficacy against various malignancies, particularly advanced and refractory tumors.
PD-1 was initially discovered to play a role in immune suppression of inflammation by serving as a negative feedback regulator. The two ligands, PD-L1 and PD-L2, activate and transmit inhibitory signals to target cells. Relative to PD-L2, PD-L1 is more widely distributed, particularly on tumor cells (27). Recent research has shed light on the elaborated and intriguing expression patterns of PD-L1, which is now known to be present not only on cell membrane, but on various cellular compartments and secreted in extracellular vesicles (30). Specifically, PD-L1 has been found to localize endocellularly on endosomes, the Golgi apparatus’s membrane, and endocytic vesicles. PD-L1 has also been detected in extracellular vesicles, which are involved in intercellular communication and the exchange of biological material between cells. These findings offer fascinating new prospects and possibilities for the development of novel therapeutic strategies targeting PD-L1 in cancer therapy (31). The PD-1/PD-L1 pathway exerts immune regulatory effects via recognition of effector T cells in the inflammatory context, with persistently high expression on activated T cells. Cytokines across the extracellular interval of tissue cells induce and modulate the expression of PD-L1, blunting the activation of T cells, and consequently resulting in immune homeostasis that the immune system eliminates exogenous microbiota while attenuating damage on normal tissue cells simultaneously. The most important inductive cytokine is IFN-γ, mainly derived from Th1 cells (32). PD-1 is persistently expressed at a high level in the circumstance of ongoing inflammation. As a result, persistently high expression of PD-1 triggers T cell exhaustion or inactivation (33).
Mechanistically, the immune system relies on a complex network of interactions between different cells and molecules to mount an effective response against invading pathogens or endogenous abnormalities, including tumor cells. One crucial aspect of this system is the ability of T cells to recognize and respond to antigens presented by other cells. In this bioprocess, MHC II molecules on antigen presentation cells (APCs) or MHC I molecules on all the karyocytes present fragments of antigens on cell membrane, which can be recognized by the T cell receptor (TCR) (34). This interaction activates T cells and triggers a cascade of signaling events that lead to the proliferation and differentiation of T cell, as well as the secretion of cytokines and other effector immune molecules. However, to prevent excessive immune activation and tissue damage, the immune system could dampen or terminate immune responses. Simultaneously with TCR-induced cascade, PD-1 begins to be expressed on the activated T cells. PD-1 and PD-L1 are brought into close proximity to each other in the microscale spatial structure. Subsequently, Immunoreceptor Tyrosine-based Inhibitory Motif (ITIM) and Immunoreceptor Tyrosine-based Switch Motif (ITSM) domain of PD-1 receptor are phosphorylated (35). The phosphorylated domains recruit tyrosine phosphatases, SHP-2 and SHP-1, which are capable of impeding critical factors in TCR signaling (36–38).
Consequently, T cell activation and function are blunted, restraining the degree and duration of the immune response. In addition to signaling repression, PD-1 can also interfere with the recognition of tumor cells by directly dampening the trimeric interaction between the TCR, pMHC, and CD8 molecules (39). This further contributes to immune evasion and tolerance by tumor cells and highlights the importance of PD-1/PD-L1 blockade as a promising immunotherapy strategy for cancer treatment.
As of March 2023, 21 PD-1/PD-L1 blockade drugs are available worldwide (Figure 1) (40–48). Herein, Nivolumab and Pembrolizumab are the most widely used PD-1/PD-L1 blockers and have received approval for the most indications. Notably, Cadonilimab is the first approved anti-PD-1/CTLA-4 BsAb for cervical cancer (44). More drugs targeting these immune checkpoints are in development, and clinical trials are underway to explore new indications for existing drugs. New drugs need to be tested against drugs already on the market to show better efficacy or performance. However, primary or acquired treatment resistance of PD-1/PD-L1 blockades has become an interactive conundrum for both physicians and tumor patients (49). Combination therapy regimens containing PD-1/PD-L1 inhibitors and other agents might be promising strategies for overcoming treatment resistance (50–52).
Figure 1 The details of approved PD-1/PD-L1 blockades in the United States of America, European Union, People’s Republic of China, and Russian Federation. Triangle symbol indicates that the corresponding indication of the drug has been approved by the United States Food and Drug Administration (FDA); Stellated symbol indicates that the corresponding indication of the drug has been approved by the National Medical Products Administration (NMPA) of People’s Republic of China. Similarly, other symbols represent drug approvals by corresponding regulatory agencies in European Union and Russian Federation. The abscissa represents the time of the approval. The color of the icon represents the approved indication.
Although PD-1/PD-L1 blockers have achieved an unprecedented breakthrough in cancer therapy, there have been increasing concerns about their disadvantages and insufficiency in the clinic (53, 54). Besides the adverse events and hyper progression associated with PD-1/PD-L1 blockade therapies (55–57), there is a prevalent concern regarding the limited response rate among cancer patients (58). Commonly, the therapeutic effect of anti-PD-1/PD-L1 antibodies mainly depends on the PD-L1 expression status, such as tumor proportion score (TPS), combined positive score (CPS), and immune cell proportion score (IPS) (59). However, a universe evaluation criterion is absent (60). Moreover, recent investigations suggest that single PD-L1 status is not a reliable indicator of predicting the response of patients to PD-1/PD-L1 blockade (61). Nevertheless, for patients without sufficient histopathologic evidence, the response rate to PD-1/PD-L1 blockades is less than 17% (62). Other responding assessing systems intervene to mount the accuracy, which hinges on the genomic instability, which is defined by tumor mutational burden (TMB) and microsatellite instable (MSI) (63–65). However, there are more or less deficiencies with these systems (66). The low response is partially attributed to the discrepancies in immune cell infiltration phenotype. In theory, the tumor immune microenvironment is classified histopathological as three phenotypes, inflamed, immune-excluded, and immune-desert, respectively. They are inextricably intertwined with immune cytokines level, which includes IFN-γ and TGF-β, fatty acid metabolism, neuroendocrine features, and EMT phenotype (67). Only the first tumor immune type benefits from the immune checkpoint inhibitors (68), but the proportion is less than 50% (67). The immune-excluded type takes up 30 to 50 percent of colorectal and ovarian cancer (69, 70). Herein, TGF-β exerts essential effects for hindrance of immune surveillance and tumor elimination in the immune-excluded type (7). The exploitation of an immunotherapeutic strategy that combines PD-1/PD-L1 blockades and TGF-β inhibiting or trap medicine is a promising direction.
TGF-β superfamily consists of more than 40 members, mainly classified as four subtypes, the TGF-β subtype, the bone morphogenetic protein-growth differentiation factor (BMP-GDM), activin-inhibin-nodal and others, orchestrates in the biological processes of carcinoma initiation, progression, and immune elimination (71–77). TGF-β, the most classic subtype, is a highly conserved and distributed breadth of the organism in the mammal. Three isoforms, TGF-β1, β2, and β3, are highly conserved with 80% of the same amino sequence, despite being encoded by separate genes. However, they still exhibit slight discrepancies in structural and bio-functional aspects, which can be recapitulated that TGF-β1 is more tendentious to immune regulation (78). It is secreted into the extracellular matrix, initially exists in an inactive form of latent precursor, and readily exerts biological functions in the tumor microenvironment via autocrine and paracrine (79). TGF-β is transcribed into a polypeptide, which is then cleaved by the Furin proteinase into two subunits. These subunits are further reassembled into an inactivated form that is secreted out of the cells. The extracellular inactivated TGF-β exists as a large complex, with the dimeric regulatory subunit called the long latency-associated peptide (LAP) forming the peripheral compound. The initial segment of LAP is a short signal peptide called the arginyl-glycyl-aspartic acid site (RGD). The bioactive catalytic subunit is ensconced internally, noncovalently combined with and wrapped around by the dimeric LAPs (72). In most cases, the cage-like complex could anchor via a disulfide bond to a compound of extracellular matrix, namely latent TGFβ-binding protein (LTBP), for stabilization. Additionally, the complex could also bind to transmembrane milieu proteins, particularly glycoprotein A repetitions predominant (GARP) on Treg and negative regulator of reactive oxygen species (NRROS) on microglia or macrophage (80–82). In the extracellular space, physical and chemical perturbance or serine protease would cleave and separate the dimeric LAPs from the complex to release the bioactive subunit. But integrin complexes capable of transmitting force derived from cytoskeleton across the cytomembrane, play the most prominent activated executor role (83). β-αv integrin heterodimer, particularly, recognizes the RGD motif, further interdigitates with the LAP, and consequently, changes autologous conformation. The allosterism tightens and gradually tears the “sleeve” of the latent TGF-β off and exposes the internal bioavailable TGF-β (83). Nevertheless, a unique kind of integrin is able to transduce the signaling without tearing LAP off and releasing the internal bioactive TGF-β (84). The free activated TGF-β or integrin αvβ8-latent TGF-β complex is capable of attaching to their three isoforms of the specific receptor, namely TGFBR1, 2, and 3, in divergent degrees of affinity (85). TGFBR3 doesn’t possess kinase activity like the others but can bind with all types of TGF-β with a high affinity therein. Therefore, it was previously believed to sequester and hinder the redundant TGF-β signaling (86). Recent studies recover its crucial roles in cellular signaling transduction in TGF-β dependent or independent manners (87, 88).
Bioavailable TGF-β could bind with TGFBR2 on the plasm membrane of specific cells. TGFBR1, subsequently, is recruited by the signaling complex, and together companies into a transmembrane heterotetrameric signaling complex. The endo-domain of the allosteric complex is phosphorylated. Then, the signaling cascade is triggered off. There are two signaling processes after the TGF-β-TGFBR1/2 tetrameric complex phosphorylated (10).
The Smad2/3 are firstly recruited by the transmembrane signaling and ulteriorly phosphorylated. The phosphorylation induces the binding of Smad4. Then, phosphorylated-Smad2/3/4 (Phospho-Smad complex) is assembled and translocates into the nucleus. As a result, the ultimate signaling complex induces downstream alterations in gene expression (89).
