- 1Laboratory for Diagnostics of Zoonoses and World Health Organisation (WHO) Center, Institute of Microbiology and Immunology, Faculty of Medicine, University of Ljubljana, Ljubljana, Slovenia
- 2Laboratory for Cellular Immunology, Institute of Microbiology and Immunology, Faculty of Medicine, University of Ljubljana, Ljubljana, Slovenia
- 3Department of Infectious Diseases, University Medical Center Ljubljana, Ljubljana, Slovenia
Tick-borne encephalitis (TBE) is a viral infection of the human central nervous system caused by the TBE virus (TBEV). The most effective protective measure against TBE is vaccination. Despite the highly immunogenic vaccine, cases of vaccine breakthroughs (VBTs) occur. One of the first targets of infection is dendritic cells (DC), which represent a fundamental bridge between innate and adaptive immunity through antigen presentation, costimulation, and cytokine production. Therefore, we investigated the activation and maturation of DCs and cytokine production after in vitro TBEV stimulation of peripheral blood mononuclear cells (PBMCs) obtained from VBT and unvaccinated TBE patients. Our results showed that the expression of HLA-DR and CD86 on DCs, was upregulated to a similar extent in both vaccinated and unvaccinated TBE patients but differed in cytokine production after stimulation with TBEV. PBMCs from patients with VBT TBE responded with lower levels of IFN-α and the proinflammatory cytokines IL-12 (p70) and IL-15 after 24- and 48-hour in vitro stimulation with TBEV, possibly facilitating viral replication and influencing the development of cell-mediated immunity. On the other hand, significantly higher levels of IL-6 in addition to an observed trend of higher expression of TNF-α measured after 6 days of in vitro stimulation of PBMC could support disruption of the blood–brain barrier and promote viral and immune cell influx into the CNS, leading to more severe disease in VBT TBE patients.
1 Introduction
Tick-borne encephalitis (TBE) is a disease of the central nervous system (CNS) caused by the tick-borne encephalitis virus (TBEV). TBEV is usually transmitted through an infected tick bite, but humans can also be infected by consumption of unpasteurized milk or dairy products from infected livestock (1, 2). The majority of infections with the European subtype of TBEV (Eu-TBEV) are asymptomatic, whereas 56% to 87% of clinically manifested infections follow a biphasic course (3–7).
Currently there is no etiologic therapy for TBE; treatment is mainly supportive. The most effective protection against TBE is regular vaccination, which successfully promotes the formation of neutralizing antibodies in 98% to 99.5% of vaccinated people (8, 9). Nevertheless, 39 TBE vaccine breakthrough (VBT) cases were reported between 2000 and 2015 in Slovenia (10). In patients with VBT TBE, the onset of neurological symptoms is accompanied by high levels of specific and neutralizing IgG, whereas IgM antibodies are absent or present only in low levels (10–12). This indicates an anamnestic immune response, but one that is likely insufficient to prevent disease. Additionally, VBT TBE patients have significantly elevated levels of proinflammatory cytokines upon admission to the hospital, specifically those associated with blood-brain barrier (BBB) impairment. This finding suggests that immune cell infiltration into the central nervous system (CNS) and the development of intrathecal inflammation may be involved in the pathogenesis of VBT TBE (13).
An adequate immune response in the early stages of infection significantly limits viral replication and spread (14, 15). Some of the first cells that come into contact with TBEV after a tick bite are thought to be dendritic cells (DCs) (16), which could thus facilitate viral spread throughout the body. Antigen uptake by DCs and its recognition by pathogen recognition receptors (PRR) triggers DC maturation and activation, leading to increased expression of the surface activation markers HLA-DR and CD86 as well as increased secretion of specific cytokines (17). Because mature DCs are responsible for initiating several antiviral defense mechanisms, including T cell activation and proliferation through antigen presentation, costimulation, and cytokine secretion, as well as isotype switching and B cell differentiation into antibody-secreting plasma cells (18, 19), they represent an important link between the innate and adaptive immune system. As the infection of DCs by several other flaviviruses—including Japanese encephalitis virus (20), dengue virus (DENV) (21), and West Nile virus (WNV) (22)—results in altered DC maturation and T cell proliferation, we hypothesize that viral interaction with DCs may potentially also affect TBE pathogenesis in VBT patients.
To gain a better understanding of the events in TBE pathogenesis, we stimulated PBMCs obtained from vaccinated and unvaccinated TBE patients with TBEV in vitro and measured DC activation through expression of activation and maturation markers and proinflammatory cytokine production.
2 Materials and methods
2.1 Study design and subjects
All TBE patients enrolled in our study were diagnosed between January 2003 and December 2018 at the Department of Infectious Diseases, University Medical Center Ljubljana, Slovenia. Following disease remission, patients were invited for a follow-up examination in which 15 ml of peripheral whole blood was collected for isolation of PBMCs. Of them, 10 VBT TBE patients that had received complete basic vaccination with or without booster doses and without delays in administration and 10 sex- and age-matched unvaccinated patients diagnosed with TBE in the same year were included in our study. Characteristics of TBE patients enrolled in our study are shown in Table 1.
PBMCs were isolated from blood samples with ethylenediaminetetraacetic acid (EDTA) using Ficoll-Paque PLUS (GE Healthcare, Uppsala, Sweden) density gradient centrifugation. Briefly, whole blood was mixed with Roswell Park Memorial Institute (RPMI) 1640 Medium in a 1:2 ratio and centrifuged in Leucosep tubes (Greiner Bio-One, GmbH, Germany) containing Ficoll-Paque PLUS at 645 × g for 10 minutes at 21 °C without brake. The buffy coat containing the PBMCs was collected, washed twice in 10 ml of fresh RPMI-1640 and resuspended and stored in RPMI-1640 (10%) + FCS (80%) + dimethyl sulfoxide (DMSO; 10%) before being aliquoted and stored at −80 °C until further use.
2.2 Virus preparation
TBEV, Ljubljana strain (EVA-GLOBAL-Ref-SKU: 007V-02198), was used as the stock virus. To prepare the virus, the VERO E6 cell line was cultured in complete Dulbecco’s modified Eagle’s medium (DMEM; GIBCO, Invitrogen, Massachusetts, United States), supplemented with 10% fetal bovine serum (FBS). Prior to inoculation, the cell supernatant was removed, and 1 ml of TBEV was transferred onto 24-hour-old VERO E6 cells (Vero 76, clone E6; ATCC-LGC, Virginia, USA), with 80% confluency. The cells were incubated for one hour at 37 °C and 5% CO2, followed by the addition of 10 ml of DMEM supplemented with 4% FBS. The cells were further incubated for seven days under the same conditions.
