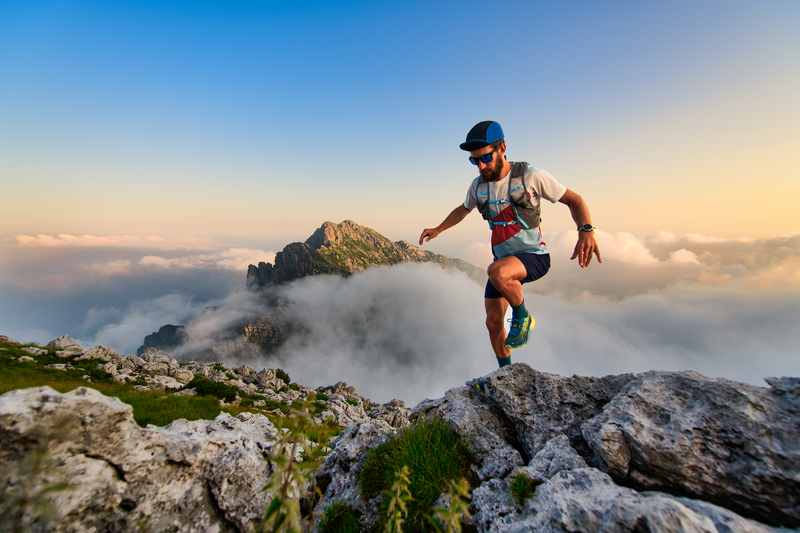
95% of researchers rate our articles as excellent or good
Learn more about the work of our research integrity team to safeguard the quality of each article we publish.
Find out more
MINI REVIEW article
Front. Immunol. , 22 May 2023
Sec. Autoimmune and Autoinflammatory Disorders : Autoimmune Disorders
Volume 14 - 2023 | https://doi.org/10.3389/fimmu.2023.1183909
This article is part of the Research Topic Monitoring immunomodulation strategies in Type 1 Diabetes View all 6 articles
The number of immunotherapeutic clinical trials in type 1 diabetes currently being conducted is expanding, and thus there is a need for robust immune-monitoring assays which are capable of detecting and characterizing islet specific immune responses in peripheral blood. Islet- specific T cells can serve as biomarkers and as such can guide drug selection, dosing regimens and immunological efficacy. Furthermore, these biomarkers can be utilized in patient stratification which can then benchmark suitability for participation in future clinical trials. This review focusses on the commonly used immune-monitoring techniques including multimer and antigen induced marker assays and the potential to combine these with single cell transcriptional profiling which may provide a greater understanding of the mechanisms underlying immuno-intervention. Although challenges remain around some key areas such as the need for harmonizing assays, technological advances mean that multiparametric information derived from a single sample can be used in coordinated efforts to harmonize biomarker discovery and validation. Moreover, the technologies discussed here have the potential to provide a unique insight on the effect of therapies on key players in the pathogenesis of T1D that cannot be obtained using antigen agnostic approaches.
Type 1 diabetes is an autoimmune disease in which islet specific T cells are thought to play a pivotal role in destroying insulin producing β cells (1). Immunotherapy clinical trials in type 1 diabetes is gaining momentum and as more and more trials are performed, there is a need for robust immuno-monitoring assays which are capable of detecting and characterising islet specific immune responses in peripheral blood.
This review will evaluate the use of established assays to monitor changes in islet specific immune responses and examine advanced multiparameter assays that give a greater insight into the functional immune response.
Islet-specific T cells serve as possible biomarkers and as such can guide drug selection and dosing regimens. Furthermore, patients can be stratified by analysing these T cell biomarkers which can then guide suitability for participation in future clinical trials. This underscores the need for robust assays to measure T cell responses in type 1 diabetes.
Assays designed to measure T cell responses in type 1 diabetes need to meet precise requirements. Firstly, they need to be able to monitor responses in peripheral blood as the target organ, the pancreas, cannot be biopsied. Secondly, they need to be sensitive enough to detect cells which occur at low frequency in the blood and have a low affinity TCR. Thirdly, T cells targeting islet antigens can also be detected in healthy controls, so a knowledge of their functional phenotypes is important. Finally, assays measuring T cell responses need to be able to work with low sample volumes as many clinical trials often involve paediatric samples. Table 1 presents a summary of the advantages and disadvantages of experimental approaches which have been extensively used to identify and characterise antigen-specific T cells.
‘Spot’ based assays can detect a wide range of cytokines and are thus commonly used to evaluate functional T cell responses both in research and as diagnostic tools (39–44); they have been used in clinical diagnostic settings. These assays are quantitative, highly sensitive, can measure low-frequency antigen-specific T cells (detection limit 0.0001%) (45) and now are increasingly used in clinical trial monitoring in a variety of settings (46–50). Spot based assays such as ELISpot assays are functional assays and have been used in clinical diagnostic settings such as in tuberculosis diagnosis (51). In type 1 diabetes, these spot-based assays have been pivotal in understanding immune responses (9, 52–56).
In the past few years, the spot-based assay has been further modified using fluorophores attached to the secondary detection antibodies, this assay known as Fluorospot allows the simultaneous detection of T cell subpopulations with distinct cytokine profiles (57). This also has advantages over the ELISpot assay, for example, all cytokines are detected in the same well and therefore fewer PBMCs are needed; it is multiparametric, allowing enumeration of cells which secrete more than one cytokine at a given time (called double or triple secretors). Recently, Fluorospot assays measuring T cells co-producing up to 4 different cytokines have been described ( (58); Jerram et al., manuscript submitted; Domino-Vial personal observations)). (Figure 1).
Figure 1 PBMCs were stimulated with the hexavalent vaccine, Infanrix and Candida albicans, and IL-22 (blue), interferon-γ (green), IL-10 (yellow) and IL-17 (red) T cell responses were measured. T cells making all 4 cytokines are shown in white.
Mechanistic assessment of immune therapies can add a unique insight into understanding clinical trial outcomes. This may be particularly important when trying to interpret trials that have failed to meet their clinical end point. For example, a trial of nasal insulin was started with the idea of reducing pathogenic immune responses to insulin (59). Patients within 1 year of diagnosis self-administered nasal insulin (at a dose was 1.6mg) or placebo (insulin diluent) in the form of a spray daily for 10 days and then weekly on 2 consecutive days for 12 months. Patients were assessed at 3 monthly intervals for 24 months.
The trial failed to meet its clinical end point but suppression of antibody responses to subcutaneous insulin was observed, and in a subgroup of patients, there was also suppression of proinsulin-specific interferonγ T cells as demonstrated using an ELISpot assay. For technical reasons, proinsulin rather than insulin was used in the ELISpot assay, which means that responses could be targeted at epitopes present in proinsulin that are absent in insulin.
Together these data imply that tolerance was induced, providing a rationale to further pursue this strategy in at risk individuals (59).
