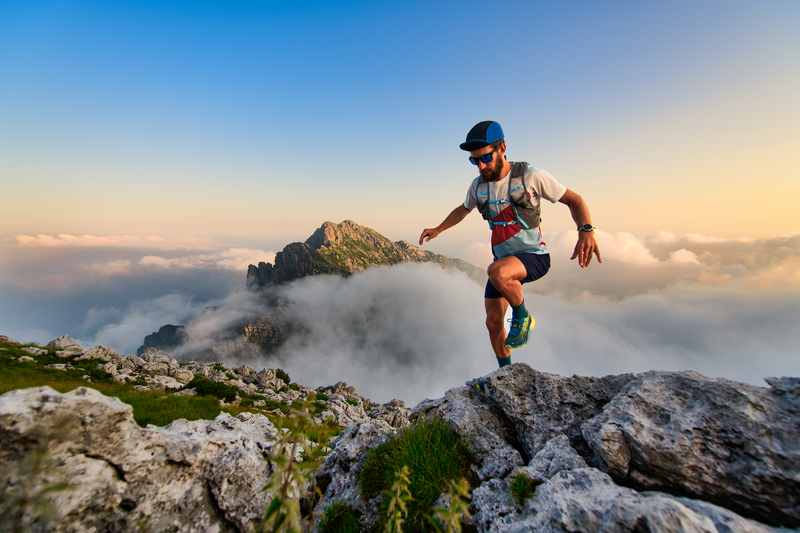
94% of researchers rate our articles as excellent or good
Learn more about the work of our research integrity team to safeguard the quality of each article we publish.
Find out more
REVIEW article
Front. Immunol. , 07 June 2023
Sec. Viral Immunology
Volume 14 - 2023 | https://doi.org/10.3389/fimmu.2023.1182217
This article is part of the Research Topic Pathogen-Induced Immunosenescence: Where do Vaccines Stand? View all 5 articles
Commencing lifelong antiretroviral therapy (ART) immediately following HIV diagnosis (Option B+) has dramatically improved the health of HIV-infected women and their children, with the majority being of HIV-exposed children born uninfected (HEU). This success has led to an increasing population of HIV-infected women receiving ART during pregnancy and children exposed to ART in utero. Nonetheless, a small proportion of children are still infected with HIV (HEI) each year. HEI children suffer from reduced immunocompetence and host-defence, due to CD4+ T lymphocyte depletion, but also dysregulation of other immune cells including CD8+ T lymphocytes, natural killer (NK) cells, macrophages including B lymphocytes. Furthermore, although HEU children are uninfected, altered immune responses are observed and associated with increased vulnerability to infections. The mechanisms underlying immune dysregulation in HEU children remain poorly described. Building on early studies, emerging data suggests that HIV/ART exposure early in life affects cell metabolic function of HEU children. Prenatal HIV/ART exposure has been associated with dysregulation of mitochondria, including impaired DNA polymerase activity. Furthermore, dysregulation of oxidative phosphorylation (OXPHOS) causes a decreased generation of adenosine triphosphate (ATP) and increased production of reactive oxygen species (ROS), resulting in oxidative stress. These altered metabolic processes can affect immune cell viability and immune responses. Recent studies have indicated that immune-metabolic dysregulation may contribute to HIV-associated pathogenesis and clinical observations associated with HIV and ART exposure in HEU/HEI children. Given the critical role metabolic processes in immune cell functioning, immune-metabolic dysregulation in HEU and HEI children may have implications in effective host-defence responses against pathogens, as well as efficacy of standard ART regimens and future novel HIV cure approaches in HEI children. At the same time, targeting metabolic pathways of immune cells may provide safer and novel approaches for HIV cure strategies. Here, we review the current literature investigating immune-metabolic dysregulation in paediatric HIV pathogenesis.
In 2020, 37.7 million people were living with HIV/AIDS (PLWHA), and of these, 1.7 million were children aged from 0 to 14 years. During the same period about 150,000 new infections occurred in children aged 0 to 9 years with up to 46% of the HIV-exposed infected (HEI) children lacking access to antiretroviral therapy (ART) (1). Without ART 50% of the HEI children are expected to succumbed to HIV by the age of 2 years as a result of adverse birth outcomes like prematurity, low birth weight (LBW) and HIV-associated impaired immunity to bacterial and viral infections early in life (2). Over the past decade the successful roll-out of ART for the prevention of mother-to-child transmission (PMTCT) of HIV has significantly reduced vertical transmission (3).
More than 90% of pregnant women globally now have access to PMTCT of HIV interventions (3). As a result, the population of maternally HIV-exposed uninfected (HEU) children is increasing with approximately a global population of 14.8 million HEU children in 2018 (4) and this figure continues to increase. However, some of the children born to HIV-infected women are still vertically infected annually with 160000 new infections recorded in the 0 to 14 year age group in 2020 (5). Dual nucleos(t)ide reverse transcriptase inhibitors (NRTIs) backbone in combination with a non-NRTI (NNRTI) or an integrase strand transfer inhibitor (INSTI) are the currently recommended ART regimens to prevent vertical transmission (6). ART can cross the placenta and detectable in cord blood (7). NRTI’s are known to dysregulate the proper functioning of mitochondria (8, 9), and concerns have been raised regarding the long term impact of in utero ART exposure and in early life after birth.
Despite having significantly improved the quality of life of PLWHA, ART has also been associated with a range of adverse effects both in children and adults, particularly metabolic changes have been reported (9–11). HEU children are exposed to maternal HIV and ART in utero, continuing throughout the breastfeeding period. Both HEU and HEI children present with higher morbidity and mortality rates as a result of altered immune responses when compared to their HIV unexposed uninfected (HUU) peers born to HIV uninfected women (12–14). Furthermore, reduced growth is observed in the early years of life in HEU and HEI children compared to their HUU counterparts (15).
Cell metabolism is critical for the functioning of cells, organs and overall human development. Metabolic dysregulation can affect the transport, utilization or storage of metabolites such as glucose, lipids and amino acid within cells. HIV infection independent of ART can induce changes in glucose, lipid and amino acid metabolism. Even in the presence of ART these observations are not fully corrected (16). Metabolic dysregulation may have clinical implications, including host-defense against pathogens, tissue growth and development, and neurological functioning. Furthermore, as proper metabolic functioning is critical for effective immune responses, hence metabolic dysregulation may have implications for the development of potential future HIV cure approaches for HEI children.
The current approach to improve health of HEI children relies on early initiation of ART (17, 18). This strategy aims to prevent the establishment of large viral reservoirs and maintenance of host immunocompetence. HIV cure approaches target the virus but also rely on a good immune system, therefore an effective host immune responses would be critical for the success of these approaches. Metabolic impaired immune cells may render potential HIV cure strategies less effective (19, 20). At present our knowledge of metabolic dysregulation and consequences of impaired immune function in HEI children is limited. However, the topic has been investigated in more depth in adults. These studies in adults may inform paediatric studies in the development of potential strategies to restore metabolic homeostasis and improve cure approaches. A better understanding of metabolic dysregulation in HEI children may also help to identify pathways that provide targets to ameliorate long-term consequences of growth impairment and tissue health. In light of this, it is crucial that paediatric studies investigating novel HIV cure strategies take into account metabolic functioning of immune cells, including the mitochondria.
Mitochondria are organelles in the cell, which serve a variety of critical functions in eukaryotic cells. The primary function of the mitochondria is energy production in the form of adenosine triphosphate (ATP) mainly through oxidative phosphorylation (OXPHOS)/electron transport chain (ETC) and the citric acid cycle. Mitochondria are furthermore involved in regulating cellular metabolism, cell signalling and maintenance of cell redox state (21, 22). Disruption of mitochondrial functioning can result in lack of energy and increased reactive oxygen species (ROS), with both triggering processes ultimately resulting in cell senescence and death (23). In the context of immune responses during activation, immune cells shift from a resting to an active state. This process requires energy in the form of ATP (24), underscoring the importance of ATP in activating immune responses. Furthermore, ROS are potent activators of proinflammatory signalling pathways. Thus the cell’s metabolism hubs, the mitochondria, is therefore central in the establishment and maintenance of immune responses (25, 26), processes that are compromised in HIV infection.
In HIV infection, both the virus and ART alter mitochondrial functioning which can have consequences for the health of PLWHA (27). HIV can directly induce reprogramming of mitochondrial functioning through its proteins including Tat, gp120, Nef and Vpr. Tat and Vpr thereby affecting the mitochondrial transmembrane potential, resulting in mitochondrial swelling and cell apoptosis (28, 29). Gp 120 furthermore affects mitochondrial fusion and mitochondrial size (30), as well as the upregulation of glycolysis as indicated by a high extracellular acidification rate (31). Furthermore, HIV proteins can also induce production of ROS through Env-mediated autophagy of peroxisomes (32) and Tat-induced DNA damage (29), in the process dysregulating oxidative stress-regulating pathways (33). These processes regulate immune responses by activating inflammatory responses through the Nod-like receptor (NLR) family of pyrin domain containing 3 (NLRP3) inflammasome that mediate antiviral responses (34, 35). Continuous signalling, however, in the context of chronic HIV infection can therefore result in prolonged signalling and inflammation associated HIV immunopathogenesis.
Not long after the introduction of NRTIs their mitochondrial toxicity became apparent (9, 36, 37). Zidovudine, a NRTI, has long been shown to cause metabolic dysregulations. A study by Blanche et al. demonstrated a low mitochondrial complex I (CI) and complex IV (CIV) activity in children exposed to AZT in utero and after birth (9) (Table 1). Since then, NRTIs, NNRTIs and protease inhibitors (PIs) have all been reported to induce mitochondrial dysfunction with more pronounced effects observed of NRTIs. NRTIs were initially thought to affect mitochondrial functioning by acting as substrates to polymerase gamma, thereby disrupting mtDNA synthesis. However, recent studies indicate alternative effects that also alter mitochondrial functioning (8, 57).
Furthermore, NRTIs can act as DNA chain terminators through their incorporation into the mtDNA leading to an aborted replication with a reduced mtDNA copy number per cell (58). Although initial studies have focused on Stavudine and Zidovudine a study by Zhao and colleagues showed that the transcription factor SSBP1 and the mitochondrial DNA helicase were both down-regulated by Tenofovir which may reduce mtDNA (8). Tenofovir is still widely used (6). PIs directly inhibit cell metabolism through decreasing the mitochondrial membrane potential which is pivotal in ATP production, but this effect may vary with cell type (59, 60). Efavirenz, like HIV infection, inhibits CI in the human ETC whilst murine studies have shown that Efavirenz inhibits CIV resulting in decreased ATP production and increased ROS production (61). It has been hypothesized that OXPHOS dysfunction is the mechanism behind lactic acidosis observed among HEI children on ART (62). A recent study reported elevated levels of methionine-sulfone, a result of increased methionine oxidation by ROS, in HEU born to women who initiated ART preconception when compared to those who initiated ART post-conception (44). This is indicative of long-term ART exposure being associated with oxidative stress in HEU.
