- 1IRCCS Humanitas Research Hospital, Milan, Italy
- 2Department of Biomedical Sciences, Humanitas University, Milan, Italy
Neutrophils are the most abundant circulating leukocytes in humans and the first immune cells recruited at the site of inflammation. Classically perceived as short-lived effector cells with limited plasticity and diversity, neutrophils are now recognized as highly heterogenous immune cells, which can adapt to various environmental cues. In addition to playing a central role in the host defence, neutrophils are involved in pathological contexts such as inflammatory diseases and cancer. The prevalence of neutrophils in these conditions is usually associated with detrimental inflammatory responses and poor clinical outcomes. However, a beneficial role for neutrophils is emerging in several pathological contexts, including in cancer. Here we will review the current knowledge of neutrophil biology and heterogeneity in steady state and during inflammation, with a focus on the opposing roles of neutrophils in different pathological contexts.
Introduction
Neutrophils represent the largest population of circulating leukocytes in humans and play a central role in acute phase responses (1). Given their inability to proliferate and their short lifespan, neutrophils are considered poorly adaptive cells with limited effector functions (2). This hypothesis has been challenged by recent discoveries made possible by new technologies. For instance, the lifespan and heterogeneity of neutrophils have been re-evaluated in the last years (3–8).
The reassessment of neutrophil biology revealed their plasticity under healthy conditions or during inflammatory processes and diseases, such as in cancer (9–11). Neutrophils are well known for their protective role in response against invading pathogens (12). However, they can sustain harmful and pathological inflammation in some conditions such as immune-mediated diseases, fibrosis, and cancer (13–15). In these pathologies, the presence of neutrophils or molecules associated with their recruitment have frequently been used as markers of severity and associated with poor clinical outcomes (9, 14–26). In contrast, recent findings have challenged this view and suggested that a better understanding of neutrophil plasticity and heterogeneity was needed to explain their different roles in pathological conditions (13, 23, 27). Here, we will discuss the current knowledge of neutrophil biology and diversity in steady state and in immune-related diseases, including in cancer.
Neutrophil development, recruitment, and effector mechanisms
Neutrophil development in steady state and inflammation
The half-life of neutrophils in circulation ranges from 6 to 8 hours. Therefore, their presence in blood requires a constant replenishment from the bone marrow (BM) (1, 28). The process of granulopoiesis is tightly regulated by the signalling of granulocyte-colony stimulating factor (G-CSF) and granulocyte–macrophage colony-stimulating factor (GM-CSF) (29). Accordingly, G-CSF and GM-CSF-deficient mice displayed severe and chronic neutropenia (30, 31).
In the bone marrow (BM), hematopoietic stem cells (HSCs) give rise to either common myeloid progenitors (CMP) or common lymphoid progenitors (CLP) (28). The CMP differentiate into granulocyte monocyte progenitors (GMP) or megakaryocyte erythroid progenitors (MEP), while CLP differentiate into T lymphocytes, B lymphocytes or NK cells (29). Then, the GMP differentiate into more mature cells referred to as myeloblasts, which subsequently differentiate into promyelocytes, myelocytes, metamyelocytes, and band neutrophils (29).
Granulopoiesis is defined by a specific and highly regulated transcriptional program where several transcription factors are involved, including CCAAT/enhancer binding proteins (C/EBPs), GATA-1 and PU.1 (28, 32, 33). C/EBPs comprise a family of six transcription factors (C/EBP-α, -β, -γ, -δ, -ε, and -ζ) characterized by a conserved leucine zipper C-terminal domain next to a positively charged DNA-binding domain (34, 35). C/EBP-α specifically induces early myeloid precursors to enter CMP differentiation pathways, whereas C-EBP-ε and Gfi-1 are implicated in the further steps of granulopoiesis, such as in the terminal phase of differentiation (36, 37). In humans, the transit time from HSC to mature neutrophils ranges from 4 to 6 days, after which neutrophils are available for release into the circulation (28, 38).
Once in the circulation, the neutrophil lifespan has been estimated to be less than 24 hours (39). However, in tissues their lifespan could be considerable prolonged, up to 2-4 days (3). In tissues and during an inflammatory process, other cells present in the microenvironment, such as macrophages, can secrete cytokines (e.g., GM-CSF, G-CSF, TNFα) that can prolong the lifespan of neutrophils (40).
The hematopoietic system is capable of rapid adaption to a variety of stimuli, such as stress, bleeding, or infections by increasing the rate of granulopoiesis (41). This program is known as “emergency granulopoiesis” and is characterized by leucocytosis, neutrophilia, and the presence of immature cells in the peripheral blood (42). Different cytokines, including G-CSF, GM-CSF, IL-3 and IL-6 are involved in the activation of emergency granulopoiesis (31, 42).
Using cutting-edge technologies such as single-cell RNA sequencing (scRNAseq) and mass-cytometry by time of flight (CyTOF), it has been possible to highlight discrete subsets of neutrophil precursors (4–7). For instance, a population of proliferating neutrophil precursors (defined as preNeu), characterized as Gr1+ CD11b+ CXCR4hi CD117int CXCR2- cells in mice and with the potential to generate non-proliferating immature which subsequently become mature neutrophils has been recently identified (4). This first observation led to the identification of preNeu progenitors. These progenitors were found both in mice and humans and represent a heterogeneous population of cells described as proNeu1, proNeu2 and preNeu (6). In humans, a recent study identified a population of CD66b−CD64dimCD115− neutrophil-committed progenitor cells (NCPs) within SSCloCD45dimCD34+ and CD34dim/− cells found in the BM (43). In Figure 1 are summarised all neutrophil developmental stages with known associated markers.
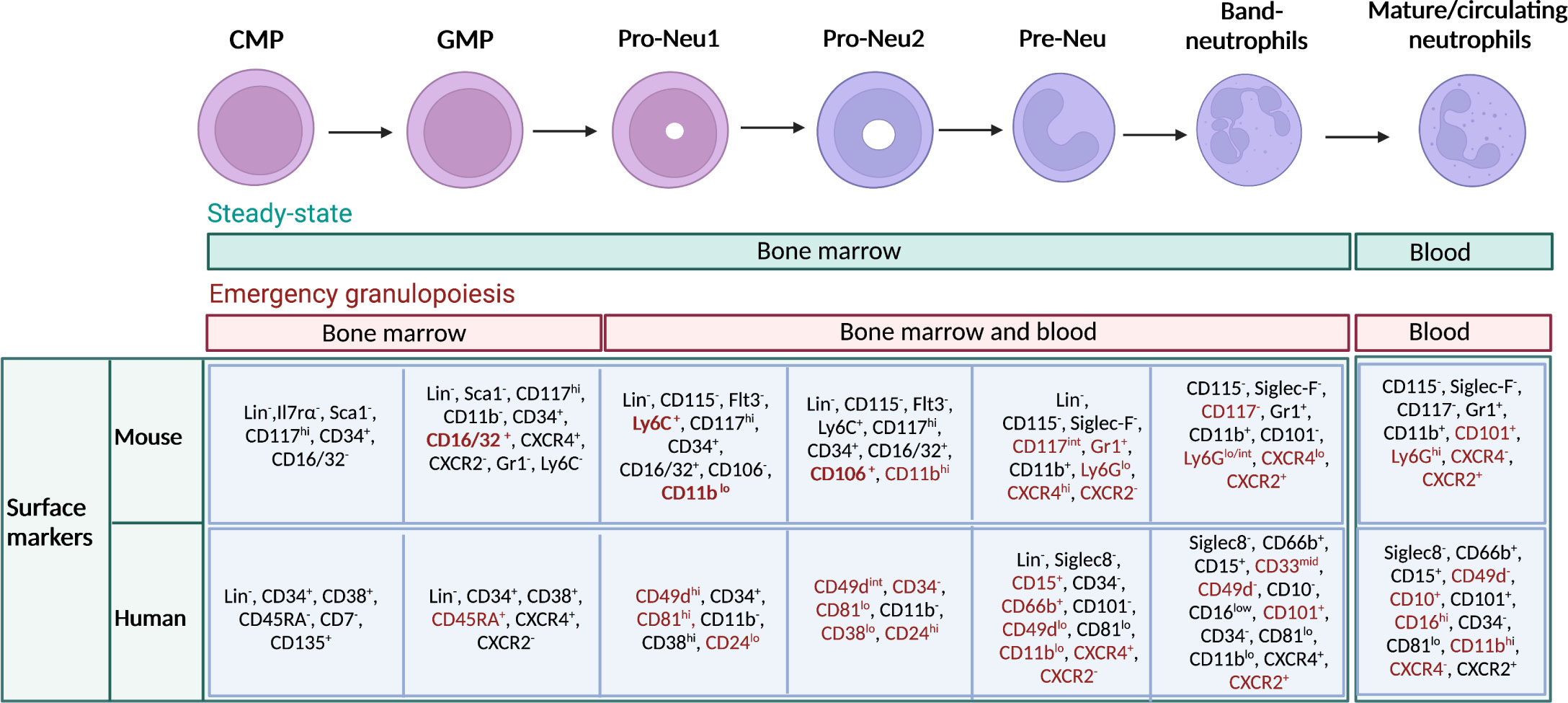
Figure 1 Maturation stages of neutrophils and immunophenotype in mouse and human. Schematic representation of neutrophil developmental stages in the bone marrow (BM), with associated known markers in human and mouse. Neutrophils arise from hematopoietic stem cells (HSCs) in the BM, which give rise to multipotent progenitors, then common myeloid progenitors (CMPs), granulocyte–monocyte progenitors (GMPs), and subsequently to neutrophil-committed precursors. In the BM, GMPs mature into proNeus and then into preNeus, which differentiate into band neutrophils. Finally, non-proliferative mature neutrophils are released in the circulation. Green bar indicates the location of neutrophil progenitors during steady state. Red bar indicates the location where neutrophil progenitors can be found during emergency granulopoiesis. Markers that particularly characterize a stage of differentiation or the transition from one stage to another have been highlighted in red. Gr1 represents a cell marker that includes both Ly6C and Ly6G. However, anti-Gr1 monoclonal antibody reacts strongly with Ly6G. and weakly with Ly6C.
Neutrophil mobilization and recruitment
The regulation of the expression of genes coding for CXCR4 and CXCR2 is essential for the final step of neutrophil maturation and their release in the bloodstream (2, 44). In steady state, stromal cells express CXCL12 in the BM, a ligand for CXCR4, which is expressed on the surface of immature neutrophils and supports their retention in the BM (12, 45). As neutrophils mature, the expression of CXCR4 is downregulated, while the expression of CXCR2 is increased. The abundance of the chemokine CXCL2, which is the ligand for CXCR2, in the circulation triggers the release of neutrophils into peripheral blood (2). A peculiar phenomenon is related to the circadian regulation of CXCR2 and CXCR4 expression. As demonstrated by Adrover José M. et al, the circadian clock transcription factor Bmal1 drives the expression of CXCL2 to induce CXCR2-dependent diurnal changes in the transcriptional landscape and migration capacity of neutrophils (46). These diurnal alterations are related to neutrophil aging and can be antagonized by CXCR4 signalling (46, 47).
The recruitment of neutrophils in inflammatory sites is initiated by the interactions between circulating leukocytes and activated endothelium. The endothelium can be directly activated by pattern-recognition receptors (PRRs) signalling (48). Neutrophils are primed by exposure to pro-inflammatory cytokines, such as tumour necrosis factor-α (TNF-α), IL-1β and IL-17, chemokines, growth factors, pathogen-associated molecular patterns (PAMPs) and by interacting with the activated endothelium (1, 38, 49). Neutrophils express the chemokine receptors CXCR1 and CXCR2 and the presence of their ligands (CXCL1, CXCL2) provides important chemotactic signalling for neutrophil recruitment into inflamed tissues (50).
Neutrophil recruitment includes the following steps: tethering, rolling, adhesion, crawling, and transmigration (49). Activated endothelial cells upregulate the expression of P-selectin and E-selectin, two molecules that interact with their glycosylated ligands expressed by neutrophils, such as the P-selectin ligand 1 (PSGL-1). The interaction leads to the tethering of neutrophils and their rolling in the direction of the blood flow (20, 51). The activation of neutrophils induces changes in the conformation of cell surface-expressed integrins, which subsequently show higher affinity for their ligands (1). In particular, neutrophils express constitutively high levels of the integrins macrophage-1 antigen (MAC1; CD11b\CD18) and lymphocyte function-associated antigen 1 (LFA1; CD11a\CD18), which undergo conformational changes to bind to the transmembrane glycoproteins endothelial intercellular adhesion molecule 1 (ICAM1) and ICAM2 expressed by endothelial cells (52). Once arrested on the endothelium, neutrophils are ready to transmigrate from the vasculature to the inflammatory site where they can exert different roles, according to tissue context (3, 13). Recent investigations showed that neutrophils can also migrate from inflamed tissues into the peripheral blood through a process known as reverse migration (53). The process of reverse migration has been proposed as a mechanism to prevent excessive inflammation and tissue damage. In the tissue, the elimination of apoptotic or aged neutrophils by macrophages promotes the resolution of the inflammation (see below) (54).
Neutrophil effector mechanisms
Neutrophils have long been viewed as primary effector cells in the elimination of pathogens and during the acute inflammatory process (55). Thus, their effector mechanisms are classically associated with antimicrobial features. However, their phenotype and function can be different in different inflammatory contexts, such as in fibrosis and cancer.
The antimicrobial potential of neutrophils includes phagocytosis, degranulation, production of reactive oxygen species (ROS) and release of neutrophils extracellular traps (NETs) (1, 56). Additionally, neutrophils secrete a broad spectrum of cytokines and chemokines that can orchestrate the adaptive and innate immune systems (1, 57, 58).
An important mechanism of pathogen elimination consists in the process of phagocytosis, where receptors, such as the FcγR, are used for the engulfment of opsonised or non-opsonised pathogens (59). After the engulfment, a phagosome is formed and its maturation mediates pathogen clearance through the presence of a variety of hydrolytic enzymes and the production of ROS (60). Additionally, neutrophils can eliminate pathogens through the extracellular release of NETs and cytotoxic granules (1). Three types of neutrophil granules have been described: primary (also called azurophilic), secondary (or specific), and tertiary (or gelatinase) granules (61). Primary granules are generally defined by their content of acidic hydrolases, myeloperoxidase (MPO), and microbicidal molecules, such as bactericidal/permeability-increasing protein (BPI) and defensins (28, 61, 62). Specific granules are rich in molecules that participate in neutrophil microbicidal activities, such as lactoferrin, cathelicidin and the long pentraxin 3 (PTX3) (63). Finally, tertiary granules, which are mobilized when neutrophils established primary rolling contact with the activated endothelium, are characterised by matrix-degrading enzymes, such as gelatinase, and membrane receptors including CD11b/CD18, CD67, CD177, fMLF-R, SCAMP, and VAMP2 (64).