The redundantly initial transmembrane signaling could cross the recognition of Smad2/3, and directly activate the downstream signaling, such as phosphoinositide‐3‐kinase‐serine/threonine kinase (PI3K-AKT) and mitogen‐activated protein kinase (MAPK). The PI3K-AKT and MAPK signaling ulteriorly cause respective downstream cascades, which regulate the physiologic and pathologic alterations (90).
The smoldering cancer-related inflammation (CRI) accompanied by the development of cancer, with multitudinous kinds of immune cells and immune regulatory cytokines and chemokines pervading across mesenchyme is the persistent intrinsic characteristic of the tumor microenvironment (91). TGF-β, as a pleiotropic cytokine, exerts nuanced, complicated, and even contradictory biological regulatory functions with the development of cancer. In general, TGF-β stimulates the proliferation, transformation, and motility of mesenchyme-originated cells, while inhibiting proliferation and promoting differentiation of epithelium-originated cells and hemopoietic cells (92, 93). In the physiologic condition and early stage of cancer, TGF-β across mesenchyme delicately induces cell cycle arrest and inhibits cell proliferation through the canonical pathway. In the progression of malignancy, the loss of function mutations across the TGF-β pathway and rewiring TGF-β bioprocess make TGF-β a mutineer against tumor suppression signal network, to elicit tumor unconfinedly growing (94). The stimulation of TGF-β, the other cytokines, and chemokines in the tumor microenvironment transforms normal fibroblasts into CAF (95, 96). CAF can prompt tumor progression versatilely (97). In this condition, the tumor cells and cancer-associated fibroblasts excessively secret the amount of TGF-β to the extracellular matrix (97, 98). TGF-β also induces tumor cell EMT, a critical biologic process for migration and invasion, and biological features robustly associated with metastasis (99, 100). Besides, TGF-β can enhance angiogenesis which is beneficial to tumor growth and metastasis through either intracellular pathways or indirectly mediating EMT (101, 102). Apart from the aforementioned direct effects, TGF-β also assumes the paramount role in tumor immunity, indirectly influencing tumor cells throughout the tumor initiation and progression (Figure 2).
Figure 2 The mechanisms of TGF-β signaling and the immunosuppressive effects of TGF-β signaling on the immune response. Cells with yellow nuclei refer to immune cells and CAFs regulated by TGF-β signaling pathway. Cells with purple nuclei refer to TGF-β-producing cells (mainly secreted by fibroblast). TGF-β is a widely distributed signaling molecule involved in the regulation of almost all kinds of immune cells in the tumor microenvironment. On the one hand, TGF-β limits the functions of T cell, NK, and dendritic cell (DC). On the other hand, TGF-β promotes the differentiation towards Treg and M2-like macrophage. Besides, TGF-β enhances cancer-associated fibroblast (CAF) to generate collagen, which hampers immune cell infiltration. Adapted from Yi et al, 2022 (10).
TGF-β is a widely distributed signaling molecule involved in the regulation of almost all kinds of immune cells in the tumor microenvironment. Foxp3 positive Treg, particularly terminally differentiated effector Treg cells, plays a crucial role in tumor immune evasion, suppressing immune recognition and diminishment and ensuring tumor development and metastasis (103). With the development of genetic tracing and multiple-colors flow cytometer analysis, the lineage derivation of Treg has been demonstrated as dual origins, thymic Treg (tTreg) or natural Treg (nTreg), and peripheral Treg (pTreg) or alias-induced Treg (iTreg) (104–106). tTreg, which constitutes 80% of the total Treg repertoire, is derived from CD4-positive T cells that are induced by moderately robust co-stimulation of the TCR and a series of soluble cytokines, such as interleukin-2 (IL-2), IL-7, and IL-15, secreted by other autoreactive immunocytes in the thymus medulla. These cells express Foxp3 more stably and strongly, which is the major component of immune regulation (107–109). The pTreg, despite minor proportion, plays a comprehensive and cryptic role in local immune regulation and immune homeostasis in a Foxp3-independent way (16, 110). TGF-β plays nuanced, complicated, and pleiotropic roles in Tregs of both origins. In thymus medulla, it is highly enriched. The activated TGF-β participates in promoting the differentiation of Treg and the negative selection of neonatal T cells (108). Nevertheless, it is reported when TGF-β signaling is depleted, medullary thymic epithelial cells are stimulated, acting as “caregivers” of Treg cells and ultimately increasing the number of Treg cells (111). It suggests the multiplicity of TGF-β signaling on tTreg.
In the induction of pTreg, TGF-β accompanied with other immune regulatory signals, is capable of boosting Foxp3 expression on mature CD4 positive T cells in the peripheral. In the tumor microenvironment, excessive TGF-β from tumor cells and CAF not only suppresses the proliferation of other normal epithelial cells and conventional immune cells, but facilitates the transformation of pTreg (11). An aforesaid TGF-β anchored transmembrane milieu protein, GARP, is located on Treg, which is essential to extracellular latent TGF-β stabilization. Besides, the GARP on Treg could interact with integrin αvβ8, which also widely distributes on the Treg (112, 113). Both transmembrane proteins orchestrate more efficiently in the signaling transduction on Treg without breaking latent TGF-β off (113). Therefore, the increased pTreg in the local tumor context accelerates the transmission of TGF-β through the GARP pathway. To summarize, TGF-β prompts the activation of Treg of both origins, through whose pathway cancer cells trigger immune evasion and immunotherapy resistance.
The understanding of tumor-infiltrating B cells and their regulatory molecules remains vague. A regulatory type of B cells is identified as IgA positive and capable of inhibiting CD8+ T cell activation in colorectal tumors (114). Breg can also secret TGF-β, contributing to the apoptosis of effector T cells in the tumor microenvironment (115).
TGF-β can inhibit immunological surveillance and conventional effector immune cells in the tumor-developing stage. On the one hand, TGF-β directly dampens immunological surveillance by targeting cytotoxic lymphocytes (CTL) (15). On the other hand, it can prevent mature inflammation dendritic cells (DC) infiltration and induces the tolerogenic DC to indirectly deceive the surveillance (116, 117). Although tumor antigen bypasses or breaks through the first immunosuppression barrier to prime and activate the antigen immunity, TGF-β can suppress igniting CRI by decreasing total effector immune cells repertoire by reducing IL-2 secreted (118). Moreover, it precludes the transformation from naïve T cells to Th1/Th2 cells (119–121), but prompts the transformation to anti-inflammation Th17 cells (122, 123). In the natural immunity of tumor context, TGF-β polarizes tumor-associated macrophages to M2 (124), and reprogram tumor-associated neutrophil (125), both of which are detrimental to tumor immune elimination (126). Additionally, TGF-β inhibits the activation and functions of natural killer cells by suppressing the mTOR pathway (81, 127).
BsAb is a powerful tool for improving the limited sensitivity and effectiveness of traditional antibodies in immunotherapy (128, 129). BsAb can broaden the range of applications by targeting two different molecules and enhancing the anti-tumor effects (130–132). There are two primary types of BsAbs, depending on the presence of the Fc region. The first type is the Fc-containing BsAb, also known as the IgG-like molecule. This type of antibody exerts Fc-mediated effects, such as antibody-dependent cellular cytotoxicity or phagocytosis, and complement-dependent cytotoxicity (133, 134). IgG-like molecules also have a longer half-life than fragment-based molecules (135). Alternatively, the antigen-binding site can be directly connected by a peptide, which lacks the Fc region, known as the fragment-based molecule. This type of antibody has demonstrated flexibility in targeting tumor cells. Additionally, fragment-based molecules have promising potential to develop multi-specific antibodies (136). BsAbs can be developed using one of three methods: genetic or protein engineering, chemical conjugation, and quadroma (137). With these manufacturing methods, a vast array of BsAbs has been developed and tested in clinical trials.
The targeted antigens of BsAb are diverse. To summarize, the main targets include EpCAM, CEA, PSMA, ErbB, GPC3, immune checkpoints like PD-1 and CTLA-4, DLL4, and VEGF (138). The therapeutic effect of BsAb mainly regulates tumor immune response, which can be divided into two parts: immune cell redirection and anti-tumor immunity enhancement. Physiologically, immune cells, especially CD8+ T cells, detect and kill potential tumor cells. During tumorigenesis, multiple dysfunctions of T cells result in cancer immune escape (139).
Some BsAbs have two types of binding antigens: a specific tumor-associated antigen (TAA) and an extracellular CD3 subunit located on the T cell surface. This kind of antibody is called T cell-engaging BsAbs (TCE). Thus, TAAs direct T cells to targeted tumor cells. TCE was first introduced in 1985 and has rapidly developed at the beginning of the 21st century (140). Catumaxomab and blinatumomab are representatives of TCE. The TAAs of the two antibodies are EpCAM and CD19 (141, 142). Additionally, other TAAs such as CD20, CEA, gpA33, EGFR variant III, PSMA, MUC-1, glypican-3, P-cadherin, B7-H3, and even intracellular antigens can be TAA of TCE (143–151). Another mechanism of BsAb is anti-tumor immunity enhancement. To achieve this effect, this kind of antibody mainly blocks immune checkpoints. The blockade of innate immune checkpoints like CD47 can disrupt the antiphagocytic signals expressed by tumor cells and enhance phagocytosis by macrophages (152–154). Immune checkpoints of T cell activity can also be blocked to enhance adaptive immunity. PD-1/PD-L1, CTLA-4, LAG3, and TIM3 are receptors of coinhibitory immune checkpoints. The blockade of these molecules resuscitates the function of tumor-infiltrating T cells in various kinds of cancers in 10%-30% of patients (155). Molecules in the TNF receptor superfamily and glucocorticoid-induced TNFR-related protein are receptors of costimulatory immune checkpoints. Using an agonist to activate the receptors can reverse the suppression of CTL and promote tumor cell death (156). The combination of blockade of coinhibitory molecules and activation of costimulatory molecules also achieves promising anti-tumor effects in vivo (40, 157, 158). The immune cell redirection and anti-tumor immunity enhancement can also be combined to achieve a robust anti-tumor effect in vivo (159).