Another trial that failed to meet its clinical endpoint was the GAD-alum trial (60). GAD65 is a commonly targeted autoantigen in type 1 diabetes, it was combined with alum as an adjuvant and subcutaneously administered to recent-onset patients with type 1 diabetes with the aim of shifting the autoimmune response from a TH1 to a TH2 response. Patients were given 20 µg GAD-alum (thrice in one cohort and twice followed by alum in a second cohort) or 3 injections of alum over 4-12 weeks and observed for a year after which it was clear that GAD-alum treatment did not show any insulin preservation (60). The trial failed in meeting its clinical endpoint but as demonstrated by IL-13 ELISpot, there was a clear induction of GAD-specific TH2 responses in a substantial proportion of patients, indicating a powerful immunological effect (61).
In other instances, mechanistic insights can validate clinical outcomes as shown in the proinsulin peptide C19-A3 trial. Patients with type 1 diabetes treated intradermally with 10µg of peptide C19-A3 either every 2 weeks or every 4 weeks (alternating with placebo) or with placebo (saline) over 6 months, showed maintenance of C-peptide levels. This was accompanied by peptide-specific IL-10 responses, measured by ELISpot, indicating that an immunoregulatory mechanism may be at play (62). In a follow-up study, using a mixture of six HLA-DRB1*0401 selective proinsulin and IA-2 peptides, C-peptide levels were preserved in half of the treated patients compared to none of the placebo treated. Immuno-monitoring in these patients using ELISpot assays, showed higher levels of antigen-specific CD4 T cells producing IL-10 and IL-17 in the treated patients indicating immunomodulatory mechanisms may be in operation (63).
ELISpot assays are useful in antigen-specific immunotherapy as they can measure cytokine responses directly to the antigen used in the clinical trial, unlike in non-antigen specific intervention strategies where the effect is generally more systemic. Furthermore, as an immune-monitoring tool, the ELISpot assay can monitor the efficacy of antigen-specific immunotherapy as demonstrated in a study designed to induce gluten-specific tolerance in patients with celiac disease (64). Patients were given gluten protein encapsulated in nanoparticles (TAK-101) or placebo by intravenous infusion and interferon-γ T cell responses to gliadin were measured by ELISpot at baseline and post therapy. In the treated patients, interferon-γ T cell responses to gliadin were reduced by nearly 90% suggesting that gluten-specific tolerance was induced by TAK-101 (64). In this setting, the ELISpot assay measured the efficacy of tolerance induction, however the method did rely on the need for in vivo gluten challenge which is not possible in type 1 diabetes.
The GAD specific TH2 response elicited by GAD-alum described above was further dissected using a Fluorospot assay which can measure cells co-producing different cytokines. It was observed that GAD specific T cells were bifunctional, producing both interferon-γ and IL-13. It is possible that these bifunctional cells were not able to sufficiently deflect the pathogenic TH1 response and this could explain the lack of clinical efficacy observed with GAD-alum (61). This is further supported by data from a preceding trial in which TH2 cytokines were detected in patients give GAD-alum but interferon-γ and IL-17 were also observed (65).
Spot based assays are also useful in interventions targeting relevant T cell derived proinflammatory cytokines in type 1 diabetes. Ustekinumab targets both IL-12 and IL-23 cytokines thereby blocking the induction of Th1 and Th17 cells respectively (66); it was used recently for the first time in a small cohort of newly diagnosed type 1 diabetes patients (67). This was primarily a safety and dose-finding study where doses of either 45mg or 90 mg were administered subcutaneously; decline in C-peptide levels were lowest at the higher dose of ustekinumab. Immuno-monitoring was conducted using a Fluorospot assay which showed a significant reduction in proinsulin specific interferon-γ and IL-17 T cells at the 90 mg dose but not at the 45 mg dose interestingly, GAD65 specific responses were not reduced at either dose. Thus, in this case, immuno-monitoring served to guide future dose selection. In terms of the mechanistic effect of ustekinumab, the Fluorospot assay demonstrated that pathogenic Th1 and Th17 cells were being restrained.
Both ELISpot and Fluorospots have been extensively used in immuno-monitoring studies in type 1 diabetes and have provided valuable insights into mechanisms underlying therapeutic interventions. These assays have used both fresh and cryopreserved PBMCs with the latter having the advantage of requiring less manpower, reducing day to day variation, and, making it possible to test all time points on the same day. On the downside, there is a loss of cell viability, loss of cell subtypes, spontaneous secretion of some cytokines (21), and loss of specific signals such as IL-10 and IL-4 responses in ELISpot (68).
There is evidence to suggest that IL-10 is especially vulnerable to cryopreservation (69) and this needs to be considered when the mechanism of intervention may be regulatory.
Proliferation assays have been extensively used in type 1 diabetes for decades to monitor immune responses. PBMCs are stimulated with antigen and proliferation tracked either by the incorporation of radiolabeled nucleoside (tritiated thymidine) or by the dilution of a florescent tracker dye (carboxyfluorescein diacetate succinimidyl ester (CFSE), cell trace violet or equivalent).
In a pilot trial, which evaluated subcutaneous versus intra-lymphatic GAD-alum administration in patients with type 1 diabetes, proliferation assays using radiolabeled thymidine showed reduced proliferation to recombinant human GAD65 in those patients given 4 µg GAD-alum intra-lymphatically compared to patients receiving it subcutaneously at 20 µg (70). The authors also reported a concurrent TH2 IL-13 dominated response in subjects with the better clinical responses implying tolerance induction. A recent follow-up study examining immune responses in these two groups after 15 months used proliferation assays with PBMCs stimulated with CD3/CD28 beads to show enhanced proliferation in most of the patients treated with intra-lymphatic GAD-alum compared to the subcutaneously treated patients (71); this also corresponded to significantly lower levels of HbA1c in the former indicating a better clinical outcome. Other parameters such as GAD autoantibodies and GAD induced cytokines also differed between the two groups demonstrating that the route of administration affects the resulting immune response.
Another trial using proinsulin peptide pulsed tolerance inducing dendritic cells (tolDCs) investigated immunological efficacy by using proliferation assays (72). Patients with long standing type 1 diabetes were given 2 escalating doses of proinsulin peptide C19-A3 pulsed tolDCs intradermally or saline (the tolDC vehicle) for one patient in each cohort and monitored for 6 months (73). Proliferation assays demonstrated reduced responses to proinsulin peptide C19-A3 in a third of patients and to whole preproinsulin in all the patients at 6 months with this persisting in the latter in some patients. The reduced proliferative responses were accompanied by an increase in a Treg subset (72), which supports the hypothesis that tolDCs polarize towards a regulatory T cell response (74). Such reduced proliferative responses upon antigen specific immunotherapy have been demonstrated in other settings including multiple sclerosis where autoantigenic peptides coupled to PBMC were used to induce antigen specific tolerance (75).
Proliferation assays in which PBMCs are labeled with fluorescent based dyes such as CFSE are becoming much more common in immuno-monitoring studies (76).