Neutrophils can undergo a unique form of cell death, called NETosis, which results in the formation of NETs (59, 65). NETs are large, extracellular, web-like structures composed of cytosolic and granule proteins that are assembled on a scaffold of decondensed chromatin (65, 66). These structures are extruded by activated neutrophils and pathogens are immobilized and killed through the exposure to high concentrations of effector proteins, including enzymes and antimicrobial molecules (e.g., defensins and cathelicidins) (63, 65).
Neutrophils are also able to synthesize different mediators that can orchestrate both innate and adaptive immunity (67). For instance, neutrophils produce pro- and anti-inflammatory cytokines (e.g., IL-6, TNF-α, IL-1β, IL-1RA, TGFβ) and chemokines (e.g., CXCL8, CXCL10) (60, 67–69).
Neutrophil functional heterogeneity
The classic view of neutrophils as poorly adaptive cells has been challenged by recent findings. Indeed, a growing number of studies have demonstrated the heterogeneity and plasticity of neutrophils. It has been reported that circulating and tissue neutrophils displayed substantial heterogeneity, in terms of phenotype, maturation\activation states and effector functions, due to circadian oscillations, aging, and the presence of microenvironment cues (3, 8, 70). Accordingly, freshly released and aged neutrophils showed considerable diversity in their repertoire of chemokines, PRRs, adhesion molecules, granule proteins, and in their ability to release NETs (46, 71–73). These results support the hypothesis that neutrophil-dependent immune response is not stable during the day and may be dependent on environmental cues (74).
Neutrophils are recruited in healthy tissues and can contribute to their physiological functions (3, 47, 75–78). Indeed, a recent report showed that neutrophils can acquire tissue-specific transcriptional programs that support tissue homeostasis in different organs. In the lung, this process is driven by the CXCL12/CXCR4 axis (3). Accordingly, the lack of CXCR4 in neutrophils impaired their acquisition of the lung-specific signature (3). Neutrophils are present in other tissues, such as in the intestine, white adipose tissue, skin, and skeletal muscle but their role and functions need to be fully elucidated.
Neutrophils with specialized functions were reported in the BM. Indeed, BM-resident neutrophils are important to sustain the regenerative capacity of medullary sinusoid via the production of TNFα (79). In addition, mature BM neutrophils expressing the histidine-decarboxylase were reported to support the maintenance of the quiescence state of HSCs, through the release of histamine and consequent activation of the H2 receptor on HSC (80). Finally, BM neutrophils can secrete prostaglandin E2 (PGE2), which stimulates the production of osteopontin by preosteoblasts and the retention of HSCs (81).
Specialized neutrophils were also found in the marginal zone of the spleen, where they can promote the immunoglobulin class switching and production of antibodies by activated B cells through the production of B-cell activating factor (BAFF), a proliferation-inducing ligand (APRIL) and IL-21 (78). In lymph nodes, MHCII+ neutrophils were found in the proximity of T and NK cells and are thought to promote T cell activation (76).
Neutrophils in systemic inflammation
Neutrophils represent the first line of defence against invading pathogens and are recruited to the site of infection and inflammation by chemokines secreted by host cells and pathogen-derived products (12). Thereafter, they can efficiently kill invading pathogens and actively recruit other immune cells to complete the clearance of foreign microorganisms (1). Neutrophils are equipped with a broad range of antimicrobial weapons to clear pathogens through the combination of different effector mechanisms including, phagocytosis, production of ROS, degranulation, and release of NETs (59) In addition, neutrophils express phagocytic receptors and a set of soluble PRRs with an opsonic activity which are considered as functional ancestors of antibodies (82) (Figures 2A–C).
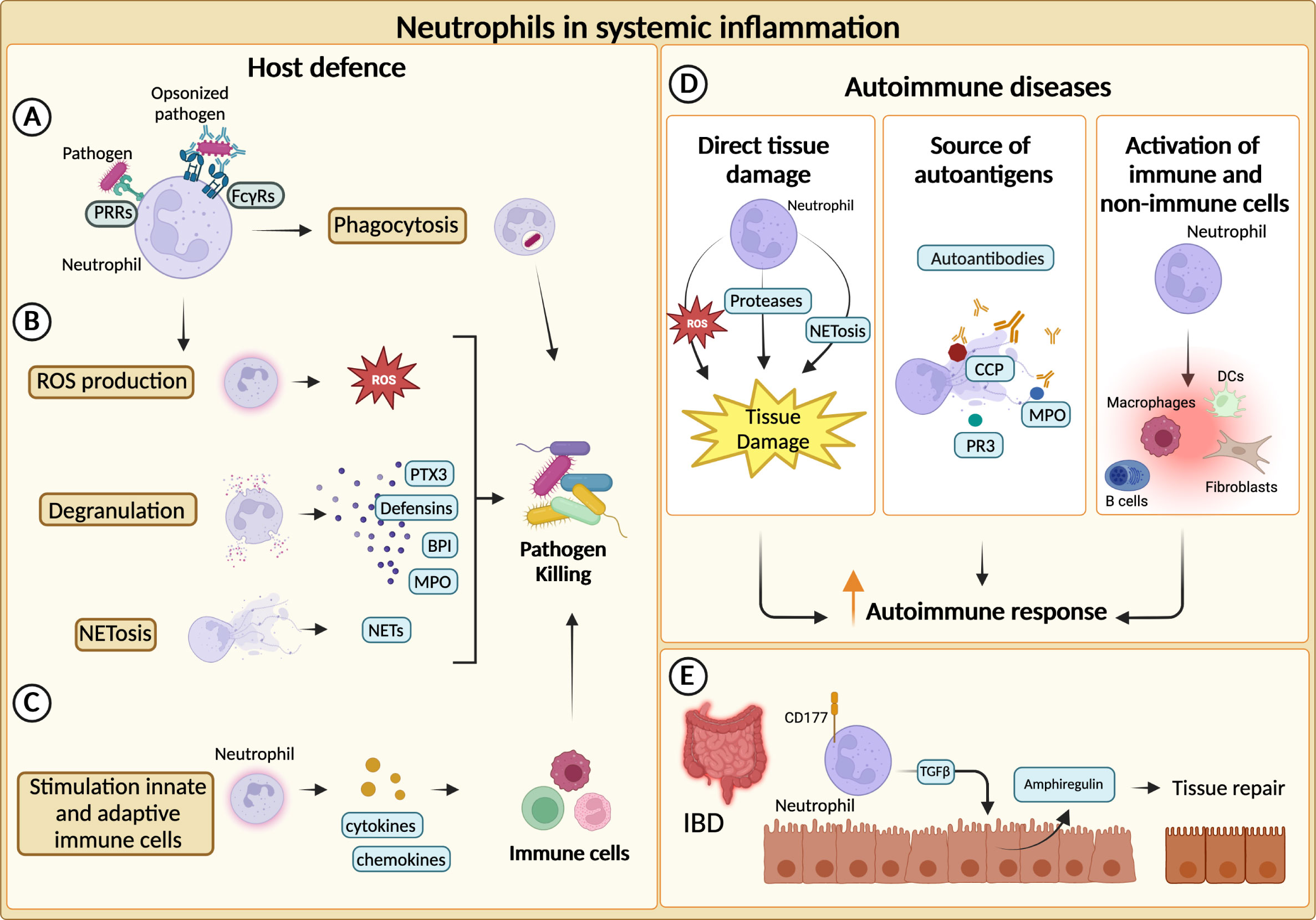
Figure 2 Neutrophils in systemic inflammation. (A) Neutrophils phagocytize and kill pathogens by using Fcγ receptors (FcγRs) and pathogen recognition receptors (PRRs) for the engulfment of opsonized or non-opsonized pathogens. (B) Neutrophils can eliminate pathogens through degranulation, production of reactive oxygen species (ROS) and release of neutrophil extracellular traps (NETs). (C) Neutrophils actively recruit and activate other innate and adaptive immune cells ensuring a complete clearance of the pathogens. (D) Neutrophils can fuel the autoimmune response and exacerbate tissue-damaging inflammation through various mechanisms. First, they can directly cause tissue damage. NETs-associated molecules, such as cyclic citrullinated peptides (CCP), myeloperoxidase (MPO) and proteinase 3 (PR3), can represent an important source of autoantibody targets. Neutrophils promote autoimmune response by recruiting and activating adaptive and innate immune cells as well as non-immune cells. (E) In inflammatory bowel diseases (IBD), CD177+ neutrophils producing TGFβ stimulate the production of the tissue repair-promoting factor amphiregulin (AREG) by intestinal epithelial cells and promote tissue repair.
Given their extraordinarily potent effector functions, excessive or uncontrolled neutrophil activation could have deleterious consequences for the host. An increasing body of evidence suggests that an abnormal neutrophil activation might play a prominent role in the pathophysiology of severe inflammation-related disorders, such as Coronavirus disease 2019 (COVID-19) (83, 84). Indeed, severe COVID-19 has been associated with increased neutrophil-to-lymphocyte ratio (85) and concentration of neutrophil-related molecules in the serum (e.g., calprotectin, NETs-associated molecules) (86–88). Therefore, neutrophilia is associated with bad prognosis in COVID-19 patients (84).
A deleterious role for neutrophils has been observed also in the context of autoimmune diseases and chronic inflammation. Diseases such as systemic lupus erythematosus (SLE), rheumatoid arthritis (RA), vasculitis, type I diabetes (T1D), multiple sclerosis (MS), idiopathic inflammatory myopathies (IIM) are characterized by the loss of immune tolerance to self-antigens. Although autoimmune diseases have been mainly ascribed to dysfunctions in adaptive immune response, an increasing number of evidence points to a possible role for innate immunity and in particular neutrophils (17, 89–91).
Increased neutrophil infiltration or augmented levels of neutrophil-specific molecules are often found within inflamed tissues of both SLE and IIM patients (92, 93). Moreover, neutrophil-related biomarkers (e.g., neutrophil serine proteinases, calprotectin, NETs) correlate with clinical parameters and response to therapy in various disease settings, further corroborating the role of neutrophils in autoimmunity (94–98). In autoimmune diseases, neutrophils can exacerbate tissue-damaging inflammation through a variety of mechanisms, including the production of ROS, proteases and the activation of immune and not immune cells (17, 99) (Figure 2D). Neutrophils from SLE patients showed cytotoxic activity towards endothelial cells in vitro. Consistently, SLE patients present an augmented risk of endothelial dysfunction (92, 100). Similarly, NETs have been found to affect the function of myoblast in IIM patients and can contribute to the cleavage of articular cartilage in RA (100). Interestingly, different components of NETs (e.g., double-stranded DNA, histones, citrullinated peptides (CCP), MPO and proteinase 3 (PR3)) are well-described targets of autoantibodies in autoimmune diseases (96, 97, 101, 102) (Figure 2D).
Neutrophils are endowed with the ability to recruit and activate a plethora of cells including adaptive and innate immune cells as well as non-immune cells. Thus, they can contribute to nourishing autoimmune responses and worsening tissue damage. In SLE, it has been described that NET-LL37-DNA complexes activated autoreactive B cells via TLR9, thereby stimulating autoantibody production (103).
Altogether, these mechanisms could explain how neutrophils can fuel autoimmune responses. However, neutrophils have also shown healing potential in some inflammatory diseases (104, 105). Indeed, the role of neutrophils in inflammatory bowel disease (IBD) appears to be paradoxical, as both beneficial and detrimental roles have been reported (23). An elevated number of circulating neutrophils has been reported in patients with IBD and can be associated with the severity of the disease (106). Similar observations were made for neutrophil-dependent mediators (107–109). Although unrestricted activation of neutrophils could trigger tissue damage and inflammation, neutrophils have been reported to be essential to maintain gut homeostasis (23). For instance, in a mouse model of colitis, neutrophils can contribute to the resolution of pathology through the production of TGFβ, which increases the levels of amphiregulin in intestinal epithelial cells (110). Moreover, neutrophils have been proposed to be a producer of IL-22, which is known to be protective in IBD (26). Consistently, a population of CD177+ neutrophils producing IL-22 and TGFβ negatively regulates IBD (25) (Figure 2E).
Neutrophil heterogeneity in systemic inflammation
Higher demand for neutrophils during systemic inflammation activates a process known as emergency granulopoiesis (see also above) (42). This phenomenon leads to the release in the circulation of immature neutrophils along with mature neutrophils, generating a mingling of populations with different features, including immunosuppressive properties (111). A part of this heterogeneous population has been empirically called low-density neutrophils (LDNs), based on sedimentation properties, to distinguish them from normal-density neutrophils (NDNs). Indeed, after density gradient sedimentation, LDNs are found in the peripheral mononuclear cell (PMBC) layer while NDNs are found on the top of the erythrocytes layer (111).
LDNs have been found in the peripheral blood of patients with a broad variety of acute and chronic inflammatory diseases, including cancer, HIV-1 infection, SARS-CoV-2 infections, sepsis, graft-versus-host disease (GvHD), trauma, or in healthy donors who received G-CSF treatment for stem cell mobilization, or hematopoietic stem cell transplantation (HSC-T) (112–114).
The presence and phenotype of the LDN population in these different contexts have been exhaustively reviewed (111, 112), but there are still open questions. Firstly, there are no specific markers for LDNs and in humans these cells are typically described as CD66b+ CD15+ CD14-/dim CD33dim HLA-DR-, a phenotypic profile identical to the one of NDNs (112). The lack of specific markers to distinguish LDNs from NDNs led to confusion and contrasting reports in the past decade (115). Recently, CD98 has been proposed as a marker of LDNs found in patients treated with G-CSF and can identify a subset of LDNs in SLE patients (116). CD98+ LDNs of SLE patients produced more proinflammatory cytokines and chemokines than NDNs and were resistant to apoptosis (116). Accordingly, the presence of CD98+ LDNs correlated with disease activity in SLE patients. Interestingly, the inhibition of CD98 reduced the metabolic flexibility of this population limiting their pathogenic capacity, thus identifying CD98 as a potential therapeutic target (116).