After the incubation period, the supernatant was transferred to a 15 ml tube. The remaining adherent cells were detached using glass beads in 5 ml of filtered saline solution supplemented with 5% FBS. The virus was then centrifuged using Amicon Ultra Centrifugal Filter Units with an ultrafiltration membrane (Merck KgaA, Darmstadt, Germany) as per the manufacturer’s instructions. The virus was transferred to the centrifugal filter unit and centrifuged at 4,000 × g for 30 minutes. The ultrafiltration membrane was then transferred to a new tube, 15 ml of RPMI-1640 medium was added, and the centrifugation procedure was repeated. The virus was resuspended in 30 ml of RPMI-1640 medium and stored in 1 ml aliquots at −80 °C until further use.
2.3 Virus quantification
Prepared TBEV stock was quantified using the median tissue culture infectious dose (TCID50) assay on VERO E6 cells. Briefly, TBEV was serially diluted (10-fold) in Dulbecco’s Modified Eagle Medium (DMEM) with 10% FBS and 50 μl of each dilution was added to VERO E6 cells in a 96-well cell culture plate, except for the last three columns which represented a negative control. After 7 days of incubation at 37 °C and 5% CO2, the cells were fixed with 100 μl of 4% formaldehyde and stained with 1% crystal violet. The TCID50 was calculated using the Spearman-Kärber method based on the dilution at which 50% of wells showed cytopathic effects.
2.4 In vitro stimulation of PBMCs with TBEV
On the day of the experiment, cryotubes containing PBMCs were thawed in a 37 °C water bath. The PBMCs were transferred to 15 ml tubes and warm RPMI-1640 supplemented with 10% inactivated human serum (IHS; Merck KgaA, Darmstadt, Germany) was slowly added with constant stirring. After centrifugation at 287 × g for 10 minutes at 21 °C, the cells were washed twice with 10% IHS in RPMI-1640, and then resuspended in 10% IHS in RPMI-1640. Cell viability was determined using trypan blue on the Countess Automated Cell Counter (Thermo Fisher Scientific, Carlsbad, CA, USA).
A working concentration of 2 × 106 viable cells/ml was prepared in RPMI-1640 containing 10% IHS (Merck KgaA, Darmstadt, Germany), 1% L-glutamine (Thermo Fisher Scientific, Carlsbad, CA, USA), 1% penicillin (100 U/ml), and 1% streptomycin (0.1 mg/ml), and 500 μl of cell suspension was seeded into 24-well cell culture plates. PBMCs were stimulated with TBEV with multiplicity of infection (MOI) 2 for 24 hours, 48 hours, and 6 days at 37 °C and 5% CO2. Unstimulated PBMCs from each individual at each time point represented the negative control.
At each stimulation time point, PBMCs were harvested and centrifuged at 287 × g for 5 min at room temperature to separate the supernatant for cytokine analysis. The supernatant was stored at −80 °C for cytokine analysis, and the pellet was resuspended in 250 μl of cell culture medium. Adherent cells were detached using ice-cold 2 mM EDTA in PBS, centrifuged at 287 × g for 5 min at room temperature and combined with the harvested cells from the first tube. The cells were thoroughly mixed, and the samples were immediately prepared for flow cytometric analysis.
2.5 Immunostaining and flow cytometry analysis
Before immunostaining, the viability of stimulated and unstimulated PBMCs was measured with trypan blue on the Countess II Automated Cell Counter (Thermo Fisher Scientific, Carlsbad, CA, USA) according to the manufacturer’s instructions. Fluorescently labeled monoclonal antibodies (mAb) to cell surface antigens used for immunostaining of PBMCs included PE-conjugated mouse anti-human CD86 (clone 2331), PerCP Cy5.5-conjugated mouse anti-human CD3 (clone SK7), CD14 (clone M5E2), CD16 (clone 3G8) and CD19 (clone SJ25C1), PE Cy7-conjugated mouse anti-human CD123 (clone 7G3), APC-conjugated mouse anti-human CD11c (clone MJ4-27G12), APC Cy7-conjugated mouse anti-human HLA-DR (clone L243), and BV510-conjugated mouse anti-human CD1c (clone F10/21A3), purchased from BD Biosciences (San Jose, CA, USA), whereas FITC-conjugated mouse anti-human CD303 (clone AC144) and ViolBlue-conjugated mouse anti-human CD141 (clone AD5-14H12) were purchased from Miltenyi Biotec (Bergisch Gladbach, Germany). A mAb combination was added to a 150 μl PBMC suspension collected 24 and 48 hours after TBEV stimulation and incubated for 20 min in the dark. The cells were then fixed using 2 ml of lysis buffer (Becton Dickinson, Franklin Lakes, NJ, USA) for 10 min in the dark. After 5 min centrifugation at 287 × g, PBMCs were washed twice with PBS and resuspended in 250 μl of PBS. Samples were acquired on a FACS Canto II flow cytometer (BD Biosciences, San Jose, CA, USA) and the results were analyzed with FACS Diva software (BD Biosciences, San Jose, CA, USA).
A minimum of 100,000 events were recorded with the flow cytometer. Doublets and dead cells were eliminated based on forward/side scatter plots, and DCs were gated based on high HLA-DR expression and the absence of surface expression of lineage markers CD3, CD14, CD16, and CD19. From the population of DCs, subpopulations were further divided based on the expression of specific surface. The combination of CD11c and CD141 was used to determine cDC1, CD11c and CD1c for cDC2, and CD123 and CD303 for pDC. FMO controls were included to define the negative gates for selected populations. The median fluorescence intensity (MFI) of HLA-DR and CD86 on each subpopulation of DCs was analyzed and served as a marker for activation after in vitro stimulation with TBEV. The gating strategy and fluorescence minus one (FMO) controls are shown in Supplementary Figure 1.
2.6 Cytokine quantification with a multiplex immunoassay
Cytokine concentrations in PBMC supernatants were measured using commercially available kits for the detection of soluble immune mediators: Human Cytokine/Chemokine Magnetic Bead Panel and Human Cytokine/Chemokine Magnetic Bead Panel IV (Millipore, Darmstadt, Germany) for the Luminex platform on a Magpix instrument (Luminex, Austin, TX, USA) according to the manufacturer’s instructions. The immunoassays detect IFN-α, IFN-β, IL-1β, IL-6, IL-12 (p70), IL-15, and TNF-α. Briefly, cell culture supernatants were thawed, centrifuged at 1,000 × g for 5 min, and diluted 1:3 with Assay Buffer (Millipore, Germany) provided in the multiplex assay kits. All measurements with a single panel were performed on the same day in one complete experiment to minimize inter-assay variation. Data analysis was performed using Milliplex Analyst 5.1 software. All values outside the upper and lower ends of the standard curve were considered maximum and minimum values, respectively.
2.7 Statistical analysis
Differences between two independent groups were analyzed using the Mann–Whitney test in GraphPad Prism 7. Each group was first compared to a negative control to determine the presence of a response, and subsequently, the extent of the response was compared between the two groups. Values with p ≤ 0.05 were interpreted as statistically significant; statistical significance levels are indicated by the number of asterisks: *p ≤ 0.05, **p ≤ 0.01, ***p ≤ 0.001, and ****p ≤ 0.0001.