Using this technique PBMC proliferative cell responses were assessed in sequential samples from autoantibody-negative children at high risk of type 1 diabetes recruited into an oral insulin trial (Pre-POINT) (77); children were administered placebo or assigned in blocks to receive insulin at escalating doses from 2.5–67.5 mg. It is thought that oral administration of antigen could promote regulatory T cells (78). The cells proliferating to insulin were subsequently stained with a panel of antibodies and proliferating CD4+ T cells were single cell sorted and gene expression profiles analyzed. The data showed that Pre-POINT administration in high-risk individuals resulted in regulatory, insulin responsive cells. This approach allowed the authors to probe the response more extensively than using proliferation alone.
MHC multimers consist of complexes of HLA molecules combined with a specific peptide and conjugated to a fluorochrome (79) or rare metal ions which can then be detected using conventional flow cytometry (80) or mass spectrometry (81) respectively. MHC multimer technology has been shown to be an effective tool in the detection of rare antigen specific CD4 and CD8 T cells in type 1 diabetes (23, 82–85). These enumerating assays can be combined with downstream processes to phenotype cells (86, 87), or gain insights into TCR sequences (88).
In type 1 diabetes clinical trials, MHC multimer technology has been used in several trials (89–91). For example, it has been used to monitor, CD4 T cell responses in GAD–alum treated patients; newly diagnosed patients were given either one of 2 doses of GAD-alum or alum as a placebo and immune responses were monitored (90). The frequency of CD4 T cells recognized by GAD peptide loaded tetramers was increased in GAD-alum treated patients compared to those given placebo; subsequent phenotyping identified these cells as effector memory cells suggesting that this was a recall response where the cells were activated specifically in response to GAD-alum (90).
Standard MHC multimer technology uses tetramers loaded with a single peptide which can be limiting when monitoring multiple epitope specific T cell populations. This can be circumvented using a combinatorial labelling approach, allowing parallel assessment of multiple specificities through the use of individual tetramers labelled with more than one marker (fluorochrome or rare element) thus facilitating the detection of a large number of epitope specific T cells (>25) (92) in a single clinical sample (93). This approach can be successfully applied to antigenic peptides presented by both HLA class I and class II molecules, and it has recently been shown to provide a more informative and discriminatory measurement of islet specific CD8+ and CD4+ T cell frequency, respectively (24, 82).
This technique was used to investigate the effect of administration of a plasmid encoding proinsulin (BHT-3021) on islet specific CD8 T cells with a range of antigen and epitope specificities (89). Patients were given either a BHT-placebo or BHT-3021 at 4 different doses (ranging from 0.3 mg to 6 mg) intramuscularly for 12 weeks. C-peptide together with antigen-specific CD8 T cell responses to several islet antigen peptides were measured thereafter. The authors reported an increase in C-peptide in the BHT-3021 treated group compared to placebo with a concurrent decrease in proinsulin specific CD8 T cells (89).
Similarly, a trial employing autologous hematopoietic stem cell transplantation (AHSCT) where recent-onset type 1 diabetes patients were given an infusion of autologous hematopoietic stem cells used this technique to show that better clinical outcome was linked to baseline autoreactivity. The levels of islet antigen specific CD8 T cells did not change upon AHSCT but higher C-peptide levels and a longer diabetes free period were observed in patients with low CD8 islet autoreactivity at baseline prior to AHSCT (91).
Activation Induced Marker (AIM) assays are used to study antigen reactive T-cells. AIM assays identify responding cells based on upregulation of cell surface markers associated with TCR engagement following incubation of PBMC with recombinant antigen or peptides. In theory, these assays make little prior assumption about the phenotype of responding cells, which can be captured for downstream analysis using methods such as single cell RNA-Seq (scRNA-Seq). The most common version of this assay uses upregulation of CD69 and CD154 to identify antigen-specific T cells (31) and has been used to study islet specific T cell responses (33, 94).
AIM assays have been used for monitoring in intervention trials in T1D (95); they are highly informative in both identifying the phenotypes of responding T cells and in providing an insight into quantitative changes in distinct cell populations. This has been demonstrated in the aforementioned intralymphatic GAD-alum trial where administration resulted in an immunomodulatory response; phenotypic profiling of the immune cells showed an induction of PD-1+ T follicular helper cells and exhausted CD8 T cells (95).
Although the inclusion of additional surface or intracellular markers in an AIM assay does provide additional information about the phenotype of the responding cells, unbiased analysis techniques may provide a greater understanding of the mechanisms underlying immuno-intervention. This can be achieved by the combination of AIM assays with single cell transcriptional profiling such as using plate-based methods (sorting directly into 96-well plates, e.g., SMART-seq), droplet-based technologies (e.g. 10x Chromium) or cartridge-based techniques (e.g. BD Rhapsody). Each technology has strengths and limitations as discussed elsewhere (96). The 10x Genomics platform has the advantage over both the plate- and cartridge- based platforms in that it can capture and process high numbers of cells in a single experiment (high-throughput sequencing) (97) however, it does incur cell loss (up to 50%) (98, 99) In contrast, cell loss is lowest when using plate-based methods, but this is significantly more expensive and time consuming (96, 100).
This has been tested on PBMCs stimulated with GAD and proinsulin from patients with type 1 diabetes and healthy controls, and in each case, 100-500 autoantigen specific cells were profiled; these were subsequently identified using cell surface markers and further characterized by examining differentially expressed genes within the various cell populations (E. Christakou et al., manuscript in preparation) (Figure 2).
Figure 2 Use of the AIM 10x assay to investigate islet-specific T cells. A schematic diagram showing combination of AIM and s.c. RNA-Seq to characterize antigen-specific T cells.
Although the cost of AIM assays combined with single cell RNA-Seq can be prohibitive when testing numerous clinical trial samples, barcoding technology allows samples from many individuals/timepoints to be pooled, and deconvoluted downstream. This together with the depth of unbiased information generated makes this technology a potential avenue to pursue when investigating mechanism of action in immunotherapy trials.
Up until now, no studies using this technology in the clinical trial setting have yet been published, however, currently a trial is underway. The IMCY-0098 Proof of ACtion in Type 1 Diabetes (IMPACT) trial (clinicaltrials.gov/NCT04524949) will test a proinsulin-derived mimotope in patients with diabetes and use AIM and RNA-Seq to characterize the subsequent immune signature to identify treatment-specific biomarkers. Single cell profiling in the absence of AIM has been used in an antigen agnostic setting; it has been used to examine the immune response in patients with type 1 diabetes after a combined low-dose IL-2 and Treg adoptive cell therapy (patients were given escalating doses of T regs from 3-20 x106/kg). Patients undergoing this treatment had high number of T regs and subsequent gene expression data showed upregulation of functional T reg markers such as FOXP3, GITR, GARP and IKAROS amongst others (101). A second trial used single cell multiomics to profile PBMCs from patients with long standing diabetes treated with low-dose IL-2 at 3-day intervals at doses ranging from 0.2 to 0.47 × 106 IU/m2 and reported an expansion of FOXP3+ HELIOS+ T regs in treated patients (102).