LDNs are generally endowed with immunosuppressive properties. Accordingly, these cells express Arginase 1 (Arg1), ROS or programmed cell death ligand 1 (PDL-1), all molecules involved in T cell suppression (115). However, neutrophils with proinflammatory properties in the LDN fraction have been reported in patients with SLE or RA (92, 100). Conversely, neutrophils with immunosuppressive features have been observed within NDNs (111, 117). Therefore, the functional properties of total LDN and NDN fractions remain redundant, and the contradictory reports could be the result of neutrophil heterogeneity.
LDNs represent a heterogeneous population of cells which can include the entire spectrum of neutrophil differentiation stages (11). Accordingly, LDNs can have different maturation stages and different functional properties. CD10 has been proposed as a marker to discriminate mature from immature neutrophils in inflammatory conditions. In G-CSF treated donors, CD10 identified two populations of LDNs with different immunological features (118). Accordingly, CD66b+ CD10- neutrophils were able to promote T cell survival, proliferation and IFNγ production, whilst CD66b+ CD10+ cells were shown to suppress T cell proliferation and IFNγ production in a CD18-mediated contact-dependent Arg1 release (118).
The extraordinary heterogeneity of LDNs could also be related to the fact that these cells are involved in different pathological contexts, spanning from infection to inflammatory disorders and HSC-T. Thus, it is conceivable that neutrophils are shaped by the specific inflammatory context giving rise to a spectrum of activation states.
A step toward a better understanding of the mechanisms allowing neutrophil diversity in humans has been recently achieved (11). The authors uncovered the diversity of human neutrophils in different settings, including G-CSF treatment, HSC-T, pancreatic ductal adenocarcinoma (PDAC) and SARS-CoV-2 syndrome. By combining multiparametric immunophenotyping, quantification of plasma cytokines, transcriptomic and computational analysis, they showed that stress-elicited neutrophils underwent profound changes in the expression dynamics of developmental genes, and they concomitantly acquired stimulus-specific gene signatures (11). Interestingly, the extent and the type of transcriptional response were dependent on neutrophil maturation stage, with the strongest dynamics observed in differentiated neutrophils. In the blood of patients receiving HSC-T, an acute IFN response has been identified. Interestingly, this response is translated into a significant upregulation of IFN-stimulated genes in neutrophils (11). These findings underlined the transcriptional plasticity of neutrophils in response to environmental cues and open to the possibility to use neutrophil transcriptome features as a biomarker.
Neutrophils in the resolution of inflammation and tissue repair
Tissue repair represents the physiological reaction that restores tissue homeostasis upon injury. The elimination of apoptotic neutrophils by macrophages contributes to the resolution of inflammation (119, 120). Efferocytosis not only eliminates dying neutrophils avoiding pro-inflammatory secondary necrosis but also actively induces a pro-resolving phenotype in macrophages. Macrophages after efferocytosis show increased production of anti-inflammatory cytokines (e.g., TGF-β and IL-10), decreased expression of pro-inflammatory mediators (e.g., TNFα, IL-12, IL-1β and IL-6) and increased biosynthesis of pro-resolving lipid mediators, including resolvin D1 (RvD1), RvD2, and RvE2 (22, 121). Beyond efferocytosis, additional pro-resolving neutrophil-mediated mechanisms have emerged. Apoptotic neutrophils express high levels of CCR5 that scavenges chemokines preventing further recruitment of inflammatory cells (122). Moreover, during the initial phases of inflammation, neutrophils can undergo a lipid mediator class switch upon PGE2 stimulation. The production of leukotrienes, which support inflammation, is shifted towards the synthesis of lipoxins (LX), which favor resolution by dampening the recruitment of neutrophils to the site of inflammation (123). Furthermore, extracellular vesicles released either by living or apoptotic neutrophils induce the production of pro-resolving lipid mediators and anti-inflammatory cytokines by macrophages, increasing their efferocytosis capacity (121). The protein annexin 1 (AnxA1) found expressed on neutrophil-derived microparticles has been implicated in this anti-inflammatory effect (124).
Neutrophils in cancer
Neutrophils have been found to infiltrate solid tumours, including non-small cell lung cancer (NSCLC), colorectal cancer (CRC), gastric cancer, hepatocellular carcinoma, melanoma, breast cancer, renal carcinoma, and sarcomas (9, 13, 21, 125–129). Although tumour infiltrating neutrophils (TANs) have been commonly associated with a poor prognosis, with few exceptions, the overall contribution of neutrophils in cancer is still unclear (9, 13).
Neutrophils in tumour promotion
Neutrophils can favour tumour development through direct mechanisms, by promoting genetic instability and cell proliferation, or by indirect mechanisms such as through the promotion of the metastatic spread or the inhibition of the anti-tumour immune response (130).
The promotion of genetic instability by neutrophils was firstly demonstrated in lung cells and linked to the production of ROS (131). This first observation has been confirmed by other studies, including in intestinal and lung cancer models, in which neutrophils have been suggested to induce tissue damage and genetic instability in a ROS-dependent manner (132, 133) (Figure 3A). In addition to ROS, recent findings showed that neutrophils promoted the accumulation of double-strand breaks (DSB) in the injured epithelium through the release of microRNAs (miR-23a and miR-155) (134) (Figure 3A).
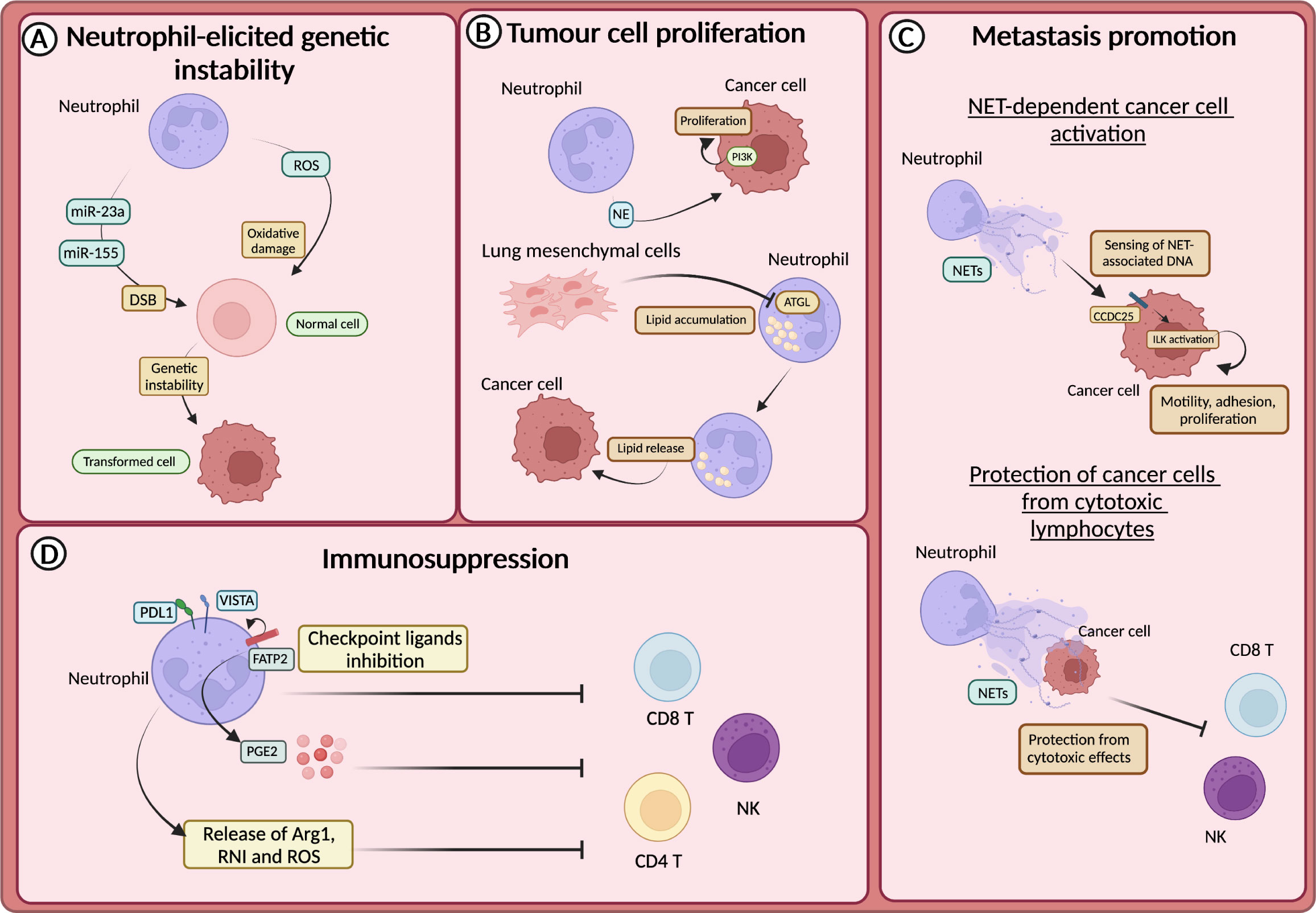
Figure 3 Neutrophils in tumour promotion (A) Neutrophil-derived reactive oxygen species (ROS) or miR23a and miR-155, by promoting the accumulation of double strand breaks (DSB), sustain genetic instability of cells. (B) Neutrophils sustain the proliferation of tumour cells through the release of neutrophils elastase (NE), and subsequent activation of PI3K. In the premetastatic niche, lung-resident mesenchymal cells forced neutrophils to accumulate lipids through the inhibition of the adipose triglyceride lipase (ATGL). Lipids are then released to feed tumour cells. (C) CCDC25 on tumour cells acts as a sensor for neutrophil extracellular trap (NET)-associated DNA and activates the integrin-linked kinase (ILK) signalling, promoting liver metastasis formation. NETs protect cancer cells from the cytotoxic activity of NK and CD8+ T cells. (D) Neutrophils drive immunosuppression and the dysfunction of T cells in various ways, including the expression of lymphocytes checkpoint (e.g., PD-L1 and VISTA), the production of ROS, arginase 1 (Arg1) or reactive nitrogen intermediate (RNI) and through fatty acid transporter protein 2 (FATP2)-dependent production of prostaglandin E2 (PGE2).
Neutrophils produce several mediators, including cytokines and growth factors that can fuel tumour growth (21, 135). It has been reported that mediators derived from neutrophil granules were involved in tumour promotion (136–138). For instance, NE can activate the proliferation of different cancer cells, including human oesophageal cell lines, mammary epithelial cells, human prostate cancer cells and human and mouse lung cancer cells (136–138). In this regard, it has been demonstrated that NE induced the degradation of the insulin receptor substrate 1 (IRS-1), which inhibits the phosphoinositide 3-kinase (PI3K) (Figure 3B). Thus, PI3K can interact with the PDGF receptor (PDGFR) and induce the proliferation of human and mouse lung cancer cells (136). Accordingly, in a model of lung adenocarcinoma driven by kras mutation, NE-deficient mice showed reduced tumour development (136).
Neutrophil metabolic reprogramming is another fascinating mechanism through which neutrophils feed tumour cells and promote metastasis (139). In the pre-metastatic niche, lung-resident mesenchymal cells co-opted neutrophils to accumulate neutral lipids via the repression of the adipose triglyceride lipase (ATGL). In turn, the transfer of lipids from neutrophils to tumour cells via a micropinocytosis-lysosome pathway dramatically potentiates their proliferative and survival capacity and metastatic potential (139) (Figure 3B).
The pro-metastatic activity of neutrophils is also achieved through the promotion of angiogenesis, the protection of circulating tumour cells (CTCs), the activation of dormant cancer cells and/or the recruitment of tumour cells in the pre-metastatic niche (140–142). For instance, neutrophil-derived mediators, such as Bv8 and MMP-9 are known for their pro-angiogenetic properties (141).
Clusters of neutrophils and CTCs were observed in the circulation of patients with breast cancer and mouse cancer models (143). Tumour cells within the cluster of neutrophil-CTCs displayed increased proliferative capacity and were more efficient in metastasis formation (143).
The release of NETs has long been associated with metastasis promotion. NETs have been observed in different types of tumours and participate in tumour-promoting inflammation, angiogenesis, extracellular matrix remodelling and proliferation of tumour cells (144–151). Several studies showed that NETs could support the formation of metastasis by entrapping CTCs and facilitating their seeding and proliferation in a distant anatomical site (144, 145). NETs can also act as a recruiting signal for CTCs expressing the coiled-coil domain containing protein-25 (CCDC25), which acts as an extracellular DNA sensor and can activate cell motility (151). Indeed, the engagement of CCDC25 by NET-associated DNA fostered the integrin-linked kinase (ILK) pathway leading to increased adhesion, motility, and growth of metastatic cancer cells in the liver (Figure 3C). Accordingly, the formation of metastasis in the liver and in the lung through this mechanism is reduced in CCDC25-deficient mice (151). NETs can also serve as a shield for CTCs and protect them from the cytotoxic activity of CD8+ T cells and natural killer (NK) cells (149). Notably, pharmacological inhibition of NETs formation synergized with anti-PD1 plus anti-CTLA4 treatment (149) (Figure 3C).
Neutrophils have long been associated with the suppression of the immune response, which can occur in several ways (152). For instance, neutrophils produce mediators that suppress the activity of T cells, such as Arg1, ROS, reactive nitrogen intermediate (RNI), and PGE2 (130) (Figure 3D). Endoplasmic reticulum (ER) stress associated with lipid metabolism alteration characterized neutrophils with immunosuppressive features (153). The expression of proteins involved in lipid trafficking and metabolism such as LOX-1, CD36 and fatty acid transport protein 2 (FATP2) were expressed by immunosuppressive neutrophils (154). In non-small cell lung cancer (NSCL) patients, neutrophils with ER stress response were observed (155). The activation of ER response induced the expression of LOX-1 on neutrophils, which are also characterized by high production of ROS and Arg1 production (155). In addition, FATP2 expression on neutrophils induced the biosynthesis of PGE2 and consequently an immunosuppressive activity (155). Indeed, PGE2 has been shown to impair cytotoxic activity and survival of NK cells and CD8+ T cells (156, 157) (Figure 3D).
