- 1Key Laboratory of Organ Regeneration and Transplantation of Ministry of Education, The First Hospital, Jilin University, Changchun, China
- 2Department of Dermatology, The First Hospital, Jilin University, Changchun, China
- 3Department of Hematology, The First Hospital, Jilin University, Changchun, China
Hematological malignancy is a disease arisen by complicate reasons that seriously endangers human health. The research on its pathogenesis and therapies depends on the usage of animal models. Conventional animal model cannot faithfully mirror some characteristics of human features due to the evolutionary divergence, whereas the mouse models hosting human hematological malignancy are more and more applied in basic as well as translational investigations in recent years. According to the construction methods, they can be divided into different types (e.g. cell-derived xenograft (CDX) and patient-derived xenograft model (PDX) model) that have diverse characteristics and application values. In addition, a variety of strategies have been developed to improve human hematological malignant cell engraftment and differentiation in vivo. Moreover, the humanized mouse model with both functional human immune system and autologous human hematological malignancy provides a unique tool for the evaluation of the efficacy of novel immunotherapeutic drugs/approaches. Herein, we first review the evolution of the mouse model of human hematological malignancy; Then, we analyze the characteristics of different types of models and summarize the ways to improve the models; Finally, the way and value of humanized mouse model of human immune system in the immunotherapy of human hematological malignancy are discussed.
1 Introduction
Hematopoietic system is formed by a complex differentiation process (e.g. from hematopoietic stem cells (HSCs) to different lineages of blood/immune subsets) and plays an important role in maintaining oxygen exchange and immune monitoring. Hematopoietic system is controlled by external and internal factors, such as the hematopoietic microenvironment, transcription factors, signal transduction pathways and chromatin modifiers (1). Abnormal changes in any factor may cause serious hematological diseases (2, 3) and endanger human health. Among these diseases, hematological malignancies have the most serious impact on human health.
Hematological malignancy refers to a group of heterogeneous and life-threatening serious diseases mainly caused by abnormal HSCs, which lead to impairment in different stages of the differentiation process, including differentiation block, apoptosis disorder and malignant proliferation (4). According to the American Cancer Society, hematological malignancies account for approximately 6%-10% of all malignancies, and the mortality rate is 5.8% of all malignancies (5). Therefore, research on the pathogenesis, drug development and therapeutic approaches is of great significance to reduce the incidence and mortality rates of hematological malignancies.
According to the fifth edition of the World Health Organization (WHO) classification of hematolymphoid tumors in 2022 (6), hematological malignancies mainly include leukemia, lymphoma, myelodysplastic tumors (MDS) and multiple myeloma (MM). Treatment methods for hematological malignancies include chemotherapy, radiotherapy, molecular targeted drug therapy, HSC transplantation, and immunotherapy. Although some types of human hematological malignancies (such as acute promyelocytic leukemia, chronic myelogenous leukemia (CML) and chronic lymphocytic leukemia (CLL)) (7) have been basically eradicated, most types of hematological malignancies still have problems such as low long-term cure rate and high recurrence rate. Moreover, the current treatment methods still require further improvement because of some associated serious side effects (8).
Animal models are an important tool for the study of pathogenesis and drug/therapy of hematological malignancy, and mouse models are most widely used. However, a large amount of evidence shows that the conclusions obtained from conventional mice or drugs/therapies developed for mice cannot be applied to humans. The main reason for this is that there are huge evolutionary differences between rodents and humans (9). To solve this problem, researchers have constructed multiple types of mouse models with human hematological malignancies and applied them to basic research and drug research, which promote the development of this field (10). Recently, immunotherapy has become an important therapy for the treatment of hematological malignancies; The humanized mouse model, which can reproduce the process of human immunotherapy, also plays an increasingly important role in the study of immunotherapy for hematological malignancies.
This review first describes the development of mouse models of human hematological malignancies and their application in preclinical research, summarizes the construction methods and technical characteristics with a comparison of their advantages and disadvantages, and finally discusses future development trends.
2 Generation of immunodeficient mouse strains for human hematological malignancy investigation
Robust xenogeneic immune reaction is the first obstacle impeding the development of mouse model with human hematological malignant cell repopulation. In this process, both innate and adaptive immune systems play an important role (11). Immunodeficient mice can be prepared by modifying key genes that regulate the development, survival and function of immune cells, which lays the foundation for replicating human hematological malignancy in mice. Therefore, immunodeficient mouse strains play an important role in promoting the development of human hematological malignancy mouse models (Figure 1).
2.1 Foxn1-/- nude mice
Foxn1-/- nude mice were the first immunodeficient mice used in the study of human malignancies. Foxn1-/- nude mice are knocked out of the forkhead box N1 (Foxn1, formerly known as Why or Hfh11) gene, with congenital absence of thymus and T cells, which can alleviate T-cell-mediated host versus gradient reaction xenograft rejection. Rygaard and Povlsen successfully used nude mice for xenotransplantation of human colon adenocarcinoma for the first time in 1969 and expanded its use to other solid tumors (12). At the end of 1970s, Franks et al. first tried to establish a subcutaneous implantation model of human acute myeloid leukemia (AML), which was the first time that nude mice were used in the study of human hematological malignancies. However, AML cells began to regress after 6 days in mice (13). The complete innate immune system and B cell humoral immune system of nude mice limit the transplantation rate of tumor cells, especially human hematological malignant cells (14). In general, Foxn1-/- nude mice are not suitable to support human hematological malignant cell engraftment because of the residual mouse versus human immunological responses mediated by mouse B cell, natural killer (NK) cell and macrophages.
2.2 C.B17-SCID and Rag1/2 deficient mice
In 1988, C.B17-SCID (C.B17-Prkdcscid, protein kinase DNA activated catalytic polypeptide) mice were generated. The prkdc mutation carried by C.B17-SCID mice prevented the development of T and B cells in the adaptive immune system, resulting in a lack of T and B cells (15). Compared with nude mice, this strain has a higher transplantation rate of human solid tumor cells (16) and was the first immunodeficient mouse that can be used to transplant human HSCs (17) and human lymphoma (18)/acute lymphoblastic leukemia (ALL) cells (19). However, with the increase of age, 2%-23% of functional T and B cells gradually develop in 3-9 months old C.B17-SCID mice. This immune leakiness (20) could inhibit human malignant cell engraftment (20). In addition, the prkdc mutation of C.B17-SCID mice can also lead to DNA repair defects, resulting in radiosensitivity (21, 22).
Similar to C.B17-SCID mice, in 1992, Mombaerts (23) and Shinkai (24) produced C57BL/6 background mice with deletion of the recombination-activating gene 1/2 (RAG1/2)- coding region. This defect prevented the recombination of antigen receptor genes and thus made RAG1/2 deficient mice lacking mature T and B lymphocytes. RAG1/2 deficient mice did not exhibit the leakiness and radiosensitivity of C.B17-SCID mice.
However, the intrinsic immune system activity (including high level of NK cell activity) of C.B17-SCID and Rag1/2-deficient mice reduced the implantation rate of human HSCs and tumor cells; NK cells from C.B17-SCID and Rag1/2-deficient mice show cytotoxicity toward human HSCs and tumor-initiating cells (25),further limiting their application in tumor models.
2.3 NOD-SCID mice
In 1995, NOD-SCID mice based on NOD background were generated. The NOD-SCID mice with NOD background have inherent immune defects, including decreased NK cell activity and lack of complement C5. The Signal regulatory protein α (SIRPA) gene is expressed in myeloid cells and encodes the suppressive immunoglobulin superfamily transmembrane protein CD172a. When combined with CD47, gene acts as a homologous ligand of “don’t eat me” signal to inhibit autoimmune recognition. SIRPA of NOD background mice can cross-react with human CD47 (26), resulting in better tolerance of macrophages to human cells. Compared with C.B17-SCID mice, NOD-SCID mice have a higher implantation efficiency of human HSCs and tumor cells of approximately five times. They could implant hematological malignant cells with lower transplantation rate in the past, including cell lines and primary tumor cells such as lymphoma (22) and leukemia (including AML (26),ALL (27)) cells.
2.4 Il2rg-/- mice
Interleukin-2 receptor γ chain (Il2rg) is responsible for the high affinity binding of IL2, IL4, IL7, IL9, IL15, and IL21 (28). Mice with IL2rg gene knockout (29), due to the complete lack of NK cells (11), further improved the rate of human tumor cell transplantation.
In 2002-2005,NSG (NOD/LtSz SCID,IL2rg-/-)/NOG(NOD/Shi SCID, IL2rg-/-) (30)mice were generated, which are NOD-SCID mice with IL2rg-/-,not only lacking T,B and NK cells but also complement C5,and showing severe immune deficiency. In addition, the NOD background of NSG/NOG can tolerate macrophage phagocytosis caused by incompatibility of CD47-SIRPA, which has advantages in modeling hematological malignancies and solid tumors (31). Except for NSG/NOG, there are other similar immunodeficient mouse models that can be applied to the study of human hematological malignancy in vivo, such as NOJ (NOD-SCID/Jak3null) (32),BRG(Balb/c Rag2-/-IL2rg-/-) (33),SRG(Transgene(Tg)(human SIRPA)Rag2-/-IL2rg-/-) (34),NRG(NOD-Rag2-/-IL2rg-/-,with radiation resistance (35)),NRGS(cross NRG with NSG-SGM3) (36). Considering their significant advantages, NSG and NOG mice are now widely used to study human hematological malignancy in vivo in recent years.
AML, acute myeloid leukemia;PDX, patient derived xenograft;SCID, severe combined immunodeficiency;HSC, hematopoietic stem cell;ALL, acute lymphoblastic leukemia;Rag, recombination-activating gene; NOD, non-obese diabetic; NK, natural killer; SIRPA, Signal regulatory proteinα;NSG,NOD/LtSz SCID,IL2rg-/-;NOG,NOD/Shi SCID,IL2rg-/-;NOJ, NOD-SCID/Jak3null ;BRG, Balb/c Rag2-/-IL2rg-/-;SRG, Transgene(Tg) (human SIRPA)Rag2-/-IL2rg/-;NRG,NOD-Rag2-/-IL2rg-/-;NRGS, cross NRG with NSG-SGM3; IL2rg, interleukin-2 receptor γ-chain;
3 Development of mouse models with human hematological malignancy development
In terms of the manners to achieve human cell engraftment, human hematological malignancy mouse models mainly include human malignant cell xenotransplantation models (cell-derived xenograft (CDX)) model, patient-derived xenograft model (PDX)), virus-induced models, induced pluripotent stem cell (iPSC)-induced models and spontaneous malignancy models (Figure 2). These models have promoted the development of research on the pathogenesis of hematological malignancies and on anti-tumor drugs/therapies.
The xenotransplantation model is defined as the model of transplanting patient cells (PDX) or cell lines (CDX) to immunodeficient mice.
3.1 CDX model
In 1990, the National Cancer Institute (NCI-60) tumor cell line became popular in tumor research. This cell line can be cultured in vitro with a fast growth rate and easy operation (37). To deeply study the physiology of the human blood-lymphatic system and their respective diseases in vivo, the human tumor CDX model was used, which can study the disease mechanism and guide treatment. Since the genotype and phenotype of tumor cell lines are homogeneous and immortal, the CDX model is convenient to study the carcinogenic mechanism, as well as diagnosis and treatment options (38). NOD-SCID or NSG or NOG mice are the strains frequently used to construct human hematological malignancy CDX model. Most CDX models can be constructed by intravenous injection of human cancer cells which may spread over the body as they act in human beings. Whereas MM CDX model requires intra-bone injection to improve cancer cell engraftment rate (39). Compared with PDX model, CDX model is easier to constructed and the stability and successful rate are markedly higher.
However, after transplantation into mice, the tumor cell line lost its original characteristics (40) and could not represent the heterogeneity of clinically complex hematological malignancies (gene expression, growth and invasion characteristics), and the value of predicting clinical prognosis was limited (41, 42).
3.2 PDX model
The PDX model of hematological malignancy was established by transplantation of a patient’s primary hematological malignant cells into immunodeficient mice. Unlike the CDX model, the PDX model preserves the original characteristics, tumor heterogeneity, and growth migration and can well represent the disease progression and treatment response of patients (43). Engraftment rates of PDX models may predict the disease outcome in patients. Since PDX models may retain the features of original tumor, harbor close genetic profiles (44), they can be used to identify the chemo-resistant tumor cell population (45, 46).
NSG and NOG mice are frequently used to construct human hematological malignancy PDX models. Human leukemia cells tend to engraft in mouse bone marrow first and then spread over mouse whole body (47). The organ engraftment tendency of other types of human hematological malignant cell may depend on their original characteristics in patients (48–51). According to disease classification, PDX models of hematological malignancies are divided into the following categories:
3.2.1 Lymphoma
Lymphoma is a common malignant proliferative disease of hematopoietic system, which can affect the whole-body organs (52). Traditionally, lymphoma is divided into Hodgkin’s lymphoma (HL) and non-Hodgkin’s lymphoma (NHL), accounting for approximately 10% and 90% of all lymphomas, respectively (53). The first PDX lymphoma model was developed in 1993 (54). In this model, human HL cells were transplanted into unirradiated CB17.SCID mice kidney/liver. The main advantage of the lymphoma PDX model is that it retains the characteristics of primary lymphoma (55), which summarizes the refractory nature of human lymphoma and non-response to treatment (44), to develop new drug targets and personalized drug treatment (56, 57). In terms of inoculation methods, in addition to intravenous injection, implantation into the renal capsule or intraperitoneal cavity has a higher success rate (48). However, it is necessary to pay attention to complications such as bleeding caused by kidney injury (58).
