- Center for Cancer Research and Comprehensive Cancer Center, Medical University of Vienna, Vienna, Austria
Long noncoding RNAs (lncRNAs) increase in genomes of complex organisms and represent the largest group of RNA genes transcribed in mammalian cells. Previously considered only transcriptional noise, lncRNAs comprise a heterogeneous class of transcripts that are emerging as critical regulators of T cell-mediated immunity. Here we summarize the lncRNA expression landscape of different T cell subsets and highlight recent advances in the role of lncRNAs in regulating T cell differentiation, function and exhaustion during homeostasis and cancer. We discuss the different molecular mechanisms of lncRNAs and highlight lncRNAs that can serve as novel targets to modulate T cell function or to improve the response to cancer immunotherapies by modulating the immunosuppressive tumor microenvironment.
1 Introduction
Estimates suggest that less than 3% of transcripts in the human genome are protein-coding (1). According to large-scale projects such as ENCODE, at least 80% of the mammalian genome is actively transcribed, and the majority is noncoding RNAs (ncRNAs) (1–3). NcRNAs are molecules that lack a protein-coding sequence. Within the noncoding transcriptome, small noncoding RNAs (sncRNAs) (< 50 bp in size) (4, 5) can be distinguished from long noncoding RNAs (lncRNAs) (> 200 bp in size) (6). Different sncRNAs have been characterized such as microRNAs (miRNAs), transfer RNAs (tRNAs), PIWI-interacting RNAs (piRNAs), small nuclear RNAs (snRNAs) and small interfering RNAs (siRNAs) (4, 5). Interestingly, ncRNAs can function as therapeutic targets, and the first RNAi drug has been approved for clinical use in 2018 (7, 8). Although numerous differentially regulated lncRNAs in immune cells and cancer have been described (2, 3, 6), no lncRNA-targeting therapeutics are in clinical use yet (8).
All lncRNAs except circular RNAs (circRNAs) are classified based on their relative position to the loci of protein-coding genes and enhancers: (1) long intergenic noncoding RNAs (lincRNAs), (2) (anti-)sense lncRNAs, (3) intronic lncRNAs, (4) bidirectional RNAs and (5) enhancer RNAs (eRNA) (9–11). Most lncRNAs are capped by 7-methyl guanosine (m7G), spliced less efficiently than mRNAs, and are polyadenylated and transcribed by RNA polymerase II. Though, eRNAs are mostly bidirectionally capped transcripts that are not spliced or polyadenylated and that are primarily unstable (12). And circRNAs are produced by back-splicing where an exon is spliced to an upstream instead of a downstream exon (13).
The question of how many lncRNAs are located in the human genome is challenging to answer as there are considerable discrepancies between automated annotations and the highly-curated ENCODE collection (10, 14). The number of lncRNAs reported in recent human genome annotations ranges from 27,919 to 58,648. Due to functional and transcriptional noise, the exact number of functional lncRNAs is still debated. Yet, the cellular function of a growing number of lncRNAs has been identified experimentally (15, 16).
Interestingly, the number of lncRNAs is associated with the complexity of an organism, and species- and cell type-specific expression is frequently observed (17, 18). Although the majority of lncRNAs are not evolutionarily conserved, there are still thousands of syntonic (conservation at genomic position) and sequence-based conserved lncRNAs (17, 19). Yet, in a study by Guo and colleagues, several conserved lncRNAs are processed differently in human embryonic stem cells (ESC) compared to murine ESC, resulting in different subcellular location and function (20). These findings indicate that the mode of lncRNA conservation across species is not yet completely understood.
Even though lncRNAs are expressed on average at a lower level compared to protein-coding genes, which generate more RNA molecules per transcriptional burst (21), lncRNAs engage through modular domains with DNA, RNA or proteins (12). Thereby, lncRNAs have crucial functions in many different cellular processes, including chromatin regulation (22, 23), genome integrity (24, 25), regulation of transcription in cis and trans (26), nuclear organization (27) and post-transcriptional functions (28). Even though thousands of lncRNAs have been identified, only a limited number of lncRNAs have been studied to determine their biological significance, such as the role of Xist in X chromosome silencing (29) and H19 in genomic imprinting (30).
T lymphocytes are a heterogeneous group of immune cells that include cytotoxic CD8+ T cells, CD4+ helper T (Th) cells, regulatory T cells (Tregs), gamma delta (γδ) T cells and natural killer T cells (NKT cells). The development, differentiation and function of these T cell subsets is tightly regulated by transcriptional programs and epigenetic processes (31–33). Naïve CD4+ T cells can differentiate into various effector cell lineages, such as Th1, Th2 and Th17 cells, which secrete different effector molecules and thereby characteristically shape the tumor microenvironment (TME) and the prognosis of cancer patients (34–36). Cytotoxic CD8+ T cells mediate tumor cell killing by recognizing tumor neoantigens and enhanced CD8+ T cell infiltration into tumors, in particular those with a high tumor mutational burden (TMB), is associated with better prognosis in almost all solid cancers (36). However, an immunosuppressive TME and chronic stimulation by tumor antigens can lead to dysfunctional CD8+ T cells, referred to as exhausted CD8+ T cells (CD8+ Tex) (37, 38). In addition, the absence of interleukin (IL)-21-expressing CD4+ T cells or increased levels of IL-10 and transforming growth factor beta (TGF-β) in the TME have been implicated in the formation and persistence of exhausted CD8+ T cells (CD8+ Tex) (39, 40). CD8+ Tex cells are incapable of effectively utilizing their effector functions even in early stages of malignancy (41, 42). CD8+ Tex cells express inhibitory receptors, such as PD-1, LAG3, CXCR5, TIM-3, CD244 (also known as 2B4 or SLAMF4), CD38, CD39 and CD101 (43–48) as well as transcription factors, such as Tox, TCF-1, T-bet and Eomes (38, 40, 49–53). So far, two major subtypes of CD8+ Tex cells have been described: early PD-1int (progenitor or stem-like) and late PD-1hi (terminally) exhausted T cells (44, 53). PD-1int early Tex cells can be defined by the expression of TCF-1 and T-bet (40, 52, 53), while PD-1hi Tim-3+ late Tex express Tox, Eomes and regulator of G protein signaling 16 (Rgs16) (38, 40, 49–51, 54, 55). A recent study even suggests a 4-stage CD8+ Tex cell model, which is regulated by the TCF-1/T-bet/Tox axis and comprises quiescent, resident Texprog1 (PD-1+ Ly108+ CD69+), Texprog2 (PD-1+ Ly108+ CD69–), Texint (PD-1+ Ly108– CD69–) and terminally exhausted, resident Texterm cells (PD-1+ Ly108– CD69+) (54). The role of lncRNAs in CD8+ T cell exhaustion and its impact on tumor immune evasion and the effectiveness of T cell-targeted therapies, such as immune checkpoint blockade or adoptive T cell therapy, will be discussed in section “2.1 CD8+ T cells”.
Here we highlight recent advances in the role of lncRNAs in regulating the differentiation and function of T cell subsets in homeostasis and cancer. We review which lncRNAs can serve as promising targets to modulate T cell function or to improve the response to cancer immunotherapies by modulating the immunosuppressive tumor microenvironment. We conclude by highlighting the challenges that still lie ahead for the translation of research findings to the clinics and the development of effective lncRNA-based anti-cancer therapies.
2 The role of lncRNAs in T cells during homeostasis and cancer
In this chapter and its associated four subchapters, we provide an overview of the expression of lncRNAs in T cell subsets (CD8+ T cells, CD4+ T cells, regulatory T cells, γδ T cells and NKT cells) during homeostasis and cancer. A large number of lncRNAs has been shown to be expressed in immune cells, including mouse and human T cell subsets (56–61). Of these studies, two demonstrated that CD4+ and CD8+ T cells can be distinguished based on their lncRNA expression profile (56, 57). Spurlock et al. performed whole-genome sequencing to identify CD4+ T cell lineage-specific lncRNAs. They reported that lncRNAs are selectively expressed in human T cells differentiated under Th1-, Th2- and Th17-polarizing conditions (56). These findings are consistent with another study by Hudson et al., demonstrating in humans and mice, that naïve, effector and memory CD8+ T cell populations display unique lncRNA expression profiles (57). They also demonstrate that lncRNAs with synteny or sequence conservation across the two species show a comparable lncRNA expression landscape during differentiation. This implies that lncRNAs may regulate cell states and cell fate decisions during T cell activation and differentiation (56, 57, 62). Figure 1 provides an overview of the expression of lncRNAs in naïve, effector and memory CD8+ and CD4+ T cell subsets in mice and humans.
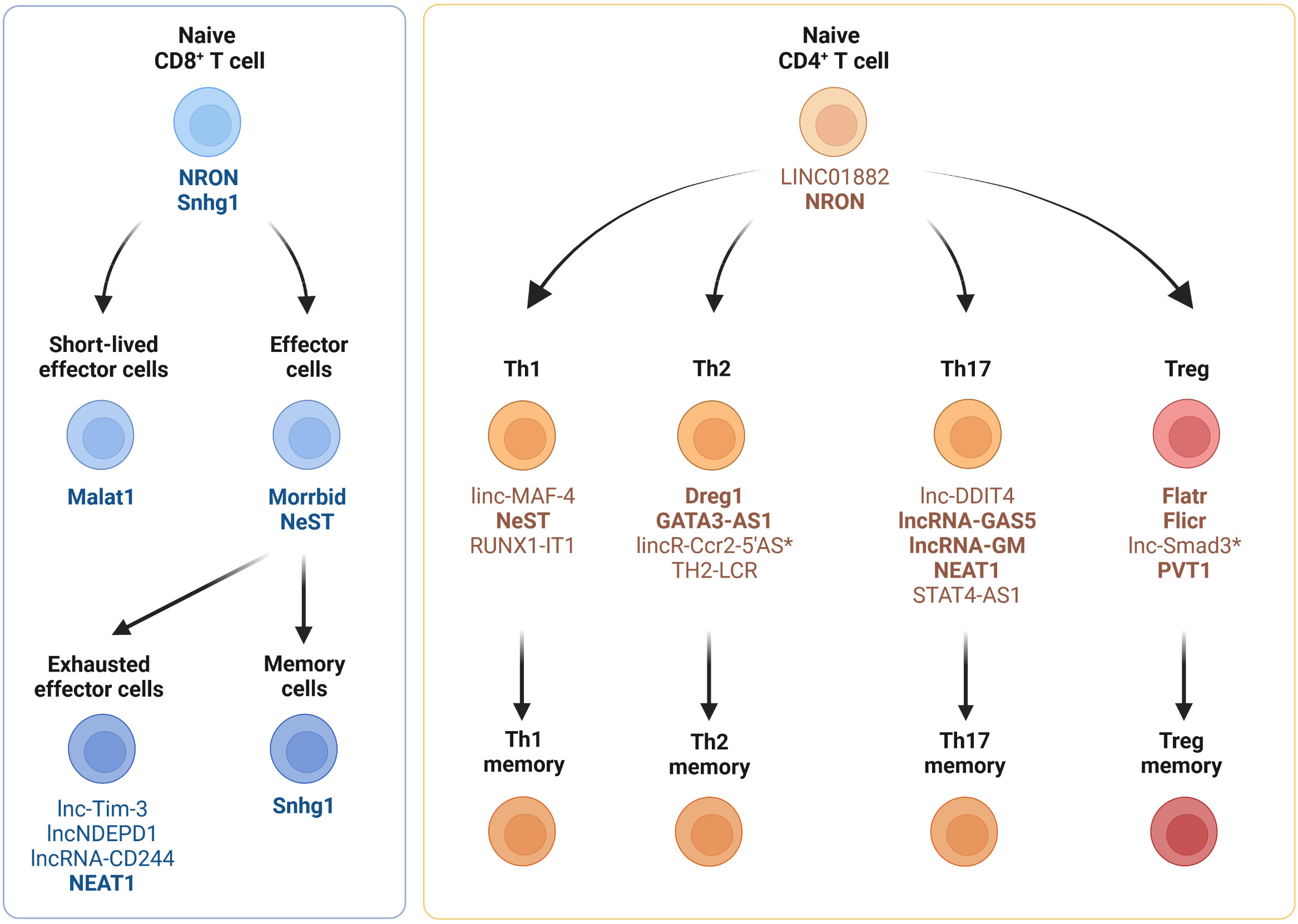
Figure 1 Expression of lncRNAs in CD4+ and CD8+ T cell subsets. Cell type-specific and differentiation-specific lncRNAs that modulate CD4+ or CD8+ T cell function or differentiation during homeostasis, infection and autoimmunity are shown. Th1, CD4+ T helper 1 cells; Th2, CD4+ T helper 2 cells; Th17, CD4+ T helper 17 cells; Treg, regulatory T cells. LncRNAs expressed in humans and mice (bold), lncRNAs expressed solely in mice (*).