Several studies have shown a decrease in mitochondrial CI and CIV protein levels in peripheral blood mononuclear cells (PBMCs) of PLWHA on ART, correlating with disease severity as assessed by the CD4/CD8 ratio (62–64). One of these studies showed that the levels of PBMCs CI and CIV protein levels were inversely related to plasma inflammatory markers (monocyte chemotactic protein-1, myeloperoxidase, serum amyloid A, serum amyloid P, soluble adhesion molecules) and inflammatory intermediate monocyte frequencies (64, 65). In return inflammatory molecules can further enhance mitochondrial dysfunction, increasing ROS production thereby driving enhanced dysregulation and inflammation. In sum, HIV and ART can alter mitochondrial functioning through different pathways of dysregulation of the cell metabolism and downstream immune responses.
Glucose is the primary source of energy in mammalian cells and is crucial in cell metabolism as it is a biosynthetic precursor which feeds into glycolysis and indirectly into the pentose phosphate pathway, Krebs cycle and OXPHOS (66, 67). These pathways generate ATP, which transfers energy by releasing a phosphate group (68). In immune cells, processes such as cell movements and effector functions all require energy in the form of ATP (69). A variety of infections such as HIV can alter transporters and enzymes involved in glucose metabolism which are known to affect the functioning of immune cells (19, 20).
HIV has been reported to interfere with energy synthesis pathways, causing an increased expression of glucose transporters. Glucose transporter-1 (GLUT-1), glucose transporter-3 (GLUT-3) which facilitates the transport of glucose across the plasma membrane, including glucose transporter-4 (GLUT-4), glucose transporter-6 (GLUT-6) and hexokinase 1 (HK1) are up-regulated in adult HIV-infected CD4+ T lymphocytes (19, 20). A study by Mason and colleagues demonstrated that GLUT-1 expression positively correlated with mitochondrial mass and mitochondrial membrane potential (70). Another study in adult PLWHA demonstrated that HIV infection caused an increase in the uptake of a fluorescent glucose analogue, 2NBDG, by infected monocytes (71). Remarkably, upregulated glucose metabolism has been associated with CD4+ T lymphocytes apoptosis although it is unknown whether these changes may be involved in the decrease of CD4+ lymphocytes in HIV infection (72, 73).
In addition, the changes in glucose transport have been associated with increased levels tumour necrosis factor (TNF) production in HIV-infected CD4+ T lymphocytes (73). Long-term viral replication and dysregulation of glucose levels have been associated with inflammation which possibly contribute to chronic immune activation in adult HIV patients. Further studies are warranted to investigate the regulation of these glucose transporters in HEU and HEI children. However, such studies in the context of obesity have shown that long term increases of glucose levels are associated with chronic inflammation and reduced antigen specific adaptive responses (74, 75).
Building on the research investigating mitochondrial toxicity of ART, Tenofovir-mediated down-regulation of the mitochondrial chaperone TRAP1 may be involved in the reprogramming of glucose metabolism with increased glycolysis and glycogen synthesis (8). ART has also been shown to induce insulin resistance in PLWHA providing the underlying conditions for the development of type 2 diabetes, which is common among this population (76). Insulin resistance, measured using the Homeostatic Model Assessment of Insulin Resistance (HOMA-IR), is also common among HIV-infected children on ART. A recent study observed insulin resistance in 20% of the HEI children with clinical end stage of the disease and long-time on ART (38, 77) (Table 1). Changes in glucose transport and metabolism contribute to impaired insulin secretion by beta cells and altered glycogen synthesis in hepatocytes which significantly increase the risk of developing type 2 diabetes among PLWHA on ART (78). There is urgent need for comprehensive research on the glucose metabolic dysregulation in HEU children with in utero and breast milk exposure to maternal HIV/ART versus HEI children with additional direct exposure to HIV and ART as these two groups may have distinct characteristics which inform on their specific health needs.
Next to glucose, lipid metabolism has been shown to be critically altered in PLWHA (79, 80). Lipids are organic compounds which play vital roles in the human body. These include storage of energy, as structural components in plasma membranes, as biomarkers, as hormones and in cell signalling in mammalian cells (81). Lipids can also be used as an alternative source of energy by the cells. The human body utilizes different types of lipids. Triglycerides are used to store energy in lipids and are increased in obese individuals (82). Studies focusing on obesity have shown that increased levels of triglycerides alter immune responses associated low level immune activation but reduced specific immune responses to pathogens or vaccines (74, 75).
HIV infection, in the absence of ART, has been shown to induce alterations in serum lipid profiles (83). HIV-associated dyslipidemia is characterized by high total cholesterol, triglyceride levels and low high-density lipoprotein cholesterol. HIV infection furthermore affects reverse cholesterol transport causing a decrease in cholesterol levels and an increase in triglycerides (84). Furthermore, cytokines can have a direct effect on lipid metabolism altering lipid profiles through modifications of lipid processing and transport in chronic inflammatory states (45, 78). HIV infection in macrophages causes an increase in lipid uptake as indicated by fluorescent lipid dye; BODIPY intensity, resulting in lipid accumulation and increased mitochondrial size, but, decreased functioning (85). Abnormal lipoprotein profiles and inflammatory markers have been shown in HIV-infected children and adolescents (86–88). These metabolic changes and chronic inflammation may have clinical implications for cardiovascular diseases (CVD) as they mature into adulthood.
The dysregulation of mitochondria also has repercussions for lipid metabolism. The excess ROS produced from mitochondrial dysfunction react with polyunsaturated fatty acids during lipid peroxidation, and levels are increased in plasma of children born to HIV-infected women compared to those born to healthy women (45). The products of lipid peroxidation, malondialdehyde (MDA) and 4-hydroxynonenal, furthermore can alter the integrity of cell membranes (89). Mitochondrial membrane lipid peroxidation destabilizes the membrane structure and changes integrity, resulting in the mitochondrial permeability transition pore formation, loss of the mitochondrial transmembrane potential, release of cytochrome c and eventually cell death (63, 90). Thus, mitochondrial dysregulation via altered lipids may further aggravate mitochondrial dysfunction, with consequences for energy production and inflammation.
PIs have long been associated with dyslipidemia in PLWHA (91). Unresolved dyslipidemia increases the risk of developing CVDs (92, 93). Elevated levels of triglycerides are observed with PLWHA on PIs (93). Exposure to ART particularly Ritonavir-boosted Lopinavir has been associated with hypertriglyceridemia, previously demonstrated in adults (94), and decreased phospholipids in children (45). Furthermore, Efavirenz, has been shown to activate AMP-activated protein kinase, subsequently promoting lipid accumulation in the cytoplasm associated with the increase in mitochondrial mass (95). In utero ART-exposed HEU-children have an altered lipid profile compared to HUU children (45). They have increased plasma concentrations of triglycerides, saturated lysophospholipids and decreased levels of unsaturated lysophospholipids compared to HUU children (Table 1). Another study reported an altered sphingolipid-ceramide ratio in HEU with long-term ART exposure than in those with medium, short or no exposure (44). This is indicative of an altered lipid metabolism with increase in the duration of in utero ART exposure. Phospholipids are important components of the cell membranes and have anti-inflammatory properties (96). The increased levels of triglycerides and subsequent decreased levels of phospholipids are important triggers for the unfolded protein response (UPR) of the endoplasmic reticulum and the pro-inflammatory milieu in early life ART-exposed HEU-children (45).
Amino acids are organic molecules, which act as building blocks for protein synthesis, e.g. enzymes. Metabolism of proteins to generate energy only occurs in situations of low carbohydrate or lipid intake such as during starvation or in situations of high energy demand (97, 98). Furthermore, glutamate is critical for the functioning of activated T lymphocytes as it provides a fuel source which sustains mitochondrial OXPHOS (99). In NK cells amino acids play less of a role as energy substrates. However, amino acids do regulate NK cell functioning. Glutamine-regulated expression of the transcription factor cMyc is critical in controlling NK cell proliferation and effector functions (100, 101).
Recent studies have reported an association between CD4+ T lymphocyte counts in PLWHA and serum glutamine indicating that this amino acid is utilized by cells as an energy source during HIV infection (72, 102). In addition, macrophages during HIV infection utilize glutamine as an alternative source of energy (19). In one study of HIV-infected macrophages showed plasticity in fuel usage by switching the source of energy when either glucose, fatty acid or glutamine was inhibited (19). Inhibition of ASCT2, a glutamine transporter, resulted in a decrease in the number of surviving HIV-infected macrophages indicating glutamine as the primary energy source in infected macrophages, hence absence of glutamine may restrict HIV infection (19).
Other amino acids are involved in the functioning of mitochondria. Cysteine and methionine residues on amino acids are easily oxidized by ROS to form disulphides (103, 104). Mitogen activated protein kinase (MAPK), a signalling complex which regulates proliferation and aerobic glycolysis, is rich in cysteine residues and formation of disulphides in the protein structure results in a dysregulated cell signalling function (104). The metabolic functioning of the mitochondria is reflected by the levels of intermediary metabolites such as acyl carnitines which are formed during fatty acid oxidation. Studies in ART-exposed HEU-children demonstrated an altered plasma metabolite profile especially acyl-carnitine profile and branched amino acids compared to HUU children (45, 46) (Table 1). One of the studies showed that postnatal exposure to Zidovudine had a greater impact on the metabolic profiles than Nevirapine (46). HIV/ART exposure has also been associated with an abnormal phenylalanine metabolism and increased phenylalanine in the circulation (57). Phenylalanine can cross the placenta and even small increases in plasma phenylalanine levels have been reported to be associated with decreased head circumference and birth length (105). Additionally, HIV-mediated changes in tryptophan metabolism have been shown to result in suppressed serotonin production and increased kynurenine production through the upregulation of indole amine 2,3-dioxygenase (IDO) expression that degrades the tryptophan (57). A study by Babu and colleagues demonstrated that an increase in kynurenine/tryptophan ratio and lower levels of serotonin in more than half of their cohort of PLWHA and both were associated with neurocognitive impairment and HIV-associated neurological disorders (106). Serotonin is essential in neurons activation and altered levels may result in neurocognitive impairment (107). Such alterations in pregnancy may affect the neurological development in the foetus.
Taken together, HIV and ART can in many ways impact the cell metabolism with consequences for immunity against pathogens, chronic inflammation and dysregulation of energy metabolism to sustain development during infancy. The HUU children have clearly distinct immunometabolism than in HEI and HEU children as illustrated in Figure 1.
Figure 1 Immunometabolism distinguishing HEI, HEU and HUU children. HEI and HEU children share many similarities in altered cell metabolism as compared to the HUU children.
Several studies have linked the metabolic dysregulations in HEI children to clinical implications such as a higher risk of CVD in HEI when compared to uninfected peers (108–110). Altered sphingolipid-ceramide profiles as reported in HEU children with long-term ART exposure (44) may predispose them to age-related neurological disease (111, 112). The latter is a CVD biomarker hence like HEI, HEU with long-term ART exposure may be at a higher risk of CVD compared to HUU children (113). However, most studies in HEI and HEU cohorts describe an increased mortality and morbidity and/or immune cell phenotypes but not linking them to metabolic assays (114, 115). This has left any association between dysregulated metabolism and clinical implication poorly described creating a need for such studies (116, 117).
