- 1Center for Vaccine Development and Global Health, University of Maryland School of Medicine, Baltimore, MD, United States
- 2Department of Medicine, University of Maryland School of Medicine, Baltimore, MD, United States
- 3Department of Pediatrics, University of Maryland School of Medicine, Baltimore, MD, United States
Introduction: Non-typhoidal Salmonella (NTS) is responsible for a high burden of foodborne infections and deaths worldwide. In the United States, NTS infections are the leading cause of hospitalizations and deaths due to foodborne illnesses, and older adults (≥65 years) are disproportionately affected by Salmonella infections. Due to this public health concern, we have developed a live attenuated vaccine, CVD 1926 (I77 ΔguaBA ΔclpP ΔpipA ΔhtrA), against Salmonella enterica serovar Typhimurium, a common serovar of NTS. Little is known about the effect of age on oral vaccine responses, and due to the decline in immune function with age, it is critical to evaluate vaccine candidates in older age groups during early product development.
Methods: In this study, adult (six-to-eight-week-old) and aged (18-month-old) C57BL/6 mice received two doses of CVD 1926 (109 CFU/dose) or PBS perorally, and animals were evaluated for antibody and cell-mediated immune responses. A separate set of mice were immunized and then pre-treated with streptomycin and challenged orally with 108 CFU of wild-type S. Typhimurium SL1344 at 4 weeks postimmunization.
Results: Compared to PBS-immunized mice, adult mice immunized with CVD 1926 had significantly lower S. Typhimurium counts in the spleen, liver, and small intestine upon challenge. In contrast, there were no differences in bacterial loads in the tissues of vaccinated versus PBS aged mice. Aged mice exhibited reduced Salmonella-specific antibody titers in the serum and feces following immunization with CVD 1926 compared to adult mice. In terms of T cell responses (T-CMI), immunized adult mice showed an increase in the frequency of IFN-γ- and IL-2-producing splenic CD4 T cells, IFN-γ- and TNF-α-producing Peyer’s Patch (PP)-derived CD4 T cells, and IFN-γ- and TNF-α-producing splenic CD8 T cells compared to adult mice administered PBS. In contrast, in aged mice, T-CMI responses were similar in vaccinated versus PBS mice. CVD 1926 elicited significantly more PP-derived multifunctional T cells in adult compared to aged mice.
Conclusion: These data suggest that our candidate live attenuated S. Typhimurium vaccine, CVD 1926, may not be sufficiently protective or immunogenic in older humans and that mucosal responses to live-attenuated vaccines decrease with increasing age.
Introduction
Non-typhoidal Salmonella (NTS) is a major cause of morbidity and mortality worldwide. The global burden of NTS disease is estimated to be 93 million diarrheal cases and 150,000 deaths each year (1). Specifically in the United States, NTS is the leading cause of hospitalizations and deaths due to foodborne infections (2). During 2021, the Foodborne Diseases Active Surveillance Network (FoodNet) reported 7,148 Salmonella laboratory-diagnosed infections, 1,974 hospitalizations and 52 deaths among 10 U.S. states (2). Alarmingly, 5,620 of the global deaths caused by infection with Salmonella spp. in 2019 were attributable to antimicrobial resistant (AMR) strains; AMR infections are associated with worse clinical outcomes (3, 4). The Centers for Disease Control and Prevention lists NTS as a serious antibiotic resistant threat that requires prompt and sustained action (5).
Most people generally experience self-limiting NTS gastroenteritis lasting 5-7 days. However, those who are malnourished, HIV-positive, infants, or older (≥65 years) are at increased risk for invasive infections that can result in bacteremia, meningitis, and/or death (6, 7). In the United States, rates of severe illness and hospitalization due to NTS have been demonstrated to be highest in individuals ≥65 years of age and increase with age (8–10). Individuals ≥65 years have a ten-fold higher case fatality rate than those aged 5-64 years (3.0% versus 0.3%) (10, 11). Older adults with gastrointestinal illness are more likely to experience extraintestinal manifestations such as septicemia, meningitis, acute renal failure, hemolytic uremic syndrome and arrhythmias, thereby resulting in further health complications for these individuals (10, 12). This poses major risks for long-term care facilities where many older individuals share living spaces and are prone to transmitting NTS to one another (12, 13).
NTS vaccines could be used to protect older adults but currently there are no licensed NTS vaccines available. We have developed a live attenuated S. Typhimurium vaccine candidate, CVD 1926 (I77 ΔguaBA ΔclpP ΔpipA ΔhtrA), against Salmonella enterica serovar Typhimurium (14, 15), one of the most common serotypes of NTS worldwide (16–18). The guaBA deletion is the primary attenuating mutation which produces an auxotrophic S. Typhimurium strain that requires guanine for growth (19). The clpP mutation further attenuates the bacteria and produces hyperflagellation. Deletion of pipA and htrA augments vaccine tolerability (15). In our previous work, we have used non-human primate and murine models to evaluate CVD 1926 (14, 15). We showed that CVD 1926 was able to protect rhesus macaques against moderate-to-severe diarrhea with a vaccine efficacy of 80%; vaccinated animals experienced diarrhea for fewer days and had significantly lower organ burden levels compared to unvaccinated animals following challenge with wild-type S. Typhimurium (15). In mice, CVD 1926 was well-tolerated and immunogenic (100% seroconversion of anti-lipopolysaccharide [LPS] serum IgG) in young adult BALB/c mice and protected them against lethal challenge with wild-type (WT) S. Typhimurium at 1 and 3 months postimmunization (14). These results indicate that CVD 1926 is a promising vaccine candidate in adult animals. However, this and other live NTS vaccines have not yet been evaluated in aged animal models.
Although vaccination is the most effective intervention in preventing infections, older adults experience reduced vaccine efficacy and/or immunogenicity (e.g., to vaccines targeting SARS-CoV-2, influenza virus, Streptococcus pneumoniae, and herpes zoster [shingles]) (20–27) due to a decline and/or dysregulation in immunity with age, termed immunosenescence. However, our current understanding of vaccine responses in older age groups is primarily derived from studies using parenteral vaccines, and little is known about peroral vaccine responses in older adults. To our knowledge, only two oral live attenuated vaccines have been administered to the elderly; a typhoid vaccine (Ty21a) and a pentavalent rotavirus vaccine (RotaTeq®) (28, 29). After Ty21a immunization, the terminal ileum resident T cells of elderly volunteers showed reduced IL-17A and IL-2 production, compared to younger adults; indicating that specific mucosal immune responses were weaker in older volunteers compared to adults. As for RotaTeq®, this vaccine was safe and well tolerated in older adults. After one dose, serum neutralizing antibodies against rotavirus were elevated in aged volunteers. However, a younger adult comparator was not included in this study; thus, the effect of age on RotaTeq® vaccine responses remain unknown. In mice, Fujihashi et al. reported that mucosal immunosenescence to soluble protein antigens can occur as early as 1 year of age; 12- to 14-month-old mice failed to generate fecal IgA responses to oral immunization with ovalbumin adjuvanted with cholera toxin (30, 31). This was associated with fewer antibody-forming cells in the gut-associated lymphoid tissue, compared to adult mice (30, 32). Therefore, there is some evidence that mucosal immunosenescence progresses with age, but it is unknown whether vaccination with live oral NTS vaccines will be less protective and immunogenic in older individuals.
The goal of the current study was to evaluate the immunogenicity of a live attenuated S. Typhimurium oral vaccine candidate, CVD 1926, in the context of immunosenescence by measuring mucosal and systemic vaccine responses in 18-month-old and 6- to 8-week-old mice. To this end, we assessed the following parameters: (i) protection of vaccinated mice against extraintestinal infection with S. Typhimurium, (ii) humoral immunity by measuring antigen-specific serum IgG and fecal IgA levels, and (iii) T cell mediated immunity (T-CMI) by measuring IFN-γ, TNF-α, and IL-2 production and/or CD107a expression in mucosal and splenic CD4 and CD8 T cells. These findings will expand our knowledge of age-associated deficits in generating local and systemic immunity to live S. Typhimurium vaccines and shed light on oral vaccine responses within older hosts.
Materials and methods
Animals and ethics statement
All animal studies were performed in facilities that are accredited by the Association for Assessment and Accreditation of Laboratory Animal Care. Mice were housed under specific pathogen–free conditions at the University of Maryland School of Medicine, and all the procedures were approved by the University of Maryland School of Medicine Institutional Animal Care and Use Committee (protocol no. 0619004). C57BL/6 mice (both sexes) were used to examine T-CMI, antibody responses and to assess protection against bacterial burden using a streptomycin mouse model. Six- to 8-week-old (adult) C57BL/6 mice were purchased from The Jackson Laboratory (Bar Harbor, ME). Eighteen-month-old (aged) C57BL/6 mice were acquired from the National Institute on Aging aged rodent colony or purchased from The Jackson Laboratory. BALB/c mice (female only) were used to examine antibody responses and to determine protection against lethal challenge. Six- to 8-week-old BALB/c mice were purchased from Charles River Laboratories (Wilmington, MA). For 18-month-old BALB/c mice, 6- to 8-week-old mice were purchased from Charles River Laboratories and housed at the University of Maryland School of Medicine until 18 months of age.