However, there are some adverse effects of BsAb that cannot be eliminated at present. Since BsAb primarily regulates the immune response through signaling pathways and cytokines, the most common adverse effect is cytokine release syndrome (CRS) (160, 161). CRS is caused by a large number of cytokines, such as IFN-γ secreted by activated T cells (162, 163). They can suppress the overstimulated inflammatory reactions without significantly reducing the anti-tumor effect (147). Cytokine receptor antagonism can block the receptors of overexpressed cytokines and relieve the symptoms of CRS (164, 165). However, to prevent the potential immune suppressive effect of corticosteroids, small molecule inhibitors have been developed. This type of inhibitor specifically targets the signaling molecules involved in CRS induction. Since most cytokines promote inflammation via the JAK/STAT pathway in CRS, preclinical studies of JAK1/2 inhibitors have shown promising efficacy in preventing CRS (166). In addition, Bruton’s tyrosine kinase inhibitors (BTK) can directly bind to B cells to reduce the overexpression of cytokines caused by BTK signaling, preventing CRS without affecting anti-tumor efficacy (167).
Given the high expression levels and specific enrichment of both PD-L1 and TGF-β in the TME, reagents that target them simultaneously could provide more precise targeting of cancerous lesions while sparing normal tissues. As a result, bispecific antibodies (BsAbs) may accumulate in the TME, reducing side effects and improving tumoral precision therapy.
YM101 is the world’s first publicly reported anti-TGF-β/PD-L1 bsAb (25). Although fusion protein M7824 has been published before, it is the first time to target these two molecules by bsAb technology. YM101 is the first molecule developed based on the Check-BODY™ technology platform (Figure 3) (25). The results showed that YM101 could effectively antagonize the biological effects of TGF-β and PD-1/PD-L1 pathways. In addition, in vivo experiments showed that the anti-tumor activity of YM101 was superior to that of anti-TGF-β and anti-PD-L1 monotherapies. Investigations into the TME found that YM101 promoted the formation of inflamed tumor: increased the number and activity of TIL and DC, and increased the ratio of M1/M2 macrophages. Additionally, hyperactive TGF-β signaling in CAF leads to thickened peritumoral collagen, which hampers immune cell infiltration and limits the efficacy of anti-PD-L1. However, YM101 suppressed the functions of CAFs and undermined the peritumoral barrier by neutralizing TGF-β in the TME. As a result, YM101 promoted T cell infiltration and relieved anti-PD-L1 resistance (25). Moreover, the combination therapy of Mn2+ and YM101 has been shown to have a synergistic anti-tumor effect, effectively reversing immunotherapy resistance in non-inflamed tumors (168). It has been validated that that Mn2+ activates the STING pathway, promotes DC maturation, and cooperates with YM101 to promote T cell activation. Moreover, in multiple mouse tumor models, the combination of Mn2+ and YM101 treatment has exhibited durable anti-tumor effects and prolonged the survival of tumor-bearing mice (168). Compared to monotherapy, the combination of Mn2+ and YM101 has a stronger anti-tumor effect and a broader anti-tumor spectrum. Mechanistically, this strategy (168). Further single-cell transcriptome analysis demonstrated that STING agonist combined with YM101 simultaneously regulates multiple components of anti-tumor immunity, promoting the transition from immune-exclude or immune-desert to inflamed tumors. This novel combined approach has the potential to be a general treatment for both inflamed and non-inflamed tumors (74). Encouraged by the positive preclinical data, the alternative molecule BiTP was constructed for further clinical trials (26). With a similar structure to YM101, BiTP is created by Check-BODY™ as well. The results of murine triple-negative breast cancer models showed that BiTP decreased peritumoral collagen generation and promoted T cell infiltration (26). A phase I clinical trial (NCT05028556) is also on recruiting to explore the optimal dose, efficacy, and safety of BiTP. There has been no observation of serious immune-related adverse events in the trial.
Figure 3 The structure of bispecific and bifunctional antibodies targeting TGF-β and PD-L1. (A) The structure of YM101 and BiTP. YM101 and BiTP contain anti-TGF-β and anti-PD-L1 domains in Fab region. (B) The structure of M7824. M7824 contains an anti-PD-L1 domain in Fab region and a TGF-β trap in Fc region. Adapted from Yi et al, 2022 (40).
As a novel bifunctional fusion protein targeting TGF-β and PD-L1, M7824 contains an anti-PD-L1 domain in Fab region and a TGF-β trap in Fc region (23). In murine cancer models, M7824 showed potent anti-tumor efficacy and significantly prolonged the survival of tumor-bearing mice (23). Further investigations showed that M7824 substantially reshaped the tumor immune microenvironment: upregulating the numbers and activities of tumor-killing effectors and decreasing the ratio of immunosuppressive subsets such as MDSC, M2-like macrophage, and Treg (23). Also, M7824 led to tumor matrix remodeling, which might contribute to improved immune cell infiltration (23). Notably, preclinical data indicated that radiotherapy or chemotherapy might enhance the anti-tumor effect of M7824 (23, 169). The successful in-vivo studies and animal studies inspire researchers to conduct clinical trials associated with M7824 as listed in Table 1. In the phase 1 trial NCT02517398, the response rate was 87.5% in patients with PD-L1 high NSCLC (170). Up to now, M7824 has undergone 19 clinical trials, with 4 completed, 1 actively not recruiting, 12 terminated and 2 withdrawn, according to the ClinicalTrials database (Supplementary File: Table S1).
The triumph of M7824 has stimulated the exploration of novel fusion protein endeavors, among which is SHR-1701, a monoclonal anti-PD-L1 domain fused with an N-terminal-truncated domain of TGFβRII that bears a resemblance to M7824 in structure (171). The linked TGFβRII domain serves as a trap and neutralizes TGF-β in the tumor microenvironment, while the Fab segment of the antibody blocks PD-L1. This dual blockade overcomes anti-PD-1 resistance in murine tumor models (172). In advanced tumors, SHR-1701 showed anti-tumor activity with objective response rate (ORR) of over 20% (173). In recurrent and metastatic cervical cancer, the ORR of SHR-1701 reached 15.6% (174). Also, fusion protein BR102 contains anti-PD-L1 antibody and TGFβRII ectodomain (175). Further animal studies confirmed the anti-tumor activity of BR102 in murine tumor models (176).
For advanced cancers, TGF-β changes from a tumor suppressor to a tumor promoter. In cancer immunology, TGF-β substantially undermines immune surveillance and immune clearance by limiting the activities of antigen-presenting cells and cytotoxic T cells. Therefore, TGF-β blockade is a promising approach to improve immunotherapy performance. Although the enhanced anti-tumor effect of TGF-β and PD-L1 dual blockade has been validated in several clinical studies, the combination therapy of two antibodies indeed complicates grouping in clinical trials.
Based on BsAb or fusion protein technology, multiple BsAbs have been developed, which could simultaneously counteract PD-1 and TGF-β signaling pathways. Commonly, these BsAbs exhibit more potent anti-tumor activities and effectively reshape the immunosuppressive microenvironment. Notably, the therapeutic effect of anti-TGF-β/PD-L1 BsAb is even superior to anti-TGF-β plus anti-PD-L1 treatment, which might be attributed to the high tumor specificity brought by BsAb structure. We believe anti-TGF-β/PD-L1 BsAb has a significant advantage in treatment effect, especially in TGF-β-driven immune-excluded tumors.
However, in a head-to-head phase III clinical study with pembrolizumab, M7824 failed to achieve the expected endpoints in patients with non-small cell lung cancer and cholangiocarcinoma. Although the reasons for the large discrepancy between the results of the phase III trial and the phase I trial have not been published, the lack of precise molecular markers to select suitable patients may be one reason for the failure of the phase III trial. For immune-desert tumors, both TGF-β and PD-1 pathways are not primary rheostats for the cancer-immunity cycle. In this case, combination therapy with agents stimulating antigen release or improving antigen-presenting cell functions is essential to overcome immunotherapy resistance. It has been confirmed that anti-TGF-β/PD-L1 BsAb combined with STING agonist effectively conquers anti-PD-1/PD-L1 resistance in immune-desert and immune-exclude tumors. Hereto, anti-TGF-β/PD-L1 BsAb-involved combination therapy might effectively broaden the anti-tumor spectrum of immunotherapy in the future.
TL and XW performed the selection of literature, drafted the manuscript and prepared the figures. MN, MW, and JZ collected the related references and participated in discussion. MY and KW designed this review and revised the manuscript. All authors contributed to this manuscript. All authors read and approved the final manuscript.
This work was supported by the National Natural Science Foundation of China (Nos. 82073370 and 82272794) and China Postdoctoral Science Foundation (No. 2022M722766).
The authors declare that the research was conducted in the absence of any commercial or financial relationships that could be construed as a potential conflict of interest.
All claims expressed in this article are solely those of the authors and do not necessarily represent those of their affiliated organizations, or those of the publisher, the editors and the reviewers. Any product that may be evaluated in this article, or claim that may be made by its manufacturer, is not guaranteed or endorsed by the publisher.
The Supplementary Material for this article can be found online at: https://www.frontiersin.org/articles/10.3389/fimmu.2023.1196970/full#supplementary-material
APC, antigen presentation cell; BiTE, bispecific T-cell engager; BsAb, bispecific antibody; BTK, Bruton’s tyrosine kinase inhibitor; CAF, carcinoma-associated fibroblast; CRS, cytokine release syndrome; CRI, cancer-related inflammation; DC, dendritic cell; IL-2, interleukin-2; MAPK, mitogen‐activated protein kinase; MDSC, myeloid-derived suppressor cell; NK, natural killer; NSCLC, non-small cell lung cancer; ORR, objective response rate; PD-1, programmed cell death protein 1; PI3K-AKT, phosphoinositide‐3‐kinase‐serine/threonine kinase; scFv, single-chain fragment variable; TGF-β, transforming growth factor-beta; TGFβRI, TGF-β type I receptor; TGFβRII, TGF-β type II receptor; TME, tumor microenvironment; Treg, regulatory T cell; TandAb, Tandem diabody.