3 Results
3.1 DCs increase expression of HLA-DR and CD86 after TBEV stimulation in vitro
Due to nonspecific symptoms in the first phase of the disease, the early immune response to TBEV infection is poorly investigated. To investigate the activation status of DC after encountering TBEV, we stimulated PBMCs from VBT and unvaccinated TBE patients in vitro with TBEV (MOI 2) for 24 and 48 hours and measured the expression of the activation and maturation markers HLA-DR and CD86 on specific DC subpopulations.
As shown in Figure 1, cDC1 from both VBT as well as unvaccinated TBE patients had statistically significant higher HLA-DR MFI at 24 and 48 hours after stimulation with TBEV, whereas only cDC2 from unvaccinated patients had significantly higher HLA-DR expression compared to unstimulated cells. On the other hand, despite the observed trend of higher HLA-DR MFI on stimulated pDC from both groups of patients, the difference compared to unstimulated cells was statistically significant only in VBT TBE patients.
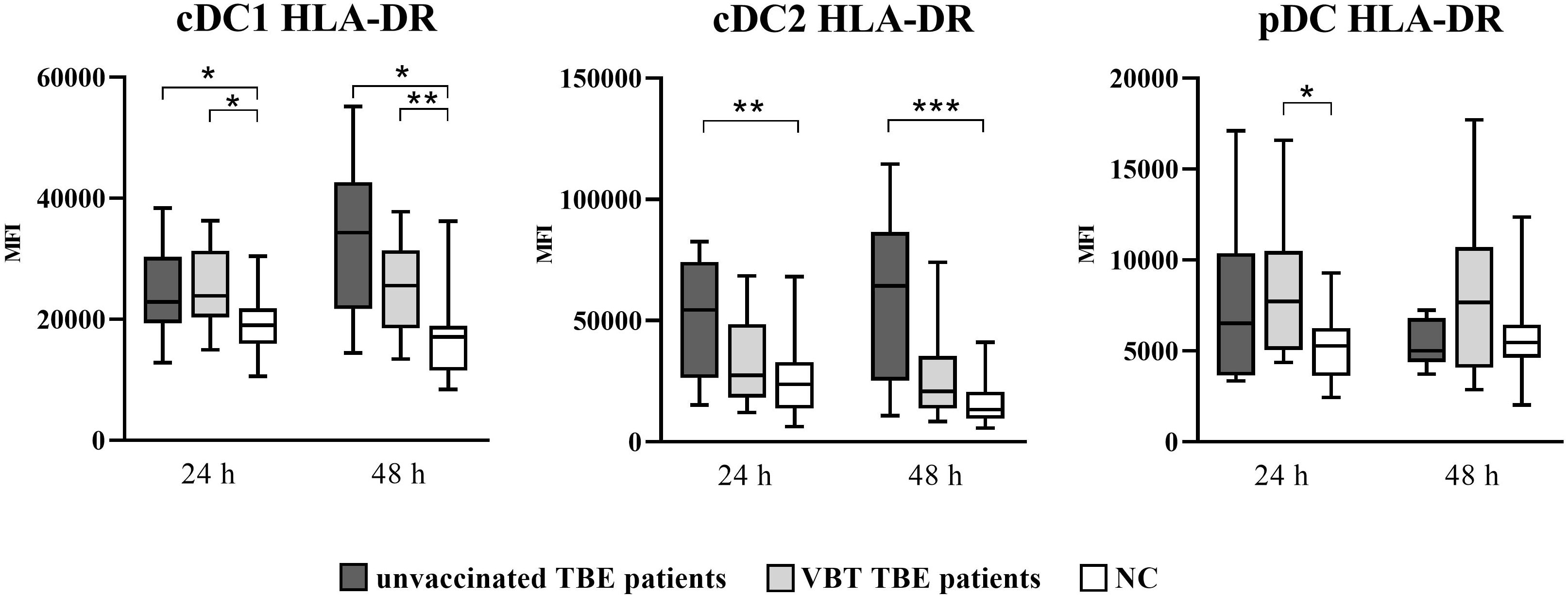
Figure 1 Expression of the activation marker HLA-DR on cDC1, cDC2, and pDC after stimulation with TBEV. To analyze the activation status of DC subpopulations upon in vitro TBEV stimulation, PBMCs were stimulated with TBEV and MOI 2, stained with mAb binding the activation/maturation marker HLA-DR for 24 and 48 hours, and analyzed with flow cytometry. The graphs show the MFI value of the respective marker. The box and whisker plots show the median (horizontal line), quartiles (box) and the whiskers, which extend to the most extreme data point. Only statistically significant differences are shown. *p ≤ 0.05, **p ≤ 0.01, and ***p ≤ 0.001. NC, negative control; MFI, median fluorescence intensity; h, hour.
Similarly, upregulation of CD86 was also observed on DC after 24- and 48-hour in vitro stimulation with TBEV, with significantly higher CD86 MFI detected on cDC1 and cDC2 of both patient groups (Figure 2). In addition, the expression of CD86 was significantly higher at 24 hours on cDC2 of VBT patients compared to nonvaccinated TBE patients. On the other hand, a significantly higher CD86 MFI on pDC compared to unstimulated cells was observed only in VBT patients.