Whilst detection of islet specific T cells remains a challenge, the technologies discussed here have the potential to provide a unique insight on the effect of therapies on key players in the pathogenesis of T1D that cannot be obtained using antigen agnostic approaches.
Although challenges remain around some key areas such as the number of cells required and the need for harmonising assays, technological advances mean that multiparametric information derived from a single sample can be used in coordinated efforts to harmonize biomarker discovery and validation (82, 103).
SA, EW, EP, EC and CD-V performed a review of the literature; SA, EW, EC and CD-V wrote the manuscript. SA conceptualized and supervised the writing of the manuscript. TT provided expertise on aspects of the manuscript and edited. All authors contributed to the article and approved the submitted version.
This work is supported by an Innovative Medicines Initiative 2 Joint Undertaking under grant agreement no. 115797 (INNODIA), which receives support from the European Union’s Horizon 2020 research and innovation programme and EFPIA, JDRF, and The Leona M. and Harry B. Helmsley Charitable Trust. Flow cytometry studies and patient recruitment were supported by the T1DUK Immunotherapy Consortium funded by grants from Diabetes UK (Ref: 15/0005232 and 15/0005233).
The authors declare that the research was conducted in the absence of any commercial or financial relationships that could be construed as a potential conflict of interest.
All claims expressed in this article are solely those of the authors and do not necessarily represent those of their affiliated organizations, or those of the publisher, the editors and the reviewers. Any product that may be evaluated in this article, or claim that may be made by its manufacturer, is not guaranteed or endorsed by the publisher.
1. Bluestone JA, Buckner JH, Herold KC. Immunotherapy: building a bridge to a cure for type 1 diabetes. Science (2021) 373(6554):510–6. doi: 10.1126/science.abh1654
2. Arif S, Gibson VB, Nguyen V, Bingley PJ, Todd JA, Guy C, et al. Beta-cell specific T-lymphocyte response has a distinct inflammatory phenotype in children with type 1 diabetes compared with adults. Diabetes Med (2017) 34(3):419–25. doi: 10.1111/dme.13153
3. Arif S, Moore F, Marks K, Bouckenooghe T, Dayan CM, Planas R, et al. Peripheral and islet interleukin-17 pathway activation characterizes human autoimmune diabetes and promotes cytokine-mediated beta-cell death. Diabetes (2011) 60(8):2112–9. doi: 10.2337/db10-1643
4. Mannering SI, Wong FS, Durinovic-Bello I, Brooks-Worrell B, Tree TI, Cilio CM, et al. Current approaches to measuring human islet-antigen specific T cell function in type 1 diabetes. Clin Exp Immunol (2010) 162(2):197–209. doi: 10.1111/j.1365-2249.2010.04237.x
5. Das A, Rouault-Pierre K, Kamdar S, Gomez-Tourino I, Wood K, Donaldson I, et al. Adaptive from innate: human IFN-gamma(+)CD4(+) T cells can arise directly from CXCL8-producing recent thymic emigrants in babies and adults. J Immunol (2017) 199(5):1696–705. doi: 10.4049/jimmunol.1700551
6. Herold KC, Brooks-Worrell B, Palmer J, Dosch HM, Peakman M, Gottlieb P, et al. Validity and reproducibility of measurement of islet autoreactivity by T-cell assays in subjects with early type 1 diabetes. Diabetes (2009) 58(11):2588–95. doi: 10.2337/db09-0249
7. Schloot NC, Meierhoff G, Karlsson Faresjo M, Ott P, Putnam A, Lehmann P, et al. Comparison of cytokine ELISpot assay formats for the detection of islet antigen autoreactive T cells. report of the third immunology of diabetes society T-cell workshop. J Autoimmun (2003) 21(4):365–76. doi: 10.1016/S0896-8411(03)00111-2
8. Meierhoff G, Ott PA, Lehmann PV, Schloot NC. Cytokine detection by ELISPOT: relevance for immunological studies in type 1 diabetes. Diabetes Metab Res Rev (2002) 18(5):367–80. doi: 10.1002/dmrr.320
9. Arif S, Leete P, Nguyen V, Marks K, Nor NM, Estorninho M, et al. Blood and islet phenotypes indicate immunological heterogeneity in type 1 diabetes. Diabetes (2014) 63(11):3835–45. doi: 10.2337/db14-0365
10. Alleva DG, Crowe PD, Jin L, Kwok WW, Ling N, Gottschalk M, et al. A disease-associated cellular immune response in type 1 diabetics to an immunodominant epitope of insulin. J Clin Invest (2001) 107(2):173–80. doi: 10.1172/JCI8525
11. Arif S, Tree TI, Astill TP, Tremble JM, Bishop AJ, Dayan CM, et al. Autoreactive T cell responses show proinflammatory polarization in diabetes but a regulatory phenotype in health. J Clin Invest (2004) 113(3):451–63. doi: 10.1172/JCI19585
12. Nakayama M, McDaniel K, Fitzgerald-Miller L, Kiekhaefer C, Snell-Bergeon JK, Davidson HW, et al. Regulatory vs. inflammatory cytokine T-cell responses to mutated insulin peptides in healthy and type 1 diabetic subjects. Proc Natl Acad Sci U S A. (2015) 112(14):4429–34. doi: 10.1073/pnas.1502967112
13. van Lummel M, Duinkerken G, van Veelen PA, de Ru A, Cordfunke R, Zaldumbide A, et al. Posttranslational modification of HLA-DQ binding islet autoantigens in type 1 diabetes. Diabetes (2014) 63(1):237–47. doi: 10.2337/db12-1214
14. Musthaffa Y, Nel HJ, Ramnoruth N, Patel S, Hamilton-Williams EE, Harris M, et al. Optimization of a method to detect autoantigen-specific T-cell responses in type 1 diabetes. Front Immunol (2020) 11:587469. doi: 10.3389/fimmu.2020.587469
15. Mannering SI, Dromey JA, Morris JS, Thearle DJ, Jensen KP, Harrison LC. An efficient method for cloning human autoantigen-specific T cells. J Immunol Methods (2005) 298(1-2):83–92. doi: 10.1016/j.jim.2005.01.001
16. Mannering SI, Morris JS, Jensen KP, Purcell AW, Honeyman MC, van Endert PM, et al. A sensitive method for detecting proliferation of rare autoantigen-specific human T cells. J Immunol Methods (2003) 283(1-2):173–83. doi: 10.1016/j.jim.2003.09.004