3.2.2 Leukemia
Leukemia is a malignant clonal disease of HSCs (59). Because of uncontrolled proliferation, impaired differentiation, and blocked apoptosis, clonal leukemia cells proliferate and accumulate in hematopoietic tissues, infiltrate other non-hematopoietic tissues and organs, and inhibit hematopoietic function. Similar to the PDX model of lymphoma, the PDX model of leukemia retains its immunophenotype, chromosome aberration, transcriptome and MRD marker expression (60, 61);The onset time of leukemia cells in mice is inversely proportional to the recurrence rate in humans; Moreover, the transplantation of leukemia stem cells (LSCs) can represent the development of leukemia (62, 63), and its efficiency reflects the prognosis of clinical patients (64). Using the LSC PDX model, researchers found genes or subclones related to drug resistance and relapse in patients with cytogenetic remission (65, 66), such as CML (67) and pediatric T cell acute lymphoblastic leukemia (T-ALL) (45). In terms of inoculation method, intravenous implantation can form a leukemia model with systemic spread, which is consistent with the clinical progress of leukemia (68); direct injection into the marrow cavity bypasses the homing process of cells and provides a bone marrow microenvironment suitable for the growth of leukemia cells, with a higher implantation rate (69).
3.2.3 MM
MM is a monoclonal malignant blood tumor characterized by abnormal proliferation of plasma cells in the blood, accompanied by anemia, hypercalcemia, renal failure and osteolytic injury. Owing to its frequent recurrence and drug resistance, the cure rate is extremely low. The MM PDX model can well represent the development process of disease and develop new treatment methods. In the past, mobilized blood mononuclear cells or CD34+-enriched cells from advanced MM patients could develop into myeloma in irradiated NOD-SCID mice by intracardiac injection, which allows injected cells to bypass lung and flow directly into bone marrow (70). At present, MM PDX is mainly constructed by injecting primary myeloma cells (MC) intravenously or intra-osseously (39). Intravenous models are easy to operate and can represent the characteristics of “diffused MM syndrome”; while intratibial models are often limited in MC growth and may not model diffused diseases. In addition, MM PDX model constructed by subcutaneous injection manner is utilized to study refractory and recurrent human MM which may own extramedullary effusion feature (71, 72). However, patient-derived MCs in the MM PDX model can grow and colonize in the bone marrow of mice but often do not have bone disease (73). Research on the efficacy of drugs in treating MM-induced bone disease is extremely limited. At this time, the MM CDX model that leads to osteolytic injury is often selected to better study the anti-MM bone disease therapy.
3.2.4 MDS
MDS are a group of heterogeneous diseases of HSCs, characterized by repeated genetic abnormalities (74) resulting in the reduction of the number of hematopoietic cells and morphological dysplasia, which are divided into high-risk MDS and low-risk MDS according to the degree of malignancy. Contrary to AML, research on MDS is hindered by the lack of a preclinical model that replicates the complexity and heterogeneity of the disease (75). The MDS CDX model that summarizes the MDS bone marrow failure status is rare and has limited genetic diversity (76). The MDS PDX model is constructed from MDS stem cells from patients (77), which reproduces human myeloid differentiation rather than lymphoid differentiation (78). By implanting high-risk MDS cells into the PDX model (79), researchers can study whether there are other abnormalities in model-derived bone marrow stem cells (80). However, the success rate of implanting low-risk MDS in NSG mice is still low, which needs to be further resolved (81).
In summary, the PDX model, as a pre-clinical bridge for potential clinical application, is used to guide treatment (82, 83);PDX can simultaneously transplant patient effector cells to evaluate individual efficiency (84) and maintain important characteristics of the original tumor, including histology, genome pattern, cell heterogeneity and drug responsive (85), cell-cell interaction (86, 87), and clonal diversity (88). In addition, through the PDX model constructed by transplanting drug-resistant primary human hematological malignant cells, researchers can determine the drug resistance mechanism or molecular target (43, 89).
3.3 iPSC-induced PDX model
The above PDX/CDX models have difficulties in building some hematological malignancy models with low invasion (such as CML), lack of cell lines (such as MDS) or insufficient donor samples. The iPSC-induced PDX model can differentiate patients’ iPSCs into HSPC that regain the ability to cause hematological malignancies in vivo (90). IPSC drug testing system (82) can be used to test the potential treatment methods to reproduce the disease DNA methylation/gene expression pattern and drug resistance (91, 92). In theory, iPSC-induced HSPC can be repeatedly used to build unlimited mouse models based on limited original samples and solve the different copy number changes of PDX models and patients (93, 94). Human CD34+ cells were isolated from primary patient bone marrow mononuclear cells cultured in cytokines, and reprogramming factors were transduced to obtain stable iPSC clones. Then, iPSC could be differentiated into HSCs in vitro (95) and transplanted into immunodeficient mice to construct the PDX model. Alternatively, iPSCs can be co-injected with OP9 stromal cells into immunodeficient mice where human iPSCs develop into HSCs; and those HSCs can be used for secondary transplantation for PDX model generation (91). At present, patient-derived chronic myelomonocytic leukemia iPSCs, juvenile myelomonocytic leukemia iPSCs (96), AML iPSCs and MDS iPSCs have successfully differentiated into HSCs and PDX models in NSG mice have been built. iPSCs derived from AML patients with MLL rearrangement (97) and MDS-iPSC differentiated HSCs (98) can be used to build PDX models in NSG mice and allow the study of characteristics, drug sensitivity, and prediction of relapse of different subclones. However, CML iPSCs with BCR-ABL gene (99) and acute B lymphoblastic leukemia (B-ALL) with MLL rearrangement (100) failed to differentiate into specific HSC, which needs further study. Some teams further glycoengineered HSCs derived from MDS iPSCs and transplanted these cells into NSG mice, obtaining strong progenitor cell activity, bone marrow transport, and exosmosis, but not long-term implantation (101).
3.4 Virus induced model
Virus infection often induces hematological malignancies, such as Epstein-Barr virus (EBV)-induced lymphoma, Human T-lymphotropic virus (HTLV) -1-induced ATL (adult T cell leukemia), EBV-induced aggressive NK-cell leukemia. Nearly 50% of HL is induced by EBV (102). The mouse model with human immune system can represent human hematological malignancy induced by a virus interference genome or non-specific activation. Injection of patient HTLV-1 virus infected human PBMCs into NSG mice resulted in T cell clonal proliferation and development of ATL (103); BLT (bone marrow-liver-thymus) humanized mice can also be used to study HTLV-1-induced-ATL (104);The humanized mouse model of lymphoma can be made by infection of EBV in human CD34+ HSCs transplanted newly born immunodeficient mice (C.B-17-SCID (105), NOD-SCID (106), NOG (107), NSG (108)). These models reshape the pathogenesis and mechanism of human lymphoma in vivo, and replicate the potential infection, T-cell-mediated immune response and humoral immune response in vivo (109, 110). Different conditions (e.g. mouse strain/age, donor cells, viral dosage and so on) may induce lymphoma into different phenotypes. When infected with EBV, the humanized mice with high numbers of human B cells trend to generate, while HL mainly occur in the humanized mice reconstituted with high levels of human T-cells (111). However, the humanized mouse model of lymphoma induced by EBV has differences in the mode of virus transmission. Oral transmission of humanized mice is not feasible because the oropharyngeal epithelial cells of humanized mice are derived from mice. Whereas human lymphoma is mainly transmitted through oral infection, which leads to the heterogeneous immune response of human and mouse. The humanized mouse model of lymphoma made by co-infection of EBV/Kaposi’s sarcoma-associated herpesvirus (KSHV) virus (112) resulted in long term viral infection in humanized NSG mice that representing lymphoma (113) of PEL (primary effusion lymphoma) type. The main manifestations were viral infection in the spleen and abdominal cavity, and the lymphoma showed plasma cell differentiation signs.
3.5 Spontaneous malignancy model
In addition to the models mentioned above, the mouse model with human hematological malignancy natural development can be made by genetic engineering modification of the target genes causing hematological malignancy in human HSCs before transplantation into immunodeficient mice. This spontaneous human malignancy mouse model shows similar performance and immune phenotype to patients with the same gene rearrangement, providing a better understanding of the molecular etiology of specific subtypes (Table 1). Based on its specific genetic abnormality, it provides the direction for potential treatment. There are many methods of HSC gene editing, including retroviral transduction, lentivirus transduction, and Clustered Regularly Interspaced Short Palindromic Repeats/Cas9 (CRISPR/Cas9) engineering transduction, which have their own characteristics in inducing hematological malignancy (Table 1). The solid tumor model requires at least three different carcinogenic genes to transform primary human cells (124, 125),and the progress is slow. Unlike the solid tumor spontaneous model, the hematological malignancy spontaneous model can quickly obtain the required additional genetic or epigenetic events, and only one or two genes are needed; For example, Lin-(lineage negative) and CD34+ human UCB (umbilical cord blood) cells transfected by MLL-AF9, MLL-ENL in NOD-SCID (β2M-/-) show pre-B-ALL and AML and mixed pedigree (114, 115);Transfection of CD34+ human HSCs with the retroviral vector containing ZYM2-FGFR1 promotes the development of MPD to AML (120) and activates the STAT signal pathway in NSG mice after transplantation, which is consistent with the development of human primary diseases. Human UCB-Lin- cells transfected with TEL-JAK2 engrafted into NOD-SCID mice showed that the graft tilted toward the myeloid and erythroid lineages and induced bone marrow fibrosis (123). Some co-expression methods further promote model construction and guide treatment, such as MLL-AF9 and NRAS co-transfection of human UCB-CD34+ cells induced faster AML progression in NSG mice (121); BCR-ABL and BMI1 co-transfected with human UCB-CD34+ cells induced B-ALL in NOD-SCID mice confirmed that BMI1 was a potential therapeutic target for CML (118);Human CD34+ cells transfected by DEK-NUP214 induced AML in NSG mice, demonstrating that the HOX family and the primary human t (6; 9) AML were highly up-regulated and became a potential target for treatment (119); Unlike conventional Notch mutation induced T-ALL mouse model (126), overexpression of Notch1 in human HSCs only resulted in alteration of human T cell differentiation tendency in NOG mice, while only 1/26 transplanted mice were found with T-ALL development (127) (Table 1, Figure 3).
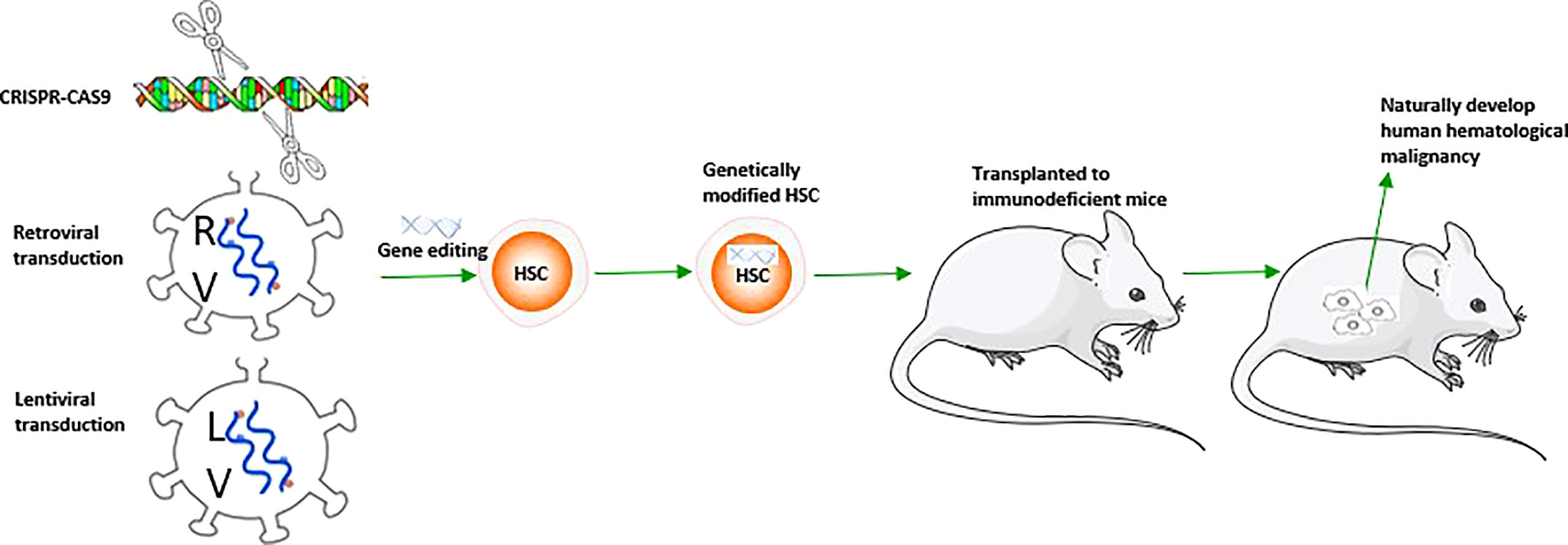
Figure 3 A schematic figure about the construction of mouse model with spontaneous human hematological malignancy development.
4 Optimization of human hematological malignancy mouse models
Other than immunological factors, non-immunological factors may also constrain the recapitulation of human malignancy in mice. Many efforts were input to optimize human hematological malignancy mouse models from this aspect in the last two decades (128). The microenvironment of hematological malignancies is composed of the bone marrow, blood vessels, and peripheral lymphoid organs providing nutrition and survival factors, which can affect the survival and growth of tumor cells. The Bone marrow microenvironment includes HSC and non-hematopoietic cells, and the latter includes endothelial cells, fibroblasts, osteoblasts, macrophages, mast cells and mesenchymal stem cells (MSCs). Because mouse bone marrow microenvironment is different from the human counterpart, it may not support the development of some less-invasive human hematological malignancies. The application of human cytokines and reconstruction of the bone marrow microenvironment further optimized the mouse model to reproduce the human microenvironment and address the limitations caused by differences between human and mouse microenvironments.
4.1 Application of human cytokine transgenic mice
The differences in amino acid sequence of cytokines/ligands between species lead to the difference in cross-reaction between humans and mice, which may affect the survival and development of human hematopoietic cells in mice. Human cytokine transgenic mice can promote hematopoietic differentiation of human myeloid cells and other cells in mice, improve the development of human hematopoietic immune system in mouse models, and increase the implantation rate of human hematological malignant cells (Table 2).