The immunosuppressive activity of neutrophils can also be achieved by direct inhibition of T cells through the engagement of checkpoint molecules. Neutrophils expressing the programmed cell death 1 ligand 1 (PD-L1) or the V-domain immunoglobulin suppressor of T-cell activation (VISTA) were reported in human and mouse models of hepatocellular carcinoma, melanoma, and gastric cancer (158–162). In a murine model of melanoma, the blockade of VISTA induced a potent pro-inflammatory response in myeloid cells and reduced their immunosuppressive potential (162) (Figure 3D). However, the treatment has no effect on neutrophil-dependent immunosuppression, suggesting the role of VISTA on neutrophils could be different from other myeloid cells and requires further investigation.
Neutrophils in the anti-tumour response
Although most of the data from patients and mouse models support the pro-tumour role of neutrophils, a growing number of studies describe their anti-tumour activity (127–129, 163–165). It has long been known that neutrophils can directly kill tumour cells through the release of ROS or NO (135, 166–168) (Figure 4A). Neutrophils expressing TNF-related apoptosis-inducing ligand (TRAIL) displayed enhanced tumour cell killing in vitro in an IFNγ-dependent manner (169). Interestingly, tumour cell derived TNFα induced the expression of the hepatocyte growth factor receptor (HGFR or Met) on neutrophils, which in response to HGF expressed the inducible nitric oxide synthase (iNOS) and NO to kill cancer cells (170) (Figure 4A). Conversely, others have reported that HGF-Met signalling in neutrophils induced an immunosuppressive phenotype associated with the inhibition of T cells and reduced response to adoptive T cell transfer and checkpoint blockade therapies (171). Therefore, the role of Met and HGF on neutrophils remains to be fully elucidated.
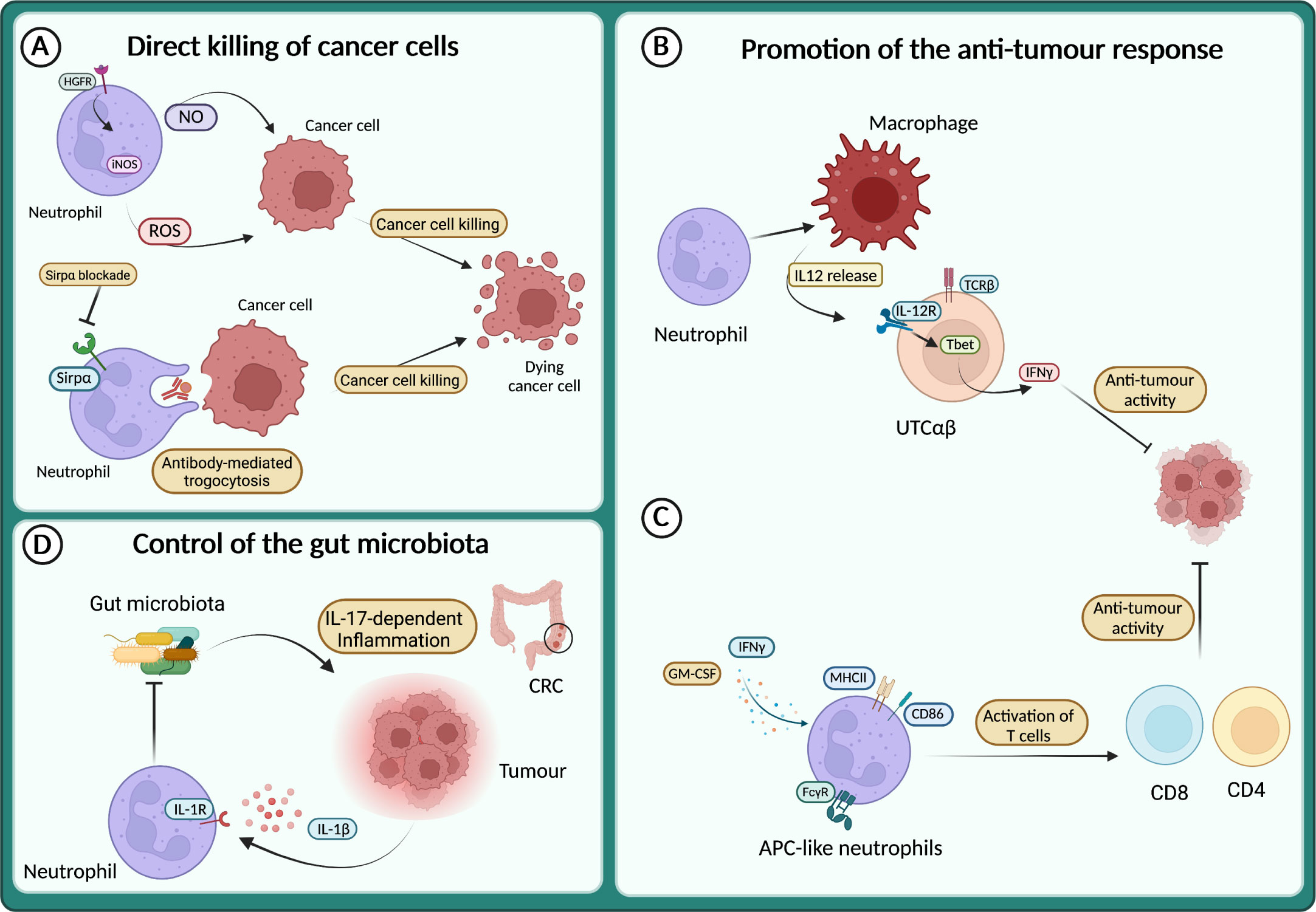
Figure 4 Neutrophils in the anti-tumour response (A) Neutrophils directly kill cancer cells through the release of reactive oxygen species (ROS) and NO or via antibody-mediated trogocytosis of antibody-opsonized cancer cells. (B) Neutrophils sustain IL-12 production by macrophages, which in turn activates a subset of unconventional αβ T cells (UTCαβ) to produce IFNγ and suppress tumour growth. (C) APC-like neutrophils can be induced in response to GM-CSF and IFNγ, or by FcγR-mediated endocytosis of antibody-antigen and can activate T cell anti-tumour response. (D) In response to IL-1β neutrophils restrict the invasion of gut microbiota and thus dampen microbiota-dependent inflammation in the context of colorectal cancer (CRC).
The direct killing of cancer cells by neutrophils can also be achieved through antibody-dependent cellular cytotoxicity (ADCC). Indeed, neutrophils can induce cell lysis via antibody-mediated trogocytosis of antibody-opsonized cancer cells (Figure 4A). Interestingly, blocking the CD47-Sirpα interaction enhanced cancer cell killing, suggesting that this pathway can be targeted to potentiate neutrophil cytotoxic activity (172). Recently, a cocktail consisting of TNFα, CD40 agonist and a tumour-specific antibody was shown to increase the ability of neutrophils to kill human tumour cells in vitro (173). In mouse models, the same combination stimulated the anti-tumour activity of neutrophils, leading to the eradication of established tumour and reduction of metastasis (173).
The neutrophil killing capacity can be potentiated also by the deletion of the atypical chemokine receptor 2 (ACKR2). Indeed, in models of breast cancer metastasis, genetic ablation of Ackr2 resulted in increased mobilization of myeloid cells, including neutrophils endowed with increased ROS-mediated cytotoxic activity (174).
Neutrophils can also act as triggers of the immune response against tumours, by recruiting and fostering the activity of other immune cells. For instance, neutrophils produce chemokines that recruit T cells or other leukocytes such as CXCL1, CXCL2, CXCL10, CCL2 and CCL3 (1). Moreover, neutrophils can engage crosstalk to activate T cells, as reported in the model of 3-methilcolantrene induced carcinogenesis (129). Here, neutrophils were essential to promote the production of IL-12 by macrophages, which in turn activated the anti-tumour activity of a subset of unconventional αβ T cells (UTCαβ) (Figure 4B).
Interestingly, a mechanism by which neutrophils can counteract carcinogenesis is represented by the acquisition of antigen-presenting cell (APC)-like phenotype. This APC-like behaviour of neutrophils has been observed in NSCL patients, where a subset of immature neutrophils (CD11b+ CD15hi CD10– CD16int/low) acquired the expression of MHCII and CD86 in response to GM-CSF and IFNγ and triggered a T cell-mediated anti-tumour response (175) (Figure 4C). Notably, FcγR-mediated endocytosis of antibody-antigen complexes converts neutrophils into a more potent APC-like cells (176) (Figure 4C). A more recent report showed the dual role of neutrophils in tumour-draining lymph nodes (LN) during head and neck cancer (HNC) progression (177). In these settings, neutrophils transmigrate and shape T cells activation in a stage-dependent manner, with neutrophils acquiring APC-like features and promoting T cell anti-tumour activity in metastasis-free patients, while at a later stage, neutrophils acquire PD-L1 expression and suppress T cell activation. Interestingly, the presence of neutrophils in LN can be used as a prognostic marker depending on the stage of the patients. In transplantable mouse models of cancer (i.e., lung adenocarcinoma and colorectal cancer), a recent study showed that neutrophils with anti-tumour properties were accumulated in tumours during successful immunotherapy with anti-PD-1 and anti-CD40 (178). In treated mice, the expanded clusters of neutrophils were characterized by a Sellhi phenotype and an interferon-stimulated gene signature. Of note, the neutrophil response requires the transcription factor IRF1 and a loss of its expression led to the failure of the treatment (178). In addition, another study showed that following melanoma-specific CD4+ T cell therapy combined with OX40 co-stimulation or anti-CTLA-4, complete tumour eradication was dependent on neutrophils killing of antigen-negative tumour cells. Interestingly, massive neutrophil activation was observed in mouse tumours and in biopsies from melanoma patients treated with immune checkpoint blockade (179).
The role of microbiota in cancer development is emerging and neutrophils have been involved in the control of microbiota in tumour context, including metastasis formation and response to therapy (180–184). For example, in a genetic mouse model of neutrophil deficiency (e.g., LysMCre;Mcl1fl/fl), enhanced intestinal tumour formation and IL-17-dependent inflammation due to increased intra-tumour bacteria have been reported (164) (Figure 4D). On the same line, blocking Il1r1 in neutrophils resulted in limiting their antibacterial potential and led to increased bacterial invasion of tumours and increased inflammation and cancer progression (165) (Figure 4D). In apparent contrast, in a lung cancer model, airway microbiota activated neutrophil release of cytokines. In turn, neutrophil-released cytokines induced the activation and proliferation of lung-resident γδ T cells that promoted inflammation and tumour growth (185).
Cancer-dependent neutrophil plasticity and heterogeneity
The results discussed above underlined the dual role of neutrophils in cancer. These controversial findings may be ascribed to the plasticity and the heterogeneity of neutrophils in the tumour context (9, 70). Indeed, a growing body of evidence challenged the view of neutrophils as short-lived effectors and poorly adaptive cells.
Cancer-dependent neutrophil perturbations, such as tumour-induced emergency granulopoiesis, are often observed during tumour progression (186–188). Indeed, neutrophil differentiation and maturation trajectories are dramatically altered in tumour-bearing mice and cancer patients, and multiple studies have reported the premature release of early neutrophil precursors and their progenitors into the peripheral blood (4, 6, 189). Neutrophilia can occur through the activation of the IL-17/G-CSF pathway. In a mouse model of breast cancer in KEP mice (i.e., K14cre; Cdh1fl/fl; Trp53fl/fl) secretion of WNT ligands induced IL-1β release from macrophages and induced the production of IL-17 by γδT cells (186, 188). IL-17 enhanced the production of G-CSF and the accumulation of neutrophils in the peripheral blood and in the lung.
Tumour-associated alterations of neutrophil metabolism, which are frequently associated with an immunosuppressive capacity, have been recently reported (see also above). The metabolic activity of neutrophils is generally based on glycolysis for their survival and function and a role for metabolic alterations in neutrophils is emerging in cancer (190). In a model of breast cancer, tumour-elicited neutrophils engage oxidative mitochondrial metabolism to maintain ROS production and suppress T cells (187). On the same line, a subset of LDNs found in the circulation of cancer patients was shown to promote liver metastasis by undergoing a metabolic shift (191). As discussed above, neutrophils in the pre-metastatic lung accumulate neutral lipids upon interaction with resident mesenchymal cells and consequently feed cancer cells by releasing the accumulated lipids (139).
The plasticity of neutrophils in cancer has been demonstrated in response to tumour derived TGFβ, which drives the polarization of neutrophils towards an immunosuppressive phenotype. In this activation state, called N2 to mirror the M1/M2 paradigm of macrophages, neutrophils produced proangiogenic factors (e.g., MMP-9) and inhibited CD8+ T cells activation through secretion of Arg1 (192). Conversely, N1 neutrophils were endowed with anti-tumour properties, including a cytotoxic activity towards tumour cells and the promotion of T cell recruitment and activation.
Another level of complexity of neutrophils heterogeneity in cancer is represented by myeloid-derived suppressor cells (MDSCs), which can be immature or mature myeloid cells (e.g., neutrophils and monocytes) endowed with immunosuppressive properties and are found in the circulation, the primary tumour, and metastases (193). MDSCs are functionally characterized by the ability to suppress T cell proliferation and activation in vitro (155). However, the phenotypic characterization of these cells is still controversial (194). Indeed, granulocyte MDSCs (G-MDSCs or PMN-MDSCs) are described as CD15+ CD66b+ CD33dim HLA-DR- in humans and as CD11b+ Ly6G+ CD84+ in mice, making them indistinguishable from other neutrophil subsets (193). Recently, a scRNAseq analysis performed on neutrophil subsets from tumour-bearing mice identified three populations of neutrophils: classic neutrophils, PMN-MDSCs and activated PMN-MDSCs, which showed potent immunosuppressive activity (195). PMN-MDSCs and activated PMN-MDSCs are found in tumours at early stages of tumorigenesis and acquired the expression of CD14, suggesting the use of this marker to distinguish classic neutrophils from PMN-MDSCs in mice.
PMN-MDSCs and N2 neutrophils share several characteristics, including phenotype and morphology, suppression of T cell activation and have been found in conditions of chronic inflammation, including cancer (161, 196–199). Therefore, the description of the different features of these two populations has become a new challenge in the study of neutrophil biology within tumour. Given their strict definition as immunosuppressive cells, we recommend the designation of MDSCs for populations with proven immunosuppressive activity.