Bacterial strains, medium, and culture conditions
Bacterial strains used in this study are shown in Table 1. All bacterial strains were maintained in animal-product-free Hy-Soy (HS) medium (10 g/L Soytone [Teknova, Hollister, CA], 5 g/L Hy-yest [Kerry Bio-Science, Beloit, WI] and 5 g/L sodium chloride [American Bio, Natick, MA]) at 37°C. When needed, agar (Sigma–Aldrich, St. Louis, MO) was added at 15 g/L. For CVD 1926, medium was supplemented with guanine (0.005% weight/volume [w/v] final concentration; Sigma–Aldrich). For S. Typhimurium SL1344, medium was supplemented with 50 μg/mL streptomycin sulfate (Research Products International, Mt. Prospect, IL).
Immunization and challenge
Salmonella strains were streaked onto HS agar (+ 0.005% guanine for CVD 1926) and incubated at 37°C for 18-20 h. Bacteria were then resuspended in sterile PBS and washed twice by centrifugation at 4°C. Bacteria were concentrated by centrifugation or diluted with sterile PBS to achieve the correct dosage. Adult and aged C57BL/6 mice were immunized by peroral gavage with either 109 colony forming units (CFU) of CVD 1926 suspended in 100 μL PBS or 100 μL PBS alone on days 0 and 28. Adult and aged BALB/c mice received either 109 CFU of CVD 1926 suspended in 100 μL PBS or 100 μL PBS by oral gavage on days 0, 21, and 42. Immunized mice were monitored for adverse effects following immunization including lethargy, difficulty breathing, inability to ambulate, and weight loss. Blood was collected one day prior to each immunization or challenge to determine serum antibody titers. Fecal pellets were collected at day -1 and day 55 (for C57BL/6 mice) or 69 (for BALB/c mice) to determine fecal antibody titers.
Immunized C57BL/6 mice were challenged using the streptomycin mouse model (33, 37). Briefly, 4 weeks after the last immunization, mice were fasted for 4 hours before being administered 20 mg streptomycin suspended in 100 µL sterile water by peroral gavage. Food and water were returned immediately. Twenty hours later, food and water were removed for 4 hours, and then mice were infected by peroral gavage with 108 CFU/100 µL of the streptomycin-resistant strain S. Typhimurium SL1344 suspended in PBS. On day 3 post-infection, mice were euthanized, and the spleen, liver, and gastrointestinal tract were harvested, homogenized, and diluted to a concentration of 100 mg tissue/mL PBS. Bacterial counts were determined by spread plating on HS agar containing 50 μg/mL streptomycin. Data are presented as CFU/organ which represents the total S. Typhimurium SL1344 recovered from the whole organ.
Immunized BALB/c mice were challenged with a lethal dose of wild-type S. Typhimurium I77 to determine vaccine efficacy. Briefly, immunized mice were challenged perorally with 100 x LD50 (3 x 106 CFU/100 µL) wild-type S. Typhimurium I77 four weeks post-immunization (day 70). Mice were monitored for up to 30 days for weight loss and signs of illness and euthanized if they met alternative endpoint criteria (e.g., lost ≥20% of their starting body weight) and scored as having succumbed to infection.
Sample collection and assessment of antibody responses
Venous blood was collected and serum separated using serum gel tubes (Sarstedt, Numbrecht, Germany). Serum was isolated after centrifugation at 10,000 x g for 5 min. Fecal pellets were weighed and suspended in ice-cold PBS containing 0.01% sodium azide and 1% protease inhibitor cocktail at a concentration of 100 mg stool/mL (MilliporeSigma, St. Louis, MO). Debris were removed by centrifugation at 15,000 x g for 10 min at 4°C, and the fecal supernatants were collected. All samples were stored at -80°C until analysis.
Vaccine-induced antibody responses were measured by enzyme-linked immunosorbent assay (ELISA). Briefly, 96-well medium binding plates (Greiner Bio-One, Monroe, NC) were coated with either S. Typhimurium core-and O-polysaccharide (COPS) or FliC antigens (19, 36) in PBS at a concentration of 5 μg/mL and incubated overnight at 4°C. Plates were washed with PBS-T (PBS containing 0.05% Tween 20) and blocked with PBS + 10% Omniblok non-fat, dry milk for 2 h at 37°C. Samples were serially diluted in PBS-T + 10% Omniblok, transferred to blocked ELISA plates, and incubated for 1 h at 37°C. Plates were washed and incubated for 1 h at 37°C with horseradish peroxidase (HRP)-labeled anti-mouse IgG (KPL, Gaithersburg, MD) or HRP-labeled anti-mouse IgA (KPL). After washing, substrate (3,3’,5,5’-tetramethylbenzidine; KPL) was added, and the plates were incubated for 10 min in darkness. The reaction was stopped with the addition of 1 M H3PO4, and the absorbance at 450 nm was recorded using a VersaMax microplate reader (Molecular Devices, San Jose, CA). ELISA titers were calculated by interpolation of absorbance values on a standard curve. The endpoint titers reported as ELISA units (EU)/mL represent the inverse of the serum dilution that produced an absorbance value of 0.2 above the blank. Seroconversion in vaccinated mice was defined as a 4-fold increase in the antibody titer compared to the pre-immunization titer.
Isolation of mononuclear cells from the spleen and gut
Single cell suspensions of the spleen and Peyer’s Patches were made by mechanical dissociation of organs through 70-µm-pore size nylon filters. Red blood cells in the spleen were lysed with Ammonium-Chloride-Potassium (ACK) lysis buffer (Gibco, Grand Island, NY). Cells were washed with PBS and then resuspended in complete RPMI 1640 medium (Gibco) supplemented with 10% fetal bovine serum (FBS; Gemini Bioproducts, West Sacramento, CA), 2 mM L-glutamine (Gibco), 1X non-essential amino acids (Gibco), 10 mM HEPES (Gibco), 2.5 mM Sodium pyruvate (Gibco), 100 U/mL penicillin (Sigma-Aldrich), 100 µg/mL streptomycin (Sigma-Aldrich), and 50 μg/mL gentamicin (Gibco).
Preparation of S. Typhimurium lysate
S. Typhimurium I77 was grown on HS agar for 18-20 h at 37°C. Bacteria were harvested, washed in sterile PBS at 4°C and heat-inactivated at 65°C for 30 minutes. Suspensions were then sonicated using Fisherbrand™ Model 120 Sonic Dismembrator (Fisher Scientific, Waltham, MA) for 10 short bursts of 10 seconds at 20 Khz frequency. After sonication, suspensions were centrifuged at 2800 x g for 15 minutes and the supernatant was collected for protein quantification. To determine the protein concentration of the lysate, the Pierce™ BCA Protein Assay Kit was used (ThermoFisher, Waltham, MA).
Ex-vivo stimulation and flow cytometry staining
One million cells from the spleen or Peyer’s Patches were stimulated with either (i) media, (ii) phorbol 12-myristate 13-acetate (PMA; 20 ng/µL) and ionomycin (1 µg/mL), or (iii) S. Typhimurium I77 cell lysate (5 μg/mL) for 16-18 h in round-bottom tubes (Corning, Kennebunk, ME). Cells were then incubated for 4 h in the presence of anti-CD107a-BV510™ (clone 1D4B; Biolegend, San Diego, CA) before overnight incubation with protein transport blockers monensin (1 μg/mL; Sigma) and brefeldin A (2 μg/mL; Biolegend). The next day, cells were transferred to V-shaped 96-well plates (Corning) and stained for flow cytometry. Briefly, 1 x 106 cells were added to a well, washed with PBS, and stained for viability using LIVE/DEAD™ Fixable Yellow stain (Invitrogen, Thermo Fisher Scientific). For cell-surface staining, cells were blocked with anti-mouse CD16/32 (clone 93, Biolegend) for 10 minutes and stained extracellularly with an antibody cocktail (Table S1) for 30 minutes on ice. Pacific Orange-Streptavidin (Invitrogen) was added for 30 minutes on ice as a secondary staining step. Cells were then permeablized and fixed using the eBioscience™ Intracellular Fixation & Permeablization Buffer Set (Invitrogen) according to the manufacturer’s instructions. An antibody cocktail against intracellular targets (Table S1) was added to cells for 30 minutes at room temperature. Cells were then washed with FACS buffer and fixed with 2% paraformaldehyde (PFA) in PBS. Samples were acquired using a Cytek® Aurora spectral flow cytometer and analyzed using SpectroFlo® Software (Cytek® Biosciences, Fremont, CA). T cell responses against S. Typhimurium I77 were expressed as a net percentage of cytokine or CD107a positive cells (i.e., percentage of S. Typhimurium-stimulated cells minus percentage of cells cultured with media only). For each cytokine and CD107a, a cutoff to determine “true responders” was included, as described (15). The cutoff point was the highest value for the cytokine/CD107a identified in the PBS group post-vaccination. Any sample with a net percentage higher than the cutoff was considered a true responder.
Statistical analysis
Data were analyzed using GraphPad Prism 7 Software (La Jolla, CA, USA). A p-value equal to or below 0.05 was considered significant for each test. For ELISA and T cell analyses, statistical comparisons were accomplished using a Mann-Whitney U test (two-tailed, α = 0.05). For assessing vaccine efficacy, seroconversion and cytokine responders, Fisher’s exact test was used. Correlations between bacterial organ burden and α-COPS IgG titers were calculated using a Spearman correlation test (two-tailed, α = 0.05). Survival curves of challenged mice were compared by the log-rank test. Vaccine efficacy (VE) was calculated based on the attack rate (AR) in control and vaccinated mice as follows: VE = (ARcontrols - ARvaccinated)/ARcontrols) × 100.