NSG-SGM3: AML and other myelopoietic hematological malignancies are difficult to transplant into mice, which may be due to the lack of hematopoietic microenvironment and cytokines supporting homing, expansion and stromal cell growth of myelopoietic hematological malignant cells in mice. NSG-SGM3 transgenic mice with human stem cell factor(SCF), granulocyte-macrophage colony stimulating factor(GM‐CSF), and interleukin (IL)‐3 (121) promote the research of human myelopoietic hematological malignancy mouse models by increasing the production of mature myeloid cells (139) and enhancing the implantation rate of AML (129),MDS (140), and CLL (141). In terms of AML, some with difficulty in migration include those with AML1-ETO and CBFβ-MYH11 AML-LSC cells that can be successfully implanted in NSG-SGM3 mice. After implantation, the level of human CD45+/CD33+myeloid cells were significantly higher than that of NSG, and the transplanted cells expand with time, providing research opportunities for diseases that are difficult to model in mice.
MISTRG: Rongvaux et al (142) knocked in human cytokines macrophage colony stimulating factor (M-CSF), IL-3, granulocyte-macrophage colony stimulating factor (GM-CSF), and thrombopoietin (TPO) into RAG-/-IL2rg-/- mice, replacing the corresponding cytokine epitopes of mice, and human SIRPA genes were knocked in at the same time to produce MISTRG mice. The lack of corresponding cytokines in mice reduces the affinity of the niche in the bone marrow to mouse HSPCs, which reasonably provides additional benefits for human hematopoiesis. MISTRG mice support the development of human bone marrow mononuclear cells, NK cells and CD163+ tumor infiltrating macrophages. MDS stem cells cannot produce colony forming units in vitro, and their proliferation and differentiation abilities are defective. MISTRG mice can implant hematological malignant cells of different risk levels, including MDS, and maintain the genetic complexity of the original samples of hematological malignancies. MISTRG mice can be transplanted twice and are superior to NSG mice in terms of implantation rate and construction percentage of myeloid system (135). For MISTRG mice, the MDS implantation rate can reach 50%, which is significantly better than 10% of NSG. They can reproduce erythrocyte and megakaryocyte dysplasia in MDS, forming circular fibroblasts and dysplastic megakaryocytes with reticulin fibrosis.
IL-6-tg: IL-6 is the key growth factor of human MM, and human and mouse IL-6 lack cross-reactivity. Human IL-6 transgenic mice (136) improved the implantation rate of MM, provided the niche key factor for MM growth in mice, and allowed the implantation of precancerous, malignant plasma cells and non-malignant cells, promoting the study of myoid-derived suppressor cells in MM immune mechanism.
TPO-tg: TPO has been proven to be a key cytokine supporting HSC maintenance and self-renewal and can promote the development of megakaryocytes and platelets. The development of bone marrow mononuclear cells in immunodeficient mice, such as NSG, is relatively small, and the implantation level usually begins to decline 4-6 months after transplantation, which may be due to the difference of TPO between humans and mice. Human TPO transgenic mice promoted the development of human bone marrow mononuclear cells (137) and CD66+ granulocytes, and may improve the transplantation rate of leukemia with specific subtypes such as t(8; 21)-ETO AML (138).
4.2 Bone marrow microenvironment optimization and reconstruction
In addition to human hematopoietic system cytokines, other complex factors (such as ligands, chemokines, and hormones) in the bone marrow microenvironment also significantly affect the occurrence of hematological malignancies. For example, the exogenous cytokine c-kit ligand can improve the implantation of normal and leukemia CP-CML (chronic phase of chronic myeloid leukemia) cells (143); SCF tg improves the implantation of irradiated NSG HSCs and eliminates radiation-related complications (131, 132),SCF/KIT ligand tg improves the development of human granulocyte lineage and human myeloid lineage (132). Therefore, remolding the mouse bone marrow microenvironment or reconstructing artificial bone marrow tissue is another important way to optimize the mouse model of human hematological malignancy.
4.3 Combined injection of MSC
MSC creates a favorable environment for human MDS-derived cells to survive in the mouse microenvironment, possibly through the physical interaction itself and the production of human cytokines (144). Co-injection of patient hematological malignant cells with MSCs can promote the successful construction of PDX mouse model (66), and successfully build a hematological malignancy model that is difficult to implant, such as MDS. The majority of MSCs used are collected from healthy people, while some MSCs were established from patients’ CD34− cell fraction (66). Injecting genetically modified MSCs platelet-derived growth factor subunit B, human IL-3 and TPO, into the tibia can further promote the survival, renewal and reduction of clone drift of HSCs (145), including the secondary transplantation (146). However, the presence of non-bone marrow-derived stromal cells (dermal fibroblasts) or non-stroma cells is not conducive to the implantation of MDS-derived cells. Also, for leukemia, co-injection of MSC and AML cells improve engraft rate, helping to evaluate key factors in the progression of leukemia in bone marrow niche (147, 148).
4.4 Reconstruction of artificial bone marrow microenvironment
Humanized bone marrow-like structures, including 3D scaffold, ossicles, and rabbit bone, can not only create artificial bone marrow microenvironment to better implant hematological malignant cells but also be used to screen for drugs, especially those targeting matrix components.
The “three-dimensional (3D) scaffold” coated with MSCs can be adjusted to form humanized bone tissue, which can also provide niche for implantation and homing of human UCB-CD34+ HSCs and the growth of cells in patients with hematological malignancies in vivo (149). The scaffold model retains the original clone structure. The flexible nature of the biocompatible cell carrier helps achieve the required size by simple cutting. Simultaneously, it also allows the use of collagenase for effective digestion, facilitating access to cells for further research. Usually, MSCs expanded in vitro are inoculated in gelatin sponge and cultured for several days, and then human hematological malignant cells are injected into the sponge, and then subcutaneously injected into non-irradiated immunodeficient mice (149), which is an effective in vivo model for studying the human hematopoiesis niche (150). In one study, MDS was only observed in mice co-injected with patient-derived MSCs and CD34+ cell bone (151), of which the regeneration rate of the method based on 3D bone was the highest (152);
“ Ossicles “ refers that bone marrow-MSC mixed with matrigel and subcutaneously injected into immunodeficient mice. After 2-3 months, they form “ossicle body”, which forms the external bone structure around the hematopoietic core (153), which also provide site for malignant and normal HSC to home and improve their engraftment and differentiation. Pievani e tal (154) created leukemia microenvironment using patient-derived MSCs in vivo and found MSCs improve the leukemia engraftment; Implantation of cartilage pellets followed by MSCs subcutaneous transplantation into NSG mice, bone marrow-stromal progenitors from AML mice have an increased adipogenic differentiation ability (155). The implantation of primary AML cells can be successfully achieved by transplanting subcutaneously injected polyurethane scaffolds, ceramic scaffolds or matrix gel coated with freshly separated human bone marrow-derived MSCs in vivo (156), which proves that tumor cells or transplanted AML cells rich in CD34+ can circulate between ossicles.
For MM, there are already advanced SCID mice models with artificial humanized bone marrow microenvironment such as SCID-hu (human fetal bone) (157),SCID-rab (rabbit bone) (158), SCID-synth-hu (the 3D polymeric scaffold previously composed with human bone marrow stromal cells) (159). SCID-rab uses rabbit bone (158) to avoid the ethical problem of human fetal bone caused by SCID-hu and uses rabbit bone subcutaneously implanted in mice. Immunohistochemical analysis shows that most bone marrow microenvironment cells originate from rabbits. Direct injection of MCs from 28 patients into the implanted bone can successfully implant tumor cells from 85% of patients with MM and lead to the production of patients’ M protein isoforms and typical myeloma manifestations. MCs only grow in rabbit bones, but can transferred to another bone in the same mouse at a distance; Cells from patients with extramedullary diseases also grow along the outer surface of the rabbit bone. The system can now be widely used to study the biology and expression of myeloma and to develop new treatment methods for the disease.
Others: Denervation of bone marrow in recipient mice by using 6-hydroxydopamine can also improve the implantation rate of AML by changing the bone marrow microenvironment (160); NHL tumor microenvironment (161) is maintained by implanting BCL2 + and CD20+ lymphocytes (48).
5 Humanized mouse models with human immune cell composition and human hematological malignancy development for cancer immunotherapy study
Immunotherapy is a powerful tool to treat a variety of human hematological malignancy clinically. Immunotherapy for hematological malignancies includes non-myeloablative transplantation, T-cell immunotherapy, NK cells, immune-agonists, monoclonal antibodies (mAb) and vaccination (1). The history of immunotherapy for hematological malignancies began with mAb therapy in B-cell lymphoma (162), and then transited to gene-modified T cell therapy. These T cells were designed to recognize and target specific antigens, such as anti-CD19 Chimeric Antigen Receptor (CAR)-T cell therapy, and produced significant results in patients with B-cell malignant tumors (163). The advantage of immunotherapy is that it reproduces the microenvironment of immune cells and improves the overall survival rate of patients. The Food and Drug Administration (FDA) has studied and approved several immunotherapy programs for hematological malignancies, but many programs are still in the late stage of clinical development, including adoptive cell transfer, antibody-based therapies, and immune checkpoint inhibitors (1). In addition, patients with hematological malignancies who receive immunotherapy, especially CAR-T therapy, will also relapse (164), and have toxic reactions (165). The mechanisms include memory response and the source of immune cytokines, and many patients cannot benefit from current immunotherapy. To sum up, to minimize the risk of clinical trials, better animal models in vivo are needed for further research (166) to develop new immunotherapy schemes and prolong the survival period of patients. The early human hematological malignancy mouse model of immunotherapy was directly infused with corresponding immunotherapy cells, such as CAR-T, on the basis of CDX/PDX to verify the curative effect (167). However, they rely on the immune system of mice and cannot represent the real human immune therapy response. Therefore, there is an urgent need for a mouse model of the human functional immune system to reproduce the immune surveillance/immune regulation of humans to further study the complex response of immunotherapy in the human body.
The mouse model with human functional immune system focuses on the study of human immune surveillance, immune regulation (84) and pro-inflammatory cytokines, which can verify the immunotherapy of various hematological malignancies, including immune-cytokine therapy (168,)bispecific antibody (169), mAb (170), and CAR-T therapy (171). At present, the humanized mouse models applied to hematological malignancy mainly include Hu-PBL (human PBMC), Hu-SRC (SCID repopulating cell) and Thy/HSC models (also known as BLT models) (10).
Hu-PBL model was a humanized PDX/CDX model constructed by transplanting human PBMCs, which were easy to process and obtain, and by inoculating hematological malignant cells. In this model, human lymphocytes were rapidly expanded in mice, which can be used to study the interaction between human immune cells and hematological malignancies (172). However, because the injected cells are human mature immune cells, human myeloid cells and B lymphocytes are rarely detected in this model. Activated human CD4+or CD8+ T cells in mice often leads to severe graft-versus-host disease (GVHD), which markedly limits the observation period and making its wide application difficult.
Another popular humanized mouse (173) model is Hu-SRC model. It was constructed by transplanting human cord blood-, fetal liver-, bone marrow-or G-CSF-mobilized peripheral blood-derived CD34+ cells into irradiated neonatal immunodeficient mice. This model has sustainable multilineage human immune cell (including T cells, B cells and myeloid cells) reconstitution. This model can reproduce the primary immune reaction of T cells in humans. Among them, human T cells develop in the mouse thymus, and after positive and negative selection, this model has mouse MHC restriction (174). However, residual innate immunity, poor human thymopoiesis, immature B cells, lack of human leukocyte antigen (HLA) restrictions, and poor T cell-dependent humoral responses also exist.
Thy/HSC model (also known as BLT model) (90) is generated by co-transplantation of human fetal liver CD34+ cells (intravenous injection) and thymus tissue (under renal capsule) into sublethal-total-body-irradiated immunodeficient mice. This model has high levels of human immune system reconstruction and secondary lymphoid organ well development. HSCs extracted from human liver tissue have more efficient transgenic expression. The Thy/HSC model can generate specific T cell and antibody responses against protein antigens, virus antigens, autoantigens, allogeneic and xenogeneic cell antigens, which proves that it can generate strong human immune responses. Transplanted human liver and thymus provide a microenvironment that supports the development of human T cells. Hassall’s corpuscles formed by human stromal cells and T progenitor cells can be detected, which can produce a human HLA-limited human T cell immune response. Mouse DCs can migrate to the human thymus and induce human T progenitor cells, reducing xenograft rejection.