Conclusions and perspectives
Neutrophils are central mediators of the innate immune response. Previously considered to be only a primary defence against invading pathogens, neutrophils have emerged as key players in many inflammatory conditions and immune-mediated diseases, including in cancer.
The role of neutrophils in these pathological contexts is often controversial, as they can exert both beneficial and detrimental functions. This dichotomous behaviour emerged clearly in the context of inflammatory diseases and cancer.
The implementation of high-throughput technologies, such as high-dimensional transcriptomic and epigenomic, and multi-dimensional cytometry, allowed the study of neutrophils at single-cell resolution. These gave rise to numerous studies revealing the heterogeneity and plasticity of neutrophils from their developmental to their effector functions. Thanks to these findings, we are now appreciating how neutrophils can rapidly respond to changes in the environment and adapt their behaviour.
Targeted therapies and immunotherapies are now the frontlines of modern medicine. In this context, it would be beneficial to develop new approaches to block the detrimental functions of neutrophils and promote their beneficial actions. Therefore, further investigations are needed to increase our understanding of the molecular drivers of neutrophil development and plasticity to open new therapeutic avenues.
Author contributions
SC, ID, GG, AR wrote the manuscript and prepare figures, these authors contributed equally to this work. EB and SJ revised the manuscript. All authors contributed to the article and approved the submitted version.
Acknowledgments
SC is recipient of a fellowship from the Italian Association for Cancer Research. Italian Ministry of Health- GR-2016-02361263 and GR-2018-12365588 to SJ, the Italian Association for Cancer Research AIRC IG-22815 to SJ and AIRC Start-Up-25828 to EB. Italian Ministry of University and Research - PRIN 2017K7FSYB to SJ, are gratefully acknowledged. Figures were created with Biorender.com.
Conflict of interest
The authors declare that the research was conducted in the absence of any commercial or financial relationships that could be construed as a potential conflict of interest.
Publisher’s note
All claims expressed in this article are solely those of the authors and do not necessarily represent those of their affiliated organizations, or those of the publisher, the editors and the reviewers. Any product that may be evaluated in this article, or claim that may be made by its manufacturer, is not guaranteed or endorsed by the publisher.
References
1. Mantovani A, Cassatella MA, Costantini C, Jaillon S. Neutrophils in the activation and regulation of innate and adaptive immunity. Nat Rev Immunol (2011) 11(8):519–31. doi: 10.1038/nri3024
2. Hidalgo A, Chilvers ER, Summers C, Koenderman L. The neutrophil life cycle. Trends Immunol (2019) 40(7):584–97. doi: 10.1016/j.it.2019.04.013
3. Ballesteros I, Rubio-Ponce A, Genua M, Lusito E, Kwok I, Fernandez-Calvo G, et al. Co-Option of neutrophil fates by tissue environments. Cell (2020) 183(5):1282–97.e18. doi: 10.1016/j.cell.2020.10.003
4. Evrard M, Kwok IWH, Chong SZ, Teng KWW, Becht E, Chen J, et al. Developmental analysis of bone marrow neutrophils reveals populations specialized in expansion, trafficking, and effector functions. Immunity (2018) 48(2):364–79.e8. doi: 10.1016/j.immuni.2018.02.002
5. Kwok I, Becht E, Xia Y, Ng M, Teh YC, Tan L, et al. Combinatorial single-cell analyses of granulocyte-monocyte progenitor heterogeneity reveals an early uni-potent neutrophil progenitor. Immunity (2020) 53(2):303–318.e5. doi: 10.1016/j.immuni.2020.06.005
6. Zhu YP, Padgett L, Dinh HQ, Marcovecchio P, Blatchley A, Wu R, et al. Identification of an early unipotent neutrophil progenitor with pro-tumoral activity in mouse and human bone marrow. Cell Rep (2018) 24(9):2329–41.e8. doi: 10.1016/j.celrep.2018.07.097
7. Giladi A, Paul F, Herzog Y, Lubling Y, Weiner A, Yofe I, et al. Single-cell characterization of haematopoietic progenitors and their trajectories in homeostasis and perturbed haematopoiesis. Nat Cell Biol (2018) 20(7):836–46. doi: 10.1038/s41556-018-0121-4
8. Palomino-Segura M, Sicilia J, Ballesteros I, Hidalgo A. Strategies of neutrophil diversification. Nat Immunol (2023) 24(4):575–84. doi: 10.1038/s41590-023-01452-x
9. Jaillon S, Ponzetta A, Di Mitri D, Santoni A, Bonecchi R, Mantovani A. Neutrophil diversity and plasticity in tumour progression and therapy. Nat Rev Cancer. (2020) 20(9):485–503. doi: 10.1038/s41568-020-0281-y
10. Grieshaber-Bouyer R, Radtke FA, Cunin P, Stifano G, Levescot A, Vijaykumar B, et al. The neutrotime transcriptional signature defines a single continuum of neutrophils across biological compartments. Nat Commun (2021) 12(1):2856. doi: 10.1038/s41467-021-22973-9
11. Montaldo E, Lusito E, Bianchessi V, Caronni N, Scala S, Basso-Ricci L, et al. Cellular and transcriptional dynamics of human neutrophils at steady state and upon stress. Nat Immunol (2022) 23(10):1470–83. doi: 10.1038/s41590-022-01311-1
12. Borregaard N. Neutrophils, from marrow to microbes. Immunity (2010) 33(5):657–70. doi: 10.1016/j.immuni.2010.11.011
13. Carnevale S, Ghasemi S, Rigatelli A, Jaillon S. The complexity of neutrophils in health and disease: focus on cancer. Semin Immunol (2020) 48:101409. doi: 10.1016/j.smim.2020.101409
14. Wang J. Neutrophils in tissue injury and repair. Cell Tissue Res (2018) 371(3):531–9. doi: 10.1007/s00441-017-2785-7
15. Soehnlein O, Steffens S, Hidalgo A, Weber C. Neutrophils as protagonists and targets in chronic inflammation. Nat Rev Immunol (2017) 17(4):248–61. doi: 10.1038/nri.2017.10
16. Garcia-Romo GS, Caielli S, Vega B, Connolly J, Allantaz F, Xu Z, et al. Netting neutrophils are major inducers of type I IFN production in pediatric systemic lupus erythematosus. Sci Transl Med (2011) 3(73):73ra20. doi: 10.1126/scitranslmed.3001201
17. Herrero-Cervera A, Soehnlein O, Kenne E. Neutrophils in chronic inflammatory diseases. Cell Mol Immunol (2022) 19(2):177–91. doi: 10.1038/s41423-021-00832-3
18. Liu J, Liu Y, Xiang P, Pu L, Xiong H, Li C, et al. Neutrophil-to-lymphocyte ratio predicts critical illness patients with 2019 coronavirus disease in the early stage. J Transl Med (2020) 18(1):206. doi: 10.1186/s12967-020-02374-0
19. Perez-Sanchez C, Ruiz-Limon P, Aguirre MA, Jimenez-Gomez Y, Arias-de la Rosa I, Abalos-Aguilera MC, et al. Diagnostic potential of NETosis-derived products for disease activity, atherosclerosis and therapeutic effectiveness in rheumatoid arthritis patients. J Autoimmun (2017) 82:31–40. doi: 10.1016/j.jaut.2017.04.007
20. Phillipson M, Kubes P. The neutrophil in vascular inflammation. Nat Med (2011) 17(11):1381–90. doi: 10.1038/nm.2514
21. Shaul ME, Fridlender ZG. Tumour-associated neutrophils in patients with cancer. Nat Rev Clin Oncol (2019) 16(10):601–20. doi: 10.1038/s41571-019-0222-4
22. Loh W, Vermeren S. Anti-inflammatory neutrophil functions in the resolution of inflammation and tissue repair. Cells (2022) 11(24):4076. doi: 10.3390/cells11244076
23. Salas A. What good can neutrophils do in UC? Gut (2022) 71(12):2375–6. doi: 10.1136/gutjnl-2021-326484
24. Wang X, Cai J, Lin B, Ma M, Tao Y, Zhou Y, et al. GPR34-mediated sensing of lysophosphatidylserine released by apoptotic neutrophils activates type 3 innate lymphoid cells to mediate tissue repair. Immunity (2021) 54(6):1123–36.e8. doi: 10.1016/j.immuni.2021.05.007
25. Zhou G, Yu L, Fang L, Yang W, Yu T, Miao Y, et al. CD177(+) neutrophils as functionally activated neutrophils negatively regulate IBD. Gut (2018) 67(6):1052–63. doi: 10.1136/gutjnl-2016-313535
26. Zindl CL, Lai JF, Lee YK, Maynard CL, Harbour SN, Ouyang W, et al. IL-22-producing neutrophils contribute to antimicrobial defense and restitution of colonic epithelial integrity during colitis. Proc Natl Acad Sci U S A (2013) 110(31):12768–73. doi: 10.1073/pnas.1300318110
27. Seignez C, Phillipson M. The multitasking neutrophils and their involvement in angiogenesis. Curr Opin Hematol (2017) 24(1):3–8. doi: 10.1097/MOH.0000000000000300
28. Lawrence SM, Corriden R, Nizet V. The ontogeny of a neutrophil: mechanisms of granulopoiesis and homeostasis. Microbiol Mol Biol Rev (2018) 82(1):e00057–17. doi: 10.1128/MMBR.00057-17
29. Yvan-Charvet L, Ng LG. Granulopoiesis and neutrophil homeostasis: a metabolic, daily balancing act. Trends Immunol (2019) 40(7):598–612. doi: 10.1016/j.it.2019.05.004
30. Liu F, Wu HY, Wesselschmidt R, Kornaga T, Link DC. Impaired production and increased apoptosis of neutrophils in granulocyte colony-stimulating factor receptor-deficient mice. Immunity (1996) 5(5):491–501. doi: 10.1016/S1074-7613(00)80504-X
31. Walker F, Zhang HH, Matthews V, Weinstock J, Nice EC, Ernst M, et al. IL6/sIL6R complex contributes to emergency granulopoietic responses in G-CSF- and GM-CSF-deficient mice. Blood (2008) 111(8):3978–85. doi: 10.1182/blood-2007-10-119636
32. Bartels M, Govers AM, Fleskens V, Lourenco AR, Pals CE, Vervoort SJ, et al. Acetylation of C/EBPepsilon is a prerequisite for terminal neutrophil differentiation. Blood (2015) 125(11):1782–92. doi: 10.1182/blood-2013-12-543850
33. Doulatov S, Notta F, Eppert K, Nguyen LT, Ohashi PS, Dick JE. Revised map of the human progenitor hierarchy shows the origin of macrophages and dendritic cells in early lymphoid development. Nat Immunol (2010) 11(7):585–93. doi: 10.1038/ni.1889
34. Hattori T, Ohoka N, Inoue Y, Hayashi H, Onozaki K. C/EBP family transcription factors are degraded by the proteasome but stabilized by forming dimer. Oncogene (2003) 22(9):1273–80. doi: 10.1038/sj.onc.1206204
35. Ohlsson E, Schuster MB, Hasemann M, Porse BT. The multifaceted functions of C/EBPalpha in normal and malignant haematopoiesis. Leukemia (2016) 30(4):767–75. doi: 10.1038/leu.2015.324
36. Hock H, Hamblen MJ, Rooke HM, Traver D, Bronson RT, Cameron S, et al. Intrinsic requirement for zinc finger transcription factor gfi-1 in neutrophil differentiation. Immunity (2003) 18(1):109–20. doi: 10.1016/S1074-7613(02)00501-0
37. Yamanaka R, Barlow C, Lekstrom-Himes J, Castilla LH, Liu PP, Eckhaus M, et al. Impaired granulopoiesis, myelodysplasia, and early lethality in CCAAT/enhancer binding protein epsilon-deficient mice. Proc Natl Acad Sci U S A (1997) 94(24):13187–92. doi: 10.1073/pnas.94.24.13187
38. Summers C, Rankin SM, Condliffe AM, Singh N, Peters AM, Chilvers ER. Neutrophil kinetics in health and disease. Trends Immunol (2010) 31(8):318–24. doi: 10.1016/j.it.2010.05.006
39. Brostjan C, Oehler R. The role of neutrophil death in chronic inflammation and cancer. Cell Death Discovery (2020) 6:26. doi: 10.1038/s41420-020-0255-6
40. Prame Kumar K, Nicholls AJ, Wong CHY. Partners in crime: neutrophils and monocytes/macrophages in inflammation and disease. Cell Tissue Res (2018) 371(3):551–65. doi: 10.1007/s00441-017-2753-2
41. Boettcher S, Manz MG. Regulation of inflammation- and infection-driven hematopoiesis. Trends Immunol (2017) 38(5):345–57. doi: 10.1016/j.it.2017.01.004
42. Manz MG, Boettcher S. Emergency granulopoiesis. Nat Rev Immunol (2014) 14(5):302–14. doi: 10.1038/nri3660
43. Calzetti F, Finotti G, Tamassia N, Bianchetto-Aguilera F, Castellucci M, Cane S, et al. CD66b(-)CD64(dim)CD115(-) cells in the human bone marrow represent neutrophil-committed progenitors. Nat Immunol (2022) 23(5):679–91. doi: 10.1038/s41590-022-01189-z
44. Eash KJ, Greenbaum AM, Gopalan PK, Link DC. CXCR2 and CXCR4 antagonistically regulate neutrophil trafficking from murine bone marrow. J Clin Invest (2010) 120(7):2423–31. doi: 10.1172/JCI41649
45. Luis TC, Killmann NM, Staal FJ. Signal transduction pathways regulating hematopoietic stem cell biology: introduction to a series of spotlight reviews. Leukemia (2012) 26(1):86–90. doi: 10.1038/leu.2011.260
46. Adrover JM, Del Fresno C, Crainiciuc G, Cuartero MI, Casanova-Acebes M, Weiss LA, et al. A neutrophil timer coordinates immune defense and vascular protection. Immunity (2019) 50(2):390–402.e10. doi: 10.1016/j.immuni.2019.01.002
47. Casanova-Acebes M, Nicolas-Avila JA, Li JL, Garcia-Silva S, Balachander A, Rubio-Ponce A, et al. Neutrophils instruct homeostatic and pathological states in naive tissues. J Exp Med (2018) 215(11):2778–95. doi: 10.1084/jem.20181468
48. Zarbock A, Ley K. Neutrophil adhesion and activation under flow. Microcirculation (2009) 16(1):31–42. doi: 10.1080/10739680802350104
49. Kolaczkowska E, Kubes P. Neutrophil recruitment and function in health and inflammation. Nat Rev Immunol (2013) 13(3):159–75. doi: 10.1038/nri3399
50. Capucetti A, Albano F, Bonecchi R. Multiple roles for chemokines in neutrophil biology. Front Immunol (2020) 11:1259. doi: 10.3389/fimmu.2020.01259