Below we describe the current understanding of altered immunity in HEI and HEU children and how metabolic dysregulation can contribute to these observations.
At birth children initially rely on their innate immune responses and passive acquired immunity from the mother thanks to the transfer of maternal antibodies from the mother to the foetus during pregnancy. During childhood immune maturation in response natural infections and vaccinations results in the acquisition of effective adaptive responses. In HEU-children antibody mediated passive as well as vaccine induced immunity is affected with HEU children showing altered vaccine-specific responses compared to HUU as previously reviewed (118). Although data has been conflicting overall HEU and HEI have lower antibody responses to vaccines shown by the low titres of anti-tetanus, measles, diphtheria, pertussis and hepatitis B surface antigen antibodies as compared to HUU (118–120), whereas conflicting reports have been published for BCG, pneumococcus and pertussis vaccines (121, 122). In HEI children the low CD4+ lymphocyte counts, further critically impairing the induction and maintenance of immunological memory to vaccines and infections. This indicates HIV-mediated poor quality and quantity of humoral responses among this group (118).
Innate immunity by NK cells and macrophages plays a crucial role in responses to infections early in life before adaptive immune responses have matured. Exposure to viruses such as HIV is associated with NK cell dysfunction (123). HIV infection in children is associated with lower NK cell numbers and altered phenotype and effector functions of NK cells in HEI children (124, 125). Exposure to HIV is furthermore associated with reduced cytolytic potential of NK cells in HEI and HEU children as indicated by the lower expression of perforin compared to HUU children (124, 125).
The expression of CD69, which is upregulated upon cell activation, is increased on NK cells in HEI compared to HUU children. This finding suggest that NK cells undergo increased activation in HEI compared to HUU children in the early months of life (125). At the same time, NK cells of HEI children express higher levels of NKG2A and KIRs inhibitory markers (126), which may inhibit NK cell activation against virus- infected cells such as HIV-infected cells. Taken together, NK cells have an altered phenotype associated with reduced cytotoxic characteristics in HEU and HEI-children, however the question remains whether metabolic changes in NK cells may underlie these observations (127).
Macrophages and dendritic cells (DC) are innate immune cells that act as antigen presenting cells (APC) and shape the adaptive immune system during an infection. Macrophages express chemokines receptors, CCR5 and CXCR4 that are co-receptors in HIV infection. HIV infection has been reported to induce changes in macrophage polarization state depending on the stage of the infection (128). Due to their plasticity during HIV infection, macrophages can polarize towards different phenotypes with increased or decreased antiviral capacity (128, 129). With regards to DCs HEU-children at birth have a significantly higher percentages of myeloid dendritic cells (mDCs) than HUU-children (130), however, the frequencies of both mDCs and plasmacytoid dendritic cells (pDCs) remain similar throughout the first year of life (130, 131). Upon stimulation with lipopolysaccharide (LPS) mDCs from HEU-children showed increased upregulation of CD80, CD86 and programmed cell death ligand 1 (PD-L1) while the mDCs from HUU children mostly upregulated PD-L1. Furthermore, mDCs of HEU-children at birth showed a higher responsiveness to LPS and polysaccharides from Atractylodes macrocephala (PAM) stimulation by secreting TNF, IL-6 and IL-12 compared to HUU children (130). Beyond 6 weeks of life mDCs responses to bacterial ligands became comparable among the two groups. In sum, innate immune cells are affected in HEU-children especially shortly after birth.
Several studies have shown that HIV-induces a switch of source of energy in innate immune cells such as macrophages (85, 132). Glucose and fatty acid utilization are reduced in adult HIV-infected macrophages while glutamine uptake is increased (19, 133). There is paucity of data regarding the implications of HIV/ART-mediated metabolic dysregulation in macrophages and DCs on the immune responses in children. Some adults’ studies have shown that HIV infection mediates IFN responses and toll-like receptor (TLR) engagement resulting in intracellular IDO expression in macrophages and DCs. This increases the levels of circulating immunomodulatory tryptophan catabolites like kynurenine and quinolinic acid which have been associated with higher production of inflammatory cytokines and increased immune activation (134). In addition, the higher glycolytic flux in infected macrophages leads to increased production of pro-inflammatory cytokines such as TNF and IL-6 further fueling chronic inflammation in PLWHA (132). These studies were in adults and the impact of such metabolic dysregulations in children are yet to be described.
Furthermore, HIV induces mitochondrial enlargement in infected macrophages. A possible cause of mitochondrial enlargement in HIV infected cells is lipid accumulation in the mitochondrial matrix (85). The clinical implications of this is not clear. In addition to enlargement, mitochondria of infected macrophages have significantly lower basal oxygen consumption rate and response to oligomycin, an ATP synthase inhibitor (85, 135). This indicates HIV-mediated mitochondrial dysfunction in infected macrophages that may persist in HEI children whereas the effects of maternal HIV may only be affecting macrophages and DCs in HEU children for shorter periods.
T lymphocytes serve as effector cells of adaptive immune responses. CD4+ T lymphocytes are susceptible to HIV infection due to their expression of CD4 and CXCR4 and CCR5 (co)-receptors. Next to the challenge of HIV associated cell death of CD4+ T lymphocytes in HEI children, chronic immune activation further affects cell populations in children and in adults (73, 136). Metabolic changes in T lymphocytes have been described in PLWHA, e.g. the glucose transporter GLUT1 is upregulated. An inverse association between the number of CD4+GLUT1+ T lymphocytes and CD4+ T lymphocyte counts has been reported (68, 73) indicating an association between cell death and glucose metabolism in CD4+ T lymphocytes.
In children, HIV infection is associated with an approximately 1.5 times higher frequencies of programmed cell death 1 (PD1)+ memory CD4 T lymphocytes compared to uninfected children (137, 138). This dysregulation was shown to be partially reversed by ART as the frequencies decreased upon ART initiation however, remaining higher than in uninfected children (138). High frequencies of programmed cell death protein 1 (PD1)+ memory CD4+ T lymphocytes predicts lower effector capacity. CD8+ T lymphocytes are important in the control of HIV infection (139–141). However, prolonged activation of CD8+ T lymphocytes results in cell exhaustion. Inhibition of PD1 on CD8+ T lymphocytes reverses cell exhaustion and restores anti-viral capacity in vitro, at one point PD1 inhibition has been suggested as a potential target in HIV cure, however adverse effects are not negligible therefore making this currently a limited option for cure (142).
Activation of CD4+ T lymphocytes in response to antigen recognition presented by macrophages or DCs during HIV infection enhances glycolysis which in turn facilitates HIV replication and establishment of a large reservoir (72). Unlike in CD4+T lymphocytes, increased glycolytic activity during activation and differentiation in CD8+ T lymphocytes is associated with viral suppression (141). GLUT1 expression is correlated with mitochondrial density and mitochondrial membrane potential (MMP) in CD4+T lymphocytes. Furthermore, the MMP has been positively correlated with ROS production in CD4+ and CD8+ T lymphocytes (70). If let unresolved, ROS can damage the cells reacting to cellular metabolites or DNA as discussed earlier (70).
In utero HIV/ART exposure also has an impact on CD4+ T lymphocytes and CD8+ T lymphocytes in HEU-children (143). HEU-children have decreased CD4+ T lymphocyte counts but increased CD8+ T lymphocyte counts (115, 144, 145) and in one longitudinal study has observed altered CD8+ count that persisted until 8 years of age (144). Furthermore, several studies have reported consistent findings of increased activated CD8+ T lymphocyte and memory CD4+CD45RO+ T lymphocytes among HEU-children compared to HUU-children (143, 146). Although CD4+ T lymphocytes in HEU-children have an activated phenotype (CD4+ HLA- DR+ CD38+), IL-2 production was reduced compared to HUU-children (146). The T lymphocytes phenotype in HEU-children reflects to a certain extend the increased activation. Studies on the metabolic dysregulations in T lymphocytes of HEU-children are warranted to understand whether metabolic dysregulation may further underlie these observations.
As PMTCT strategies are not reaching all women in time, paediatric HIV infection still occurs. Therefore, there is a need for HIV cure approaches in children. The success of an HIV cure depends on the eradication of viral reservoir in latent infected cells. Early ART initiation was one of the first strategies to be tested for a functional cure among children (147–149). The early ART initiation approach aims to limit the size of the viral reservoir.
In efforts to cure HIV, the “shock and kill” approach has been suggested where latent infected cells are reactivated and next are recognised and eliminated by immune cells. Another HIV cure approach is the use of therapeutic vaccination which aims to enhance HIV-specific T lymphocyte responses (149–151). Finally, antibody based approaches have been suggested (152, 153). All the above cure approaches depend on a functional immune system to finally eliminate infected cells. The “surge and purge” approach which combines early ART, passive antibody administration and immune stimulation has been hypothesized to affect reservoir establishment (154). This approach and the “block and lock” may be successful in children with small viral reservoirs by blocking HIV production. In children with large HIV reservoirs it has been suggested that a combination of reversal agent, to reactivate viral expression, and clearance (kick and kill) of the infected cells by the immune system may be successful (155, 156). Considering the metabolic dysregulation of immune cells in HIV infection and upon ART exposure, the immune system of HEI-children is moderately to severely impaired. This then acts as a barrier to the eradication of the virus by the different HIV cure approaches (149).
The growing body of evidence of HIV and/or ART-mediated metabolic dysregulation has formed the foundation for the possibility of cellular metabolism as a potential target for complimentary approaches for HIV cure strategies (Figure 2) (141, 157). As discussed earlier HIV exposure induces a decrease in the levels of acyl carnitines. Several studies have shown the potential benefits of carnitines supplements as therapy for HIV/ART-induced metabolic dysregulations and mitochondrial dysfunction (158–161). Use of carnitine among PLWHA has been shown to improve symptoms of lactic acidosis, reduce serum triglyceride levels and delay CD4+ T lymphocyte apoptosis in HIV infected adults (159–162). Although these studies were in adult populations, carnitine supplementation may also be beneficial to the paediatric populations.
Figure 2 Targets in the cell that could represent a potential strategy to improve immunity for cure in HIV infection. Targeting immunometabolism may affect HIV pathogenesis by inhibiting specific metabolic pathways which are altered by the virus, suppressing inflammation and enhancing immune responses to infection. Targeting glucose and glutamine metabolism can block viral replication. Inhibition of mTOR, which is upregulated in HIV infection, reduce the mTOR-mediated increase in glucose uptake. Suboptimal glycolysis produces less pyruvate which in return controls ROS overproduction and reduces inflammation in HIV infection. The use of therapeutic approaches which improve mitochondrial function may rejuvenate the cells and enhance immune cell mediated antiviral capacity as seen in long-lived memory T lymphocytes. The above strategies may contribute to decreasing the number of infected cells, enhance immune responses to target remaining infected cells and prevention of chronic inflammation. ASCT, amino acid transporter; GLUT1, glucose transporter 1; TCA cycle, tricarboxylic acid cycle; mTOR, mechanistic target of rapamycin. Potential targets which block early steps in HIV replication.