Results
CVD 1926 elicits poor protection against challenge with S. Typhimurium in aged versus adult C57BL/6 mice
We found that CVD 1926 was safe, and no adverse effects were observed following immunization of 6- to 8-week-old (adult) or 18-month-old (aged) C57BL/6 mice (n=10). We subsequently used the streptomycin challenge model to evaluate protection against colonization with S. Typhimurium (33, 37). At day 3 after challenge, the spleen, liver, and small intestine of CVD 1926-immunized adult mice contained significantly less S. Typhimurium, ~3 log10 CFU lower, compared to the bacterial load of PBS mice (Figure 1). In contrast, CVD 1926 failed to confer protection in aged mice, as there were no significant differences in S. Typhimurium levels within the spleen, liver, and small intestine between vaccinated and unvaccinated animals (Figure 1). Further, for CVD 1926-immunized animals, the adult mice showed significantly lower organ burden in the spleen, liver, and small intestine compared to aged mice (spleen: p = 0.003; liver: p = 0.02; small intestine p = 0.0004). To determine if this phenotype is consistent in a different mouse strain and using a lethal infection model, we assessed vaccine efficacy in BALB/c mice. Three immunizations were used here to compare results to previous findings using this model (14). We observed a vaccine efficacy of 64% (p = 0.0007) against lethal challenge in adult BALB/c mice, but only 33% vaccine efficacy (p = 0.093) in aged BALB/c mice (Table S2). However, by log-rank test, there was a significant difference in survival curves between CVD 1926- and PBS-immunized animals for both the adult and aged mice (Figure S1).
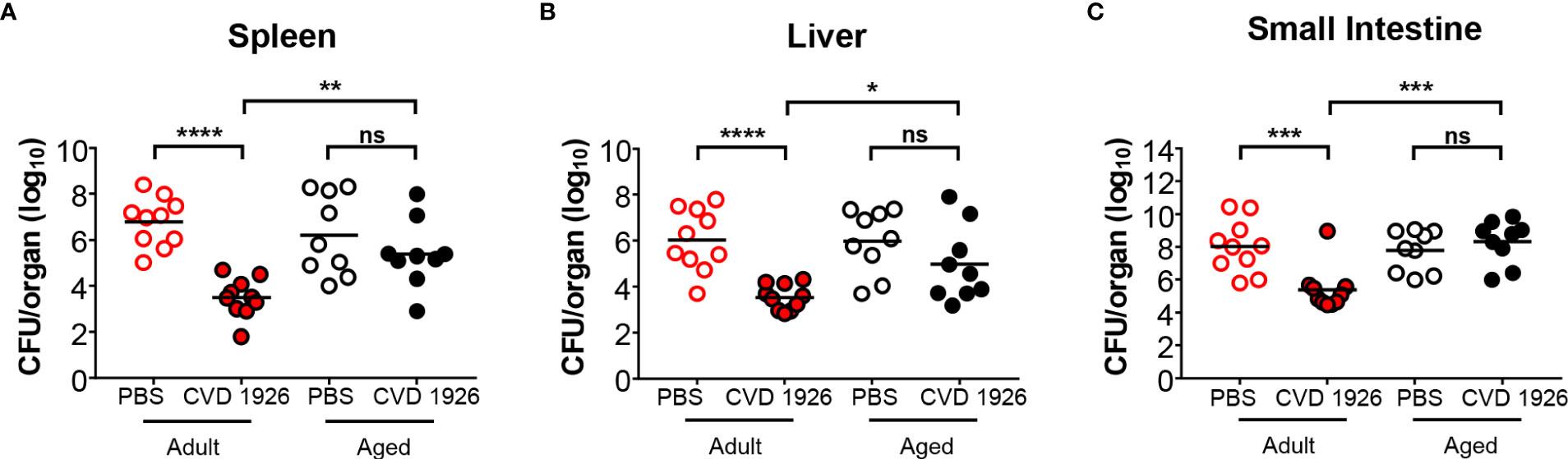
Figure 1 Bacterial burden of adult and aged C57BL/6 mice after challenge with wild-type S. Typhimurium. Bacterial burden in the (A) spleen, (B) liver, and (C) small intestine of adult and aged mice (n=5 of each sex) immunized with either PBS or CVD 1926, and then subsequently challenged perorally with 108 CFU of S. Typhimurium SL1344. Twenty-four hours prior to challenge, mice were pretreated with peroral streptomycin. Median represented by bar (ns, not significant; *p ≤ 0.05; **p ≤ 0.01 ***p ≤ 0.001; ****p ≤ 0.0001 by Mann-Whitney).
Aged C57BL/6 mice fail to produce robust antibody responses following immunization with CVD 1926
CVD 1926 elicited robust anti-COPS and anti-FliC serum IgG antibody titers in adult compared with aged C57BL/6 mice (anti-COPS: p = 0.0009; anti-FliC: p = 0.0003) (Figures 2A, B). Anti-COPS and anti-FliC serum titers in CVD 1926-immunized adult mice were significantly higher than unvaccinated mice at day 55 (anti-COPS: p < 0.0001; anti-FliC: p = 0.0001). Although aged mice vaccinated with CVD 1926 elicited significantly higher anti-COPS serum IgG titers compared to unvaccinated mice (p = 0.011), there was no difference in anti-FliC serum IgG titers (Figures 2A, B). The majority of vaccinated adult mice seroconverted to serum anti-COPS IgG (100%) and anti-FliC serum IgG (90%); however, seroconversion in the aged cohort was significantly diminished in comparison, with 56% of mice seroconverting for anti-COPS (p = 0.033) and 11% (p = 0.001) for anti-FliC serum IgG titers (Table 2). One day prior to challenge, fecal samples from adult mice contained significantly higher anti-COPS IgA levels compared to aged mice (p = 0.003) although there was no significant difference between groups in terms of seroconversion (Figure 2C and Table 2).
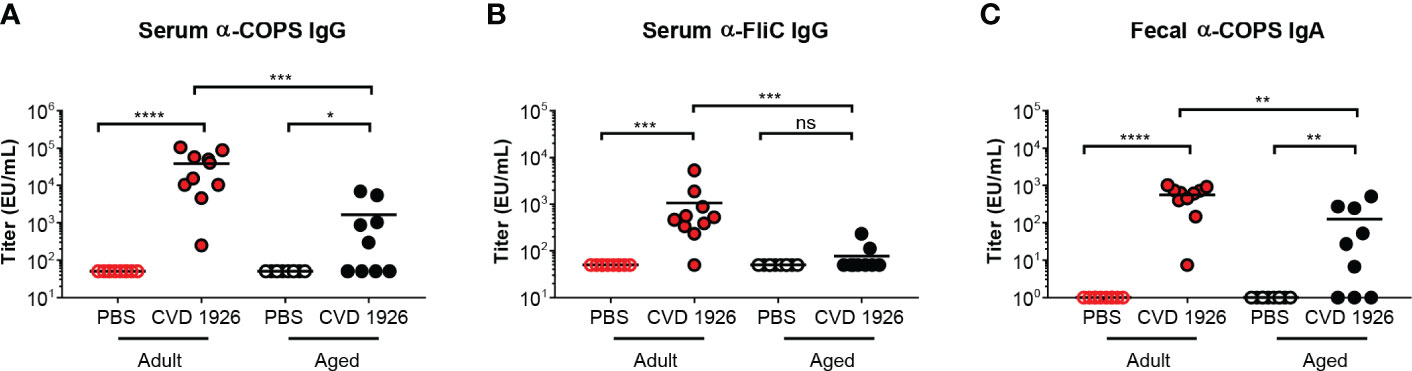
Figure 2 Serum IgG and fecal IgA responses from adult and aged C57BL/6 mice immunized with CVD 1926. (A) Anti-COPS and (B) anti-FliC serum IgG responses and (C) anti-COPS fecal IgA responses after 2 doses of PBS or CVD 1926 (day 55). Geometric mean titer represented by bar (ns, not significant; *p ≤ 0.05; **p ≤ 0.01; ***p ≤ 0.001; ****p ≤ 0.0001 by Mann-Whitney).
Both adult and aged BALB/c mice showed a dose-response increase in anti-COPS serum IgG levels post-vaccination, in comparison to PBS-immunized mice (Figures S2A, C). Although both adult and aged vaccinated mice produced high fecal IgA titers by day 69, adult mice produced significantly higher anti-COPS IgA levels than aged mice with a geometric mean titer (GMT) of 1,122.33 and 180.34, respectively (p < 0.0001) (Figures S2B, D). We investigated the relationship between anti-COPS antibody levels and S. Typhimurium organ burden following challenge in adult and aged C57BL/6 mice. Anti-COPS serum IgG levels and S. Typhimurium CFU from the spleens of adult and aged mice were negatively correlated (r = -0.519, p = 0.027) (Figure S3A). For the liver and small intestine, there was a moderate negative association between anti-COPS serum IgG levels and organ burden but the p-values were not significant (liver: r = -0.455, p = 0.058; small intestine: r = -0.450, p = 0.061) (Figures S3B, C). Anti-COPS fecal IgA levels and CFU counts in the spleen (r = -0.479, p = 0.045) and liver (r = -0.584, p = 0.011) were negatively correlated as well (Figures S4A, B). Likewise, there was a negative but non-significant association between the fecal IgA titers and bacteria present within the small intestine (r = -0.461, p = 0.054) (Figure S4C).