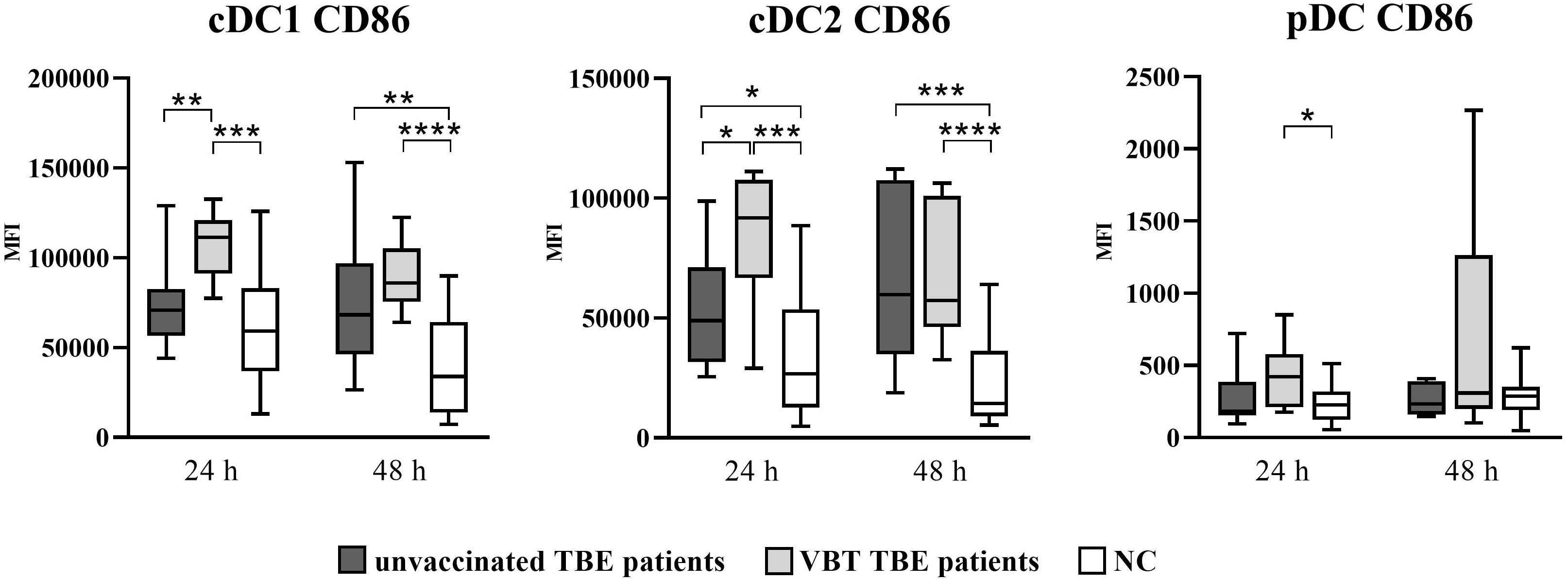
Figure 2 Expression of the activation marker CD86 on cDC1, cDC2, and pDC after stimulation with TBEV. To analyze the activation status of DC subpopulations after in vitro TBEV stimulation, PBMCs were stimulated with TBEV MOI 2 for 24 and 48 hours, stained for the activation/maturation marker CD86, and analyzed with flow cytometry. The graphs show the MFI value of the respective marker. The box and whisker plots show the median (horizontal line), quartiles (box) and the whiskers, which extend to the most extreme data point. Only statistically significant differences are shown. *p ≤ 0.05, **p ≤ 0.01, ***p ≤ 0.001, and ****p ≤ 0.0001. NC, negative control; MFI, median fluorescence intensity; h, hour.
Thus, TBEV stimulation of PBMC in vitro resulted in significant DC activation observed by profound upregulation of surface activation molecules, especially on cDC1 and cDC2 from both groups of TBE patients, indicating possible involvement of DC in the early immune response during TBEV stimulation.
3.2 Differential cytokine expression in vitro after TBEV stimulation
To investigate the innate immune response to TBEV at early stages of pathogenesis, we analyzed the production of the pro-inflammatory cytokines IFN-α, IL-1β, IL-6, IL-12 (p70), IL-15, and TNF-α after in vitro stimulation of PBMCs. PBMCs were stimulated with TBEV (MOI 2) for 24 hours, 48 hours, and 6 days. At each time point, supernatants were collected and cytokines were analyzed with a multiplex immunoassay on a Magpix instrument. Supernatants from untreated cells served as negative controls.
As early as 24 hours after stimulation with TBEV in vitro, statistically significant upregulation of IFN-α, IL-12 (p70), IL-15, and TNF-α was observed in the supernatants of stimulated PBMCs from VBT and unvaccinated patients compared to unstimulated cells (Figure 3). In addition, IL-15 and TNF-α remained significantly elevated after 48-hour PBMC stimulation with TBEV compared to unstimulated cells from both groups of patients. Secretion of IFN-α and IL-12 (p70) was not significantly increased in stimulated PBMCs from VBT patients, whereas it was significantly higher in stimulated PBMCs from unvaccinated patients compared to unstimulated cells. Moreover, the concentrations of IFN-α, IL-12 (p70), and IL-15 measured in PBMC cultures from VBT patients at 48 hours and 6 days were significantly lower compared to unvaccinated patients. In addition, IL-6 was upregulated in the supernatants of stimulated PBMCs from both patient groups at all time points and was significantly higher compared to unstimulated cells 48 hours and 6 days post stimulation. However, PBMCs from VBT patients produced significantly higher levels of IL-6 than in unvaccinated patients after 6 days of in vitro stimulation. A trend toward increased TNF-α was also observed in the supernatants of stimulated PBMC from VBT patients at all time points, especially 6 days after stimulation, whereas the concentration of IL-1β did not increase in PBMC cultures from both groups of TBE patients.
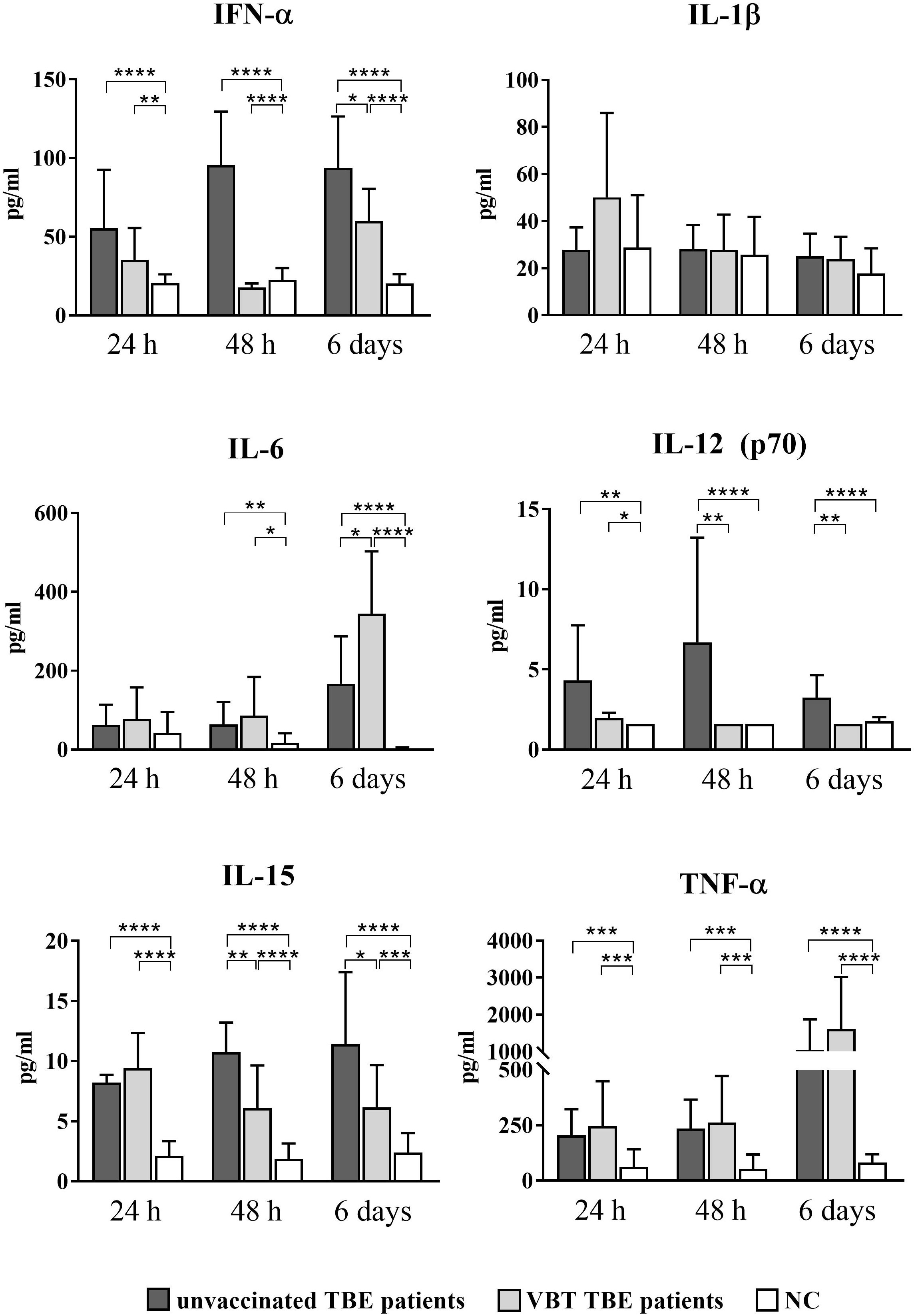
Figure 3 Cytokine expression after stimulation of PBMCs from VBT and unvaccinated TBE patients with TBEV. Supernatants of PBMC were harvested 24 hours, 48 hours, and 6 days post stimulation, and induction of IFN-α, IL-1β, IL6, IL-12 (p70), IL-15, and TNF-α was analyzed with a multiplex immunoassay. Untreated cells served as negative control (NC). The graphs show the concentration of each cytokine in pg/ml. The column plots show the mean with SD. Only statistically significant differences are shown. *p ≤ 0.05, **p ≤ 0.01, ***p ≤ 0.001, and ****p ≤ 0.0001.