17. So M, Elso CM, Tresoldi E, Pakusch M, Pathiraja V, Wentworth JM, et al. Proinsulin c-peptide is an autoantigen in people with type 1 diabetes. Proc Natl Acad Sci U S A. (2018) 115(42):10732–7. doi: 10.1073/pnas.1809208115
18. Di Carluccio AR, Tresoldi E, So M, Mannering SI. Quantification of proliferating human antigen-specific CD4+ T cells using carboxyfluorescein succinimidyl ester. J Vis Exp (2019) 148). doi: 10.3791/59545-v
19. Velthuis JH, Unger WW, Abreu JR, Duinkerken G, Franken K, Peakman M, et al. Simultaneous detection of circulating autoreactive CD8+ T-cells specific for different islet cell-associated epitopes using combinatorial MHC multimers. Diabetes (2010) 59(7):1721–30. doi: 10.2337/db09-1486
20. Dolton G, Zervoudi E, Rius C, Wall A, Thomas HL, Fuller A, et al. Optimized peptide-MHC multimer protocols for detection and isolation of autoimmune T-cells. Front Immunol (2018) 9:1378. doi: 10.3389/fimmu.2018.01378
21. Mallone R, Mannering SI, Brooks-Worrell BM, Durinovic-Bello I, Cilio CM, Wong FS, et al. Isolation and preservation of peripheral blood mononuclear cells for analysis of islet antigen-reactive T cell responses: position statement of the T-cell workshop committee of the immunology of diabetes society. Clin Exp Immunol (2011) 163(1):33–49. doi: 10.1111/j.1365-2249.2010.04272.x
22. James EA, Mallone R, Schloot NC, Gagnerault MC, Thorpe J, Fitzgerald-Miller L, et al. Immunology of diabetes society T-cell workshop: HLA class II tetramer-directed epitope validation initiative. Diabetes Metab Res Rev (2011) 27(8):727–36. doi: 10.1002/dmrr.1244
23. Blahnik G, Uchtenhagen H, Chow IT, Speake C, Greenbaum C, Kwok WW, et al. Analysis of pancreatic beta cell specific CD4+ T cells reveals a predominance of proinsulin specific cells. Cell Immunol (2019) 335:68–75. doi: 10.1016/j.cellimm.2018.11.004
24. Uchtenhagen H, Rims C, Blahnik G, Chow IT, Kwok WW, Buckner JH, et al. Efficient ex vivo analysis of CD4+ T-cell responses using combinatorial HLA class II tetramer staining. Nat Commun (2016) 7:12614. doi: 10.1038/ncomms12614
25. Hadrup SR, Bakker AH, Shu CJ, Andersen RS, van Veluw J, Hombrink P, et al. Parallel detection of antigen-specific T-cell responses by multidimensional encoding of MHC multimers. Nat Methods (2009) 6(7):520–6. doi: 10.1038/nmeth.1345
26. Unger WW, Velthuis J, Abreu JR, Laban S, Quinten E, Kester MG, et al. Discovery of low-affinity preproinsulin epitopes and detection of autoreactive CD8 T-cells using combinatorial MHC multimers. J Autoimmun (2011) 37(3):151–9. doi: 10.1016/j.jaut.2011.05.012
27. Spanier JA, Sahli NL, Wilson JC, Martinov T, Dileepan T, Burrack AL, et al. Increased effector memory insulin-specific CD4(+) T cells correlate with insulin autoantibodies in patients with recent-onset type 1 diabetes. Diabetes (2017) 66(12):3051–60. doi: 10.2337/db17-0666
28. Mallone R, Scotto M, Janicki CN, James EA, Fitzgerald-Miller L, Wagner R, et al. Immunology of diabetes society T-cell workshop: HLA class I tetramer-directed epitope validation initiative T-cell workshop report-HLA class I tetramer validation initiative. Diabetes Metab Res Rev (2011) 27(8):720–6. doi: 10.1002/dmrr.1243
29. Chow IT, Yang J, Gates TJ, James EA, Mai DT, Greenbaum C, et al. Assessment of CD4+ T cell responses to glutamic acid decarboxylase 65 using DQ8 tetramers reveals a pathogenic role of GAD65 121-140 and GAD65 250-266 in T1D development. PloS One (2014) 9(11):e112882. doi: 10.1371/journal.pone.0112882
30. Reiss S, Baxter AE, Cirelli KM, Dan JM, Morou A, Daigneault A, et al. Comparative analysis of activation induced marker (AIM) assays for sensitive identification of antigen-specific CD4 T cells. PloS One (2017) 12(10):e0186998. doi: 10.1371/journal.pone.0186998
31. Chattopadhyay PK, Yu J, Roederer M. Live-cell assay to detect antigen-specific CD4+ T-cell responses by CD154 expression. Nat Protoc (2006) 1(1):1–6. doi: 10.1038/nprot.2006.1
32. Grifoni A, Voic H, Dhanda SK, Kidd CK, Brien JD, Buus S, et al. T Cell responses induced by attenuated flavivirus vaccination are specific and show limited cross-reactivity with other flavivirus species. J Virol (2020) 94(10). doi: 10.1128/JVI.00089-20
33. Cerosaletti K, Barahmand-Pour-Whitman F, Yang J, DeBerg HA, Dufort MJ, Murray SA, et al. Single-cell RNA sequencing reveals expanded clones of islet antigen-reactive CD4(+) T cells in peripheral blood of subjects with type 1 diabetes. J Immunol (2017) 199(1):323–35. doi: 10.4049/jimmunol.1700172
34. Schoenbrunn A, Frentsch M, Kohler S, Keye J, Dooms H, Moewes B, et al. A converse 4-1BB and CD40 ligand expression pattern delineates activated regulatory T cells (Treg) and conventional T cells enabling direct isolation of alloantigen-reactive natural Foxp3+ treg. J Immunol (2012) 189(12):5985–94. doi: 10.4049/jimmunol.1201090
35. Osterdahl MF, Christakou E, Hart D, Harris F, Shahrabi Y, Pollock E, et al. Concordance of b- and T-cell responses to SARS-CoV-2 infection, irrespective of symptoms suggestive of COVID-19. J Med Virol (2022) 94(11):5217–24. doi: 10.1002/jmv.28016
36. Sauerwein KMT, Geier CB, Stemberger RF, Akyaman H, Illes P, Fischer MB, et al. Antigen-specific CD4(+) T-cell activation in primary antibody deficiency after BNT162b2 mRNA COVID-19 vaccination. Front Immunol (2022) 13:827048. doi: 10.3389/fimmu.2022.827048
37. Grifoni A, Weiskopf D, Ramirez SI, Mateus J, Dan JM, Moderbacher CR, et al. Targets of T cell responses to SARS-CoV-2 coronavirus in humans with COVID-19 disease and unexposed individuals. Cell (2020) 181(7):1489–501 e15. doi: 10.1016/j.cell.2020.05.015
38. Chattopadhyay PK, Yu J, Roederer M. A live-cell assay to detect antigen-specific CD4+ T cells with diverse cytokine profiles. Nat Med (2005) 11(10):1113–7. doi: 10.1038/nm1293
39. Ranieri E, Netti GS, Gigante M. CTL ELISPOT assay and T cell detection. Methods Mol Biol (2021) 2325:65–77. doi: 10.1007/978-1-0716-1507-2_5