51. Zarbock A, Ley K, McEver RP, Hidalgo A. Leukocyte ligands for endothelial selectins: specialized glycoconjugates that mediate rolling and signaling under flow. Blood (2011) 118(26):6743–51. doi: 10.1182/blood-2011-07-343566
52. Phillipson M, Heit B, Colarusso P, Liu L, Ballantyne CM, Kubes P. Intraluminal crawling of neutrophils to emigration sites: a molecularly distinct process from adhesion in the recruitment cascade. J Exp Med (2006) 203(12):2569–75. doi: 10.1084/jem.20060925
53. Nourshargh S, Renshaw SA, Imhof BA. Reverse migration of neutrophils: where, when, how, and why? Trends Immunol (2016) 37(5):273–86. doi: 10.1016/j.it.2016.03.006
54. de Oliveira S, Rosowski EE, Huttenlocher A. Neutrophil migration in infection and wound repair: going forward in reverse. Nat Rev Immunol (2016) 16(6):378–91. doi: 10.1038/nri.2016.49
55. Jaillon S, Galdiero MR, Del Prete D, Cassatella MA, Garlanda C, Mantovani A. Neutrophils in innate and adaptive immunity. Semin Immunopathol (2013) 35(4):377–94. doi: 10.1007/s00281-013-0374-8
56. Gierlikowska B, Stachura A, Gierlikowski W, Demkow U. Phagocytosis, degranulation and extracellular traps release by neutrophils-the current knowledge, pharmacological modulation and future prospects. Front Pharmacol (2021) 12:666732. doi: 10.3389/fphar.2021.666732
57. Griffin GK, Newton G, Tarrio ML, Bu DX, Maganto-Garcia E, Azcutia V, et al. IL-17 and TNF-alpha sustain neutrophil recruitment during inflammation through synergistic effects on endothelial activation. J Immunol (2012) 188(12):6287–99. doi: 10.4049/jimmunol.1200385
58. Tecchio C, Micheletti A, Cassatella MA. Neutrophil-derived cytokines: facts beyond expression. Front Immunol (2014) 5:508. doi: 10.3389/fimmu.2014.00508
59. Burn GL, Foti A, Marsman G, Patel DF, Zychlinsky A. The neutrophil. Immunity (2021) 54(7):1377–91. doi: 10.1016/j.immuni.2021.06.006
60. Amulic B, Cazalet C, Hayes GL, Metzler KD, Zychlinsky A. Neutrophil function: from mechanisms to disease. Annu Rev Immunol (2012) 30:459–89. doi: 10.1146/annurev-immunol-020711-074942
61. Sheshachalam A, Srivastava N, Mitchell T, Lacy P, Eitzen G. Granule protein processing and regulated secretion in neutrophils. Front Immunol (2014) 5:448. doi: 10.3389/fimmu.2014.00448
62. Huang H, Zhang H, Onuma AE, Tsung A. Neutrophil elastase and neutrophil extracellular traps in the tumor microenvironment. Adv Exp Med Biol (2020) 1263:13–23. doi: 10.1007/978-3-030-44518-8_2
63. Jaillon S, Peri G, Delneste Y, Fremaux I, Doni A, Moalli F, et al. The humoral pattern recognition receptor PTX3 is stored in neutrophil granules and localizes in extracellular traps. J Exp Med (2007) 204(4):793–804. doi: 10.1084/jem.20061301
64. Cowland JB, Borregaard N. Granulopoiesis and granules of human neutrophils. Immunol Rev (2016) 273(1):11–28. doi: 10.1111/imr.12440
65. Brinkmann V, Reichard U, Goosmann C, Fauler B, Uhlemann Y, Weiss DS, et al. Neutrophil extracellular traps kill bacteria. Science (2004) 303(5663):1532–5. doi: 10.1126/science.1092385
66. Papayannopoulos V. Neutrophil extracellular traps in immunity and disease. Nat Rev Immunol (2018) 18(2):134–47. doi: 10.1038/nri.2017.105
67. Tamassia N, Bianchetto-Aguilera F, Arruda-Silva F, Gardiman E, Gasperini S, Calzetti F, et al. Cytokine production by human neutrophils: revisiting the "dark side of the moon". Eur J Clin Invest (2018) 48 Suppl 2:e12952. doi: 10.1111/eci.12952
68. George ST, Lai J, Ma J, Stacey HD, Miller MS, Mullarkey CE. Neutrophils and influenza: a thin line between helpful and harmful. Vaccines (Basel) (2021) 9(6):597. doi: 10.3390/vaccines9060597
69. Witko-Sarsat V, Rieu P, Descamps-Latscha B, Lesavre P, Halbwachs-Mecarelli L. Neutrophils: molecules, functions and pathophysiological aspects. Lab Invest (2000) 80(5):617–53. doi: 10.1038/labinvest.3780067
70. Ng LG, Ostuni R, Hidalgo A. Heterogeneity of neutrophils. Nat Rev Immunol (2019) 19(4):255–65. doi: 10.1038/s41577-019-0141-8
71. Casanova-Acebes M, Pitaval C, Weiss LA, Nombela-Arrieta C, Chevre R, AG N, et al. Rhythmic modulation of the hematopoietic niche through neutrophil clearance. Cell (2013) 153(5):1025–35. doi: 10.1016/j.cell.2013.04.040
72. Xie X, Shi Q, Wu P, Zhang X, Kambara H, Su J, et al. Single-cell transcriptome profiling reveals neutrophil heterogeneity in homeostasis and infection. Nat Immunol (2020) 21(9):1119–33. doi: 10.1038/s41590-020-0736-z
73. Zhang D, Chen G, Manwani D, Mortha A, Xu C, Faith JJ, et al. Neutrophil ageing is regulated by the microbiome. Nature (2015) 525(7570):528–32. doi: 10.1038/nature15367
74. Adrover JM, Aroca-Crevillen A, Crainiciuc G, Ostos F, Rojas-Vega Y, Rubio-Ponce A, et al. Programmed 'disarming' of the neutrophil proteome reduces the magnitude of inflammation. Nat Immunol (2020) 21(2):135–44. doi: 10.1038/s41590-019-0571-2
75. Devi S, Wang Y, Chew WK, Lima R, AG N, Mattar CN, et al. Neutrophil mobilization via plerixafor-mediated CXCR4 inhibition arises from lung demargination and blockade of neutrophil homing to the bone marrow. J Exp Med (2013) 210(11):2321–36. doi: 10.1084/jem.20130056
76. Lok LSC, Dennison TW, Mahbubani KM, Saeb-Parsy K, Chilvers ER, Clatworthy MR. Phenotypically distinct neutrophils patrol uninfected human and mouse lymph nodes. Proc Natl Acad Sci U S A (2019) 116(38):19083–9. doi: 10.1073/pnas.1905054116
77. Nicolas-Avila JA, Adrover JM, Hidalgo A. Neutrophils in homeostasis, immunity, and cancer. Immunity (2017) 46(1):15–28. doi: 10.1016/j.immuni.2016.12.012
78. Puga I, Cols M, Barra CM, He B, Cassis L, Gentile M, et al. B cell-helper neutrophils stimulate the diversification and production of immunoglobulin in the marginal zone of the spleen. Nat Immunol (2011) 13(2):170–80. doi: 10.1038/ni.2194
79. Bowers E, Slaughter A, Frenette PS, Kuick R, Pello OM, Lucas D. Granulocyte-derived TNFalpha promotes vascular and hematopoietic regeneration in the bone marrow. Nat Med (2018) 24(1):95–102. doi: 10.1038/nm.4448
80. Chen X, Deng H, Churchill MJ, Luchsinger LL, Du X, Chu TH, et al. Bone marrow myeloid cells regulate myeloid-biased hematopoietic stem cells via a histamine-dependent feedback loop. Cell Stem Cell (2017) 21(6):747–60.e7. doi: 10.1016/j.stem.2017.11.003
81. Kawano Y, Fukui C, Shinohara M, Wakahashi K, Ishii S, Suzuki T, et al. G-CSF-induced sympathetic tone provokes fever and primes antimobilizing functions of neutrophils via PGE2. Blood (2017) 129(5):587–97. doi: 10.1182/blood-2016-07-725754
82. Jaillon S, Ponzetta A, Magrini E, Barajon I, Barbagallo M, Garlanda C, et al. Fluid phase recognition molecules in neutrophil-dependent immune responses. Semin Immunol (2016) 28(2):109–18. doi: 10.1016/j.smim.2016.03.005
83. Jose RJ, Manuel A. COVID-19 cytokine storm: the interplay between inflammation and coagulation. Lancet Respir Med (2020) 8(6):e46–e7. doi: 10.1016/S2213-2600(20)30216-2
84. McKenna E, Wubben R, Isaza-Correa JM, Melo AM, Mhaonaigh AU, Conlon N, et al. Neutrophils in COVID-19: not innocent bystanders. Front Immunol (2022) 13:864387. doi: 10.3389/fimmu.2022.864387
85. Carissimo G, Xu W, Kwok I, Abdad MY, Chan YH, Fong SW, et al. Whole blood immunophenotyping uncovers immature neutrophil-to-VD2 T-cell ratio as an early marker for severe COVID-19. Nat Commun (2020) 11(1):5243. doi: 10.1038/s41467-020-19080-6
86. Li L, Li J, Gao M, Fan H, Wang Y, Xu X, et al. Interleukin-8 as a biomarker for disease prognosis of coronavirus disease-2019 patients. Front Immunol (2020) 11:602395. doi: 10.3389/fimmu.2020.602395
87. Silvin A, Chapuis N, Dunsmore G, Goubet AG, Dubuisson A, Derosa L, et al. Elevated calprotectin and abnormal myeloid cell subsets discriminate severe from mild COVID-19. Cell (2020) 182(6):1401–18.e18. doi: 10.1016/j.cell.2020.08.002
88. Middleton EA, He XY, Denorme F, Campbell RA, Ng D, Salvatore SP, et al. Neutrophil extracellular traps contribute to immunothrombosis in COVID-19 acute respiratory distress syndrome. Blood (2020) 136(10):1169–79. doi: 10.1182/blood.2020007008
89. Fu X, Liu H, Huang G, Dai SS. The emerging role of neutrophils in autoimmune-associated disorders: effector, predictor, and therapeutic targets. MedComm (2020) (2021) 2(3):402–13. doi: 10.1002/mco2.69
90. Ma S, Jiang W, Zhang X, Liu W. Insights into the pathogenic role of neutrophils in systemic lupus erythematosus. Curr Opin Rheumatol (2023) 35(2):82–8. doi: 10.1097/BOR.0000000000000912
91. Wang L, Luqmani R, Udalova IA. The role of neutrophils in rheumatic disease-associated vascular inflammation. Nat Rev Rheumatol (2022) 18(3):158–70. doi: 10.1038/s41584-021-00738-4
92. Villanueva E, Yalavarthi S, Berthier CC, Hodgin JB, Khandpur R, Lin AM, et al. Netting neutrophils induce endothelial damage, infiltrate tissues, and expose immunostimulatory molecules in systemic lupus erythematosus. J Immunol (2011) 187(1):538–52. doi: 10.4049/jimmunol.1100450
93. Wu S, Peng W, Zhang Y, Guo J, Fu J, Wang W. Correlation of PMN elastase and PMN elastase-to-neutrophil ratio with disease activity in patients with myositis. J Transl Med (2019) 17(1):420. doi: 10.1186/s12967-019-02176-z
94. Gao S, Zuo X, Liu D, Xiao Y, Zhu H, Zhang H, et al. The roles of neutrophil serine proteinases in idiopathic inflammatory myopathies. Arthritis Res Ther (2018) 20(1):134. doi: 10.1186/s13075-018-1632-x
95. Hammer HB, Fagerhol MK, Wien TN, Kvien TK. The soluble biomarker calprotectin (an S100 protein) is associated to ultrasonographic synovitis scores and is sensitive to change in patients with rheumatoid arthritis treated with adalimumab. Arthritis Res Ther (2011) 13(5):R178. doi: 10.1186/ar3503
96. Kessenbrock K, Krumbholz M, Schonermarck U, Back W, Gross WL, Werb Z, et al. Netting neutrophils in autoimmune small-vessel vasculitis. Nat Med (2009) 15(6):623–5. doi: 10.1038/nm.1959
97. Khandpur R, Carmona-Rivera C, Vivekanandan-Giri A, Gizinski A, Yalavarthi S, Knight JS, et al. NETs are a source of citrullinated autoantigens and stimulate inflammatory responses in rheumatoid arthritis. Sci Transl Med (2013) 5(178):178ra40. doi: 10.1126/scitranslmed.3005580
98. Seto N, Torres-Ruiz JJ, Carmona-Rivera C, Pinal-Fernandez I, Pak K, Purmalek MM, et al. Neutrophil dysregulation is pathogenic in idiopathic inflammatory myopathies. JCI Insight (2020) 5(3):e134189. doi: 10.1172/jci.insight.134189
99. Gupta S, Kaplan MJ. The role of neutrophils and NETosis in autoimmune and renal diseases. Nat Rev Nephrol (2016) 12(7):402–13. doi: 10.1038/nrneph.2016.71
100. Carmona-Rivera C, Zhao W, Yalavarthi S, Kaplan MJ. Neutrophil extracellular traps induce endothelial dysfunction in systemic lupus erythematosus through the activation of matrix metalloproteinase-2. Ann Rheum Dis (2015) 74(7):1417–24. doi: 10.1136/annrheumdis-2013-204837
101. O'Neil LJ, Barrera-Vargas A, Sandoval-Heglund D, Merayo-Chalico J, Aguirre-Aguilar E, Aponte AM, et al. Neutrophil-mediated carbamylation promotes articular damage in rheumatoid arthritis. Sci Adv (2020) 6(44):eabd2688. doi: 10.1126/sciadv.abd2688
102. Sangaletti S, Tripodo C, Chiodoni C, Guarnotta C, Cappetti B, Casalini P, et al. Neutrophil extracellular traps mediate transfer of cytoplasmic neutrophil antigens to myeloid dendritic cells toward ANCA induction and associated autoimmunity. Blood (2012) 120(15):3007–18. doi: 10.1182/blood-2012-03-416156
103. Gestermann N, Di Domizio J, Lande R, Demaria O, Frasca L, Feldmeyer L, et al. Netting neutrophils activate autoreactive b cells in lupus. J Immunol (2018) 200(10):3364–71. doi: 10.4049/jimmunol.1700778
104. Chen H, Wu X, Xu C, Lin J, Liu Z. Dichotomous roles of neutrophils in modulating pathogenic and repair processes of inflammatory bowel diseases. Precis Clin Med (2021) 4(4):246–57. doi: 10.1093/pcmedi/pbab025
105. Headland SE, Jones HR, Norling LV, Kim A, Souza PR, Corsiero E, et al. Neutrophil-derived microvesicles enter cartilage and protect the joint in inflammatory arthritis. Sci Transl Med (2015) 7(315):315ra190. doi: 10.1126/scitranslmed.aac5608
106. Bressenot A, Peyrin-Biroulet L. Histologic features predicting postoperative crohn's disease recurrence. Inflammation Bowel Dis (2015) 21(2):468–75. doi: 10.1097/MIB.0000000000000224
107. Li Y, Zhu L, Chu Z, Yang T, Sun HX, Yang F, et al. Characterization and biological significance of IL-23-induced neutrophil polarization. Cell Mol Immunol (2018) 15(5):518–30. doi: 10.1038/cmi.2017.39
108. Wang Y, Wang K, Han GC, Wang RX, Xiao H, Hou CM, et al. Neutrophil infiltration favors colitis-associated tumorigenesis by activating the interleukin-1 (IL-1)/IL-6 axis. Mucosal Immunol (2014) 7(5):1106–15. doi: 10.1038/mi.2013.126
109. Zhu F, He H, Fan L, Ma C, Xu Z, Xue Y, et al. Blockade of CXCR2 suppresses proinflammatory activities of neutrophils in ulcerative colitis. Am J Transl Res (2020) 12(9):5237–51.