Reduced T-CMI responses in aged C57BL/6 mice receiving CVD 1926 compared to C57BL/6 adult mice
For evaluation of systemic and mucosal T-CMI to CVD 1926 in C57BL/6 mice, splenic and Peyer’s Patch (PP)-derived cells were stimulated ex-vivo with wild-type S. Typhimurium lysate 10 days following the second immunization, and cytokine production (IFN-γ, TNF-α, IL-2) and CD107a upregulation (a T cell degranulation marker) by CD4 and CD8 T cells were assessed by intracellular flow cytometry (Figure S5). There were no differences in the frequencies of CD4 and CD8 T cells within the spleen and PPs of adult and aged mice (Figure S6). However, within the CD4 and CD8 T cell compartments, naïve aged mice had significantly more T cells expressing CD44 (Figure S7). S. Typhimurium-specific T-CMI was recorded in the spleens of adult mice as shown by higher levels of IFN-γ+ and IL-2+ CD4 T cells in the spleens of immunized adult mice compared to PBS-immunized mice (Figure 3A). Likewise, frequencies of antigen-specific IFN-γ+ and TNF-α+ PP-derived CD4 T cells were significantly higher in CVD 1926-immunized mice compared to unvaccinated mice (Figure 3C). Importantly, CVD 1926 did not elicit S. Typhimurium-specific T-CMI in aged mice, as there were no differences in CD4 T cell cytokine production between vaccinated and unvaccinated aged mice in the spleen or PP (Figures 3B, D respectively). Splenic CD8 T cells were also evaluated, but there were too few CD8 T cells in the PP to evaluate statistical differences between individual mice. In the CD8 compartment, CVD 1926-immunized adult mice demonstrated higher frequencies of TNF-α+ CD8 T spleen cells compared to non-immunized animals (Figure 4A). CVD 1926 did not elicit strong CD8 T-CMI in aged mice (Figure 4B). The proportion of CD4 and CD8 T cell responders is shown in Table 3.
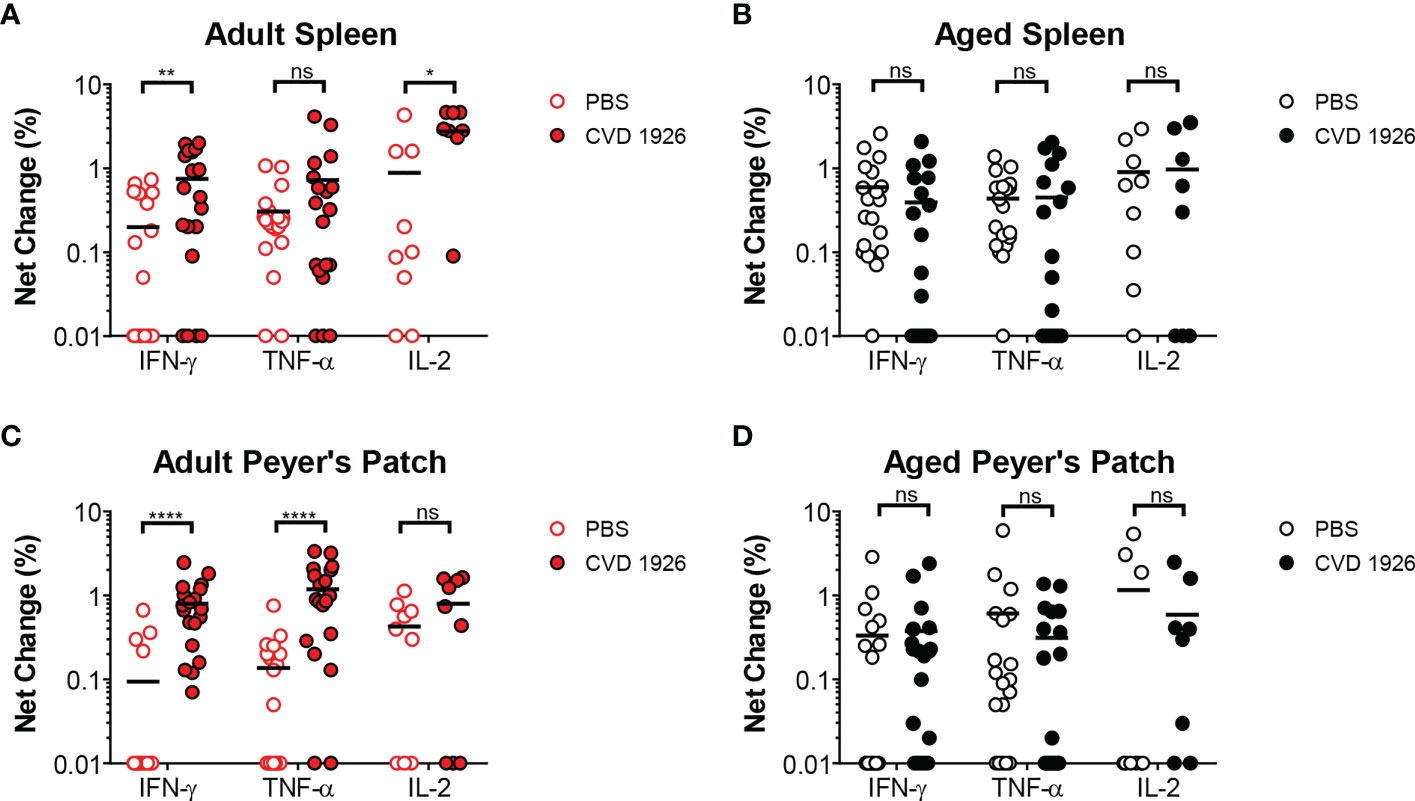
Figure 3 Evaluation of CD4 T cell responses elicited by CVD 1926 in C57BL/6 mice. Fourteen days following the second immunization with CVD 1926, cytokine production from CD4 T cells were assessed in the spleens (A, B) and Peyer’s Patches (PP; C, D) of adult (A, C) and aged (B, D) mice. Changes in these markers were assessed upon ex-vivo stimulation with S. Typhimurium lysate. Each point represents data from one mouse. Median represented by bar (ns, not significant; *p ≤ 0.05; **p ≤ 0.01; ****p ≤ 0.0001 by Mann-Whitney).
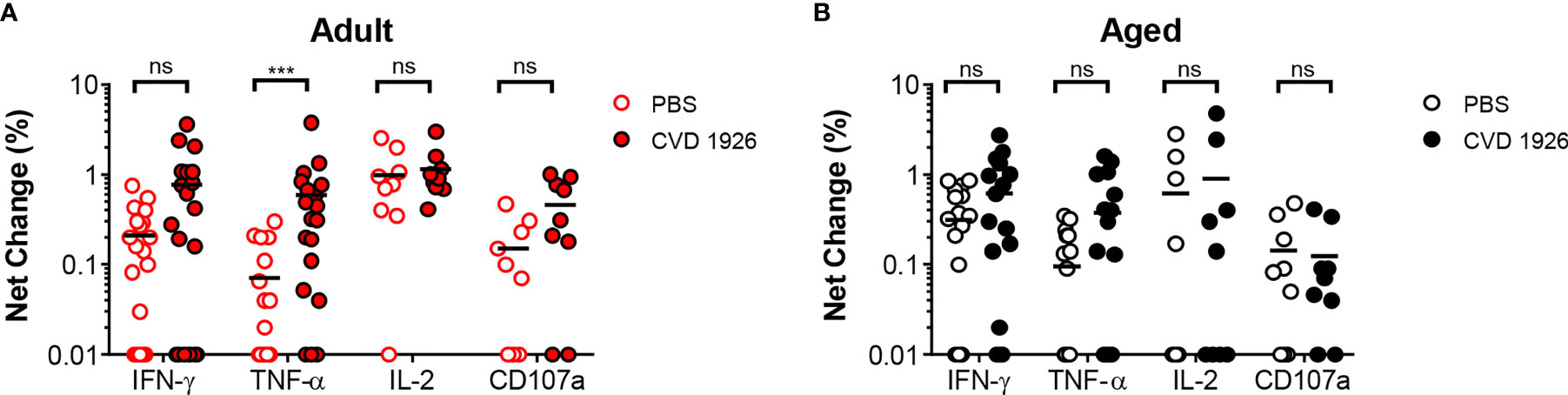
Figure 4 Evaluation of CD8 T cell responses elicited by CVD 1926 in C57BL/6 mice. Fourteen days following the second immunization with CVD 1926, cytokine production and CD107a upregulation from CD8 T cells were assessed in the spleens of (A) adult and (B) aged mice. Changes in these markers were assessed upon ex-vivo stimulation with S. Typhimurium lysate. Each point represents data from one mouse. Median represented by bar (ns, not significant; ***p ≤ 0.001 by Mann-Whitney).