HIV infection has been associated with increased glycolysis and glutaminolysis in infected lymphocytes (20, 141). Because activated T lymphocytes are largely dependent on glycolysis to fulfil their energy requirements for proliferation and function, these cells are particularly sensitive to a dysregulated metabolism. The plasticity of immune cells such as T and macrophages in their varied energy source during HIV infection has prompted studies on the effects of glutamine/glutamate inhibitors on HIV replication and reservoir size (85, 163).
Several in vitro studies have demonstrated the effect of glycolysis and glutaminolysis inhibitors on immune cells functions, HIV infection, replication and reservoir size. Metabolic inhibitors of the enzymes involved in energy metabolism have been demonstrated to reduce HIV infection by inhibiting glycolysis and glutaminolysis (164). Reduced HIV infection is observed in CD4+ T lymphocytes when glycolysis and glutaminolysis are inhibited. Glutamine deprivation resulted in a higher reduction in HIV infected cells compared to glucose deprivation (163). Culturing HIV-infected CD4+ T lymphocytes with poor glycolysis substrates, such as galactose or 2-deoxyglucose, decreases both the cell proliferation and the release of virions in the culture supernatant when compared to culturing in the presence of glucose (72, 165). This indicates a reduced viral latency and replication respectively in a glycolysis-limited environment which is a vulnerability that can be targeted in novel metabolic inhibitors for HIV cure (Figure 2). However, given the important role of glycolysis in T lymphocyte functioning, the extent of glycolysis inhibition may decrease T lymphocyte efficacy in eliminating the reservoir and the host defences against other pathogens.
The mammalian (mechanistic) target of rapamycin (mTOR) regulates growth in response to nutrients levels and cellular stress can lead to an increased AMP/ATP ratio, which in turn promotes the phosphorylation and activation of adenosine monophosphate-(AMP)-activated protein kinase (AMPK) (166). Activation of AMPK promotes glucose uptake, glycolysis, fatty acid uptake and fatty acid oxidation and at the same time inhibiting anabolism (gluconeogenesis, synthesis of glycogen, fatty acids and triglycerides) to maintain energy (167). Thus, another potential novel strategy is to optimize immune activity is the treatment with metformin, a drug prescribed to diabetics (168). It is an indirect inhibitor of mTOR which functions by targeting the mitochondrial respiratory chain complex 1 resulting in decreased ATP to ADP ratio (169, 170). This in turn activates AMPK to phosphorylate the raptor subunit on mTOR and alters glycolysis in T lymphocytes (171, 172). The drug has been shown to normalize mitochondrial dysfunction in CD4+ T lymphocytes (168).
The mechanism of action of Metformin in non-diabetic PLWHA is based on this drug’s ability to normalise mitochondrial function through improving ATP production and providing an anti-inflammatory environment, in the process restoring the effective functioning of immune cells (172, 173). Furthermore, the viral reservoir and replication inhibition through Metformin-mediated glycolysis inhibition may play a role in the “block and lock” HIV cure strategy (141). Combining metformin and ART may assist in limiting viral reservoirs. In a recent LILAC pilot study, treatment with metformin among PLWHA showed a preferential activation or phosphorylation of Th17-polarized CCR6+ CD4+ T lymphocytes (171). This subset of CD4+ T lymphocytes is highly targeted by the HIV virus and implicated in the blood and colon viral reservoirs among PLWHA. Interestingly, in the same study treatment with Metformin reduced inflammation and the HIV RNA/HIV DNA ratios indicating a reduced viral transcription (171). It is yet to be explored if there is a synergy between metformin and latency reversal agents, an approach which could be used in combination with ART for viral reservoir eradication.
Early life exposures to HIV and ART pose a risk on the health outcomes of children born to women living with HIV when compared to those born to healthy women. The impact of these factors on immune cell metabolism and the development of the immune system remain poorly described. There is need for long-term follow-up of these children to monitor clinical implications of metabolic dysregulations. A deeper understanding on how optimal cell metabolism is a central in the functioning of the immune system is critical for exploring alternative novel and safer immunotherapies for HIV cure.
HM wrote the first draft. All authors were involved in manuscript revisions and approved the final draft.
The authors declare that the research was conducted in the absence of any commercial or financial relationships that could be construed as a potential conflict of interest.
All claims expressed in this article are solely those of the authors and do not necessarily represent those of their affiliated organizations, or those of the publisher, the editors and the reviewers. Any product that may be evaluated in this article, or claim that may be made by its manufacturer, is not guaranteed or endorsed by the publisher.
1. Global HIV & AIDS statistics. Fact sheet (2022). Available at: https://www.unaids.org/en/resources/fact-sheet.
2. Newell ML, Coovadia H, Cortina-Borja M, Rollins N, Gaillard P, Dabis F, et al. Mortality of infected and uninfected infants born to HIV-infected mothers in Africa: a pooled analysis. Lancet Lond Engl (2004) 364(9441):1236–43. doi: 10.1016/S0140-6736(04)17140-7
4. UNAIDS. Start free stay free AIDS free 2019 report (2019). Available at: https://www.unaids.org/en/resources/documents/2019/20190722_UNAIDS_SFSFAF_2019.
5. AIDSinfo. UNAIDS (2023). Available at: https://aidsinfo.unaids.org/.
6. World Health Organization. Consolidated guidelines on the use of antiretroviral drugs for treating and preventing HIV infection: recommendations for a public health approach. 2nd ed. Geneva: World Health Organization (2016). Available at: https://apps.who.int/iris/handle/10665/208825.
7. Else LJ, Taylor S, Back DJ, Khoo SH. Pharmacokinetics of antiretroviral drugs in anatomical sanctuary sites: the fetal compartment (Placenta and amniotic fluid). Antivir Ther (2011) 16(8):1139–47. doi: 10.3851/IMP1918
8. Zhao X, Sun K, Lan Z, Wenxin S, Cheng L, Chi W, et al. Tenofovir and adefovir down-regulate mitochondrial chaperone TRAP1 and succinate dehydrogenase subunit b to metabolically reprogram glucose metabolism and induce nephrotoxicity. Sci Rep (2017) 7:46344. doi: 10.1038/srep46344
9. Blanche S, Tardieu M, Rustin P, Slama A, Barret B, Firtion G, et al. Persistent mitochondrial dysfunction and perinatal exposure to antiretroviral nucleoside analogues. Lancet (1999) 354(9184):1084–9. doi: 10.1016/S0140-6736(99)07219-0
10. Brinkman K, Smeitink JA, Romijn JA, Reiss P. Mitochondrial toxicity induced by nucleoside-analogue reverse-transcriptase inhibitors is a key factor in the pathogenesis of antiretroviral-therapy-related lipodystrophy. Lancet (1999) 354(9184):1112–5. doi: 10.1016/S0140-6736(99)06102-4
11. van der Valk M, Casula M, Weverlingz GJ, van Kuijk K, van Eck-Smit B, Hulsebosch HJ, et al. Prevalence of lipoatrophy and mitochondrial DNA content of blood and subcutaneous fat in HIV-1-infected patients randomly allocated to zidovudine- or stavudine-based therapy. Antivir Ther (2004) 9(3):385–93. doi: 10.1177/135965350400900317
12. Dauby N, Goetghebuer T, Kollmann TR, Levy J, Marchant A. Uninfected but not unaffected: chronic maternal infections during pregnancy, fetal immunity, and susceptibility to postnatal infections. Lancet Infect Dis (2012) 12(4):330–40. doi: 10.1016/S1473-3099(11)70341-3
13. Slogrove AL, Goetghebuer T, Cotton MF, Singer J, Bettinger JA. Pattern of infectious morbidity in HIV-exposed uninfected infants and children. Front Immunol (2016) 7:164. doi: 10.3389/fimmu.2016.00164
14. Afran L, Garcia Knight M, Nduati E, Urban BC, Heyderman RS, Rowland-Jones SL. HIV-Exposed uninfected children: a growing population with a vulnerable immune system? Clin Exp Immunol (2014) 176(1):11–22. doi: 10.1111/cei.12251
15. Isanaka S, Duggan C, Fawzi WW. Patterns of postnatal growth in HIV-infected and HIV-exposed children. Nutr Rev (2009) 67(6):343–59. doi: 10.1111/j.1753-4887.2009.00207.x
16. Deme P, Rubin LH, Yu D, Xu Y, Nakigozi G, Nakasujja N, et al. Immunometabolic reprogramming in response to HIV infection is not fully normalized by suppressive antiretroviral therapy. Viruses (2022) 14(6):1313. doi: 10.3390/v14061313
17. Kuhn L, Strehlau R, Shiau S, Patel F, Shen Y, Technau KG, et al. Early antiretroviral treatment of infants to attain HIV remission. eClinicalMedicine (2020). Available at: https://www.thelancet.com/journals/eclinm/article/PIIS2589-5370(19)30246-9/fulltext.