Application of humanized mice in the immunotherapy of hematological malignancies: mainly embodied in the verification and exploration of immune checkpoint inhibitors, cytokine therapy, and cell therapy, such as CAR-T. (Table 3)
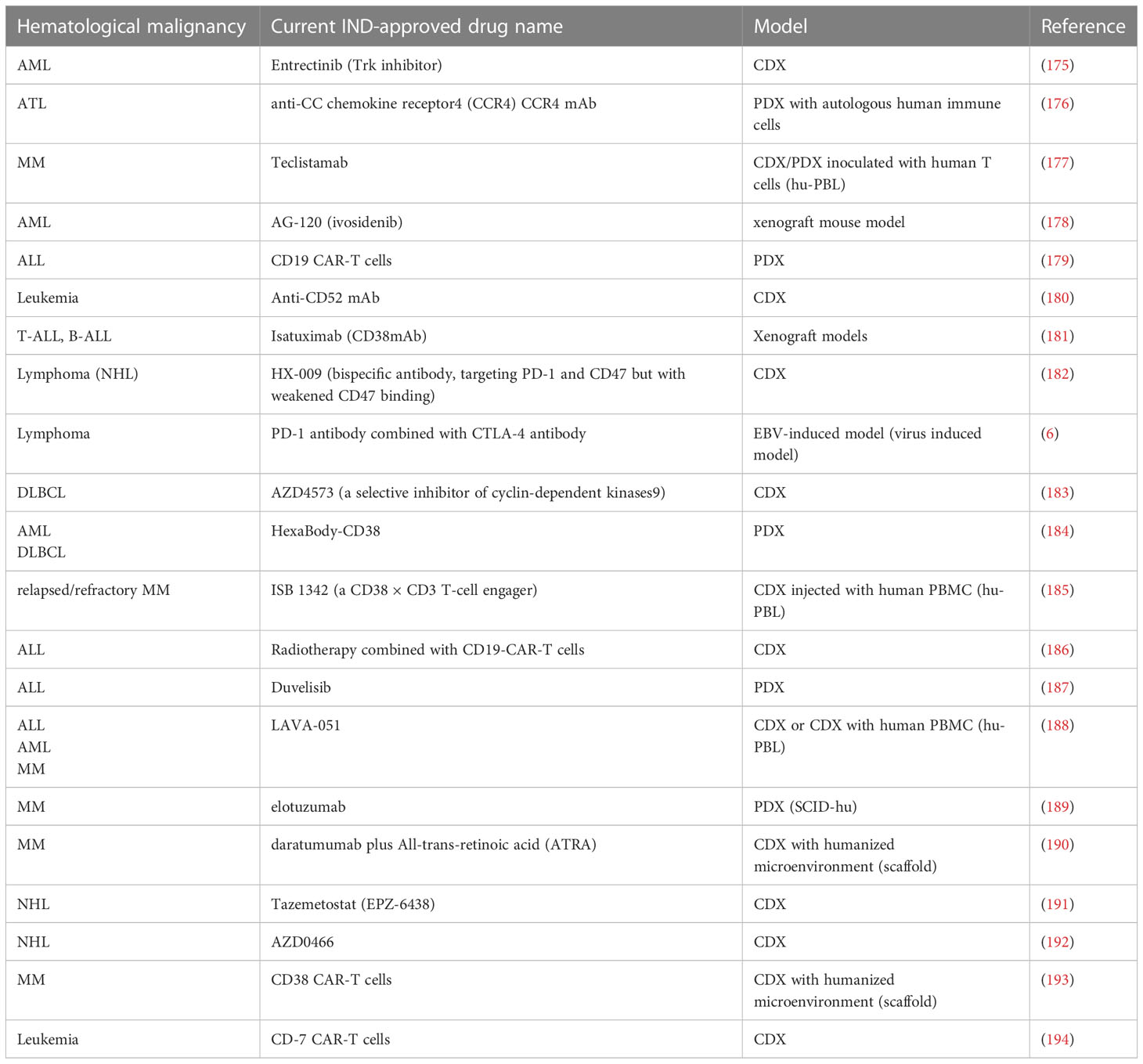
Table 3 Part of Investigational New Drug (IND)-approved drugs that utilize mouse models with human hematological malignancy for pre-clinical tests between 2020-2023.
Immune checkpoint inhibitors: The application of immune checkpoint inhibitors in hematological malignancies mainly includes CD137 antibody, programmed cell death protein-1 (PD-1) antibody, or cytolytic T lymphocyte-associated antigen (CTLA)-4 antibody, and their effect can be verified in humanized mouse models, with either single therapy or combined therapy for different types of tumors. The efficacy of anti-CD137 antibody was tested in MM CDX humanized mice models with inoculation of human healthy NK cells (hu-PBL) (195). The effect of PD-1 and CTLA-4 antibodies were evaluated in EBV induced B cell lymphoma humanized mouse models (196) before clinical trial. The human immune cells of humanized mice can mediate the immune response against therapeutic antibodies. For example, the effectiveness of bi-specific CD20/CD3 antibody can also be verified in a lymphoma CDX hu-PBL humanized mice model (169). However, these humanized mouse models have different immune cells from human donors, which cannot completely replicate the immune response in patients with tumors.
For cytokine therapy, humanized mice with cytokines can reproduce the immune response in patients during immunotherapy. For example, human IL-2 transgenic mice (197) produce various NK cells, which can reproduce human NK cells targeted to implanted leukemia and lymphoma cells (198);Human IL-15 knock-in (199, 200) humanized mice better mimic human NK (201)and CD8+T cells that remain in human circulation and tissue to target Burkitt lymphoma, as well as ADCC effect on CD20 antibody (68, 133).
For cell therapy, humanized mice can represent its immune effect and toxicity in humans in vivo. Recently Caruso et al. described a humanized mice model system constructed with human HSCs, AML cells and human endothelial tissues to evaluate the on target-off tumor toxicity of CAR.CD123-NK cells. This is a new model for studying the off-tumor toxicity of immunotherapy for hematological malignancy, especially for relapsed/refractory pediatric AML (202). Additionally, humanized mice can reproduce cytokine release syndrome (CRS) and neurotoxicity in patients after infusion of CAR-T cells. For example, Mhaidly et al (203) experimented in NSG mice with CD19+human B-lymphoblastic leukemia, delivering anti-CD19 CAR by CD8 targeting lentivirus and injecting human PBMC (204). Compared to the control, a single injection was sufficient to eliminate tumor cells. However, its CAR-T cells came from allogeneic and HLA donors (205). Although the occurrence of CRS was the same as that of CAR-T patients (206), CRS could not be distinguished from allogeneic reactions. Therefore, it is necessary to verify the humanized spontaneous model of human hematological malignancy by monitoring auto-functional human immunity.
The humanized mouse model with homologous immune system and spontaneous human leukemia development is a novel mouse model recently developed by our group for human hematological malignancy investigation. It is mainly based on the Thy/Liv SCID-Hu mouse model (207). The model was constructed by transplanting CD34+cells from human fetal liver and human fetal thymus from the same embryo donor into NSG mice with sublethal radiation. CD34+ cells were transduced by a retrovirus carrying the MLL-AF9 fusion gene and GFP. About 10-12 weeks after humanization, human immune cells were reconstructed, human CD3+ T cells from spleen were isolated, transferred with a virus vector encoding human CD19 specific CAR, expanded in vitro for about 2 weeks, and then returned to leukemic humanized mice with adoptive immunotherapy (208, 209). In most previous studies, the reconstructed human immune system and inoculated human tumors are allogeneic. The allogeneic response of human T cells to malignant tumors may damage the value of data collected from these models, making it difficult to predict the clinical immune effects of anticancer drugs (10). While this model allows the evaluation of anti-tumor response in “immune-active” hosts without allogeneic or xenogeneic immune response. CAR-T cells are modified autologous T cells developing in human thymus. In mouse blood, we can detect anti-CD19 CAR-T cells, whose dynamics and levels are similar to those of patients receiving CAR-T, and are closely related to the burden of leukemia, B-cell destruction and mouse survival, which is helpful to identify new and more effective CARs (210), and inhibit side effects, such as CRS and neurotoxicity. In this model, human immune cells and leukemia cells were derived from CD34+ HSCs from the same human fetal liver. These cells are considered autologous and have a homologous immune monitoring system. Even the CAR-T produced by humanized mice will not attack mice, nor will it cause strong xenograft rejection. The humanized mouse model of B-ALL induced by MLL-AF9 can also verify the treatment of receptor leukocyte infusion (RLI) without GVHD (211),which has a similar immune response to patients. For instance, increase in the proportion of cytokines produced by T cells and mononuclear macrophages and Treg reveals that GM-CSF may play a key role in CRS after CAR-T cell treatment; and RLI can improve the anti-tumor efficiency by depletion of human T cells (211). In addition, Leskov et al. developed a “double-hit” lymphoma model and constructed a humanized mouse model of lymphoma with the human immune system by inducing joint overexpression of c-MYC and BCL2 in human HSC-derived B-lineage cells through lentivirus transduction. This model accurately summarizes the histopathological and clinical features of human “double-hit” lymphoma resistant to steroids, chemotherapy and rituximab (212), and can evaluate immunotherapy.
6 Discussion and summary
Advancement in mouse models with human hematological malignancy recapitulation intensively contributes to exploring the pathological mechanism of related human diseases and facilitates invention of effective drugs and therapeutic approaches in last two decades. Development of mouse strains with severe deficiency of murine immune system (such as NSG mice) almost completely prevent mouse versus human immunological rejection after human cell/tissue transplantation and markedly elevate the successful rates of CDX and PDX model construction. Whereas non-immunological factors still constrain the capability of immunodeficient mice to accept and repopulate less aggressive human hematological malignant cells, especially the ones closely rely on human specific microenvironment to survive and differentiate. Thus, development of immunodeficient mouse strains with delicate human cytokine/chemokine/ligand expression or reconstruction of artificial human bone marrow microenvironment would further promote their application in human blood disease study. Importantly, proper combination of humanized mouse model with functional human immune system and the mouse model with human hematological malignancy development may further generate a powerful tool to precisely evaluate the efficacy of new drugs or immunotherapies and speed up their translational paces in clinic.
Author contributions
YL and YNL performed literature search and wrote the manuscript. YT and ZH conceived the framework of this article, wrote, and edited the manuscript. All authors contributed to the article and approved the submitted version.
Funding
This work was supported by grants from NFSC (82241224) and Jilin Provincial Science and Technology Development Plan Project (20230505029ZP; 20190201005JC).
Conflict of interest
The authors declare that the research was conducted in the absence of any commercial or financial relationships that could be construed as a potential conflict of interest.
Publisher’s note
All claims expressed in this article are solely those of the authors and do not necessarily represent those of their affiliated organizations, or those of the publisher, the editors and the reviewers. Any product that may be evaluated in this article, or claim that may be made by its manufacturer, is not guaranteed or endorsed by the publisher.
References
1. Tawfik EA, Aldrak NA, Albrahim SH, Alzahrani DA, Alfassam HA, Alkoblan SM, et al. Immunotherapy in hematological malignancies: recent advances and open questions. Immunotherapy (2021) 13:1215–29. doi: 10.2217/imt-2021-0065
2. Haas S, Trumpp A, Milsom MD. Causes and consequences of hematopoietic stem cell heterogeneity. Cell Stem Cell (2018) 22:627–38. doi: 10.1016/j.stem.2018.04.003
3. Liggett LA, Sankaran VG. Unraveling hematopoiesis through the lens of genomics. Cell (2020) 182:1384–400. doi: 10.1016/j.cell.2020.08.030
4. Siegel RL, Miller KD, Jemal A. Cancer statistics, 2015. CA Cancer J Clin (2015) 65:5–29. doi: 10.3322/caac.21254
5. Mattiuzzi C, Lippi G. Current cancer epidemiology. J Epidemiol Glob Health (2019) 9:217–22. doi: 10.2991/jegh.k.191008.001
6. Alaggio R, Amador C, Anagnostopoulos I, Attygalle AD, Araujo IB, Berti E, et al. The 5th edition of the world health organization classification of haematolymphoid tumours: Lymphoid neoplasms. Leukemia (2022) 36:1720–48. doi: 10.1038/s41375-022-01620-2
7. Kantarjian HM, Jain N, Garcia-Manero G, Welch MA, Ravandi F, Wierda WG, et al. The cure of leukemia through the optimist's prism. Cancer (2022) 128:240–59. doi: 10.1002/cncr.33933
8. Chen DS, Mellman I. Elements of cancer immunity and the cancer-immune set point. Nature (2017) 541:321–30. doi: 10.1038/nature21349
9. Perrin S. Preclinical research: Make mouse studies work. Nature (2014) 507:423–5. doi: 10.1038/507423a
10. De La Rochere P, Guil-Luna S, Decaudin D, Azar G, Sidhu SS, Piaggio E, et al. Humanized mice for the study of immuno-oncology. Trends Immunol (2018) 39:748–63. doi: 10.1016/j.it.2018.07.001
11. Shultz LD, Ishikawa F, Greiner DL. Humanized mice in translational biomedical research. Nat Rev Immunol (2007) 7:118–30. doi: 10.1038/nri2017
12. Rygaard J, Povsen CO. Heterotransplantation of a human Malignant tumour to "nude" mice. APMIS (1969) 115:604–606; discussion 607-608. doi: 10.1111/j.1600-0463.2007.apm_689a.x
13. Franks CR, Bishop D, Balkwill FR, Oliver RT, Spector WG. Growth of acute myeloid leukaemia as discrete subcutaneous tumours in immune-deprived mice. Br J Cancer (1977) 35:697–700. doi: 10.1038/bjc.1977.107
14. Shultz LD, Goodwin N, Ishikawa F, Hosur V, Lyons BL, Greiner DL, et al. Human cancer growth and therapy in immunodeficient mouse models. Cold Spring Harb Protoc (2014) 2014:694–708. doi: 10.1101/pdb.top073585
15. Bosma GC, Custer RP, Bosma MJ. A severe combined immunodeficiency mutation in the mouse. Nature (1983) 301:527–30. doi: 10.1038/301527a0
16. Phillips RA, Jewett MA, Gallie BL. Growth of human tumors in immune-deficient scid mice and nude mice. Curr Top Microbiol Immunol (1989) 152:259–63. doi: 10.1007/978-3-642-74974-2_31
17. Kamel-Reid S, Dick JE. Engraftment of immune-deficient mice with human hematopoietic stem cells. Science (1988) 242:1706–9. doi: 10.1126/science.2904703
18. Flavell DJ. Modelling human leukemia and lymphoma in severe combined immunodeficient (SCID) mice: practical applications. Hematol Oncol (1996) 14:67–82. doi: 10.1002/(SICI)1099-1069(199606)14:2<67::AID-HON567>3.0.CO;2-U
19. Kamel-Reid S, Letarte M, Sirard C, Doedens M, Grunberger T, Fulop G, et al. A model of human acute lymphoblastic leukemia in immune-deficient SCID mice. Science (1989) 246:1597–600. doi: 10.1126/science.2595371
20. Bosma GC, Fried M, Custer RP, Carroll A, Gibson DM, Bosma MJ. Evidence of functional lymphocytes in some (leaky) scid mice. J Exp Med (1988) 167:1016–33. doi: 10.1084/jem.167.3.1016
21. Fulop GM, Phillips RA. The scid mutation in mice causes a general defect in DNA repair. Nature (1990) 347:479–82. doi: 10.1038/347479a0
22. Hudson WA, Li Q, Le C, Kersey JH. Xenotransplantation of human lymphoid Malignancies is optimized in mice with multiple immunologic defects. Leukemia (1998) 12:2029–33. doi: 10.1038/sj.leu.2401236
23. Mombaerts P, Iacomini J, Johnson RS, Herrup K, Tonegawa S, Papaioannou VE. RAG-1-deficient mice have no mature b and t lymphocytes. Cell (1992) 68:869–77. doi: 10.1016/0092-8674(92)90030-g
24. Shinkai Y, Rathbun G, Lam KP, Oltz EM, Stewart V, Mendelsohn M, et al. RAG-2-deficient mice lack mature lymphocytes owing to inability to initiate V(D)J rearrangement. Cell (1992) 68:855–67. doi: 10.1016/0092-8674(92)90029-c
25. Greiner DL, Hesselton RA, Shultz LD. SCID mouse models of human stem cell engraftment. Stem Cells (1998) 16:166–77. doi: 10.1002/stem.160166
26. Lumkul R, Gorin NC, Malehorn MT, Hoehn GT, Zheng R, Baldwin B, et al. Human AML cells in NOD/SCID mice: engraftment potential and gene expression. Leukemia (2002) 16:1818–26. doi: 10.1038/sj.leu.2402632
27. Baersch G, Mollers T, Hotte A, Dockhorn-Dworniczak B, Rube C, Ritter J, et al. Good engraftment of b-cell precursor ALL in NOD-SCID mice. Klin Padiatr (1997) 209:178–85. doi: 10.1055/s-2008-1043947
28. Pino S, Brehm MA, Covassin-Barberis L, King M, Gott B, Chase TH, et al. Development of novel major histocompatibility complex class i and class II-deficient NOD-SCID IL2R gamma chain knockout mice for modeling human xenogeneic graft-versus-host disease. Methods Mol Biol (2010) 602:105–17. doi: 10.1007/978-1-60761-058-8_7