T cells capable of upregulating at least two cytokines/CD107a expression (termed multifunctional [MF]) were assessed in a subset of mice in response to S. Typhimurium stimulation. Amongst adult vaccine recipients, 44% demonstrated MF responses in splenic CD4 T cells, 66% in PP-derived CD4 T cells, and 33% in splenic CD8 T cells (Table 4). In contrast, single functionality (or no functionality) predominated in aged mice; 11% of splenic CD4 T cell responders, 0% of PP-derived CD4 T cell responders, and 22% of splenic CD8 T cell responders were MF. The number of CVD 1926-immunized animals demonstrating multifunctionality in the PP was significantly greater for adult mice than aged mice (p = 0.009) (Table 4). For MF splenic CD4 T cells from adult mice, IL-2 production was detected from all animals displaying multifunctionality (Figure 5A). No dominant cytokine expression profile was observed in PP-derived MF CD4 T cells since MF cells producing different combinations of IFN-γ, TNF-α and/or IL-2 were all detected (Figure 5B). For multifunctionality in the CD8 T cell compartment, 3/9 (33%) adult mice and 2/9 (22%) aged mice demonstrated detectable MF CD8 T cells (Table 4). TNF-α production (with IFN-γ, IL-2 and/or CD107) was dominant in MF CD8 T cells from adult mice, whilst IFN-γ and IL-2 production was consistent among MF CD8 T cells from aged mice (Figure 5C).

Table 4 Proportion of mice that show multifunctional (MF) T cell responses to CVD 1926 immunization.
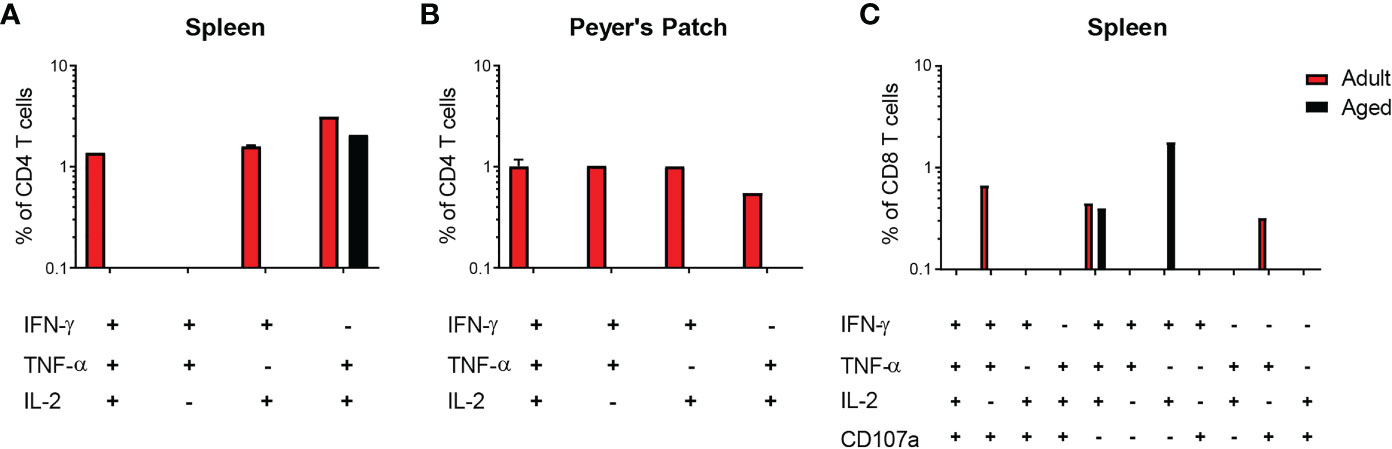
Figure 5 Cytokine profiles of multifunctional (MF) CD4 and CD8 T cells elicited by CVD 1926 in C57BL/6 mice. Induction of MF CD4 and CD8 T cells following immunization with CVD 1926 in vaccine responders. Fourteen days following the second immunization with CVD 1926, the ability of CD4 T cells in the (A) spleens and (B) Peyer’s Patches as well as (C) CD8 T cells in the spleen to produce more than one cytokine and/or CD107a expression was assessed. Only data from animals demonstrating multifunctionality are shown.
Discussion
Development of an effective NTS vaccine for high-risk target groups, including the elderly, remains a public health priority. In this study, we explored the impact of age-related immunosenescence on the protective ability of CVD 1926, a live-attenuated vaccine candidate for S. Typhimurium. We demonstrated that aged mice immunized with CVD 1926 failed to reduce bacterial burden upon challenge with wild-type S. Typhimurium, which was associated with lower vaccine-induced antibody titers and weaker T cell responses. Our data suggest that CVD 1926 is poorly immunogenic and less efficacious in this age group.
Aged mice immunized with CVD 1926 were unable to lower the bacterial load upon challenge which is consistent with other studies showing that aged mice failed to clear influenza, SARS-CoV-2 or Streptococcus pneumoniae infection after immunization (38–40). Moreover, we showed that aged mice produced poor antigen-specific antibody and T cell responses following vaccination, which are both involved in protective immunity to NTS infection in susceptible mouse strains (41); hence, consistent with the inability to control the bacterial burden.
Decreased antigen-specific antibody levels have been implicated in weak vaccine responses in older individuals (27, 42). In line with these observations, we found that aged mice produced significantly lower anti-COPS and anti-FliC serum IgG responses compared to adult mice. Notably, CVD 1926 did not elicit an anti-FliC IgG response altogether in aged mice, as evidenced by comparable titers between CVD 1926- and PBS-immunized mice. Antibodies directed at the O-antigen of LPS and flagellin protein of Salmonella play a critical role in mediating protection in mice and humans by promoting phagocytic uptake and/or lysing bacteria directly via complement fixation (43–46). Since prevention of bacterial dissemination relies on robust preexisting antibody responses, the inability of CVD 1926-immunzed aged mice to clear bacteria upon infection may be explained by modest levels of anti-COPS and anti-FliC serum IgG. Indeed, we observed a significant relationship between S. Typhimurium CFUs in the spleen and anti-COPS serum IgG levels, which is consistent with reports from sub-Saharan Africa where Salmonella-specific antibody titers were shown to be associated with decreased disease in humans (47, 48).
Aged C57BL/6 mice produced lower levels of anti-COPS fecal IgA with only 67% of mice seroconverting, as compared to 100% of adult mice. Similarly, fecal IgA levels from aged BALB/c mice were lower compared to adult mice post-immunization. Secretory IgA (SIgA) is proposed to entrap antigens and pathogens within the intestinal lumen, which restricts colonization of the gut mucosa, as well as invasion to extraintestinal tissues (49). Despite comparable anti-COPS serum IgG titers between adult and aged BALB/c mice post-immunization, aged BALB/c mice achieved a vaccine efficacy of only 33% (versus 64% in adult mice), suggesting a role for mucosal IgA in protection against lethality. Richards et al. demonstrated that a human SIgA monoclonal antibody against S. Typhimurium LPS, Sal4, limited S. Typhimurium invasion of Peyer’s Patches in BALB/c mice (50). Lastly, in humans, the quantity and avidity of IgA specific for S. Typhi Vi polysaccharide was correlated with protection against typhoid fever, thereby highlighting the importance of IgA mediated immunity (51, 52). With these studies and our finding that IgA titers in the stool negatively correlate with S. Typhimurium burden in the spleen and liver, we postulate that, in addition to serum IgG, antigen-specific IgA in the intestinal lumen assists in preventing bacterial dissemination to deep tissues upon oral infection. In line with this hypothesis, the age-associated decrease in fecal IgA titers likely contributes to the high bacterial burden in aged C57BL/6 mice after challenge.
CVD 1926 elicited fewer T cells capable of producing pro-inflammatory cytokines in aged mice compared to young animals. The number of T cell responders, the magnitude of cytokine production, and number of animals with MF T cells was weak in aged mice, suggesting that, collectively, CVD 1926 failed to elicit a robust T-CMI in aged mice. This is consistent with data from older adult humans that received influenza and SARS-CoV-2 mRNA vaccines and may explain the decreased protection in older animals (53). Compared to PBS-treated adult mice, CVD 1926-immunized animals showed an increase in the frequency of splenic IFN-γ and IL-2 producing CD4 T cells and IFN-γ and TNF-α producing PP-derived CD4 T cells following in vitro stimulation with S. Typhimurium lysate. Since cytokine production upon re-stimulation is a known property of effector T cells and Salmonella clearance requires a robust Th1 response, we postulate that the T cells of vaccinated adult mice secrete pro-inflammatory cytokines to promote bacterial killing by immune inflammatory cells in the gut, limiting extra-intestinal spread during infection (54–57). This is supported by a study showing that convalescent typhoid fever patients produced higher levels of IFN-γ, TNF-α and MIP-1β (58). Moreover, extensive studies using specimens from participants exposed to wild-type S. Typhi showed striking associations in the specific production of IFN-γ, TNF-α, IL-2, and other cytokines, as well as the expression of CD107, and protection from disease (59–61). MF T cells are an indicator of efficient effector responses and there is evidence that secretion of multiple cytokines synergistically support phagocytic killing (62, 63). CVD 1926 elicited multifunctionality in 44%, 66% and 33% of adult mice for splenic CD4, PP-derived CD4, and splenic CD8 T cell responses, respectively. In contrast, only 11%, 0%, and 22% of aged mice showed multifunctional cytokine production for splenic CD4, PP-derived CD4, and splenic CD8 T cell compartments, respectively. IL-2 is essential for T cell survival and its production was detected alongside IFN-γ or TNF-α in all splenic and most PP-derived CD4 MF T cells in adult mice (64). Therefore, the presence of IL-2-secreting T cells in the spleen and PP of adult mice may contribute to the robust T cell responses observed in adult but not aged mice. In addition to functional defects, T cells experience phenotypic alterations with age; the frequency of naïve CD4 and CD8 T cell populations in peripheral blood and in the terminal ileum are lower for older adults (29, 65, 66). This is often accompanied by an increase in T memory cells (66). In fact, we found that naïve aged mice harbored high levels of CD44+ CD4 and CD8 T cells in the spleen and PP compared to adult mice, signifying a greater population of antigen-experienced T cells present prior to vaccination. A proposed explanation for weaker vaccine responses in older individuals is that the naïve T cell pool is reduced and limits primary vaccine responses to novel antigens (20, 67). Altogether, the high bacterial loads observed in aged mice post challenge may be due in part to their inferior T cell responses to CVD 1926.