Thousands of lncRNAs have been shown to be expressed in cancer cells, metastases and tumor stroma (5, 63–65). Intriguingly, many of the genomic mutations in cancer cells reside inside regions that are transcribed into lncRNAs (66, 67), and the expression and dysregulation of lncRNAs are strikingly cancer-type specific (68). In Chapter 4, we will therefore highlight tumor cell-expressed lncRNAs that drive immune escape by modulating T cell infiltration or T cell dysfunction/exhaustion, and that may therefore represent promising targets for therapeutic intervention.
However, only limited information is available on the expression and role of lncRNAs in tumor-infiltrating T cell subsets and how they affect T cell function, tumor immune escape and the efficacy of T cell-based cancer immunotherapies. Recently, the first comprehensive catalog and functional collection of lncRNAs in human tumor-infiltrating T cells was generated by performing single-cell RNA-sequencing (scRNA-seq) of T cell libraries for three cancer types (62). In this study, 154 lncRNA signature genes were associated with effector, exhausted and regulatory T cell stages of which 84 lncRNAs may modulate T cell function according to functional annotation. Even though the expression profiles and mechanisms were not addressed, this study provides the foundation for future studies of lncRNAs regulating effector and exhausted tumor-infiltrating T cells. In the following four subchapters, we will highlight the most important lncRNAs expressed in T cell subsets (CD8+ T cells, CD4+ T cells, regulatory T cells, γδ T cells and NKT cells), describe their mechanism and summarize their relevance during homeostasis and cancer.
2.1 CD8+ T cells
Although many lncRNAs have been shown to be differentially expressed between CD8+ T cell subsets in mice and humans, only a few lncRNAs have been experimentally validated for their biological relevance to CD8+ T cell differentiation and function (Figure 1 and Table 1). Resting naïve CD8+ and CD4+ T cells express lncRNA NRON (noncoding repressor of NFAT), which limits excessive T cell activation (79–81) and nuclear factor of activated T cells (NFAT)-dependent cytokine production (81). Effector CD8+ T cell differentiation and function are regulated by several other lncRNAs, such as Malat1, NeST and Morrbid (Figure 1). The chromatin-associated lncRNA Malat1 promotes differentiation of short-lived effector cells (SLECs), thereby impairing the generation of memory precursor effector cells (MPEC) and long-lived memory cells (74). Mechanistically, Malat1 directly interacts with EZH2, the catalytic subunit of the polycomb repressive complex 2 (PRC2), thus leading to increased H3K27me3 deposition at memory cell-associated genes. The lncRNA NeST (also known as IFNG-AS1 or Tmevpg1) controls microbial susceptibility by epigenetic activation of the IFN-γ locus in CD8+ T cells (77) and regulates IFN-γ in Th1 cells (78). NeST RNA binds to WDR5, a component of the histone H3-lysine 4 (H3K4) methyltransferase complex, thus altering histone 3 methylation at the IFN-γ locus. The lncRNA Morrbid and its locus have been shown to control CD8+ T cell expansion, survival and effector function during viral infection by regulating the pro-apoptotic factor Bcl2l11 (also known as Bim) and PI3K-AKT signaling strength (84). However, Morrbid is not only expressed in CD8+ T cells but also regulates the survival of neutrophils, eosinophils and classical monocytes via transcriptional regulation of Bcl2l11 (84). Finally, lncRNA SNHG1 has been shown to promote memory CD8+ T cell differentiation via IL-7-mediated signaling as well as initiation of a transcriptional memory differentiation program via the STAT3-TCF1-Blimp1 axis (85).
In cancer, cytotoxic CD8+ T cells mediate tumor cell killing by recognizing tumor neoantigens and enhanced infiltration of tumors by CD8+ T cells is associated with better prognosis in most solid cancers (36). Importantly, TMB and a T cell-inflamed gene expression profile can be used as a pan-cancer biomarker to identify responders and non-responders to anti-PD-1 antibody therapy (86, 87). However, an immunosuppressive TME and chronic stimulation by tumor antigens can lead to dysfunctional CD8+ Tex cells. LncRNAs have been shown to play a key role in regulating CD8+ T cell function and dysfunction in cancer (Table 1 and Figures 1, 2). Here we highlight the role and molecular mechanism of lncRNAs associated with tumor-infiltrating CD8+ T cells, including lncRNAs that modulate CD8+ T cell exhaustion (69, 73, 75) and tumor immune evasion (28, 76, 88). This is of therapeutic significance, since knockdown of lncRNAs lncNDEPD1 and INCR improved the effectiveness of CAR T cell therapy (71, 89).
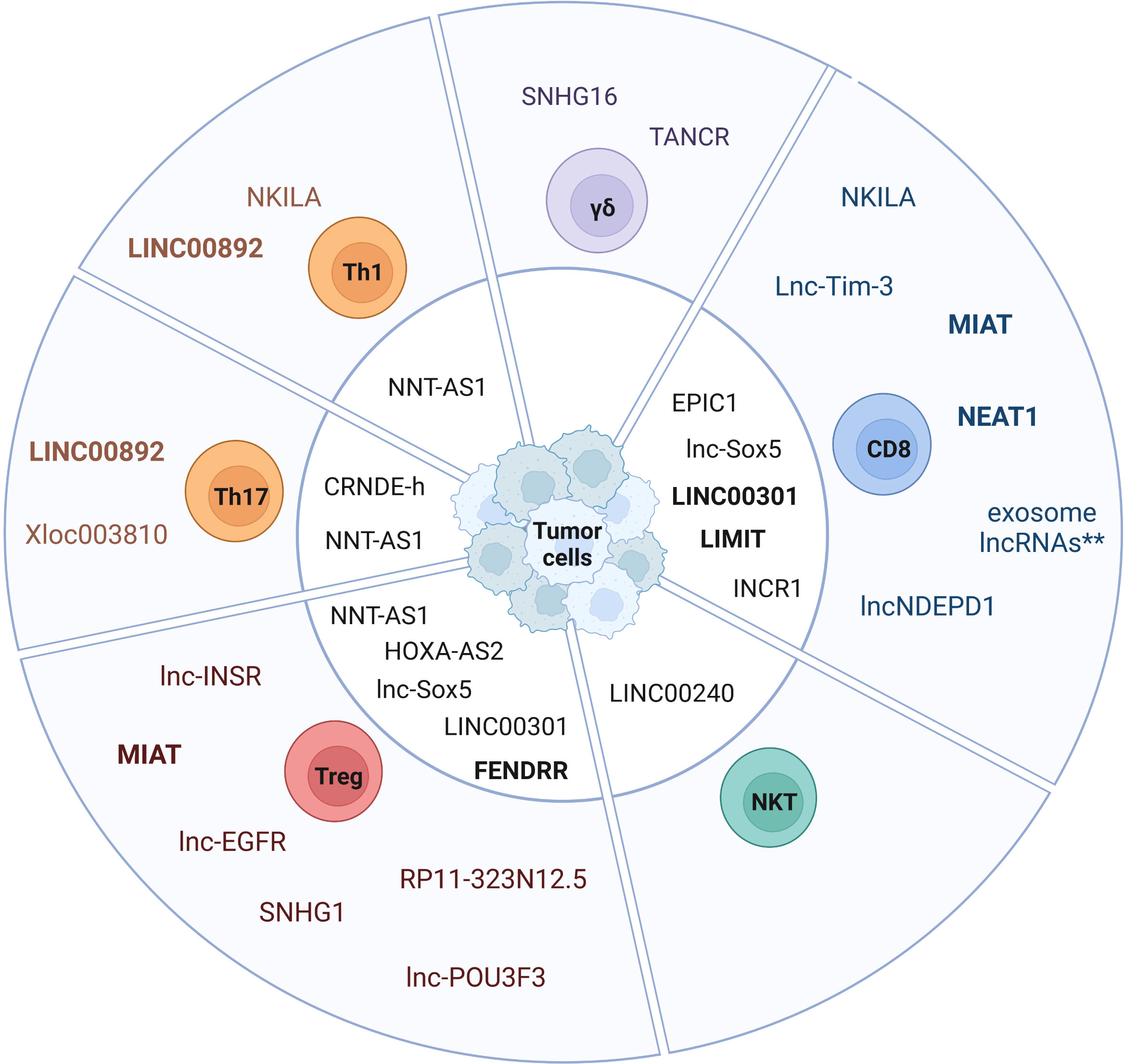
Figure 2 Expression of lncRNAs in tumor-infiltrating T cell subsets. The pie chart depicts lncRNAs expressed in tumor-infiltrating T cell subsets and tumor cell-expressed lncRNAs affecting TIL-specific functions (center). Exosome-derived lncRNAs represent a cluster of lncRNAs that are transmitted from exhausted CD8+ T cells via exosomes to non-exhausted CD8+ T cells (**) (69). LncRNAs expressed in humans and mice are labeled in bold.
High expression of PD-1 and co-expression of molecules such as Tim-3 or CD244 on CD8+ T cells has been associated with chronic T cell receptor activation and impaired effector function (38, 90). In a recent study, lncNDEPD1 has been shown to regulate PD-1 expression on CD8+ T cells in NSCLC patients. Interestingly, knockdown of lncNDEPD1 in chimeric antigen receptor (CAR) T cells reduced tumor growth (71). LncNDEPD1 may therefore represent an interesting target to improve adoptive T cell therapy.
Two lncRNAs that modulate CD8+ T cell exhaustion via the co-inhibitory receptor Tim-3 have been identified in hepatocellular carcinoma (HCC) patients (73, 76). Lnc-Tim-3, a NF-κB-modulating lncRNA, was uncovered by high-throughput screening of CD8+ T cells originating from TILs of HCC patient samples. Lnc-Tim-3 expression highly correlated with Tim-3+ Tex cells and specific binding of the lncRNA to Tim-3 blocked its ligand Bat3 from binding. Consequently, Bat3 facilitated the expression of NF-κB-targeted genes like Bcl-2 and MDM2, thereby promoting survival and exhaustion (73). LncRNA NEAT1 was identified in CD8+ T cells from peripheral blood mononuclear cells (PBMCs) of HCC patients. Mechanistically, NEAT1 increases Tim-3 levels by binding miR-155. SiRNA-mediated knockdown of Neat1 limits apoptosis of CD8+ T cells and improves tumor cell killing (76).
CD244 is another molecule co-expressed on exhausted PD-1+ Tox+ CD8+ T cells in cancer (38) and chronic viral infection (48). Blockade of CD244 signaling using an anti-CD244 antibody reversed exhaustion in virus-specific CD8+ T cells (91). Mechanistically, lncRNA-CD244 is induced by signaling via CD244 in CD8+ T cells and represses IFN-γ and TNF transcription by recruiting the N-methyltransferase EZH2 to IFN-γ and TNF promoters to induce H3K27 methylation (72).
In a study by Huang et al., lncRNA NKILA was shown to promote tumor immune escape. Through functional assays in breast and lung cancer, they uncovered that NKILA sensitizes T cells (cytotoxic CD8+ T cells and CD4+ Th1 cells) to activation-induced cell death (AICD), and high expression of NKILA was associated with reduced patient survival (28). Following T cell activation, IFNγ-JAK-STAT1 signaling induces NKILA transcription. NKILA then binds and inactivates NF-κB (Figure 3A), thereby initiating AICD in CD8+ T cells and CD4+ Th1 cells but not CD4+ Tregs and CD4+ Th2 cells, which shifts the balance towards an immunosuppressive TME (28). Inhibition of NKILA expression may thus represent a promising target for adoptive T cell therapy and improve therapeutic outcome.