29. Cao X, Shores EW, Hu-Li J, Anver MR, Kelsall BL, Russell SM, et al. Defective lymphoid development in mice lacking expression of the common cytokine receptor gamma chain. Immunity (1995) 2:223–38. doi: 10.1016/1074-7613(95)90047-0
30. Ishikawa F, Yasukawa M, Lyons B, Yoshida S, Miyamoto T, Yoshimoto G, et al. Development of functional human blood and immune systems in NOD/SCID/IL2 receptor gamma chain(null) mice. Blood (2005) 106:1565–73. doi: 10.1182/blood-2005-02-0516
31. Ito M, Hiramatsu H, Kobayashi K, Suzue K, Kawahata M, Hioki K, et al. NOD/SCID/gamma(c)(null) mouse: an excellent recipient mouse model for engraftment of human cells. Blood (2002) 100:3175–82. doi: 10.1182/blood-2001-12-0207
32. Okada S, Harada H, Ito T, Saito T, Suzu S. Early development of human hematopoietic and acquired immune systems in new born NOD/Scid/Jak3null mice intrahepatic engrafted with cord blood-derived CD34 + cells. Int J Hematol (2008) 88:476–82. doi: 10.1007/s12185-008-0215-z
33. Brehm MA, Cuthbert A, Yang C, Miller DM, DiIorio P, Laning J, et al. Parameters for establishing humanized mouse models to study human immunity: analysis of human hematopoietic stem cell engraftment in three immunodeficient strains of mice bearing the IL2rgamma(null) mutation. Clin Immunol (2010) 135:84–98. doi: 10.1016/j.clim.2009.12.008
34. Strowig T, Rongvaux A, Rathinam C, Takizawa H, Borsotti C, Philbrick W, et al. Transgenic expression of human signal regulatory protein alpha in Rag2-/-gamma(c)-/- mice improves engraftment of human hematopoietic cells in humanized mice. Proc Natl Acad Sci USA (2011) 108:13218–23. doi: 10.1073/pnas.1109769108
35. Pearson T, Shultz LD, Miller D, King M, Laning J, Fodor W, et al. Non-obese diabetic-recombination activating gene-1 (NODRag1 null) interleukin (IL)-2 receptor common gamma chain (IL2r gamma null) null mice: a radioresistant model for human lymphohaematopoietic engraftment. Clin Exp Immunol (2008) 154:270–84. doi: 10.1111/j.1365-2249.2008.03753.x
36. Goyama S, Wunderlich M, Mulloy JC. Xenograft models for normal and Malignant stem cells. Blood (2015) 125:2630–40. doi: 10.1182/blood-2014-11-570218
37. Weinstein JN, Pommier Y. Transcriptomic analysis of the NCI-60 cancer cell lines. C R Biol (2003) 326:909–20. doi: 10.1016/j.crvi.2003.08.005
38. Gazdar AF, Hirsch FR, Minna JD. From mice to men and back: An assessment of preclinical model systems for the study of lung cancers. J Thorac Oncol (2016) 11:287–99. doi: 10.1016/j.jtho.2015.10.009
39. Libouban H. The use of animal models in multiple myeloma. Morphologie (2015) 99:63–72. doi: 10.1016/j.morpho.2015.01.003
40. Aparicio S, Hidalgo M, Kung AL. Examining the utility of patient-derived xenograft mouse models. Nat Rev Cancer (2015) 15:311–6. doi: 10.1038/nrc3944
41. Hidalgo M, Amant F, Biankin AV, Budinska E, Byrne AT, Caldas C, et al. Patient-derived xenograft models: an emerging platform for translational cancer research. Cancer Discovery (2014) 4:998–1013. doi: 10.1158/2159-8290.CD-14-0001
42. Zhong C, Kayamori K, Koide S, Shinoda D, Oshima M, Nakajima-Takagi Y, et al. Efficacy of the novel tubulin polymerization inhibitor PTC-028 for myelodysplastic syndrome. Cancer Sci (2020) 111:4336–47. doi: 10.1111/cas.14684
43. Farge T, Saland E, de Toni F, Aroua N, Hosseini M, Perry R, et al. Chemotherapy-resistant human acute myeloid leukemia cells are not enriched for leukemic stem cells but require oxidative metabolism. Cancer Discovery (2017) 7:716–35. doi: 10.1158/2159-8290.CD-16-0441
44. Cho SY, Kang W, Han JY, Min S, Kang J, Lee A, et al. An integrative approach to precision cancer medicine using patient-derived xenografts. Mol Cells (2016) 39:77–86. doi: 10.14348/molcells.2016.2350
45. Clappier E, Gerby B, Sigaux F, Delord M, Touzri F, Hernandez L, et al. Clonal selection in xenografted human t cell acute lymphoblastic leukemia recapitulates gain of malignancy at relapse. J Exp Med (2011) 208:653–61. doi: 10.1084/jem.20110105
46. Ebinger S, Ozdemir EZ, Ziegenhain C, Tiedt S, Castro Alves C, Grunert M, et al. Characterization of rare, dormant, and therapy-resistant cells in acute lymphoblastic leukemia. Cancer Cell (2016) 30:849–62. doi: 10.1016/j.ccell.2016.11.002
47. Schueler J, Greve G, Lenhard D, Pantic M, Edinger A, Oswald E, et al. Impact of the injection site on growth characteristics, phenotype and sensitivity towards cytarabine of twenty acute leukaemia patient-derived xenograft models. Cancers (Basel) (2020) 12:1349. doi: 10.3390/cancers12051349
48. Townsend EC, Murakami MA, Christodoulou A, Christie AL, Koster J, DeSouza TA, et al. The public repository of xenografts enables discovery and randomized phase II-like trials in mice. Cancer Cell (2016) 29:574–86. doi: 10.1016/j.ccell.2016.03.008
49. Poglio S, Prochazkova-Carlotti M, Cherrier F, Gros A, Laharanne E, Pham-Ledard A, et al. Xenograft and cell culture models of sezary syndrome reveal cell of origin diversity and subclonal heterogeneity. Leukemia (2021) 35:1696–709. doi: 10.1038/s41375-020-01068-2
50. Schueler J, Wider D, Klingner K, Siegers GM, May AM, Wasch R, et al. Intratibial injection of human multiple myeloma cells in NOD/SCID IL-2Rgamma(null) mice mimics human myeloma and serves as a valuable tool for the development of anticancer strategies. PloS One (2013) 8:e79939. doi: 10.1371/journal.pone.0079939
51. Farre L, Sanz G, Ruiz-Xiville N, Castro de Moura M, Martin-Tejera JF, Goncalves-Ribeiro S, et al. Extramedullary multiple myeloma patient-derived orthotopic xenograft with a highly altered genome: combined molecular and therapeutic studies. Dis Model Mech (2021) 14:dmm048223. doi: 10.1242/dmm.048223
52. Shankland KR, Armitage JO, Hancock BW. Non-hodgkin lymphoma. Lancet (2012) 380:848–57. doi: 10.1016/S0140-6736(12)60605-9
53. Armitage JO, Gascoyne RD, Lunning MA, Cavalli F. Non-hodgkin lymphoma. Lancet (2017) 390:298–310. doi: 10.1016/S0140-6736(16)32407-2
54. Kapp U, Wolf J, Hummel M, Pawlita M, von Kalle C, Dallenbach F, et al. Hodgkin's lymphoma-derived tissue serially transplanted into severe combined immunodeficient mice. Blood (1993) 82:1247–56. doi: 10.1182/blood.V82.4.1247.1247
55. Chapuy B, Cheng H, Watahiki A, Ducar MD, Tan Y, Chen L, et al. Diffuse large b-cell lymphoma patient-derived xenograft models capture the molecular and biological heterogeneity of the disease. Blood (2016) 127:2203–13. doi: 10.1182/blood-2015-09-672352
56. Zhang L, Nomie K, Zhang H, Bell T, Pham L, Kadri S, et al. B-cell lymphoma patient-derived xenograft models enable drug discovery and are a platform for personalized therapy. Clin Cancer Res (2017) 23:4212–23. doi: 10.1158/1078-0432.CCR-16-2703
57. Wong NC, Bhadri VA, Maksimovic J, Parkinson-Bates M, Ng J, Craig JM, et al. Stability of gene expression and epigenetic profiles highlights the utility of patient-derived paediatric acute lymphoblastic leukaemia xenografts for investigating molecular mechanisms of drug resistance. BMC Genomics (2014) 15:416. doi: 10.1186/1471-2164-15-416
58. Shultz LD, Goodwin N, Ishikawa F, Hosur V, Lyons BL, Greiner DL. Subcapsular transplantation of tissue in the kidney. Cold Spring Harb Protoc (2014) 2014:737–40. doi: 10.1101/pdb.prot078089
59. Ma XY, Wei L, Lei Z, Chen Y, Ding Z, Chen ZS. Recent progress on targeting leukemia stem cells. Drug Discovery Today (2021) 26:1904–13. doi: 10.1016/j.drudis.2021.05.009
60. Richter-Pechanska P, Kunz JB, Bornhauser B, von Knebel Doeberitz C, Rausch T, Erarslan-Uysal B, et al. PDX models recapitulate the genetic and epigenetic landscape of pediatric t-cell leukemia. EMBO Mol Med (2018) 10:e9443. doi: 10.15252/emmm.201809443
61. Woiterski J, Ebinger M, Witte KE, Goecke B, Heininger V, Philippek M, et al. Engraftment of low numbers of pediatric acute lymphoid and myeloid leukemias into NOD/SCID/IL2Rcgammanull mice reflects individual leukemogenecity and highly correlates with clinical outcome. Int J Cancer (2013) 133:1547–56. doi: 10.1002/ijc.28170
62. Ishikawa F, Yoshida S, Saito Y, Hijikata A, Kitamura H, Tanaka S, et al. Chemotherapy-resistant human AML stem cells home to and engraft within the bone-marrow endosteal region. Nat Biotechnol (2007) 25:1315–21. doi: 10.1038/nbt1350
63. Ninomiya M, Abe A, Katsumi A, Xu J, Ito M, Arai F, et al. Homing, proliferation and survival sites of human leukemia cells in vivo in immunodeficient mice. Leukemia (2007) 21:136–42. doi: 10.1038/sj.leu.2404432
64. Lock RB, Liem N, Farnsworth ML, Milross CG, Xue C, Tajbakhsh M, et al. The nonobese diabetic/severe combined immunodeficient (NOD/SCID) mouse model of childhood acute lymphoblastic leukemia reveals intrinsic differences in biologic characteristics at diagnosis and relapse. Blood (2002) 99:4100–8. doi: 10.1182/blood.v99.11.4100
65. Silvestri G, Trotta R, Stramucci L, Ellis JJ, Harb JG, Neviani P, et al. Persistence of drug-resistant leukemic stem cells and impaired NK cell immunity in CML patients depend on MIR300 antiproliferative and PP2Aactivating functions. Blood Cancer Discovery (2020) 1:48–67. doi: 10.1158/0008-5472.BCD-19-0039
66. Muguruma Y, Matsushita H, Yahata T, Yumino S, Tanaka Y, Miyachi H, et al. Establishment of a xenograft model of human myelodysplastic syndromes. Haematologica (2011) 96:543–51. doi: 10.3324/haematol.2010.027557
67. Eppert K, Takenaka K, Lechman ER, Waldron L, Nilsson B, van Galen P, et al. Stem cell gene expression programs influence clinical outcome in human leukemia. Nat Med (2011) 17:1086–93. doi: 10.1038/nm.2415
68. Sawyers CL, Gishizky ML, Quan S, Golde DW, Witte ON. Propagation of human blastic myeloid leukemias in the SCID mouse. Blood (1992) 79:2089–98. doi: 10.1182/blood.V79.8.2089.2089
69. Mazurier F, Doedens M, Gan OI, Dick JE. Rapid myeloerythroid repopulation after intrafemoral transplantation of NOD-SCID mice reveals a new class of human stem cells. Nat Med (2003) 9:959–63. doi: 10.1038/nm886
70. Pilarski LM, Hipperson G, Seeberger K, Pruski E, Coupland RW, Belch AR. Myeloma progenitors in the blood of patients with aggressive or minimal disease: engraftment and self-renewal of primary human myeloma in the bone marrow of NOD SCID mice. Blood (2000) 95:1056–65. doi: 10.1182/blood.V95.3.1056.003k26_1056_1065
71. Yue Y, Cao Y, Mao X, Wang F, Fan P, Qian L, et al. Novel myeloma patient-derived xenograft models unveil the potency of anlotinib to overcome bortezomib resistance. Front Oncol (2022) 12:894279. doi: 10.3389/fonc.2022.894279
72. Blade J, Fernandez de Larrea C, Rosinol L, Cibeira MT, Jimenez R, Powles R. Soft-tissue plasmacytomas in multiple myeloma: incidence, mechanisms of extramedullary spread, and treatment approach. J Clin Oncol (2011) 29:3805–12. doi: 10.1200/JCO.2011.34.9290
73. Lawson MA, Paton-Hough JM, Evans HR, Walker RE, Harris W, Ratnabalan D, et al. NOD/SCID-GAMMA mice are an ideal strain to assess the efficacy of therapeutic agents used in the treatment of myeloma bone disease. PloS One (2015) 10:e0119546. doi: 10.1371/journal.pone.0119546
74. Haase D, Germing U, Schanz J, Pfeilstocker M, Nosslinger T, Hildebrandt B, et al. New insights into the prognostic impact of the karyotype in MDS and correlation with subtypes: evidence from a core dataset of 2124 patients. Blood (2007) 110:4385–95. doi: 10.1182/blood-2007-03-082404
75. Liu W, Teodorescu P, Halene S, Ghiaur G. The coming of age of preclinical models of MDS. Front Oncol (2022) 12:815037. doi: 10.3389/fonc.2022.815037
76. Drexler HG, Dirks WG, Macleod RA. Many are called MDS cell lines: one is chosen. Leuk Res (2009) 33:1011–6. doi: 10.1016/j.leukres.2009.03.005
77. Pang WW, Pluvinage JV, Price EA, Sridhar K, Arber DA, Greenberg PL, et al. Hematopoietic stem cell and progenitor cell mechanisms in myelodysplastic syndromes. Proc Natl Acad Sci USA (2013) 110:3011–6. doi: 10.1073/pnas.1222861110
78. Woll PS, Kjallquist U, Chowdhury O, Doolittle H, Wedge DC, Thongjuea S, et al. Myelodysplastic syndromes are propagated by rare and distinct human cancer stem cells in vivo. Cancer Cell (2014) 25:794–808. doi: 10.1016/j.ccr.2014.03.036
79. Strupp C, Nachtkamp K, Hildebrandt B, Giagounidis A, Haas R, Gattermann N, et al. New proposals of the WHO working group (2016) for the diagnosis of myelodysplastic syndromes (MDS): Characteristics of refined MDS types. Leuk Res (2017) 57:78–84. doi: 10.1016/j.leukres.2017.02.008
80. Kunimoto H, Fukuchi Y, Murakami K, Ikeda J, Teranaka H, Kato I, et al. Establishment of a high-risk MDS/AML cell line YCUAML1 and its xenograft model harboring t(3;3) and monosomy 7. Hemasphere (2020) 4:e469. doi: 10.1097/HS9.0000000000000469
81. Martin MG, Welch JS, Uy GL, Fehniger TA, Kulkarni S, Duncavage EJ, et al. Limited engraftment of low-risk myelodysplastic syndrome cells in NOD/SCID gamma-c chain knockout mice. Leukemia (2010) 24:1662–4. doi: 10.1038/leu.2010.156
82. Shalapour S, Zelmer A, Pfau M, Moderegger E, Costa-Blechschmidt C, van Landeghem FK, et al. The thalidomide analogue, CC-4047, induces apoptosis signaling and growth arrest in childhood acute lymphoblastic leukemia cells in vitro and in vivo. Clin Cancer Res (2006) 12:5526–32. doi: 10.1158/1078-0432.CCR-06-0719
83. Irving J, Matheson E, Minto L, Blair H, Case M, Halsey C, et al. Ras pathway mutations are prevalent in relapsed childhood acute lymphoblastic leukemia and confer sensitivity to MEK inhibition. Blood (2014) 124:3420–30. doi: 10.1182/blood-2014-04-531871
84. Wege AK. Humanized mouse models for the preclinical assessment of cancer immunotherapy. BioDrugs (2018) 32:245–66. doi: 10.1007/s40259-018-0275-4
85. Seal JB, Bohorquez H, Battula N, DeGregorio L, Bugeaud E, Bruce DS, et al. Balloon-occlusion technique for managing portal vein hemorrhage in liver transplantation. Ochsner J (2017) 17:76–9.