We observed that CVD 1926 successfully induced antigen-specific fecal IgA responses and functional T cells from the PPs in adult mice, which are characteristic of an effective mucosal immune response. The effect of age on tissue-specific vaccine responses is becoming increasingly appreciated (68). We found that aged mice produced lower antigen-specific IgA levels and weaker PP-derived T cell responses, which are indicative of an impaired mucosal immunity. Consistent with our findings, adults ≥60 years of age that received the live oral vaccine, S. Typhi Ty21a also showed lower intestinal T cell cytokine responses compared to younger counterparts (29). As for cell-extrinsic age-related deficiencies, the spleen and lymph nodes of older adults become fibrotic and lose confined stromal networks of the lymphoid tissue (69, 70). It is unknown whether these microenvironment alterations extend to the lymphoid structure of mucosa (e.g., Peyer’s Patches). If so, this may account for blunted mucosal responses to CVD 1926, similarly to how lymphoid tissue fibrosis was associated with impaired immunological responses to yellow fever vaccination (71). Future studies on oral vaccine responses are warranted to better understand mucosal immunosenescence.
In this study, we assessed the effect of vaccination with a live oral S. Typhimurium vaccine on several immunological parameters in aged versus adult mice. In addition to immunological changes, other factors could potentially contribute to reduced immunogenicity and efficacy observed in aged mice. For example, increased gut permeability and shifts in intestinal microbiota composition have been shown to be associated with advanced age and may negatively impact vaccine responses (72).
Previous live attenuated S. Typhimurium strains that were immunogenic in murine models elicited similar vaccine responses in human volunteers; suggesting that preclinical testing of NTS vaccines in mice can translate clinically (73). Although there are some phenotypic differences in B and T cell populations between older humans and aged mice, the antibody and T cell responses to several licensed vaccines in older adults reflects what has been observed in aged murine models (74). Thus, we anticipate that antibody and T cell responses to CVD 1926 in humans will resemble what we observed herein.
Older adults would highly benefit from vaccines targeting enteric illnesses caused by Salmonella spp., Escherichia coli, Clostridium spp., and others. Live-attenuated vaccines given via the oral route can elicit immunity at the local level within the gastrointestinal tract as well as systemically and are easy to administer and manufacture. Overall, this work provides the first head-to-head comparison of NTS vaccine responses between adult and aged mice and shows that the live oral CVD 1926 vaccine was poorly immunogenic in aged mice. The findings herein are important from a translational perspective, as these data indicate that our live NTS vaccine candidate CVD 1926 requires further optimization to be sufficiently immunogenic for older adults. Strategies may include the addition of a mucosal adjuvant, use of a heterologous live oral prime followed by a parenteral boost regimen, and/or mutating Salmonella immune evasion genes to increase immunogenicity. Our data also highlight the issue of mucosal immunosenescence and suggest that more research is needed to understand the mechanisms underlying poor immune responses to oral vaccines in aged hosts.
Data availability statement
The original contributions presented in the study are included in the article/Supplementary Material. Further inquiries can be directed to the corresponding author.
Ethics statement
The animal study was reviewed and approved by University of Maryland School of Medicine IACUC.
Author contributions
JA designed and performed experiments, analyzed data, prepared figures and prepared the original manuscript draft. FT and SB provided scientific input and technical training. MS provided scientific input. ST conceived and designed experiments, acquired funds for the study, and supervised the study. FT, SB, MS and ST reviewed/edited the manuscript. All authors contributed to the article and approved the submitted version.
Funding
This work was funded by National Institutes of Health (NIH)/National Institute of Allergy and Infectious Diseases (NIAID) under Project 2: Multivalent vaccines effective against MDR Salmonella (project leader: ST) of U19 AI142725 awarded to Myron M. Levine.
Conflict of interest
ST is a holder of the following patents that describe development of NTS vaccines: US patent 9,050,283, “Broad spectrum vaccine against non-typhoidal Salmonella”; US patent 9,011,871, “Broad spectrum vaccine against typhoidal and non-typhoidal Salmonella disease”; and European Patent Number 2387417, “Broad spectrum vaccine against non-typhoidal Salmonella”.
The remaining authors declare that the research was conducted in the absence of any commercial or financial relationships that could be construed as a potential conflict of interest.
Publisher’s note
All claims expressed in this article are solely those of the authors and do not necessarily represent those of their affiliated organizations, or those of the publisher, the editors and the reviewers. Any product that may be evaluated in this article, or claim that may be made by its manufacturer, is not guaranteed or endorsed by the publisher.
Supplementary material
The Supplementary Material for this article can be found online at: https://www.frontiersin.org/articles/10.3389/fimmu.2023.1190339/full#supplementary-material
References
1. Majowicz SE, Musto J, Scallan E, Angulo FJ, Kirk M, O'Brien SJ, et al. The global burden of nontyphoidal Salmonella gastroenteritis. Clin Infect Dis (2010) 50:882–9. doi: 10.1086/650733
2. Collins JP, Shah HJ, Weller DL, Ray LC, Smith K, McGuire S, et al. Preliminary incidence and trends of infections caused by pathogens transmitted commonly through food - foodborne diseases active surveillance network, 10 U.S. sites, 2016-2021. MMWR Morb Mortal Wkly Rep (2022) 71:1260–4. doi: 10.15585/mmwr.mm7140a2
3. Collaborators AR. Global burden of bacterial antimicrobial resistance in 2019: a systematic analysis. Lancet (2022) 399:629–55. doi: 10.1016/s0140-6736(21)02724-0
4. Parisi A, Crump JA, Glass K, Howden BP, Furuya-Kanamori L, Vilkins S, et al. Health outcomes from multidrug-resistant Salmonella infections in high-income countries: a systematic review and meta-analysis. Foodborne Pathog Dis (2018) 15:428–36. doi: 10.1089/fpd.2017.2403
5. CDC. Antibiotic resistance threats in the United States, 2019. Atlanta, GA: U.S: Department of Health and Human Services (2019).