40. Tang Y, Zhu L, Xu Q, Zhang X, Li B, Lee LJ. The co-stimulation of anti-CD28 and IL-2 enhances the sensitivity of ELISPOT assays for detection of neoantigen-specific T cells in PBMC. J Immunol Methods (2020) 484-485:112831. doi: 10.1016/j.jim.2020.112831
41. Dissanayake K, Jayasinghe C, Wanigasekara P, Sominanda A. Potential applicability of cytokines as biomarkers of disease activity in rheumatoid arthritis: enzyme-linked immunosorbent spot assay-based evaluation of TNF-alpha, IL-1beta, IL-10 and IL-17A. PloS One (2021) 16(1):e0246111. doi: 10.1371/journal.pone.0246111
42. Thummler L, Schwarzkopf S, Knop D, Ross JA, Berg V, Horn PA, et al. Comparison of SARS-CoV-2- and HCoV-specific T cell response using IFN-gamma ELISpot. Diagnostics (Basel) (2021) 11(8). doi: 10.3390/diagnostics11081439
43. Chen C, Jiang X, Liu X, Guo L, Wang W, Gu S, et al. Identification of the association between HBcAg-specific T cell and viral control in chronic HBV infection using a cultured ELISPOT assay. J Leukoc Biol (2021) 109(2):455–65. doi: 10.1002/JLB.5MA0620-023RR
44. von Essen MR, Ammitzboll C, Bornsen L, Sellebjerg F. Assessment of commonly used methods to determine myelin-reactivity of T cells in multiple sclerosis. Clin Immunol (2021) 230:108817. doi: 10.1016/j.clim.2021.108817
45. Lehmann PV, Zhang W. Unique strengths of ELISPOT for T cell diagnostics. Methods Mol Biol (2012) 792:3–23. doi: 10.1007/978-1-61779-325-7_1
46. Langat RK, Farah B, Indangasi J, Ogola S, Omosa-Manyonyi G, Anzala O, et al. Performance of international AIDS vaccine initiative African clinical research laboratories in standardised ELISpot and peripheral blood mononuclear cell processing in support of HIV vaccine clinical trials. Afr J Lab Med (2021) 10(1):1056. doi: 10.4102/ajlm.v10i1.1056
47. De Keersmaecker B, Claerhout S, Carrasco J, Bar I, Corthals J, Wilgenhof S, et al. TriMix and tumor antigen mRNA electroporated dendritic cell vaccination plus ipilimumab: link between T-cell activation and clinical responses in advanced melanoma. J Immunother Cancer (2020) 8(1). doi: 10.1136/jitc-2019-000329
48. Eberhardson M, Hall S, Papp KA, Sterling TM, Stek JE, Pang L, et al. Safety and immunogenicity of inactivated varicella-zoster virus vaccine in adults with autoimmune disease: a phase 2, randomized, double-blind, placebo-controlled clinical trial. Clin Infect Dis (2017) 65(7):1174–82. doi: 10.1093/cid/cix484
49. Kister I, Patskovsky Y, Curtin R, Pei J, Perdomo K, Rimler Z, et al. Cellular and humoral immunity to SARS-CoV-2 infection in multiple sclerosis patients on ocrelizumab and other disease-modifying therapies: a multi-ethnic observational study. Ann Neurol (2022) 91(6):782–95. doi: 10.1002/ana.26346
50. Curtis JR, Cofield SS, Bridges SL Jr., Bassler J, Deodhar A, Ford TL, et al. The safety and immunologic effectiveness of the live varicella-zoster vaccine in patients receiving tumor necrosis factor inhibitor therapy : a randomized controlled trial. Ann Intern Med (2021) 174(11):1510–8. doi: 10.7326/M20-6928
51. Meier T, Enders M. High reproducibility of the interferon-gamma release assay T-SPOT.TB in serial testing. Eur J Clin Microbiol Infect Dis (2021) 40(1):85–93. doi: 10.1007/s10096-020-03997-3
52. Arif S, Pujol-Autonell I, Kamra Y, Williams E, Yusuf N, Domingo-Vila C, et al. Mapping T cell responses to native and neo-islet antigen epitopes in at risk and type 1 diabetes subjects. Front Immunol (2021) 12:675746. doi: 10.3389/fimmu.2021.675746
53. Arif S, Yusuf N, Domingo-Vila C, Liu YF, Bingley PJ, Peakman M. Evaluating T cell responses prior to the onset of type 1 diabetes. Diabetes Med (2022) 39(9):e14860. doi: 10.1111/dme.14860
54. Hedman M, Ludvigsson J, Faresjo MK. Nicotinamide reduces high secretion of IFN-gamma in high-risk relatives even though it does not prevent type 1 diabetes. J Interferon Cytokine Res (2006) 26(4):207–13. doi: 10.1089/jir.2006.26.207
55. Karlsson MG, Lawesson SS, Ludvigsson J. Th1-like dominance in high-risk first-degree relatives of type I diabetic patients. Diabetologia (2000) 43(6):742–9. doi: 10.1007/s001250051372
56. Karlsson MG, Ludvigsson J. The ABBOS-peptide from bovine serum albumin causes an IFN-gamma and IL-4 mRNA response in lymphocytes from children with recent onset of type 1 diabetes. Diabetes Res Clin Pract (2000) 47(3):199–207. doi: 10.1016/S0168-8227(99)00127-8
57. Gazagne A, Claret E, Wijdenes J, Yssel H, Bousquet F, Levy E, et al. A fluorospot assay to detect single T lymphocytes simultaneously producing multiple cytokines. J Immunol Methods (2003) 283(1-2):91–8. doi: 10.1016/j.jim.2003.08.013
58. Karulin AY, Megyesi Z, Caspell R, Hanson J, Lehmann PV. Multiplexing T- and b-cell FLUOROSPOT assays: experimental validation of the multi-color ImmunoSpot((R)) software based on center of mass distance algorithm. Methods Mol Biol (2018) 1808:95–113. doi: 10.1007/978-1-4939-8567-8_9
59. Fourlanos S, Perry C, Gellert SA, Martinuzzi E, Mallone R, Butler J, et al. Evidence that nasal insulin induces immune tolerance to insulin in adults with autoimmune diabetes. Diabetes (2011) 60(4):1237–45. doi: 10.2337/db10-1360
60. Wherrett DK, Bundy B, Becker DJ, DiMeglio LA, Gitelman SE, Goland R, et al. Antigen-based therapy with glutamic acid decarboxylase (GAD) vaccine in patients with recent-onset type 1 diabetes: a randomised double-blind trial. Lancet (2011) 378(9788):319–27. doi: 10.1016/S0140-6736(11)60895-7
61. Arif S, Gomez-Tourino I, Kamra Y, Pujol-Autonell I, Hanton E, Tree T, et al. GAD-alum immunotherapy in type 1 diabetes expands bifunctional Th1/Th2 autoreactive CD4 T cells. Diabetologia (2020) 63(6):1186–98. doi: 10.1007/s00125-020-05130-7
62. Alhadj Ali M, Liu YF, Arif S, Tatovic D, Shariff H, Gibson VB, et al. Metabolic and immune effects of immunotherapy with proinsulin peptide in human new-onset type 1 diabetes. Sci Transl Med (2017) 9(402). doi: 10.1126/scitranslmed.aaf7779