1. Zou W, Wolchok JD, Chen L. PD-L1 (B7-H1) and PD-1 pathway blockade for cancer therapy: mechanisms, response biomarkers, and combinations. Sci Transl Med (2016) 8(328):328rv324. doi: 10.1126/scitranslmed.aad7118
2. Dong H, Strome SE, Salomao DR, Tamura H, Hirano F, Flies DB, et al. Tumor-associated B7-H1 promotes T-cell apoptosis: a potential mechanism of immune evasion. Nat Med (2002) 8(8):793–800. doi: 10.1038/nm730
3. Zha H, Jiang Y, Wang X, Shang J, Wang N, Yu L, et al. Non-canonical PD-1 signaling in cancer and its potential implications in clinic. J Immunother Cancer (2021) 9(2):e001230. doi: 10.1136/jitc-2020-001230
4. Wang Y, Zhang H, Liu C, Wang Z, Wu W, Zhang N, et al. Immune checkpoint modulators in cancer immunotherapy: recent advances and emerging concepts. J Hematol Oncol (2022) 15(1):111. doi: 10.1186/s13045-022-01225-3
5. Marin-Acevedo JA, Kimbrough EO, Lou Y. Next generation of immune checkpoint inhibitors and beyond. J Hematol Oncol (2021) 14(1):45. doi: 10.1186/s13045-021-01056-8
6. Yi M, Jiao D, Xu H, Liu Q, Zhao W, Han X, et al. Biomarkers for predicting efficacy of PD-1/PD-L1 inhibitors. Mol Cancer (2018) 17(1):129. doi: 10.1186/s12943-018-0864-3
7. Mariathasan S, Turley SJ, Nickles D, Castiglioni A, Yuen K, Wang Y, et al. TGFβ attenuates tumour response to PD-L1 blockade by contributing to exclusion of T cells. Nature (2018) 554(7693):544–8. doi: 10.1038/nature25501
8. Tauriello DVF, Palomo-Ponce S, Stork D, Berenguer-Llergo A, Badia-Ramentol J, Iglesias M, et al. TGFβ drives immune evasion in genetically reconstituted colon cancer metastasis. Nature (2018) 554(7693):538–43. doi: 10.1038/nature25492
9. Bai X, Yi M, Jiao Y, Chu Q, Wu K. Blocking TGF-β signaling to enhance the efficacy of immune checkpoint inhibitor. Onco Targets Ther (2019) 12:9527–38. doi: 10.2147/OTT.S224013
10. Yi M, Li T, Niu M, Wu Y, Zhao Z, Wu K. TGF-β: a novel predictor and target for anti-PD-1/PD-L1 therapy. Front Immunol (2022) 13:1061394. doi: 10.3389/fimmu.2022.1061394
11. Batlle E, Massagué J. Transforming growth factor-β signaling in immunity and cancer. Immunity (2019) 50(4):924–40. doi: 10.1016/j.immuni.2019.03.024
12. Derynck R, Turley SJ, Akhurst RJ. TGFβ biology in cancer progression and immunotherapy. Nat Rev Clin Oncol (2021) 18(1):9–34. doi: 10.1038/s41571-020-0403-1
13. Larson C, Oronsky B, Carter CA, Oronsky A, Knox SJ, Sher D, et al. TGF-beta: a master immune regulator. Expert Opin Ther Targets (2020) 24(5):427–38. doi: 10.1080/14728222.2020.1744568
14. Chen J, Gingold JA, Su X. Immunomodulatory TGF-β signaling in hepatocellular carcinoma. Trends Mol Med (2019) 25(11):1010–23. doi: 10.1016/j.molmed.2019.06.007
15. Thomas DA, Massagué J. TGF-beta directly targets cytotoxic T cell functions during tumor evasion of immune surveillance. Cancer Cell (2005) 8(5):369–80. doi: 10.1016/j.ccr.2005.10.012
16. Kanamori M, Nakatsukasa H, Okada M, Lu Q, Yoshimura A. Induced regulatory T cells: their development, stability, and applications. Trends Immunol (2016) 37(11):803–11. doi: 10.1016/j.it.2016.08.012
17. Park BV, Freeman ZT, Ghasemzadeh A, Chattergoon MA, Rutebemberwa A, Steigner J, et al. TGFβ1-mediated SMAD3 enhances PD-1 expression on antigen-specific T cells in cancer. Cancer Discovery (2016) 6(12):1366–81. doi: 10.1158/2159-8290.CD-15-1347
18. Tauriello DVF, Sancho E, Batlle E. Overcoming TGFβ-mediated immune evasion in cancer. Nat Rev Cancer (2022) 22(1):25–44. doi: 10.1038/s41568-021-00413-6
19. Bagati A, Kumar S, Jiang P, Pyrdol J, Zou AE, Godicelj A, et al. Integrin αvβ6-TGFβ-SOX4 pathway drives immune evasion in triple-negative breast cancer. Cancer Cell (2021) 39(1):54–67.e59. doi: 10.1016/j.ccell.2020.12.001
20. Busenhart P, Montalban-Arques A, Katkeviciute E, Morsy Y, Van Passen C, Hering L, et al. Inhibition of integrin αvβ6 sparks T-cell antitumor response and enhances immune checkpoint blockade therapy in colorectal cancer. J Immunother Cancer (2022) 10(2):e003465. doi: 10.1136/jitc-2021-003465
21. Terabe M, Robertson FC, Clark K, De Ravin E, Bloom A, Venzon DJ, et al. Blockade of only TGF-β 1 and 2 is sufficient to enhance the efficacy of vaccine and PD-1 checkpoint blockade immunotherapy. Oncoimmunology (2017) 6(5):e1308616. doi: 10.1080/2162402X.2017.1308616
22. Larson C, Oronsky B, Reid T. AdAPT-001, an oncolytic adenovirus armed with a TGF-β trap, overcomes in vivo resistance to PD-L1-immunotherapy. Am J Cancer Res (2022) 12(7):3141–7.
23. Lan Y, Zhang D, Xu C, Hance KW, Marelli B, Qi J, et al. Enhanced preclinical antitumor activity of M7824, a bifunctional fusion protein simultaneously targeting PD-L1 and TGF-β. Sci Transl Med (2018) 10(424):eaan5488. doi: 10.1126/scitranslmed.aan5488
24. Strauss J, Heery CR, Schlom J, Madan RA, Cao L, Kang Z, et al. Phase I trial of M7824 (MSB0011359C), a bifunctional fusion protein targeting PD-L1 and TGFβ, in advanced solid tumors. Clin Cancer Res (2018) 24(6):1287–95. doi: 10.1158/1078-0432.CCR-17-2653
25. Yi M, Zhang J, Li A, Niu M, Yan Y, Jiao Y, et al. The construction, expression, and enhanced anti-tumor activity of YM101: a bispecific antibody simultaneously targeting TGF-β and PD-L1. J Hematol Oncol (2021) 14(1):27. doi: 10.1186/s13045-021-01045-x
26. Yi M, Wu Y, Niu M, Zhu S, Zhang J, Yan Y, et al. Anti-TGF-β/PD-L1 bispecific antibody promotes T cell infiltration and exhibits enhanced antitumor activity in triple-negative breast cancer. J Immunother Cancer (2022) 10(12):e005543. doi: 10.1136/jitc-2022-005543
27. Keir ME, Butte MJ, Freeman GJ, Sharpe AH. PD-1 and its ligands in tolerance and immunity. Annu Rev Immunol (2008) 26:677–704. doi: 10.1146/annurev.immunol.26.021607.090331
28. Zhang Y, Zhang Z. The history and advances in cancer immunotherapy: understanding the characteristics of tumor-infiltrating immune cells and their therapeutic implications. Cell Mol Immunol (2020) 17(8):807–21. doi: 10.1038/s41423-020-0488-6
29. Wang D, Fu B, Shen X, Guo C, Liu Y, Zhang J, et al. Restoration of HBV-specific CD8(+) T-cell responses by sequential low-dose IL-2 treatment in non-responder patients after IFN-α therapy. Signal Transduct Target Ther (2021) 6(1):376. doi: 10.1038/s41392-021-00776-0
30. Niu M, Liu Y, Yi M, Jiao D, Wu K. Biological characteristics and clinical significance of soluble PD-1/PD-L1 and exosomal PD-L1 in cancer. Front Immunol (2022) 13:827921. doi: 10.3389/fimmu.2022.827921
31. Zou W, Luo X, Gao M, Yu C, Wan X, Yu S, et al. Optimization of cancer immunotherapy on the basis of programmed death ligand-1 distribution and function. Br J Pharmacol (2023). doi: 10.1111/bph.16054
32. Alspach E, Lussier DM, Schreiber RD. Interferon γ and its important roles in promoting and inhibiting spontaneous and therapeutic cancer immunity. Cold Spring Harb Perspect Biol (2019) 11(3):a028480. doi: 10.1101/cshperspect.a028480
33. Sun C, Mezzadra R, Schumacher TN. Regulation and function of the PD-L1 checkpoint. Immunity (2018) 48(3):434–52. doi: 10.1016/j.immuni.2018.03.014
34. Jardim DL, Goodman A, de Melo Gagliato D, Kurzrock R. The challenges of tumor mutational burden as an immunotherapy biomarker. Cancer Cell (2021) 39(2):154–73. doi: 10.1016/j.ccell.2020.10.001
35. Hui E, Cheung J, Zhu J, Su X, Taylor MJ, Wallweber HA, et al. T Cell costimulatory receptor CD28 is a primary target for PD-1-mediated inhibition. Science (2017) 355(6332):1428–33. doi: 10.1126/science.aaf1292
36. Marasco M, Berteotti A, Weyershaeuser J, Thorausch N, Sikorska J, Krausze J, et al. Molecular mechanism of SHP2 activation by PD-1 stimulation. Sci Adv (2020) 6(5):eaay4458. doi: 10.1126/sciadv.aay4458
37. Peled M, Tocheva AS, Sandigursky S, Nayak S, Philips EA, Nichols KE, et al. Affinity purification mass spectrometry analysis of PD-1 uncovers SAP as a new checkpoint inhibitor. Proc Natl Acad Sci USA (2018) 115(3):E468–e477. doi: 10.1073/pnas.1710437115