Lower IFN-α, IL-12 (p70), and IL-15 levels measured 24 hours and 48 hours after in vitro stimulation of PBMCs indicate a weaker innate immune response early after TBEV contact, whereas higher expression of IL-6 and TNF-α at 6 days suggests a more extensive inflammatory response in the late stages of TBEV encounter in VBT patients compared to unvaccinated TBE patients.
4 Discussion
TBE is a disease of the CNS caused by TBEV. Because no etiologic treatment for TBE is available, the most effective protection against TBE is regular vaccination. Although the vaccine is highly immunogenic, vaccine breakthrough TBE cases have been reported in several European countries, including Slovenia (10). Despite increasing evidence of immune system involvement in immunopathological manifestations of TBE, a sufficient immune response in the early stages of infection importantly suppresses viral replication and spread (14, 15). Some of the first cells encountering TBEV during natural infection are dendritic cells, which represent an important link between innate and adaptive immunity. To gain insight in the pathogenesis of TBE, we stimulated PBMCs from vaccinated and unvaccinated TBE patients in vitro with TBEV and measured DC cell activation by expression of activation and maturation markers and cytokine production.
We found that in vitro TBEV stimulation of PBMCs from vaccinated and unvaccinated TBE patients increased the expression of the activation molecules HLA-DR and CD86 on DCs, particularly on a subpopulation of cDCs. cDC cells are the main antigen-presenting cells capable of recognizing both viral single- and double-stranded RNA via Toll-like receptors (TLR) 3 and 8, resulting in differential cytokine secretion leading to T cell proliferation and activation. In addition to producing IFN I, cDC1 cells are also known to produce large amounts of IFN III, whereas cDC2 cells produce higher amounts of the inflammatory cytokines IL-6, IL-8, and TNF-α (17). In both groups of TBE patients, cDC1 cells displayed a mature phenotype by significantly increasing the expression of HLA-DR and CD86 compared to unstimulated cells. On the other hand, we observed a significantly higher CD86 MFI on cDC2 from vaccinated TBE patients compared to unvaccinated patients 24 hours post stimulation, indicating a more extensive costimulatory function of this subpopulation of cells in vaccinated patients during the early phase of the anti-viral immune response. However, in contrast to unvaccinated TBE patients, we did not observe a statistically significant difference in HLA-DR MFI on stimulated cDC2 from vaccinated compared to unstimulated PBMCs. Our findings indicate that although CD86 expression on cDC2 is significantly increased in VBT TBE patients, this cell subset’s antigen presentation function may be impaired, potentially hindering the priming of T cells and promoting viral pathogenesis. Studies have shown that several flaviviruses, including JEV (20), DENV (21), WNV (22), and LGTV (23), have been found to impair DC maturation, with immature DCs showing reduced capacity to induce T cell proliferation. As HLA-DR upregulation is observed in unvaccinated patients, we hypothesize that reduced HLA-DR expression on cDC2s in vaccinated patients may be a characteristic of this group, rather than a consequence of viral manipulation of DC function. However, to fully comprehend the capabilities of cDC2s in inducing T cell priming and proliferation among vaccinated TBE patients, more studies are necessary. Similarly, it remains to be investigated whether cDC1 cells from VBT patients may be able to compensate for the diminished antigen presentation by cDC2 cells and play a dominant role in this regard.
Additionally, VBT patients responded with significantly lower concentrations of IFN-α, IL-12p70, and IL-15 compared to unvaccinated patients 48 hours after the initiating stimulation. IFN-α plays a central role in inhibiting viral replication through interferon-stimulated genes (ISG) with antiviral activity (24, 25), as well as by activating other immune cells and inducing secretion of proinflammatory cytokines (26). The absence of an IFN I response in mice infected with Langat virus (LGTV) resulted in uncontrolled viral replication in all organs, early disruption of BBB, and a fatal outcome (27). Similar to other flaviviruses, TBEV has developed various evasion strategies to circumvent the host immune system. One of them is the active reduction of IFN I production, which, however, is limited to the first 24 hours of infection (28, 29). Therefore, we hypothesize that a reduced early IFN-α response is a characteristic of VBT patients that could lead to higher viral load and viral dissemination, contributing to earlier neuroinvasion and onset of disease symptoms.
IFN-α, in combination with IL-6 and BAFF, also promotes differentiation of B cells into plasma cells and antibody production after stimulation with inactivated TBEV in vitro (30). The amount of IFN-α production has been shown to influence the antibody response to yellow fever vaccine (31) and influenza vaccine (32). Older individuals develop a lower antibody response after vaccination against TBE (33), and antibody levels induced by TBE vaccination decline more rapidly over time compared to younger individuals (34–37). Patients with VBT TBE are usually older and often develop illness before the recommended time for booster vaccination (10). Therefore, the lower IFN-α response observed upon stimulation of PBMCs obtained from patients with VBT TBE could potentially be a contributing factor to an inadequate antibody response following TBE vaccination and shorter protection against the disease than expected. Nonetheless, vaccinated TBE patients develop a more robust antibody response after infection with TBEV in comparison to unvaccinated patients (10–12), indicating that vaccination induced the formation of memory B cells that differentiate into antibody-producing plasma cells after exposure to TBEV. However, the immune response in VBT patients was presumably not fast or effective enough to prevent disease.