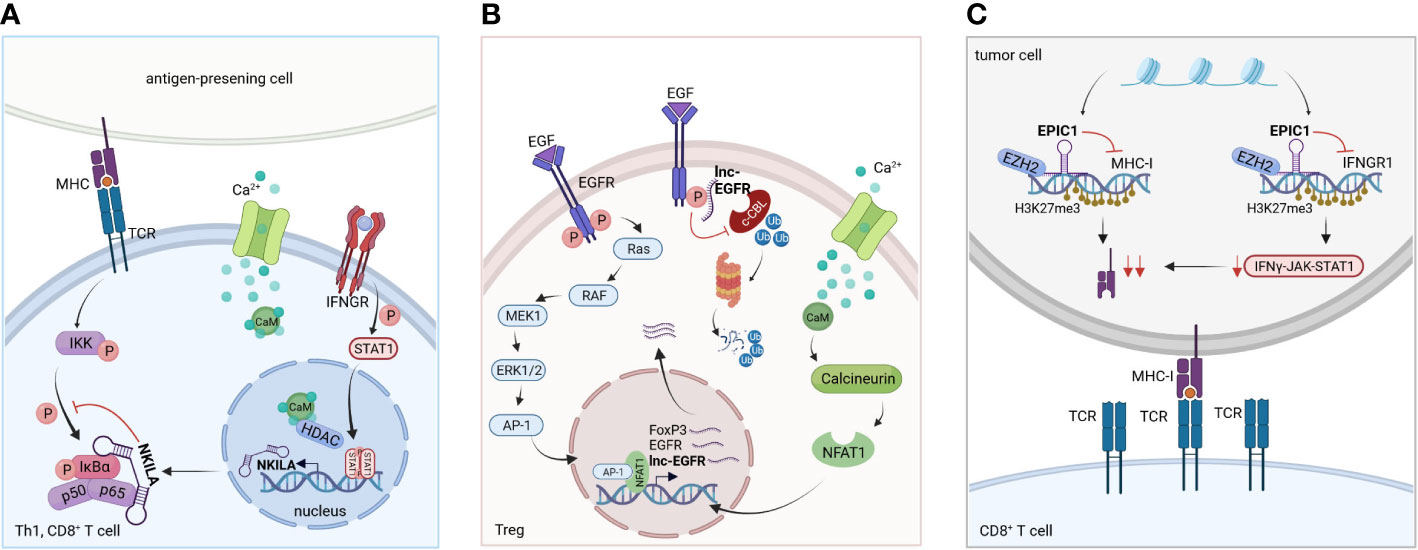
Figure 3 Molecular mechanism of selected lncRNAs. (A) LncRNA NKILA: Following T cell activation, IFNγ-JAK-STAT1 signaling triggers NKILA transcription. STAT1-mediated transcription of NKILA is induced by Ca2+ influx and activation of calmodulin, which removes HDACs from the promotor. Consequently, NKILA directly interacts with the NFκB-subunit p65 and IκBα, regulating the sensitivity to AICD of Th1 and CD8+ T cells. Based on Huang et al. (28). (B) LncRNA EPIC1: EPIC1 together with EZH2 enhances H3K27me3 chromatin modifications at the loci of IFNGR1 and antigen presentation genes, downregulating their transcription. This leads to tumor immune evasion and promotes resistance to immune checkpoint inhibitor therapy by blockage of IFNγ-JAK-STAT1 signaling. Based on Guo et al. (92). (C) LncRNA lnc-EGFR: EGFR is stabilized by the bound lnc-EGFR, thereby blocking its ubiquitination by c-CBL. EGFR activation and signaling via AP-1/NF-AT1 is enhanced, thereby promoting EGFR expression, Treg cell differentiation and tumor progression. Based on Jiang et al. (93).
Altogether, these studies showcase how lncRNAs regulate exhaustion, apoptosis and cytotoxicity in tumor-infiltrating CD8+ T cells. This could open up possibilities to modify these processes to circumvent tumor immune evasion and increase the effectiveness of T cell-targeted immunotherapies, including adoptive T cell therapies.
2.2 CD4+ T cells
CD4+ helper T cells (Th) are highly versatile and polyfunctional cells that display phenotypic plasticity and heterogeneity depending on the microenvironment. Naïve CD4+ T cells can differentiate into various subsets, such as (1) inflammatory Th1 cells, which promote anti-tumor activity through IFN-γ and TNF-α (94), (2) Th2 cells that may contribute to tumorigenesis and tumor progression (95, 96), (3) Th17 cells that have been associated with poor prognosis in hepatocellular carcinoma and thyroid cancer (97), and (4) regulatory T cells that suppress immune responses in the TME (98).
A multitude of lncRNAs has been shown to regulate different CD4+ T cell lineages, which is summarized in Figures 1, 2 and Table 2. Naïve CD4+ T cells express lncRNAs LINC01882 (103) and NRON (79). Mechanistically, lncRNA NRON restricts excessive activation in CD4+ and CD8+ T cells (80, 81). Well-characterized lncRNAs that are expressed in Th1 cells or that modulate Th1 cell differentiation are linc-MAF-4 (105), NeST (77, 117), and RUNX1-IT1 (111). LncRNAs expressed in Th2 cells or that regulate Th2 cell differentiation are Dreg1 (100), GATA3-AS1 (101), lincR-Ccr2-5’AS (104) and TH2-LCR (56). LncRNAs expressed in Th17 cells or that modulate Th17 cell differentiation are lnc-DDIT4 (106), lncRNA-GAS5 (107), lncRNA-GM (108), NEAT1 (109) and STAT4-AS1 (112). LncRNAs that are expressed in Tregs or affect Treg cell differentiation will be discussed in Chapter 2.3.
In CD4+ Th1 cells, deep RNA-seq analysis led to the identification of the chromatin-associated linc-MAF-4. This lncRNA is crucial for proper Th1 lineage development, as siRNA-mediated knockdown of linc-MAF-4 induced differentiation into the Th2 lineage. Mechanistically, the 5’ and 3’ region of linc-MAF-4 interacts through chromatin-looping with the promoter of MAF (a Th2-associated transcription factor). The authors go on to show that linc-MAF-4 represents a scaffold to recruit the chromatin modifiers EZH2 and LSD1, which induces repressive H3K27me3 epigenetic marks on the MAF promoter (105). This study beautifully demonstrates the essential Th1 lineage-determining function of linc-MAF-4.
CD4+ Th2 cells harbor a lineage-specific Th2-LCR lncRNA cluster, which consists of 4 alternatively spliced transcripts, and regulates the Th2 cytokine locus (IL-4, IL-5 and IL-13) (56, 113, 114). Interestingly, LCR-knockout studies in mice using the Cre-LoxP system revealed that the Th2-LCR lncRNA cluster downregulates IFN-γ (56, 113, 114), a cytokine that is crucial for promoting anti-tumor immune responses (118). Similar to the TH2-LCR lncRNA cluster, the two lncRNAs linc-Ccr2-5’ AS and GATA3-AS1 were shown to be essential for Th2-specific lineage commitment (101, 104). GATA3-AS1 is crucial for the transcription of IL-5, IL-13 and GATA3. Mechanistically, GATA3-AS1 establishes H3K4-methylation and H3K27-acetylation marks at the GATA3 locus during Th2 polarizing conditions by recruiting an MLL methyltransferase via WDR5 (101). GATA3 itself guides the expression of linc-Ccr2-5’ AS, which induces several downstream target genes including the chemokine receptor encoding genes Ccr2 and Ccr3, thereby modulating Th2 cell migration (104). It is intriguing to speculate whether the Th2-LCR lncRNA cluster (linc-Ccr2-5’ AS and GATA3-AS1) is part of the same Th2 regulatory circuit, and whether the TME induces the Th2-LCR lncRNA cluster to facilitate tumor immune evasion by promoting Th2 cell differentiation.
LncRNAs modulating CD4+ T cell subsets in a tumor context-dependent manner is a newly emerging field of research. So far, only few lncRNAs have been studied to determine their functional importance in tumor-infiltrating CD4+ T cell subsets (Table 2). However, lncRNA colorectal neoplasia differentially expressed (CRNDE) is highly expressed in multiple cancer types, and in CRC, lncRNA isoform CRNDE-h is transmitted via tumor-exosomes to induce Th17 differentiation in tumor-infiltrating CD4+ T cells. LncRNA CRNDE-h targets the PPXY motif of ROR-γt inhibiting Itch-mediated ubiquitination and degradation of ROR-γt, thereby inducing IL-17 expression (99). This highlights another mechanism of how lncRNAs from tumor cell-derived exosomes are able to modulate the tumor microenvironment to facilitate cancer progression.
Although these findings highlight the relevance of lncRNAs in governing CD4+ T cell differentiation into distinct T helper cell lineages, the exact role of these and other lncRNAs in shaping CD4+ T cell responses in the TME awaits further investigation.
2.3 Regulatory T cells
Treg cell development occurs in the thymus by self-antigen recognition with moderate avidity (thymic Tregs; tTregs) or alternatively, in specialized peripheral tissues (peripheral Tregs; pTregs). In the gut, pTregs develop from CD4+ T cells in the presence of TGF-β and retinoic acid (119, 120). The T cell receptor diversity distinguishes tTregs from pTregs. While tTregs primarily engage self-antigens, pTregs can also bind commensal microbiota antigens thereby providing mucosal tolerance (120). Tregs typically express the transcription factor Forkhead box protein 3 (FoxP3), the high affinity IL-2 receptor alpha (CD25) and low levels of IL-7Rα (CD127). However, also FoxP3-independent, IL-10 producing type 1 regulatory cells (Tr1) have been described. Tr1 cells originate extra-thymically, lack FoxP3 but express CD49b and lymphocyte activation gene 3 (LAG-3), produce IL-10 and TGF-β thereby maintaining immune homeostasis and preventing autoimmune diseases (121). Although a recent study discovered that the transcriptional program of pTregs is independent of FoxP3, FoxP3 is still crucial for a specific pTreg function, namely to suppress inflammatory Th17 cells (122). Another FoxP3-dependent subtype are tissue Tregs. They reside in non-lymphoid tissues and originate from a common FoxP3+ CD4+ precursor in lymphoid organs. Aside from immune surveillance, tissue Tregs regulate non-lymphoid progenitors and function during tissue homeostasis (123).
In cancer, Tregs dampen anti-tumor immune responses by secreting suppressive cytokines (TGF-β and IL-10), consuming excessive amounts of the T cell growth factor IL-2 (thereby impairing effector T cell proliferation), expressing inhibitory receptors (CTLA-4, PD-1 and LAG3) and inducing tolerogenic dendritic cells (98). Generally, intratumoral Tregs are associated with poor prognosis in patients, although differences have been observed between cancer types and studies (36). Treg cell depletion has shown promising results in preclinical cancer models, and anti-CTLA-4 therapy not only increases tumor-specific effector CD4+ T cells but also depletes intratumoral CTLA-4+ Tregs (98, 124).
Numerous lncRNAs have been shown to be expressed in Tregs or to regulate Treg cell differentiation are Flatr (125), Flicr (126), lnc-SMAD3 (127) and PVT1 (128) (Figure 1). Some of these lncRNAs may severe as novel targets for cancer immunotherapy as they have been shown to modulate the complex immunosuppressive TME (Table 3).
For example, lnc-epidermal growth factor receptor (lnc-EGFR) has been described to be highly expressed in Tregs in HCC (93). Mechanistically, lnc-EGFR interacts with EGFR and inhibits its ubiquitination by c-CBL and subsequent degradation while amplifying downstream signaling via AP-1/NFAT1. NFAT1 and AP-1 binding sites were identified in the promotor regions of Foxp3, lnc-EGFR and EGFR. NFAT1 and AP-1 signaling increased the expression of all three genes, indicating a feed forward loop of lnc-EGFR/EGFR/NFAT1/AP-1 in Tregs in HCC (Figure 3B) (93). The multi-kinase inhibitor sorafenib and the receptor tyrosine kinase inhibitor lenvatinib are currently being used as first-line treatments against HCC (134) and blocking EGFR has shown to further increase the efficacy of those two drugs (135, 136). Targeting lnc-EGFR and its signaling axis may therefore represent a promising strategy to improve the anti-tumor activities of sorafenib and lenvatinib (93). This model showcases how lnc-EGFR connects an immunosuppressive state to cancer by inducing Treg cell differentiation, suppressing cytotoxic T cells and promoting tumor immune evasion.
Another interesting lncRNA that indirectly modulates the suppressive function of Tregs in HCC is lncRNA fetal-lethal non-coding developmental regulatory RNA (FENDRR) (129). Microarray analysis revealed that lncRNA FENDRR is downregulated in HCC as compared to adjacent normal tissue. Mechanistically, overexpressed lncRNA FENDRR competitively binds miR-423-5p and upregulates “growth arrest and DNA-damage-inducible beta protein” (GADD45B). GADD45B has an anti-tumorigenic function and inversely correlates with Treg cell number. Consequently, its upregulation reduced the Treg cell-associated immunosuppressive cytokines TGF-β and IL-10, and induced apoptosis in tumor cells via Bax and caspase-3 (129). This mechanism nicely demonstrates how downregulation of lncRNA FENDRR in HCC enhances the immunosuppressive ability of Tregs to promote immune escape.
In pediatric acute lymphoblastic leukemia (ALL), lnc-insulin receptor precursor (lnc-INSR) was identified to play a crucial role in promoting suppressive immune cells. High throughput screening revealed an increased expression of lnc-INSR in Tregs in the bone marrow of pediatric ALL patients. A combination of FISH and RIP assays identified the cytoplasmic domain of INSR as the target of lnc-INSR. Consequently, INSR and its downstream signaling pathway PI3K/Akt is abnormally activated causing immune suppression and tumor growth via enhanced Treg cell differentiation and a reduction in cytotoxic T cells (131).