110. Chen F, Yang W, Huang X, Cao AT, Bilotta AJ, Xiao Y, et al. Neutrophils promote amphiregulin production in intestinal epithelial cells through TGF-beta and contribute to intestinal homeostasis. J Immunol (2018) 201(8):2492–501. doi: 10.4049/jimmunol.1800003
111. Silvestre-Roig C, Fridlender ZG, Glogauer M, Scapini P. Neutrophil diversity in health and disease. Trends Immunol (2019) 40(7):565–83. doi: 10.1016/j.it.2019.04.012
112. Scapini P, Marini O, Tecchio C, Cassatella MA. Human neutrophils in the saga of cellular heterogeneity: insights and open questions. Immunol Rev (2016) 273(1):48–60. doi: 10.1111/imr.12448
113. Morrissey SM, Geller AE, Hu X, Tieri D, Ding C, Klaes CK, et al. A specific low-density neutrophil population correlates with hypercoagulation and disease severity in hospitalized COVID-19 patients. JCI Insight (2021) 6(9):e148435. doi: 10.1172/jci.insight.148435
114. Matthews NC, Burton CS, Alfred A. Low-density neutrophils in chronic graft versus host disease (cGVHD) are primarily immature CD10(-) and enhance T cell activation. Clin Exp Immunol (2021) 205(2):257–73. doi: 10.1111/cei.13612
115. Jablonska J, Granot Z. Neutrophil, quo vadis? J Leukoc Biol (2017) 102(3):685–8. doi: 10.1189/jlb.3MR0117-015R
116. Martin KR, Day JA, Hansen JA, D'Silva DB, Wong HL, Garnham A, et al. CD98 defines a metabolically flexible, proinflammatory subset of low-density neutrophils in systemic lupus erythematosus. Clin Transl Med (2023) 13(1):e1150. doi: 10.1002/ctm2.1150
117. Tsuda Y, Fukui H, Asai A, Fukunishi S, Miyaji K, Fujiwara S, et al. An immunosuppressive subtype of neutrophils identified in patients with hepatocellular carcinoma. J Clin Biochem Nutr (2012) 51(3):204–12. doi: 10.3164/jcbn.12-32
118. Marini O, Costa S, Bevilacqua D, Calzetti F, Tamassia N, Spina C, et al. Mature CD10(+) and immature CD10(-) neutrophils present in G-CSF-treated donors display opposite effects on T cells. Blood (2017) 129(10):1343–56. doi: 10.1182/blood-2016-04-713206
119. Doran AC, Yurdagul A Jr., Tabas I. Efferocytosis in health and disease. Nat Rev Immunol (2020) 20(4):254–67. doi: 10.1038/s41577-019-0240-6
120. Kourtzelis I, Hajishengallis G, Chavakis T. Phagocytosis of apoptotic cells in resolution of inflammation. Front Immunol (2020) 11:553. doi: 10.3389/fimmu.2020.00553
121. Dalli J, Serhan CN. Specific lipid mediator signatures of human phagocytes: microparticles stimulate macrophage efferocytosis and pro-resolving mediators. Blood (2012) 120(15):e60–72. doi: 10.1182/blood-2012-04-423525
122. Ariel A, Fredman G, Sun YP, Kantarci A, Van Dyke TE, Luster AD, et al. Apoptotic neutrophils and T cells sequester chemokines during immune response resolution through modulation of CCR5 expression. Nat Immunol (2006) 7(11):1209–16. doi: 10.1038/ni1392
123. Levy BD, Clish CB, Schmidt B, Gronert K, Serhan CN. Lipid mediator class switching during acute inflammation: signals in resolution. Nat Immunol (2001) 2(7):612–9. doi: 10.1038/89759
124. Dalli J, Norling LV, Renshaw D, Cooper D, Leung KY, Perretti M. Annexin 1 mediates the rapid anti-inflammatory effects of neutrophil-derived microparticles. Blood (2008) 112(6):2512–9. doi: 10.1182/blood-2008-02-140533
125. Bindea G, Mlecnik B, Tosolini M, Kirilovsky A, Waldner M, Obenauf AC, et al. Spatiotemporal dynamics of intratumoral immune cells reveal the immune landscape in human cancer. Immunity (2013) 39(4):782–95. doi: 10.1016/j.immuni.2013.10.003
126. Gentles AJ, Newman AM, Liu CL, Bratman SV, Feng W, Kim D, et al. The prognostic landscape of genes and infiltrating immune cells across human cancers. Nat Med (2015) 21(8):938–45. doi: 10.1038/nm.3909
127. Galdiero MR, Bianchi P, Grizzi F, Di Caro G, Basso G, Ponzetta A, et al. Occurrence and significance of tumor-associated neutrophils in patients with colorectal cancer. Int J Cancer. (2016) 139(2):446–56. doi: 10.1002/ijc.30076
128. Governa V, Trella E, Mele V, Tornillo L, Amicarella F, Cremonesi E, et al. The interplay between neutrophils and CD8(+) T cells improves survival in human colorectal cancer. Clin Cancer Res (2017) 23(14):3847–58. doi: 10.1158/1078-0432.CCR-16-2047
129. Ponzetta A, Carriero R, Carnevale S, Barbagallo M, Molgora M, Perucchini C, et al. Neutrophils driving unconventional T cells mediate resistance against murine sarcomas and selected human tumors. Cell (2019) 178(2):346–60.e24. doi: 10.1016/j.cell.2019.05.047
130. Hedrick CC, Malanchi I. Neutrophils in cancer: heterogeneous and multifaceted. Nat Rev Immunol (2022) 22(3):173–87. doi: 10.1038/s41577-021-00571-6
131. Knaapen AM, Seiler F, Schilderman PA, Nehls P, Bruch J, Schins RP, et al. Neutrophils cause oxidative DNA damage in alveolar epithelial cells. Free Radic Biol Med (1999) 27(1-2):234–40. doi: 10.1016/S0891-5849(98)00285-8
132. Canli O, Nicolas AM, Gupta J, Finkelmeier F, Goncharova O, Pesic M, et al. Myeloid cell-derived reactive oxygen species induce epithelial mutagenesis. Cancer Cell (2017) 32(6):869–83.e5. doi: 10.1016/j.ccell.2017.11.004
133. Wculek SK, Bridgeman VL, Peakman F, Malanchi I. Early neutrophil responses to chemical carcinogenesis shape long-term lung cancer susceptibility. iScience (2020) 23(7):101277. doi: 10.1016/j.isci.2020.101277
134. Butin-Israeli V, Bui TM, Wiesolek HL, Mascarenhas L, Lee JJ, Mehl LC, et al. Neutrophil-induced genomic instability impedes resolution of inflammation and wound healing. J Clin Invest (2019) 129(2):712–26. doi: 10.1172/JCI122085
135. Granot Z, Jablonska J. Distinct functions of neutrophil in cancer and its regulation. Mediators Inflamm (2015) 2015:701067. doi: 10.1155/2015/701067
136. Houghton AM, Rzymkiewicz DM, Ji H, Gregory AD, Egea EE, Metz HE, et al. Neutrophil elastase-mediated degradation of IRS-1 accelerates lung tumor growth. Nat Med (2010) 16(2):219–23. doi: 10.1038/nm.2084
137. Lerman I, Garcia-Hernandez ML, Rangel-Moreno J, Chiriboga L, Pan C, Nastiuk KL, et al. Infiltrating myeloid cells exert protumorigenic actions via neutrophil elastase. Mol Cancer Res (2017) 15(9):1138–52. doi: 10.1158/1541-7786.MCR-17-0003
138. Wada Y, Yoshida K, Tsutani Y, Shigematsu H, Oeda M, Sanada Y, et al. Neutrophil elastase induces cell proliferation and migration by the release of TGF-alpha, PDGF and VEGF in esophageal cell lines. Oncol Rep (2007) 17(1):161–7.
139. Li P, Lu M, Shi J, Gong Z, Hua L, Li Q, et al. Lung mesenchymal cells elicit lipid storage in neutrophils that fuel breast cancer lung metastasis. Nat Immunol (2020) 21(11):1444–55. doi: 10.1038/s41590-020-0783-5
140. El Rayes T, Catena R, Lee S, Stawowczyk M, Joshi N, Fischbach C, et al. Lung inflammation promotes metastasis through neutrophil protease-mediated degradation of tsp-1. Proc Natl Acad Sci U S A (2015) 112(52):16000–5. doi: 10.1073/pnas.1507294112
141. Kowanetz M, Wu X, Lee J, Tan M, Hagenbeek T, Qu X, et al. Granulocyte-colony stimulating factor promotes lung metastasis through mobilization of Ly6G+Ly6C+ granulocytes. Proc Natl Acad Sci U S A (2010) 107(50):21248–55. doi: 10.1073/pnas.1015855107
142. Wculek SK, Malanchi I. Neutrophils support lung colonization of metastasis-initiating breast cancer cells. Nature (2015) 528(7582):413–7. doi: 10.1038/nature16140
143. Szczerba BM, Castro-Giner F, Vetter M, Krol I, Gkountela S, Landin J, et al. Neutrophils escort circulating tumour cells to enable cell cycle progression. Nature (2019) 566(7745):553–7. doi: 10.1038/s41586-019-0915-y
144. Albrengues J, Shields MA, Ng D, Park CG, Ambrico A, Poindexter ME, et al. Neutrophil extracellular traps produced during inflammation awaken dormant cancer cells in mice. Science (2018) 361(6409):eaao4227. doi: 10.1126/science.aao4227
145. Cools-Lartigue J, Spicer J, McDonald B, Gowing S, Chow S, Giannias B, et al. Neutrophil extracellular traps sequester circulating tumor cells and promote metastasis. J Clin Invest (2013) 123(8):3446–58. doi: 10.1172/JCI67484
146. Guglietta S, Chiavelli A, Zagato E, Krieg C, Gandini S, Ravenda PS, et al. Coagulation induced by C3aR-dependent NETosis drives protumorigenic neutrophils during small intestinal tumorigenesis. Nat Commun (2016) 7:11037. doi: 10.1038/ncomms11037
147. Lee W, Ko SY, Mohamed MS, Kenny HA, Lengyel E, Naora H. Neutrophils facilitate ovarian cancer premetastatic niche formation in the omentum. J Exp Med (2019) 216(1):176–94. doi: 10.1084/jem.20181170
148. Park J, Wysocki RW, Amoozgar Z, Maiorino L, Fein MR, Jorns J, et al. Cancer cells induce metastasis-supporting neutrophil extracellular DNA traps. Sci Transl Med (2016) 8(361):361ra138. doi: 10.1126/scitranslmed.aag1711
149. Teijeira A, Garasa S, Gato M, Alfaro C, Migueliz I, Cirella A, et al. CXCR1 and CXCR2 chemokine receptor agonists produced by tumors induce neutrophil extracellular traps that interfere with immune cytotoxicity. Immunity (2020) 52(5):856–71.e8. doi: 10.1016/j.immuni.2020.03.001
150. van der Windt DJ, Sud V, Zhang H, Varley PR, Goswami J, Yazdani HO, et al. Neutrophil extracellular traps promote inflammation and development of hepatocellular carcinoma in nonalcoholic steatohepatitis. Hepatology (2018) 68(4):1347–60. doi: 10.1002/hep.29914
151. Yang L, Liu Q, Zhang X, Liu X, Zhou B, Chen J, et al. DNA Of neutrophil extracellular traps promotes cancer metastasis via CCDC25. Nature (2020) 583(7814):133–8. doi: 10.1038/s41586-020-2394-6
152. Coffelt SB, Wellenstein MD, de Visser KE. Neutrophils in cancer: neutral no more. Nat Rev Cancer. (2016) 16(7):431–46. doi: 10.1038/nrc.2016.52
153. Condamine T, Kumar V, Ramachandran IR, Youn JI, Celis E, Finnberg N, et al. ER stress regulates myeloid-derived suppressor cell fate through TRAIL-r-mediated apoptosis. J Clin Invest (2014) 124(6):2626–39. doi: 10.1172/JCI74056
154. Veglia F, Tyurin VA, Blasi M, De Leo A, Kossenkov AV, Donthireddy L, et al. Fatty acid transport protein 2 reprograms neutrophils in cancer. Nature (2019) 569(7754):73–8. doi: 10.1038/s41586-019-1118-2
155. Veglia F, Perego M, Gabrilovich D. Myeloid-derived suppressor cells coming of age. Nat Immunol (2018) 19(2):108–19. doi: 10.1038/s41590-017-0022-x
156. Zelenay S, van der Veen AG, Bottcher JP, Snelgrove KJ, Rogers N, Acton SE, et al. Cyclooxygenase-dependent tumor growth through evasion of immunity. Cell (2015) 162(6):1257–70. doi: 10.1016/j.cell.2015.08.015
157. Bonavita E, Bromley CP, Jonsson G, Pelly VS, Sahoo S, Walwyn-Brown K, et al. Antagonistic inflammatory phenotypes dictate tumor fate and response to immune checkpoint blockade. Immunity (2020) 53(6):1215–29.e8. doi: 10.1016/j.immuni.2020.10.020
158. Cheng Y, Li H, Deng Y, Tai Y, Zeng K, Zhang Y, et al. Cancer-associated fibroblasts induce PDL1+ neutrophils through the IL6-STAT3 pathway that foster immune suppression in hepatocellular carcinoma. Cell Death Dis (2018) 9(4):422. doi: 10.1038/s41419-018-0458-4