Upon activation, DCs also upregulate the production of several proinflammatory cytokines, including IL-6, IL-12, IL-15, and TNF-α (17). In this study, PBMCs obtained from patients with VBT TBE responded with lower IL-12 (p70) and IL-15 production after 2 and 6 days of stimulation with TBEV compared to unvaccinated patients, suggesting a weaker functional capacity of DCs in VBT patients in the early stages of TBEV stimulation. Both IL-12 and IL-15 are known activators of NK cells, which control infection through the secretion of perforin and granzyme B and production of IFN-γ and TNF-α (38). Blom et al. (39) have shown that TBEV likely triggers NK cell activation at an earlier stage of infection because elevated Ki67 levels in NK cells were associated with elevated levels of IFN-γ and TNF-α in plasma from TBE patients. In addition, NK cells are thought to significantly influence the course and outcome of the disease caused by other flaviviruses, including Dengue virus (40, 41), West Nile virus (42), and Japanese encephalitis virus (43), as well as vaccination with live attenuated yellow fever virus (44). Therefore, lower expression of IL-12 (p70) and IL-15 in VBT patients could lead to lower NK cell activation, promoting viral dissemination at an early stage of infection. However, to better understand the involvement of NK cells in VBT TBE, further studies investigating the activation and effector function of this immune cell subset are needed.
In vitro stimulation of PBMCs with TBEV showed that, in addition to lower proinflammatory response, there was a marked upregulation of the proinflammatory cytokines IL-6 and TNF-α in VBT patients compared to unvaccinated patients after 6 days of PBMC stimulation in vitro, which was statistically significant in the case of IL-6. Although the production of IL-6 is generally associated with a protective immune response, it could also contribute to an increased inflammatory response and disease progression. Elevated levels of IL-6 have been measured in clinical samples from patients that developed a more severe form of Japanese encephalitis (45). Excessive synthesis of IL-6 in combination with other inflammatory mediators such as TNF-α is thought to lead to increased BBB permeability and neuronal damage (46–49). The more extensive disruption of the BBB could facilitate the entry of virus and immune cells to the CNS and consequently contribute to a more severe manifestation of the disease in VBT patients.
Our in vitro results show that VBT and unvaccinated TBE patients upregulate the expression of activating molecules on DCs, particularly cDCs, to a similar extent, but differ in cytokine production after stimulation with TBEV. PBMCs from VBT patients responded with lower levels of IFN-α and the proinflammatory cytokines IL-12 (p70) and IL-15 after in vitro stimulation, which could facilitate viral replication and lead to poorer activation of other immune cells. On the other hand, the measured elevated levels of IL-6 as well as observed trend of higher expression of TNF-α after 6 days of PBMC stimulation could possibly contribute to disruption of the BBB and facilitate the influx of TBEV and immune cells into the CNS, which in turn could contribute to a more severe disease.
Although this study has provided valuable insight into the immune response of VBT TBE patients, certain limitations remain. Specifically, the lack of samples from VBT patients prior to their encounter with TBE virus and subsequent development of TBE impedes our ability to fully understand the immune status of these individuals at the time of tick bite. There is a limited number of samples available from VBT patients in this study, which may limit the generalizability of the findings. Additionally, the inclusion of a control group consisting of vaccinated individuals who have been exposed to the virus but remain asymptomatic would provide crucial information regarding the immune system’s ability to combat TBEV. However, practical constraints make it difficult to obtain blood samples from such individuals, as they are unlikely to seek medical attention. Moreover, it should be emphasized that investigating other cell types, in addition to dendritic cells, may be crucial to gain a complete understanding of the immune response to TBEV and the mechanisms involved in VBT TBE.
Despite these limitations, this study is the first and only VBT TBE study to date, and the samples collected are extremely rare and valuable. The findings of this study provide important insights into the immune response of individuals who develop VBT TBE, which can inform future research and potentially lead to the development of more effective treatments and prevention strategies of TBE.
Data availability statement
The original contributions presented in the study are included in the article/Supplementary Material. Further inquiries can be directed to the corresponding author.
Ethics statement
The studies involving human participants were reviewed and approved by National Medical Ethics Committee (approval no. 0120-564/2018/13). The patients/participants provided their written informed consent to participate in this study.
Author contributions
MM, MK, NK and TAZ designed the study. PB and FS included patients in the study and obtained clinical information. MM performed the experiments and formal analysis. MM, MK, NK, TAZ, ANK, AI, PB, and FS performed review and editing. MM performed the data analysis and interpretation. MM wrote the original draft. MM, MK, NK, TAZ, ANK, AI, PB and FS performed review and editing. All authors contributed to the article and approved the submitted version.
Funding
This work was supported by the Slovenian Research and Innovation Agency (grant numbers P3-0083 and P3-0296), the European Union’s Horizon 2020 research and innovation program - EVA GLOBAL project (Grant agreement no. 871029) and Network of infrastructure Centres of University of Ljubljana (MRIC-UL-IC-BSL3+). This publication reflects only the author’s view and the Commission is not responsible for any use that may be made of the information it contains.
Conflict of interest
The authors declare that the research was conducted in the absence of any commercial or financial relationships that could be construed as a potential conflict of interest.
Publisher’s note
All claims expressed in this article are solely those of the authors and do not necessarily represent those of their affiliated organizations, or those of the publisher, the editors and the reviewers. Any product that may be evaluated in this article, or claim that may be made by its manufacturer, is not guaranteed or endorsed by the publisher.
Supplementary material
The Supplementary Material for this article can be found online at: https://www.frontiersin.org/articles/10.3389/fimmu.2023.1190803/full#supplementary-material
Abbreviations
BBB, blood-brain barrier; CNS, central nervous system; CSF, cerebrospinal fluid; CD, cluster of differentiation; DC, dendritic cell; HLA-DR, major histocompatibility complex class II cell surface molecule; IFN, interferon; Ig, immunoglobulin; IL, interleukin; TLR, toll-like receptor; TNF, tumor necrosis factor; TBE, tick-borne encephalitis; TBEV, tick-borne encephalitis virus; VBT, vaccine breakthrough.