Additionally, lncRNA MIAT, SNHG1, lnc-POU3F3 and RP11-323N12.5 have been associated with Tregs in cancer. LncRNAs linc-POU3F3 and RP11-323N12.5 are both associated with gastric cancer (GC) (130, 132). Linc-POU3F3 was upregulated in peripheral blood CD4+ CD25+ FoxP3+ Tregs of GC patients and could recruit TGF-β to induce its downstream signaling pathway which triggered Treg proliferation (130). RP11-323N12.5 was highly expressed in human GC cells. Functionally, RP11-323N12.5 stimulates the expression of the transcription factor YAP1. YAP1 itself promotes lncRNA RP11-323N12.5 expression as part of a positive feedback loop. RP11-323N12.5 was also detected in tumor-infiltrating lymphocytes such as Tregs, and enhanced Treg cell differentiation thereby shifting the balance towards an immunosuppressive TME (132). Another lncRNA, lncRNA SNHG1 was identified in breast cancer-infiltrating CD4+ T cells. LncRNA SNHG1 bound miR-448, which decreased IDO expression and increased FoxP3 and IL-10 levels in Tregs. SiRNA-mediated knockdown of SNHG1 decreased tumor progression indicating that SNHG1 promotes tumor immune escape by stimulating Treg cell differentiation (133). In HCC, lncRNA MIAT expression was upregulated in tumor cells, FoxP3+ Tregs, PD-1+ CD8+ T cells and GZMK+ CD8+ T cells (75). MIAT expression correlated with the responsiveness to anticancer drugs, such as sorafenib, and with the expression of PD-L1. However, additional functional analyses are needed to reveal the molecular mechanism and the role of MIAT in tumor cells and different immune cell types. Additionally, two lncRNAs, Flatr and Flicr both modulate FoxP3 expression on Tregs. While Flatr is part of the upstream signaling cascade triggering FoxP3 expression (125), Flicr functions as a negative regulator modulating FoxP3 expression under IL-2 deficient conditions. Thereby, Flicr limits Treg activity promoting autoimmunity (126). Consequently, this mechanism could be exploited for Treg-cell therapy to reduce immunosuppression by Tregs in cancer.
Another lncRNA that affects the Treg/CD8+ T cell balance in the TME is lnc-Sox5. In colorectal cancer (CRC), lnc-Sox5 induces indoleamine 2,3-dioxygenase 1 (IDO1) expression, which is known to promote Treg cell differentiation. Conversely, the inhibition of lnc-Sox5 limits Treg cell differentiation thereby reducing tumorigenesis (88). Similarly, LINC00301 induces an immunosuppressive tumor microenvironment by modulating the CD8+ T cell/Tregs ratio in non-small cell lung cancer (NSCLC) (137). Interestingly, this lncRNA regulates Tregs by targeting hypoxia-inducible factor 1α (HIF-1α) in two different ways: nuclear LINC00301 binds to EZH2, which downregulates the EAF2 promoter and increases HIF-1α expression, whereas cytoplasmic LINC00301 targets HIF-1α via miR-1276.
Altogether, these studies demonstrate the relevance of lncRNAs in Treg cell differentiation and in establishing an immunosuppressive TME to promote tumor growth and tumor immune evasion. Several lncRNAs have been linked to the complex interplay between Tregs and the TME, and may therefore represent interesting therapeutic targets.
2.4 γδ T cells and NKT cells
In humans, two major subsets of γδ T cells have been identified. Vδ1 T cells are found in the thymus and peripheral tissues, and recognize stress-related antigens. And Vδ2 T cells are found in the circulation and mostly recognize phospho-antigens. One feature that distinguishes γδ T cells from conventional αβ T cells is their ability to identify tumor cells regardless of human leukocyte antigen (HLA) restriction. In a number of cancer entities, a considerable part of the tumor-infiltrating lymphocyte population consists of γδ T cells (138). The abundance of γδ T cells, in particular Vδ1 T cells, has been associated with favorable prognosis in some cancers, such as non-small cell lung cancer (NSCLC) (139), triple-negative breast cancer (TNBC) (140) and ovarian cancer (141). However, tumor-infiltrating γδ T cells can also differentiate into pro-tumorigenic IL-17+ γδ T cells or immunosuppressive γδ T regulatory cells (138) thereby being associated with poor patient prognosis. A recent study indicates that TCR-Vγδ usage distinguishes pro-tumor from anti-tumor intestinal γδ T cell subsets (142). Due to their better functional characterization and their frequent abundance in numerous cancer types, γδ T cells are recognized as a promising cellular target for cancer immunotherapy (143), and lncRNAs could act as molecular targets to specifically modulate γδ T cell function in the TME (Table 1).
In a study by Ni et al. (82), the exosomal lncRNA SNHG16 was identified in γδ T cells of breast cancer (BC) patients. Taking advantage of co-culture experiments, microarray and mechanistic analyses, the authors demonstrated that breast tumor cell-derived exosomes can transfer lncRNA SNHG16 to γδ T cells. The SNHG16/miR-16-5p/TGF-β1/SMAD5 pathway increased CD73 expression in γδ T cells, thereby inducing an immunosuppressive CD73+ Vδ1 T cell subpopulation (82). This work nicely exemplifies exosomal transmission of a lncRNA from a tumor cell to γδ T cells, which promotes a regulatory CD73+ Vδ1 T cell subset and thus facilitates tumor immune evasion.
LncRNA TANCR is another recently identified human γδ T cell-specific lncRNA. Deep RNA sequencing, led to the discovery of TANCR in isopentenyl pyrophosphate (IPP)-activated γδ T cells generated from human PBMCs. This study indicates that TANCR regulates γδ T cell activation and modulates tumor necrosis factor-related apoptosis-inducing ligand (TRAIL) expression (83). Thereby, TANCR may induce apoptosis of target cells, which could be utilized for therapeutic purposes. However, additional functional studies will be required to identify the molecular mechanism of TANCR and its regulation in the TME.
NKT cells are another T cell subpopulation that participates in the immune surveillance of tumors. In cancer patients, exogenous NKT cell activation triggers a considerable immune response and NKT cell infiltration into tumors correlates with a favorable prognosis in a variety of cancer types (144). Currently, only one lncRNA has been identified that modulates NKT cell function in cancer. In a recent study, the intergenic lncRNA LINC00240 was found to be increased in cervical cancer. Mechanistic analyses demonstrated that LIN00240 promotes cancer progression by sponging miR-124-3p and activating STAT3, which caused NKT cell tolerance (70).
3 The impact of tumor cell-expressed lncRNAs on T cell responses
LncRNAs have been shown to be expressed in all major cancer types, contribute to the hallmarks of cancer, and can act as oncogenes (“onco-lncRNAs”, such as HOTAIR, NEAT1 and MALAT1) or tumor suppressors (e.g. GAS5, MEG3, NBAT and LINC-PINT) (67, 145–147). Numerous lncRNAs have been shown to regulate tumorigenesis, epithelial-mesenchymal transition and metastasis, and some of those lncRNAs may prove useful for risk stratification of cancer patients or may be included in the molecular classification of cancer subtypes (63, 65). In this section, we will highlight tumor cell-expressed lncRNAs that drive immune escape by modulating T cell infiltration or T cell dysfunction/exhaustion (Table 4) and that may therefore represent promising targets for therapeutic intervention.
Using whole-transcriptome analysis, lncRNA IFN-stimulated non-coding RNA 1 (INCR1) was identified as a crucial modulator of IFN-γ signaling in multiple tumors (89). INCR1 confers its function by post-transcriptionally regulating PD-L1 and JAK2 activity. Mechanistically, the nuclear riboprotein HNRNPH1 binds and downregulates PD-L1 and JAK2. However, INCR1 can competitively bind HNRNPH1, which enables PD-L1 and JAK2 expression. Knockdown of INCR1 repressed IFN-γ-stimulated genes (ISGs) and increased T cell-mediated killing of tumor cells. Intriguingly, in vivo silencing of INCR1 improved CAR T cell therapy responses (89).
While INCR1 serves as an inhibitory target to promote IFN-γ response and T cell activity, another cancer immunogenic lncRNA, lncRNA inducing MHC-I and immunogenicity of tumor (LIMIT), has been identified recently (150). The IFN-γ-induced lncRNA LIMIT stimulates the guanylate-binding protein (GBP) gene cluster in cis. Consequently, the HSP90 and heat shock factor-1 (HSF1) complex dissociate, activating HSF1 and increasing MHC-I expression but not PD-L1. Li et al. also performed RNA-guided CRISPR activation of LIMIT, which boosted GBPs and MHC-I, and potentiated tumor immunogenicity and anti-PD-L1 antibody therapy. Remarkably, the authors performed CRISPR activation of LIMIT in a B16 melanoma model, which characteristically lacks responsiveness to PD-L1 blockade, and demonstrated resensitization of the tumor to anti-PD-L1 therapy. Since the loss of MHC-I and IFN-γ is commonly observed in cancer (150), lncRNA LIMIT provides a novel therapeutic opportunity to increase MHC I on tumors and to resensitize cancers to anti-PD-L1 therapy.
Another lncRNA that may act as a target for immunotherapy is lncRNA EPIC1 (Figure 3C). For the identification of lncRNA EPIC1, Guo et al. employed an interesting new approach to dissect the landscape of interactions between lncRNAs and tumor immunity (92). Based on bulk tumor RNA-seq data collected in The Cancer Genome Atlas (TCGA) database comprising 32 cancer types and more than 9000 tumor samples, they performed an integrative analysis of lncRNA expression and immunogenomics profiles of the TME. This led to the development of a lncRNA-based immune response (LIMER) score which, predicts lymphocyte infiltration of tumors and patient prognosis. Tumor tissue-specific lncRNA EPIC1 correlated with lower infiltration and activation of CD8+ T cells as well as decreased tumor antigen presentation in multiple cancers. Mechanistically, lncRNA EPIC1 interacts with EZH2, a histone methyltransferase that epigenetically silences IFNGR1 and consequently IFN-γ signaling (92). Thereby, the authors propose a novel and distinct therapeutic target for immunotherapy across multiple cancer types.
The lncRNA LINK-A (LINC01139) acts as an oncogene by downregulating cancer cell antigen presentation as well as the tumor suppressors p53 and Rb (151). LINK-A is upregulated in multiple cancer types, including triple-negative breast cancer, and high LINK-A expression correlates with low CD8+ T cell abundance in basal-like breast cancer. Mechanistically, LINK-A binds to phosphatidylinositol-(3,4,5)-triphosphate, suppressing protein kinase A-mediated phosphorylation of the E3 ubiquitin ligase TRIM71. As a consequence, LINK-A enhances K48-polyubiquitination-mediated degradation of the antigen peptide-loading complex as well as p53 and Rb. Using a mouse model, treatment with LINK-A locked nucleic acids (LNAs) sensitized breast tumors to immune checkpoint blockade therapy (anti-PD-1 + anti-CTLA-4) thus providing a basis for a rational combination immunotherapy regimen (151).
Apart from tumor-expressed lncRNAs that impair effector CD8+ T cells (88, 89, 92, 137, 150), several lncRNAs have been described that induce an immunosuppressive TME by altering tumor-infiltrating CD4+ T cells (137, 159), Tregs (88, 129, 137, 159, 160), γδ T cells (82, 83) and NKT cells (70). For example, lncRNA NNT-AS1 impairs TGF-β signaling and reduces CD4+ T cell tumor infiltration, and thereby promotes HCC progression and metastasis (159, 164). Another lncRNA, HOXA-AS2 has been shown to contribute to Treg cell proliferation and immune tolerance in glioma through the miR-302a/KDM2A/JAG1 axis (160).
The above-mentioned studies demonstrate how tumor cell-expressed lncRNAs promote an immunosuppressive TME by modulating MHC I expression or several signaling pathways, such as IFN-γ and TGF-β. As IFN-γ signaling is an integral part of anti-tumor immunity, these lncRNAs, in particular lncRNA EPIC1 due to its pan-cancer applicability, may represent a promising target for therapeutic intervention.
4 Challenges for lncRNA-based therapeutics
Several siRNAs that target mRNA transcripts are currently in clinical use or development (8, 165). Although no lncRNA-based therapeutics have entered the clinic yet, there are several strategies to inhibit lncRNAs, depending on their mode of action. Second-generation antisense oligonucleotides (ASOs), which target pre-mRNA splicing, as well as third-generation LNA oligonucleotides represent promising therapeutics to inhibit lncRNAs. In general, small-molecule inhibitors have two modes of action to block lncRNA function: Interaction element blockers (IEBs), that block RNA/DNA/protein-docking sites on lncRNAs, and structural element lockers (SELs), which affect the conformation of a lncRNA thereby inhibiting functional interactions with interactor molecules (8). One example of an IEB is NP-C86 that stabilizes lncRNA GAS5 by preventing its interaction with UPF1, which normally mediates nonsense-mediated decay of GAS5 (166). NP-C86 may thus prevent the decay of the tumor suppressor GAS5 and inhibit Th17 cell differentiation (Figure 1) (107). There are also SELs being developed for MALAT1, which aim to destabilize and down-regulate MALAT1, albeit their efficacy and specificity still need to be evaluated (167). MALAT1 also contributes to enzalutamide resistance in castration-resistant prostate cancer, and a MALAT1 siRNA reduced growth of enzalutamide-resistant tumor xenografts (168). Despite the frequently reported pro-tumorigenic and metastasis-promoting role of MALAT1 (169), a study by Kim et al. found that MALAT1 can also act as a tumor suppressor in a mouse model of breast cancer (170). Since MALAT1 is expressed in various cell types, and affects the function of CD8+ and CD4+ T cells (74, 171), it remains to be seen whether SELs or siRNAs that target MALAT1 are effective in inhibiting the growth of different tumor types. In summary, some progress has been made to advance therapeutics to target noncoding RNAs. However, there are still some important challenges ahead, such as improving specificity and delivery as well as reducing immunogenicity and toxicity of lncRNA-based therapeutics. A detailed description of RNA therapeutics that are in clinical development or that have been approved as well as the challenges that remain for the development of RNA-based therapeutics has been summarized in an excellent perspectives article by Winkle and colleagues (8).