38. Wang Q, Bardhan K, Boussiotis VA, Patsoukis N. The PD-1 interactome. Adv Biol (Weinh) (2021) 5(9):e2100758. doi: 10.1002/adbi.202100758
39. Li K, Yuan Z, Lyu J, Ahn E, Davis SJ, Ahmed R, et al. PD-1 suppresses TCR-CD8 cooperativity during T-cell antigen recognition. Nat Commun (2021) 12(1):2746. doi: 10.1038/s41467-021-22965-9
40. Yi M, Zheng X, Niu M, Zhu S, Ge H, Wu K. Combination strategies with PD-1/PD-L1 blockade: current advances and future directions. Mol Cancer (2022) 21(1):28. doi: 10.1186/s12943-021-01489-2
41. Dhillon S. Penpulimab: first approval. Drugs (2021) 81(18):2159–66. doi: 10.1007/s40265-021-01640-9
42. Paik J. Nivolumab plus relatlimab: first approval. Drugs (2022) 82(8):925–31. doi: 10.1007/s40265-022-01723-1
43. Lee A. Serplulimab: first approval. Drugs (2022) 82(10):1137–41. doi: 10.1007/s40265-022-01740-0
44. Keam SJ. Cadonilimab: first approval. Drugs (2022) 82(12):1333–9. doi: 10.1007/s40265-022-01761-9
45. Dhillon S. Pucotenlimab: first approval. Drugs (2022) 82(15):1557–64. doi: 10.1007/s40265-022-01787-z
46. Yan W, Zhong WZ, Liu YH, Chen Q, Xing W, Zhang Q, et al. Adebrelimab (SHR-1316) in combination with chemotherapy as perioperative treatment in patients with resectable stage II to III NSCLCs: an open-label, multicenter, phase 1b trial. J Thorac Oncol (2023) 18(2):194–203. doi: 10.1016/j.jtho.2022.09.222
47. Dhillon S, Duggan S. Sugemalimab: first approval. Drugs (2022) 82(5):593–9. doi: 10.1007/s40265-022-01693-4
48. Markham A. Envafolimab: first approval. Drugs (2022) 82(2):235–40. doi: 10.1007/s40265-022-01671-w
49. Kim JM, Chen DS. Immune escape to PD-L1/PD-1 blockade: seven steps to success (or failure). Ann Oncol (2016) 27(8):1492–504. doi: 10.1093/annonc/mdw217
50. Yi M, Jiao D, Qin S, Chu Q, Wu K, Li A. Synergistic effect of immune checkpoint blockade and anti-angiogenesis in cancer treatment. Mol Cancer (2019) 18(1):60. doi: 10.1186/s12943-019-0974-6
51. Li A, Yi M, Qin S, Song Y, Chu Q, Wu K. Activating cGAS-STING pathway for the optimal effect of cancer immunotherapy. J Hematol Oncol (2019) 12(1):35. doi: 10.1186/s13045-019-0721-x
52. Yi M, Yu S, Qin S, Liu Q, Xu H, Zhao W, et al. Gut microbiome modulates efficacy of immune checkpoint inhibitors. J Hematol Oncol (2018) 11(1):47. doi: 10.1186/s13045-018-0592-6
53. Shergold AL, Millar R, Nibbs RJB. Understanding and overcoming the resistance of cancer to PD-1/PD-L1 blockade. Pharmacol Res (2019) 145:104258. doi: 10.1016/j.phrs.2019.104258
54. Ramos-Casals M, Brahmer JR, Callahan MK, Flores-Chávez A, Keegan N, Khamashta MA, et al. Immune-related adverse events of checkpoint inhibitors. Nat Rev Dis Primers (2020) 6(1):38. doi: 10.1038/s41572-020-0160-6
55. Champiat S, Marabelle A. Paradoxical cancer cell stimulation by IFNγ drives tumor hyperprogression upon checkpoint blockade immunotherapy. Cancer Cell (2023) 41(2):229–31. doi: 10.1016/j.ccell.2023.01.006
56. Li G, Choi JE, Kryczek I, Sun Y, Liao P, Li S, et al. Intersection of immune and oncometabolic pathways drives cancer hyperprogression during immunotherapy. Cancer Cell (2023) 41(2):304–322.e307. doi: 10.1016/j.ccell.2022.12.008
57. Baxi S, Yang A, Gennarelli RL, Khan N, Wang Z, Boyce L, et al. Immune-related adverse events for anti-PD-1 and anti-PD-L1 drugs: systematic review and meta-analysis. Bmj (2018) 360:k793. doi: 10.1136/bmj.k793
58. Bareche Y, Kelly D, Abbas-Aghababazadeh F, Nakano M, Esfahani PN, Tkachuk D, et al. Leveraging big data of immune checkpoint blockade response identifies novel potential targets. Ann Oncol (2022) 33(12):1304–17. doi: 10.1016/j.annonc.2022.08.084
59. Yoon HH, Jin Z, Kour O, Kankeu Fonkoua LA, Shitara K, Gibson MK, et al. Association of PD-L1 expression and other variables with benefit from immune checkpoint inhibition in advanced gastroesophageal cancer: systematic review and meta-analysis of 17 phase 3 randomized clinical trials. JAMA Oncol (2022) 8(10):1456–65. doi: 10.1001/jamaoncol.2022.3707
60. Ilie M, Hofman V, Dietel M, Soria JC, Hofman P. Assessment of the PD-L1 status by immunohistochemistry: challenges and perspectives for therapeutic strategies in lung cancer patients. Virchows Arch (2016) 468(5):511–25. doi: 10.1007/s00428-016-1910-4
61. Shen X, Zhao B. Efficacy of PD-1 or PD-L1 inhibitors and PD-L1 expression status in cancer: meta-analysis. Bmj (2018) 362:k3529. doi: 10.1136/bmj.k3529
62. Khagi Y, Kurzrock R, Patel SP. Next generation predictive biomarkers for immune checkpoint inhibition. Cancer Metastasis Rev (2017) 36(1):179–90. doi: 10.1007/s10555-016-9652-y
63. Rizvi NA, Hellmann MD, Snyder A, Kvistborg P, Makarov V, Havel JJ, et al. Cancer immunology. mutational landscape determines sensitivity to PD-1 blockade in non-small cell lung cancer. Science (2015) 348(6230):124–8. doi: 10.1126/science.aaa1348
64. Cristescu R, Mogg R, Ayers M, Albright A, Murphy E, Yearley J, et al. Pan-tumor genomic biomarkers for PD-1 checkpoint blockade-based immunotherapy. Science (2018) 362(6411):eaar3593. doi: 10.1126/science.aar3593
65. Chung J, Maruvka YE, Sudhaman S, Kelly J, Haradhvala NJ, Bianchi V, et al. DNA Polymerase and mismatch repair exert distinct microsatellite instability signatures in normal and malignant human cells. Cancer Discovery (2021) 11(5):1176–91. doi: 10.1158/2159-8290.CD-20-0790
66. Jackson SS, Van Dyke AL, Zhu B, Pfeiffer RM, Petrick JL, Adami HO, et al. Anthropometric risk factors for cancers of the biliary tract in the biliary tract cancers pooling project. Cancer Res (2019) 79(15):3973–82. doi: 10.1158/0008-5472.CAN-19-0459
67. Hegde PS, Chen DS. Top 10 challenges in cancer immunotherapy. Immunity (2020) 52(1):17–35. doi: 10.1016/j.immuni.2019.12.011
68. Binnewies M, Roberts EW, Kersten K, Chan V, Fearon DF, Merad M, et al. Understanding the tumor immune microenvironment (TIME) for effective therapy. Nat Med (2018) 24(5):541–50. doi: 10.1038/s41591-018-0014-x
69. Picard E, Verschoor CP, Ma GW, Pawelec G. Relationships between immune landscapes, genetic subtypes and responses to immunotherapy in colorectal cancer. Front Immunol (2020) 11:369. doi: 10.3389/fimmu.2020.00369
70. Zhang L, Conejo-Garcia JR, Katsaros D, Gimotty PA, Massobrio M, Regnani G, et al. Intratumoral T cells, recurrence, and survival in epithelial ovarian cancer. N Engl J Med (2003) 348(3):203–13. doi: 10.1056/NEJMoa020177
71. Guignabert C, Humbert M. Targeting transforming growth factor-β receptors in pulmonary hypertension. Eur Respir J (2021) 57(2):2002341. doi: 10.1183/13993003.02341-2020
72. Morikawa M, Derynck R, Miyazono K. TGF-β and the TGF-β family: context-dependent roles in cell and tissue physiology. Cold Spring Harb Perspect Biol (2016) 8(5):a021873. doi: 10.1101/cshperspect.a021873
73. Li Y, Yan J, Chang HM, Chen ZJ, Leung PCK. Roles of TGF-β superfamily proteins in extravillous trophoblast invasion. Trends Endocrinol Metab (2021) 32(3):170–89. doi: 10.1016/j.tem.2020.12.005
74. Yi M, Niu M, Wu Y, Ge H, Jiao D, Zhu S, et al. Combination of oral STING agonist MSA-2 and anti-TGF-β/PD-L1 bispecific antibody YM101: a novel immune cocktail therapy for non-inflamed tumors. J Hematol Oncol (2022) 15(1):142. doi: 10.1186/s13045-022-01363-8
75. Kobayashi H, Gieniec KA, Wright JA, Wang T, Asai N, Mizutani Y, et al. The balance of stromal BMP signaling mediated by GREM1 and ISLR drives colorectal carcinogenesis. Gastroenterology (2021) 160(4):1224–1239.e1230. doi: 10.1053/j.gastro.2020.11.011
76. Cangkrama M, Wietecha M, Werner S. Wound repair, scar formation, and cancer: converging on activin. Trends Mol Med (2020) 26(12):1107–17. doi: 10.1016/j.molmed.2020.07.009
77. Zhu S, Li Y, Bennett S, Chen J, Weng IZ, Huang L, et al. The role of glial cell line-derived neurotrophic factor family member artemin in neurological disorders and cancers. Cell Prolif (2020) 53(7):e12860. doi: 10.1111/cpr.12860