63. Liu YF, Powrie J, Arif S, Yang JHM, Williams E, Khatri L, et al. Immune and metabolic effects of antigen-specific immunotherapy using multiple beta-cell peptides in type 1 diabetes. Diabetes (2022) 71(4):722–32. doi: 10.2337/db21-0728
64. Kelly CP, Murray JA, Leffler DA, Getts DR, Bledsoe AC, Smithson G, et al. TAK-101 nanoparticles induce gluten-specific tolerance in celiac disease: a randomized, double-blind, placebo-controlled study. Gastroenterology (2021) 161(1):66–80 e8. doi: 10.1053/j.gastro.2021.03.014
65. Axelsson S, Cheramy M, Akerman L, Pihl M, Ludvigsson J, Casas R. Cellular and humoral immune responses in type 1 diabetic patients participating in a phase III GAD-alum intervention trial. Diabetes Care (2013) 36(11):3418–24. doi: 10.2337/dc12-2251
66. Benson JM, Sachs CW, Treacy G, Zhou H, Pendley CE, Brodmerkel CM, et al. Therapeutic targeting of the IL-12/23 pathways: generation and characterization of ustekinumab. Nat Biotechnol (2011) 29(7):615–24. doi: 10.1038/nbt.1903
67. Marwaha AK, Chow S, Pesenacker AM, Cook L, Sun A, Long SA, et al. A phase 1b open-label dose-finding study of ustekinumab in young adults with type 1 diabetes. Immunother Adv (2022) 2(1):ltab022. doi: 10.1093/immadv/ltab022
68. Kvarnstrom M, Jenmalm MC, Ekerfelt C. Effect of cryopreservation on expression of Th1 and Th2 cytokines in blood mononuclear cells from patients with different cytokine profiles, analysed with three common assays: an overall decrease of interleukin-4. Cryobiology (2004) 49(2):157–68. doi: 10.1016/j.cryobiol.2004.06.003
69. Shreffler WG, Visness CM, Burger M, Cruikshank WW, Lederman HM, de la Morena M, et al. Standardization and performance evaluation of mononuclear cell cytokine secretion assays in a multicenter study. BMC Immunol (2006) 7:29. doi: 10.1186/1471-2172-7-29
70. Tavira B, Barcenilla H, Wahlberg J, Achenbach P, Ludvigsson J, Casas R. Intralymphatic glutamic acid decarboxylase-alum administration induced Th2-Like-Specific immunomodulation in responder patients: a pilot clinical trial in type 1 diabetes. J Diabetes Res (2018) 2018:9391845. doi: 10.1155/2018/9391845
71. Dietrich F, Barcenilla H, Tavira B, Wahlberg J, Achenbach P, Ludvigsson J, et al. Immune response differs between intralymphatic or subcutaneous administration of GAD-alum in individuals with recent onset type 1 diabetes. Diabetes Metab Res Rev (2022) 38(3):e3500. doi: 10.1002/dmrr.3500
72. Nikolic T, Suwandi JS, Wesselius J, Laban S, Joosten AM, Sonneveld P, et al. Tolerogenic dendritic cells pulsed with islet antigen induce long-term reduction in T-cell autoreactivity in type 1 diabetes patients. Front Immunol (2022) 13:1054968. doi: 10.3389/fimmu.2022.1054968
73. Nikolic T, Zwaginga JJ, Uitbeijerse BS, Woittiez NJ, de Koning EJ, Aanstoot HJ, et al. Safety and feasibility of intradermal injection with tolerogenic dendritic cells pulsed with proinsulin peptide-for type 1 diabetes. Lancet Diabetes Endocrinol (2020) 8(6):470–2. doi: 10.1016/S2213-8587(20)30104-2
74. Manicassamy S, Pulendran B. Dendritic cell control of tolerogenic responses. Immunol Rev (2011) 241(1):206–27. doi: 10.1111/j.1600-065X.2011.01015.x
75. Lutterotti A, Martin R. Antigen-specific tolerization approaches in multiple sclerosis. Expert Opin Investig Drugs (2014) 23(1):9–20. doi: 10.1517/13543784.2014.844788
76. Ten Brinke A, Marek-Trzonkowska N, Mansilla MJ, Turksma AW, Piekarska K, Iwaszkiewicz-Grzes D, et al. Monitoring T-cell responses in translational studies: optimization of dye-based proliferation assay for evaluation of antigen-specific responses. Front Immunol (2017) 8:1870. doi: 10.3389/fimmu.2017.01870
77. Bonifacio E, Ziegler AG, Klingensmith G, Schober E, Bingley PJ, Rottenkolber M, et al. Effects of high-dose oral insulin on immune responses in children at high risk for type 1 diabetes: the pre-POINT randomized clinical trial. JAMA (2015) 313(15):1541–9. doi: 10.1001/jama.2015.2928
78. Syed A, Garcia MA, Lyu SC, Bucayu R, Kohli A, Ishida S, et al. Peanut oral immunotherapy results in increased antigen-induced regulatory T-cell function and hypomethylation of forkhead box protein 3 (FOXP3). J Allergy Clin Immunol (2014) 133(2):500–10. doi: 10.1016/j.jaci.2013.12.1037
79. Altman JD, Moss PA, Goulder PJ, Barouch DH, McHeyzer-Williams MG, Bell JI, et al. Phenotypic analysis of antigen-specific T lymphocytes. Science (1996) 274(5284):94–6. doi: 10.1126/science.274.5284.94
80. Klenerman P, Tolfvenstam T, Price DA, Nixon DF, Broliden K, Oxenius A. T Lymphocyte responses against human parvovirus B19: small virus, big response. Pathol Biol (Paris) (2002) 50(5):317–25. doi: 10.1016/S0369-8114(02)00306-1
81. Newell EW, Sigal N, Nair N, Kidd BA, Greenberg HB, Davis MM. Combinatorial tetramer staining and mass cytometry analysis facilitate T-cell epitope mapping and characterization. Nat Biotechnol (2013) 31(7):623–9. doi: 10.1038/nbt.2593
82. James EA, Abreu JRF, McGinty JW, Odegard JM, Fillie YE, Hocter CN, et al. Combinatorial detection of autoreactive CD8(+) T cells with HLA-A2 multimers: a multi-centre study by the immunology of diabetes society T cell workshop. Diabetologia (2018) 61(3):658–70. doi: 10.1007/s00125-017-4508-8
83. Perri V, Gianchecchi E, Cifaldi L, Pellegrino M, Giorda E, Andreani M, et al. Identification of GAD65 AA 114-122 reactive 'memory-like' NK cells in newly diagnosed type 1 diabetic patients by HLA-class I pentamers. PloS One (2017) 12(12):e0189615. doi: 10.1371/journal.pone.0189615
84. Anderson AM, Landry LG, Alkanani AA, Pyle L, Powers AC, Atkinson MA, et al. Human islet T cells are highly reactive to preproinsulin in type 1 diabetes. Proc Natl Acad Sci U.S.A. (2021) 118(41). doi: 10.1073/pnas.2107208118