5 Conclusions
LncRNAs have been recognized for their unique ability to regulate gene expression, nuclear architecture and cellular function. LncRNAs have been identified as critical regulators of T cell-mediated immunity during homeostasis, infection and cancer, highlighting the use of lncRNAs as potential biomarkers and/or more precise therapeutic targets. Advances in CRISPR-Cas genome editing have enabled high throughput functional screens and accelerated the generation of knockout and transgene mouse models to properly address the functional significance of lncRNAs (145, 172). This will facilitate uncovering the complex role of lncRNAs in shaping an immunosuppressive tumor microenvironment or in contributing to metastasis and immunotherapy resistance. Combining different technologies will be of great importance in order to investigate whether a lncRNA locus has multiple functional modalities, such as mediating its function through the RNA molecule itself, DNA elements encoded in the locus or via transcription (173). A lncRNA may have many molecular interactions and multiple context-specific and/or cell-type specific functions. Additionally, large lncRNA loci may overlap with other regulatory elements and may therefore generate multiple different RNAs or encode multiple different DNA elements. PVT1 and MALAT1 are examples of two lncRNA loci that have multiple and/or opposing effects (63). In conclusion, lncRNAs have been shown to be key regulators of T cell-mediated responses, and the recent technological advances will prove useful to provide definitive evidence for the functional relevance of lncRNAs in different cell types and context-specific environments. However, major challenges remain before transferring research results to the clinic, such as improving specificity and delivery as well as reducing immunogenicity and toxicity of lncRNA-targeting therapeutics.
Author contributions
Conceptualization: DH-B. Writing - original draft: JE. Writing - review and editing: DH-B. Funding acquisition: DH-B. All authors read and approved the submitted version.
Funding
This work was supported by the Austrian Science Fund (FWF; P33340-B to DH-B).
Acknowledgments
Figures were created with BioRender.com.
Conflict of interest
The authors declare that the research was conducted in the absence of any commercial or financial relationships that could be construed as a potential conflict of interest.
Publisher’s note
All claims expressed in this article are solely those of the authors and do not necessarily represent those of their affiliated organizations, or those of the publisher, the editors and the reviewers. Any product that may be evaluated in this article, or claim that may be made by its manufacturer, is not guaranteed or endorsed by the publisher.
References
1. Dunham I, Kundaje A, Aldred SF, Collins PJ, Davis CA, Doyle F, et al. An integrated encyclopedia of DNA elements in the human genome. Nature (2012) 489(7414):57–74. doi: 10.1038/nature11247
2. Hon CC, Ramilowski JA, Harshbarger J, Bertin N, Rackham OJL, Gough J, et al. An atlas of human long non-coding RNAs with accurate 5′ ends. Nature (2017) 543(7644):199–204. doi: 10.1038/nature21374
3. Moore JE, Purcaro MJ, Pratt HE, Epstein CB, Shoresh N, Adrian J, et al. Expanded encyclopaedias of DNA elements in the human and mouse genomes. Nature (2020) 583(7818):699–710. doi: 10.1038/s41586-020-2493-4
4. Liu L, Wang Q, Qiu Z, Kang Y, Liu J, Ning S, et al. Noncoding RNAs: the shot callers in tumor immune escape. Signal Transduct Target Ther (2020) 5(1):102. doi: 10.1038/s41392-020-0194-y
5. Slack FJ, Chinnaiyan AM. The role of non-coding RNAs in oncology. Cell (2019) 179(5):1033–55. doi: 10.1016/j.cell.2019.10.017
6. Kopp F, Mendell JT. Functional classification and experimental dissection of long noncoding RNAs. Cell (2018) 172(3):393–407. doi: 10.1016/j.cell.2018.01.011
7. Adams D, Gonzalez-Duarte A, O'Riordan WD, Yang CC, Ueda M, Kristen AV, et al. Patisiran, an RNAi therapeutic, for hereditary transthyretin amyloidosis. N Engl J Med (2018) 379(1):11–21. doi: 10.1056/NEJMoa1716153
8. Winkle M, El-Daly SM, Fabbri M, Calin GA. Noncoding RNA therapeutics {{/amp]]mdash; challenges and potential solutions. Nat Rev Drug Discov (2021) 20(8):629–51. doi: 10.1038/s41573-021-00219-z
9. Chen YG, Satpathy AT, Chang HY. Gene regulation in the immune system by long noncoding RNAs. Nat Immunol (2017) 18(9):962–72. doi: 10.1038/ni.3771
11. Heidari R, Akbariqomi M, Asgari Y, Ebrahimi D, Alinejad-Rokny H. A systematic review of long non-coding RNAs with a potential role in breast cancer. Mutat Res Rev Mutat Res (2021) 787:108375–. doi: 10.1016/j.mrrev.2021.108375
12. Statello L, Guo C-J, Chen L-L, Huarte M. Gene regulation by long non-coding RNAs and its biological functions. Nat Rev Mol Cell Biol (2021) 22(2):96–118. doi: 10.1038/s41580-020-00315-9
13. Kristensen LS, Jakobsen T, Hager H, Kjems J. The emerging roles of circRNAs in cancer and oncology. Nat Rev Clin Oncol (2022) 19(3):188–206. doi: 10.1038/s41571-021-00585-y
14. Zhao Y, Li H, Fang S, Kang Y, Wu W, Hao Y, et al. NONCODE 2016: an informative and valuable data source of long non-coding RNAs. Nucleic Acids Res (2016) 44(D1):D203–D8. doi: 10.1093/NAR/GKV1252
15. Quinn JJ, Chang HY. Unique features of long non-coding RNA biogenesis and function. Nat Rev Genet (2015) 17(1):47–62. doi: 10.1038/nrg.2015.10
16. Uszczynska-Ratajczak B, Lagarde J, Frankish A, Guigó R, Johnson R. Towards a complete map of the human long non-coding RNA transcriptome. Nat Rev Genet (2018) 19(9):535–48. doi: 10.1038/s41576-018-0017-y
17. Hezroni H, Koppstein D, Schwartz Matthew G, Avrutin A, Bartel David P, Ulitsky I. Principles of long noncoding RNA evolution derived from direct comparison of transcriptomes in 17 species. Cell Rep (2015) 11(7):1110–22. doi: 10.1016/j.celrep.2015.04.023
18. Sarropoulos I, Marin R, Cardoso-Moreira M, Kaessmann H. Developmental dynamics of lncRNAs across mammalian organs and species. Nature (2019) 571(7766):510–4. doi: 10.1038/s41586-019-1341-x
19. Amaral PP, Leonardi T, Han N, Viré E, Gascoigne DK, Arias-Carrasco R, et al. Genomic positional conservation identifies topological anchor point RNAs linked to developmental loci. Genome Biol (2018) 19(1):32–. doi: 10.1186/s13059-018-1405-5
20. Guo C-J, Ma X-K, Xing Y-H, Zheng C-C, Xu Y-F, Shan L, et al. Distinct processing of lncRNAs contributes to non-conserved functions in stem cells. Cell (2020) 181(3):621–36.e22. doi: 10.1016/j.cell.2020.03.006
21. Johnsson P, Ziegenhain C, Hartmanis L, Hendriks G-J, Hagemann-Jensen M, Reinius B, et al. Transcriptional kinetics and molecular functions of long noncoding RNAs. Nat Genet (2022) 54(3):306–17. doi: 10.1038/s41588-022-01014-1
22. Wang KC, Yang YW, Liu B, Sanyal A, Corces-Zimmerman R, Chen Y, et al. A long noncoding RNA maintains active chromatin to coordinate homeotic gene expression. Nature (2011) 472(7341):120–4. doi: 10.1038/nature09819
23. Luo H, Zhu G, Xu J, Lai Q, Yan B, Guo Y, et al. HOTTIP lncRNA promotes hematopoietic stem cell self-renewal leading to AML-like disease in mice. Cancer Cell (2019) 36(6):645–59 e8. doi: 10.1016/j.ccell.2019.10.011
24. Hu WL, Jin L, Xu A, Wang YF, Thorne RF, Zhang XD, et al. GUARDIN is a p53-responsive long non-coding RNA that is essential for genomic stability. Nat Cell Biol (2018) 20(4):492–502. doi: 10.1038/s41556-018-0066-7
25. Munschauer M, Nguyen CT, Sirokman K, Hartigan CR, Hogstrom L, Engreitz JM, et al. The NORAD lncRNA assembles a topoisomerase complex critical for genome stability. Nature (2018) 561(7721):132–6. doi: 10.1038/s41586-018-0453-z
26. Jeon Y, Lee JT. YY1 tethers xist RNA to the inactive X nucleation center. Cell (2011) 146(1):119–33. doi: 10.1016/j.cell.2011.06.026
27. Hacisuleyman E, Goff LA, Trapnell C, Williams A, Henao-Mejia J, Sun L, et al. Topological organization of multichromosomal regions by the long intergenic noncoding RNA firre. Nat Struct Mol Biol (2014) 21(2):198–206. doi: 10.1038/nsmb.2764
28. Huang D, Chen J, Yang L, Ouyang Q, Li J, Lao L, et al. NKILA lncRNA promotes tumor immune evasion by sensitizing T cells to activation-induced cell death. Nat Immunol (2018) 19(10):1112–25. doi: 10.1038/s41590-018-0207-y
29. Brown CJ, Ballabio A, Rupert JL, Lafreniere RG, Grompe M, Tonlorenzi R, et al. A gene from the region of the human X inactivation centre is expressed exclusively from the inactive X chromosome. Nature (1991) 349(6304):38–44. doi: 10.1038/349038a0
30. Brannan CI, Dees Ec Fau - Ingram RS, Ingram Rs Fau - Tilghman SM, Tilghman SM. The product of the H19 gene may function as an RNA. Mol Cell Biol (1990) 10(1)(0270-7306(1):28–36. doi: 10.1128/mcb.10.1.28-36.1990
31. Kaech SM, Cui W. Transcriptional control of effector and memory CD8+ T cell differentiation. Nat Rev Immunol (2012) 12(11):749–61. doi: 10.1038/nri3307
32. Henning AN, Roychoudhuri R, Restifo NP. Epigenetic control of CD8(+) T cell differentiation. Nat Rev Immunol (2018) 18(5):340–56. doi: 10.1038/nri.2017.146
33. Herndler-Brandstetter D, Ishigame H, Shinnakasu R, Plajer V, Stecher C, Zhao J, et al. KLRG1(+) effector CD8(+) T cells lose KLRG1, differentiate into all memory T cell lineages, and convey enhanced protective immunity. Immunity (2018) 48(4):716–29 e8. doi: 10.1016/j.immuni.2018.03.015
34. Wilson CB, Rowell E, Sekimata M. Epigenetic control of T-helper-cell differentiation. Nat Rev Immunol (2009) 9(2):91–105. doi: 10.1038/nri2487
35. Borst J, Ahrends T, Babala N, Melief CJM, Kastenmuller W. CD4(+) T cell help in cancer immunology and immunotherapy. Nat Rev Immunol (2018) 18(10):635–47. doi: 10.1038/s41577-018-0044-0
36. Bruni D, Angell HK, Galon J. The immune contexture and immunoscore in cancer prognosis and therapeutic efficacy. Nat Rev Cancer (2020) 20(11):662–80. doi: 10.1038/s41568-020-0285-7
37. Wherry EJ, Kurachi M. Molecular and cellular insights into T cell exhaustion. Nat Rev Immunol (2015) 15(8):486–99. doi: 10.1038/nri3862
38. Scott AC, Dundar F, Zumbo P, Chandran SS, Klebanoff CA, Shakiba M, et al. TOX is a critical regulator of tumour-specific T cell differentiation. Nature (2019) 571(7764):270–4. doi: 10.1038/s41586-019-1324-y