78. Sun T, Huang Z, Liang WC, Yin J, Lin WY, Wu J, et al. TGFβ2 and TGFβ3 isoforms drive fibrotic disease pathogenesis. Sci Transl Med (2021) 13(605):eabe0407. doi: 10.1126/scitranslmed.abe0407
79. Shi M, Zhu J, Wang R, Chen X, Mi L, Walz T, et al. Latent TGF-β structure and activation. Nature (2011) 474(7351):343–9. doi: 10.1038/nature10152
80. Metelli A, Salem M, Wallace CH, Wu BX, Li A, Li X, et al. Immunoregulatory functions and the therapeutic implications of GARP-TGF-β in inflammation and cancer. J Hematol Oncol (2018) 11(1):24. doi: 10.1186/s13045-018-0570-z
81. Wang D, Sun Z, Zhu X, Zheng X, Zhou Y, Lu Y, et al. GARP-mediated active TGF-β1 induces bone marrow NK cell dysfunction in AML patients with early relapse post-allo-HSCT. Blood (2022) 140(26):2788–804. doi: 10.1182/blood.2022015474
82. Duan Z, Lin X, Wang L, Zhen Q, Jiang Y, Chen C, et al. Specificity of TGF-β1 signal designated by LRRC33 and integrin α(V)β(8). Nat Commun (2022) 13(1):4988. doi: 10.1038/s41467-022-32655-9
83. Dong X, Zhao B, Iacob RE, Zhu J, Koksal AC, Lu C, et al. Force interacts with macromolecular structure in activation of TGF-β. Nature (2017) 542(7639):55–9. doi: 10.1038/nature21035
84. Campbell MG, Cormier A, Ito S, Seed RI, Bondesson AJ, Lou J, et al. Cryo-EM reveals integrin-mediated TGF-β activation without release from latent TGF-β. Cell (2020) 180(3):490–501.e416. doi: 10.1016/j.cell.2019.12.030
85. Vander Ark A, Cao J, Li X. TGF-β receptors: in and beyond TGF-β signaling. Cell Signalling (2018) 52:112–20. doi: 10.1016/j.cellsig.2018.09.002
86. Blobe GC, Liu X, Fang SJ, How T, Lodish HF. A novel mechanism for regulating transforming growth factor β (TGF-β) signaling: FUNCTIONAL MODULATION OF TYPE III TGF-β RECEPTOR EXPRESSION THROUGH INTERACTION WITH THE PDZ DOMAIN PROTEIN, GIPC*. J Biol Chem (2001) 276(43):39608–17. doi: 10.1074/jbc.M106831200
87. Nishida J, Miyazono K, Ehata S. Decreased TGFBR3/betaglycan expression enhances the metastatic abilities of renal cell carcinoma cells through TGF-β-dependent and -independent mechanisms. Oncogene (2018) 37(16):2197–212. doi: 10.1038/s41388-017-0084-0
88. Dalin MG, Katabi N, Persson M, Lee KW, Makarov V, Desrichard A, et al. Multi-dimensional genomic analysis of myoepithelial carcinoma identifies prevalent oncogenic gene fusions. Nat Commun (2017) 8(1):1197. doi: 10.1038/s41467-017-01178-z
89. Moreau JM, Velegraki M, Bolyard C, Rosenblum MD, Li Z. Transforming growth factor-β1 in regulatory T cell biology. Sci Immunol (2022) 7(69):eabi4613. doi: 10.1126/sciimmunol.abi4613
90. Liu Q, Sun H, Liu Y, Li X, Xu B, Li L, et al. HTR1A inhibits the progression of triple-negative breast cancer via TGF-β canonical and noncanonical pathways. Adv Sci (Weinh) (2022) 9(12):e2105672. doi: 10.1002/advs.202105672
91. Balkwill FR, Mantovani A. Cancer-related inflammation: common themes and therapeutic opportunities. Semin Cancer Biol (2012) 22(1):33–40. doi: 10.1016/j.semcancer.2011.12.005
92. Frangogiannis N. Transforming growth factor-β in tissue fibrosis. J Exp Med (2020) 217(3):e20190103. doi: 10.1084/jem.20190103
93. Massagué J. TGFβ signalling in context. Nat Rev Mol Cell Biol (2012) 13(10):616–30. doi: 10.1038/nrm3434
94. Yu Y, Feng X-H. TGF-β signaling in cell fate control and cancer. Curr Opin Cell Biol (2019) 61:56–63. doi: 10.1016/j.ceb.2019.07.007
95. Yoshida GJ. Regulation of heterogeneous cancer-associated fibroblasts: the molecular pathology of activated signaling pathways. J Exp Clin Cancer Res (2020) 39(1):112. doi: 10.1186/s13046-020-01611-0
96. Buechler MB, Pradhan RN, Krishnamurty AT, Cox C, Calviello AK, Wang AW, et al. Cross-tissue organization of the fibroblast lineage. Nature (2021) 593(7860):575–9. doi: 10.1038/s41586-021-03549-5
97. Chen Y, McAndrews KM, Kalluri R. Clinical and therapeutic relevance of cancer-associated fibroblasts. Nat Rev Clin Oncol (2021) 18(12):792–804. doi: 10.1038/s41571-021-00546-5
98. Liu J, Chen S, Wang W, Ning BF, Chen F, Shen W, et al. Cancer-associated fibroblasts promote hepatocellular carcinoma metastasis through chemokine-activated hedgehog and TGF-β pathways. Cancer Lett (2016) 379(1):49–59. doi: 10.1016/j.canlet.2016.05.022
99. De Craene B, Berx G. Regulatory networks defining EMT during cancer initiation and progression. Nat Rev Cancer (2013) 13(2):97–110. doi: 10.1038/nrc3447
100. Lamouille S, Xu J, Derynck R. Molecular mechanisms of epithelial-mesenchymal transition. Nat Rev Mol Cell Biol (2014) 15(3):178–96. doi: 10.1038/nrm3758
101. Wang X, Abraham S, McKenzie JAG, Jeffs N, Swire M, Tripathi VB, et al. LRG1 promotes angiogenesis by modulating endothelial TGF-β signalling. Nature (2013) 499(7458):306–11. doi: 10.1038/nature12345
102. Unterleuthner D, Neuhold P, Schwarz K, Janker L, Neuditschko B, Nivarthi H, et al. Cancer-associated fibroblast-derived WNT2 increases tumor angiogenesis in colon cancer. Angiogenesis (2020) 23(2):159–77. doi: 10.1007/s10456-019-09688-8
103. Tanaka A, Sakaguchi S. Regulatory T cells in cancer immunotherapy. Cell Res (2017) 27(1):109–18. doi: 10.1038/cr.2016.151
104. Curotto de Lafaille MA, Lafaille JJ. Natural and adaptive foxp3+ regulatory T cells: more of the same or a division of labor? Immunity (2009) 30(5):626–35. doi: 10.1016/j.immuni.2009.05.002
105. Klein L, Robey EA, Hsieh CS. Central CD4(+) T cell tolerance: deletion versus regulatory T cell differentiation. Nat Rev Immunol (2019) 19(1):7–18. doi: 10.1038/s41577-018-0083-6
106. Seed RI, Kobayashi K, Ito S, Takasaka N, Cormier A, Jespersen JM, et al. A tumor-specific mechanism of t(reg) enrichment mediated by the integrin αvβ8. Sci Immunol (2021) 6(57):eabf0558. doi: 10.1126/sciimmunol.abf0558
107. Hemmers S, Schizas M, Azizi E, Dikiy S, Zhong Y, Feng Y, et al. IL-2 production by self-reactive CD4 thymocytes scales regulatory T cell generation in the thymus. J Exp Med (2019) 216(11):2466–78. doi: 10.1084/jem.20190993
108. Hinterberger M, Aichinger M, Prazeres da Costa O, Voehringer D, Hoffmann R, Klein L. Autonomous role of medullary thymic epithelial cells in central CD4(+) T cell tolerance. Nat Immunol (2010) 11(6):512–9. doi: 10.1038/ni.1874
109. Irla M. Instructive cues of thymic T cell selection. Annu Rev Immunol (2022) 40:95–119. doi: 10.1146/annurev-immunol-101320-022432
110. van der Veeken J, Campbell C, Pritykin Y, Schizas M, Verter J, Hu W, et al. Genetic tracing reveals transcription factor Foxp3-dependent and Foxp3-independent functionality of peripherally induced treg cells. Immunity (2022) 55(7):1173–1184.e1177. doi: 10.1016/j.immuni.2022.05.010
111. Hauri-Hohl M, Zuklys S, Holländer GA, Ziegler SF. A regulatory role for TGF-β signaling in the establishment and function of the thymic medulla. Nat Immunol (2014) 15(6):554–61. doi: 10.1038/ni.2869
112. Edwards JP, Thornton AM, Shevach EM. Release of active TGF-β1 from the latent TGF-β1/GARP complex on T regulatory cells is mediated by integrin β8. J Immunol (2014) 193(6):2843–9. doi: 10.4049/jimmunol.1401102
113. Worthington JJ, Kelly A, Smedley C, Bauché D, Campbell S, Marie JC, et al. Integrin αvβ8-mediated TGF-β activation by effector regulatory T cells is essential for suppression of T-Cell-Mediated inflammation. Immunity (2015) 42(5):903–15. doi: 10.1016/j.immuni.2015.04.012
114. Wang Z, Lu Z, Lin S, Xia J, Zhong Z, Xie Z, et al. Leucine-tRNA-synthetase-2-expressing b cells contribute to colorectal cancer immunoevasion. Immunity (2022) 55(6):1067–1081.e1068. doi: 10.1016/j.immuni.2022.04.017
115. Rosser EC, Mauri C. Regulatory b cells: origin, phenotype, and function. Immunity (2015) 42(4):607–12. doi: 10.1016/j.immuni.2015.04.005
116. Ramalingam R, Larmonier CB, Thurston RD, Midura-Kiela MT, Zheng SG, Ghishan FK, et al. Dendritic cell-specific disruption of TGF-β receptor II leads to altered regulatory T cell phenotype and spontaneous multiorgan autoimmunity. J Immunol (2012) 189(8):3878–93. doi: 10.4049/jimmunol.1201029