18. Payne H, Chan MK, Watters SA, Otwombe K, Hsiao NY, Babiker A, et al. Early ART-initiation and longer ART duration reduces HIV-1 proviral DNA levels in children from the CHER trial. AIDS Res Ther (2021) 18(1):63. doi: 10.1186/s12981-021-00389-1
19. Hollenbaugh JA, Munger J, Kim B. Metabolite profiles of human immunodeficiency virus infected CD4+ T cells and macrophages using LC–MS/MS analysis. Virology (2011) 415(2):153–9. doi: 10.1016/j.virol.2011.04.007
20. Kavanagh Williamson M, Coombes N, Juszczak F, Athanasopoulos M, Khan MB, Eykyn TR, et al. Upregulation of glucose uptake and hexokinase activity of primary human CD4+ T cells in response to infection with HIV-1. Viruses (2018) 10(3):114. doi: 10.3390/v10030114
21. Murphy E, Ardehali H, Balaban RS, DiLisa F, Dorn GW, Kitsis RN, et al. Mitochondrial function, biology, and role in disease. Circ Res (2016) 118(12):1960–91. doi: 10.1161/RES.0000000000000104
22. Kühlbrandt W. Structure and function of mitochondrial membrane protein complexes. BMC Biol (2015) 13(1):89. doi: 10.1186/s12915-015-0201-x
23. Quinzii CM, López LC, Gilkerson RW, Dorado B, Coku J, Naini AB, et al. Reactive oxygen species, oxidative stress, and cell death correlate with level of CoQ10 deficiency. FASEB J (2010) 24(10):3733–43. doi: 10.1096/fj.09-152728
24. Angajala A, Lim S, Phillips JB, Kim JH, Yates C, You Z, et al. Diverse roles of mitochondria in immune responses: novel insights into immuno-metabolism. Front Immunol (2018). 9 doi: 10.3389/fimmu.2018.01605
25. Breda CN de S, Davanzo GG, Basso PJ, Saraiva Câmara NO, Moraes-Vieira PMM. Mitochondria as central hub of the immune system. Redox Biol (2019) 26:101255. doi: 10.1016/j.redox.2019.101255
26. Weinberg SE, Sena LA, Chandel NS. Mitochondria in the regulation of innate and adaptive immunity. Immunity (2015) 42(3):406–17. doi: 10.1016/j.immuni.2015.02.002
27. Kallianpur KJ, Walker M, Gerschenson M, Shikuma CM, Gangcuangco LMA, Kohorn L, et al. Systemic mitochondrial oxidative phosphorylation protein levels correlate with neuroimaging measures in chronically HIV-infected individuals. AIDS Res Hum Retroviruses (2020) 36(1):83–91. doi: 10.1089/aid.2019.0240
28. Wang Y, Santerre M, Tempera I, Martin K, Mukerjee R, Sawaya BE. HIV-1 vpr disrupts mitochondria axonal transport and accelerates neuronal aging. Neuropharmacology (2017) 117:364–75. doi: 10.1016/j.neuropharm.2017.02.008
29. El-Amine R, Germini D, Zakharova VV, Tsfasman T, Sheval EV, Louzada RAN, et al. HIV-1 tat protein induces DNA damage in human peripheral blood b-lymphocytes via mitochondrial ROS production. Redox Biol (2018) 15:97–108. doi: 10.1016/j.redox.2017.11.024
30. Avdoshina V, Fields JA, Castellano P, Dedoni S, Palchik G, Trejo M, et al. The HIV protein gp120 alters mitochondrial dynamics in neurons. Neurotox Res (2016) 29(4):583–93. doi: 10.1007/s12640-016-9608-6
31. Valentín-Guillama G, López S, Kucheryavykh YV, Chorna NE, Pérez J, Ortiz-Rivera J, et al. HIV-1 envelope protein gp120 promotes proliferation and the activation of glycolysis in glioma cell. Cancers (2018) 10(9):301. doi: 10.3390/cancers10090301
32. Daussy CF, Galais M, Pradel B, Robert-Hebmann V, Sagnier S, Pattingre S, et al. HIV-1 env induces pexophagy and an oxidative stress leading to uninfected CD4+ T cell death. Autophagy (2021) 17(9):2465–74. doi: 10.1080/15548627.2020.1831814
33. Rozzi SJ, Borelli G, Ryan K, Steiner JP, Reglodi D, Mocchetti I, et al. PACAP27 is protective against tat-induced neurotoxicity. J Mol Neurosci (2014) 54(3):485–93. doi: 10.1007/s12031-014-0273-z
34. Billingham LK, Stoolman JS, Vasan K, Rodriguez AE, Poor TA, Szibor M, et al. Mitochondrial electron transport chain is necessary for NLRP3 inflammasome activation. Nat Immunol (2022) 23(5):692–704. doi: 10.1038/s41590-022-01185-3
35. Latz E, Xiao TS, Stutz A. Activation and regulation of the inflammasomes. Nat Rev Immunol (2013) 13(6):397–411. doi: 10.1038/nri3452
37. Hamilton JD, Hartigan PM, Simberkoff MS, Day PL, Diamond GR, Dickinson GM, et al. A controlled trial of early versus late treatment with zidovudine in symptomatic human immunodeficiency virus infection. N Engl J Med (1992) 326(7):437–43. doi: 10.1056/NEJM199202133260703
38. Dirajlal-Fargo S, Shan L, Sattar A, Bowman E, Gabriel J, Kulkarni M, et al. Insulin resistance and intestinal integrity in children with and without HIV infection in Uganda. HIV Med (2020) 21(2):119–27. doi: 10.1111/hiv.12808
39. Geffner ME, Patel K, Miller TL, Hazra R, Silio M, Van Dyke RB, et al. Factors associated with insulin resistance among children and adolescents perinatally infected with HIV-1 in the pediatric HIV/AIDS cohort study. Horm Res Paediatr (2011) 76(6):386–91. doi: 10.1159/000332957
40. Bengtson AM, Pellowski J, McGarvey S, McGinty R, Botha M, Burd T, et al. In-utero HIV exposure and cardiometabolic health among children 5–8 years: findings from a prospective birth cohort in south Africa. AIDS (2023) 37(1):173. doi: 10.1097/QAD.0000000000003412
41. Davies C, Vaida F, Otwombe K, Cotton MF, Browne S, Innes S. Longitudinal comparison of insulin resistance and dyslipidemia in children with and without perinatal HIV infection in south Africa. AIDS Lond Engl (2023) 37(3):523–33. doi: 10.1097/QAD.0000000000003452
42. Nkinda L, Buberwa E, Memiah P, Ntagalinda A, George M, Msafiri F, et al. Impaired fasting glucose levels among perinatally HIV-infected adolescents and youths in dar es salaam, Tanzania. Front Endocrinol (2022) 13:1045628. doi: 10.3389/fendo.2022.1045628
43. Oyenusi EE, Micondo KH, Dainguy ME, Abodo RJ, Folquet MA, Oduwole AO. Fasting blood glucose profile of children living with HIV taking first-line antiretroviral treatment in Abidjan, cote D’Ivoire: a cross-sectional study. Romanian J Diabetes Nutr Metab Dis (2020) 27(2):90–8. doi: 10.46389/rjd-2020-1016
44. Zhang Z, Duri K, Duisters KLW, Schoeman JC, Chandiwana P, Lindenburg P, et al. Altered methionine-sulfone levels are associated with impaired growth in HEU-children. AIDS. (2023) doi: 10.1097/QAD.0000000000003574
45. Schoeman JC, Moutloatse GP, Harms AC, Vreeken RJ, Scherpbier HJ, Van Leeuwen L, et al. Fetal metabolic stress disrupts immune homeostasis and induces proinflammatory responses in human immunodeficiency virus type 1-and combination antiretroviral therapy-exposed infants. J Infect Dis (2017) 216(4):436–46. doi: 10.1093/infdis/jix291
46. Jao J, Kirmse B, Yu C, Qiu Y, Powis K, Nshom E, et al. Lower preprandial insulin and altered fuel use in HIV/Antiretroviral-exposed infants in Cameroon. J Clin Endocrinol Metab (2015) 100(9):3260–9. doi: 10.1210/JC.2015-2198
47. Vilaseca M, Artuch R, Sierra C, Pineda J, López-Vilches M, Muñoz-Almagro C, et al. Low serum carnitine in HIV-infected children on antiretroviral treatment. Eur J Clin Nutr (2003) 57:1317–22. doi: 10.1038/sj.ejcn.1601694
48. Kirmse B, Yao TJ, Hofherr S, Kacanek D, Williams PL, Hobbs CV, et al. Acylcarnitine profiles in HIV-exposed, uninfected neonates in the united states. AIDS Res Hum Retroviruses (2016) 32(4):339–48. doi: 10.1089/aid.2015.0112
49. Farley J, Gona P, Crain M, Cervia J, Oleske J, Seage G, et al. Prevalence of elevated cholesterol and associated risk factors among perinatally HIV-infected children (4-19 years old) in pediatric AIDS clinical trials group 219C. J Acquir Immune Defic Syndr (1999) 38(4):480–7. doi: 10.1097/01.qai.0000139397.30612.96.
50. Claudio CC, Patin RV, Palchetti CZ, Machado DM, Succi RC de M, Oliveira FLC. Nutritional status and metabolic disorders in HIV-exposed uninfected prepubertal children. Nutr Burbank Los Angel Cty Calif (2013) 29(7–8):1020–3. doi: 10.1016/j.nut.2013.01.019
51. Shen J, Liberty A, Shiau S, Strehlau R, Pierson S, Patel F, et al. Mitochondrial impairment in well-suppressed children with perinatal HIV-infection on antiretroviral therapy. AIDS Res Hum Retroviruses (2020) 36(1):27. doi: 10.1089/aid.2018.0182
52. Jao J, Powis KM, Kirmse B, Yu C, Epie F, Nshom E, et al. Lower mitochondrial DNA and altered mitochondrial fuel metabolism in HIV-exposed uninfected infants in Cameroon. AIDS (2017) 31(18):2475–81. doi: 10.1097/QAD.0000000000001647
53. Desquiret-Dumas V, D’Ottavi M, Monnin A, Goudenège D, Méda N, Vizeneux A, et al. Long-term persistence of mitochondrial DNA instability in HIV-exposed uninfected children during and after exposure to antiretroviral drugs and HIV. Biomedicines (2022) 10(8):1786. doi: 10.3390/biomedicines10081786
54. Divi RL, Walker VE, Wade NA, Nagashima K, Seilkop SK, Adams ME, et al. Mitochondrial damage and DNA depletion in cord blood and umbilical cord from infants exposed in utero to combivir. AIDS (2004) 18(7):1013. doi: 10.1097/00002030-200404300-00009
55. Brogly SB, DiMauro S, Van Dyke RB, Williams PL, Naini A, Libutti DE, et al. Short communication: transplacental nucleoside analogue exposure and mitochondrial parameters in HIV-uninfected children. AIDS Res Hum Retroviruses (2011) 27(7):777–83. doi: 10.1089/aid.2010.0204
56. Gojanovich GS, Jacobson DL, Jao J, Russell JS, Van Dyke RB, Libutti DE, et al. Mitochondrial dysfunction and insulin resistance in pubertal youth living with perinatally acquired HIV. AIDS Res Hum Retroviruses (2020) 36(9):703–11. doi: 10.1089/aid.2020.0067
57. Ahmed D, Roy D, Cassol E. Examining relationships between metabolism and persistent inflammation in HIV patients on antiretroviral therapy. Mediators Inflamm (2018) 2018:e6238978. doi: 10.1155/2018/6238978
58. Smith RL, Tan JME, Jonker MJ, Jongejan A, Buissink T, Veldhuijzen S, et al. Beyond the polymerase-γ theory: production of ROS as a mode of NRTI-induced mitochondrial toxicity. PloS One (2017) 12(11):e0187424. doi: 10.1371/journal.pone.0187424
59. Ganta K, Chaubey B. Mitochondrial dysfunctions in HIV infection and antiviral drug treatment. Expert Opin Drug Metab Toxicol (2019) 12:15. doi: 10.1080/17425255.2019.1692814
60. Ganta KK, Chaubey B. Endoplasmic reticulum stress leads to mitochondria-mediated apoptosis in cells treated with anti-HIV protease inhibitor ritonavir. Cell Biol Toxicol (2018). 35(3), 189–204 doi: 10.1007/s10565-018-09451-7
61. Li M, Sopeyin A, Paintsil E. Combination of tenofovir and emtricitabine with efavirenz does not moderate inhibitory effect of efavirenz on mitochondrial function and cholesterol biosynthesis in human T lymphoblastoid cell line. Antimicrob Agents Chemother (2018) 62(9):e00691–18. doi: 10.1128/AAC.00691-18
62. Jao J, Jacobson DL, Russell JS, Wang J, Yu W, Gojanovich GS, et al. Perinatally acquired HIV infection is associated with abnormal blood mitochondrial function during childhood/adolescence. AIDS Lond Engl (2021) 35(9):1385–94. doi: 10.1097/QAD.0000000000002884
63. Schank M, Zhao J, Moorman JP, Yao ZQ. The impact of HIV- and ART-induced mitochondrial dysfunction in cellular senescence and aging. Cells (2021) 10(1):174. doi: 10.3390/cells10010174
64. Gangcuangco LMA, Mitchell BI, Siriwardhana C, Kohorn LB, Chew GM, Bowler S, et al. Correction: mitochondrial oxidative phosphorylation in peripheral blood mononuclear cells is decreased in chronic HIV and correlates with immune dysregulation. PLoS One (2021) 16(3):e0249428. doi: 10.1371/journal.pone.0249428
65. Gangcuangco LMA, Mitchell BI, Siriwardhana C, Kohorn LB, Chew GM, Bowler S, et al. Mitochondrial oxidative phosphorylation in peripheral blood mononuclear cells is decreased in chronic HIV and correlates with immune dysregulation. PLoS One (2020) 15(4):e0231761. doi: 10.1371/journal.pone.0231761
66. Hantzidiamantis PJ, Lappin SL. Physiology, glucose. StatPearls Publishing: Treasure Island (FL) (2021). Available at: https://www.ncbi.nlm.nih.gov/books/NBK545201/.