References
1. Lindquist L, Vapalahti O. Tick-borne encephalitis. Lancet (2008) 371:18611871. doi: 10.1016/S0140-6736(08)60800-4
2. Hudopisk N, Korva M, Janet E, Simetinger M, Grgič-Vitek M, Gubenšek J, et al. Tick-borne encephalitis associated with consumption of raw goat milk, Slovenia. Emerg Infect Dis (2012) 19(5):806–8. doi: 10.3201/eid1905.121442
3. Gustafson R, Svenungsson B, Forsgren M, Gardulf A, Granström M. Two-year survey of the incidence of Lyme borreliosis and tick-borne encephalitis in a high-risk population in Sweden. Eur J Clin Microbiol Infect Dis (1992) 11(10):894–900. doi: 10.1007/BF01962369
4. Tomažič J, Pikelj F, Schwartz B, Kunze M, Kraigher A, Matjašiš M, et al. The clinical features of tick-borne encephalitis in slovenia. a study of 492 cases in 1994. Antibiot. Monitor (1996) 12:115–20. doi: 10.3201/eid1905.121442
5. Kaiser R. Tick-borne encephalitis. Infect Dis Clin North Am (2008) 22(3):561–75. doi: 10.1016/j.idc.2008.03.013
6. Bogovič P, Strle F. Tick-borne encephalitis: a review of epidemiology, clinical characteristics, and management. World J Clin cases (2015) 3(5):430–41. doi: 10.12998/wjcc.v3.i5.430
7. Kohlmaier B, Schweintzger NA, Sagmeister MG, Švendová V, Kohlfürst DS, Sonnleitner A, et al. Clinical characteristics of patients with tick-borne encephalitis (TBE): a European multicentre study from 2010 to 2017. Microorganisms (2021) 9(7):1420. doi: 10.3390/microorganisms9071420
8. Heinz FX, Holzmann H, Essl A, Kundi M. Field effectiveness of vaccination against tick-borne encephalitis. Vaccine (2007) 25(43):7559–67. doi: 10.1016/j.vaccine.2007.08.024
9. Loew-Baselli A, Poellabauer EM, Pavlova BG, Fritsch S, Firth C, Petermann R, et al. Prevention of tick-borne encephalitis by FSME-IMMUN vaccines: review of a clinical development programme. Vaccine (2011) 29(43):7307–19. doi: 10.1016/j.vaccine.2011.07.089
10. Lotric-Furlan S, Bogovič P, Avšič-Županc T, Jelovšek M, Lusa L, Strle F. Tick-borne encephalitis in patients vaccinated against this disease. J Intern Med (2017) 282:142–55. doi: 10.1111/joim.12625
11. Stiasny K, Holzmann H, Heinz FX. Characteristics of antibody responses in tick-borne encephalitis vaccination breakthroughs. Vaccine (2009) 27(50):7021–6. doi: 10.1016/j.vaccine.2009.09.069
12. Andersson CR, Vene S, Insulander M, Lindquist L, Lundkvist A, Günther G. Vaccine failures after active immunisation against tick-borne encephalitis. Vaccine (2010) 28:2827–31. doi: 10.1016/j.vaccine.2010.02.001
13. Pavletič M, Korva M, Knap N, Bogovič P, Lusa L, Strle K, et al. Upregulated intrathecal expression of VEGF-a and long lasting global upregulation of proinflammatory immune mediators in vaccine breakthrough tick-borne encephalitis. Front Cell Infect Microbiol (2021) 11:696337. doi: 10.3389/fcimb.2021.696337
14. King NJ, Getts DR, Getts MT, Rana S, Shrestha B, Kesson AM. Immunopathology of flavivirus infections. Immunol Cell Biol (2007) 85(1):33–42. doi: 10.1038/sj.icb.7100012
15. Lindqvist R, Mundt F, Gilthorpe JD, Wölfel S, Gekara NO, Kröger A, et al. Fast type I interferon response protects astrocytes from flavivirus infection and virus-induced cytopathic effects. J Neuroinflamm (2016) 13(1):277. doi: 10.1186/s12974-016-0748-7
16. Labuda M, Austyn JM, Zuffova E, Kozuch O, Fuchsberger N, Lysy J, et al. Importance of localized skin infection in tick-borne encephalitis virus transmission. Virology (1996) 219(2):357–66. doi: 10.1006/viro.1996.0261
17. Collin M, Bigley V. Human dendritic cell subsets: an update. Immunology (2018) 154(1):3–20. doi: 10.1111/imm.12888
18. Van Gool SW, Vandenberghe P, de Boer M, Ceuppens JL. CD80, CD86 and CD40 provide accessory signals in a multiple-step T-cell activation model. Immunol Rev (1996) 153:47–83. doi: 10.1111/j.1600-065X.1996.tb00920.x
19. Iwasaki A, Medzhitov R. Toll-like receptor control of the adaptive immune responses. Nat Immunol (2004) 5(10):987–95. doi: 10.1038/ni1112
20. Cao S, Li, Y, Ye, J, Yang, X, Chen, L, Liu, X, et al. Japanese Encephalitis virus wild strain infection suppresses dendritic cells maturation and function, and causes the expansion of regulatory T cells. Virol J (2011) 8:39. doi: 10.1186/1743-422X-8-39
21. Nightingale ZD, Patkar, C, Rothman, AL. Viral replication and paracrine effects result in distinct, functional responses of dendritic cells following infection with dengue 2 virus. J Leukoc. Biol (2008) 84:1028–38. doi: 10.1189/jlb.0208105
22. Qian F, Wang, X, Zhang L., Lin, A, Zhao, H, Fikrig E., et al. Impaired interferon signaling in dendritic cells from older donors infected in vitro with West Nile virus. J Infect Dis (2011) 203:1415–24. doi: 10.1093/infdis/jir048
23. Robertson SJ, Lubick KJ, Freedman BA, Carmody AB, Best SM. Tick-borne flaviviruses antagonize both IRF-1 and type I IFN signaling to inhibit dendritic cell function. J Immunol (2014) 192(6):2744–55. doi: 10.4049/jimmunol.1302110
24. Taylor RT, Lubick KJ, Robertson SJ, Broughton JP, Bloom ME, Bresnahan WA, et al. TRIM79α, an interferon-stimulated gene product, restricts tick-borne encephalitis virus replication by degrading the viral RNA polymerase. Cell Host Microbe (2011) 10(3):185–96. doi: 10.1016/j.chom.2011.08.004
25. Panayiotou C, Lindqvist R, Kurhade C, Vonderstein K, Pasto J, Edlund K, et al. Viperin restricts zika virus and tick-borne encephalitis virus replication by targeting NS3 for proteasomal degradation. J Virol (2018) 92(7):e02054–17. doi: 10.1128/JVI.02054-17
26. Haller O, Kochs G, Weber F. The interferon response circuit: induction and suppression by pathogenic viruses. Virology (2006) 344(1):119–30. doi: 10.1016/j.virol.2005.09.024
27. Weber E, Finsterbusch K, Lindquist R, Nair S, Lienenklaus S, Gekara NO, et al. Type I interferon protects mice from fatal neurotropic infection with langat virus by systemic and local antiviral responses. J Virol (2014) 88(21):12202–12. doi: 10.1128/JVI.01215-14
28. Carletti T, Zakaria MK, Marcello A. The host cell response to tick-borne encephalitis virus. Biochem Biophys Res Commun (2017) 492(4):533–40. doi: 10.1016/j.bbrc.2017.02.006