117. Morante-Palacios O, Fondelli F, Ballestar E, Martínez-Cáceres EM. Tolerogenic dendritic cells in autoimmunity and inflammatory diseases. Trends Immunol (2021) 42(1):59–75. doi: 10.1016/j.it.2020.11.001
118. Das L, Levine AD. TGF-beta inhibits IL-2 production and promotes cell cycle arrest in TCR-activated effector/memory T cells in the presence of sustained TCR signal transduction. J Immunol (2008) 180(3):1490–8. doi: 10.4049/jimmunol.180.3.1490
119. Gorelik L, Constant S, Flavell RA. Mechanism of transforming growth factor beta-induced inhibition of T helper type 1 differentiation. J Exp Med (2002) 195(11):1499–505. doi: 10.1084/jem.20012076
120. Liu M, Kuo F, Capistrano KJ, Kang D, Nixon BG, Shi W, et al. TGF-β suppresses type 2 immunity to cancer. Nature (2020) 587(7832):115–20. doi: 10.1038/s41586-020-2836-1
121. Li S, Liu M, Do MH, Chou C, Stamatiades EG, Nixon BG, et al. Cancer immunotherapy via targeted TGF-β signalling blockade in T(H) cells. Nature (2020) 587(7832):121–5. doi: 10.1038/s41586-020-2850-3
122. Bettelli E, Carrier Y, Gao W, Korn T, Strom TB, Oukka M, et al. Reciprocal developmental pathways for the generation of pathogenic effector TH17 and regulatory T cells. Nature (2006) 441(7090):235–8. doi: 10.1038/nature04753
123. Geginat J, Paroni M, Kastirr I, Larghi P, Pagani M, Abrignani S. Reverse plasticity: TGF-β and IL-6 induce Th1-to-Th17-cell transdifferentiation in the gut. Eur J Immunol (2016) 46(10):2306–10. doi: 10.1002/eji.201646618
124. Zhang M, Pan X, Fujiwara K, Jurcak N, Muth S, Zhou J, et al. Pancreatic cancer cells render tumor-associated macrophages metabolically reprogrammed by a GARP and DNA methylation-mediated mechanism. Signal Transduct Target Ther (2021) 6(1):366. doi: 10.1038/s41392-021-00461-2
125. Fridlender ZG, Sun J, Kim S, Kapoor V, Cheng G, Ling L, et al. Polarization of tumor-associated neutrophil phenotype by TGF-beta: "N1" versus "N2" TAN. Cancer Cell (2009) 16(3):183–94. doi: 10.1016/j.ccr.2009.06.017
126. Jaillon S, Ponzetta A, Di Mitri D, Santoni A, Bonecchi R, Mantovani A. Neutrophil diversity and plasticity in tumour progression and therapy. Nat Rev Cancer (2020) 20(9):485–503. doi: 10.1038/s41568-020-0281-y
127. Viel S, Marçais A, Guimaraes FS, Loftus R, Rabilloud J, Grau M, et al. TGF-β inhibits the activation and functions of NK cells by repressing the mTOR pathway. Sci Signal (2016) 9(415):ra19. doi: 10.1126/scisignal.aad1884
128. Kegyes D, Constantinescu C, Vrancken L, Rasche L, Gregoire C, Tigu B, et al. Patient selection for CAR T or BiTE therapy in multiple myeloma: which treatment for each patient? J Hematol Oncol (2022) 15(1):78. doi: 10.1186/s13045-022-01296-2
129. Wang Z, Yin C, Lum LG, Simons A, Weiner GJ. Bispecific antibody-activated T cells enhance NK cell-mediated antibody-dependent cellular cytotoxicity. J Hematol Oncol (2021) 14(1):204. doi: 10.1186/s13045-021-01216-w
130. Yu S, Zhang J, Yan Y, Yao X, Fang L, Xiong H, et al. A novel asymmetrical anti-HER2/CD3 bispecific antibody exhibits potent cytotoxicity for HER2-positive tumor cells. J Exp Clin Cancer Res (2019) 38(1):355. doi: 10.1186/s13046-019-1354-1
131. Wu Y, Yi M, Zhu S, Wang H, Wu K. Recent advances and challenges of bispecific antibodies in solid tumors. Exp Hematol Oncol (2021) 10(1):56. doi: 10.1186/s40164-021-00250-1
132. Tian Z, Liu M, Zhang Y, Wang X. Bispecific T cell engagers: an emerging therapy for management of hematologic malignancies. J Hematol Oncol (2021) 14(1):75. doi: 10.1186/s13045-021-01084-4
133. Weiner LM, Surana R, Wang S. Monoclonal antibodies: versatile platforms for cancer immunotherapy. Nat Rev Immunol (2010) 10(5):317–27. doi: 10.1038/nri2744
134. Lameris R, de Bruin RC, Schneiders FL, van Bergen en Henegouwen PM, Verheul HM, de Gruijl TD, et al. Bispecific antibody platforms for cancer immunotherapy. Crit Rev Oncol Hematol (2014) 92(3):153–65. doi: 10.1016/j.critrevonc.2014.08.003
135. Carter PJ. Potent antibody therapeutics by design. Nat Rev Immunol (2006) 6(5):343–57. doi: 10.1038/nri1837
136. Brinkmann U, Kontermann RE. The making of bispecific antibodies. MAbs (2017) 9(2):182–212. doi: 10.1080/19420862.2016.1268307
137. Zhang X, Yang Y, Fan D, Xiong D. The development of bispecific antibodies and their applications in tumor immune escape. Exp Hematol Oncol (2017) 6:12. doi: 10.1186/s40164-017-0072-7
138. Yu S, Liu Q, Han X, Qin S, Zhao W, Li A, et al. Development and clinical application of anti-HER2 monoclonal and bispecific antibodies for cancer treatment. Exp Hematol Oncol (2017) 6:31. doi: 10.1186/s40164-017-0091-4
139. Philip M, Schietinger A. CD8(+) T cell differentiation and dysfunction in cancer. Nat Rev Immunol (2022) 22(4):209–23. doi: 10.1038/s41577-021-00574-3
140. Staerz UD, Kanagawa O, Bevan MJ. Hybrid antibodies can target sites for attack by T cells. Nature (1985) 314(6012):628–31. doi: 10.1038/314628a0
141. Goebeler ME, Bargou R. Blinatumomab: a CD19/CD3 bispecific T cell engager (BiTE) with unique anti-tumor efficacy. Leuk Lymphoma (2016) 57(5):1021–32. doi: 10.3109/10428194.2016.1161185
142. Sebastian M, Kiewe P, Schuette W, Brust D, Peschel C, Schneller F, et al. Treatment of malignant pleural effusion with the trifunctional antibody catumaxomab (Removab) (anti-EpCAM x anti-CD3): results of a phase 1/2 study. J Immunother (2009) 32(2):195–202. doi: 10.1097/CJI.0b013e318195b5bb
143. Clynes RA, Desjarlais JR. Redirected T cell cytotoxicity in cancer therapy. Annu Rev Med (2019) 70:437–50. doi: 10.1146/annurev-med-062617-035821
144. Luke JJ, Patel MR, Hamilton EP, Chmielowski B, Ulahannan SV, Kindler HL, et al. First-in-human, open-label, dose-escalation study of MGD013, a bispecific DART molecule binding PD-1 and LAG-3, in patients with unresectable or metastatic neoplasms. J Clin Oncol (2020) 38(15_suppl):3004–4. doi: 10.1200/JCO.2020.38.15_suppl.3004
145. Schaller TH, Snyder DJ, Spasojevic I, Gedeon PC, Sanchez-Perez L, Sampson JH. First in human dose calculation of a single-chain bispecific antibody targeting glioma using the MABEL approach. J Immunother Cancer (2020) 8(1):e000213. doi: 10.1136/jitc-2019-000213
146. Hummel HD, Kufer P, Grüllich C, Seggewiss-Bernhardt R, Deschler-Baier B, Chatterjee M, et al. Pasotuxizumab, a BiTE(®) immune therapy for castration-resistant prostate cancer: phase I, dose-escalation study findings. Immunotherapy (2021) 13(2):125–41. doi: 10.2217/imt-2020-0256
147. Ishiguro T, Sano Y, Komatsu SI, Kamata-Sakurai M, Kaneko A, Kinoshita Y, et al. An anti-glypican 3/CD3 bispecific T cell-redirecting antibody for treatment of solid tumors. Sci Transl Med (2017) 9(410):eaal4291. doi: 10.1126/scitranslmed.aal4291
148. Root AR, Cao W, Li B, LaPan P, Meade C, Sanford J, et al. Development of PF-06671008, a highly potent anti-P-cadherin/Anti-CD3 bispecific DART molecule with extended half-life for the treatment of cancer. Antibodies (Basel) (2016) 5(1):6. doi: 10.3390/antib5010006
149. Dao T, Pankov D, Scott A, Korontsvit T, Zakhaleva V, Xu Y, et al. Therapeutic bispecific T-cell engager antibody targeting the intracellular oncoprotein WT1. Nat Biotechnol (2015) 33(10):1079–86. doi: 10.1038/nbt.3349
150. Tolcher AW, Alley EW, Chichili G, Baughman JE, Moore PA, Bonvini E, et al. Phase 1, first-in-human, open label, dose escalation ctudy of MGD009, a humanized B7-H3 x CD3 dual-affinity re-targeting (DART) protein in patients with B7-H3-expressing neoplasms or B7-H3 expressing tumor vasculature. J Clin Oncol (2016) 34(15_suppl):TPS3105–TPS3105. doi: 10.1200/JCO.2016.34.15_suppl.TPS3105
151. Chang AY, Dao T, Gejman RS, Jarvis CA, Scott A, Dubrovsky L, et al. A therapeutic T cell receptor mimic antibody targets tumor-associated PRAME peptide/HLA-I antigens. J Clin Invest (2017) 127(7):2705–18. doi: 10.1172/JCI92335