67. Lema-Pérez L. Main organs involved in glucose metabolism. In: Sugar intake - risks and benefits and the global diabetes epidemic. IntechOpen: Rijeka (2021). Available at: https://www.intechopen.com/chapters/74163.
68. Palmer CS, Hussain T, Duette G, Weller TJ, Ostrowski M, Sada-Ovalle I, et al. Regulators of glucose metabolism in CD4+ and CD8+ T cells. Int Rev Immunol (2016) 35(6):477–88. doi: 10.3109/08830185.2015.1082178
69. Amitrano AM, Berry BJ, Lim K, Kim KD, Waugh RE, Wojtovich AP, et al. Optical control of CD8+ T cell metabolism and effector functions. Front Immunol (2021) 12:666231. doi: 10.3389/fimmu.2021.666231
70. Masson J, Murphy A, Lee M, Ostrowski M, Crowe S, Palmer C. Assessment of metabolic and mitochondrial dynamics in CD4+ and CD8+ T cells in virologically suppressed HIV-positive individuals on combination antiretroviral therapy. PloS One (2017) 12:e0183931. doi: 10.1371/journal.pone.0183931
71. Palmer CS, Anzinger JJ, Butterfield TR, McCune JM, Crowe SM. A simple flow cytometric method to measure glucose uptake and glucose transporter expression for monocyte subpopulations in whole blood. JoVE J Vis Exp (2016) 114):e54255. doi: 10.3791/54255
72. Hegedus A, Kavanagh Williamson M, Huthoff H. HIV-1 pathogenicity and virion production are dependent on the metabolic phenotype of activated CD4+ T cells. Retrovirology (2014) 11:98. doi: 10.1186/s12977-014-0098-4
73. Palmer CS, Ostrowski M, Gouillou M, Tsai L, Yu D, Zhou J, et al. Increased glucose metabolic activity is associated with CD4+ T-cell activation and depletion during chronic HIV infection. AIDS Lond Engl (2014) 28(3):297–309. doi: 10.1097/QAD.0000000000000128
74. Painter SD, Ovsyannikova IG, Poland GA. The weight of obesity on the human immune response to vaccination. Vaccine (2015) 33(36):4422–9. doi: 10.1016/j.vaccine.2015.06.101
75. Andersen CJ, Murphy KE, Fernandez ML. Impact of obesity and metabolic syndrome on Immunity12. Adv Nutr (2016) 7(1):66–75. doi: 10.3945/an.115.010207
76. Hernandez-Romieu AC, Garg S, Rosenberg ES, Thompson-Paul AM, Skarbinski J. Is diabetes prevalence higher among HIV-infected individuals compared with the general population? evidence from MMP and NHANES 2009–2010. BMJ Open Diabetes Res Care (2017) 5(1):e000304. doi: 10.1136/bmjdrc-2016-000304
77. Ketha F, Beatriz P, Sandra T, Thomas N, Ruth N, Lucy M, et al. Insulin resistance and glucose intolerance in HIV infected children on antiretroviral therapy at lubango pediatric hospital - Angola. Int J Virol AIDS (2020) 7(3):71. doi: 10.23937/2469-567X/1510071
78. Lagathu C, Béréziat V, Gorwood J, Fellahi S, Bastard JP, Vigouroux C, et al. Metabolic complications affecting adipose tissue, lipid and glucose metabolism associated with HIV antiretroviral treatment. Expert Opin Drug Saf. (2019) 18(9):829–40. doi: 10.1080/14740338.2019.1644317
79. Mulligan K, Grunfeld C, Tai VW, Algren H, Pang M, Chernoff DN, et al. Hyperlipidemia and insulin resistance are induced by protease inhibitors independent of changes in body composition in patients with HIV infection. JAIDS J Acquir Immune Defic Syndr (2000) 23(1):35. doi 10.1097/00126334-200001010-00005
80. Behrens G, Dejam A, Schmidt H, Balks HJ, Brabant G, Körner T, et al. Impaired glucose tolerance, beta cell function and lipid metabolism in HIV patients under treatment with protease inhibitors. AIDS (1999) 13(10):F63. doi: 10.1097/00002030-199907090-00001
81. Natesan V, Kim SJ. Lipid metabolism, disorders and therapeutic drugs – review. Biomol Ther (2021) 29(6):596–604. doi: 10.4062/biomolther.2021.122
82. Feingold KR. Obesity and dyslipidemia. In: Endotext. MDText.com, Inc: South Dartmouth (MA). (2020) Available at: https://www.ncbi.nlm.nih.gov/books/NBK305895/.
83. Singh J, Verma M, Ghalaut PS, Verma R, Soni A, Ghalaut VS. Alteration in lipid profile in treatment-naive HIV-infected patients and changes following HAART initiation in haryana. J Endocrinol Metab (2014) 4(1–2):25–31. doi: 10.14740/jem207w
84. Godfrey C, Bremer A, Alba D, Apovian C, Koethe JR, Koliwad S, et al. Obesity and fat metabolism in human immunodeficiency virus-infected individuals: immunopathogenic mechanisms and clinical implications. J Infect Dis (2019) 220(3):420–31. doi: 10.1093/infdis/jiz118
85. Castellano P, Prevedel L, Valdebenito S, Eugenin E. HIV Infection and latency induce a unique metabolic signature in human macrophages. Sci Rep (2019) 9:3941. doi: 10.1038/s41598-019-39898-5
86. Dapena M, Jiménez B, Noguera-Julian A, Soler-Palacín P, Fortuny C, Lahoz R, et al. Metabolic disorders in vertically HIV-infected children: future adults at risk for cardiovascular disease. J Pediatr Endocrinol Metab (2012) 25(5–6):529–35. doi: 10.1515/jpem-2012-0005
87. Miller TL, Borkowsky W, DiMeglio LA, Dooley L, Geffner ME, Hazra R, et al. Metabolic abnormalities and viral replication is associated with biomarkers of vascular dysfunction in HIV-infected children. HIV Med (2012) 13(5):264–75. doi: 10.1111/j.1468-1293.2011.00970.x
88. Jacobson DL, Williams P, Tassiopoulos K, Melvin A, Hazra R, Farley J. Clinical management and follow-up of hypercholesterolemia among perinatally HIV-infected children enrolled in the PACTG 219C study. J Acquir Immune Defic Syndr (1999) 57(5):413–20. doi: 10.1097/QAI.0b013e31822203f5
89. Niki E. Biomarkers of lipid peroxidation in clinical material. Biochim Biophys Acta BBA - Gen Subj (2014) 1840(2):809–17. doi: 10.1016/j.bbagen.2013.03.020
90. Yadav N, Kumar S, Marlowe T, Chaudhary AK, Kumar R, Wang J, et al. Oxidative phosphorylation-dependent regulation of cancer cell apoptosis in response to anticancer agents. Cell Death Dis (2015) 6(11):e1969–9. doi: 10.1038/cddis.2015.305
91. Périard D, Telenti A, Sudre P, Cheseaux JJ, Halfon P, Reymond MJ, et al. Atherogenic dyslipidemia in HIV-infected individuals treated with protease inhibitors. Circulation (1999) 100(7):700–5. doi: 10.1161/01.CIR.100.7.700
92. Zha BS, Wan X, Zhang X, Zha W, Zhou J, Wabitsch M, et al. HIV Protease inhibitors disrupt lipid metabolism by activating endoplasmic reticulum stress and inhibiting autophagy activity in adipocytes. PloS One (2013) 8(3):e59514. doi: 10.1371/journal.pone.0059514
93. Levy AR, McCandless L, Harrigan PR, Hogg RS, Bondy G, Iloeje UH, et al. Changes in lipids over twelve months after initiating protease inhibitor therapy among persons treated for HIV/AIDS. Lipids Health Dis (2005) 4(1):4. doi: 10.1186/1476-511X-4-4
94. Waters DD, Hsue PY. Lipid abnormalities in persons living with HIV infection. Can J Cardiol (2019) 35(3):249–59. doi: 10.1016/j.cjca.2018.11.005
95. Apostolova N, Blas-García A, Esplugues JV. Mitochondrial interference by anti-HIV drugs: mechanisms beyond pol-γ inhibition. Trends Pharmacol Sci (2011) 32(12):715–25. doi: 10.1016/j.tips.2011.07.007
96. Lordan R, Tsoupras A, Zabetakis I. Phospholipids of animal and marine origin: structure, function, and anti-inflammatory properties. Molecules (2017) 22(11):1964. doi: 10.3390/molecules22111964
97. Hayamizu K. 21 - amino acids and energy metabolism: an overview. In: Bagchi D, editor. Sustained energy for enhanced human functions and activity. Academic Press (2017). p. 339–49. Available at: https://www.sciencedirect.com/science/article/pii/B9780128054130000211.