85. Hanna SJ, Powell WE, Long AE, Robinson EJS, Davies J, Megson C, et al. Slow progressors to type 1 diabetes lose islet autoantibodies over time, have few islet antigen-specific CD8(+) T cells and exhibit a distinct CD95(hi) b cell phenotype. Diabetologia (2020) 63(6):1174–85. doi: 10.1007/s00125-020-05114-7
86. Yeo L, Pujol-Autonell I, Baptista R, Eichmann M, Kronenberg-Versteeg D, Heck S, et al. Circulating beta cell-specific CD8(+) T cells restricted by high-risk HLA class I molecules show antigen experience in children with and at risk of type 1 diabetes. Clin Exp Immunol (2020) 199(3):263–77. doi: 10.1111/cei.13391
87. Wiedeman AE, Muir VS, Rosasco MG, DeBerg HA, Presnell S, Haas B, et al. Autoreactive CD8+ T cell exhaustion distinguishes subjects with slow type 1 diabetes progression. J Clin Invest (2020) 130(1):480–90. doi: 10.1172/JCI126595
88. Zhang SQ, Ma KY, Schonnesen AA, Zhang M, He C, Sun E, et al. High-throughput determination of the antigen specificities of T cell receptors in single cells. Nat Biotechnol (2018) 36, 1156–1159. doi: 10.1101/457069
89. Roep BO, Solvason N, Gottlieb PA, Abreu JRF, Harrison LC, Eisenbarth GS, et al. Plasmid-encoded proinsulin preserves c-peptide while specifically reducing proinsulin-specific CD8(+) T cells in type 1 diabetes. Sci Transl Med (2013) 5(191):191ra82. doi: 10.1126/scitranslmed.3006103
90. Pihl M, Barcenilla H, Axelsson S, Cheramy M, Akerman L, Johansson I, et al. GAD-specific T cells are induced by GAD-alum treatment in type-1 diabetes patients. Clin Immunol (2017) 176:114–21. doi: 10.1016/j.clim.2017.01.010
91. Malmegrim KC, de Azevedo JT, Arruda LC, Abreu JR, Couri CE, de Oliveira GL, et al. Immunological balance is associated with clinical outcome after autologous hematopoietic stem cell transplantation in type 1 diabetes. Front Immunol (2017) 8:167. doi: 10.3389/fimmu.2017.00167
92. Hadrup SR, Schumacher TN. MHC-based detection of antigen-specific CD8+ T cell responses. Cancer Immunol Immunother (2010) 59(9):1425–33. doi: 10.1007/s00262-010-0824-2
93. Newell EW, Klein LO, Yu W, Davis MM. Simultaneous detection of many T-cell specificities using combinatorial tetramer staining. Nat Methods (2009) 6(7):497–9. doi: 10.1038/nmeth.1344
94. Estorninho M, Gibson VB, Kronenberg-Versteeg D, Liu YF, Ni C, Cerosaletti K, et al. A novel approach to tracking antigen-experienced CD4 T cells into functional compartments via tandem deep and shallow TCR clonotyping. J Immunol (2013) 191(11):5430–40. doi: 10.4049/jimmunol.1300622
95. Barcenilla H, Pihl M, Wahlberg J, Ludvigsson J, Casas R. Intralymphatic GAD-alum injection modulates b cell response and induces follicular helper T cells and PD-1+ CD8+ T cells in patients with recent-onset type 1 diabetes. Front Immunol (2021) 12:797172. doi: 10.3389/fimmu.2021.797172
96. Williams JW, Winkels H, Durant CP, Zaitsev K, Ghosheh Y, Ley K. Single cell RNA sequencing in atherosclerosis research. Circ Res (2020) 126(9):1112–26. doi: 10.1161/CIRCRESAHA.119.315940
97. Zheng GX, Terry JM, Belgrader P, Ryvkin P, Bent ZW, Wilson R, et al. Massively parallel digital transcriptional profiling of single cells. Nat Commun (2017) 8:14049. doi: 10.1038/ncomms14049
98. Kolodziejczyk AA, Kim JK, Svensson V, Marioni JC, Teichmann SA. The technology and biology of single-cell RNA sequencing. Mol Cell (2015) 58(4):610–20. doi: 10.1016/j.molcel.2015.04.005
99. Griffiths JA, Scialdone A, Marioni JC. Using single-cell genomics to understand developmental processes and cell fate decisions. Mol Syst Biol (2018) 14(4):e8046. doi: 10.15252/msb.20178046
100. Haque A, Engel J, Teichmann SA, Lonnberg T. A practical guide to single-cell RNA-sequencing for biomedical research and clinical applications. Genome Med (2017) 9(1):75. doi: 10.1186/s13073-017-0467-4
101. Dong S, Hiam-Galvez KJ, Mowery CT, Herold KC, Gitelman SE, Esensten JH, et al. The effect of low-dose IL-2 and treg adoptive cell therapy in patients with type 1 diabetes. JCI Insight (2021) 6(18). doi: 10.1172/jci.insight.147474
102. Zhang JY, Hamey F, Trzupek D, Mickunas M, Lee M, Godfrey L, et al. Low-dose IL-2 reduces IL-21(+) T cell frequency and induces anti-inflammatory gene expression in type 1 diabetes. Nat Commun (2022) 13(1):7324. doi: 10.1038/s41467-022-34162-3
Keywords: immuno-monitor, ELISpot, multimer, immunotherapy, trial, T cell
Citation: Arif S, Domingo-Vila C, Pollock E, Christakou E, Williams E and Tree TIM (2023) Monitoring islet specific immune responses in type 1 diabetes clinical immunotherapy trials. Front. Immunol. 14:1183909. doi: 10.3389/fimmu.2023.1183909
Received: 10 March 2023; Accepted: 02 May 2023;
Published: 22 May 2023.
Edited by:
Arnaud Zaldumbide, Leiden University Medical Center (LUMC), NetherlandsReviewed by:
Karen Cerosaletti, Benaroya Research Institute, United StatesCopyright © 2023 Arif, Domingo-Vila, Pollock, Christakou, Williams and Tree. This is an open-access article distributed under the terms of the Creative Commons Attribution License (CC BY). The use, distribution or reproduction in other forums is permitted, provided the original author(s) and the copyright owner(s) are credited and that the original publication in this journal is cited, in accordance with accepted academic practice. No use, distribution or reproduction is permitted which does not comply with these terms.
*Correspondence: Sefina Arif, c2VmaW5hLmFyaWZAa2NsLmFjLnVr
Disclaimer: All claims expressed in this article are solely those of the authors and do not necessarily represent those of their affiliated organizations, or those of the publisher, the editors and the reviewers. Any product that may be evaluated in this article or claim that may be made by its manufacturer is not guaranteed or endorsed by the publisher.
Research integrity at Frontiers
Learn more about the work of our research integrity team to safeguard the quality of each article we publish.