159. He G, Zhang H, Zhou J, Wang B, Chen Y, Kong Y, et al. Peritumoural neutrophils negatively regulate adaptive immunity via the PD-L1/PD-1 signalling pathway in hepatocellular carcinoma. J Exp Clin Cancer Res (2015) 34:141. doi: 10.1186/s13046-015-0256-0
160. Noman MZ, Desantis G, Janji B, Hasmim M, Karray S, Dessen P, et al. PD-L1 is a novel direct target of HIF-1alpha, and its blockade under hypoxia enhanced MDSC-mediated T cell activation. J Exp Med (2014) 211(5):781–90. doi: 10.1084/jem.20131916
161. Wang TT, Zhao YL, Peng LS, Chen N, Chen W, Lv YP, et al. Tumour-activated neutrophils in gastric cancer foster immune suppression and disease progression through GM-CSF-PD-L1 pathway. Gut (2017) 66(11):1900–11. doi: 10.1136/gutjnl-2016-313075
162. Xu W, Dong J, Zheng Y, Zhou J, Yuan Y, Ta HM, et al. Immune-checkpoint protein VISTA regulates antitumor immunity by controlling myeloid cell-mediated inflammation and immunosuppression. Cancer Immunol Res (2019) 7(9):1497–510. doi: 10.1158/2326-6066.CIR-18-0489
163. Blaisdell A, Crequer A, Columbus D, Daikoku T, Mittal K, Dey SK, et al. Neutrophils oppose uterine epithelial carcinogenesis via debridement of hypoxic tumor cells. Cancer Cell (2015) 28(6):785–99. doi: 10.1016/j.ccell.2015.11.005
164. Triner D, Devenport SN, Ramakrishnan SK, Ma X, Frieler RA, Greenson JK, et al. Neutrophils restrict tumor-associated microbiota to reduce growth and invasion of colon tumors in mice. Gastroenterology (2019) 156(5):1467–82. doi: 10.1053/j.gastro.2018.12.003
165. Dmitrieva-Posocco O, Dzutsev A, Posocco DF, Hou V, Yuan W, Thovarai V, et al. Cell-Type-Specific responses to interleukin-1 control microbial invasion and tumor-elicited inflammation in colorectal cancer. Immunity (2019) 50(1):166–80.e7. doi: 10.1016/j.immuni.2018.11.015
166. Gershkovitz M, Caspi Y, Fainsod-Levi T, Katz B, Michaeli J, Khawaled S, et al. TRPM2 mediates neutrophil killing of disseminated tumor cells. Cancer Res (2018) 78(10):2680–90. doi: 10.1158/0008-5472.CAN-17-3614
167. Gershkovitz M, Fainsod-Levi T, Zelter T, Sionov RV, Granot Z. TRPM2 modulates neutrophil attraction to murine tumor cells by regulating CXCL2 expression. Cancer Immunol Immunother (2019) 68(1):33–43. doi: 10.1007/s00262-018-2249-2
168. Granot Z, Henke E, Comen EA, King TA, Norton L, Benezra R. Tumor entrained neutrophils inhibit seeding in the premetastatic lung. Cancer Cell (2011) 20(3):300–14. doi: 10.1016/j.ccr.2011.08.012
169. Koga Y, Matsuzaki A, Suminoe A, Hattori H, Hara T. Neutrophil-derived TNF-related apoptosis-inducing ligand (TRAIL): a novel mechanism of antitumor effect by neutrophils. Cancer Res (2004) 64(3):1037–43. doi: 10.1158/0008-5472.CAN-03-1808
170. Finisguerra V, Di Conza G, Di Matteo M, Serneels J, Costa S, Thompson AA, et al. MET is required for the recruitment of anti-tumoural neutrophils. Nature (2015) 522(7556):349–53. doi: 10.1038/nature14407
171. Glodde N, Bald T, van den Boorn-Konijnenberg D, Nakamura K, O'Donnell JS, Szczepanski S, et al. Reactive neutrophil responses dependent on the receptor tyrosine kinase c-MET limit cancer immunotherapy. Immunity (2017) 47(4):789–802.e9. doi: 10.1016/j.immuni.2017.09.012
172. Matlung HL, Babes L, Zhao XW, van Houdt M, Treffers LW, van Rees DJ, et al. Neutrophils kill antibody-opsonized cancer cells by trogoptosis. Cell Rep (2018) 23(13):3946–59.e6. doi: 10.1016/j.celrep.2018.05.082
173. Linde IL, Prestwood TR, Qiu J, Pilarowski G, Linde MH, Zhang X, et al. Neutrophil-activating therapy for the treatment of cancer. Cancer Cell (2023) 41(2):356–72.e10. doi: 10.1016/j.ccell.2023.01.002
174. Massara M, Bonavita O, Savino B, Caronni N, Mollica Poeta V, Sironi M, et al. ACKR2 in hematopoietic precursors as a checkpoint of neutrophil release and anti-metastatic activity. Nat Commun (2018) 9(1):676. doi: 10.1038/s41467-018-03080-8
175. Singhal S, Bhojnagarwala PS, O'Brien S, Moon EK, Garfall AL, Rao AS, et al. Origin and role of a subset of tumor-associated neutrophils with antigen-presenting cell features in early-stage human lung cancer. Cancer Cell (2016) 30(1):120–35. doi: 10.1016/j.ccell.2016.06.001
176. Mysore V, Cullere X, Mears J, Rosetti F, Okubo K, Liew PX, et al. FcgammaR engagement reprograms neutrophils into antigen cross-presenting cells that elicit acquired anti-tumor immunity. Nat Commun (2021) 12(1):4791. doi: 10.1038/s41467-021-24591-x
177. Pylaeva E, Korschunow G, Spyra I, Bordbari S, Siakaeva E, Ozel I, et al. During early stages of cancer, neutrophils initiate anti-tumor immune responses in tumor-draining lymph nodes. Cell Rep (2022) 40(7):111171. doi: 10.1016/j.celrep.2022.111171
178. Gungabeesoon J, Gort-Freitas NA, Kiss M, Bolli E, Messemaker M, Siwicki M, et al. A neutrophil response linked to tumor control in immunotherapy. Cell (2023) 186(7):1448–64.e20. doi: 10.1016/j.cell.2023.02.032
179. Hirschhorn D, Budhu S, Kraehenbuehl L, Gigoux M, Schroder D, Chow A, et al. T Cell immunotherapies engage neutrophils to eliminate tumor antigen escape variants. Cell (2023) 186(7):1432–47.e17. doi: 10.1016/j.cell.2023.03.007
180. Belkaid Y, Hand TW. Role of the microbiota in immunity and inflammation. Cell (2014) 157(1):121–41. doi: 10.1016/j.cell.2014.03.011
181. Bertocchi A, Carloni S, Ravenda PS, Bertalot G, Spadoni I, Lo Cascio A, et al. Gut vascular barrier impairment leads to intestinal bacteria dissemination and colorectal cancer metastasis to liver. Cancer Cell (2021) 39(5):708–24.e11. doi: 10.1016/j.ccell.2021.03.004
182. Brennan CA, Garrett WS. Gut microbiota, inflammation, and colorectal cancer. Annu Rev Microbiol (2016) 70:395–411. doi: 10.1146/annurev-micro-102215-095513
183. McQuade JL, Daniel CR, Helmink BA, Wargo JA. Modulating the microbiome to improve therapeutic response in cancer. Lancet Oncol (2019) 20(2):e77–91. doi: 10.1016/S1470-2045(18)30952-5
184. Zagato E, Pozzi C, Bertocchi A, Schioppa T, Saccheri F, Guglietta S, et al. Endogenous murine microbiota member faecalibaculum rodentium and its human homologue protect from intestinal tumour growth. Nat Microbiol (2020) 5(3):511–24. doi: 10.1038/s41564-019-0649-5
185. Jin C, Lagoudas GK, Zhao C, Bullman S, Bhutkar A, Hu B, et al. Commensal microbiota promote lung cancer development via gammadelta T cells. Cell (2019) 176(5):998–1013.e16. doi: 10.1016/j.cell.2018.12.040
186. Coffelt SB, Kersten K, Doornebal CW, Weiden J, Vrijland K, Hau CS, et al. IL-17-producing gammadelta T cells and neutrophils conspire to promote breast cancer metastasis. Nature (2015) 522(7556):345–8. doi: 10.1038/nature14282
187. Rice CM, Davies LC, Subleski JJ, Maio N, Gonzalez-Cotto M, Andrews C, et al. Tumour-elicited neutrophils engage mitochondrial metabolism to circumvent nutrient limitations and maintain immune suppression. Nat Commun (2018) 9(1):5099. doi: 10.1038/s41467-018-07505-2
188. Wellenstein MD, Coffelt SB, Duits DEM, van Miltenburg MH, Slagter M, de Rink I, et al. Loss of p53 triggers WNT-dependent systemic inflammation to drive breast cancer metastasis. Nature (2019) 572(7770):538–42. doi: 10.1038/s41586-019-1450-6
189. Dinh HQ, Eggert T, Meyer MA, Zhu YP, Olingy CE, Llewellyn R, et al. Coexpression of CD71 and CD117 identifies an early unipotent neutrophil progenitor population in human bone marrow. Immunity (2020) 53(2):319–34.e6. doi: 10.1016/j.immuni.2020.07.017
190. Kumar S, Dikshit M. Metabolic insight of neutrophils in health and disease. Front Immunol (2019) 10:2099. doi: 10.3389/fimmu.2019.02099
191. Hsu BE, Tabaries S, Johnson RM, Andrzejewski S, Senecal J, Lehuede C, et al. Immature low-density neutrophils exhibit metabolic flexibility that facilitates breast cancer liver metastasis. Cell Rep (2019) 27(13):3902–15.e6. doi: 10.1016/j.celrep.2019.05.091
192. Fridlender ZG, Sun J, Kim S, Kapoor V, Cheng G, Ling L, et al. Polarization of tumor-associated neutrophil phenotype by TGF-beta: "N1" versus "N2" TAN. Cancer Cell (2009) 16(3):183–94. doi: 10.1016/j.ccr.2009.06.017
193. Veglia F, Sanseviero E, Gabrilovich DI. Myeloid-derived suppressor cells in the era of increasing myeloid cell diversity. Nat Rev Immunol (2021) 21(8):485–98. doi: 10.1038/s41577-020-00490-y
194. Cassetta L, Baekkevold ES, Brandau S, Bujko A, Cassatella MA, Dorhoi A, et al. Deciphering myeloid-derived suppressor cells: isolation and markers in humans, mice and non-human primates. Cancer Immunol Immunother (2019) 68(4):687–97. doi: 10.1007/s00262-019-02302-2
195. Veglia F, Hashimoto A, Dweep H, Sanseviero E, De Leo A, Tcyganov E, et al. Analysis of classical neutrophils and polymorphonuclear myeloid-derived suppressor cells in cancer patients and tumor-bearing mice. J Exp Med (2021) 218(4):e20201803. doi: 10.1084/jem.20201803
196. Gabrilovich DI, Nagaraj S. Myeloid-derived suppressor cells as regulators of the immune system. Nat Rev Immunol (2009) 9(3):162–74. doi: 10.1038/nri2506
197. Zhou SL, Zhou ZJ, Hu ZQ, Huang XW, Wang Z, Chen EB, et al. Tumor-associated neutrophils recruit macrophages and T-regulatory cells to promote progression of hepatocellular carcinoma and resistance to sorafenib. Gastroenterology (2016) 150(7):1646–58.e17. doi: 10.1053/j.gastro.2016.02.040
198. Hoechst B, Ormandy LA, Ballmaier M, Lehner F, Kruger C, Manns MP, et al. A new population of myeloid-derived suppressor cells in hepatocellular carcinoma patients induces CD4(+)CD25(+)Foxp3(+) T cells. Gastroenterology (2008) 135(1):234–43. doi: 10.1053/j.gastro.2008.03.020
Keywords: neutrophil, inflammation, cancer, innate immunity, tumor immunology
Citation: Carnevale S, Di Ceglie I, Grieco G, Rigatelli A, Bonavita E and Jaillon S (2023) Neutrophil diversity in inflammation and cancer. Front. Immunol. 14:1180810. doi: 10.3389/fimmu.2023.1180810
Received: 06 March 2023; Accepted: 11 April 2023;
Published: 26 April 2023.
Edited by:
Denisa Baci, University of Insubria, ItalyReviewed by:
Daniel Hargbøl Madsen, Herlev Hospital, DenmarkChristian Con Yost, The University of Utah, United States
Copyright © 2023 Carnevale, Di Ceglie, Grieco, Rigatelli, Bonavita and Jaillon. This is an open-access article distributed under the terms of the Creative Commons Attribution License (CC BY). The use, distribution or reproduction in other forums is permitted, provided the original author(s) and the copyright owner(s) are credited and that the original publication in this journal is cited, in accordance with accepted academic practice. No use, distribution or reproduction is permitted which does not comply with these terms.
*Correspondence: Sebastien Jaillon, c2ViYXN0aWVuLmphaWxsb25AaHVtYW5pdGFzcmVzZWFyY2guaXQ=; Eduardo Bonavita, ZWR1YXJkby5ib25hdml0YUBodW1hbml0YXNyZXNlYXJjaC5pdA==
†These authors have contributed equally to this work