152. Jaiswal S, Jamieson CH, Pang WW, Park CY, Chao MP, Majeti R, et al. CD47 is upregulated on circulating hematopoietic stem cells and leukemia cells to avoid phagocytosis. Cell (2009) 138(2):271–85. doi: 10.1016/j.cell.2009.05.046
153. Piccione EC, Juarez S, Liu J, Tseng S, Ryan CE, Narayanan C, et al. A bispecific antibody targeting CD47 and CD20 selectively binds and eliminates dual antigen expressing lymphoma cells. MAbs (2015) 7(5):946–56. doi: 10.1080/19420862.2015.1062192
154. Dheilly E, Moine V, Broyer L, Salgado-Pires S, Johnson Z, Papaioannou A, et al. Selective blockade of the ubiquitous checkpoint receptor CD47 is enabled by dual-targeting bispecific antibodies. Mol Ther (2017) 25(2):523–33. doi: 10.1016/j.ymthe.2016.11.006
155. Wei SC, Duffy CR, Allison JP. Fundamental mechanisms of immune checkpoint blockade therapy. Cancer Discovery (2018) 8(9):1069–86. doi: 10.1158/2159-8290.CD-18-0367
156. Chester C, Sanmamed MF, Wang J, Melero I. Immunotherapy targeting 4-1BB: mechanistic rationale, clinical results, and future strategies. Blood (2018) 131(1):49–57. doi: 10.1182/blood-2017-06-741041
157. Kuang Z, Pu P, Wu M, Wu Z, Wang L, Li Y, et al. A novel bispecific antibody with PD-L1-assisted OX40 activation for cancer treatment. Mol Cancer Ther (2020) 19(12):2564–74. doi: 10.1158/1535-7163.MCT-20-0226
158. Zhu S, Zhang T, Zheng L, Liu H, Song W, Liu D, et al. Combination strategies to maximize the benefits of cancer immunotherapy. J Hematol Oncol (2021) 14(1):156. doi: 10.1186/s13045-021-01164-5
159. Herrmann M, Krupka C, Deiser K, Brauchle B, Marcinek A, Ogrinc Wagner A, et al. Bifunctional PD-1 × αCD3 × αCD33 fusion protein reverses adaptive immune escape in acute myeloid leukemia. Blood (2018) 132(23):2484–94. doi: 10.1182/blood-2018-05-849802
160. Topp MS, Gökbuget N, Stein AS, Zugmaier G, O'Brien S, Bargou RC, et al. Safety and activity of blinatumomab for adult patients with relapsed or refractory b-precursor acute lymphoblastic leukaemia: a multicentre, single-arm, phase 2 study. Lancet Oncol (2015) 16(1):57–66. doi: 10.1016/S1470-2045(14)71170-2
161. von Stackelberg A, Locatelli F, Zugmaier G, Handgretinger R, Trippett TM, Rizzari C, et al. Phase I/Phase II study of blinatumomab in pediatric patients with Relapsed/Refractory acute lymphoblastic leukemia. J Clin Oncol (2016) 34(36):4381–9. doi: 10.1200/JCO.2016.67.3301
162. Shimabukuro-Vornhagen A, Gödel P, Subklewe M, Stemmler HJ, Schlößer HA, Schlaak M, et al. Cytokine release syndrome. J Immunother Cancer (2018) 6(1):56. doi: 10.1186/s40425-018-0343-9
163. Obstfeld AE, Frey NV, Mansfield K, Lacey SF, June CH, Porter DL, et al. Cytokine release syndrome associated with chimeric-antigen receptor T-cell therapy: clinicopathological insights. Blood (2017) 130(23):2569–72. doi: 10.1182/blood-2017-08-802413
164. Giavridis T, van der Stegen SJC, Eyquem J, Hamieh M, Piersigilli A, Sadelain M. CAR T cell-induced cytokine release syndrome is mediated by macrophages and abated by IL-1 blockade. Nat Med (2018) 24(6):731–8. doi: 10.1038/s41591-018-0041-7
165. Sterner RM, Sakemura R, Cox MJ, Yang N, Khadka RH, Forsman CL, et al. GM-CSF inhibition reduces cytokine release syndrome and neuroinflammation but enhances CAR-T cell function in xenografts. Blood (2019) 133(7):697–709. doi: 10.1182/blood-2018-10-881722
166. Kenderian SS, Ruella M, Shestova O, Kim MY, Klichinsky M, Chen F, et al. Ruxolitinib prevents cytokine release syndrome after CART cell therapy without impairing the anti-tumor effect in a xenograft model. Blood (2016) 128(22):652. doi: 10.1182/blood.V128.22.652.652
167. Ruella M, Kenderian SS, Shestova O, Klichinsky M, Melenhorst JJ, Wasik MA, et al. Kinase inhibitor ibrutinib to prevent cytokine-release syndrome after anti-CD19 chimeric antigen receptor T cells for b-cell neoplasms. Leukemia (2017) 31(1):246–8. doi: 10.1038/leu.2016.262
168. Yi M, Niu M, Zhang J, Li S, Zhu S, Yan Y, et al. Combine and conquer: manganese synergizing anti-TGF-β/PD-L1 bispecific antibody YM101 to overcome immunotherapy resistance in non-inflamed cancers. J Hematol Oncol (2021) 14(1):146. doi: 10.1186/s13045-021-01155-6
169. Knudson KM, Hicks KC, Luo X, Chen JQ, Schlom J, Gameiro SR. M7824, a novel bifunctional anti-PD-L1/TGFβ trap fusion protein, promotes anti-tumor efficacy as monotherapy and in combination with vaccine. Oncoimmunology (2018) 7(5):e1426519. doi: 10.1080/2162402X.2018.1426519
170. Paz-Ares L, Kim TM, Vicente D, Felip E, Lee DH, Lee KH, et al. Bintrafusp Alfa, a bifunctional fusion protein targeting TGF-β and PD-L1, in second-line treatment of patients with NSCLC: results from an expansion cohort of a phase 1 trial. J Thorac Oncol (2020) 15(7):1210–22. doi: 10.1016/j.jtho.2020.03.003
171. Zhang J, Yi J, Zhou P. Development of bispecific antibodies in China: overview and prospects. Antib Ther (2020) 3(2):126–45. doi: 10.1093/abt/tbaa011
172. Cheng B, Ding K, Chen P, Ji J, Luo T, Guo X, et al. Anti-PD-L1/TGF-βR fusion protein (SHR-1701) overcomes disrupted lymphocyte recovery-induced resistance to PD-1/PD-L1 inhibitors in lung cancer. Cancer Commun (Lond) (2022) 42(1):17–36. doi: 10.1002/cac2.12244
173. Liu D, Zhou J, Wang Y, Li M, Jiang H, Liu Y, et al. Bifunctional anti-PD-L1/TGF-βRII agent SHR-1701 in advanced solid tumors: a dose-escalation, dose-expansion, and clinical-expansion phase 1 trial. BMC Med (2022) 20(1):408. doi: 10.1186/s12916-022-02605-9
174. Feng J, Tang D, Wang J, Zhou Q, Peng J, Lou H, et al. SHR-1701, a bifunctional fusion protein targeting PD-L1 and TGFβ, for recurrent or metastatic cervical cancer: a clinical expansion cohort of a phase I study. Clin Cancer Res (2022) 28(24):5297–305. doi: 10.1158/1078-0432.CCR-22-0346
175. Gao ZZ, Li C, Chen G, Yuan JJ, Zhou YQ, Jiao JY, et al. Optimization strategies for expression of a novel bifunctional anti-PD-L1/TGFBR2-ECD fusion protein. Protein Expr Purif (2022) 189:105973. doi: 10.1016/j.pep.2021.105973
Keywords: cancer immunotherapy, immunotherapy resistance, immune checkpoint inhibitor, bispecific antibody, TGF-β, PD-L1
Citation: Li T, Wang X, Niu M, Wang M, Zhou J, Wu K and Yi M (2023) Bispecific antibody targeting TGF-β and PD-L1 for synergistic cancer immunotherapy. Front. Immunol. 14:1196970. doi: 10.3389/fimmu.2023.1196970
Received: 30 March 2023; Accepted: 28 June 2023;
Published: 13 July 2023.
Edited by:
Prakash Radhakrishnan, University of Nebraska Medical Center, United StatesReviewed by:
Dongyao Wang, University of Science and Technology of China, ChinaCopyright © 2023 Li, Wang, Niu, Wang, Zhou, Wu and Yi. This is an open-access article distributed under the terms of the Creative Commons Attribution License (CC BY). The use, distribution or reproduction in other forums is permitted, provided the original author(s) and the copyright owner(s) are credited and that the original publication in this journal is cited, in accordance with accepted academic practice. No use, distribution or reproduction is permitted which does not comply with these terms.
*Correspondence: Ming Yi, bWluZ3lpX29uY29Ab3V0bG9vay5jb20=; MTMyMjA2OEB6anUuZWR1LmNu; Kongming Wu, a213dUB0amgudGptdS5lZHUuY24=; d3VrbV9sYWJAMTYzLmNvbQ==
†These authors have contributed equally to this work
Disclaimer: All claims expressed in this article are solely those of the authors and do not necessarily represent those of their affiliated organizations, or those of the publisher, the editors and the reviewers. Any product that may be evaluated in this article or claim that may be made by its manufacturer is not guaranteed or endorsed by the publisher.
Research integrity at Frontiers
Learn more about the work of our research integrity team to safeguard the quality of each article we publish.