86. Jung J, Seol HS, Chang S. The generation and application of patient-derived xenograft model for cancer research. Cancer Res Treat (2018) 50:1–10. doi: 10.4143/crt.2017.307
87. Okada S, Vaeteewoottacharn K, Kariya R. Application of highly immunocompromised mice for the establishment of patient-derived xenograft (PDX) models. Cells (2019) 8:889. doi: 10.3390/cells8080889
88. Byrne AT, Alferez DG, Amant F, Annibali D, Arribas J, Biankin AV, et al. Interrogating open issues in cancer precision medicine with patient-derived xenografts. Nat Rev Cancer (2017) 17:254–68. doi: 10.1038/nrc.2016.140
89. Ishizawa J, Nakamaru K, Seki T, Tazaki K, Kojima K, Chachad D, et al. Predictive gene signatures determine tumor sensitivity to MDM2 inhibition. Cancer Res (2018) 78:2721–31. doi: 10.1158/0008-5472.CAN-17-0949
90. Rongvaux A, Takizawa H, Strowig T, Willinger T, Eynon EE, Flavell RA, et al. Human hemato-lymphoid system mice: current use and future potential for medicine. Annu Rev Immunol (2013) 31:635–74. doi: 10.1146/annurev-immunol-032712-095921
91. Taoka K, Arai S, Kataoka K, Hosoi M, Miyauchi M, Yamazaki S, et al. Using patient-derived iPSCs to develop humanized mouse models for chronic myelomonocytic leukemia and therapeutic drug identification, including liposomal clodronate. Sci Rep (2018) 8:15855. doi: 10.1038/s41598-018-34193-1
92. Kumano K, Arai S, Hosoi M, Taoka K, Takayama N, Otsu M, et al. Generation of induced pluripotent stem cells from primary chronic myelogenous leukemia patient samples. Blood (2012) 119:6234–42. doi: 10.1182/blood-2011-07-367441
93. Woo XY, Giordano J, Srivastava A, Zhao ZM, Lloyd MW, de Bruijn R, et al. Conservation of copy number profiles during engraftment and passaging of patient-derived cancer xenografts. Nat Genet (2021) 53:86–99. doi: 10.1038/s41588-020-00750-6
94. Ben-David U, Ha G, Tseng YY, Greenwald NF, Oh C, Shih J, et al. Patient-derived xenografts undergo mouse-specific tumor evolution. Nat Genet (2017) 49:1567–75. doi: 10.1038/ng.3967
95. Takayama N, Eto K. In vitro generation of megakaryocytes and platelets from human embryonic stem cells and induced pluripotent stem cells. Methods Mol Biol (2012) 788:205–17. doi: 10.1007/978-1-61779-307-3_15
96. Gandre-Babbe S, Paluru P, Aribeana C, Chou ST, Bresolin S, Lu L, et al. Patient-derived induced pluripotent stem cells recapitulate hematopoietic abnormalities of juvenile myelomonocytic leukemia. Blood (2013) 121:4925–9. doi: 10.1182/blood-2013-01-478412
97. Chao MP, Gentles AJ, Chatterjee S, Lan F, Reinisch A, Corces MR, et al. Human AML-iPSCs reacquire leukemic properties after differentiation and model clonal variation of disease. Cell Stem Cell (2017) 20:329–344.e327. doi: 10.1016/j.stem.2016.11.018
98. Reinisch A, Thomas D, Corces MR, Zhang X, Gratzinger D, Hong WJ, et al. A humanized bone marrow ossicle xenotransplantation model enables improved engraftment of healthy and leukemic human hematopoietic cells. Nat Med (2016) 22:812–21. doi: 10.1038/nm.4103
99. Amabile G, Di Ruscio A, Muller F, Welner RS, Yang H, Ebralidze AK, et al. Dissecting the role of aberrant DNA methylation in human leukaemia. Nat Commun (2015) 6:7091. doi: 10.1038/ncomms8091
100. Munoz-Lopez A, Romero-Moya D, Prieto C, Ramos-Mejia V, Agraz-Doblas A, Varela I, et al. Development refractoriness of MLL-rearranged human b cell acute leukemias to reprogramming into pluripotency. Stem Cell Rep (2016) 7:602–18. doi: 10.1016/j.stemcr.2016.08.013
101. Lee J, Dykstra B, Spencer JA, Kenney LL, Greiner DL, Shultz LD, et al. mRNA-mediated glycoengineering ameliorates deficient homing of human stem cell-derived hematopoietic progenitors. J Clin Invest (2017) 127:2433–7. doi: 10.1172/JCI92030
102. Zhang Y, Peng J, Tang Y, He J, Peng J, Zhao Q, et al. The prevalence of epstein-barr virus infection in different types and sites of lymphomas. Jpn J Infect Dis (2010) 63:132–5. doi: 10.7883/yoken.63.132
103. Peres E, Bagdassarian E, This S, Villaudy J, Rigal D, Gazzolo L, et al. From immunodeficiency to humanization: The contribution of mouse models to explore HTLV-1 leukemogenesis. Viruses (2015) 7:6371–86. doi: 10.3390/v7122944
104. Galli V, Nixon CC, Strbo N, Artesi M, de Castro-Amarante MF, McKinnon K, et al. Essential role of human t cell leukemia virus type 1 orf-i in lethal proliferation of CD4(+) cells in humanized mice. J Virol (2019) 93:e00565–19. doi: 10.1128/JVI.00565-19
105. Mosier DE, Gulizia RJ, Baird SM, Wilson DB. Transfer of a functional human immune system to mice with severe combined immunodeficiency. Nature (1988) 335:256–9. doi: 10.1038/335256a0
106. Yuling H, Ruijing X, Li L, Xiang J, Rui Z, Yujuan W, et al. EBV-induced human CD8+ NKT cells suppress tumorigenesis by EBV-associated malignancies. Cancer Res (2009) 69:7935–44. doi: 10.1158/0008-5472.CAN-09-0828
107. Yajima M, Imadome K, Nakagawa A, Watanabe S, Terashima K, Nakamura H, et al. A new humanized mouse model of epstein-barr virus infection that reproduces persistent infection, lymphoproliferative disorder, and cell-mediated and humoral immune responses. J Infect Dis (2008) 198:673–82. doi: 10.1086/590502
108. Ma SD, Hegde S, Young KH, Sullivan R, Rajesh D, Zhou Y, et al. A new model of epstein-barr virus infection reveals an important role for early lytic viral protein expression in the development of lymphomas. J Virol (2011) 85:165–77. doi: 10.1128/JVI.01512-10
109. Fujiwara S, Matsuda G, Imadome K. Humanized mouse models of epstein-barr virus infection and associated diseases. Pathogens (2013) 2:153–76. doi: 10.3390/pathogens2010153
110. Munz C. Humanized mouse models for Epstein Barr virus infection. Curr Opin Virol (2017) 25:113–8. doi: 10.1016/j.coviro.2017.07.026
111. Lee EK, Joo EH, Song KA, Choi B, Kim M, Kim SH, et al. Effects of lymphocyte profile on development of EBV-induced lymphoma subtypes in humanized mice. Proc Natl Acad Sci USA (2015) 112:13081–6. doi: 10.1073/pnas.1407075112
112. Fujiwara S, Nakamura H. Animal models for gammaherpesvirus infections: recent development in the analysis of virus-induced pathogenesis. Pathogens (2020) 9:116. doi: 10.3390/pathogens9020116
113. Jenner RG, Maillard K, Cattini N, Weiss RA, Boshoff C, Wooster R, et al. Kaposi's sarcoma-associated herpesvirus-infected primary effusion lymphoma has a plasma cell gene expression profile. Proc Natl Acad Sci USA (2003) 100:10399–404. doi: 10.1073/pnas.1630810100
114. Wei J, Wunderlich M, Fox C, Alvarez S, Cigudosa JC, Wilhelm JS, et al. Microenvironment determines lineage fate in a human model of MLL-AF9 leukemia. Cancer Cell (2008) 13:483–95. doi: 10.1016/j.ccr.2008.04.020
115. Barabe F, Kennedy JA, Hope KJ, Dick JE. Modeling the initiation and progression of human acute leukemia in mice. Science (2007) 316:600–4. doi: 10.1126/science.1139851
116. Kim WT, Hennick K, Johnson J, Finnerty B, Choo S, Short SB, et al. Cancer-associated POT1 mutations lead to telomere elongation without induction of a DNA damage response. EMBO J (2021) 40:e107346. doi: 10.15252/embj.2020107346
117. Askmyr M, Agerstam H, Lilljebjorn H, Hansen N, Karlsson C, von Palffy S, et al. Modeling chronic myeloid leukemia in immunodeficient mice reveals expansion of aberrant mast cells and accumulation of pre-b cells. Blood Cancer J (2014) 4:e269. doi: 10.1038/bcj.2014.89
118. Rizo A, Horton SJ, Olthof S, Dontje B, Ausema A, van Os R, et al. BMI1 collaborates with BCR-ABL in leukemic transformation of human CD34+ cells. Blood (2010) 116:4621–30. doi: 10.1182/blood-2010-02-270660
119. Qin H, Malek S, Cowell JK, Ren M. Transformation of human CD34+ hematopoietic progenitor cells with DEK-NUP214 induces AML in an immunocompromised mouse model. Oncogene (2016) 35:5686–91. doi: 10.1038/onc.2016.118
120. Ren M, Qin H, Wu Q, Savage NM, George TI, Cowell JK. Development of ZMYM2-FGFR1 driven AML in human CD34+ cells in immunocompromised mice. Int J Cancer (2016) 139:836–40. doi: 10.1002/ijc.30100
121. Wunderlich M, Chou FS, Link KA, Mizukawa B, Perry RL, Carroll M, et al. AML xenograft efficiency is significantly improved in NOD/SCID-IL2RG mice constitutively expressing human SCF, GM-CSF and IL-3. Leukemia (2010) 24:1785–8. doi: 10.1038/leu.2010.158
122. Ren M, Qin H, Kitamura E, Cowell JK. Dysregulated signaling pathways in the development of CNTRL-FGFR1-induced myeloid and lymphoid Malignancies associated with FGFR1 in human and mouse models. Blood (2013) 122:1007–16. doi: 10.1182/blood-2013-03-489823
123. Kennedy JA, Barabe F, Patterson BJ, Bayani J, Squire JA, Barber DL, et al. Expression of TEL-JAK2 in primary human hematopoietic cells drives erythropoietin-independent erythropoiesis and induces myelofibrosis in vivo. Proc Natl Acad Sci USA (2006) 103:16930–5. doi: 10.1073/pnas.0604902103
124. Chudnovsky Y, Adams AE, Robbins PB, Lin Q, Khavari PA. Use of human tissue to assess the oncogenic activity of melanoma-associated mutations. Nat Genet (2005) 37:745–9. doi: 10.1038/ng1586
125. Elenbaas B, Spirio L, Koerner F, Fleming MD, Zimonjic DB, Donaher JL, et al. Human breast cancer cells generated by oncogenic transformation of primary mammary epithelial cells. Genes Dev (2001) 15:50–65. doi: 10.1101/gad.828901
126. Chiang MY, Shestova O, Xu L, Aster JC, Pear WS. Divergent effects of supraphysiologic Notch signals on leukemia stem cells and hematopoietic stem cells. Blood (2013) 121:905–17. doi: 10.1182/blood-2012-03-416503
127. Haji Y, Suzuki M, Moriya K, So T, Hozumi K, Mizuma M, et al. Activation of Notch1 promotes development of human CD8(+) single positive t cells in humanized mice. Biochem Biophys Res Commun (2014) 447:346–51. doi: 10.1016/j.bbrc.2014.04.003
128. Arranz L. The hematology of tomorrow is here-preclinical models are not: Cell therapy for hematological Malignancies. Cancers (Basel) (2022) 14:580. doi: 10.3390/cancers14030580
129. Medyouf H, Mossner M, Jann JC, Nolte F, Raffel S, Herrmann C, et al. Myelodysplastic cells in patients reprogram mesenchymal stromal cells to establish a transplantable stem cell niche disease unit. Cell Stem Cell (2014) 14:824–37. doi: 10.1016/j.stem.2014.02.014
130. Klco JM, Spencer DH, Miller CA, Griffith M, Lamprecht TL, O'Laughlin M, et al. Functional heterogeneity of genetically defined subclones in acute myeloid leukemia. Cancer Cell (2014) 25:379–92. doi: 10.1016/j.ccr.2014.01.031