39. Zander R, Schauder D, Xin G, Nguyen C, Wu X, Zajac A, et al. CD4+ T cell help is required for the formation of a cytolytic CD8+ T cell subset that protects against chronic infection and cancer. Immunity (2019) 51(6):1028–42.e4. doi: 10.1016/j.immuni.2019.10.009
40. McLane LM, Abdel-Hakeem MS, Wherry EJ. CD8 T cell exhaustion during chronic viral infection and cancer. Annu Rev Immunol (2019) 37(1):457–95. doi: 10.1146/annurev-immunol-041015-055318
41. Philip M, Schietinger A. CD8+ T cell differentiation and dysfunction in cancer. Nat Rev Immunol (2022), 22(4):209–23. doi: 10.1038/s41577-021-00574-3
42. Paijens ST, Vledder A, de Bruyn M, Nijman HW. Tumor-infiltrating lymphocytes in the immunotherapy era. Cell Mol Immunol (2020) 18(4):842–59. doi: 10.1038/s41423-020-00565-9
43. Philip M, Fairchild L, Sun L, Horste EL, Camara S, Shakiba M, et al. Chromatin states define tumour-specific T cell dysfunction and reprogramming. Nature (2017) 545(7655):452–6. doi: 10.1038/nature22367
44. Miller BC, Sen DR, Al Abosy R, Bi K, Virkud YV, LaFleur MW, et al. Subsets of exhausted CD8+ T cells differentially mediate tumor control and respond to checkpoint blockade. Nat Immunol (2019) 20(3):326–36. doi: 10.1038/s41590-019-0312-6
45. Paley Michael A, Kroy Daniela C, Odorizzi Pamela M, Johnnidis Jonathan B, Dolfi Douglas V, Barnett Burton E, et al. Progenitor and terminal subsets of CD8+ T cells cooperate to contain chronic viral infection. Science (2012) 338(6111):1220–5. doi: 10.1126/science.1229620
46. Hudson WH, Gensheimer J, Hashimoto M, Wieland A, Valanparambil RM, Li P, et al. Proliferating transitory T cells with an effector-like transcriptional signature emerge from PD-1+ stem-like CD8+ T cells during chronic infection. Immunity (2019) 51(6):1043–58.e4. doi: 10.1016/j.immuni.2019.11.002
47. Duhen T, Duhen R, Montler R, Moses J, Moudgil T, de Miranda NF, et al. Co-Expression of CD39 and CD103 identifies tumor-reactive CD8 T cells in human solid tumors. Nat Commun (2018) 9(1):2724. doi: 10.1038/s41467-018-05072-0
48. Blackburn SD, Shin H, Haining WN, Zou T, Workman CJ, Polley A, et al. Coregulation of CD8+ T cell exhaustion by multiple inhibitory receptors during chronic viral infection. Nat Immunol (2009) 10(1):29–37. doi: 10.1038/ni.1679
49. Alfei F, Kanev K, Hofmann M, Wu M, Ghoneim HE, Roelli P, et al. TOX reinforces the phenotype and longevity of exhausted T cells in chronic viral infection. Nature (2019) 571(7764):265–9. doi: 10.1038/s41586-019-1326-9
50. Khan O, Giles JR, McDonald S, Manne S, Ngiow SF, Patel KP, et al. TOX transcriptionally and epigenetically programs CD8+ T cell exhaustion. Nature (2019) 571(7764):211–8. doi: 10.1038/s41586-019-1325-x
51. Yao C, Sun H-W, Lacey NE, Ji Y, Moseman EA, Shih H-Y, et al. Single-cell RNA-seq reveals TOX as a key regulator of CD8+ T cell persistence in chronic infection. Nat Immunol (2019) 20(7):890–901. doi: 10.1038/s41590-019-0403-4
52. Utzschneider DT, Charmoy M, Chennupati V, Pousse L, Ferreira DP, Calderon-Copete S, et al. T Cell factor 1-expressing memory-like CD8+ T cells sustain the immune response to chronic viral infections. Immunity (2016) 45(2):415–27. doi: 10.1016/j.immuni.2016.07.021
53. Im SJ, Hashimoto M, Gerner MY, Lee J, Kissick HT, Burger MC, et al. Defining CD8+ T cells that provide the proliferative burst after PD-1 therapy. Nature (2016) 537(7620):417–21. doi: 10.1038/nature19330
54. Beltra J-C, Manne S, Abdel-Hakeem MS, Kurachi M, Giles JR, Chen Z, et al. Developmental relationships of four exhausted CD8+ T cell subsets reveals underlying transcriptional and epigenetic landscape control mechanisms. Immunity (2020) 52(5):825–41.e8. doi: 10.1016/j.immuni.2020.04.014
55. Weisshaar N, Wu J, Ming Y, Madi A, Hotz-Wagenblatt A, Ma S, et al. Rgs16 promotes antitumor CD8+ T cell exhaustion. Sci Immunol (2022) 7(71):eabh1873. doi: 10.1126/sciimmunol.abh1873
56. Spurlock CF 3rd, Tossberg JT, Guo Y, Collier SP, Crooke PS 3rd, Aune TM. Expression and functions of long noncoding RNAs during human T helper cell differentiation. Nat Commun (2015) 6:6932. doi: 10.1038/ncomms7932
57. Hudson WH, Prokhnevska N, Gensheimer J, Akondy R, McGuire DJ, Ahmed R, et al. Expression of novel long noncoding RNAs defines virus-specific effector and memory CD8(+) T cells. Nat Commun (2019) 10(1):196. doi: 10.1038/s41467-018-07956-7
58. Kanduri K, Tripathi S, Larjo A, Mannerstrom H, Ullah U, Lund R, et al. Identification of global regulators of T-helper cell lineage specification. Genome Med (2015) 7:122. doi: 10.1186/s13073-015-0237-0
59. Tuomela S, Rautio S, Ahlfors H, Oling V, Salo V, Ullah U, et al. Comparative analysis of human and mouse transcriptomes of Th17 cell priming. Oncotarget (2016) 7(12):13416–28. doi: 10.18632/oncotarget.7963
60. Ubaid U, Andrabi SBA, Tripathi SK, Dirasantha O, Kanduri K, Rautio S, et al. Transcriptional repressor HIC1 contributes to suppressive function of human induced regulatory T cells. Cell Rep (2018) 22(8):2094–106. doi: 10.1016/j.celrep.2018.01.070
61. Hrdlickova B, Kumar V, Kanduri K, Zhernakova DV, Tripathi S, Karjalainen J, et al. Expression profiles of long non-coding RNAs located in autoimmune disease-associated regions reveal immune cell-type specificity. Genome Med (2014) 6(10):88. doi: 10.1186/s13073-014-0088-0
62. Luo H, Bu D, Shao L, Li Y, Sun L, Wang C, et al. Single-cell long non-coding RNA landscape of T cells in human cancer immunity. Genomics Proteomics Bioinf (2021) 19(3):377–93. doi: 10.1016/j.gpb.2021.02.006
63. Goodall GJ, Wickramasinghe VO. RNA In cancer. Nat Rev Cancer (2021) 21(1):22–36. doi: 10.1038/s41568-020-00306-0
64. Uddin MN, Wang X. The landscape of long non-coding RNAs in tumor stroma. Life Sci (2021) 264:118725. doi: 10.1016/j.lfs.2020.118725
65. Liu SJ, Dang HX, Lim DA, Feng FY, Maher CA. Long noncoding RNAs in cancer metastasis. Nat Rev Cancer (2021) 21(7):446–60. doi: 10.1038/s41568-021-00353-1
66. Dietlein F, Wang AB, Fagre C, Tang A, Besselink NJM, Cuppen E, et al. Genome-wide analysis of somatic noncoding mutation patterns in cancer. Science (2022) 376(6589):eabg5601. doi: 10.1126/science.abg5601
67. Huarte M. The emerging role of lncRNAs in cancer. Nat Med (2015) 21(11):1253–61. doi: 10.1038/nm.3981
68. Yan X, Hu Z, Feng Y, Hu X, Yuan J, Zhao SD, et al. Comprehensive genomic characterization of long non-coding RNAs across human cancers. Cancer Cell (2015) 28(4):529–40. doi: 10.1016/j.ccell.2015.09.006
69. Wang X, Shen H, He Q, Tian W, Xia A, Lu X-J. Exosomes derived from exhausted CD8+ T cells impaired the anticancer function of normal CD8+ T cells. J Med Genet (2019) 56(1):29 LP–31. doi: 10.1136/jmedgenet-2018-105439
70. Zhang Y, Li X, Zhang J, Liang H. Natural killer T cell cytotoxic activity in cervical cancer is facilitated by the LINC00240/microRNA-124-3p/STAT3/MICA axis. Cancer Lett (2020) 474:63–73. doi: 10.1016/J.CANLET.2019.12.038
71. Cheng S, Li F, Qin H, Ping Y, Zhao Q, Gao Q, et al. Long noncoding RNA lncNDEPD1 regulates PD-1 expression via miR-3619-5p in CD8(+) T cells. J Immunol (2022) 208(6):1483–92. doi: 10.4049/jimmunol.2100602
72. Wang Y, Zhong H, Xie X, Chen CY, Huang D, Shen L, et al. Long noncoding RNA derived from CD244 signaling epigenetically controls CD8+ T-cell immune responses in tuberculosis infection. Proc Natl Acad Sci U.S.A. (2015) 112(29):E3883–92. doi: 10.1073/pnas.1501662112
73. Ji J, Yin Y, Ju H, Xu X, Liu W, Fu Q, et al. Long non-coding RNA lnc-Tim3 exacerbates CD8 T cell exhaustion via binding to Tim-3 and inducing nuclear translocation of Bat3 in HCC. Cell Death Dis (2018) 9(5):478–. doi: 10.1038/s41419-018-0528-7
74. Kanbar JN, Ma S, Kim ES, Kurd NS, Tsai MS, Tysl T, et al. The long noncoding RNA Malat1 regulates CD8+ T cell differentiation by mediating epigenetic repression. J Exp Med (2022) 219(6):e20211756. doi: 10.1084/jem.20211756
75. Peng L, Chen Y, Ou Q, Wang X, Tang N. LncRNA MIAT correlates with immune infiltrates and drug reactions in hepatocellular carcinoma. Int Immunopharmacol (2020) 89:107071–. doi: 10.1016/J.INTIMP.2020.107071
76. Yan K, Fu Y, Zhu N, Wang Z, J-l H, Li Y, et al. Repression of lncRNA NEAT1 enhances the antitumor activity of CD8+T cells against hepatocellular carcinoma via regulating miR-155/Tim-3. Int J Biochem Cell Biol (2019) 110:1–8. doi: 10.1016/j.biocel.2019.01.019
77. Gomez JA, Wapinski OL, Yang YW, Bureau JF, Gopinath S, Monack DM, et al. The NeST long ncRNA controls microbial susceptibility and epigenetic activation of the interferon-gamma locus. Cell (2013) 152(4):743–54. doi: 10.1016/j.cell.2013.01.015
78. Collier SP, Collins PL, Williams CL, Boothby MR, Aune TM. Cutting edge: influence of Tmevpg1, a long intergenic noncoding RNA, on the expression of ifng by Th1 cells. J Immunol (2012) 189(5):2084–8. doi: 10.4049/jimmunol.1200774
79. Li J, Chen C, Ma X, Geng G, Liu B, Zhang Y, et al. Long noncoding RNA NRON contributes to HIV-1 latency by specifically inducing tat protein degradation. Nat Commun (2016) 7(1):11730. doi: 10.1038/ncomms11730
80. Willingham AT, Orth AP, Batalov S, Peters EC, Wen BG, Aza-Blanc P, et al. A strategy for probing the function of noncoding RNAs finds a repressor of NFAT. Science (2005) 309(5740):1570–3. doi: 10.1126/science.1115901
81. Sharma S, Findlay GM, Bandukwala HS, Oberdoerffer S, Baust B, Li Z, et al. Dephosphorylation of the nuclear factor of activated T cells (NFAT) transcription factor is regulated by an RNA-protein scaffold complex. Proc Natl Acad Sci USA (2011) 108(28):11381–6. doi: 10.1073/pnas.1019711108
82. Ni C, Fang QQ, Chen WZ, Jiang JX, Jiang Z, Ye J, et al. Breast cancer-derived exosomes transmit lncRNA SNHG16 to induce CD73+γδ1 treg cells. Signal Transduct Targeted Ther (2020) 5(1):1–14. doi: 10.1038/s41392-020-0129-7
83. Yang C, Feng T, Lin F, Gong T, Yang S, Tao Y, et al. Long noncoding RNA TANCR promotes γδ T cells activation by regulating TRAIL expression in cis. Cell Biosci (2020) 10(1):15. doi: 10.1186/s13578-020-00383-6
84. Kotzin JJ, Iseka F, Wright J, Basavappa MG, Clark ML, Ali MA, et al. The long noncoding RNA morrbid regulates CD8 T cells in response to viral infection. Proc Natl Acad Sci U.S.A. (2019) 116(24):11916–25. doi: 10.1073/pnas.1819457116