6. GBD 2017 Non-Typhoidal Salmonella Invasive Disease Collaborators. The global burden of non-typhoidal Salmonella invasive disease: a systematic analysis for the global burden of disease study 2017. Lancet Infect Dis (2019) 19:1312–24. doi: 10.1016/s1473-3099(19)30418-9
7. Gordon MA, Banda HT, Gondwe M, Gordon SB, Boeree MJ, Walsh AL, et al. Non-typhoidal Salmonella bacteraemia among HIV-infected Malawian adults: high mortality and frequent recrudescence. Aids (2002) 16:1633–41. doi: 10.1097/00002030-200208160-00009
8. Scallan E, Crim SM, Runkle A, Henao OL, Mahon BE, Hoekstra RM, et al. Bacterial enteric infections among older adults in the United States: foodborne diseases active surveillance network, 1996-2012. Foodborne Pathog Dis (2015) 12:492–9. doi: 10.1089/fpd.2014.1915
9. Chen Y, Glass K, Liu B, Hope K, Kirk M. Salmonella infection in middle-aged and older adults: incidence and risk factors from the 45 and up study. Foodborne Pathog Dis (2016) 13:689–94. doi: 10.1089/fpd.2016.2170
10. White AE, Ciampa N, Chen Y, Kirk M, Nesbitt A, Bruce BB, et al. Characteristics of Campylobacter and Salmonella infections and acute gastroenteritis in older adults in Australia, Canada, and the United States. Clin Infect Dis (2019) 69:1545–52. doi: 10.1093/cid/ciy1142
11. Behravesh CB, Jones TF, Vugia DJ, Long C, Marcus R, Smith K, et al. Deaths associated with bacterial pathogens transmitted commonly through food: foodborne diseases active surveillance network (FoodNet), 1996-2005. J Infect Dis (2011) 204:263–7. doi: 10.1093/infdis/jir263
12. Kirk MD, Veitch MG, Hall GV. Gastroenteritis and food-borne disease in elderly people living in long-term care. Clin Infect Dis (2010) 50:397–404. doi: 10.1086/649878
13. Greig JD, Lee MB. Enteric outbreaks in long-term care facilities and recommendations for prevention: a review. Epidemiol Infect (2009) 137:145–55. doi: 10.1017/s0950268808000757
14. Higginson EE, Ramachandran G, Panda A, Shipley ST, Kriel EH, DeTolla LJ, et al. Improved tolerability of a Salmonella enterica serovar Typhimurium live-attenuated vaccine strain achieved by balancing inflammatory potential with immunogenicity. Infect Immun (2018) 86:e00440-18. doi: 10.1128/iai.00440-18
15. Higginson EE, Panda A, Toapanta FR, Terzi MC, Jones JA, Sen S, et al. Immunogenicity and efficacy of live-attenuated Salmonella Typhimurium vaccine candidate CVD 1926 in a rhesus macaque model of gastroenteritis. Infect Immun (2021) 89:e0008721. doi: 10.1128/iai.00087-21
16. European Food Safety Authority and European Centre for Disease Prevention and Control (EFSA and ECDC).The European union summary report on trends and sources of zoonoses, zoonotic agents and food-borne outbreaks in 2017. EFSA J (2018) 16:e05500. doi: 10.2903/j.efsa.2018.5500
17. Zhang S, Li S, Gu W, den Bakker H, Boxrud D, Taylor A, et al. Zoonotic source attribution of Salmonella enterica serotype Typhimurium using genomic surveillance data, United States. Emerg Infect Dis (2019) 25:82–91. doi: 10.3201/eid2501.180835
18. Ferrari RG, Rosario DKA, Cunha-Neto A, Mano SB, Figueiredo EES, Conte-Junior CA. Worldwide epidemiology of Salmonella serovars in animal-based foods: a meta-analysis. Appl Environ Microbiol (2019) 85:e00440-18. doi: 10.1128/aem.00591-19
19. Tennant SM, Wang JY, Galen JE, Simon R, Pasetti MF, Gat O, et al. Engineering and preclinical evaluation of attenuated nontyphoidal Salmonella strains serving as live oral vaccines and as reagent strains. Infect Immun (2011) 79:4175–85. doi: 10.1128/iai.05278-11
20. Allen JC, Toapanta FR, Chen W, Tennant SM. Understanding immunosenescence and its impact on vaccination of older adults. Vaccine (2020) 38:8264–72. doi: 10.1016/j.vaccine.2020.11.002
21. Goodwin K, Viboud C, Simonsen L. Antibody response to influenza vaccination in the elderly: a quantitative review. Vaccine (2006) 24:1159–69. doi: 10.1016/j.vaccine.2005.08.105
22. Schenkein JG, Park S, Nahm MH. Pneumococcal vaccination in older adults induces antibodies with low opsonic capacity and reduced antibody potency. Vaccine (2008) 26:5521–6. doi: 10.1016/j.vaccine.2008.07.071
23. Siegrist CA, Aspinall R. B-cell responses to vaccination at the extremes of age. Nat Rev Immunol (2009) 9:185–94. doi: 10.1038/nri2508
24. Young B, Zhao X, Cook AR, Parry CM, Wilder-Smith A, MC IC. Do antibody responses to the influenza vaccine persist year-round in the elderly? a systematic review and meta-analysis. Vaccine (2017) 35:212–21. doi: 10.1016/j.vaccine.2016.11.013
25. Levin MJ, Oxman MN, Zhang JH, Johnson GR, Stanley H, Hayward AR, et al. Varicella-zoster virus-specific immune responses in elderly recipients of a herpes zoster vaccine. J Infect Dis (2008) 197:825–35. doi: 10.1086/528696
26. Bonten MJ, Huijts SM, Bolkenbaas M, Webber C, Patterson S, Gault S, et al. Polysaccharide conjugate vaccine against pneumococcal pneumonia in adults. N Engl J Med (2015) 372:1114–25. doi: 10.1056/NEJMoa1408544
27. Brockman MA, Mwimanzi F, Lapointe HR, Sang Y, Agafitei O, Cheung PK, et al. Reduced magnitude and durability of humoral immune responses to COVID-19 mRNA vaccines among older adults. J Infect Dis (2022) 225:1129–40. doi: 10.1093/infdis/jiab592
28. Lawrence J, He S, Martin J, Schödel F, Ciarlet M, Murray AV. Safety and immunogenicity of pentavalent rotavirus vaccine in a randomized, double-blind, placebo-controlled study in healthy elderly subjects. Hum Vaccin Immunother (2014) 10:2247–54. doi: 10.4161/hv.29107
29. Booth JS, Goldberg E, Patil SA, Barnes RS, Greenwald BD, Sztein MB. Age-dependency of terminal ileum tissue resident memory T cell responsiveness profiles to S. Typhi following oral Ty21a immunization in humans. Immun Ageing (2021) 18:19. doi: 10.1186/s12979-021-00227-y
30. Fujihashi K, Koga T, McGhee JR. Mucosal vaccination and immune responses in the elderly. Vaccine (2000) 18:1675–80. doi: 10.1016/s0264-410x(99)00505-8
31. Fujihashi K, McGhee JR. Mucosal immunity and tolerance in the elderly. Mech Ageing Dev (2004) 125:889–98. doi: 10.1016/j.mad.2004.05.009
32. Koga T, McGhee JR, Kato H, Kato R, Kiyono H, Fujihashi K. Evidence for early aging in the mucosal immune system. J Immunol (2000) 165:5352–9. doi: 10.4049/jimmunol.165.9.5352
33. Barthel M, Hapfelmeier S, Quintanilla-Martínez L, Kremer M, Rohde M, Hogardt M, et al. Pretreatment of mice with streptomycin provides a Salmonella enterica serovar Typhimurium colitis model that allows analysis of both pathogen and host. Infect Immun (2003) 71:2839–58. doi: 10.1128/iai.71.5.2839-2858.2003
34. Hoiseth SK, Stocker BA. Aromatic-dependent Salmonella Typhimurium are non-virulent and effective as live vaccines. Nature (1981) 291:238–9. doi: 10.1038/291238a0
35. Levy H, Diallo S, Tennant SM, Livio S, Sow SO, Tapia M, et al. PCR method to identify Salmonella enterica serovars Typhi, Paratyphi A, and Paratyphi B among Salmonella isolates from the blood of patients with clinical enteric fever. J Clin Microbiol (2008) 46:1861–6. doi: 10.1128/jcm.00109-08
36. Hegerle N, Bose J, Ramachandran G, Galen JE, Levine MM, Simon R, et al. Overexpression of O-polysaccharide chain length regulators in Gram-negative bacteria using the wzx-/Wzy-dependent pathway enhances production of defined modal length O-polysaccharide polymers for use as haptens in glycoconjugate vaccines. J Appl Microbiol (2018) 125:575–85. doi: 10.1111/jam.13772
37. Ren Z, Gay R, Thomas A, Pae M, Wu D, Logsdon L, et al. Effect of age on susceptibility to Salmonella Typhimurium infection in C57BL/6 mice. J Med Microbiol (2009) 58:1559–67. doi: 10.1099/jmm.0.013250-0
38. Baldwin SL, Hsu FC, Van Hoeven N, Gage E, Granger B, Guderian JA, et al. Improved immune responses in young and aged mice with adjuvanted vaccines against H1N1 influenza infection. Front Immunol (2018) 9:295. doi: 10.3389/fimmu.2018.00295
39. Chen Y, Li C, Liu F, Ye Z, Song W, Lee ACY, et al. Age-associated SARS-CoV-2 breakthrough infection and changes in immune response in a mouse model. Emerg Microbes Infect (2022) 11:368–83. doi: 10.1080/22221751.2022.2026741
40. Simmons SR, Tchalla EYI, Bhalla M, Bou Ghanem EN. The age-driven decline in neutrophil function contributes to the reduced efficacy of the pneumococcal conjugate vaccine in old hosts. Front Cell Infect Microbiol (2022) 12:849224. doi: 10.3389/fcimb.2022.849224
41. Simon R, Tennant SM, Galen JE, Levine MM. Mouse models to assess the efficacy of non-typhoidal Salmonella vaccines: revisiting the role of host innate susceptibility and routes of challenge. Vaccine (2011) 29:5094–106. doi: 10.1016/j.vaccine.2011.05.022
42. Frasca D, Blomberg BB. Aging induces B cell defects and decreased antibody responses to influenza infection and vaccination. Immun Ageing (2020) 17:37. doi: 10.1186/s12979-020-00210-z