131. Brehm MA, Racki WJ, Leif J, Burzenski L, Hosur V, Wetmore A, et al. Engraftment of human HSCs in nonirradiated newborn NOD-scid IL2rgamma null mice is enhanced by transgenic expression of membrane-bound human SCF. Blood (2012) 119:2778–88. doi: 10.1182/blood-2011-05-353243
132. Takagi S, Saito Y, Hijikata A, Tanaka S, Watanabe T, Hasegawa T, et al. Membrane-bound human SCF/KL promotes in vivo human hematopoietic engraftment and myeloid differentiation. Blood (2012) 119:2768–77. doi: 10.1182/blood-2011-05-353201
133. Herndler-Brandstetter D, Shan L, Yao Y, Stecher C, Plajer V, Lietzenmayer M, et al. Humanized mouse model supports development, function, and tissue residency of human natural killer cells. Proc Natl Acad Sci USA (2017) 114:E9626–34. doi: 10.1073/pnas.1705301114
134. Hanazawa A, Ito R, Katano I, Kawai K, Goto M, Suemizu H, et al. Generation of human immunosuppressive myeloid cell populations in human interleukin-6 transgenic NOG mice. Front Immunol (2018) 9:152. doi: 10.3389/fimmu.2018.00152
135. Song Y, Rongvaux A, Taylor A, Jiang T, Tebaldi T, Balasubramanian K, et al. A highly efficient and faithful MDS patient-derived xenotransplantation model for pre-clinical studies. Nat Commun (2019) 10:366. doi: 10.1038/s41467-018-08166-x
136. Das R, Strowig T, Verma R, Koduru S, Hafemann A, Hopf S, et al. Microenvironment-dependent growth of preneoplastic and malignant plasma cells in humanized mice. Nat Med (2016) 22:1351–7. doi: 10.1038/nm.4202
137. Rongvaux A, Willinger T, Takizawa H, Rathinam C, Auerbach W, Murphy AJ, et al. Human thrombopoietin knockin mice efficiently support human hematopoiesis in vivo. Proc Natl Acad Sci USA (2011) 108:2378–83. doi: 10.1073/pnas.1019524108
138. Chou FS, Griesinger A, Wunderlich M, Lin S, Link KA, Shrestha M, et al. The thrombopoietin/MPL/Bcl-xL pathway is essential for survival and self-renewal in human preleukemia induced by AML1-ETO. Blood (2012) 120:709–19. doi: 10.1182/blood-2012-01-403212
139. Billerbeck E, Barry WT, Mu K, Dorner M, Rice CM, Ploss A. Development of human CD4+FoxP3+ regulatory t cells in human stem cell factor-, granulocyte-macrophage colony-stimulating factor-, and interleukin-3-expressing NOD-SCID IL2Rgamma(null) humanized mice. Blood (2011) 117:3076–86. doi: 10.1182/blood-2010-08-301507
140. Theocharides AP, Rongvaux A, Fritsch K, Flavell RA, Manz MG. Humanized hemato-lymphoid system mice. Haematologica (2016) 101:5–19. doi: 10.3324/haematol.2014.115212
141. Zhang Y, He L, Selimoglu-Buet D, Jego C, Morabito M, Willekens C, et al. Engraftment of chronic myelomonocytic leukemia cells in immunocompromised mice supports disease dependency on cytokines. Blood Adv (2017) 1:972–9. doi: 10.1182/bloodadvances.2017004903
142. Rongvaux A, Willinger T, Martinek J, Strowig T, Gearty SV, Teichmann LL, et al. Development and function of human innate immune cells in a humanized mouse model. Nat Biotechnol (2014) 32:364–72. doi: 10.1038/nbt.2858
143. Sirard C, Lapidot T, Vormoor J, Cashman JD, Doedens M, Murdoch B, et al. Normal and leukemic SCID-repopulating cells (SRC) coexist in the bone marrow and peripheral blood from CML patients in chronic phase, whereas leukemic SRC are detected in blast crisis. Blood (1996) 87:1539–48. doi: 10.1182/blood.V87.4.1539.bloodjournal8741539
144. Muguruma Y, Yahata T, Miyatake H, Sato T, Uno T, Itoh J, et al. Reconstitution of the functional human hematopoietic microenvironment derived from human mesenchymal stem cells in the murine bone marrow compartment. Blood (2006) 107:1878–87. doi: 10.1182/blood-2005-06-2211
145. Antonelli A, Noort WA, Jaques J, de Boer B, de Jong-Korlaar R, Brouwers-Vos AZ, et al. Establishing human leukemia xenograft mouse models by implanting human bone marrow-like scaffold-based niches. Blood (2016) 128:2949–59. doi: 10.1182/blood-2016-05-719021
146. Yin X, Hu L, Zhang Y, Zhu C, Cheng H, Xie X, et al. PDGFB-expressing mesenchymal stem cells improve human hematopoietic stem cell engraftment in immunodeficient mice. Bone Marrow Transplant (2020) 55:1029–40. doi: 10.1038/s41409-019-0766-z
147. Abarrategi A, Mian SA, Passaro D, Rouault-Pierre K, Grey W, Bonnet D. Modeling the human bone marrow niche in mice: From host bone marrow engraftment to bioengineering approaches. J Exp Med (2018) 215:729–43. doi: 10.1084/jem.20172139
148. Le PM, Andreeff M, Battula VL. Osteogenic niche in the regulation of normal hematopoiesis and leukemogenesis. Haematologica (2018) 103:1945–55. doi: 10.3324/haematol.2018.197004
149. Abarrategi A, Foster K, Hamilton A, Mian SA, Passaro D, Gribben J, et al. Versatile humanized niche model enables study of normal and malignant human hematopoiesis. J Clin Invest (2017) 127:543–8. doi: 10.1172/JCI89364
150. Groen RW, Noort WA, Raymakers RA, Prins HJ, Aalders L, Hofhuis FM, et al. Reconstructing the human hematopoietic niche in immunodeficient mice: opportunities for studying primary multiple myeloma. Blood (2012) 120:e9–e16. doi: 10.1182/blood-2012-03-414920
151. Reinisch A, Hernandez DC, Schallmoser K, Majeti R. Generation and use of a humanized bone-marrow-ossicle niche for hematopoietic xenotransplantation into mice. Nat Protoc (2017) 12:2169–88. doi: 10.1038/nprot.2017.088
152. Altrock E, Sens-Albert C, Jann JC, Flach J, Riabov V, Schmitt N, et al. Humanized three-dimensional scaffold xenotransplantation models for myelodysplastic syndromes. Exp Hematol (2022) 107:38–50. doi: 10.1016/j.exphem.2021.12.395
153. Come C, Balhuizen A, Bonnet D, Porse BT. Myelodysplastic syndrome patient-derived xenografts: from no options to many. Haematologica (2020) 105:864–9. doi: 10.3324/haematol.2019.233320
154. Pievani A, Donsante S, Tomasoni C, Corsi A, Dazzi F, Biondi A, et al. Acute myeloid leukemia shapes the bone marrow stromal niche. vivo. Haematologica (2021) 106:865–70. doi: 10.3324/haematol.2020.247205
155. Kumar B, Garcia M, Weng L, Jung X, Murakami JL, Hu X, et al. Acute myeloid leukemia transforms the bone marrow niche into a leukemia-permissive microenvironment through exosome secretion. Leukemia (2018) 32:575–87. doi: 10.1038/leu.2017.259
156. Vaiselbuh SR, Edelman M, Lipton JM, Liu JM. Ectopic human mesenchymal stem cell-coated scaffolds in NOD/SCID mice: an in vivo model of the leukemia niche. Tissue Eng Part C Methods (2010) 16:1523–31. doi: 10.1089/ten.tec.2010.0179
157. Yaccoby S, Barlogie B, Epstein J. Primary myeloma cells growing in SCID-hu mice: a model for studying the biology and treatment of myeloma and its manifestations. Blood (1998) 92:2908–13. doi: 10.1182/blood.V92.8.2908
158. Yata K, Yaccoby S. The SCID-rab model: a novel in vivo system for primary human myeloma demonstrating growth of CD138-expressing Malignant cells. Leukemia (2004) 18:1891–7. doi: 10.1038/sj.leu.2403513
159. Amodio N, Leotta M, Bellizzi D, Di Martino MT, D'Aquila P, Lionetti M, et al. DNA-demethylating and anti-tumor activity of synthetic miR-29b mimics in multiple myeloma. Oncotarget (2012) 3:1246–58. doi: 10.18632/oncotarget.675
160. Hanoun M, Zhang D, Mizoguchi T, Pinho S, Pierce H, Kunisaki Y, et al. Acute myelogenous leukemia-induced sympathetic neuropathy promotes malignancy in an altered hematopoietic stem cell niche. Cell Stem Cell (2014) 15:365–75. doi: 10.1016/j.stem.2014.06.020
161. Burack WR, Spence JM, Spence JP, Spence SA, Rock PJ, Shenoy GN, et al. Patient-derived xenografts of low-grade b-cell lymphomas demonstrate roles of the tumor microenvironment. Blood Adv (2017) 1:1263–73. doi: 10.1182/bloodadvances.2017005892
162. Miller RA, Maloney DG, Warnke R, Levy R. Treatment of B-cell lymphoma with monoclonal anti-idiotype antibody. N Engl J Med (1982) 306:517–22. doi: 10.1056/NEJM198203043060906
163. Park JH, Riviere I, Gonen M, Wang X, Senechal B, Curran KJ, et al. Long-term follow-up of CD19 CAR therapy in acute lymphoblastic leukemia. N Engl J Med (2018) 378:449–59. doi: 10.1056/NEJMoa1709919
164. Jackson HJ, Brentjens RJ. Overcoming antigen escape with CAR T-cell therapy. Cancer Discovery (2015) 5:1238–40. doi: 10.1158/2159-8290.CD-15-1275
165. Neelapu SS, Tummala S, Kebriaei P, Wierda W, Gutierrez C, Locke FL, et al. Chimeric antigen receptor t-cell therapy - assessment and management of toxicities. Nat Rev Clin Oncol (2018) 15:47–62. doi: 10.1038/nrclinonc.2017.148
166. Kalos M, Levine BL, Porter DL, Katz S, Grupp SA, Bagg A, et al. T cells with chimeric antigen receptors have potent antitumor effects and can establish memory in patients with advanced leukemia. Sci Transl Med (2011) 3:95ra73. doi: 10.1126/scitranslmed.3002842
167. Barrett DM, Zhao Y, Liu X, Jiang S, Carpenito C, Kalos M, et al. Treatment of advanced leukemia in mice with mRNA engineered t cells. Hum Gene Ther (2011) 22:1575–86. doi: 10.1089/hum.2011.070
168. Cany J, van der Waart AB, Tordoir M, Franssen GM, Hangalapura BN, de Vries J, et al. Natural killer cells generated from cord blood hematopoietic progenitor cells efficiently target bone marrow-residing human leukemia cells in NOD/SCID/IL2Rg(null) mice. PloS One (2013) 8:e64384. doi: 10.1371/journal.pone.0064384
169. Smith EJ, Olson K, Haber LJ, Varghese B, Duramad P, Tustian AD, et al. A novel, native-format bispecific antibody triggering t-cell killing of b-cells is robustly active in mouse tumor models and cynomolgus monkeys. Sci Rep (2015) 5:17943. doi: 10.1038/srep17943
170. Ito A, Ishida T, Yano H, Inagaki A, Suzuki S, Sato F, et al. Defucosylated anti-CCR4 monoclonal antibody exercises potent ADCC-mediated antitumor effect in the novel tumor-bearing humanized NOD/Shi-scid, IL-2Rgamma(null) mouse model. Cancer Immunol Immunother (2009) 58:1195–206. doi: 10.1007/s00262-008-0632-0
171. Gill S, Tasian SK, Ruella M, Shestova O, Li Y, Porter DL, et al. Preclinical targeting of human acute myeloid leukemia and myeloablation using chimeric antigen receptor-modified t cells. Blood (2014) 123(15):2343–54. doi: 10.1182/blood-2016-10-745448
172. Chu Y, Hochberg J, Yahr A, Ayello J, van de Ven C, Barth M, et al. Targeting CD20+ aggressive b-cell non-hodgkin lymphoma by anti-CD20 CAR mRNA-modified expanded natural killer cells in vitro and in NSG mice. Cancer Immunol Res (2015) 3:333–44. doi: 10.1158/2326-6066.CIR-14-0114
173. Shultz LD, Lyons BL, Burzenski LM, Gott B, Chen X, Chaleff S, et al. Human lymphoid and myeloid cell development in NOD/LtSz-scid IL2R gamma null mice engrafted with mobilized human hemopoietic stem cells. J Immunol (2005) 174:6477–89. doi: 10.4049/jimmunol.174.10.6477