85. Zhang Y, Li B, Bai Q, Wang P, Wei G, Li Z, et al. The lncRNA Snhg1-Vps13D vesicle trafficking system promotes memory CD8 T cell establishment via regulating the dual effects of IL-7 signaling. Signal Transduct Target Ther (2021) 6(1):126. doi: 10.1038/s41392-021-00492-9
86. Cristescu R, Mogg R, Ayers M, Albright A, Murphy E, Yearley J, et al. Pan-tumor genomic biomarkers for PD-1 checkpoint blockade-based immunotherapy. Science (2018) 362(6411):eaar3593. doi: 10.1126/science.aar3593
87. Rizvi NA, Hellmann MD, Snyder A, Kvistborg P, Makarov V, Havel JJ, et al. Cancer immunology. mutational landscape determines sensitivity to PD-1 blockade in non-small cell lung cancer. Science (2015) 348(6230):124–8. doi: 10.1126/science.aaa1348
88. Wu K, Zhao Z, Liu K, Zhang J, Li G, Wang L. Long noncoding RNA lnc-sox5 modulates CRC tumorigenesis by unbalancing tumor microenvironment. Cell Cycle (2017) 16(13):1295–301. doi: 10.1080/15384101.2017.1317416
89. Mineo M, Lyons SM, Zdioruk M, von Spreckelsen N, Ferrer-Luna R, Ito H, et al. Tumor interferon signaling is regulated by a lncRNA INCR1 transcribed from the PD-L1 locus. Mol Cell (2020) 78(6):1207–23 e8. doi: 10.1016/j.molcel.2020.05.015
90. Barber DL, Wherry EJ, Masopust D, Zhu B, Allison JP, Sharpe AH, et al. Restoring function in exhausted CD8 T cells during chronic viral infection. Nature (2006) 439(7077):682–7. doi: 10.1038/nature04444
91. Raziorrouh B, Schraut W, Gerlach T, Nowack D, Gruner NH, Ulsenheimer A, et al. The immunoregulatory role of CD244 in chronic hepatitis b infection and its inhibitory potential on virus-specific CD8+ T-cell function. Hepatology (2010) 52(6):1934–47. doi: 10.1002/hep.23936
92. Guo W, Wang Y, Yang M, Wang Z, Wang Y, Chaurasia S, et al. LincRNA-immunity landscape analysis identifies EPIC1 as a regulator of tumor immune evasion and immunotherapy resistance. Sci Adv (2021) 7(7):eabb3555. doi: 10.1126/sciadv.abb3555
93. Jiang R, Tang J, Chen Y, Deng L, Ji J, Xie Y, et al. The long noncoding RNA lnc-EGFR stimulates T-regulatory cells differentiation thus promoting hepatocellular carcinoma immune evasion. Nat Commun (2017) 8(1):15129–. doi: 10.1038/ncomms15129
94. Tay RE, Richardson EK, Toh HC. Revisiting the role of CD4+ T cells in cancer immunotherapy–new insights into old paradigms. Cancer Gene Ther (2020) 28(1):5–17. doi: 10.1038/s41417-020-0183-x
95. Feng Q, Wei H, Morihara J, Stern J, Yu M, Kiviat N, et al. Th2 type inflammation promotes the gradual progression of HPV-infected cervical cells to cervical carcinoma. Gynecol Oncol (2012) 127(2):412–9. doi: 10.1016/J.YGYNO.2012.07.098
96. Schreiber S, Hammers CM, Kaasch AJ, Schraven B, Dudeck A, Kahlfuss S. Metabolic interdependency of Th2 cell-mediated type 2 immunity and the tumor microenvironment. Front Immunol (2021) 12:632581/BIBTEX. doi: 10.3389/FIMMU.2021.632581/BIBTEX
97. Asadzadeh Z, Mohammadi H, Safarzadeh E, Hemmatzadeh M, Mahdian-shakib A, Jadidi-Niaragh F, et al. The paradox of Th17 cell functions in tumor immunity. Cell Immunol (2017) 322:15–25. doi: 10.1016/J.CELLIMM.2017.10.015
98. Togashi Y, Shitara K, Nishikawa H. Regulatory T cells in cancer immunosuppression - implications for anticancer therapy. Nat Rev Clin Oncol (2019) 16(6):356–71. doi: 10.1038/s41571-019-0175-7
99. Sun J, Jia H, Bao X, Wu Y, Zhu T, Li R, et al. Tumor exosome promotes Th17 cell differentiation by transmitting the lncRNA CRNDE-h in colorectal cancer. Cell Death Dis (2021) 12(1):123–. doi: 10.1038/s41419-020-03376-y
100. Chan WF, Coughlan HD, Iannarella N, Smyth GK, Johanson TM, Keenan CR, et al. Identification and characterization of the long noncoding RNA Dreg1 as a novel regulator of Gata3. Immunol Cell Biol (2021) 99(3):323–32. doi: 10.1111/imcb.12408
101. Gibbons HR, Shaginurova G, Kim LC, Chapman N, Spurlock CF, Aune TM. Divergent lncRNA GATA3-AS1 regulates GATA3 transcription in T-helper 2 cells. Front Immunol (2018) 9:2512. doi: 10.3389/fimmu.2018.02512
102. Iaccarino I, Mourtada F, Reinke S, Patil P, Doose G, Monaco G, et al. LINC00892 is an lncRNA induced by T cell activation and expressed by follicular lymphoma-resident T helper cells. Non-Coding RNA (2022) 8(3). doi: 10.3390/ncrna8030040
103. Houtman M, Shchetynsky K, Chemin K, Hensvold AH, Ramsköld D, Tandre K, et al. T Cells are influenced by a long non-coding RNA in the autoimmune associated PTPN2 locus. J Autoimmun (2018) 90:28–38. doi: 10.1016/j.jaut.2018.01.003
104. Hu G, Tang Q, Sharma S, Yu F, Escobar TM, Muljo SA, et al. Expression and regulation of intergenic long noncoding RNAs during T cell development and differentiation. Nat Immunol (2013) 14(11):1190–8. doi: 10.1038/ni.2712
105. Ranzani V, Rossetti G, Panzeri I, Arrigoni A, Bonnal RJP, Curti S, et al. The long intergenic noncoding RNA landscape of human lymphocytes highlights the regulation of T cell differentiation by linc-MAF-4. Nat Immunol (2015) 16(3):318–25. doi: 10.1038/ni.3093
106. Zhang F, Liu G, Li D, Wei C, Hao J. DDIT4 and associated lncDDIT4 modulate Th17 differentiation through the DDIT4/TSC/mTOR pathway. J Immunol (2018) 200(5):1618–26. doi: 10.4049/jimmunol.1601689
107. Li J, Tian J, Lu J, Wang Z, Ling J, Wu X, et al. LncRNA GAS5 inhibits Th17 differentiation and alleviates immune thrombocytopenia via promoting the ubiquitination of STAT3. Int Immunopharmacol (2020) 80:106127. doi: 10.1016/j.intimp.2019.106127
108. Chen Y, Liu J, Zhang X, Zhu H, Wang Y, Li Z, et al. lncRNA-GM targets Foxo1 to promote T cell–mediated autoimmunity. Sci Adv (2022) 8(31):eabn9181. doi: 10.1126/sciadv.abn9181
109. Shui X, Chen S, Lin J, Kong J, Zhou C, Wu J. Knockdown of lncRNA NEAT1 inhibits Th17/CD4+ T cell differentiation through reducing the STAT3 protein level. J Cell Physiol (2019) 234(12):22477–84. doi: 10.1002/jcp.28811
110. Liu AY, Migeon BR, Siliciano RF. The human NTT gene: identification of a novel 17-kb noncoding nuclear RNA expressed in activated CD4+ T cells. Genomics (1997) 39(2):171-84. doi: 10.1006/geno.1996.4463
111. Huang F-J, Liu Y-L, Wang J, Zhou Y-Y, Zhao S-Y, Qin G-J. LncRNA RUNX1-IT1 affects the differentiation of Th1 cells by regulating NrCAM transcription in graves’ disease. Cell Cycle (2022) 21(9):921–33. doi: 10.1080/15384101.2022.2034431
112. He H, Qiu X, Qi M, Bajinka O, Qin L, Tan Y. lncRNA STAT4-AS1 inhibited TH17 cell differentiation by targeting RORgammat protein. J Immunol Res (2022) 2022:8307280. doi: 10.1155/2022/8307280
113. Hwang SS, Kim K, Lee W, Lee GR. Aberrant expression of IFN-γ in Th2 cells from Th2 LCR-deficient mice. Biochem Biophys Res Commun (2012) 424(3):512–8. doi: 10.1016/j.bbrc.2012.06.146
114. Koh BH, Hwang SS, Kim JY, Lee W, Kang MJ, Lee CG, et al. Th2 LCR is essential for regulation of Th2 cytokine genes and for pathogenesis of allergic asthma. Proc Natl Acad Sci U.S.A. (2010) 107(23):10614–9. doi: 10.1073/pnas.1005383107
115. Niu L, Jiang J, Yin Y, Hu B. LncRNA XLOC_003810 modulates thymic Th17/Treg balance in myasthenia gravis with thymoma. Clin Exp Pharmacol Physiol (2020) 47(6):989–96. doi: 10.1111/1440-1681.13280
116. Hu B, Niu L, Jiang Z, Xu S, Hu Y, Cao K. LncRNA XLOC_003810 promotes T cell activation and inhibits PD-1/PD-L1 expression in patients with myasthenia gravis-related thymoma. Scand J Immunol (2020) 92(1):e12886. doi: 10.1111/sji.12886
117. Peng H, Liu Y, Tian J, Ma J, Tang X, Rui K, et al. The long noncoding RNA IFNG-AS1 promotes T helper type 1 cells response in patients with hashimoto’s thyroiditis. Sci Rep (2015) 5(1):17702. doi: 10.1038/srep17702
118. Briukhovetska D, Dörr J, Endres S, Libby P, Dinarello CA, Kobold S. Interleukins in cancer: from biology to therapy. Nat Rev Cancer (2021) 21(8):481–99. doi: 10.1038/s41568-021-00363-z
119. Lucca LE, Dominguez-Villar M. Modulation of regulatory T cell function and stability by co-inhibitory receptors. Nat Rev Immunol (2020) 20(11):680–93. doi: 10.1038/s41577-020-0296-3
120. Raffin C, Vo LT, Bluestone JA. Treg cell-based therapies: challenges and perspectives. Nat Rev Immunol (2020) 20(3):158–72. doi: 10.1038/s41577-019-0232-6
121. Roncarolo MG, Gregori S, Bacchetta R, Battaglia M, Gagliani N. The biology of T regulatory type 1 cells and their therapeutic application in immune-mediated diseases. Immunity (2018) 49(6):1004–19. doi: 10.1016/j.immuni.2018.12.001
122. van der Veeken J, Campbell C, Pritykin Y, Schizas M, Verter J, Hu W, et al. Genetic tracing reveals transcription factor Foxp3-dependent and Foxp3-independent functionality of peripherally induced treg cells. Immunity (2022) 55(7):1173–84.e7. doi: 10.1016/j.immuni.2022.05.010
123. Muñoz-Rojas AR, Mathis D. Tissue regulatory T cells: regulatory chameleons. Nat Rev Immunol (2021) 21(9):597–611. doi: 10.1038/s41577-021-00519-w
124. Simpson TR, Li F, Montalvo-Ortiz W, Sepulveda MA, Bergerhoff K, Arce F, et al. Fc-dependent depletion of tumor-infiltrating regulatory T cells co-defines the efficacy of anti-CTLA-4 therapy against melanoma. J Exp Med (2013) 210(9):1695–710. doi: 10.1084/jem.20130579
125. Brajic A, Franckaert D, Burton O, Bornschein S, Calvanese AL, Demeyer S, et al. The long non-coding RNA flatr anticipates Foxp3 expression in regulatory T cells. Front Immunol (2018) 9:1989(SEP). doi: 10.3389/fimmu.2018.01989
126. Zemmour D, Pratama A, Loughhead SM, Mathis D, Benoist C. Flicr, a long noncoding RNA, modulates Foxp3 expression and autoimmunity. Proc Natl Acad Sci U.S.A. (2017) 114(17):E3472 LP–E80. doi: 10.1073/pnas.1700946114
127. Xia M, Liu J, Liu S, Chen K, Lin H, Jiang M, et al. Ash1l and lnc-Smad3 coordinate Smad3 locus accessibility to modulate iTreg polarization and T cell autoimmunity. Nat Commun (2017) 8:15818. doi: 10.1038/ncomms15818
128. Fu J, Shi H, Wang B, Zhan T, Shao Y, Ye L, et al. LncRNA PVT1 links myc to glycolytic metabolism upon CD4+ T cell activation and sjögren's syndrome-like autoimmune response. J Autoimmun (2020) 107:102358. doi: 10.1016/j.jaut.2019.102358
129. Yu Z, Zhao H, Feng X, Li H, Qiu C, Yi X, et al. Long non-coding RNA FENDRR acts as a miR-423-5p sponge to suppress the treg-mediated immune escape of hepatocellular carcinoma cells. Mol Ther Nucleic Acids (2019) 17:516–29. doi: 10.1016/j.omtn.2019.05.027
130. Xiong G, Yang L, Chen Y, Fan Z. Linc-POU3F3 promotes cell proliferation in gastric cancer via increasing T-reg distribution. Am J Transl Res (2015) 7(11):2262–9.