29. Signorazzi A, Pennings JLA, Etna MP, Noya M, Coccia EM, Huckriede A. In vitro characterization of the innate immune pathways engaged by live and inactivated tick-borne encephalitis virus. Vaccines (2021) 9(6):664. doi: 10.3390/vaccines9060664
30. Etna MP, Signorazzi A, Ricci D, Severa M, Rizzo F, Giacomini E, et al. Human plasmacytoid dendritic cells at the crossroad of type I interferon-regulated b cell differentiation and antiviral response to tick-borne encephalitis virus. PloS Pathog (2021) 17(4):e1009505. doi: 10.1371/journal.ppat.1009505
31. Querec TD, Akondy RS, Lee EK, Cao W, Nakaya HI, Teuwen D, et al. Systems biology approach predicts immunogenicity of the yellow fever vaccine in humans. Nat Immunol (2009) 10(1):116–25. doi: 10.1038/ni.1688
32. Nakaya HI, Wrammert J, Lee EK, Racioppi L, Marie-Kunze S, Haining WN, et al. Systems biology of vaccination for seasonal influenza in humans. Nat Immunol (2011) 12(8):786–95. doi: 10.1038/ni.2067
33. Stiasny K, Aberle JH, Keller M, Grubeck-Loebenstein B, Heinz FX. Age affects quantity but not quality of antibody responses after vaccination with an inactivated flavivirus vaccine against tick-borne encephalitis. PloS One (2012) 7:e34145. doi: 10.1371/journal.pone.0034145
34. Hainz U, Jenewein B, Asch E, Pfeiffer KP, Berger P, Grubeck-Loebenstein B. Insufficient protection for healthy elderly adults by tetanus and TBE vaccines. Vaccine (2005) 23(25):3232–5. doi: 10.1016/j.vaccine.2005.01.085
35. Loew-Baselli A, Poellabauer EM, Pavlova BG, Fritsch S, Koska M, Bobrovsky R, et al. Seropersistence of tick-borne encephalitis antibodies, safety and booster response to FSME-IMMUN 0.5 ml in adults aged 18–67 years. Hum Vaccin. (2009) 5(8):551–6. doi: 10.4161/hv.5.8.8571
36. Paulke-Korinek M, Rendi-Wagner P, Kundi M, Laaber B, Wiedermann U, Kollaritsch H. Booster vaccinations against tick-borne encephalitis: 6 years follow-up indicates long-term protection. Vaccine (2009) 27:7027–30. doi: 10.1016/j.vaccine.2009.09.068
37. Baldovin T, Mel R, Bertoncelloj C, Carpenè G, Soppelsa F, Giliberti A, et al. Persistence of immunity to tick-borne encephalitis after vaccination and natural infection. J Med Virol (2012) 84(8):1274–8. doi: 10.1002/jmv.23313
38. Jost S, Altfeld M. Control of human viral infections by natural killer cells. Annu Rev Immunol (2013) 31:163–94. doi: 10.1146/annurev-immunol-032712-100001
39. Blom K, Braun M, Pakalniene J, Lunemann S, Enqvist M, Dailidyte L, et al. NK cell responses to human tick-borne encephalitis virus infection. J Immunol (2016) 197(7):2762–71. doi: 10.4049/jimmunol.1600950
40. Azeredo EL, De Oliveira-Pinto LM, Zagne SM, Cerqueira DI, Nogueira RM, Kubelka CF. NK cells, displaying early activation, cytotoxicity and adhesion molecules, are associated with mild dengue disease. Clin Exp Immunol (2006) 143(2):345–56. doi: 10.1111/j.1365-2249.2006.02996.x
41. Petitdemange C, Wauquier N, Devilliers H, Yssel H, Mombo I, Caron M, et al. Longitudinal analysis of natural killer cells in dengue virus-infected patients in comparison to chikungunya and chikungunya/dengue virus-infected patients. PloS Negl Trop Dis (2016) 10:e0004499. doi: 10.1371/journal.pntd.0004499
42. Strauss-Albee DM, Fukuyama J, Liang EC, Yao Y, Jarrell JA, Drake AL, et al. Human NK cell repertoire diversity reflects immune experience and correlates with viral susceptibility. Sci Transl Med (2015) 7(297):297ra115. doi: 10.1126/scitranslmed.aac5722
43. Larena M, Regner M, Lobigs M. Cytolytic effector pathways and IFN-g help protect against Japanese encephalitis. Eur J Immunol (2013) 43:1789–98. doi: 10.1002/eji.201243152
44. Neves PC, Matos DC, Marcovistz R, Galler R. TLR expression and NK cell activation after human yellow fever vaccination. Vaccine (2009) 27:5543–5549. doi: 10.1016/j.vaccine.2009.07.028
45. Atrasheuskaya AV, Fredeking TM, Ignatyev GM. Changes in immune parameters and their correction in human cases of tick-borne encephalitis. Clin Exp Immunol (2003) 131:148–54. doi: 10.1046/j.1365-2249.2003.02050.x
46. Jeohn GH, Kong LY, Wilson B, Hudson P, Hong JS. Synergistic neurotoxic effects of combined treatments with cytokines in murine primary mixed neuron/glia cultures. J Neuroimmunol. (1998) 85:1–10. doi: 10.1016/S0165-5728(97)00204-X
47. de Vries HE, Blom-Roosemalen MC, van Oosten M, de Boer AG, van Berkel TJ, Breimer DD, et al. The influence of cytokines on the integrity of the blood-brain barrier in vitro. J Neuroimmunol (1996) 64(1):37–43. doi: 10.1016/0165-5728(95)00148-4
48. Wang T, Town T, Alexopoulou L, Anderson JF, Fikrig E, Flavell RA. Toll-like receptor 3 mediates West Nile virus entry into the brain causing lethal encephalitis. Nat Med (2004) 10(12):1366–73. doi: 10.1038/nm1140
Keywords: tick-borne encephalitis, vaccine breakthrough, cytokines, dendritic cells, immune response in vitro
Citation: Marušić M, Kopitar AN, Korva M, Knap N, Bogovič P, Strle F, Ihan A and Avšič-Županc T (2023) Dendritic cell activation and cytokine response in vaccine breakthrough TBE patients after in vitro stimulation with TBEV. Front. Immunol. 14:1190803. doi: 10.3389/fimmu.2023.1190803
Received: 21 March 2023; Accepted: 03 May 2023;
Published: 16 May 2023.
Edited by:
Xiaoquan Rao, Case Western Reserve University, United StatesReviewed by:
Juliano Bordignon, Oswaldo Cruz Foundation (Fiocruz), BrazilRuochen Dong, Stowers Institute for Medical Research, United States
Copyright © 2023 Marušić, Kopitar, Korva, Knap, Bogovič, Strle, Ihan and Avšič-Županc. This is an open-access article distributed under the terms of the Creative Commons Attribution License (CC BY). The use, distribution or reproduction in other forums is permitted, provided the original author(s) and the copyright owner(s) are credited and that the original publication in this journal is cited, in accordance with accepted academic practice. No use, distribution or reproduction is permitted which does not comply with these terms.
*Correspondence: Tatjana Avšič-Županc, dGF0amFuYS5hdnNpY0BtZi51bmktbGouc2k=