174. Halkias J, Yen B, Taylor KT, Reinhartz O, Winoto A, Robey EA, et al. Conserved and divergent aspects of human t-cell development and migration in humanized mice. Immunol Cell Biol (2015) 93:716–26. doi: 10.1038/icb.2015.38
175. Smith KM, Fagan PC, Pomari E, Germano G, Frasson C, Walsh C, et al. Antitumor activity of entrectinib, a pan-TRK, ROS1, and ALK inhibitor, in ETV6-NTRK3-positive acute myeloid leukemia. Mol Cancer Ther (2018) 17:455–63. doi: 10.1158/1535-7163.MCT-17-0419
176. Ito A, Ishida T, Utsunomiya A, Sato F, Mori F, Yano H, et al. Defucosylated anti-CCR4 monoclonal antibody exerts potent ADCC against primary ATLL cells mediated by autologous human immune cells in NOD/Shi-scid, IL-2R gamma(null) mice. vivo. J Immunol (2009) 183:4782–91. doi: 10.4049/jimmunol.0900699
177. Pillarisetti K, Powers G, Luistro L, Babich A, Baldwin E, Li Y, et al. Teclistamab is an active t cell-redirecting bispecific antibody against b-cell maturation antigen for multiple myeloma. Blood Adv (2020) 4:4538–49. doi: 10.1182/bloodadvances.2020002393
178. Chen Y, Nagaraja NV, Fan B, Utley L, Lemieux RM, Popovici-Muller J, et al. Preclinical drug metabolism, pharmacokinetic, and pharmacodynamic profiles of ivosidenib, an inhibitor of mutant isocitrate dehydrogenase 1 for treatment of isocitrate dehydrogenase 1-mutant malignancies. Drug Metab Dispos (2021) 49:870–81. doi: 10.1124/dmd.120.000234
179. Manriquez Roman C, Sakemura RL, Jin F, Khadka RH, Adada MM, Siegler EL, et al. Assessment of chimeric antigen receptor t cell- Q20 associated toxicities using an acute lymphoblastic leukemia patient-derived xenograft mouse model. J Vis Exp (2023) 192. doi: 10.3791/64535
180. Wang Y, Zheng C, Zhuang C, Fu Q, Qin J, Zhang B, et al. Preclinical pharmacology and toxicology evaluation of an anti-CD52 monoclonal antibody produced by perfusion fermentation process. J Ind Microbiol Biotechnol (2021) 48:kuab078. doi: 10.1093/jimb/kuab078
181. Wang A, Song Z, Zheng G, Nicolazzi C, Fromm JR, Shehu E, et al. Evaluation of preclinical activity of isatuximab in patients with acute lymphoblastic leukemia. Mol Cancer Ther (2021) 20:1916–25. doi: 10.1158/1535-7163.MCT-21-0058
182. Ke H, Zhang F, Wang J, Xiong L, An X, Tu X, et al. HX009, a novel BsAb dual targeting PD1 x CD47, demonstrates potent anti-lymphoma activity in preclinical models. Sci Rep (2023) 13:5419. doi: 10.1038/s41598-023-32547-y
183. Thieme E, Bruss N, Sun D, Dominguez EC, Coleman D, Liu T, et al. CDK9 inhibition induces epigenetic reprogramming revealing strategies to circumvent resistance in lymphoma. Mol Cancer (2023) 22:64. doi: 10.1186/s12943-023-01762-6
184. Hiemstra IH, Santegoets KCM, Janmaat ML, De Goeij B, Ten Hagen W, van Dooremalen S, et al. Preclinical anti-tumour activity of HexaBody-CD38, a next-generation CD38 antibody with superior complement-dependent cytotoxic activity. EBioMedicine (2023) 93:104663. doi: 10.1016/j.ebiom.2023.104663
185. Pouleau B, Estoppey C, Suere P, Nallet E, Laurendon A, Monney T, et al. Preclinical characterization of ISB 1342, a CD38 x CD3 t-cell engager for relapsed/refractory multiple myeloma. Blood (2023) 142:260–73. doi: 10.1182/blood.2022019451
186. Sugita M, et al. Radiation therapy improves CAR T cell activity in acute lymphoblastic leukemia. Cell Death Dis (2023) 14:305. doi: 10.1038/s41419-023-05829-6
187. Randall J, et al. In vivo activity of the dual PI3Kdelta and PI3Kgamma inhibitor duvelisib against pediatric acute lymphoblastic leukemia xenografts. Pediatr Blood Cancer (2023) 70:e30398. doi: 10.1002/pbc.30398
188. Lameris R, et al. A bispecific T cell engager recruits both type 1 NKT and Vgamma9Vdelta2-T cells for the treatment of CD1d-expressing hematological Malignancies. Cell Rep Med (2023) 4:100961. doi: 10.1016/j.xcrm.2023.100961
189. van Rhee F, Szmania SM, Dillon M, van Abbema AM, Li X, Stone MK, et al. Combinatorial efficacy of anti-CS1 monoclonal antibody elotuzumab (HuLuc63) and bortezomib against multiple myeloma. Mol Cancer Ther (2009) 8:2616–24. doi: 10.1158/1535-7163.MCT-09-0483
190. Nijhof IS, Groen RW, Lokhorst HM, van Kessel B, Bloem AC, van Velzen J, et al. Upregulation of CD38 expression on multiple myeloma cells by all-trans retinoic acid improves the efficacy of daratumumab. Leukemia (2015) 29:2039–49. doi: 10.1038/leu.2015.123
191. Knutson SK, Kawano S, Minoshima Y, Warholic NM, Huang KC, Xiao Y, et al. Selective inhibition of EZH2 by EPZ-6438 leads to potent antitumor activity in EZH2-mutant non-hodgkin lymphoma. Mol Cancer Ther (2014) 13:842–54. doi: 10.1158/1535-7163.MCT-13-0773
192. Patterson CM, Balachander SB, Grant I, Pop-Damkov P, Kelly B, McCoull W, et al. Design and optimisation of dendrimer-conjugated bcl-2/x (L) inhibitor, AZD0466, with improved therapeutic index for cancer therapy. Commun Biol (2021) 4:112. doi: 10.1038/s42003-020-01631-8
193. Drent E, Groen RW, Noort WA, Themeli M, Lammerts van Bueren JJ, Parren PW, et al. Pre-clinical evaluation of CD38 chimeric antigen receptor engineered t cells for the treatment of multiple myeloma. Haematologica (2016) 101:616–25. doi: 10.3324/haematol.2015.137620
194. Lu Y, Liu Y, Wen S, Kuang N, Zhang X, Li J, et al. Naturally selected CD7 CAR-t therapy without genetic editing demonstrates significant antitumour efficacy against relapsed and refractory acute myeloid leukaemia (R/R-AML). J Transl Med (2022) 20:600. doi: 10.1186/s12967-022-03797-7
195. Pei Y, Xiang Z, Wen K, Tu CR, Wang X, Zhang Y, et al. CD137 costimulation enhances the antitumor activity of vgamma9Vdelta2-t cells in IL-10-mediated immunosuppressive tumor microenvironment. Front Immunol (2022) 13:872122. doi: 10.3389/fimmu.2022.872122
196. Ma SD, Xu X, Jones R, Delecluse HJ, Zumwalde NA, Sharma A, et al. PD-1/CTLA-4 blockade inhibits epstein-barr virus-induced lymphoma growth in a cord blood humanized-mouse model. PloS Pathog (2016) 12:e1005642. doi: 10.1371/journal.ppat.1005642
197. Jin KT, Du WL, Lan HR, Liu YY, Mao CS, Du JL, et al. Development of humanized mouse with patient-derived xenografts for cancer immunotherapy studies: A comprehensive review. Cancer Sci (2021) 112:2592–606. doi: 10.1111/cas.14934
198. Katano I, Takahashi T, Ito R, Kamisako T, Mizusawa T, Ka Y, et al. Predominant development of mature and functional human NK cells in a novel human IL-2-producing transgenic NOG mouse. J Immunol (2015) 194:3513–25. doi: 10.4049/jimmunol.1401323
199. Katano I, Nishime C, Ito R, Kamisako T, Mizusawa T, Ka Y, et al. Long-term maintenance of peripheral blood derived human NK cells in a novel human IL-15- transgenic NOG mouse. Sci Rep (2017) 7:17230. doi: 10.1038/s41598-017-17442-7
200. Matsuda M, Ono R, Iyoda T, Endo T, Iwasaki M, Tomizawa-Murasawa M, et al. Human NK cell development in hIL-7 and hIL-15 knockin NOD/SCID/IL2rgKO mice. Life Sci Alliance (2019) 2:e201800195. doi: 10.26508/lsa.201800195
201. Pek EA, Chan T, Reid S, Ashkar AA. Characterization and IL-15 dependence of NK cells in humanized mice. Immunobiology (2011) 216:218–24. doi: 10.1016/j.imbio.2010.04.008
202. Caruso S, De Angelis B, Del Bufalo F, Ciccone R, Donsante S, Volpe G, et al. Safe and effective off-the-shelf immunotherapy based on CAR.CD123-NK cells for the treatment of acute myeloid leukaemia. J Hematol Oncol (2022) 15:163. doi: 10.1186/s13045-022-01376-3
203. Mhaidly R, Verhoeyen E. The future: In Vivo CAR T cell gene therapy. Mol Ther (2019) 27:707–9. doi: 10.1016/j.ymthe.2019.03.012
204. Agarwal S, Weidner T, Thalheimer FB, Buchholz CJ. In vivo generated human CAR T cells eradicate tumor cells. Oncoimmunology (2019) 8:e1671761. doi: 10.1080/2162402X.2019.1671761
205. Mo F, Watanabe N, McKenna MK, Hicks MJ, Srinivasan M, Gomes-Silva D, et al. Engineered off-the-shelf therapeutic t cells resist host immune rejection. Nat Biotechnol (2021) 39:56–63. doi: 10.1038/s41587-020-0601-5
206. Diaconu I, Ballard B, Zhang M, Chen Y, West J, Dotti G, et al. Inducible caspase-9 selectively modulates the toxicities of CD19-specific chimeric antigen receptor-modified t cells. Mol Ther (2017) 25:580–92. doi: 10.1016/j.ymthe.2017.01.011
207. McCune JM, Namikawa R, Kaneshima H, Shultz LD, Lieberman M, Weissman IL. The SCID-hu mouse: murine model for the analysis of human hematolymphoid differentiation and function. Science (1988) 241:1632–9. doi: 10.1126/science.241.4873.1632
208. Tammana S, Huang X, Wong M, Milone MC, Ma L, Levine BL, et al. 4-1BB and CD28 signaling plays a synergistic role in redirecting umbilical cord blood t cells against b-cell malignancies. Hum Gene Ther (2010) 21:75–86. doi: 10.1089/hum.2009.122
209. Dudley ME, Wunderlich JR, Robbins PF, Yang JC, Hwu P, Schwartzentruber DJ, et al. Cancer regression and autoimmunity in patients after clonal repopulation with antitumor lymphocytes. Science (2002) 298:850–4. doi: 10.1126/science.1076514
210. Jin CH, Xia J, Rafiq S, Huang X, Hu Z, Zhou X, et al. Modeling anti-CD19 CAR t cell therapy in humanized mice with human immunity and autologous leukemia. EBioMedicine (2019) 39:173–81. doi: 10.1016/j.ebiom.2018.12.013
211. Xia J, Hu Z, Yoshihara S, Li Y, Jin CH, Tan S, et al. Modeling human leukemia immunotherapy in humanized mice. EBioMedicine (2016) 10:101–8. doi: 10.1016/j.ebiom.2016.06.028
Keywords: humanized mice, PDX model, hematological malignancy, immune therapy, immune system
Citation: Lang Y, Lyu Y, Tan Y and Hu Z (2023) Progress in construction of mouse models to investigate the pathogenesis and immune therapy of human hematological malignancy. Front. Immunol. 14:1195194. doi: 10.3389/fimmu.2023.1195194
Received: 28 March 2023; Accepted: 27 July 2023;
Published: 14 August 2023.
Edited by:
Chunhua Song, The Ohio State University, United StatesReviewed by:
Marta Serafini, Fondazione Matilde Tettamanti Menotti De Marchi, ItalyNatalia Baran, University of Texas MD Anderson Cancer Center, United States
Linghua Zheng, The Ohio State University, United States
Copyright © 2023 Lang, Lyu, Tan and Hu. This is an open-access article distributed under the terms of the Creative Commons Attribution License (CC BY). The use, distribution or reproduction in other forums is permitted, provided the original author(s) and the copyright owner(s) are credited and that the original publication in this journal is cited, in accordance with accepted academic practice. No use, distribution or reproduction is permitted which does not comply with these terms.
*Correspondence: Zheng Hu, emhlbmdodUBqbHUuZWR1LmNu; Yehui Tan, eWh0YW5Aamx1LmVkdS5jbg==