43. MacLennan CA, Tennant SM. Comparing the roles of antibodies to nontyphoidal Salmonella enterica in high- and low-income countries and implications for vaccine development. Clin Vaccine Immunol (2013) 20:1487–90. doi: 10.1128/cvi.00465-13
44. Trebicka E, Jacob S, Pirzai W, Hurley BP, Cherayil BJ. Role of antilipopolysaccharide antibodies in serum bactericidal activity against Salmonella enterica serovar Typhimurium in healthy adults and children in the United States. Clin Vaccine Immunol (2013) 20:1491–8. doi: 10.1128/cvi.00289-13
45. Goh YS, Grant AJ, Restif O, McKinley TJ, Armour KL, Clark MR, et al. Human IgG isotypes and activating fcγ receptors in the interaction of Salmonella enterica serovar Typhimurium with phagocytic cells. Immunology (2011) 133:74–83. doi: 10.1111/j.1365-2567.2011.03411.x
46. Simon R, Tennant SM, Wang JY, Schmidlein PJ, Lees A, Ernst RK, et al. Salmonella enterica serovar Enteritidis core O polysaccharide conjugated to h:g,m flagellin as a candidate vaccine for protection against invasive infection with S. Enteritidis. Infect Immun (2011) 79:4240–9. doi: 10.1128/iai.05484-11
47. MacLennan CA, Gondwe EN, Msefula CL, Kingsley RA, Thomson NR, White SA, et al. The neglected role of antibody in protection against bacteremia caused by nontyphoidal strains of Salmonella in African children. J Clin Invest (2008) 118:1553–62. doi: 10.1172/jci33998
48. Nyirenda TS, Gilchrist JJ, Feasey NA, Glennie SJ, Bar-Zeev N, Gordon MA, et al. Sequential acquisition of T cells and antibodies to nontyphoidal Salmonella in Malawian children. J Infect Dis (2014) 210:56–64. doi: 10.1093/infdis/jiu045
49. Richards AF, Baranova DE, Pizzuto MS, Jaconi S, Willsey GG, Torres-Velez FJ, et al. Recombinant human secretory IgA induces Salmonella Typhimurium agglutination and limits bacterial invasion into gut-associated lymphoid tissues. ACS Infect Dis (2021) 7:1221–35. doi: 10.1021/acsinfecdis.0c00842
50. Richards AF, Doering JE, Lozito SA, Varrone JJ, Willsey GG, Pauly M, et al. Inhibition of invasive Salmonella by orally administered IgA and IgG monoclonal antibodies. PloS Negl Trop Dis (2020) 14:e0007803. doi: 10.1371/journal.pntd.0007803
51. Jin C, Hill J, Gunn BM, Yu WH, Dahora LC, Jones E, et al. Vi-specific serological correlates of protection for typhoid fever. J Exp Med (2021) 218:e00440-18. doi: 10.1084/jem.20201116
52. Dahora LC, Jin C, Spreng RL, Feely F, Mathura R, Seaton KE, et al. IgA and IgG1 specific to Vi polysaccharide of Salmonella Typhi correlate with protection status in a typhoid fever controlled human infection model. Front Immunol (2019) 10:2582. doi: 10.3389/fimmu.2019.02582
53. Collier DA, Ferreira I, Kotagiri P, Datir RP, Lim EY, Touizer E, et al. Age-related immune response heterogeneity to SARS-CoV-2 vaccine BNT162b2. Nature (2021) 596:417–22. doi: 10.1038/s41586-021-03739-1
54. Hess J, Ladel C, Miko D, Kaufmann SH. Salmonella Typhimurium aroA- infection in gene-targeted immunodeficient mice: major role of CD4+ TCR-alpha beta cells and IFN-gamma in bacterial clearance independent of intracellular location. J Immunol (1996) 156:3321–6. doi: 10.4049/jimmunol.156.9.3321
55. MacLennan C, Fieschi C, Lammas DA, Picard C, Dorman SE, Sanal O, et al. Interleukin (IL)-12 and IL-23 are key cytokines for immunity against Salmonella in humans. J Infect Dis (2004) 190:1755–7. doi: 10.1086/425021
56. McSorley SJ, Cookson BT, Jenkins MK. Characterization of CD4+ T cell responses during natural infection with Salmonella Typhimurium. J Immunol (2000) 164:986–93. doi: 10.4049/jimmunol.164.2.986
57. Gordon MA, Jack DL, Dockrell DH, Lee ME, Read RC. Gamma interferon enhances internalization and early nonoxidative killing of Salmonella enterica serovar Typhimurium by human macrophages and modifies cytokine responses. Infect Immun (2005) 73:3445–52. doi: 10.1128/iai.73.6.3445-3452.2005
58. Bhuiyan S, Sayeed A, Khanam F, Leung DT, Rahman Bhuiyan T, Sheikh A, et al. Cellular and cytokine responses to Salmonella enterica serotype Typhi proteins in patients with typhoid fever in Bangladesh. Am J Trop Med Hyg (2014) 90:1024–30. doi: 10.4269/ajtmh.13-0261
59. Fresnay S, McArthur MA, Magder L, Darton TC, Jones C, Waddington CS, et al. Salmonella Typhi-specific multifunctional CD8+ T cells play a dominant role in protection from typhoid fever in humans. J Transl Med (2016) 14:62. doi: 10.1186/s12967-016-0819-7
60. Fresnay S, McArthur MA, Magder LS, Darton TC, Jones C, Waddington CS, et al. Importance of Salmonella Typhi-responsive CD8+ T cell immunity in a human typhoid fever challenge model. Front Immunol (2017) 8:208. doi: 10.3389/fimmu.2017.00208
61. Sztein MB, Booth JS. Controlled human infectious models, a path forward in uncovering immunological correlates of protection: lessons from enteric fevers studies. Front Microbiol (2022) 13:983403. doi: 10.3389/fmicb.2022.983403
62. Liew FY, Li Y, Millott S. Tumor necrosis factor-alpha synergizes with IFN-gamma in mediating killing of Leishmania major through the induction of nitric oxide. J Immunol (1990) 145:4306–10. doi: 10.4049/jimmunol.145.12.4306
63. Westerhof LM, McGuire K, MacLellan L, Flynn A, Gray JI, Thomas M, et al. Multifunctional cytokine production reveals functional superiority of memory CD4 T cells. Eur J Immunol (2019) 49:2019–29. doi: 10.1002/eji.201848026
64. Ross SH, Cantrell DA. Signaling and function of interleukin-2 in T lymphocytes. Annu Rev Immunol (2018) 36:411–33. doi: 10.1146/annurev-immunol-042617-053352
65. Dock J, Ramirez CM, Hultin L, Hausner MA, Hultin P, Elliott J, et al. Distinct aging profiles of CD8+ T cells in blood versus gastrointestinal mucosal compartments. PloS One (2017) 12:e0182498. doi: 10.1371/journal.pone.0182498
66. Sansoni P, Vescovini R, Fagnoni F, Biasini C, Zanni F, Zanlari L, et al. The immune system in extreme longevity. Exp Gerontol (2008) 43:61–5. doi: 10.1016/j.exger.2007.06.008
67. Gustafson CE, Kim C, Weyand CM, Goronzy JJ. Influence of immune aging on vaccine responses. J Allergy Clin Immunol (2020) 145:1309–21. doi: 10.1016/j.jaci.2020.03.017
68. Crooke SN, Ovsyannikova IG, Poland GA, Kennedy RB. Immunosenescence and human vaccine immune responses. Immun Ageing (2019) 16:25. doi: 10.1186/s12979-019-0164-9
69. Masters AR, Hall A, Bartley JM, Keilich SR, Lorenzo EC, Jellison ER, et al. Assessment of lymph node stromal cells as an underlying factor in age-related immune impairment. J Gerontol A Biol Sci Med Sci (2019) 74:1734–43. doi: 10.1093/gerona/glz029
70. Masters AR, Jellison ER, Puddington L, Khanna KM, Haynes L. Attrition of T cell zone fibroblastic reticular cell number and function in aged spleens. Immunohorizons (2018) 2:155–63. doi: 10.4049/immunohorizons.1700062
71. Kityo C, Makamdop KN, Rothenberger M, Chipman JG, Hoskuldsson T, Beilman GJ, et al. Lymphoid tissue fibrosis is associated with impaired vaccine responses. J Clin Invest (2018) 128:2763–73. doi: 10.1172/jci97377
72. Salazar N, Valdés-Varela L, González S, Gueimonde M, de Los Reyes-Gavilán CG. Nutrition and the gut microbiome in the elderly. Gut Microbes (2017) 8:82–97. doi: 10.1080/19490976.2016.1256525
73. Hindle Z, Chatfield SN, Phillimore J, Bentley M, Johnson J, Cosgrove CA, et al. Characterization of Salmonella enterica derivatives harboring defined aroC and Salmonella pathogenicity island 2 type III secretion system (ssaV) mutations by immunization of healthy volunteers. Infect Immun (2002) 70:3457–67. doi: 10.1128/iai.70.7.3457-3467.2002
Keywords: Salmonella, vaccine, live-attenuated, aged, immunosenescence
Citation: Allen JC, Toapanta FR, Baliban SM, Sztein MB and Tennant SM (2023) Reduced immunogenicity of a live Salmonella enterica serovar Typhimurium vaccine in aged mice. Front. Immunol. 14:1190339. doi: 10.3389/fimmu.2023.1190339
Received: 20 March 2023; Accepted: 13 April 2023;
Published: 03 May 2023.
Edited by:
Javier Leceta, Complutense University of Madrid, SpainReviewed by:
Jing Ouyang, Chongqing Public Health Medical Center, ChinaJonathan Lalsiamthara, Oregon Health and Science University, United States
Copyright © 2023 Allen, Toapanta, Baliban, Sztein and Tennant. This is an open-access article distributed under the terms of the Creative Commons Attribution License (CC BY). The use, distribution or reproduction in other forums is permitted, provided the original author(s) and the copyright owner(s) are credited and that the original publication in this journal is cited, in accordance with accepted academic practice. No use, distribution or reproduction is permitted which does not comply with these terms.
*Correspondence: Sharon M. Tennant, c3Rlbm5hbnRAc29tLnVtYXJ5bGFuZC5lZHU=