131. Wang Y, Yang X, Sun X, Rong L, Kang M, Wu P, et al. Bone marrow infiltrated lnc-INSR induced suppressive immune microenvironment in pediatric acute lymphoblastic leukemia. Cell Death Dis (2018) 9(10):1043–. doi: 10.1038/s41419-018-1078-8
132. Wang J, Huang F, Shi Y, Zhang Q, Xu S, Yao Y, et al. RP11-323N12.5 promotes the malignancy and immunosuppression of human gastric cancer by increasing YAP1 transcription. Gastric Cancer (2021) 24(1):85–102. doi: 10.1007/s10120-020-01099-9
133. Pei X, Wang X, Li H. LncRNA SNHG1 regulates the differentiation of treg cells and affects the immune escape of breast cancer via regulating miR-448/IDO. Int J Biol Macromol (2018) 118:24–30. doi: 10.1016/j.ijbiomac.2018.06.033
134. Huang A, Yang X-R, Chung W-Y, Dennison AR, Zhou J. Targeted therapy for hepatocellular carcinoma. Signal Transduct Target Ther (2020) 5(1):146. doi: 10.1038/s41392-020-00264-x
135. Jin H, Shi Y, Lv Y, Yuan S, Ramirez CFA, Lieftink C, et al. EGFR activation limits the response of liver cancer to lenvatinib. Nature (2021) 595(7869):730–4. doi: 10.1038/s41586-021-03741-7
136. Blivet-Van Eggelpoël MJ, Fartoux L, Fartoux L, Aoudjehane L, Barbu V, Rey C, et al. Epidermal growth factor receptor and HER-3 restrict cell response to sorafenib in hepatocellular carcinoma cells. J Hepatol (2012) 57(1):108-15. doi: 10.1016/j.jhep.2012.02.019
137. Sun CC, Zhu W, Li SJ, Hu W, Zhang J, Zhuo Y, et al. FOXC1-mediated LINC00301 facilitates tumor progression and triggers an immune-suppressing microenvironment in non-small cell lung cancer by regulating the HIF1α pathway. Genome Med (2020) 12(1):77. doi: 10.1186/s13073-020-00773-y
138. Lawand M, Dechanet-Merville J, Dieu-Nosjean MC. Key features of gamma-delta T-cell subsets in human diseases and their immunotherapeutic implications. Front Immunol (2017) 8:761. doi: 10.3389/fimmu.2017.00761
139. Wu Y, Biswas D, Usaite I, Angelova M, Boeing S, Karasaki T, et al. A local human Vdelta1 T cell population is associated with survival in nonsmall-cell lung cancer. Nat Cancer (2022) 3(6):696–709. doi: 10.1038/s43018-022-00376-z
140. Wu Y, Kyle-Cezar F, Woolf RT, Naceur-Lombardelli C, Owen J, Biswas D, et al. An innate-like Vdelta1(+) gammadelta T cell compartment in the human breast is associated with remission in triple-negative breast cancer. Sci Transl Med (2019) 11(513):eaax9364. doi: 10.1126/scitranslmed.aax9364
141. Foord E, Arruda LCM, Gaballa A, Klynning C, Uhlin M. Characterization of ascites- and tumor-infiltrating gammadelta T cells reveals distinct repertoires and a beneficial role in ovarian cancer. Sci Transl Med (2021) 13(577):eabb0192. doi: 10.1126/scitranslmed.abb0192
142. Reis BS, Darcy PW, Khan IZ, Moon CS, Kornberg AE, Schneider VS, et al. TCR-vgammadelta usage distinguishes protumor from antitumor intestinal gammadelta T cell subsets. Science (2022) 377(6603):276–84. doi: 10.1126/science.abj8695
143. Kabelitz D, Serrano R, Kouakanou L, Peters C, Kalyan S. Cancer immunotherapy with γδ T cells: many paths ahead of us. Cell Mol Immunol (2020) 17(9):925–39. doi: 10.1038/s41423-020-0504-x
144. Nelson A, Lukacs JD, Johnston B. The current landscape of NKT cell immunotherapy and the hills ahead. Cancers (2021) 13(20):5174–. doi: 10.3390/CANCERS13205174
145. Esposito R, Bosch N, Lanzos A, Polidori T, Pulido-Quetglas C, Johnson R. Hacking the cancer genome: profiling therapeutically actionable long non-coding RNAs using CRISPR-Cas9 screening. Cancer Cell (2019) 35(4):545–57. doi: 10.1016/j.ccell.2019.01.019
146. Lin C, Yang L. Long noncoding RNA in cancer: wiring signaling circuitry. Trends Cell Biol (2018) 28(4):287–301. doi: 10.1016/j.tcb.2017.11.008
147. Hanahan D, Weinberg RA. Hallmarks of cancer: the next generation. Cell (2011) 144(5):646–74. doi: 10.1016/j.cell.2011.02.013
148. Zhang C, Jiang F, Su C, Xie P, Xu L. Upregulation of long noncoding RNA SNHG20 promotes cell growth and metastasis in esophageal squamous cell carcinoma via modulating ATM-JAK-PD-L1 pathway. J Cell Biochem (2019) 120(7):11642–50. doi: 10.1002/jcb.28444
149. Wang QM, Lian GY, Song Y, Huang YF, Gong Y. LncRNA MALAT1 promotes tumorigenesis and immune escape of diffuse large b cell lymphoma by sponging miR-195. Life Sci (2019) 231:116335. doi: 10.1016/j.lfs.2019.03.040
150. Li G, Kryczek I, Nam J, Li X, Li S, Li J, et al. LIMIT is an immunogenic lncRNA in cancer immunity and immunotherapy. Nat Cell Biol (2021) 23(5):526–37. doi: 10.1038/s41556-021-00672-3
151. Hu Q, Ye Y, Chan LC, Li Y, Liang K, Lin A, et al. Oncogenic lncRNA downregulates cancer cell antigen presentation and intrinsic tumor suppression. Nat Immunol (2019) 20(7):835–51. doi: 10.1038/s41590-019-0400-7
152. Ma F, Lei YY, Ding MG, Luo LH, Xie YC, Liu XL. LncRNA NEAT1 interacted with DNMT1 to regulate malignant phenotype of cancer cell and cytotoxic T cell infiltration via epigenetic inhibition of p53, cGAS, and STING in lung cancer. Front Genet (2020) 11:250/BIBTEX. doi: 10.3389/FGENE.2020.00250/BIBTEX
153. Chen Z, Chen Z, Xu S, Zhang Q. LncRNA SOX2-OT/miR-30d-5p/PDK1 regulates PD-L1 checkpoint through the mTOR signaling pathway to promote non-small cell lung cancer progression and immune escape. Front Genet (2021) 12:674856. doi: 10.3389/fgene.2021.674856
154. Lin Z-b, Long P, Zhao Z, Zhang Y-r, Chu X-d, Zhao X-x, et al. Long noncoding RNA KCNQ1OT1 is a prognostic biomarker and mediates CD8+ T cell exhaustion by regulating CD155 expression in colorectal cancer. Int J Biol Sci (2021) 17(7):1757–68. doi: 10.7150/ijbs.59001
155. Zhou WY, Zhang MM, Liu C, Kang Y, Wang JO, Yang XH. Long noncoding RNA LINC00473 drives the progression of pancreatic cancer via upregulating programmed death-ligand 1 by sponging microRNA-195-5p. J Cell Physiol (2019) 234(12):23176–89. doi: 10.1002/JCP.28884
156. Zhao L, Liu Y, Zhang J, Liu Y, Qi Q. LncRNA SNHG14/miR-5590-3p/ZEB1 positive feedback loop promoted diffuse large b cell lymphoma progression and immune evasion through regulating PD-1/PD-L1 checkpoint. Cell Death Dis (2019) 10(10):731–. doi: 10.1038/s41419-019-1886-5
157. Xu Y-J, Zhao J-M, Ni X-F, Wang W, Hu W-W, Wu C-P. LncRNA HCG18 suppresses CD8+ T cells to confer resistance to cetuximab in colorectal cancer via miR-20b-5p/PD-L1 axis. Epigenomics (2021) 13(16):1283–99. doi: 10.2217/epi-2021-0130
158. Tian P, Wei J-X, Li J, Ren J-K, Yang J-J. LncRNA SNHG1 regulates immune escape of renal cell carcinoma by targeting miR-129-3p to activate STAT3 and PD-L1. Cell Biol Int (2021) 45(7):1546–60. doi: 10.1002/cbin.11595
159. Wang Y, Yang L, Dong X, Yang X, Zhang X, Liu Z, et al. Overexpression of NNT-AS1 activates TGF-beta signaling to decrease tumor CD4 lymphocyte infiltration in hepatocellular carcinoma. BioMed Res Int (2020) 2020:8216541. doi: 10.1155/2020/8216541
160. Zhong C, Tao B, Li X, Xiang W, Peng L, Peng T, et al. HOXA-AS2 contributes to regulatory T cell proliferation and immune tolerance in glioma through the miR-302a/KDM2A/JAG1 axis. Cell Death Dis (2022) 13(2):160. doi: 10.1038/s41419-021-04471-4
161. Wang J, Zhao X, Wang Y, Ren F, Sun D, Yan Y, et al. circRNA-002178 act as a ceRNA to promote PDL1/PD1 expression in lung adenocarcinoma. Cell Death Dis (2020) 11(1):32. doi: 10.1038/s41419-020-2230-9
162. Liu Z, Wang T, She Y, Wu K, Gu S, Li L, et al. N6-methyladenosine-modified circIGF2BP3 inhibits CD8+ T-cell responses to facilitate tumor immune evasion by promoting the deubiquitination of PD-L1 in non-small cell lung cancer. Mol Cancer (2021) 20(1):105. doi: 10.1186/s12943-021-01398-4
163. Chen S-W, Zhu S-Q, Pei X, Qiu B-Q, Xiong D, Long X, et al. Cancer cell-derived exosomal circUSP7 induces CD8+ T cell dysfunction and anti-PD1 resistance by regulating the miR-934/SHP2 axis in NSCLC. Mol Cancer (2021) 20(1):144. doi: 10.1186/s12943-021-01448-x
164. Lu YB, Jiang Q, Yang MY, Zhou JX, Zhang Q. Long noncoding RNA NNT-AS1 promotes hepatocellular carcinoma progression and metastasis through miR-363/CDK6 axis. Oncotarget (2017) 8(51):88804–14. doi: 10.18632/oncotarget.21321
165. Roberts TC, Langer R, Wood MJA. Advances in oligonucleotide drug delivery. Nat Rev Drug Discovery (2020) 19(10):673–94. doi: 10.1038/s41573-020-0075-7
166. Shi Y, Parag S, Patel R, Lui A, Murr M, Cai J, et al. Stabilization of lncRNA GAS5 by a small molecule and its implications in diabetic adipocytes. Cell Chem Biol (2019) 26(3):319–30 e6. doi: 10.1016/j.chembiol.2018.11.012
167. Abulwerdi FA, Xu W, Ageeli AA, Yonkunas MJ, Arun G, Nam H, et al. Selective small-molecule targeting of a triple helix encoded by the long noncoding RNA, MALAT1. ACS Chem Biol (2019) 14(2):223–35. doi: 10.1021/acschembio.8b00807
168. Wang R, Sun Y, Li L, Niu Y, Lin W, Lin C, et al. Preclinical study using Malat1 small interfering RNA or androgen receptor splicing variant 7 degradation enhancer ASC-J9((R)) to suppress enzalutamide-resistant prostate cancer progression. Eur Urol (2017) 72(5):835–44. doi: 10.1016/j.eururo.2017.04.005
169. Gutschner T, Hammerle M, Eissmann M, Hsu J, Kim Y, Hung G, et al. The noncoding RNA MALAT1 is a critical regulator of the metastasis phenotype of lung cancer cells. Cancer Res (2013) 73(3):1180–9. doi: 10.1158/0008-5472.CAN-12-2850
170. Kim J, Piao HL, Kim BJ, Yao F, Han Z, Wang Y, et al. Long noncoding RNA MALAT1 suppresses breast cancer metastasis. Nat Genet (2018) 50(12):1705–15. doi: 10.1038/s41588-018-0252-3
171. Hewitson JP, West KA, James KR, Rani GF, Dey N, Romano A, et al. Malat1 suppresses immunity to infection through promoting expression of maf and IL-10 in Th cells. J Immunol (2020) 204(11):2949–60. doi: 10.4049/jimmunol.1900940
172. Andergassen D, Rinn JL. From genotype to phenotype: genetics of mammalian long non-coding RNAs in vivo. Nat Rev Genet (2022) 23(4):229–43. doi: 10.1038/s41576-021-00427-8
Keywords: noncoding RNA, tumor-infiltrating T cell, regulatory T cell, gamma delta T cell, exhaustion, dysfunction, tumor immune evasion, adoptive cell therapy
Citation: Erber J and Herndler-Brandstetter D (2023) Regulation of T cell differentiation and function by long noncoding RNAs in homeostasis and cancer. Front. Immunol. 14:1181499. doi: 10.3389/fimmu.2023.1181499
Received: 07 March 2023; Accepted: 02 May 2023;
Published: 06 June 2023.
Edited by:
Ashor Kumar, All India Institute of Medical Sciences, Bhopal, IndiaReviewed by:
Subhash Kumar Tripathi, Seattle Children’s Research Institute, United StatesZhiyuan Zhang, Zhongshan Hospital, Fudan University, China
Ghanbar Mahmoodi Chalbatani, Tehran University of Medical Sciences, Iran
Copyright © 2023 Erber and Herndler-Brandstetter. This is an open-access article distributed under the terms of the Creative Commons Attribution License (CC BY). The use, distribution or reproduction in other forums is permitted, provided the original author(s) and the copyright owner(s) are credited and that the original publication in this journal is cited, in accordance with accepted academic practice. No use, distribution or reproduction is permitted which does not comply with these terms.
*Correspondence: Dietmar Herndler-Brandstetter, ZGlldG1hci5oZXJuZGxlci1icmFuZHN0ZXR0ZXJAbWVkdW5pd2llbi5hYy5hdA==