98. Meisenberg G, Simmons W. Amino acid metabolism. (2012), E-book Published by Saunders Elsevier 3rd edition 441–62. doi: 10.1016/B978-0-323-07155-0.00026-5
99. Kedia-Mehta N, Finlay DK. Competition for nutrients and its role in controlling immune responses. Nat Commun (2019) 10:2123. doi: 10.1038/s41467-019-10015-4
100. Choi C, Finlay DK. Optimising NK cell metabolism to increase the efficacy of cancer immunotherapy. Stem Cell Res Ther (2021) 12(1):320. doi: 10.1186/s13287-021-02377-8
101. Loftus RM, Assmann N, Kedia-Mehta N, O’Brien KL, Garcia A, Gillespie C, et al. Amino acid-dependent cMyc expression is essential for NK cell metabolic and functional responses in mice. Nat Commun (2018) 9:2341. doi: 10.1038/s41467-018-04719-2
102. Ziegler TR, Judd SE, Ruff JH, McComsey GA, Eckard AR. Amino acid concentrations in HIV-infected youth compared to healthy controls and associations with CD4 counts and inflammation. AIDS Res Hum Retroviruses (2017) 33(7):681–9. doi: 10.1089/aid.2015.0369
103. Höhn A, Jung T, Grune T. Pathophysiological importance of aggregated damaged proteins. Free Radic Biol Med (2014) 71:70–89. doi: 10.1016/j.freeradbiomed.2014.02.028
104. Bin P, Huang R, Zhou X. Oxidation resistance of the sulfur amino acids: methionine and cysteine. BioMed Res Int (2017) 2017:e9584932. doi: 10.1155/2017/9584932
105. Kirmse B, Baumgart S, Rakhmanina N. Metabolic and mitochondrial effects of antiretroviral drug exposure in pregnancy and postpartum: implications for fetal and future health. Semin Fetal Neonatal Med (2013) 18(1):48–55. doi: 10.1016/j.siny.2012.10.005
106. Babu H, Sperk M, Ambikan AT, Rachel G, Viswanathan VK, Tripathy SP, et al. Plasma metabolic signature and abnormalities in HIV-infected individuals on long-term successful antiretroviral therapy. Metabolites (2019) 9(10):E210. doi: 10.3390/metabo9100210
107. Chaudhury S, Mayondi GK, Williams PL, Leidner J, Shapiro R, Diseko M, et al. In utero exposure to antiretrovirals and neurodevelopment among HIV-exposed uninfected children in Botswana. AIDS Lond Engl (2018) 32(9):1173–83. doi: 10.1097/QAD.0000000000001790
108. Ruamtawee W, Tipayamongkholgul M, Aimyong N, Manosuthi W. Prevalence and risk factors of cardiovascular disease among people living with HIV in the Asia-pacific region: a systematic review. BMC Public Health (2023) 23(1):477. doi: 10.1186/s12889-023-15321-7
109. Bonnet D. Cardiovascular complications in HIV-infected children. In: Cardiovascular disease in AIDS. Milano: Springer (2009). doi: 10.1007/978-88-470-0761-1_14
110. McCrary AW, Nyandiko WM, Ellis AM, Chakraborty H, Muehlbauer MJ, Koech MM, et al. Early cardiac dysfunction in children and young adults with perinatally acquired HIV. AIDS Lond Engl (2020) 34(4):539–48. doi: 10.1097/QAD.0000000000002445
111. Nixon GF. Sphingolipids in inflammation: pathological implications and potential therapeutic targets. Br J Pharmacol (2009) 158(4):982–93. doi: 10.1111/j.1476-5381.2009.00281.x
112. Olsen ASB, Færgeman NJ. Sphingolipids: membrane microdomains in brain development, function and neurological diseases. Open Biol (2017) 7(5):170069. doi: 10.1098/rsob.170069
113. McGurk KA, Keavney BD, Nicolaou A. Circulating ceramides as biomarkers of cardiovascular disease: evidence from phenotypic and genomic studies. Atherosclerosis (2021) 327:18–30. doi: 10.1016/j.atherosclerosis.2021.04.021
114. Kakkar F, Lamarre V, Ducruet T, Boucher M, Valois S, Soudeyns H, et al. Impact of maternal HIV-1 viremia on lymphocyte subsets among HIV-exposed uninfected infants: protective mechanism or immunodeficiency. BMC Infect Dis (2014) 14:236. doi: 10.1186/1471-2334-14-236
115. Borges-Almeida E, Milanez HM, Vilela MMS, Cunha FG, Abramczuk BM, Reis-Alves SC, et al. The impact of maternal HIV infection on cord blood lymphocyte subsets and cytokine profile in exposed non-infected newborns. BMC Infect Dis (2011) 11(1):38. doi: 10.1186/1471-2334-11-38
116. Ruck C, Reikie BA, Marchant A, Kollmann TR, Kakkar F. Linking susceptibility to infectious diseases to immune system abnormalities among HIV-exposed uninfected infants. Front Immunol (2016) 7:310. doi: 10.3389/fimmu.2016.00310
117. Goetghebuer T, Rowland-Jones SL, Kollmann TR. Editorial: immune mechanisms underlying the increased morbidity and mortality of HIV-exposed uninfected (HEU) children. Front Immunol (2017) 8:1060. doi: 10.3389/fimmu.2017.01060
118. Falconer O, Newell ML, Jones CE. The effect of human immunodeficiency virus and cytomegalovirus infection on infant responses to vaccines: a review. Front Immunol (2018) 9:328. doi: 10.3389/fimmu.2018.00328
119. Abramczuk BM, Mazzola TN, Moreno YMF, Zorzeto TQ, Quintilio W, Wolf PS, et al. Impaired humoral response to vaccines among HIV-exposed uninfected infants ▿. Clin Vaccine Immunol CVI. (2011) 8(9):1406–9. doi: 10.1128/CVI.05065-11
120. Baroncelli S, Galluzzo CM, Liotta G, Andreotti M, Orlando S, Ciccacci F, et al. HIV-Exposed infants with EBV infection have a reduced persistence of the immune response to the HBV vaccine. AIDS Res Ther (2021) 18:48. doi: 10.1186/s12981-021-00375-7
121. Saso A, Kampmann B. Vaccine responses in newborns. Semin Immunopathol (2017) 39(6):627–42. doi: 10.1007/s00281-017-0654-9
122. Jones CE, Hesseling AC, Tena-Coki NG, Scriba TJ, Chegou NN, Kidd M, et al. The impact of HIV exposure and maternal mycobacterium tuberculosis infection on infant immune responses to bacille calmette-guerin vaccination. Aids (2015) 29(2):155–65. doi: 10.1097/QAD.0000000000000536
123. Scully E, Alter G. NK cells in HIV disease. Curr HIV/AIDS Rep (2016) 13:85–94. doi: 10.1007/s11904-016-0310-3
124. Smith C, Jalbert E, de Almeida V, Canniff J, Lenz LL, Mussi-Pinhata MM, et al. Altered natural killer cell function in HIV-exposed uninfected infants. Front Immunol (2017) 8:470–0. doi: 10.3389/fimmu.2017.00470
125. Slyker JA, Lohman-Payne B, John-Stewart GC, Dong T, Mbori-Ngacha D, Tapia K, et al. The impact of HIV-1 infection and exposure on natural killer (NK) cell phenotype in Kenyan infants during the first year of life. Front Immunol (2012) 3:399. doi: 10.3389/fimmu.2012.00399
126. Ballan WM, Vu BAN, Long BR, Loo CP, Michaëlsson J, Barbour JD, et al. Natural killer cells in perinatally HIV-1-Infected children exhibit less degranulation compared to HIV-1-Exposed uninfected children and their expression of KIR2DL3, NKG2C, and NKp46 correlates with disease severity. J Immunol (2007) 179(5):3362–70. doi: 10.4049/jimmunol.179.5.3362
127. Korencak M, Byrne M, Richter E, Schultz BT, Juszczak P, Ake JA, et al. Effect of HIV infection and antiretroviral therapy on immune cellular functions. JCI Insight (2019) 4(12):e126675. doi: 10.1172/jci.insight.126675
128. Cassol E, Cassetta L, Rizzi C, Alfano M, Poli G. M1 and M2a polarization of human monocyte-derived macrophages inhibits HIV-1 replication by distinct mechanisms. J Immunol (2009) 182(10):6237–46. doi: 10.4049/jimmunol.0803447
129. Galvão-Lima LJ, Espíndola MS, Soares LS, Zambuzi FA, Cacemiro M, Fontanari C, et al. Classical and alternative macrophages have impaired function during acute and chronic HIV-1 infection. Braz J Infect Dis (2017) 21(1):42–50. doi: 10.1016/j.bjid.2016.10.004
130. Velilla PA, Montoya CJ, Hoyos A, Moreno ME, Chougnet C, Rugeles MT. Effect of intrauterine HIV-1 exposure on the frequency and function of uninfected newborns’ dendritic cells. Clin Immunol (2008) 126(3):243–50. doi: 10.1016/j.clim.2007.11.004
131. Reikie BA, Adams RCM, Leligdowicz A, Ho K, Naidoo S, Ruck CE, et al. Altered innate immune development in HIV-exposed uninfected infants. JAIDS J Acquir Immune Defic Syndr (2014) 66(3):245–55. doi: 10.1097/QAI.0000000000000161
132. Freemerman AJ, Johnson AR, Sacks GN, Milner JJ, Kirk EL, Troester MA, et al. Metabolic reprogramming of macrophages: glucose transporter 1 (GLUT1)-mediated glucose metabolism drives a proinflammatory phenotype. J Biol Chem (2014) 289(11):7884–96. doi: 10.1074/jbc.M113.522037
133. Datta PK, Deshmane S, Khalili K, Merali S, Gordon JC, Fecchio C, et al. Glutamate metabolism in HIV-1 infected macrophages: role of HIV-1 vpr. Cell Cycle (2016) 15(17):2288–98. doi: 10.1080/15384101.2016.1190054
134. Jenabian MA, Patel M, Kema I, Kanagaratham C, Radzioch D, Thébault P, et al. Distinct tryptophan catabolism and Th17/Treg balance in HIV progressors and elite controllers. PloS One (2013) 8(10):e78146. doi: 10.1371/journal.pone.0078146
135. Wallace J, Gonzalez H, Rajan R, Narasipura SD, Virdi AK, Olali AZ, et al. Anti-HIV drugs cause mitochondrial dysfunction in monocyte-derived macrophages. Antimicrob Agents Chemother (2022) 66(4):e01941–21. doi: 10.1128/aac.01941-21
136. Ssewanyana I, Elrefaei M, Dorsey G, Ruel T, Jones NG, Gasasira A, et al. Profile of T cell immune responses in HIV-infected children from Uganda. J Infect Dis (2007) 196(11):1667–70. doi: 10.1086/522013
137. Miyamoto M, Gouvêa AFTB, Ono E, Succi RCM, Pahwa S, Moraes-Pinto MIde. Immune development in HIV-exposed uninfected children born to HIV-infected women. Rev Inst Med Trop Sao Paulo (2017) 59. doi: 10.1590/s1678-9946201759030
138. Foldi J, Kozhaya L, McCarty B, Mwamzuka M, Marshed F, Ilmet T, et al. HIV-Infected children have elevated levels of PD-1+ memory CD4 T cells with low proliferative capacity and high inflammatory cytokine effector functions. J Infect Dis (2017) 216(6):641–50. doi: 10.1093/infdis/jix341
139. Sears JD, Waldron KJ, Wei J, Chang CH. Targeting metabolism to reverse T-cell exhaustion in chronic viral infections. Immunology (2021) 162(2):135–44. doi: 10.1111/imm.13238
140. Rahman ANur, Liu J, Mujib S, Kidane S, Ali A, Szep S, et al. Elevated glycolysis imparts functional ability to CD8+ T cells in HIV infection. Life Sci Alliance (2021) 4(11). doi: 10.26508/lsa.202101081
141. Kang S, Tang H. HIV-1 infection and glucose metabolism reprogramming of T cells: another approach toward functional cure and reservoir eradication. Front Immunol (2020) 11:2621. doi: 10.3389/fimmu.2020.572677
142. Trautmann L, Janbazian L, Chomont N, Said EA, Gimmig S, Bessette B, et al. Upregulation of PD-1 expression on HIV-specific CD8+ T cells leads to reversible immune dysfunction. Nat Med (2006) 12(10):1198–202. doi: 10.1038/nm1482
143. Clerici M, Saresella M, Colombo F, Fossati S, Sala N, Bricalli D, et al. T-Lymphocyte maturation abnormalities in uninfected newborns and children with vertical exposure to HIV. Blood (2000) 96(12):3866–71. doi: 10.1182/blood.V96.12.3866
144. Bunders M, Thorne C, Newell ML, Study for the EC. Maternal and infant factors and lymphocyte, CD4 and CD8 cell counts in uninfected children of HIV-1-infected mothers. AIDS (2005) 19(10):1071–9. doi: 10.1097/01.aids.0000174454.63250.22
145. Le Chenadec J, Mayaux MJ, Guihenneuc-Jouyaux C, Blanche S. Perinatal antiretroviral treatment and hematopoiesis in HIV-uninfected infants. Aids (2003) 17(14):2053–61. doi: 10.1097/00002030-200309260-00006
146. Rich KC, Siegel JN, Jennings C, Rydman RJ, Landay AL. Function and phenotype of immature CD4(+) lymphocytes in healthy infants and early lymphocyte activation in uninfected infants of human immunodeficiency virus-infected mothers. Clin Diagn Lab Immunol (1997) 4(3):358–61. doi: 10.1128/cdli.4.3.358-361.1997
147. Deng K, Pertea M, Rongvaux A, Wang L, Durand CM, Ghiaur G, et al. Broad CTL response is required to clear latent HIV-1 due to dominance of escape mutations. Nature (2015) 517(7534):381–5. doi: 10.1038/nature14053
148. Bailon L, Mothe B, Berman L, Brander C. Novel approaches towards a functional cure of HIV/AIDS. Drugs (2020) 80(9):859–68. doi: 10.1007/s40265-020-01322-y
149. Ward AR, Mota TM, Jones RB. Immunological approaches to HIV cure. Semin Immunol (2021) 51:101412. doi: 10.1016/j.smim.2020.101412
150. Maina EK, Adan AA, Mureithi H, Muriuki J, Lwembe RM. A review of current strategies towards the elimination of latent HIV-1 and subsequent HIV-1 cure. Curr HIV Res (2021) 19(1):14–26. doi: 10.2174/1570162X18999200819172009
151. Thomas J, Ruggiero A, Paxton WA, Pollakis G. Measuring the success of HIV-1 cure strategies. Front Cell Infect Microbiol (2020) 10. doi: 10.3389/fcimb.2020.00134
152. Kufel WD. Antibody-based strategies in HIV therapy. Int J Antimicrob Agents (2020) 56(6):106186. doi: 10.1016/j.ijantimicag.2020.106186
153. Moldt B, Chandrashekar A, Borducchi EN, Nkolola JP, Stephenson H, Nagel M, et al. HIV Envelope antibodies and TLR7 agonist partially prevent viral rebound in chronically SHIV-infected monkeys. PloS Pathog (2022) 18(4):e1010467. doi: 10.1371/journal.ppat.1010467
154. Singh V, Dashti A, Mavigner M, Chahroudi A. Latency reversal 2.0: giving the immune system a seat at the table. Curr HIV/AIDS Rep (2021) 18(2):117–27. doi: 10.1007/s11904-020-00540-z.
155. Nixon CC, Mavigner M, Sampey GC, Brooks AD, Spagnuolo RA, Irlbeck DM, et al. Systemic HIV and SIV latency reversal via non-canonical NF-κB signalling in vivo. Nature (2020) 578(7793):160–5. doi: 10.1038/s41586-020-1951-3
156. Dashti A, Waller C, Mavigner M, Schoof N, Bar KJ, Shaw GM, et al. SMAC mimetic plus triple-combination bispecific HIVxCD3 retargeting molecules in SHIV.C.CH505-infected, antiretroviral therapy-suppressed rhesus macaques. J Virol (2020) 94(21):e00793–20. doi: 10.1128/JVI.00793-20
157. Guo H, Wang Q, Ghneim K, Wang L, Rampanelli E, Holley-Guthrie E, et al. Multi-omics analyses reveal that HIV-1 alters CD4+ T cell immunometabolism to fuel virus replication. Nat Immunol (2021) 22(4):423–33. doi: 10.1038/s41590-021-00898-1
158. Rezaee H, Khalili H, Salamzadeh J, Jafari S, Abdollahi A. Potential benefits of carnitine in HIV-positive patients. Future Virol (2012) 7(1):73–83. doi: 10.2217/fvl.11.133
159. Loignon M, Toma E. L-carnitine for the treatment of highly active antiretroviral therapy-related hypertriglyceridemia in HIV-infected adults. AIDS (2001) 15(9):1194. doi: 10.1097/00002030-200106150-00024
160. De Simone C, Tzantzoglou S, Famularo G, Moretti S, Paoletti F, Vullo V, et al. High dose l-carnitine improves immunologic and metabolic parameters in AIDS patients. Immunopharmacol Immunotoxicol (1993) 15(1):1–12. doi: 10.3109/08923979309066930
161. Claessens YE, Chiche JD, Mira JP, Cariou A. Bench-to-bedside review: severe lactic acidosis in HIV patients treated with nucleoside analogue reverse transcriptase inhibitors. Crit Care (2003) 7(3):226. doi: 10.1186/cc2162
162. Di Marzio L, Moretti S, D’Alò S, Zazzeroni F, Marcellini S, Smacchia C, et al. Acetyl-l-carnitine administration increases insulin-like growth factor 1 levels in asymptomatic HIV-1-infected subjects: correlation with its suppressive effect on lymphocyte apoptosis and ceramide generation. Clin Immunol Orlando Fla (1999) 92(1):103–10. doi: 10.1006/clim.1999.4727
163. Clerc I, Moussa DA, Vahlas Z, Tardito S, Oburoglu L, Hope TJ, et al. Entry of glucose- and glutamine-derived carbons into the citric acid cycle supports early steps of HIV-1 infection in CD4 T cells. Nat Metab (2019) 1(7):717–30. doi: 10.1038/s42255-019-0084-1
164. Shytaj IL, Procopio FA, Tarek M, Carlon-Andres I, Tang HY, Goldman AR, et al. Glycolysis downregulation is a hallmark of HIV-1 latency and sensitizes infected cells to oxidative stress. EMBO Mol Med (2021) 13(8):e13901. doi: 10.15252/emmm.202013901
165. Valle-Casuso JC, Angin M, Volant S, Passaes C, Monceaux V, Mikhailova A, et al. Cellular metabolism is a major determinant of HIV-1 reservoir seeding in CD4+ T cells and offers an opportunity to tackle infection. Cell Metab (2019) 29(3):611–26.e5. doi: 10.1016/j.cmet.2018.11.015
166. Kennedy BK, Lamming DW. The mechanistic target of rapamycin: the grand conducTOR of metabolism and aging. Cell Metab (2016) 23(6):990–1003. doi: 10.1016/j.cmet.2016.05.009
167. Li J, Zhong L, Wang F, Zhu H. Dissecting the role of AMP-activated protein kinase in human diseases. Acta Pharm Sin B (2017) 7(3):249–59. doi: 10.1016/j.apsb.2016.12.003
168. Bharath LP, Agrawal M, McCambridge G, Nicholas DA, Hasturk H, Liu J, et al. Metformin enhances autophagy and normalizes mitochondrial function to alleviate aging-associated inflammation. Cell Metab (2020) 32(1):44–55.e6. doi: 10.1016/j.cmet.2020.04.015
169. Arbor S. Where and how in the mTOR pathway inhibitors fight aging: rapamycin, resveratrol, and metformin. In: Resveratrol - adding life to years, not adding years to life. IntechOpen: Rijeka (2018). Available at: https://www.intechopen.com/chapters/62579.
170. Besnard E, Hakre S, Kampmann M, Lim HW, Hosmane NN, Martin A, et al. The mTOR complex controls HIV latency. Cell Host Microbe (2016) 20(6):785–97. doi: 10.1016/j.chom.2016.11.001
171. Planas D, Pagliuzza A, Ponte R, Fert A, Marchand LR, Massanella M, et al. LILAC pilot study: effects of metformin on mTOR activation and HIV reservoir persistence during antiretroviral therapy. EBioMedicine (2021) 65:103270. doi: 10.1016/j.ebiom.2021.103270
172. Routy JP, Isnard S, Mehraj V, Ostrowski M, Chomont N, Ancuta P, et al. Effect of metformin on the size of the HIV reservoir in non-diabetic ART-treated individuals: single-arm non-randomised lilac pilot study protocol. BMJ Open (2019) 9(4):e028444. doi: 10.1136/bmjopen-2018-028444
Keywords: early life antiretroviral therapy exposure, mother to child transmission of HIV, immune-metabolic dysregulation, mitochondrial toxicity, immunity
Citation: Mataramvura H, Bunders MJ and Duri K (2023) Human immunodeficiency virus and antiretroviral therapy-mediated immune cell metabolic dysregulation in children born to HIV-infected women: potential clinical implications. Front. Immunol. 14:1182217. doi: 10.3389/fimmu.2023.1182217
Received: 08 March 2023; Accepted: 25 May 2023;
Published: 07 June 2023.
Edited by:
Nilu Goonetilleke, University of North Carolina at Chapel Hill, United StatesReviewed by:
Mireille Laforge, INSERM U1141 Neuroprotection du Cerveau en Développement, FranceCopyright © 2023 Mataramvura, Bunders and Duri. This is an open-access article distributed under the terms of the Creative Commons Attribution License (CC BY). The use, distribution or reproduction in other forums is permitted, provided the original author(s) and the copyright owner(s) are credited and that the original publication in this journal is cited, in accordance with accepted academic practice. No use, distribution or reproduction is permitted which does not comply with these terms.
*Correspondence: Kerina Duri, a2VyaW5hLmR1cmlAZ21haWwuY29t, a2R1cmlAbWVkc2NoLnV6LmFjLnp3
Disclaimer: All claims expressed in this article are solely those of the authors and do not necessarily represent those of their affiliated organizations, or those of the publisher, the editors and the reviewers. Any product that may be evaluated in this article or claim that may be made by its manufacturer is not guaranteed or endorsed by the publisher.
Research integrity at Frontiers
Learn more about the work of our research integrity team to safeguard the quality of each article we publish.